- 1Institute of Microbiology, Technische Universität Braunschweig, Braunschweig, Germany
- 2Department Structure and Function of Proteins, Helmholtz Centre for Infection Research, Braunschweig, Germany
- 3Institute of Biochemistry, Biotechnology and Bioinformatic, Technische Universität Braunschweig, Braunschweig, Germany
- 4Braunschweig Centre of Integrated Systems Biology, Technische Universität Braunschweig, Braunschweig, Germany
Acinetobacter baumannii is an opportunistic human pathogen which can use host-derived L-carnitine as sole carbon and energy source. Recently, an L-carnitine transporter (Aci1347) and a specific monooxygense (CntA/CntB) for the intracellular cleavage of L-carnitine have been characterized. Subsequent conversion of the resulting malic semialdehyde into the central metabolite L-malate was hypothesized. Alternatively, L-carnitine degradation via D-malate with subsequent oxidation into pyruvate was proposed. Here we describe the in vitro and in vivo reconstitution of the entire pathway, starting from the as yet uncharacterized gene products of the carnitine degradation gene operon. Using recombinantly purified enzymes, enantiomer-specific formation of D-malate by the NAD(P)+-dependent malic semialdehyde dehydrogenase (MSA-DH) is demonstrated. The solved X-ray crystal structure of tetrameric MSA-DH reveals the key catalytic residues Cys290 and Glu256, accessible through opposing substrate and cofactor funnels. Specific substrate binding is enabled by Arg166, Arg284 and Ser447 while dual cofactor specificity for NAD+ and NADP+ is mediated by Asn184. The subsequent conversion of the unusual D-malate reaction product by an uncharacterized NAD+-dependent malate dehydrogenase (MDH) is shown. Tetrameric MDH is a β-decarboxylating dehydrogenase that synthesizes pyruvate. MDH experiments with alternative substrates showed a high degree of substrate specificity. Finally, the entire A. baumannni pathway was heterologously reconstituted, allowing E. coli to grow on L-carnitine as a carbon and energy source. Overall, the metabolic conversion of L-carnitine via malic semialdehyde and D-malate into pyruvate, CO2 and trimethylamine was demonstrated. Trimethylamine is also an important gut microbiota-dependent metabolite that is associated with an increased risk of cardiovascular disease. The pathway reconstitution experiments allowed us to assess the TMA forming capacity of gut microbes which is related to human cardiovascular health.
1 Introduction
Acinetobacter baumannii is an emerging pathogen causing opportunistic infections in healthcare facilities worldwide (Villegas and Hartstein, 2003). The Gram-negative organism is known for the development of high antibiotic resistance and the ability to survive and persist under a wide range of environmental conditions (Dijkshoorn et al., 2007; Ayoub Moubareck and Hammoudi Halat, 2020). A. baumannii is a nosocomial pathogen that thrives due to its remarkable ability to withstand desiccation and to persist in the human host (Jawad et al., 1998; Parra-Millan et al., 2018; Zeidler and Muller, 2019). The versatile metabolism of A. baumannii facilitates for the utilization of multiple host-derived carbon and nitrogen sources promoting survival in host niches such as the urinary tract, bloodstream or wounds (Dijkshoorn et al., 2007; Soares et al., 2009). Therefore, understanding the employed metabolic pathways is crucial for developing effective strategies to combat infections. One such pathway that might play a vital role in the metabolism of A. baumannii is responsible for the degradation for L-carnitine (Zhu et al., 2014; Breisch et al., 2022). This quaternary amine compound is highly available in host tissues (e.g., blood ~50 μM, liver or heart ~1.5 or 5 μmol/g tissue) (Hanai et al., 2020). In the human body, L-carnitine mainly functions as a shuttle for the translocation of long-chain fatty acids into mitochondria for subsequent β-oxidation (Longo et al., 2016). Growth experiments revealed that L-carnitine serves as a sole carbon and energy source of A. baumannii (Zhu et al., 2014; Breisch et al., 2022) and a gene cluster for the metabolism of L-carnitine was bioinformatically predicted (Figure 1). Infection studies clearly demonstrated that the corresponding genes are important for the virulence of the opportunistic pathogen (Breisch et al., 2022). The cluster comprises the carR gene which encodes for a LysR-type transcriptional activator (lysR). L-carnitine dependent binding of the purified CarR protein to the intergenic region between carR and the consecutive carnitine catabolic operon was demonstrated (Breisch et al., 2022). Among the components of this operon, the biological function of the encoded transporter Aci01347 was elucidated on the basis of substrate uptake experiments. The transporter belongs to the betaine/choline/carnitine (BCCT) family and mediates the energy-dependent import of L-carnitine but also of choline into the A. baumannii cell (Breisch et al., 2019). Furthermore, genes cntA and cntB of the operon encode for a two-component carnitine monooxygenase which facilitates the initial C-N bond cleavage of L-carnitine which results in the formation of trimethylamine (TMA) and malic semialdehyde (MSA) (Zhu et al., 2014). Our laboratory contributed to the molecular understanding of the catalytic mechanism of the enzyme which comprises the Rieske-type oxygenase CntA and the corresponding reductase CntB (Massmig et al., 2020; Piskol et al., 2022). On the basis of theoretical considerations, it was hypothesized that the operon encodes for a malic semialdehyde dehydrogenase (MSA-DH) which enables for the subsequent conversion of MSA into L-malate which might be channelled into the tricarboxylic acid cycle (Zhu et al., 2014). Alternative L-carnitine degradation via MSA and D-malate with subsequent oxidation into pyruvate by virtue of a D-malate dehydrogenase (MDH) was postulated. Deletion of the mdh gene abolished A. baumannii growth on L-carnitine which demonstrates the involvement of the postulated MDH in the degradation pathway. Growth of the mdh mutant on L-malate was still possible due to the presence of an L-malate dehydrogenase as part of the TCA cycle. Further analysis of the proposed D-malate pathway intermediate was hampered by the possible involvement of a cellular malate racemase and the unavailability of a msadh mutant strain. Growth experiments on D-malate for the mdh mutant revealed reduced growth (instead of abolished growth) which might be attributed to a theoretical malate racemase activity of A. baumannii (Breisch et al., 2022). To date, biochemical evidence for the proposed conversion of L-carnitine via MSA and D-malate into pyruvate and CO2 is still pending.
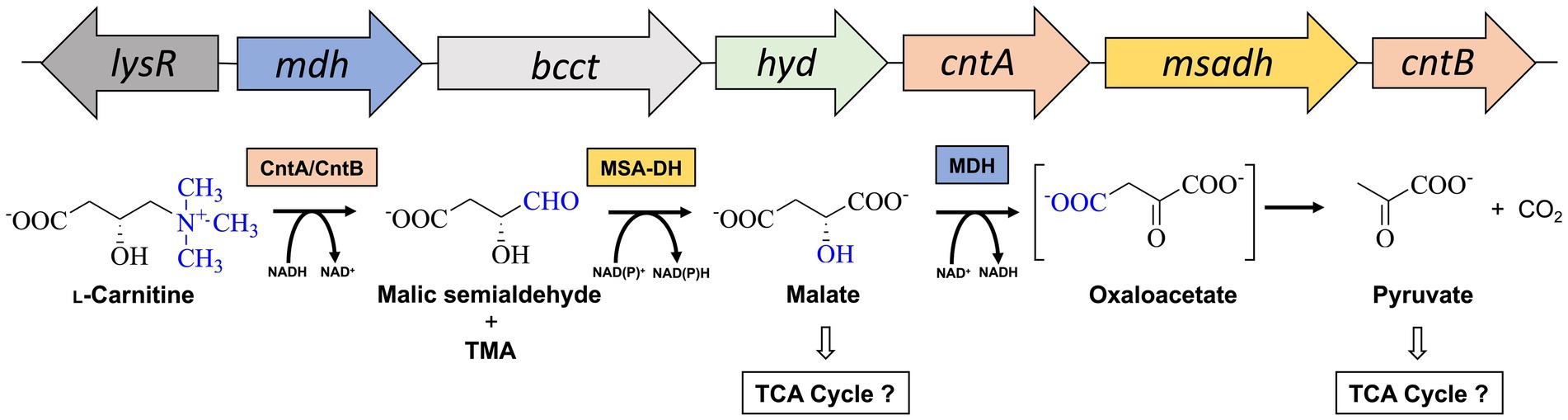
Figure 1. Metabolism of L-carnitine in A. baumannii. Structure of the L-carnitine degradation gene operon (top) and proposed enzymatic reaction sequence(s) (bottom). The cleavage of the C-N bond of L-carnitine is facilitated by carnitine monooxygenase (CntA/CntB), resulting in the formation of trimethylamine (TMA) and malic semialdehyde (Zhu et al., 2014). The latter is converted to malate by malic semialdehyde dehydrogenase (MSA-DH). Pyruvate is formed by the reaction of a potential malate dehydrogenase (MDH). Channeling of reaction products into the central metabolism is indicated. Genes lysR (also termed carR) and bcct encode for a LysR-type transcriptional activator and a transporter of the betaine/choline/carnitine family.
In the present study, we make use of recombinantly purified carnitine monooxygenase, MSA-DH and MDH for the in vitro reconstitution of the overall carnitine degradation pathway. Enzyme activities in the presence of variant substrates allow for the elucidation of the (stereo)specificity of the individual reactions. X-ray protein crystallography and/or biochemical experiments are employed for the molecular understanding of the respective enzymes. Finally, the overall pathway is reconstituted under in vitro and in vivo conditions.
2 Materials and methods
2.1 Plasmid construction
Constructs pGEX-6P-1-cntA and pACYCDuet-1-cntB (for the individual production of CntA and CntB) and also plasmid pGEX-6P-1-cntAB (for the co-production of CntA and CntB) were described recently (Massmig et al., 2020). Codon-optimized genes for A. baumannii ATCC 19606 MSA-DH and MDH were synthesized by GeneArt (Thermo Fisher). Both Genes were cloned via NcoI and NotI restriction sites into plasmid pETM20 and/or pETM11 (Dummler et al., 2005) yielding constructs pETM20-msadh or pETM11-msadh and pETM11-mdh.
For pathway reconstitution experiments, the codon-optimized BCCT gene (Aci1347) was cloned into plasmid pGEX-6P-1-cntAB using the In-Fusion cloning system (Takara) yielding construct pGEX-6P-1-cntAB-bcct. Plasmid pETM11-cntAB-bcct was obtained by subcloning. cntAB-bcct. The gene for MSA-DH was cloned into MCS1 of pACYCDuet-1 via NotI and NcoI restriction sites and the gene for MDH was cloned into MCS2 via NdeI and XhoI restriction sites yielding pACYCDuet-1-msadh-mdh.
2.2 Production and purification of carnitine monooxygenase
The individual subunits CntA and CntB of carnitine monooxygenase were produced and purified as described elsewhere (Massmig et al., 2020).
2.3 Overproduction and purification of MSA-DH and MDH
MSA-DH fused to an N-terminal His-thioredoxin tag or MDH fused to an N-terminal His-tag was overproduced in E. coli Tuner (DE3) cells. An overnight culture was used to inoculate 4 × 500 mL of LB medium containing 100 μg ml−1 ampicillin (pETM20-msadh) or 50 μg ml−1 kanamycin (pETM11-msadh pETM11-mdh), respectively. Cells were cultivated at 37°C and 200 rpm in baffled flasks until an optical density at 578 nm of 0.5 was reached. Subsequently, the production of target proteins was initiated by addition of 50 μM isopropyl-β-d-thiogalactopyranoside (IPTG). After 16 h of cultivation at 17°C and 180 rpm, the cells were harvested by centrifugation at 4,000 × g for 20 min at 4°C. Cells of 2 L culture were resuspended in buffer 1 (100 mM HEPES-NaOH pH 7.5, 10 mM MgCl2,150 mM NaCl) or in buffer 1 containing 50 mM NaCl, supplemented with 2.5 units/mL Turbo Nuclease (Jena Bioscience) and disrupted by a single passage through a french press at 14,500 psi. Lysates were clarified by centrifugation at 27,500 × g for 65 min at 4°C and subsequent 0.45 μm filtration. Soluble extracts were applied to 2 mL Ni2+-loaded Ni-NTA Agarose (MACHEREY-NAGEL). Columns were washed with 8 mL buffer 1, 8 mL buffer 1 containing 50 mM imidazole and 8 mL buffer 1 containing 100 mM imidazole. Proteins MSA-DH or MDH were eluted with 3 × 2 mL buffer 1 containing 150 mM imidazole. Target protein fractions were identified by SDS-PAGE.
2.4 Dialysis of MDH
The apo form of MDH was obtained by EDTA treatment. Purified MDH (~1 mL) was dialyzed for 4 h in 1 L buffer 2 (20 mM MOPS-KOH pH 7.5, 150 mM NaCl) containing 10 mM EDTA followed by three times dialysis against 1 L of buffer 2.
2.5 Determination of protein concentration
The concentration of purified proteins was determined using Bradford reagent (Sigma-Aldrich) according to the manufacturer’s instructions with bovine serum albumin as a standard.
