- 1Molecular Enzyme Technology and Biochemistry (MEB), Environmental Microbiology and Biotechnology (EMB), Centre for Water and Environmental Research (CWE), Faculty of Chemistry, University of Duisburg-Essen, Essen, Germany
- 2Microbial Genomics and Biotechnology, Center for Biotechnology (CeBiTec), Bielefeld University, Bielefeld, Germany
Sulfolobus acidocaldarius, a thermoacidophilic archaeon of the phylum Thermoproteota (former Crenarchaeota), is a widely used model organism for gene deletion studies and recombinant protein production. Previous research has demonstrated the efficacy of the saci_2122 promoter (Para), providing low basal activity and high pentose-dependent induction. However, the available expression vector does not include a 5′-terminal untranslated region (5’-UTR), a typical element found in bacterial expression vectors that usually enhances protein production in bacteria. To establish S. acidocaldarius as a production strain in biotechnology in the long term, it is intrinsically relevant to optimize its tools and capacities to increase production efficiencies. Here we show that protein production is increased by the integration of S. acidocaldarius 5’-UTRs into Para expression plasmids. Using the esterase Saci_1116 as a reporter protein, we observed a four-fold increase in soluble and active protein yield upon insertion of the saci_1322 (alba) 5’-UTR. Screening of four additional 5’-UTRs from other highly abundant proteins (thα, slaA, slaB, saci_0330) revealed a consistent enhancement in target protein production. Additionally, site-directed mutagenesis of the Shine-Dalgarno (SD) motif within the alba 5’-UTR revealed its significance for protein synthesis. Ultimately, the alba 5’-UTR optimized expression vector improved the expression of various proteins, including six glycosyltransferases and one hydroxyacyl-CoA dehydratase from S. acidocaldarius, and a malto-oligosyltrehalose trehalohydrolase from Saccharolobus solfataricus, demonstrating its applicability. Our results show that the integration of SD-motif containing 5’-UTRs significantly enhanced plasmid-based protein production in S. acidocaldarius. This advancement in recombinant expression not only broadens the utility of S. acidocaldarius as an archaeal expression platform but also marks an important step toward potential biotechnological applications.
1 Introduction
The efficient production of properly folded, functional proteins of interest (POIs) is fundamental in both basic research and biotechnology. A range of microorganisms and gene expression vectors are available for protein production. Most commonly, yeast or bacteria are used for gene overexpression, with Escherichia coli standing out as the most commonly used bacterial expression host (Rosano and Ceccarelli, 2014). Despite continuous advancements in expression systems, challenges such as suboptimal codon usage and posttranslational modifications, protein misfolding, and the formation of insoluble aggregates persist, hindering successful gene overexpression (de Lise et al., 2023).
Selecting an appropriate expression host is crucial for successful POI production. Besides eukaryotic or bacterial strains, Archaea offer high potential as protein production platforms. Archaea are characterized by a mosaic nature, sharing cellular features with both Bacteria and Eukaryotes, while additionally exhibiting unique archaeal properties. As Bacteria, Archaea are unicellular, lack organelles, and possess similar DNA structures (e.g., operon structures, one circular chromosome, and plasmids). However, archaeal information processing (e.g., transcription and translation) and posttranslational modifications resemble simplified versions of eukaryotic processes (Lewis et al., 2021; Schocke et al., 2019; Esser et al., 2016; Jarrell et al., 2014; Eichler and Adams, 2005). Some archaeal proteins can be efficiently produced in E. coli. In particular, hyperthermophilic proteins can be easily purified by heat precipitation, which removes unwanted heat-unstable proteins of E. coli by denaturation, allowing their separation from the heat-stable, soluble target protein. However, the production of thermophilic and/or archaeal proteins is also often challenging using classical bacterial expression hosts (e.g., Martínez-Espinosa, 2019; Straub et al., 2018). In these cases, proper expression or protein folding and stability may depend on special cofactors, posttranslational modifications, or suitable expression conditions, such as temperature (Eichler and Adams, 2005; Martínez-Espinosa, 2019; Straub et al., 2018). Thus, expression in the native or a closely related organism was suggested as alternative for efficient protein synthesis (Straub et al., 2018).
With its established genetic system and genomic stability, the thermoacidophilic, aerobic archaeon Sulfolobus acidocaldarius (Topt 70–75°C, pHopt 2–3; Brock et al., 1972; Chen et al., 2005) offers a versatile platform for gene deletion studies and overexpression (de Lise et al., 2023; Wagner et al., 2012; Lewis et al., 2021). Inducible expression vectors utilizing sugar-inducible promoters (e.g., Para from saci_2122, Pxyl from saci_1938 (both pentose inducible by L-/D-arabinose and D-xylose) or Pmal (maltose) from saci_1165) have been developed, featuring various N-or C-terminal protein tags (see van der Kolk et al., 2020 for an overview) for successful protein expression (e.g., Stracke et al., 2020; Zweerink et al., 2017). In a comparative promoter screening, Para was identified as the most efficient promoter, exhibiting the highest pentose-dependent induction with D-xylose and L-/D-arabinose and low basal activity (van der Kolk et al., 2020). While D-xylose and L-arabinose are metabolized by S. acidocaldarius, D-arabinose does not serve as a carbon source and can therefore act as an artificial inducer.
In general, mRNAs can have UTRs at their 5′ and 3′ ends. Both are known to be involved in important regulatory processes, including transcription and translation initiation, transcript stability, mRNA secondary structure formation, and posttranscriptional regulation, e.g., through the action of RNA binding proteins or small regulatory RNAs (Ao et al., 2013; Brenneis and Soppa, 2009; Gomes-Filho et al., 2018; Ren et al., 2017). Notably, under the control of the native Para sequence, S. acidocaldarius generates leaderless gene transcripts, lacking a 5′-untranslated region (UTR) (Cohen et al., 2016). Leaderless mRNAs may either directly start with the initiation codon or possess up to five (Babski et al., 2016) or ten (Brenneis et al., 2007; Chen et al., 2015) 5′-terminal nucleotides upstream of the translation start codon (Figure 1). In contrast, leadered mRNAs carry a 5′- UTR, i.e., a non-coding regulatory sequence at the 5′-end of the transcript. 5’-UTRs may contain a Shine-Dalgarno (SD) sequence motif (Hering et al., 2009; Srivastava et al., 2016; Wurtzel et al., 2010). If present, the SD sequence [core sequence: GGAGGU (Shine and Dalgarno, 1975)] enables base pairing with the anti-Shine-Dalgarno sequence (aSD) at the 3′-end of the 16S rRNA of the small ribosomal subunit (Ma et al., 2002; Torarinsson et al., 2005), thereby supporting translation initiation. Based on the direct interaction of SD to aSD, the SD is referred to as ribosome binding site (RBS). However, in the process of translation initiation, the ribosome covers an mRNA region of approximately 30 nucleotides (nts), referred to as the ribosome docking site (RDS). This area includes the SD sequence, the translation start codon, an intermediate spacer region (of variable length and nucleotide composition), and the first nucleotides of the coding sequence (Reeve et al., 2014; Chen et al., 1994) (Figure 1).
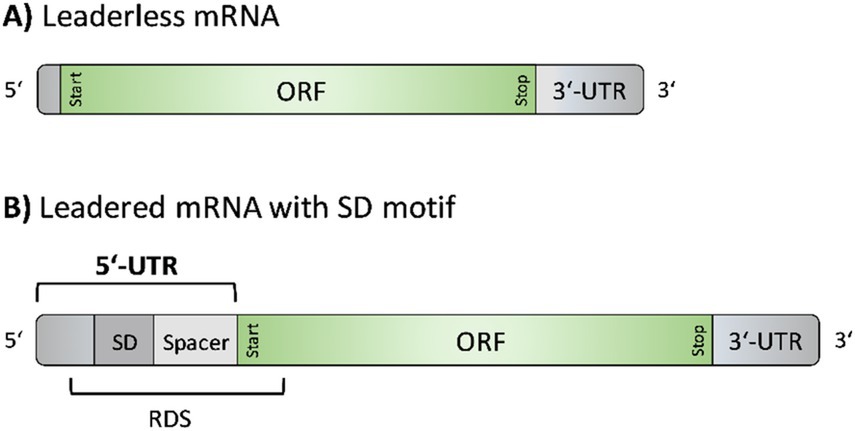
Figure 1. Structural elements of leaderless mRNAs and leadered mRNAs with a Shine-Dalgarno motif. (A) Leaderless mRNAs consist of an open reading frame (ORF), defined by a start and stop codon, followed by a 3′ untranslated region (UTR). Up to 10 nucleotides may optionally precede the ORF at the 5′ end (Brenneis et al., 2007). (B) Leadered mRNAs contain a 5’-UTR longer than 10 nucleotides, which may include a Shine-Dalgarno (SD) sequence. The region between the SD sequence and the start codon is known as spacer region. The ribosome docking site (RDS) includes the SD sequence, spacer and the first nucleotides of the coding region (Reeve et al., 2014; Chen et al., 1994).
The use of a 5’-UTR, including a preserved SD sequence, is common for many bacterial expression vectors, and in Bacteria the insertion, enlargement, or modification of the 5’-UTR (RBS) has been shown to increase plasmid-encoded protein production significantly (e.g., Liebeton et al., 2014; Vellanoweth and Rabinowitz, 1992; Volkenborn et al., 2020). In contrast, their utilization in archaeal expression plasmids remains less explored (e.g., Brenneis et al., 2007; Akinyemi et al., 2021). Notably, Wurtzel et al. (2010) reported that 5’-UTRs are overrepresented in Sulfolobales genes involved in translation, which are generally highly expressed. Thus, in this study, we systematically analyze the impact of 5’-UTR insertions of highly expressed genes into S. acidocaldarius expression plasmids on protein production, primarily utilizing esterase Saci_1116 and β-galactosidase LacS from Saccharolobus solfataricus (formerly Sulfolobus solfataricus) as reporters. Additionally, we employ the 5’-UTR-optimized expression vectors for the production of diverse proteins of different enzyme classes, including S. acidocaldarius glycosyltransferases as well as a hydroxyacyl-CoA dehydratase and S. solfataricus malto-oligosyltrehalose trehalohydrolase. Furthermore, we provide initial findings on the relevance of spacer nucleotides (located directly upstream of the translation start codon) and the SD sequence motif.
2 Materials and methods
2.1 Strains and growth conditions
The uracil auxotrophic mutant S. acidocaldarius MW001 (Wagner et al., 2012) was used as the expression host. The strain originates from S. acidocaldarius DSM639, but lacks 322 bp of pyrE (saci_1597), enabling genetic selection. Cultures were grown aerobically in Brock medium (Brock et al., 1972) at pH 3.0, 140 rpm, and 76°C (New Brunswick Innova 44 incubator, Eppendorf, Germany). Cell growth was monitored by turbidity measurements at 600 nm. S. acidocaldarius MW001 cultures were supplemented with 10 μg/mL uracil (min. 99%, Merck, Germany). Transformed clones of S. acidocaldarius MW001, carrying an expression plasmid with pyrEF from Saccharolobus solfataricus, were grown without the addition of uracil.
Escherichia coli DH5α (DSMZ 6897) was used for cloning and plasmid amplification. E. coli ER1821 (New England Biolabs, USA), harbouring pM.EsaBC4I, which encodes a DNA methylase and kanamycin resistance gene, was applied for the methylation of S. acidocaldarius expression plasmids. The strains were grown in Lysogeny broth (LB) medium (Carl Roth, Germany), supplemented with respective antibiotics, at 37°C (and 180 rpm for liquid cultures).
2.2 Plasmid construction
pBSaraFX-xxxUTR-Nt/Ct-SS plasmids (with xxx for the different UTRs tested) are originally based on pSVAaraFX-Nt/Ct-SS expression vectors (van der Kolk et al., 2020) (Supplementary Table S1). They possess the vector backbone of Sulfolobus islandicus plasmid pRN1 (Lipps, 2004) and contain basal shuttle vector elements such as origins of replication for S. acidocaldarius and E. coli, as well as pyrEF from S. solfataricus and an ampicillin resistance gene (β-lactamase) for selection in S. acidocaldarius or E. coli, respectively. The expression cassette for a gene of interest is flanked by different restriction sites and is composed of the pentose-inducible promoter of saci_2122 (Para) and lacI, lacZ as a reporter system enabling blue/white screening in E. coli. The vector encodes a Twin-Strep tag, enabling N-or C-terminal protein tagging (pSVAaraFX-NtSS or-CtSS; van der Kolk et al., 2020). “FX” indicates that the FX cloning strategy can be applied (class IIS restriction enzyme SapI; Geertsma and Dutzler, 2011).