2.6 Carnitine monooxygenase activity assays
The standard L-carnitine depletion assay was performed as detailed under (Piskol et al., 2022) in the presence 2 mM L-carnitine and 2 mM NADH. An alternative carnitine monooxygenase assay was developed which monitors the time-dependent formation of the reaction product MSA. The assay is based on the determination of aldehydes using 3-methyl-2-benzothiazolinone hydrochloride (MBTH) (Cummins and Hauser, 1964; Zurek and Karst, 1997). A typical 400-μl standard aldehyde formation assay was performed in 20 mM HEPES-NaOH pH 7.5, 10 mM MgCl2, 150 mM NaCl containing 5 μM purified CntA, 15 μM purified CntB, and 2 mM L-carnitine at 27°C. Reactions were initiated by the addition of 2 mM NADH. At defined time points (0, 30, 60, 90 and 120 s), 40-μl samples were heat inactivated by the addition of 360 μL pre-heated water (95°C). After 5 min at 95°C followed by centrifugation for 10 min at 12,000 × g, 20 μL of the resulting supernatant was diluted with 80 μL deionized water for subsequent aldehyde determination. A 0.1% (w/v) MBTH solution and an Fe solution consisting of 1% (w/v) iron(III) perchlorate hydrate and 1.6% (w/v) sulfamic acid were each prepared in deionized water. Aldehyde containing samples of 100 μL were mixed with 100 μL MBTH solution on a microplate and incubated at RT for 25 min. Subsequently, 40 μL Fe solution were added and incubated for 30 min before the absorption at 630 nm was determined. Samples with a defined concentration of formaldehyde (2.5 to 50 μM) were processed accordingly. All kinetic experiments were performed in triplicate.
2.7 Enzymatic synthesis of MSA
MSA was generated by quantitative turnover of 20 mM L-carnitine in the presence of 20 mM NADH in a 1 mL carnitine monooxygenase reaction as mainly detailed under (Massmig et al., 2020). Resulting aldehyde concentrations were determined as described above. Stoichiometric turnover of the NADH cofactor was verified spectroscopically.
2.8 Spectroscopic MSA-DH or MDH activity assays
The activity of MSA-DH or MDH was measured in a continuous spectroscopic assay by determining the initial rate of NADH formation at 340 nm, at a temperature of 27°C using an extinction coefficient of ϵ340 = 6.2 mM−1 cm−1 (Hossain et al., 1984). Standard assays containing 0.2 μM MSA-DH or MDH in 300 μL buffer 1 containing 20 mM NAD(P)+ were performed in the presence of 0.5–12 or 20 mM of the respective substrate MSA or D-malate. Experiments (in triplicate) were completed by control reactions in the absence of substrate. Specific activities were determined in the linear range of the assay. Kinetic parameters were calculated according to the Michaelis–Menten equation.
2.9 MSA-DH depletion assay
The activity of MSA-DH was also followed by monitoring MSA turnover at 27°C. A typical assay in a volume of 500 μL buffer 1 contained 400 μM MSA, 0.2 μM MSA-DH and 2 mM NAD+. At defined time points (0, 30, 60, and 90 s), 40 μL samples were heat inactivated and the aldehyde concentration was determined as detailed above. Experiments in the absence of MSA-DH were processed accordingly. The slope of this control reaction was subtracted to calculate the specific MSA depletion activity in the linear range of the assay. All experiments were performed in triplicate.
2.10 Identification of reaction products by HPLC
Samples from MSA-DH assays, from MDH assays or from pathway reconstitution experiments were separated on a Repromer H column (300 × 4,6 mm, 9 μm, TECHLAB GmbH) using a HPLC-system equipped with a degasser (DG-1580-54, Jasco), a gradient unit (LG-1580-04, Jasco), a pump (PU-1580, Jasco), a sampler (AS-1555, Jasco), a column oven (CO-1560, Jasco) and a multi wavelength detector (MD-1515, Jasco). Isocratic separation (~ 50 μL) was performed using 3 mM sulfuric acid as liquid phase at a flow rate of 0.5 mL min−1 at room temperature, measuring absorbance at 210 nm. Samples were treated by heat precipitation (99°C, 10 min) followed by centrifugation (12,000 g, 10 min). Authentic malate or pyruvate samples (Sigma-Aldrich) at a concentration of 10 mM were analyzed accordingly. A 50 μL sample from a MSA-DH assay was subjected to preparative separation with subsequent fractionation.
2.11 HPLC separation of stereoisomers
The separation of D- and L-malate was performed on a Chirex 3126 (D)-penicillamine column (150 × 4.6 mm, Phenomenex Inc.) using the above chromatography system as detailed mainly under application note TN-1005. Isocratic enantiomer separation was at a flow rate of 1 mL min−1 at 27°C using a mobile phase consisting of 85% (v/v) 100 mM ammonium acetate, 1 mM Cu(II)-acetate (pH 4.5, with acetic acid) and 15% (v/v) 2-propanol. The HPLC purified malate fraction from the MSA-DH assay was diluted 1:1 in the mobile phase and subjected to enantiomer separation (25 μL). Authentic L-malate and D-malate samples (5 μL, 10 mM, Sigma Aldrich) were analyzed as a reference.
2.12 UV–vis spectroscopy
Purified protein fractions at a concentration of 20–30 μM were subjected to UV–vis spectroscopy using a V-650 UV–Vis spectrophotometer (Jasco).
2.13 Analytical size exclusion chromatography
The native molecular mass of MSA-DH and MDH was determined by analytical size exclusion chromatography. A Superdex 200 increase 5/150 GL column (GE Healthcare) was calibrated with protein standards (molecular weight marker kit MWGF200 and thyroglobulin and apoferritin from Sigma) in the presence of 20 mM HEPES-NaOH pH 7.5, 150 mM NaCl at a flow rate of 0.45 mL min−1. Samples of MSA-DH and MDH at a concentration of 3 mg mL−1 (50 μL) were injected. Elution was monitored at 280 nm.
2.14 Mass photometry
The mass photometry experiments were performed on a Refeyn TwoMP mass photometer (Refeyn Ltd). Movies of 60 s (regular FOV) were recorded for 25 nM MDH or 25 nM MSA-DH, respectively in PBS with filtered PBS as a reference using AquireMP (Refeyn Ltd). Evaluation was performed in DiscoverMP (Refeyn Ltd) and molecular masses were determined by matching histogram contrast against a molecular weight standard (Invitrogen/Thermo NativeMark™, LC0725).
2.15 Protein crystallography
Crystallization trials were set up at room temperature with a Crystal Gryphon crystallization robot (Art Robbins Instruments) in Intelli 96–3 plates (Art Robbins Instruments) with 200 nL protein solution at a concentration of 40 mg/mL supplemented with 7 mM D-malate and 200 nL reservoir solution. Well-diffracting crystals were obtained after a few days in condition G8 of the Pi-PEG screen (Jena Bioscience), containing 34.3% (w/v) PEG 550 monomethyl ether and 2.9% (w/v) PEG 300. Crystals were after harvesting flash cooled in liquid N2.
Data collection was performed at beamline P11 at the Petra III storage ring (Deutsches Elektronen-Synchrotron, Hamburg, Germany) (Burkhardt et al., 2016). Datasets were recorded at a temperature of 100 K. Data processing was carried out using the AutoPROC (Vonrhein et al., 2011) toolbox (Global Phasing) executing XDS (Kabsch, 2010), Pointless (Evans, 2006), and Aimless (Evans and Murshudov, 2013).
The structure of MSA-DH was determined by molecular replacement using a model generated by Alpha-Fold2 (Jumper et al., 2021) as a search-model for Phaser (McCoy et al., 2007) from the Phenix suite (Adams et al., 2010). The structural models were built using Coot (Emsley et al., 2010) and crystallographic refinement was performed with Phenix.refine (Afonine et al., 2012) including the addition of hydrogens in riding positions and TLS-refinement. 5% of random reflections were flagged for the calculation of Rfree. The model of MSA-DH was at 2.6 Å resolution refined to R/Rfree of 18/21% in space group P212121. The structural model contains 12 polypeptide chains in the asymmetric unit, corresponding to three tetramers of MSA-DH. Data collection and refinement statistics are summarized in Supplementary Table S1.
NADP+ as a ligand was extracted from E. coli succinic semialdehyde dehydrogenase [PDB: 3JZ4, (Langendorf et al., 2010)] after structural superimposition with MSA-DH. MSA as a ligand was generated by addition of a hydroxy group to succinic semialdehyde (SSA) using Maestro (Schrödinger Suite 2023–4, Schrödinger, LLC), subsequent to the extraction of SSA from human SSA-DH [PDB: 2W8Q, (Kim et al., 2009)] superimposed with MSA-DH. The crystal structure including both added ligands was then energy minimized using Maestro. Figures of crystal structures were prepared using the PyMOL Molecular Graphics System version 2.4.0 (Schrödinger, LLC). Cavities were calculated using CavitOmiX (v. 1.0, 2022, Innophore GmbH). The corresponding hydrophobicity module of the program VASCo (Steinkellner et al., 2009) was used to analyze the hydrophobicity of the cavities. The cavities were calculated using a modified LIGSITE algorithm (Hendlich et al., 1997).
2.16 Pathway reconstitution in E. coli
E. coli BL21 carrying IPTG inducible plasmids pETM11-cntAB-bcct and pACYCDuet-1-msadh-mdh was used for the heterologous in vivo reconstitution of the overall carnitine degradation pathway of A. baumannii. Cells from a 50 mL overnight culture in LB medium were harvested by centrifugation (4,000 g, 10 min) and resuspended in 20 mL MOPS minimal medium (Neidhardt et al., 1974). The cell suspension was used to inoculate 50 mL of 20 mM L-carnitine supplemented MOPS minimal medium (OD578 ~ 0.2) containing 50 μg mL−1 ampicillin (pGEX-6P-1-cntAB-bcct), 17 μg mL−1 chloramphenicol (pACYCDuet-1-msadh-mdh) and 500 μM IPTG. E. coli strains BL21(DE3), B and K12 served as a control. Growth experiments were reproduced with three independent cultures.
3 Results
3.1 Recombinant production and purification of carnitine monooxygenase and MSA-DH
The catalytic component CntA and the related reductase CntB of carnitine monooxygenase from A. baumannii were individually produced and purified as N-terminal GST-tagged and His-tagged fusion proteins. Proteolytic on-column cleavage of the CntA fusion was performed with PreScission protease (SDS-PAGE Figure 2A, lanes 1–3, left and right) (Massmig et al., 2020).
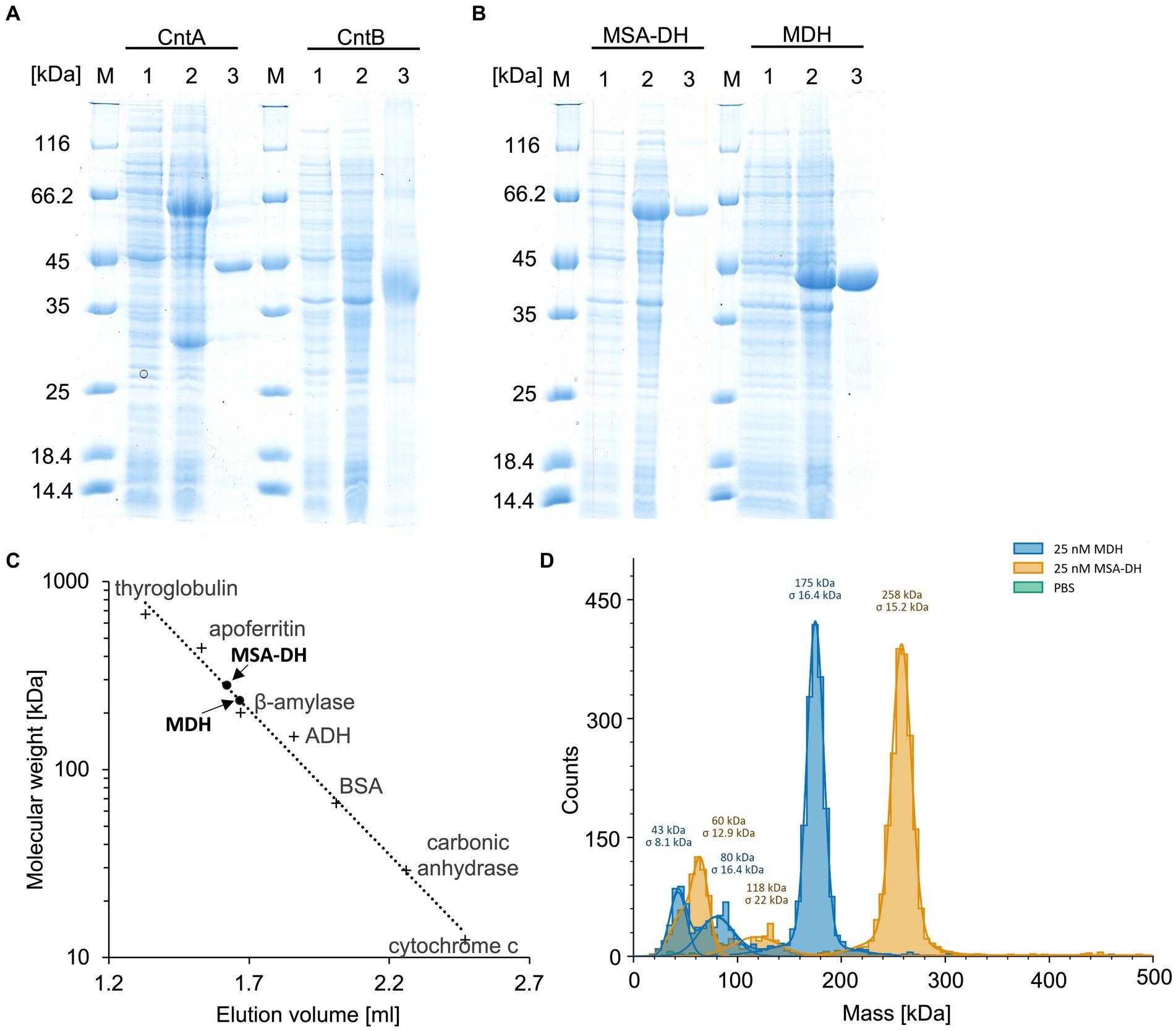
Figure 2. Purification and characterization of the enzymes of the L-carnitine degrading pathway. (A,B) SDS-PAGE analysis of the affinity purification of carnitine monooxygenase subunits CntA and CntB, MSA-DH and MDH. Lanes 1 and 2, E. coli cells before and after IPTG induction; lanes 3, elution fractions after cell disruption, ultracentrifugation and affinity chromatography; lanes M, molecular mass marker, relative molecular masses are indicated. (C) Native molecular mass determination of MSA-DH and MDH by analytical gel filtration. Purified proteins were analyzed on a Superdex 200 increase 5/150 GL column at a flow rate of 0.45 mL min−1 monitoring the absorption at 280 nm. The column was calibrated with thyroglobulin (Mr = 660,000), apoferritin (Mr = 440,000), ß-amylase (Mr = 200,000), alcohol dehydrogenase (ADH, Mr. = 150,000), albumin (Mr = 66,000), carbonic anhydrase (Mr = 29,000) and cytochrome c (Mr = 12,400). (D) Molecular mass histograms of MSA-DH and MDH obtained from mass photometry measurements using a Refeyn TwoMP instrument. The average molecular mass is given.