2.2.1 Integration of different 5’-UTR sequences into saci_1116 expression plasmid
In this study, the pSVAaraFX-saci_1116-CtSS expression plasmid was expanded by the insertion of different 5′-untranslated region sequences that were integrated directly upstream of the translation start codon of the reporter gene saci_1116 (Supplementary Figures S1, S2). The insertion of a 5’-UTR was achieved using standard cloning procedures: modified promoter-5’-UTR fragments were PCR amplified with a fwd-primer (UTR-fwd, Supplementary Table S2), that bound to the vector backbone upstream of the saci_2122 promoter, in combination with a rev-primer containing the desired 5’-UTR sequence and a NcoI restriction site that bound upstream of the translation start codon (Supplementary Table S2). pSVAaraFX-CtSS served as PCR template. The resulting PCR product consisted of a fragment of the vector backbone, carrying a SacII restriction site, Para, and the newly integrated 5’-UTR sequence with a NcoI restriction site. The fragment was cloned into pSVAaraFX-saci_1116-CtSS by restriction with SacII and NcoI (NEB) followed by ligation (T4 DNA ligase, NEB). The resulting saci_1116 expression plasmid, containing a 5’-UTR, was named pBSaraFX-xxxUTR-saci_1116-CtSS. Successful cloning was confirmed by sequencing (LGC genomics, Germany).
2.2.2 Generation of lacS expression constructs with different 5’-UTR sequences
The series of expression plasmids for saci_1116, each containing distinct 5’-UTR sequences, served as the basis for the development of corresponding lacS expression plasmids. Amplification of lacS from S. solfataricus (SSO3019) was conducted using primers lacS-SSO-NcoI-fwd and lacS-SSO-XhoI-rev (Supplementary Table S2). Subsequently, the resulting PCR product and the pBSaraFX-xxxUTR-saci_1116-CtSS expression plasmids (Supplementary Table S1) underwent restriction enzyme digestion with NcoI and XhoI. This process facilitated the excision of saci_1116 from the xxxUTR-plasmid backbone, excluding it from subsequent cloning steps through agarose gel extraction of the plasmid fragment. The NcoI-XhoI digested pBSaraFX-xxxUTR-CtSS fragment and lacS were ligated. Verification of the resultant plasmid set, designated as pBSaraFX-xxxUTR-lacS-CtSS, was conducted through sequencing. Subsequently, this plasmid set was utilized to investigate LacS production in S. acidocaldarius.
2.2.3 Site-directed mutagenesis of alba 5’-UTR
To assess the impact of -2 and -1 nucleotides, located directly upstream of the translation start codon within the alba 5’-UTR, on gene expression, we employed site-directed mutagenesis. Plasmid pBSaraFX-albaUTR-saci_1116-CtSS served as the template for modifications (Supplementary Table S1). Each primer contained the desired mutation and was complementary to the other (Supplementary Table S2). To prevent the formation of primer homodimers in the reaction mixture, DNA amplification was performed in single-primer reactions, following the protocol outlined by Edelheit et al., 2009. Q5® High-Fidelity DNA Polymerase and its recommended reaction buffer (NEB) were utilized for the amplification process.
Correspondingly, the SD sequence motif within the alba 5’-UTR was modified by site-directed mutagenesis, as described by Edelheit et al. (2009) (Supplementary Table S2) using pBSaraFX-albaUTR-saci_1116-CtSS as template for the “noSD” and “8SDmut” variants of alba 5’-UTR (Supplementary Tables S1, S2). The “8SDins” variant, designated as pBSaraFX-albaUTR-8SDins-saci_1116-CtSS, was constructed via restriction and ligation-dependent cloning. Initially, PCR was conducted using primers SD-fwd and 8SD-ins-rev with pSVAaraFX-CtSS serving as the template (Supplementary Tables S1, S2). Subsequently, the resulting PCR product and the vector pSVAaraFX-saci_1116-CtSS underwent restriction using SacII and NcoI, followed by ligation.
2.2.4 Generation of cloning vectors pBSaraFX-albaUTR-CtSS and -NtSS
Generally, S. acidocaldarius expression vectors contain lacI and lacZ in the expression cassette for blue/white screening in E. coli. To enable this feature to be used in the cloning process of alba 5’-UTR containing plasmids, two alba 5’-UTR cloning vectors with inserted lacI, lacZ, and an N-or C-terminal Twin-Strep tag were generated. pBSaraFX-albaUTR-NtSS (N-terminal Twin-Strep tag) was generated by the insertion of the original alba (saci_1322) 5’-UTR (5′ GATAGGTGGTTTAA 3′) directly upstream of the gene expression cassette start codon into pSVAaraFX-NtSS by overlap PCR using the primer pair saci_1322-UTR-NtSS-fwd and -rev (Supplementary Table S2). To obtain the cloning vector pBSaraFX-albaUTR-CtSS (C-terminal Twin-Strep tag), the saci_1116 expression plasmid pBSaraFX-albaUTR-saci_1116-CtSS as well as the vector pSVAaraFX-CtSS, containing lacI and lacZ, were restricted with NcoI and XhoI. The obtained vector backbone and the lacI-lacZ fragment were ligated, resulting in cloning vector pBSaraFX-albaUTR-CtSS (containing the modified alba 5’-UTR: 5’ GATAGGTGGTTTCC 3′).
2.2.5 Cloning of gene expression plasmids for different target proteins
Genes of interest, namely saci_1904, saci_1909, saci_1911, saci_1915, saci_1916, saci_1921, saci_2033, and saci_1085 from S. acidocaldarius and SSO3019 (lacS) and SSO2093 (treZ) from S. solfataricus were cloned into pSVAaraFX-NtSS and/or-CtSS as well as pBSaraFX-albaUTR-NtSS and/or-CtSS expression vectors (Supplementary Table S1). All cloning primers and the used restriction sites are included in Supplementary Table S2.
2.3 Transformation of S. acidocaldarius MW001
Competent cells were prepared according to Wagner et al. (2012), and transformed with 300–500 ng of methylated plasmid DNA using a Gene Pulser Xcell (Bio-Rad, Germany) at 2,000 V, 600 Ω and 25 μF in 1 mm cuvettes. Cell recovery was performed in Brock medium (pH 5.0), supplemented with 0.1% (w/v) N-Z-amine (casein enzymatic hydrolysate N-Z-Amine® AS, Merck, Germany), for up to 4 hours at 76°C in a heat block (speed of 300 rpm; ThermoMixer F1.5, Eppendorf, Germany) (Wagner et al., 2014). Cells were plated on Gelrite-Brock plates with 0.6% (w/v) Gelrite® (Gellan Gum: K9A-40, Serva Electrophoresis, Heidelberg, Germany), 0.1% (w/v) N-Z-amine and 0.2% (w/v) dextrin (pure, Carl Roth, Germany), lacking uracil. Plates were incubated in a closed metal box for 5–6 d at 76°C.
2.4 S. acidocaldarius MW001 expression cultures
After transformation of S. acidocaldarius MW001 and incubation of cells on Gelrite-Brock plates, clones were transferred to liquid Brock medium (0.1% (w/v) N-Z-amine (Merck, Germany) and 0.2% (w/v) dextrin (Carl Roth, Germany), pH 3.0), and cultures were grown to the late log growth phase (OD600nm 0.9–1.2) at 140 rpm and 76°C. The presence of the expression plasmid was verified by colony PCR using gene-specific primer pairs (Supplementary Table S2). Cell cultures, carrying an expression plasmid with SSOpyrEF, were used to inoculate pre-cultures for S. acidocaldarius expression. Pre-cultures were cultivated in Brock medium supplemented with 0.1% (w/v) N-Z-amine (Merck, Germany) and 0.2% (w/v) dextrin (Carl Roth, Germany) without uracil until the log growth phase. Expression cultures were inoculated to an OD600nm of 0.05–0.1, and 0.1% (w/v) N-Z-amine as well as 0.3% (w/v) D-xylose (min. 99%, Carl Roth, Germany) were added to the medium. Expression cultures were grown at 140 rpm and 76°C (New Brunswick Innova 44 incubator, Eppendorf, Germany). Cells were harvested by centrifugation (7,000 xg, 4°C, 20 min) at OD600nm values of 0.6 or 0.9–1.2, as stated in the results section. Cell pellets were stored at −80°C until further use.
2.5 Sodium dodecyl sulfate-polyacrylamide gel electrophoresis (SDS-PAGE) and immunoblotting of S. acidocaldarius cell lysate samples
For the comparison of protein expression in presence and absence of a certain 5’-UTR sequence, S. acidocaldarius cell samples were adjusted to a theoretical OD600nm of 10 by resuspension of cells in appropriate volumes of 50 mM TRIS/HCl pH 7.5 with 0.5% (v/v) Triton X-100 (Gerbu, Germany). Samples of protein purification were applied to SDS-PAGE. 5x SDS-PAGE loading dye (125 mM TRIS/HCl pH 6.8, 4% (w/v) SDS, 20% (v/v) glycerol, 10% (v/v) β-mercaptoethanol, 0.01% (w/v) Bromophenol Blue) was added and samples were heated for 5 min at 99°C. 5 μL of PageRuler Unstained or PageRuler (Plus) Prestained Protein Ladder (Thermo Fisher Scientific, USA) and 15 or 20 μL of each cell lysate sample (approximately 30 μg of protein) were applied to SDS-PAGE. Gels were either stained by Coomassie Brilliant Blue staining (0.05% (w/v) Coomassie Brilliant Blue G-250 (Merck, Germany), 40% (v/v) ethanol, 10% (v/v) acetic acid) and imaged using the Molecular Imager Gel Doc XR System and the Quantity One Software Package (Bio-Rad, Germany), or they were used for immunodetection. Proteins were transferred on a PVDF membrane (Roti-PVDF, pore size 0.45 μm, Carl Roth, Germany) using blotting buffer (50 mM TRIS, 40 mM glycine, 0.1% (w/v) SDS, 20% (v/v) EtOH (p.a.)) and the Bio-Rad trans-blot® TurboTM Trans System (25 V, 0.5–1 A, 25 min; Bio-Rad, Germany). Afterwards, the membrane was blocked with 0.2% (w/v) Tropix® I-BLOCK (Applied Biosystems, Thermo Fisher Scientific, USA) in TBS-T buffer (20 mM TRIS–HCl pH 7.5, 500 mM NaCl, 0.05% (v/v) Tween 20) at 4°C overnight. The next day, the membranes were floated in a solution of 1:50,000 Strep-Tactin HRP conjugate (IBA Lifesciences, Germany) with 0.1% (w/v) I-BLOCK in TBS-T buffer and incubated while shaking at RT for 1–2 h. The membrane was washed twice using 0.1% (w/v) I-BLOCK in TBS-T buffer for 10 min each, followed by two washing steps using TBS-T and TBS buffer, respectively. Clarity Western ECL Substrate (Bio-Rad, Germany) and the VersaDoc MP-4000 imaging system (Bio-Rad, Germany) were used for immunodetection and documentation.
2.6 Bicinchoninic acid (BCA) protein assay of S. acidocaldarius cell lysates
S. acidocaldarius cell suspensions were adjusted to a theoretical OD600nm of 5 by resuspension of cells in 50 mM TRIS/HCl pH 7.5 with 0.5% (v/v) Triton X-100 (Gerbu, Germany). Cells were lysed by sonication [ultrasonic processor UP 200 s (Hielscher Ultrasonics, Germany)] with a cycle of 0.5 s−1 and an amplitude of 50% for 1 min on ice. Protein concentrations in cell lysate samples were determined using the bicinchoninic acid (BCA) assay (Uptima BC assay protein quantification kit, France). Cell lysates (25 μL) and bovine serum albumin (BSA) protein standards (0–1 mg/mL BSA (Merck, Germany) in resuspension buffer) were transferred in the wells of a 96-well microtiter plate (MTP; flat bottom, polystyrene, Sarstedt, Germany) and mixed with 200 μL BCA solution each. For each biological replicate three technical replicates were analyzed. After 3 h of incubation at room temperature absorption at 562 nm was determined in a Tecan plate reader (Tecan Infinite® M200, NanoQuant PlateTM; Tecan, Switzerland).
2.7 Esterase activity assay (Saci_1116)
To determine the esterase activity of Saci_1116 in cell lysates of S. acidocaldarius cultures, para-nitrophenyl acetate (pNPA, Merck, Germany) was used as a substrate (Sobek and Görisch, 1988, 1989). 175 μL of 50 mM TRIS/HCl (pH 7.5) and 5 μL of 10-fold diluted S. acidocaldarius cell lysate sample (diluted with reaction buffer) were pipetted into the wells of a 96-well MTP (flat bottom, polystyrene, Sarstedt, Germany) and pre-warmed in the Tecan plate reader at 42°C for 10 min (Tecan Infinite® M200, NanoQuant PlateTM; Tecan, Switzerland). Afterward, 20 μL of 10 mM pNPA (dissolved in acetonitrile) were added to the wells by automatic dispersion, yielding a substrate concentration of 1 mM pNPA in the assay. Measurements were performed in 60 s cycles at 410 nm for 1 h, following the formation of pNP (4-nitrophenol). pNP (Merck, Germany) calibration curves were conducted under the same conditions. Experiments were performed in three biological replicates and for each biological replicate three technical replicates were analysed. The specific activity for biological replicates was calculated and displayed as data points in the bar charts. Enzyme activity measurements of purified esterase Saci_1116-CtSS were performed under the same assay conditions, using 40 and 20 ng of pure protein. The initial reaction velocities were used for the calculation of activities. pNP production was calculated using the established pNP calibration curve. Specific enzyme activities (U/mg of protein) were determined by the protein concentration obtained in the BCA assay.