Overproduction of MSA-DH in E. coli as an N-terminal His-thioredoxin fusion yielded an additional protein band with a relative molecular mass of ∼64,000 Da (calculated molecular mass, 66,592 Da). The recombinant A. baumannii protein was affinity purified using Ni2+-loaded chelating Sepharose (SDS-PAGE Figure 2B, lanes 1–3 left). Approximately 30 mg purified MSA-DH was obtained per liter cell culture.
3.2 New carnitine monooxygenase assay
Published carnitine monooxygenase assays rely on the enzymatic quantification of the L-carnitine substrate (Massmig et al., 2020; Piskol et al., 2022) or on the spectroscopic quantification of the co-substrate NADH (Quareshy et al., 2020). These depletion assays either require a coupled detection reaction or, on the other hand, may be affected by a nonproductive NADH consumption (Piskol et al., 2022). Alternatively, sophisticated GC–MS-based approaches were used for the quantification of TMA (Kalnins et al., 2018).
Here, a simple and efficient assay was established which is based on the quantification of the second reaction product of carnitine monooxygenase (Figures 3A,B). MSA formation was monitored using 3-methyl-2-benzothiazolinone (MBTH). This reagent enables for the spectrophotometric quantification of aliphatic aldehydes at 630 nm in a microplate reader (Cummins and Hauser, 1964; Zurek and Karst, 1997). Formaldehyde samples of a defined concentration were used to calibrate the system. In Figure 3B, the linear time course of the established carnitine monooxygenase assay is shown which yields a specific activity of 2.8 ± 0.2 μmol min−1 mg−1. A closely related value of 2.7 ± 0.4 μmol min−1 mg−1 was calculated on the basis of the well-established L-carnitine depletion assay (Figure 3A) [previously calculated value of 771 nmol min−1 mg−1 (Massmig et al., 2020)]. The new assay was used for the analysis of the substrate profile of the A. baumannii carnitine monooxygenase. Kinetic experiments in the presence of γ-butyrobetaine revealed a high activity of 2.1 ± 0.2 μmol min−1 mg−1 which is in agreement with a recently published structural investigation (Quareshy et al., 2020). The observed aldehyde formation suggests an analogous reaction mechanism for the conversion of L-carnitine and γ-butyrobetaine. No detectable activity was observed in the presence of choline or glycine betaine (Figure 3B). From this data we conclude that MBTH-based kinetic experiments are a good alternative to the kinetic determinations described so far.
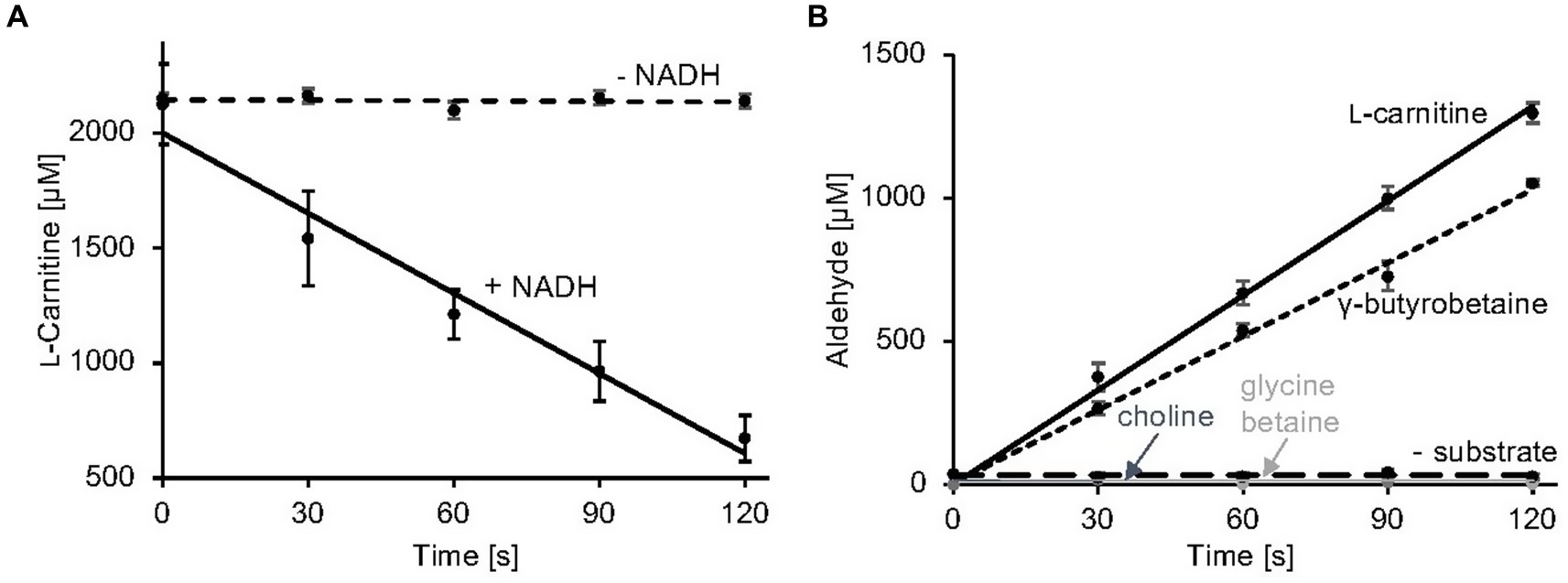
Figure 3. Carnitine monooxygenase activity assays. (A) The standard L-carnitine depletion assay containing purified CntA and CntB was initiated by the addition of NADH. L-carnitine concentrations were quantified colorimetrically in a coupled carnitine acetyltransferase reaction as recently described (Piskol et al., 2022) R2 values: 0.98 (−NADH), 0.96 (+NADH). (B) The newly established carnitine monooxygenase assay monitors MSA production spectrophotometrically using 3-methyl-2-benzothiazolinone (MBTH). Aliphatic aldehyde concentrations were determined in a microplate reader at a wavelength of 630 nm. A control reaction was performed in the absence of substrate. High specific activity was also observed in the presence of the alternative substrate γ-butyrobetaine. No detectable activity was measured in the presence of choline or glycine betaine. R2 values: 0.99 (L-carnitine), 0.98 (γ-butyrobetaine), 0.99 (choline), 0.99 (glycine betaine), 0.99 (− substrate). Data shown as mean ± SD from three independent experiments.
3.3 Characterization of MSA-DH
UV–visible absorption spectroscopy of concentrated protein samples did not show the presence of a chromophoric cofactor. Analytical size exclusion chromatography revealed a native molecular mass of 281 kDa (Figure 2C), indicative for a tetrameric quaternary structure of MSA-DH (266 kDa calculated theoretically). However, the methodology used is primarily based on the Stokes radius of the analyzed (fusion) protein. This hydrodynamic value might be strongly influenced by the molecular shape of the MSA-DH fusion protein.
Mass photometry is an alternative experimental technique which allows for the mass measurement of native molecules in solution. Particles are illuminated with a focused laser beam, and their motion is captured by a camera. By analyzing the fluctuations in the scattered light, it’s possible to derive the mass of biomolecules (Soltermann et al., 2020). As indicated in the histogram of Figure 2D, a native mass of 258 kDa for MSA-DH was determined by mass photometry. This result is in good agreement with the analytical size exclusion experiments. Accordingly, A. baumannii MSA-DH was found to function as a biological tetramer.
3.4 Enzymatic activity of MSA-DH
The aldehyde substrate of the proposed MSA-DH is not commercially available. Therefore, the enzymatic activity of MSA-DH was initially shown in a coupled assay containing carnitine monooxygenase (5 μM CntA and 15 μM CntB) and 5 μM MSA-DH in the presence of the respective cofactors NADH (10 mM) and NAD+ (10 mM). By addition of 10 mM L-carnitine, formation of substantial amounts of malate was demonstrated in the HPLC-based approach depicted in Figure 4A (yellow line). Obviously, the reaction of carnitine monooxygenase (or of MSA-DH) is not affected by the presence of high NAD+ (or NADH) concentrations. This is a good prerequisite for the intended in vitro reconstitution of the overall pathway.
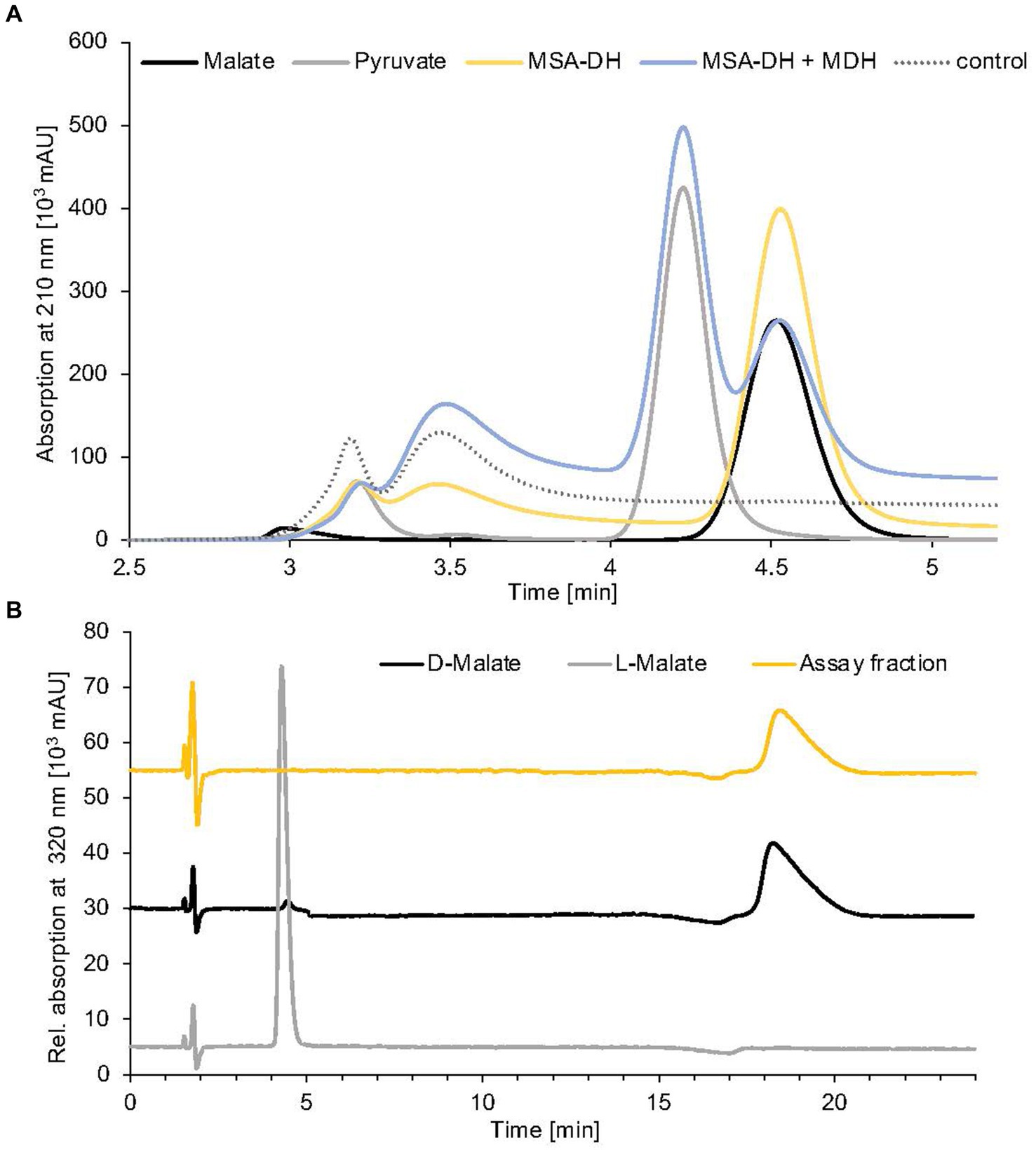
Figure 4. Identification of MSA-DH and MDH reaction products. (A) HPLC analysis of pathway reconstitution experiments containing MSA-DH (yellow) or MSA-DH and MDH (blue). The substrate MSA was synthesized in situ by the carnitine monooxygenase subunits CntA and CntB. An authentic malate (black) or pyruvate (grey) standard and a control reaction in the absence of MSA-DH and MDH (dotted) were analyzed accordingly. HPLC separation was performed on a Repromer H column using 3 mM sulfuric acid as a mobile phase at a flow rate of 0.5 mL min−1 at room temperature. Absorption was monitored at 210 nm. (B) Chiral HPLC analysis of the MSA-DH derived reaction product malate. The MSA-DH containing pathway reconstitution experiment from A was further analyzed. The malate-containing fraction was subjected to enantiomer separation on a Chirex 3126 ligand exchange phase (yellow). Authentic D- and L-malate samples were analyzed as standards (black and grey). Isocratic separation was performed at a flow rate of 1 mL min−1 at 27°C using 85% (v/v) 100 mM ammonium acetate, 1 mM Cu(II)-acetate (pH 4.5) and 15% (v/v) 2-propanol as a mobile phase.