2.8 β-galactosidase activity assay (LacS)
Cells of S. acidocaldarius expression cultures were resuspended in 50 mM sodium phosphate buffer (pH 6.5) supplemented with 50 mM NaCl and disrupted using prefilled 0.1 mm Glass Bead tubes and the Precellys 24 tissue homogenizer (Bertin technologies, France). The cell lysis process involved three cycles of homogenization at 6.500 xg for 20 s each, with intermittent cooling on ice for 1 min between cycles. Subsequently, cell lysates were centrifuged at 21,428 xg at 4°C for 40 min to obtain the supernatant, the crude extract, which was next utilized for protein concentration determination. Protein concentration was assessed using the Bradford protein assay (QuickStart™, Bio-Rad, Germany) with BSA (Sigma-Aldrich, USA) as protein standard (Zor and Selinger, 1996). LacS activity in crude extracts was determined using a continuous assay in the BioTek Synergy H1 microplate reader with 96-well microtiter plates (flat bottom, polystyrene, Thermo Fisher Scientific, USA). The wells of a 96-well MTP were filled with 177.5 μL of 50 mM sodium phosphate buffer (pH 6.5) and 2.5 μL of crude extract and pre-warmed in the reader at 70°C for 20 min. Then, 20 μL of 10 mM para-nitrophenyl β-D-galactopyranoside (dissolved in buffer) were automatically dispensed into the wells, resulting in a final substrate concentration of 1 mM pNPG in the assay. The plate was shaken for 5 s, and measurements were performed in 60 s cycles at 410 nm for 30 min to monitor the formation of pNP (4-nitrophenol). Calibration curves for pNP were prepared under the same conditions. For each biological replicate three technical replicates were analyzed. Finally, the initial linear range of pNP formation following the enzymatic reaction (8 min) was utilized to calculate specific enzyme activity.
2.9 Purification of Twin-Strep-tagged proteins from S. acidocaldarius crude extracts
Purification was performed according to the manufacturer’s instructions (IBA Lifesciences, Germany). Briefly, S. acidocaldarius cell suspensions were adjusted to a theoretical OD600nm of 5 by resuspension in buffer W (100 mM TRIS/HCl pH 8.0, 150 mM NaCl). Cells were disrupted by sonication (UP 200 s, Hielscher Ultrasonics, Germany) with a cycle of 0.5 s−1 and an amplitude of 60% three times for 5 min each on ice (cooling pauses of 1 min each) and cell lysates were centrifuged at 16,000 xg and 4°C for 40 min to remove cell debris. Supernatants were filtered (0.45 μm polyvinylidene fluoride membrane, Carl Roth, Germany) and Twin-Strep tagged proteins of interest were purified from crude extracts (soluble fraction) using the Strep-Tactin®XT Superflow® Kit (IBA Lifesciences, Germany). Purification was performed according to the manufacturer’s instructions, using buffer BXT (100 mM TRIS/HCl pH 8.0, 150 mM NaCl, 1 mM EDTA, 50 mM biotin) as elution buffer. Protein purity was analyzed by SDS-PAGE and the protein concentration was determined using the Bradford assay (QuickStart™, Bio-Rad, Germany) with BSA as the protein standard (Zor and Selinger, 1996).
3 Results
3.1 Influence of the alba 5’-UTR on the plasmid-encoded production of esterase Saci_1116
In earlier studies, the Para promoter (saci_2122) has demonstrated its effectiveness in enabling protein production in S. acidocaldarius, with pentose-dependent induction and low basal activity (van der Kolk et al., 2020). Notably, RNA sequencing data (Cohen et al., 2016; https://exploration.weizmann.ac.il/TCOL/) indicate that this promoter sequence leads to the generation of leaderless gene transcripts. This prompted our investigation into whether the incorporation of a 5’-UTR (RBS) could enhance plasmid-based protein production. To assess the impact of a 5’-UTR on protein yield, we utilized the 5’-UTR sequence of the Alba encoding gene saci_1322 (alba) from S. acidocaldarius. Alba (Acetylation Lowers Binding Affinity) (Laurens et al., 2012) is a well-conserved archaeal double-stranded DNA/RNA binding protein (Wardleworth et al., 2002; Cajili and Prieto, 2022) and it was already reported to be highly abundant in Sulfolobus [4.8% of cellular protein in Sulfolobus shibatae (Mai et al., 1998)]. In agreement, available full proteome data from S. acidocaldarius identified Alba as the most abundant cellular protein under different growth conditions (label-free quantification intensity, 0.2% (w/v) NZA and 0.2% (w/v) D-xylose; Schmerling et al., 2024a). The alba transcript comprises a 14-nucleotide long 5’-UTR (5’ GAUAGGUGGUUUAA 3′) (Cohen et al., 2016; Schmerling et al., 2024a). Based on previous literature on Sulfolobus SD consensus as well as S. acidocaldarius aSD sequence (e.g., Tolstrup et al., 2000; Schmitt et al., 2020), we identified a 5-nucleotide long SD sequence motif (bold), followed by a 6-nucleotide long spacer in alba 5’-UTR. To evaluate the impact of this 5’-UTR on protein production, we selected the esterase Saci_1116 as reporter protein. The enzyme was previously characterized (Sobek and Görisch, 1988, 1989), and was used as a model protein in the optimization of the S. acidocaldarius expression system before (van der Kolk et al., 2020). We used existing expression plasmids containing the Para promoter and encoding saci_1116, with either an N-or C-terminal Twin-Strep tag (NtSS or CtSS, respectively; Supplementary Table S1), as templates for inserting the alba 5’-UTR and as reference for expression analysis. The alba 5’-UTR was inserted upstream of the reporter gene start codon (“ATG”; Supplementary Figure S1), resulting in the generation of S. acidocaldarius expression plasmids termed pBSaraFX-albaUTR-saci_1116-NtSS or-CtSS, respectively (Supplementary Figures S1, S2; Supplementary Table S1).