For the subsequent characterization of MSA-DH, substantial amounts of the enzyme substrate were produced enzymatically. The CntA/CntB reaction was carried out on a large scale in the presence of high concentrations of L-carnitine (20 mM) and NADH (20 mM). After an incubation time of 60 min, quantitative substrate and NADH conversion with equimolar MSA production was verified by absorption spectroscopy in combination with the MBTH-based aldehyde quantification method. Subsequent precipitation of CntA/CntB revealed an MSA-containing fraction which was used to provide the enzyme substrate for further biochemical experiments.
In a first MSA-DH in vitro assay, substrate-dependent formation of NADH was monitored spectroscopically (Figure 5A). These experiments indicated a pH optimum of pH 7.5–8 in the presence of 400 μM MSA and 2 mM NAD+. A specific MSA-DH activity of 7.5 ± 0.2 μmol min−1 mg−1 was determined. An alternative MSA-DH in vitro activity assay was developed which monitors the depletion of the aldehyde substrate using an MBTH-based approach (Figure 5B). A comparable specific MSA-DH activity of 9.7 ± 1.1 μmol min−1 mg−1 was measured.
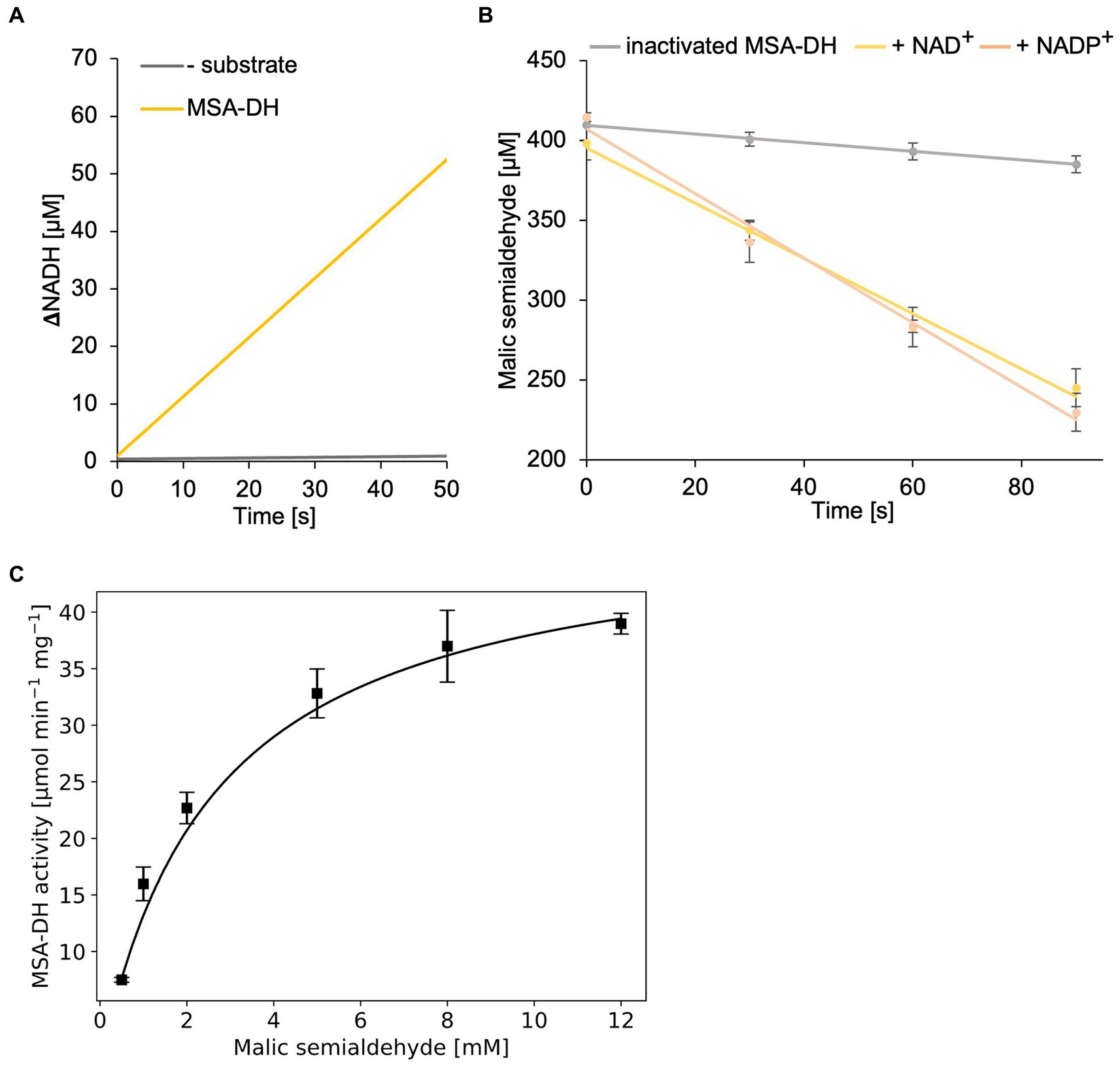
Figure 5. In vitro enzyme kinetics and cofactor specificity of MSA-DH. (A) Continuous measurement of in vitro MSA-DH activity (0.2 μM) in the presence of 400 μM MSA and 20 mM NAD+. A linear increase of the NADH concentration (yellow) was determined by measuring the absorption at 340 nm. (B) MSA-DH dependent depletion of the MSA substrate in the presence of 2 mM NAD+ (yellow) or NADP+ (light orange). MSA concentrations were determined spectrophotometrically using the 3-methyl-2-benzothiazolinone (MBTH) method. A control reaction was performed with inactivated MSA-DH (grey). R2 values: 0.99 (inactivated MSA-DH), 0.99 NAD+, 0.99 NADP+. (C) Michaelis–Menten kinetics of MSA-DH. Initial rates were measured as in A with MSA concentrations ranging from 0.5–12 mM in the presence of 0.2 μM enzyme. R2 value: 0.98. (B,C) Each point represents the mean of three independent experiments.
MSA-DH activity experiments in the presence of the cofactor NAD+ or NADP+ (2 mM) revealed closely related enzyme activities (Figure 5B). Thus it was concluded that MSA-DH can use NAD+ or NADP+ as a cofactor for the oxidation of the MSA substrate under in vivo conditions. Experiments with varying substrate concentrations revealed Michaelis–Menten type kinetics with a Vmax of 48.1 ± 1.4 μmol min−1 mg−1 and a Km of 2.6 ± 0.14 mM MSA (Figure 5C).
3.5 Specific formation of D-malate by MSA-DH
Conversion of MSA into malate in MSA-DH activity experiments was confirmed by standard HPLC experiments (Figure 4A, yellow line). However, the understanding of the stereochemistry of the MSA-DH reaction is crucial for the elucidation of the overall carnitine degradation pathway: L-malate might be directly channeled into the tricarboxylic acid cycle whereas D-malate would require a specific oxidation step that potentially yields the central metabolite oxaloacetate or pyruvate.
Reaction products from a standard MSA-DH assay were fractionated by preparative HPLC separation (compare Figure 4A) and the malate containing fraction was subjected to enantiomeric separation on a Chirex ligand exchange phase 3126. Authentic L-malate or D-malate samples reproducibly eluted at 4.4 min or 18.3 min (Figure 4B, grey and black line). MSA-DH dependent formation of D-malate was indicated at a retention time of 18.4 min (yellow line). Based on these findings, it was concluded that A. baumannii MSA-DH catalyzes the stereospecific formation of D-malate which might be further metabolized as part of the degradation pathway.
3.6 The three-dimensional crystal structure of MSA-DH
To further investigate the molecular mechanism and cofactor utilization of MSA-DH, the X-ray protein structure was determined at 2.6 Å (data collection and refinement statistics in Supplementary Table S1). The asymmetric unit contains an overall of three individual MSA-DH homotetramers which is consistent with our gel filtration and mass photometry experiments. The biological unit is composed of two obligate dimers (e.g., AB and CD colored brown/blue and green/pink) which are arranged to generate a tetramer with almost perfect D2 symmetry (Figure 6). Corresponding monomers show a root-mean-square deviation (r.m.s.d of all Cα’s) of 0.116 to 0.212 Å.
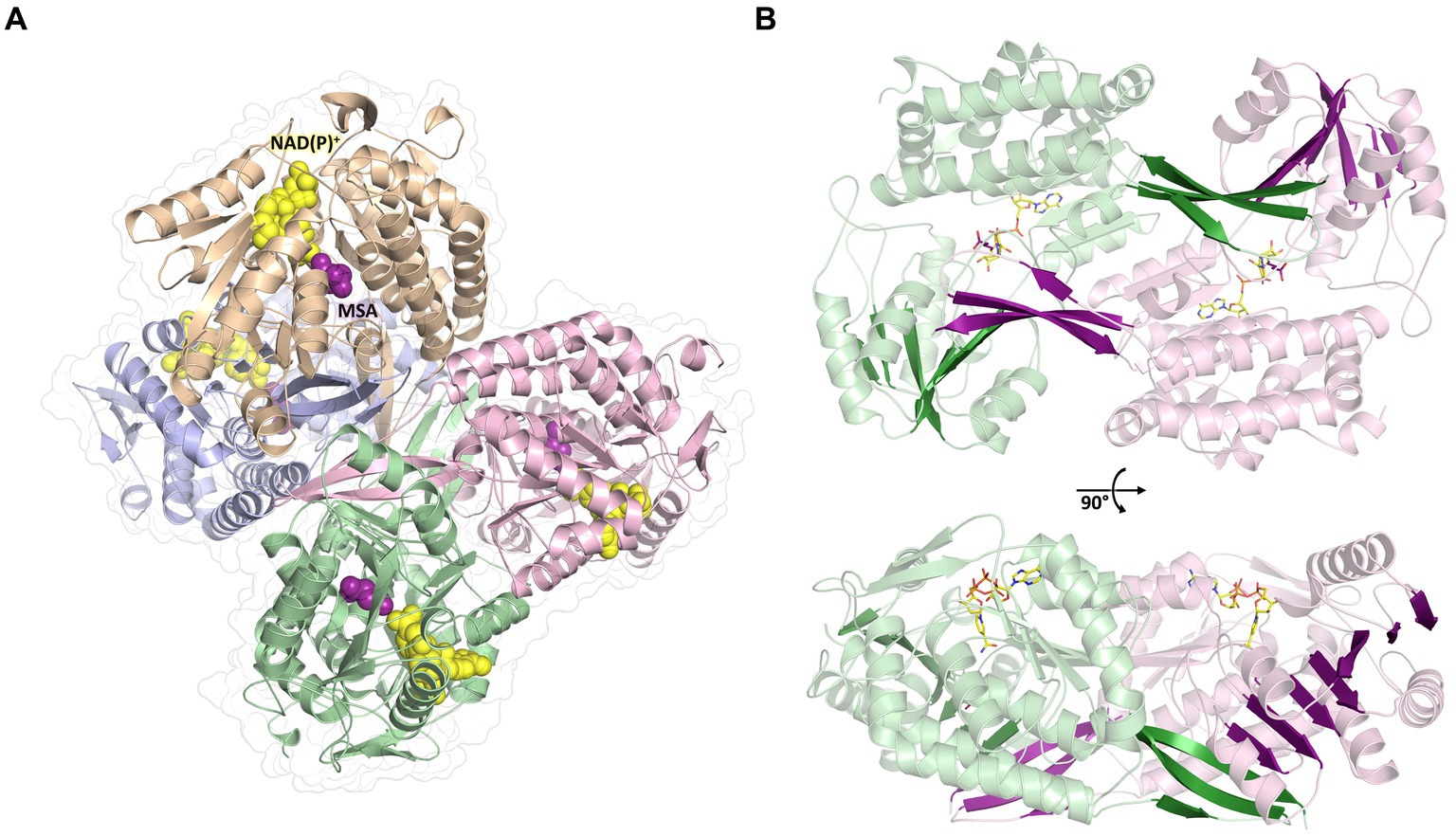
Figure 6. Overall crystal structure of MSA-DH. (A) Structure of the native tetramer. In silico modeled NAD(P)+ and MSA are shown as yellow and purple spheres. (B) Obligate dimer with modeled ligands shown as sticks. The 10-stranded β-sheets formed by domain swapping are highlighted.
The overall fold of MSA-DH is similar to other proteins of the aldehyde dehydrogenase family (Shortall et al., 2021). The L-shaped monomer (Figure 7) consists of the N-terminal Rossmann dinucleotide-binding domain (green, residues 1–126 and 150–261), the α/β catalytic domain (blue, residues 262–441), and the oligomerization domain (brown, residues 127–149 and 441–483). The latter part of the molecule is responsible for the domain-swapping dimerization of AB or CD. Three β-strands of the oligomerization domain and seven β-strands from the catalytic domain form a common 10-stranded β-sheet, respectively (Figure 6B, highlighted green and purple).
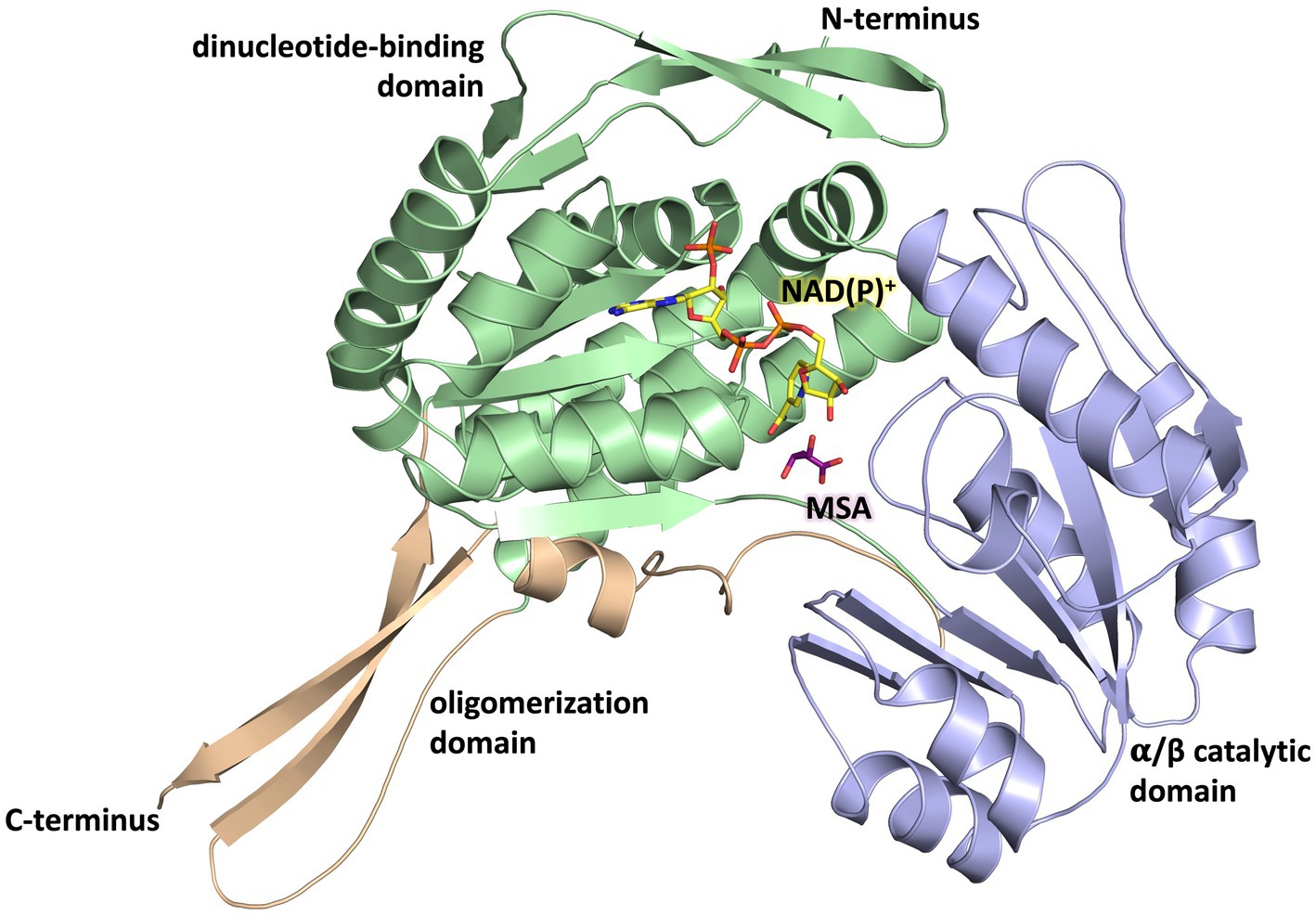
Figure 7. Domain architecture of the MSA-DH monomer. The Rossmann dinucleotide-binding domain is shown in green (residues 1–126, 150–261), the α/β catalytic domain is shown in blue (residues 262–441) and the oligomerisation domain is shown in orange (residues 127–149, 441–483). The in silico modeled NAD(P)+ and MSA are shown in yellow and purple.
Every monomer possesses two funel-like invaginations (Figure 8) which were proposed as potential entry sites for the MSA substrate and the cofactor NAD(P)+, respectively. Starting from opposite sides, both channels meet in a region where the two highly conserved amino acids Cys290 and Glu256 are localised. The corresponding cystein and glutamate residues have been described as key catalytic residues of the aldehyde dehydrogenase family (Shortall et al., 2021). Subsequently, the potential cofactor and substrate binding sites of MSA-DH were explored on the basis of structurally related aldehyde dehydrogenase protein structures.
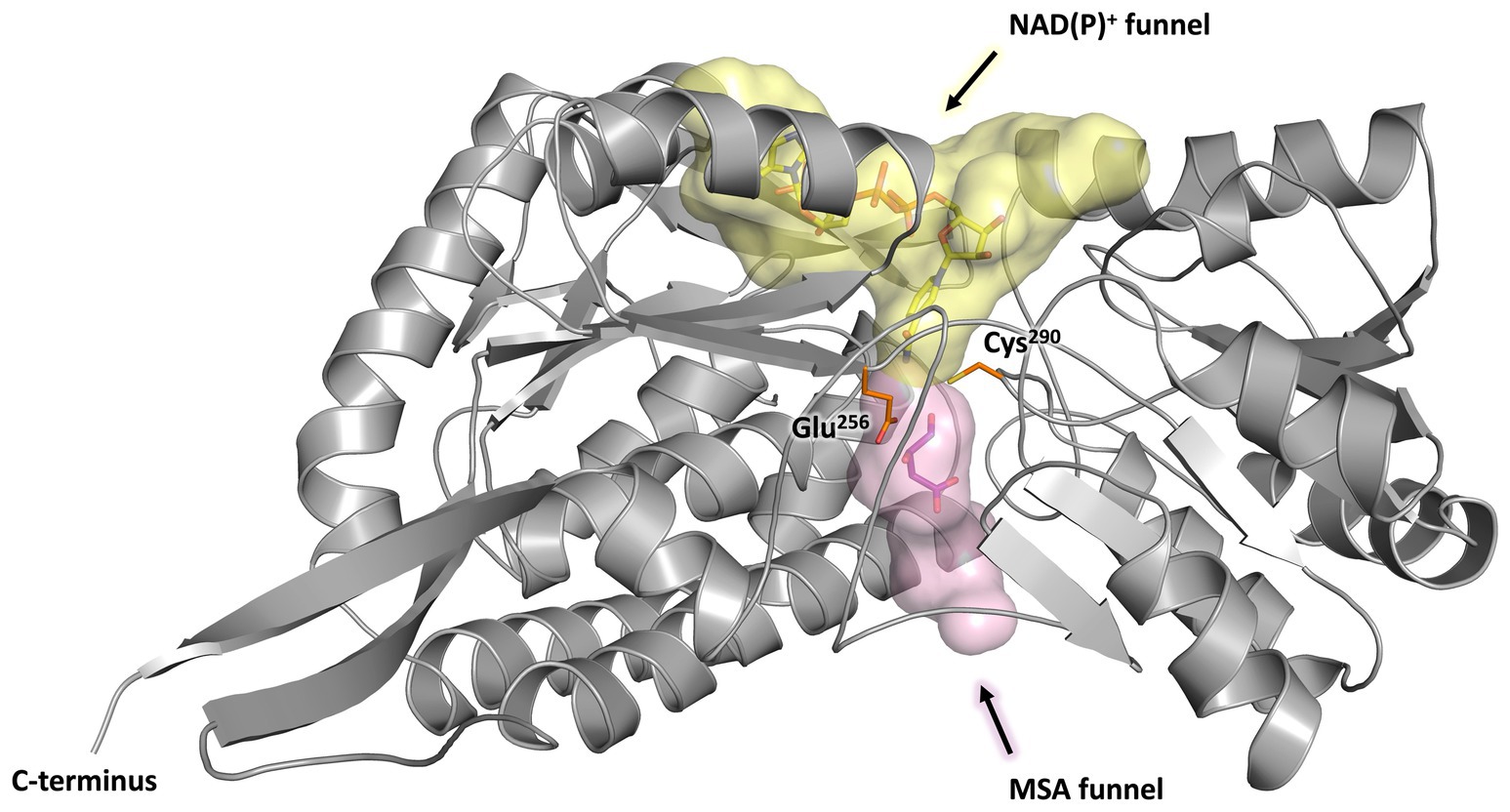
Figure 8. Substrate and co-substrate funnels of MSA-DH. A monomer of MSA-DH (grey) with the NAD(P)+ funnel (depicted as yellow spheres) and the MSA funnel (purple spheres) as found by the PyMOL plugin CavitOmiX (v. 1.0, 2022, Innophore GmbH) is shown. NAD(P)+ and MSA have been modeled into the corresponding funnels and are shown as yellow and purple sticks. The key catalytic residues Glu256 and Cys290 are shown as orange sticks.
3.7 Cofactor utilization of MSA-DH
Coordinates of E. coli succinic semialdehyde dehydrogenase in complex with NADP+ (PDB ID: 3JZ4) (Langendorf et al., 2010) were structurally superimposed to the native MSA-DH structure (Supplementary Figure S1). As indicated in Figures 7–9, the NADP+ molecule was located in a well-defined cofactor binding pocked which did not produce steric clashes. Residues Trp157, Lys181, Ser235 and Glu387 of the proposed nucleotide binding pocket (Supplementary Figures S1, S2) of MSA-DH revealed a direct counterpart in the E. coli co-crystal structure (residues Trp155, Lys179, Ser233 and Glu385). Accordingly, the solved protein structure with the superimposed NADP+ molecule was used to assess the cofactor specificity of A. baumannii MSA-DH. Based on related structural investigations, one key residue of aldehyde dehydrogenases was identified that is of importance for the recognition (or discrimination) of NAD+ and/or NADP+ (Yuan et al., 2013). The respective amino acid of MSA-DH was identified as Asn184. This asparagine residue does not allow discrimination of the extra phosphate group of NADP+ (as observed in the presence of a glutamate). Furthermore, Asn184 cannot mediate a favorable interaction with the NADP+ phosphate group (as observed in the presence of a serine or threonine). Accordingly, a relaxed NAD(P)+ cofactor specificity was expected on the basis of the solved MSA-DH structure. Our structural work thus explains the almost identical MSA-DH activities in the presence of either NAD+ or NADP+.
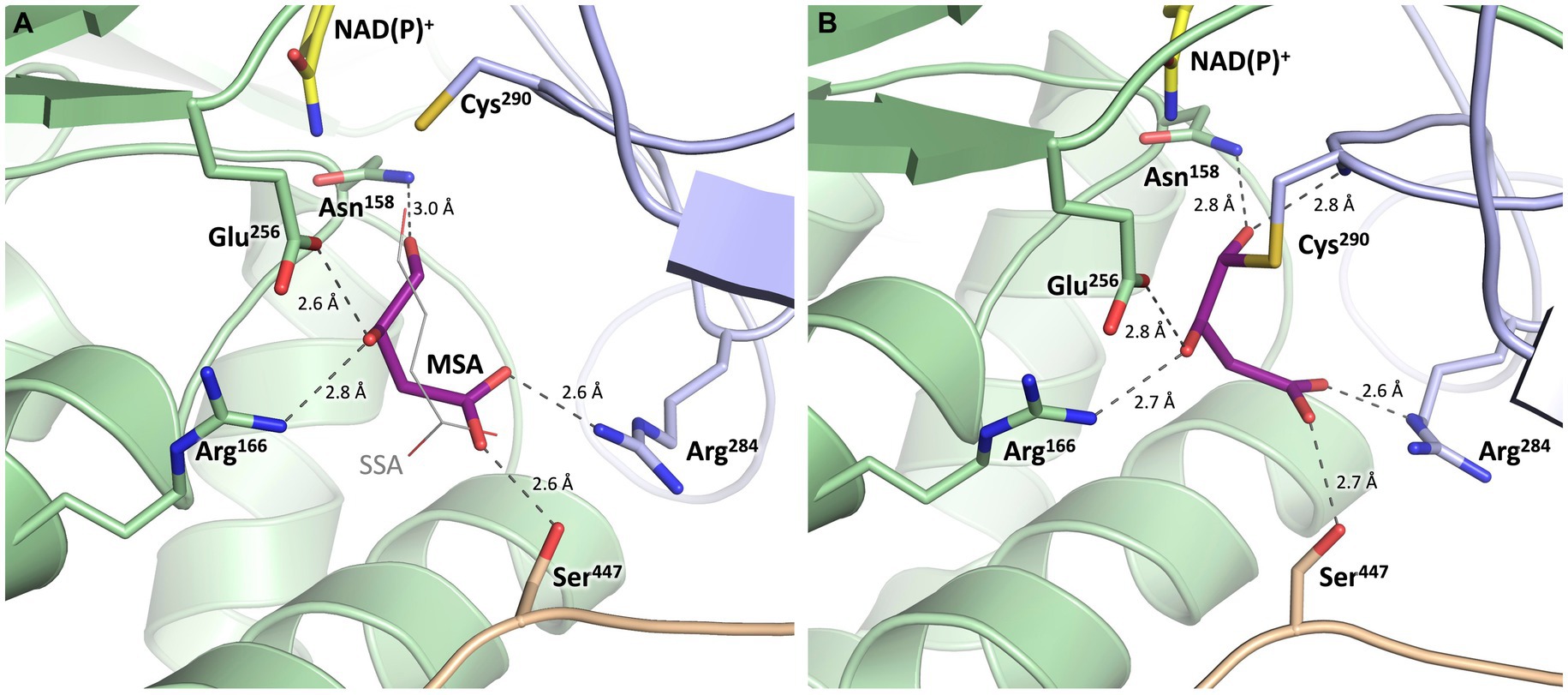
Figure 9. Substrate binding site of MSA-DH with in silico modeled ligands. Hydrogen bonds are shown as dashed lines. (A) Succinic semialdehyde (SSA, thin, grey) was superimposed using human succinic semialdehyde dehydrogenase (2W8Q). MSA (purple) was modeled by addition of the 3-hydroxyl group and energy minimization. (B) Model of a reaction intermediate (purple, modeled from A) covalently bound to Cys290.