Insertion of the alba 5’-UTR into the saci_1116-CtSS expression plasmid markedly enhanced reporter protein yield, as evidenced by SDS-PAGE and immunodetection analysis (Figure 2A). In line with previous observations (van der Kolk et al., 2020), the C-terminal tag yielded higher Saci_1116 production in contrast to the N-terminal protein tag. Using the C-terminal Twin-Strep tag, Saci_1116 was purified from the soluble protein fraction from the UTR expression culture (Figure 2B), and protein quantification revealed a four-fold increase in reporter protein yield using the alba 5’-UTR modified expression plasmid compared to the vector lacking the 5’-UTR (Supplementary Figure S3). The purified esterase Saci_1116-CtSS exhibited a specific activity of 124.5 U/mg using pNP-acetate as a substrate at 42°C. In total, the incorporation of the alba 5’-UTR into the saci_1116-CtSS expression plasmid significantly enhanced the production of soluble, active esterase reporter protein.
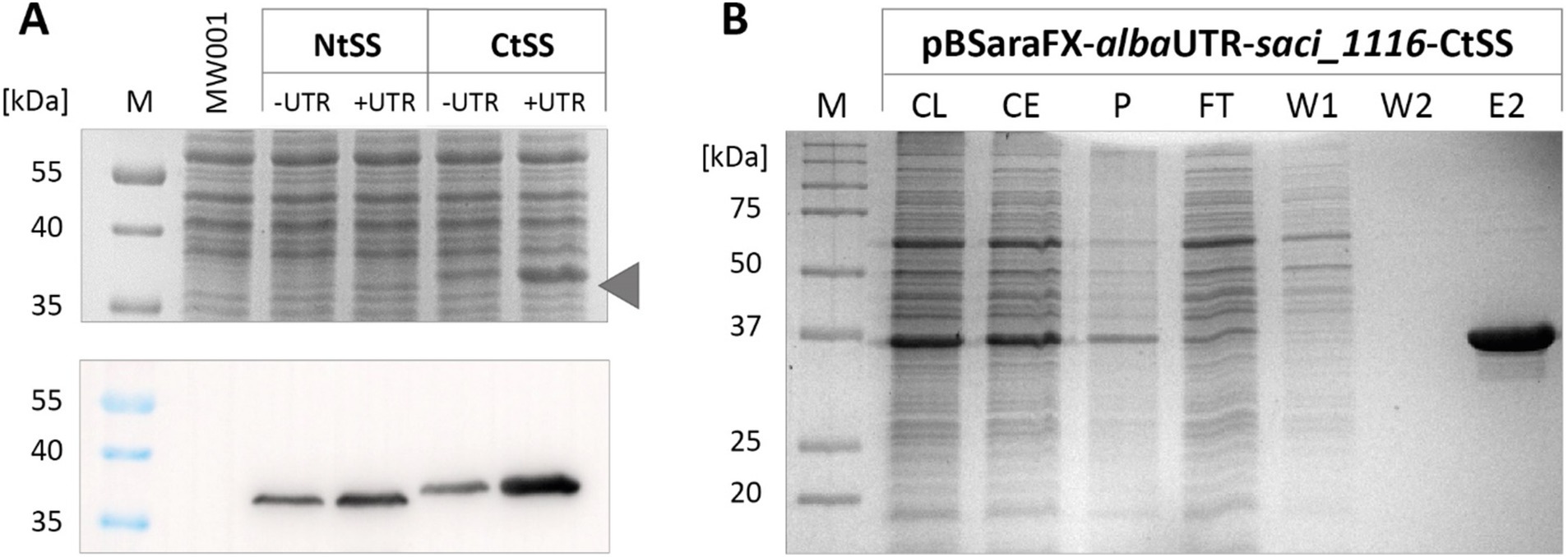
Figure 2. Effect of alba 5’-UTR on esterase Saci_1116 production in S. acidocaldarius. (A) Coomassie-stained SDS-PAGE and immunoblot of Twin-Strep-tagged Saci_1116 in cell lysates from S. acidocaldarius expressing saci_1116 without and with alba 5’-UTR, and with N-terminal (Nt) or C-terminal (Ct) Twin-Strep tag (SS). The arrow indicates the height of N-terminal tagged Saci_1116 bands in the Coomassie-stained SDS-PAGE. S. acidocaldarius MW001 without plasmid served as reference (MW001). For all cell samples, OD600nm was adjusted to 10, and 15 μL of each lysate were applied to SDS-PAGE. (B) SDS-PAGE of Saci_1116-CtSS purification from crude extract of S. acidocaldarius MW001 pBSaraFX-albaUTR-saci_1116-CtSS via affinity chromatography. M: marker, CL: cell lysate; CE: crude extract (after centrifugation); P: cell pellet and debris after lysis; FT: flow-through; W: wash fraction; E: elution, 3 μg protein. Theoretical molecular weight of Saci_1116-SS: 37 kDa.
3.2 Effect of 5’-UTR insertion on promoter activity
To assess the impact of alba 5’-UTR insertion on the functionality and selective induction of the pentose-inducible promoter (saci_2122 promoter Para), we investigated protein production under the influence of different inducing and non-inducing sugars (Supplementary Figure S4). While S. acidocaldarius metabolizes the inducing pentoses D-xylose and L-arabinose, D-arabinose is not utilized as carbon source and hence acts as an artificial inducer and was therefore applied at a lower concentration. Our findings demonstrate that the functionality of the pentose-inducible promoter remained unaffected by the insertion of the alba 5’-UTR into the expression construct (Supplementary Figure S4). In agreement with previous studies, gene expression was induced by the addition of pentoses, while minimal basal expression was observed with sucrose, the disaccharide comprising D-glucose and D-fructose (Supplementary Figure S4; van der Kolk et al., 2020). Thus, alba 5’-UTR insertion resulted in a profound increase in reporter protein levels, while maintaining Para’s inducibility and pentose specificity.