3.8 Proposed substrate recognition and enzyme mechanism of MSA-DH
The potential substrate binding site of MSA-DH was explored by superimposing the three-dimensional structure of human succinic semialdehyde dehydrogenase in complex with its substrate (PDB ID: 2W8Q) (Kim et al., 2009) (Supplementary Figure S1). Succinic semialdehyde (4-oxobutanoate) acts as a suitable substrate ´analog` as this molecule only lacks the 3-hydroxy group of MSA (3-hydroxy-4-oxobutanoate) which could be added in silico.
In Figure 9, the resulting model with MSA (purple) in the proposed active site of MSA-DH is depicted. The in silico experiment revealed a well-defined substrate binding pocked that did not cause steric conflicts when the 3-hydroxy group of MSA was added and the model was energy minimized. The obtained active site model was further substantiated as amino acid residues Arg166, Arg284 and Ser447 were located at an appropriate distance for hydrogen bonding interaction with the carboxylate group of the substrate. A very similar network of hydrogen bonds was also observed in the complex structure of the human succinic semialdehyde dehydrogenase (residues Arg213, Arg334 and Ser498, compare Supplementary Figure S1) (Kim et al., 2009).
Subsequently, the enzymatic mechanism of MSA-DH was deduced from the solved X-ray crystal structure with the superimposed MSA and NADP+ molecule. As can be seen from Figure 9A, the highly conserved residues Cys290 and Glu256 are located in a suitable spatial arrangement to the carbonyl of the MSA substrate. Therefore, a typical aldehyde dehydrogenase catalytic mechanism was concluded. Cys290 in the active center is poised to nucleophilically attack the MSA substrate which results in the formation of a covalent hemithioacetal. Subsequently, hydride transfer from the covalent intermediate to the NAD(P)+ cofactor results in the formation of a thioacyl intermediate (compare the modelled reaction intermediate depicted in Figure 9B). Finally, a water molecule which is deprotonated by Glu256 attacks the thioacyl enzyme intermediate. This results in the formation of malate and the regeneration of residue Cys290. As also shown in related structural studies, the general base Glu256 could further support the hydride transfer step of MSA-DH catalysis (Kim et al., 2009; Vorobieva et al., 2014; Shortall et al., 2021). Supplementary Figure S3 illustrates the spatial interplay of cofactor and substrate in the MSA-DH active site on the basis of the NAD(P)+ and the MSA funnel.
For human succinic semialdehyde dehydrogenase, reversible disulfide bond formation of the active site cysteine (Cys340) and an adjacent residue (Cys342) was described as regulatory redox-switch mechanism. The purified enzyme was completely inactive in the presence of molecular oxygen and the respective crystal structure revealed a substantial rearrangement of the active site loop region. For this enzyme, in vitro activity was only observed under reducing conditions. The active site residue Cys290 of A. baumannii MSA-DH is also neighbored by the fully conserved Cys292. Our protein was purified, crystallized and kinetically characterized in the absence of any reducing agents. The solved crystal structure places the active site loop in a conformation which closely resembles the reduced `active state` of human succinic semialdehyde dehydrogenase. Extensive exposure to molecular oxygen, but also the addition of reducing agents did not influence the specific activity of MSA-DH (air exposure for four days, 7.3 ± 0.3 μmol min−1 mg−1; 10 mM DTT for one h, 7.4 ± 0.3 μmol min−1 mg−1). Accordingly, our structural and biochemical data do not support a regulatory switch mechanism for the MSA-DH enzyme from A. baumannii.
3.9 Recombinant production and purification of the postulated MDH
Subsequent investigations were performed to elucidate the involvement of the postulated MDH enzyme in the L-carnitine degradation pathway. E. coli cells were used to produce MDH from A. baumannii fused to an N-terminal His-tag. SDS-PAGE analysis revealed an induction band with a relative molecular mass of ∼43,000 Da (calculated molecular mass, 44,007 Da). Ni2+-loaded chelating Sepharose was used for the affinity purification of the target protein (Figure 2B, lanes 1–3 right). A yield of approximately 40 mg purified MDH was obtained per liter cell culture.
3.10 Characterization of the proposed MDH
Concentrated protein samples of MDH did not indicate the presence of a chromophoric cofactor as judged bei UV–visible absorption spectroscopy. Analytical size exclusion chromatography revealed a native molecular mass of 234,000 Da, which is in agreement with a tetrameric or a pentameric MDH architecture (Figure 2C). Accordingly, mass photometry was used to verify the quaternary MDH structure. The histogram of Figure 2D indicates a native mass of 175 kDa (calculated native mass, 176,028 Da). These findings clearly show that A. baumannii MDH is a functional tetramer.
3.11 Enzymatic activity of MDH
The enzymatic activity of the proposed MDH was initially demonstrated in pathway reconstitution experiments. Therefore, a coupled assay containing carnitine monooxygenase, MSA-DH and 5 μM MDH in the presence of the respective cofactors NADH (10 mM) and NAD+ (10 mM) was performed (Figure 4A). HPLC analysis revealed L-carnitine dependent formation of malate with the simultaneous appearance of a second dominant peak at a retention time of 4.2 min. By comparison with an authentic sample, the MDH-dependent formation of pyruvate was demonstrated (blue trace). No formation of oxaloacetate was detected in the experiments performed. Thus, it was concluded that A. baumannii MDH belongs to the family of β-decarboxylating dehydrogenases which exclusively form pyruvate as a reaction product (Vorobieva et al., 2014).
Alternatively, MDH activity was followed in a spectroscopic assay which monitors the formation of NADH (Figure 10A). In the presence of 2 mM D-malate and 20 mM NAD+, a specific MDH activity of 7.0 ± 0.3 μmol min−1 mg−1 was determined. Michaelis–Menten type kinetics were determined with a Vmax of 14.2 ± 0.4 μmol min−1 mg−1 and a Km of 2.1 ± 0.2 mM for D-malate (Figure 10B).
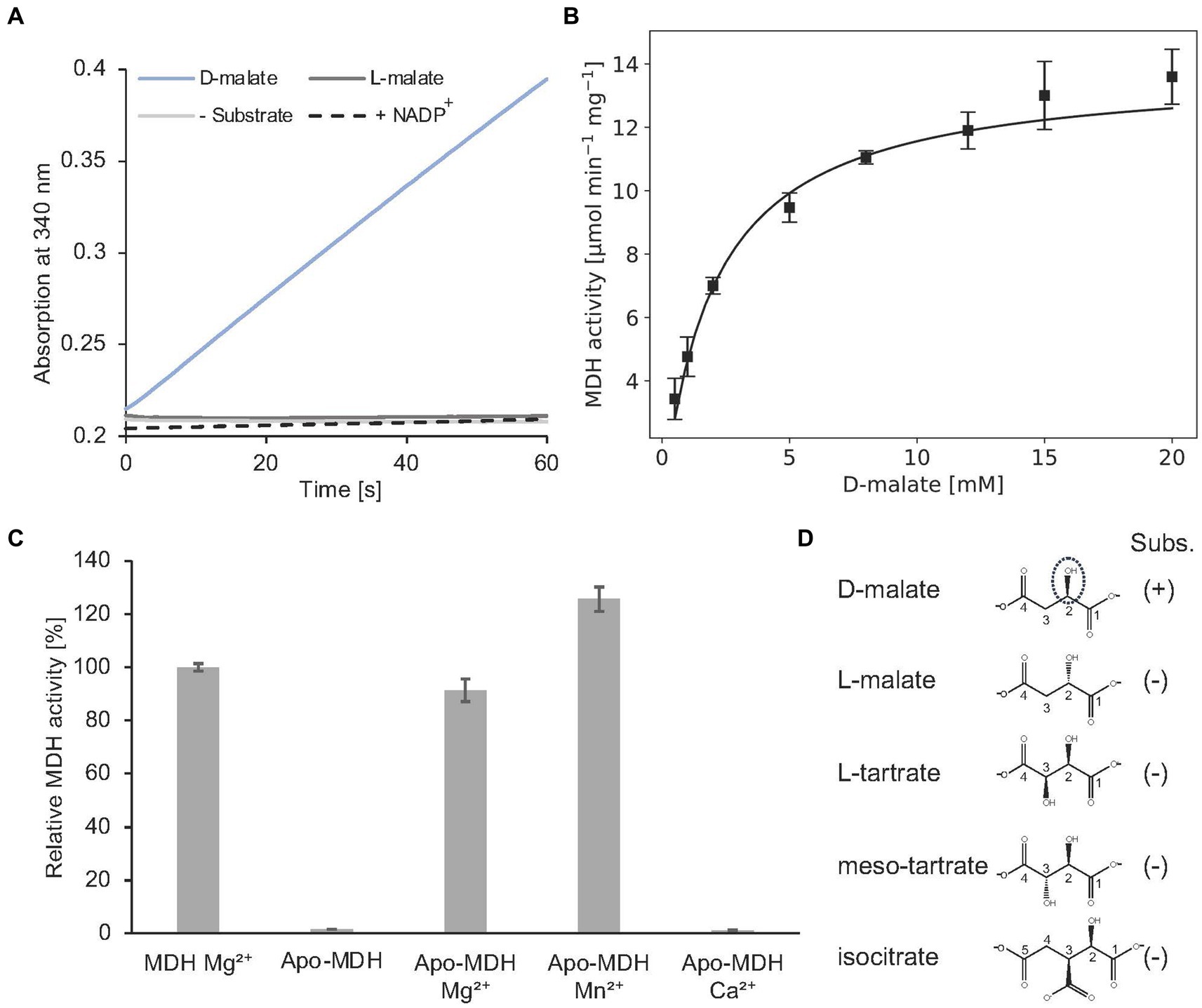
Figure 10. In vitro enzyme kinetics, metal cofactor and substrate specificity of MDH. (A) Continuous in vitro MDH experiments (0.2 μM) in the presence of 400 μM D- or L-malate (blue or dark grey) and 20 mM NAD+. The absorption increase at 340 nm due to the formation of NADH was monitored. A control reaction was carried out without substrate (grey). NADP+ was tested as an alternative cofactor in the presence of D-malate (dashed). (B) Michaelis–Menten kinetics of MDH. Initial rates were measured as in A with D-malate concentrations ranging from 0.5–20 mM in the presence of 0.2 μM enzyme. R2 value: 0.94. (C) Enzymatically active MDH (MDH Mg2+) showed a complete loss of enzyme activity after EDTA treatment and dialysis (Apo-MDH), which was efficiently restored by the addition of Mg2+ (Apo-MDH Mg2+) or Mn2+ (Apo-MDH Mn2+). Ca2+ supplementation did not restore MDH activity (Apo-MDH Ca2+). (D), Compounds L-malate, L-tartrate, meso tartrate and isocitrate were tested as alternative substrates of MDH as described under A. (B,C), Each point represents the average of three independent experiments.
Complete loss of activity was observed after EDTA treatment and dialysis of MDH. Enzymatic activity of this apo enzyme was fully restored upon Mg2+ or Mn2+ addition. No MDH activity was observed by Ca2+ addition (Figure 10C). From these data a Mg2+ or Mn2+-dependent catalysis was concluded as also demonstrated for other members of the enzyme superfamily (Hurley et al., 1991; Vorobieva et al., 2014).
3.12 MDH catalysis is highly specific
The orthologous D-malate dehydrogenase from E. coli (DmlA) was described as a generalist enzyme that contributes to two distinct physiological pathways as indicated by high catalytic activity on alternative substrates (L-tartrate, D-malate, and isocitrate). Substrate promiscuity has been also demonstrated for related enzymes of the superfamily (Miyazaki, 2005b,a).
As indicated in Figure 10A, A. baumannii MDH is not able to convert L-malate. Furthermore, NADP+ cannot serve as a substitute for the employed NAD+ cofactor of MDH. The following compounds with a substitution at the C-3 position were tested as alternative MDH substrates: L-tartrate, meso-tartrate and isocitrate (Figure 10D). These molecules did not reveal detectable enzyme activity.