3.3 Maintenance of cloning sites—influence of −2 and −1 nucleotide identities on reporter protein production
Expression vectors should facilitate the seamless integration of genes of interest (GOIs) through the presence of suitable restriction sites for restriction-and ligation-dependent cloning. In the pSVAara expression vectors, a NcoI restriction site (5’ C’CATGG 3′) serves as the upstream cloning site for GOI integration. For vectors featuring N-terminal protein tags, this NcoI site is positioned downstream of the tag-encoding nucleotide sequence. Thus, the integration of a 5’-UTR upstream of the N-terminal tag-coding sequence does not affect the NcoI cloning site (Supplementary Figure S1). However, in vectors with C-terminal protein tags, the direct insertion of the native alba 5’-UTR sequence (5′ GATAGGTGGTTTAA 3′) upstream of the translation start codon (“ATG”) would disrupt the NcoI restriction site used for GOI integration, which simultaneously encodes the translation start codon in C-terminal tag vectors (NcoI site: 5’ C’CATGG 3′, start codon in bold) (Supplementary Figure S1). Therefore, for convenient use, the two nucleotides located at the 3′-end of the 5’-UTR, immediately upstream of the start codon, hereafter referred to as −2 and − 1 nucleotides, were modified to preserve the NcoI restriction site in C-terminal protein tag vectors. Specifically, the identities of the −2 and −1 nucleotides were changed from “AA” to “CC,” while maintaining the overall length of the alba 5’-UTR. We assessed the impact of these nucleotide changes (i.e., from “AA” to “AC” or “CC”) on reporter protein production through enzyme activity assays, SDS-PAGE, and immunodetection (Figure 3).
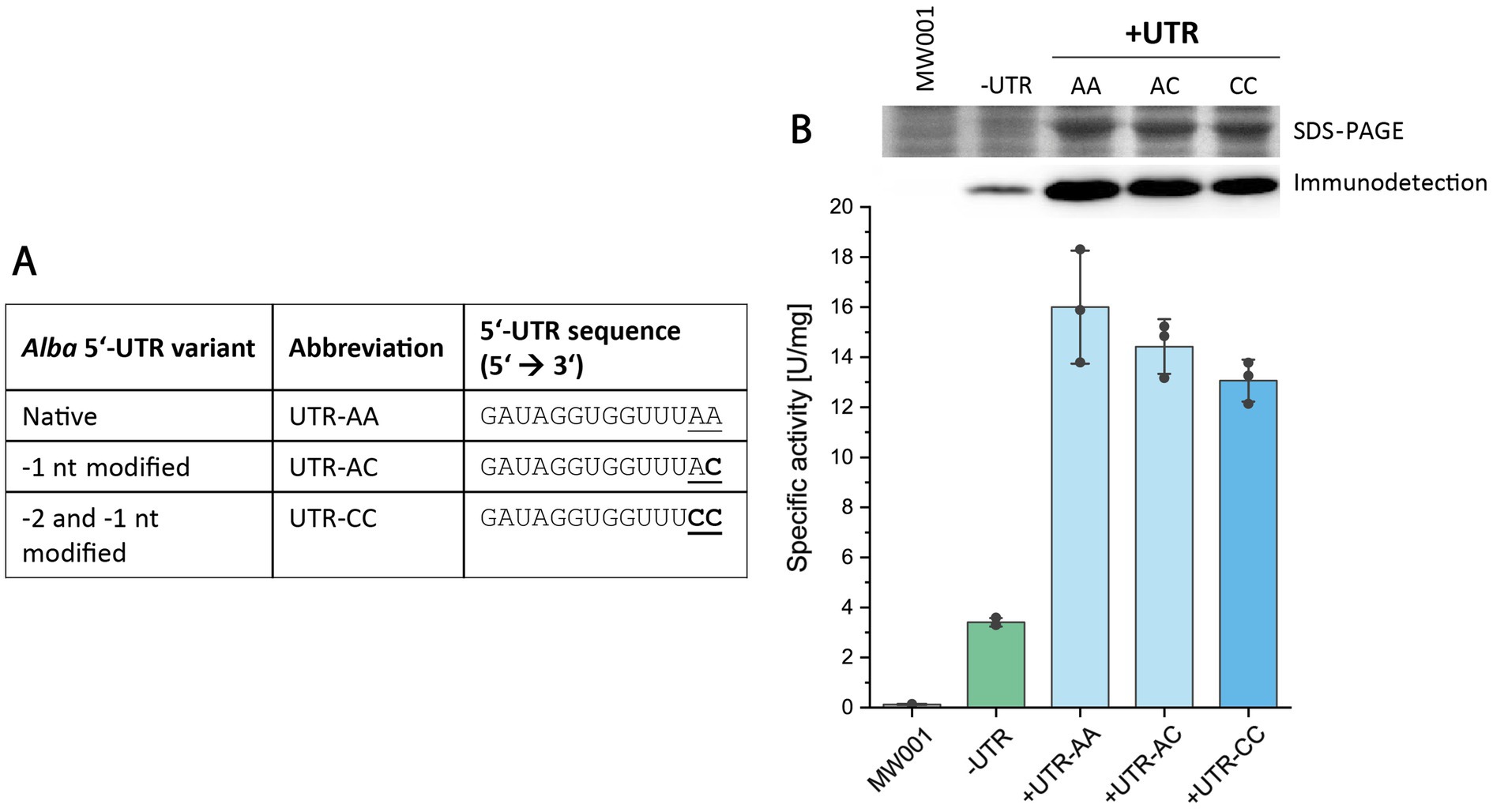
Figure 3. Influence of-2 and -1 nucleotide identities in the alba 5’-UTR on esterase production. (A) Overview of different alba 5’-UTR variants tested. (B) Expression analysis of Saci_1116-CtSS using constructs either without a 5’-UTR (-UTR) or with the alba 5’-UTR variants. Esterase activity was quantified in cell lysates using a continuous assay with pNP-acetate as substrate, monitoring pNP formation at 410 nm and 42°C. Error bars represent the standard deviation from three biological replicates (n = 3), shown as data points. Image sections of the respective SDS-PAGE (upper row) and Saci_1116-CtSS immunodetection (lower row) are provided (uncropped images in Supplementary Figure S5). Cultures were harvested at OD600nm values of 0.6 (log phase). S. acidocaldarius MW001 without plasmid served as a reference (MW001).
Similar values of specific esterase activities were obtained in the cell lysates of respective S. acidocaldarius expression cultures for all tested −2 and −1 nucleotide identities (“AA,” “AC” and “CC”) (Figure 3, Supplementary Table S3). SDS-PAGE analysis and esterase immunodetection confirmed the results of activity measurements (Supplementary Figure S5). In comparison to expression conditions lacking a 5’-UTR, constituting 2.5% of the total cell protein, the insertion of the alba 5’-UTR variants resulted in a 3.9–4.7-fold increase in esterase activity, with the reporter enzyme constituting 10–13% of cellular protein (Supplementary Table S3). This share was calculated by comparing the specific activities in cell lysates to the specific activity of purified esterase (124.5 U/mg).