Presence of 2 mM meso-tartrate in the standard MDH activity assay (containing 2 mM D-malate) revealed a significant reduction of the enzyme activity (0.93 ± 0.1 μmol min−1 mg−1). Experiments with varying inhibitor concentrations revealed an inhibition constant Ki of 0.32 ± 0.08 mM. Competitive inhibition of MDH in the presence of high meso-tartrate concentrations was concluded (Supplementary Figure S4).
In summary, it has been shown that the MDH of A. baumannii is a highly specific β-decarboxylating dehydrogenase. Accordingly, catabolism of L-carnitine proceeds via MSA and D-malate and leads to the formation of trimethylamine and pyruvate.
3.13 The cntA containing gene cluster of E. coli
Bacterial L-carnitine metabolism is important for understanding the resilience of potentially pathogenic organisms. However, the overall contribution of the CntA-mediated conversion of L-carnitine has been controversial in the context of microbiome investigations (Zhu et al., 2014; Rath et al., 2018, 2019). For a specific organism, it is not clear whether the presence of the cntA gene directly correlates with substantial L-carnitine metabolism and the formation of TMA.
Based on bioinformatics investigations, orthologous cntA genes were identified in different genomic contexts (Zhu et al., 2014). The carnitine degradation cluster of A. baumannii comprises the LysR-type transcriptional regulator carR, mdh, the betaine/choline/carnitine transporter gene bcct, the gene for a proposed acylcarnitin hydrolase hyd which is followed by cntA, msadh and cntB. The related cntA containing cluster of E. coli only reveals an overall of five orthologous gens: carR, mdh, bcct, cntA, and cntB showing substantial protein sequence identity of 45, 72, 56, 72 and 52%, respectively (Supplementary Figure S5). The gene cluster clearly differs due to the absence of the hyd and the msadh gene. However, at a distant site in the genome, E. coli also encodes for a reductase protein (GabD) showing 62% identity when compared to A. baumannii MSA-DH. Based on these theoretical investigations, it is not clear whether E. coli is capable of using the same carnitine degradation pathway as A. baumannii. In the current literature, there is no experimental evidence of E. coli growth using L-carnitine as the sole carbon and energy source (Jung et al., 1987).
3.14 No growth of E. coli strains on L-carnitine
High in vitro enzyme activity of recombinantly overproduced carnitine monooxygenase from E. coli was recently demonstrated (Piskol et al., 2022). However, a manual search of high throughput gene expression data (NCBI GEO database) (Edgar et al., 2002) did not indicate experimental conditions that revealed substantial cntA gene transcription under in vivo conditions. This was confirmed when the based carnitine monooxygenase assay was performed using a cellular extract of E. coli BL21. The laboratory strain after cultivation in complex medium did not indicate detectable carnitine monooxygenase activity.
Subsequently, growth experiments on carnitine containing minimal medium were initiated using different strains of E. coli. Strains BL21, B and K12 did not showed measurable growth (Supplementary Figure S6). These initial experiments might indicate that the cntA containing operon of E. coli serves a different physiological function when compared to the related A. baumannii gene cluster. However, these findings show that, e.g., strain E. coli BL21 is a suitable platform for the investigation of the A. baumannii carnitine degradation pathway.
3.15 In vivo reconstitution of the carnitine degradation pathway
E. coli BL21 carrying two IPTG inducible overexpression plasmids was used for the heterologous in vivo reconstitution of the overall carnitine degradation pathway of A. baumannii. Overproduction of carnitine monooxygenase subunits CntA and CntB, MSA-DH, MDH and BCCT resulted in a strain that was able to grow slowly on L-carnitine as the sole carbon and energy source (Supplementary Figure S6). After a prolonged lag phase, an OD578 of 0.6 was achieved after 24 h of incubation. Carnitine metabolism of this strain was widely recognizable by a distinct TMA odor of the respective culture. Obviously, the alternative metabolic pathway has been successfully implemented in E. coli. In order to achieve a more efficient growth of the culture, it may be necessary to have a precisely balanced ratio of the reconstituted protein components. In vitro reconstitution of the pathway was only observed upon IPTG induction.
4 Discussion
L-carnitine is a quaternary amine compound that serves a variety of physiological functions. Due to its zwitterionic nature, it plays an important role in protecting microbes from osmotic, thermal, cryogenic, and barometric stresses by acting as a compatible solute (Kets et al., 1994; Jebbar et al., 1998; Verheul et al., 1998). Furthermore, L-carnitine has been also described as a final electron acceptor under anaerobic conditions in the presence of additional carbon and nitrogen sources (Seim et al., 1982; Jung et al., 1987; Walt and Kahn, 2002; Meadows and Wargo, 2015).
For A. baumannii, a gene cluster for the metabolism of L-carnitine under aerobic conditions was predicted and bacterial growth on L-carnitine as the sole carbon and energy source was demonstrated (Zhu et al., 2014; Breisch et al., 2022). This degradation pathway was also linked to the virulence of the opportunistic pathogen (Breisch et al., 2022). Previous biochemical studies addressed the LysR-type transcriptional activator (lysR), the energy-dependent import system BCCT and the two-component carnitine monooxygenase which catalyzes the oxygen-dependent cleavage of L-carnitine into TMA and MSA (Zhu et al., 2014; Breisch et al., 2019; Massmig et al., 2020; Breisch et al., 2022; Piskol et al., 2022). Here we focussed on the remaining pathway enzymes and the funnelling of the reaction intermediates into the central metabolism. The protein crystal structure of MSA-DH in combination with biochemical studies revealed a covalent catalytic mechanism leading to the enantiomer-specific formation of D-malate. The stereochemistry of the reaction product D-malate hampers direct channelling into the tricarboxylic acid cycle. We were able to show that the further required enzyme MDH is a β-decarboxylating dehydrogenase with unusually high substrate specificity. Finally, our in vivo and in vitro reconstitution experiments clearly demonstrated the conversion of L-carnitine via MSA and D-malate to pyruvate, CO2 and TMA.
From the evolutionary perspective, the formation of the unusual D-malate intermediate prevents the direct channeling of malate into the TCA cycle which requires the presence of one further enzyme (MDH). But why has no synthetic pathway evolved that leads directly to the synthesis of L-malate? As can be seen in Figure 1, the stereo center of L-carnitine remains unaltered throughout the individual reaction steps. Neither the enzymatic mechanism of CntA/CntB, nor the reaction sequence of MSA-DH relate to this atom. This shows that a metabolic pathway leading to L-malate would only be possible with the involvement of an additional racemase.
In recent years, the bacterial formation of TMA has attracted much attention due to its medical relevance. TMA has been shown to be a gut microbiota-dependent metabolite that is associated with an increased risk of cardiovascular disease. TMA formed from dietary nutrients such as carnitine or choline is absorbed by the intestinal epithelium and subsequently oxidized to TMAO by hepatic flavin monooxygenases. Several studies have found a positive association between elevated plasma TMAO levels and mortality, particularly deaths due to cardiovascular and renal disease (Kanitsoraphan et al., 2018; Chen et al., 2022). In this context, the capacity of the gut microbiota for TMA production was assessed, e.g., by detecting the presence of the following enzymes: Choline-TMA lyase (cutC), betaine reductase (grdH) and carnitine monooxygenase (cntA) (Falony et al., 2015; Rath et al., 2017, 2019). Based on the results of the present investigation, we propose that cntA dependent TMA synthesis should not be judged solely by the presence of the respective gene. We suggest that the genomic context of the cntA gene must be also taken into account. In Supplementary Figure S5 the structure of five different types of cntA containing operons from representative genomes of human gut microbiota is compared to the carnitine degradation cluster of A. baumanni and to the related gene cluster of E. coli (Zhu et al., 2014) (A-E). Judging from the results of the present investigation, only the operon structure C and E which is found in Providencia stuartii and Sporosarcina newyorkensis might facilitate the synthesis of substantial amounts of TMA. Organisms with a cntA gene cluster which is devoid of the msadh and/or the mdh gene as in the operon D of Achromobacter piechaudii were expected to mainly use the respective genes for the appropriate adjustment of intracellular L-carnitine concentrations. These organisms might use L-carnitine mainly as a compatible solute to provide protection against various environmental stress conditions. This hypothesis is in line with a previous study showing the absence of cntA expression in human fecal samples (Rath et al., 2018) or with another investigation that demonstrated poor correlation between cntA abundance and TMAO plasma levels (Wu et al., 2019). Further biochemical studies are required to quantitatively assess the contribution of the encoded TMA-producing enzymes within a given microbiome.
Data availability statement
The datasets presented in this study can be found in online repositories. The names of the repository/repositories and accession number(s) can be found below: Protein structure coordinates are deposited in the Protein Data Bank under the PDB entry code 8S33.
Author contributions
FP: Data curation, Formal analysis, Investigation, Validation, Writing – original draft, Writing – review & editing. PL: Data curation, Formal analysis, Investigation, Validation, Writing – original draft, Writing – review & editing. LK: Data curation, Formal analysis, Investigation, Validation, Writing – original draft, Writing – review & editing. AH: Data curation, Formal analysis, Investigation, Validation, Writing – original draft, Writing – review & editing. WB: Data curation, Formal analysis, Investigation, Validation, Writing – original draft, Writing – review & editing. DJ: Conceptualization, Data curation, Formal analysis, Investigation, Validation, Writing – original draft, Writing – review & editing. JM: Conceptualization, Data curation, Formal analysis, Investigation, Validation, Writing – original draft, Writing – review & editing, Project administration, Supervision.
Funding
The author(s) declare that no financial support was received for the research, authorship, and/or publication of this article.
Acknowledgments
We thank Simone Virus and Ute Widow for their excellent technical assistance.
Conflict of interest
The authors declare that the research was conducted in the absence of any commercial or financial relationships that could be construed as a potential conflict of interest.
The author(s) declared that they were an editorial board member of Frontiers, at the time of submission. This had no impact on the peer review process and the final decision.
Publisher’s note
All claims expressed in this article are solely those of the authors and do not necessarily represent those of their affiliated organizations, or those of the publisher, the editors and the reviewers. Any product that may be evaluated in this article, or claim that may be made by its manufacturer, is not guaranteed or endorsed by the publisher.
Supplementary material
The Supplementary material for this article can be found online at: https://www.frontiersin.org/articles/10.3389/fmicb.2024.1446595/full#supplementary-material
Abbreviations
BCCT, betaine/choline/carnitine transporter; HEPES, 4-(2-hydroxyethyl)-1-piperazineethanesulfonic acid; MBTH, 3-methyl-2-benzothiazolinone; IPTG, isopropyl-β-D-thiogalactopyranoside; MDH, D-malate dehydrogenase; MSA, malic semialdehyde; MSA-DH, malic semialdehyde dehydrogenase; TMA, trimethylamine
References
Adams, P. D., Afonine, P. V., Bunkóczi, G., Chen, V. B., Davis, I. W., Echols, N., et al. (2010). PHENIX: a comprehensive Python-based system for macromolecular structure solution. Acta Crystallogr. D Biol. Crystallogr. 66, 213–221. doi: 10.1107/s0907444909052925
Afonine, P. V., Grosse-Kunstleve, R. W., Echols, N., Headd, J. J., Moriarty, N. W., Mustyakimov, M., et al. (2012). Towards automated crystallographic structure refinement with phenix. Refine. Acta Crystallogr. D Biol. Crystallogr. 68, 352–367. doi: 10.1107/S0907444912001308
Ayoub Moubareck, C., and Hammoudi Halat, D. (2020). Insights into Acinetobacter baumannii: a review of microbiological, virulence, and resistance traits in a threatening nosocomial pathogen. Antibiotics 9:119. doi: 10.3390/antibiotics9030119
Breisch, J., Schumm, C., Poehlein, A., Daniel, R., and Averhoff, B. (2022). The carnitine degradation pathway of Acinetobacter baumannii and its role in virulence. Environ. Microbiol. 24, 4437–4448. doi: 10.1111/1462-2920.16075
Breisch, J., Waclawska, I., and Averhoff, B. (2019). Identification and characterization of a carnitine transporter in Acinetobacter baumannii. Microbiology 8:e00752. doi: 10.1002/mbo3.752
Burkhardt, A., Pakendorf, T., Reime, B., Meyer, J., Fischer, P., Stübe, N., et al. (2016). Status of the crystallography beamlines at PETRA III. Eur. Phys. J. Plus 131:56. doi: 10.1140/epjp/i2016-16056-0
Chen, G., He, L., Dou, X., and Liu, T. (2022). Association of Trimethylamine-N-oxide levels with risk of cardiovascular disease and mortality among elderly subjects: a systematic review and Meta-analysis. Cardiorenal Med. 12, 39–54. doi: 10.1159/000520910
Cummins, R., and Hauser, T. (1964). Increasing sensitivity of 3-methyl-2-benzothiazolone hydrazone. Test for analysis of aliphatic aldehydes in air. Anal. Chem. 36, 679–681. doi: 10.1021/ac60209a067
Dijkshoorn, L., Nemec, A., and Seifert, H. (2007). An increasing threat in hospitals: multidrug-resistant Acinetobacter baumannii. Nat. Rev. Microbiol. 5, 939–951. doi: 10.1038/nrmicro1789
Dummler, A., Lawrence, A. M., and de Marco, A. (2005). Simplified screening for the detection of soluble fusion constructs expressed in E. coli using a modular set of vectors. Microb. Cell Factories 4:34. doi: 10.1186/1475-2859-4-34
Edgar, R., Domrachev, M., and Lash, A. E. (2002). Gene expression omnibus: NCBI gene expression and hybridization array data repository. Nucleic Acids Res. 30, 207–210. doi: 10.1093/nar/30.1.207
Emsley, P., Lohkamp, B., Scott, W. G., and Cowtan, K. (2010). Features and development of coot. Acta Crystallogr. D Biol. Crystallogr. 66, 486–501. doi: 10.1107/S0907444910007493
Evans, P. (2006). Scaling and assessment of data quality. Acta Crystallogr. Sect. D 62, 72–82. doi: 10.1107/S0907444905036693
Evans, P. R., and Murshudov, G. N. (2013). How good are my data and what is the resolution? Acta Crystallogr. Sect. D 69, 1204–1214. doi: 10.1107/S0907444913000061
Falony, G., Vieira-Silva, S., and Raes, J. (2015). Microbiology meets big data: the case of gut microbiota-derived trimethylamine. Ann. Rev. Microbiol. 69, 305–321. doi: 10.1146/annurev-micro-091014-104422
Hanai, T., Shiraki, M., Imai, K., Suetugu, A., Takai, K., and Shimizu, M. (2020). Usefulness of carnitine supplementation for the complications of liver cirrhosis. Nutrients 12:195. doi: 10.3390/nu12071915
Hendlich, M., Rippmann, F., and Barnickel, G. (1997). LIGSITE: automatic and efficient detection of potential small molecule-binding sites in proteins. J. Mol. Graph. Model. 15, 359–389. doi: 10.1016/s1093-3263(98)00002-3
Hossain, M. A., Nakano, Y., and Asada, K. (1984). Monodehydroascorbate reductase in spinach chloroplasts and its participation in regeneration of ascorbate for scavenging hydrogen peroxide. Plant Cell Physiol. 25, 385–395. doi: 10.1093/oxfordjournals.pcp.a076726
Hurley, J. H., Dean, A. M., Koshland, D. E. Jr., and Stroud, R. M. (1991). Catalytic mechanism of NADP(+)-dependent isocitrate dehydrogenase: implications from the structures of magnesium-isocitrate and NADP+ complexes. Biochemistry 30, 8671–8678. doi: 10.1021/bi00099a026
Jawad, A., Seifert, H., Snelling, A. M., Heritage, J., and Hawkey, P. M. (1998). Survival of Acinetobacter baumannii on dry surfaces: comparison of outbreak and sporadic isolates. J. Clin. Microbiol. 36, 1938–1941. doi: 10.1128/JCM.36.7.1938-1941.1998
Jebbar, M., Champion, C., Blanco, C., and Bonnassie, S. (1998). Carnitine acts as a compatible solute in Brevibacterium linens. Res. Microbiol. 149, 211–219. doi: 10.1016/s0923-2508(98)80081-8
Jumper, J., Evans, R., Pritzel, A., Green, T., Figurnov, M., Ronneberger, O., et al. (2021). Highly accurate protein structure prediction with AlphaFold. Nature 596, 583–589. doi: 10.1038/s41586-021-03819-2
Jung, K., Jung, H., and Kleber, H. P. (1987). Regulation of L-carnitine metabolism in Escherichia coli. J. Basic Microbiol. 27, 131–137. doi: 10.1002/jobm.3620270303
Kabsch, W. (2010). XDS. Acta Crystallogr. D Biol. Crystallogr. 66, 125–132. doi: 10.1107/s0907444909047337
Kalnins, G., Sevostjanovs, E., Hartmane, D., Grinberga, S., and Tars, K. (2018). CntA oxygenase substrate profile comparison and oxygen dependency of TMA production in Providencia rettgeri. J. Basic Microbiol. 58, 52–59. doi: 10.1002/jobm.201700428
Kanitsoraphan, C., Rattanawong, P., Charoensri, S., and Senthong, V. (2018). Trimethylamine N-oxide and risk of cardiovascular disease and mortality. Curr. Nutr. Rep. 7, 207–213. doi: 10.1007/s13668-018-0252-z
Kets, E. P. W., Galinski, E. A., and de Bont, J. A. M. (1994). Carnitine: a novel compatible solute in Lactobacillus plantarum. Arch. Microbiol. 162, 243–248. doi: 10.1007/BF00301845
Kim, Y. G., Lee, S., Kwon, O. S., Park, S. Y., Lee, S. J., Park, B. J., et al. (2009). Redox-switch modulation of human SSADH by dynamic catalytic loop. EMBO J. 28, 959–968. doi: 10.1038/emboj.2009.40
Langendorf, C. G., Key, T. L., Fenalti, G., Kan, W. T., Buckle, A. M., Caradoc-Davies, T., et al. (2010). The X-ray crystal structure of Escherichia coli succinic semialdehyde dehydrogenase; structural insights into NADP+/enzyme interactions. PLoS One 5:e9280. doi: 10.1371/journal.pone.0009280
Longo, N., Frigeni, M., and Pasquali, M. (2016). Carnitine transport and fatty acid oxidation. Biochim. Biophys. Acta 1863, 2422–2435. doi: 10.1016/j.bbamcr.2016.01.023
Massmig, M., Reijerse, E., Krausze, J., Laurich, C., Lubitz, W., Jahn, D., et al. (2020). Carnitine metabolism in the human gut: characterization of the two-component carnitine monooxygenase CntAB from Acinetobacter baumannii. J. Biol. Chem. 295, 13065–13078. doi: 10.1074/jbc.RA120.014266
McCoy, A. J., Grosse-Kunstleve, R. W., Adams, P. D., Winn, M. D., Storoni, L. C., and Read, R. J. (2007). Phaser crystallographic software. J. Appl. Crystallogr. 40, 658–674. doi: 10.1107/S0021889807021206
Meadows, J. A., and Wargo, M. J. (2015). Carnitine in bacterial physiology and metabolism. Microbiology 161, 1161–1174. doi: 10.1099/mic.0.000080
Miyazaki, K. (2005a). Bifunctional isocitrate-homoisocitrate dehydrogenase: a missing link in the evolution of beta-decarboxylating dehydrogenase. Biochem. Biophys. Res. Commun. 331, 341–346. doi: 10.1016/j.bbrc.2005.03.169
Miyazaki, K. (2005b). Identification of a novel trifunctional homoisocitrate dehydrogenase and modulation of the broad substrate specificity through site-directed mutagenesis. Biochem. Biophys. Res. Commun. 336, 596–602. doi: 10.1016/j.bbrc.2005.08.139
Neidhardt, F. C., Bloch, P. L., and Smith, D. F. (1974). Culture medium for enterobacteria. J. Bacteriol. 119, 736–747. doi: 10.1128/jb.119.3.736-747.1974
Parra-Millan, R., Guerrero-Gomez, D., Ayerbe-Algaba, R., Pachon-Ibanez, M. E., Miranda-Vizuete, A., Pachon, J., et al. (2018). Intracellular trafficking and persistence of Acinetobacter baumannii requires transcription factor EB. mSphere 3:106. doi: 10.1128/mSphere.00106-18
Piskol, F., Neubauer, K., Eggers, M., Bode, L. M., Jasper, J., Slusarenko, A., et al. (2022). Two-component carnitine monooxygenase from Escherichia coli: functional characterization, inhibition and mutagenesis of the molecular interface. Biosci. Rep. 42:102. doi: 10.1042/BSR20221102
Quareshy, M., Shanmugam, M., Townsend, E., Jameson, E., Bugg, T. D. H., Cameron, A. D., et al. (2020). Structural basis of carnitine monooxygenase CntA substrate specificity, inhibition and inter-subunit electron transfer. J. Biol. Chem. 296:100038. doi: 10.1074/jbc.RA120.016019
Rath, S., Heidrich, B., Pieper, D. H., and Vital, M. (2017). Uncovering the trimethylamine-producing bacteria of the human gut microbiota. Microbiome 5:54. doi: 10.1186/s40168-017-0271-9
Rath, S., Rud, T., Karch, A., Pieper, D. H., and Vital, M. (2018). Pathogenic functions of host microbiota. Microbiome 6:174. doi: 10.1186/s40168-018-0542-0, Pathogenic functions of host microbiota
Rath, S., Rud, T., Pieper, D. H., and Vital, M. (2019). Potential TMA-producing Bacteria are ubiquitously found in Mammalia. Front. Microbiol. 10:2966. doi: 10.3389/fmicb.2019.02966
Seim, H., Loster, H., Claus, R., Kleber, H. P., and Strack, E. (1982). Stimulation of the anaerobic growth of Salmonella typhimurium by reduction of L-carnitine, carnitine derivatives and structure-related trimethylammonium compounds. Arch. Microbiol. 132, 91–95. doi: 10.1007/BF00690825
Shortall, K., Djeghader, A., Magner, E., and Soulimane, T. (2021). Insights into aldehyde dehydrogenase enzymes: a structural perspective. Front. Mol. Biosci. 8:659550. doi: 10.3389/fmolb.2021.659550
Soares, N. C., Cabral, M. P., Parreira, J. R., Gayoso, C., Barba, M. J., and Bou, G. (2009). 2-DE analysis indicates that Acinetobacter baumannii displays a robust and versatile metabolism. Proteome Sci. 7:37. doi: 10.1186/1477-5956-7-37
Soltermann, F., Foley, E. D. B., Pagnoni, V., Galpin, M., Benesch, J. L. P., Kukura, P., et al. (2020). Quantifying protein-protein interactions by molecular counting with mass photometry. Angew. Chem. Int. Ed. Engl. 59, 10774–10779. doi: 10.1002/anie.202001578
Steinkellner, G., Rader, R., Thallinger, G. G., Kratky, C., and Gruber, K. (2009). VASCo: computation and visualization of annotated protein surface contacts. BMC Bioinformatics 10:32. doi: 10.1186/1471-2105-10-32
Verheul, A., Wouters, J. A., Rombouts, F. M., and Abee, T. (1998). A possible role of ProP, ProU and CaiT in osmoprotection of Escherichia coli by carnitine. J. Appl. Microbiol. 85, 1036–1046. doi: 10.1111/j.1365-2672.1998.tb05269.x
Villegas, M. V., and Hartstein, A. I. (2003). Acinetobacter outbreaks, 1977-2000. Infect. Control Hosp. Epidemiol. 24, 284–295. doi: 10.1086/502205
Vonrhein, C., Flensburg, C., Keller, P., Sharff, A., Smart, O., Paciorek, W., et al. (2011). Data processing and analysis with the autoPROC toolbox. Acta Crystallogr. D Biol. Crystallogr. 67, 293–302. doi: 10.1107/s0907444911007773
Vorobieva, A. A., Khan, M. S., and Soumillion, P. (2014). Escherichia coli D-malate dehydrogenase, a generalist enzyme active in the leucine biosynthesis pathway. J. Biol. Chem. 289, 29086–29096. doi: 10.1074/jbc.M114.595363
Walt, A., and Kahn, M. L. (2002). The fixA and fixB genes are necessary for anaerobic carnitine reduction in Escherichia coli. J. Bacteriol. 184, 4044–4047. doi: 10.1128/jb.184.14.4044-4047.2002
Wu, W.-K., Chen, C.-C., Liu, P.-Y., Panyod, S., Liao, B.-Y., Chen, P.-C., et al. (2019). Identification of TMAO-producer phenotype and host–diet–gut dysbiosis by carnitine challenge test in human and germ-free mice. Gut 68, 1439–1449. doi: 10.1136/gutjnl-2018-317155
Yuan, Z., Yin, B., Wei, D., and Yuan, Y.-R. A. (2013). Structural basis for cofactor and substrate selection by cyanobacterium succinic semialdehyde dehydrogenase. J. Struct. Biol. 182, 125–135. doi: 10.1016/j.jsb.2013.03.001
Zeidler, S., and Muller, V. (2019). Coping with low water activities and osmotic stress in Acinetobacter baumannii: significance, current status and perspectives. Environ. Microbiol. 21, 2212–2230. doi: 10.1111/1462-2920.14565
Zhu, Y., Jameson, E., Crosatti, M., Schafer, H., Rajakumar, K., Bugg, T. D., et al. (2014). Carnitine metabolism to trimethylamine by an unusual Rieske-type oxygenase from human microbiota. Proc. Natl. Acad. Sci. USA 111, 4268–4273. doi: 10.1073/pnas.1316569111
Keywords: Acinetobacter baumannii, carnitine, carnitine monooxygenase, trimethylamine, malic semialdehyde dehydrogenase, D-malate dehydrogenase, X-ray crystal structure
Citation: Piskol F, Lukat P, Kaufhold L, Heger A, Blankenfeldt W, Jahn D and Moser J (2024) Biochemical and structural elucidation of the L-carnitine degradation pathway of the human pathogen Acinetobacter baumannii. Front. Microbiol. 15:1446595. doi: 10.3389/fmicb.2024.1446595
Edited by:
Daniela De Biase, Sapienza University of Rome, ItalyReviewed by:
Wolfgang Buckel, University of Marburg, GermanyMalte Sinn, University of Konstanz, Germany
Copyright © 2024 Piskol, Lukat, Kaufhold, Heger, Blankenfeldt, Jahn and Moser. This is an open-access article distributed under the terms of the Creative Commons Attribution License (CC BY). The use, distribution or reproduction in other forums is permitted, provided the original author(s) and the copyright owner(s) are credited and that the original publication in this journal is cited, in accordance with accepted academic practice. No use, distribution or reproduction is permitted which does not comply with these terms.
*Correspondence: Jürgen Moser, j.moser@tu-bs.de