3.4 Screening of different UTR candidates for efficient reporter protein production
In the next step, we sought to ascertain whether the efficacy of the expression system could be augmented by utilizing alternative 5’-UTR sequences. Leveraging literature and available proteomic data, we identified proteins of notable abundance in S. acidocaldarius and extracted their respective 5’-UTR sequences from RNA sequencing data (Weizmann Exploration, https://exploration.weizmann.ac.il/TCOL/; Cohen et al., 2016). Four 5’-UTR sequences, derived from genes saci_0330 (encoding a hypothetical protein), saci_1401 (thermosome α subunit), saci_2355 (surface-layer protein A), and saci_2354 (S-layer protein B), were chosen for comparative analysis alongside the alba 5’-UTR (Figure 4A).
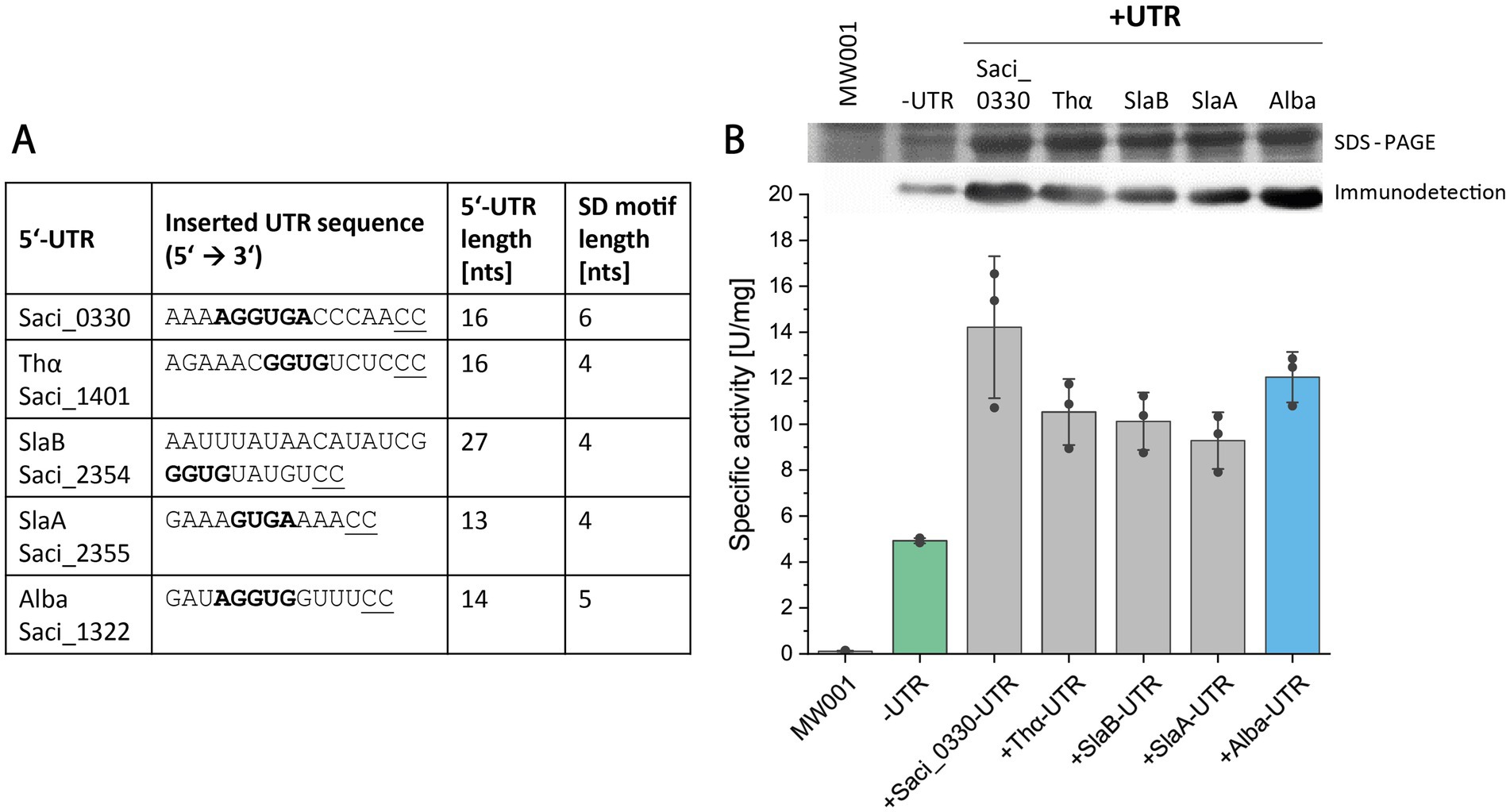
Figure 4. Screening of five 5’-UTR sequences for Saci_1116-CtSS production. (A) Overview of tested 5’-UTR sequences, including gene IDs, 5’-UTR sequences with their respective SD motifs (bold), UTR lengths, and SD motif lengths. (B) Saci_1116-CtSS production was analyzed using constructs without (-UTR) or with a 5’-UTR (+UTR). The 5’-UTR sequences from saci_0330, saci_1401 (Thα), saci_2354 (SlaB), saci_2355 (SlaA), and saci_1322 (Alba) were inserted upstream of the saci_1116-CtSS start codon in the expression plasmid. Specific esterase activity was determined in cell lysates using the continuous pNPA assay. Error bars represent the standard deviation from three biological replicates (n = 3), shown as data points. Sections of the respective SDS-PAGE of cell lysates (upper row) and immunodetection (lower row) are shown (uncropped images in Supplementary Figure S6). Cultures were harvested at OD600nm values of 0.8–1.2 (log phase). S. acidocaldarius MW001 without plasmid served as a reference (MW001).
The 5’-UTR sequence of saci_0330 corresponds to the transcript leader sequence of the operon saci_0330–0333, with saci_0331 annotated to encode a pyridine nucleotide-disulphide oxidoreductase, while the others are annotated as conserved proteins of unknown function (Chen et al., 2005; Esser et al., 2011). Saci_2355 and saci_2354, encoding S-layer proteins A and B, with their respective 5’-UTR sequences are encoded in a single operon (slaAB) on the complementary DNA strand (Veith et al., 2009). However, in addition to the promoter upstream of SlaA, available transcriptome data revealed a second promoter and a weak 5’-UTR signal upstream of slaB, consistent with RNA sequencing data (Cohen et al., 2016). The 5’-UTR of saci_1401, the gene encoding the thermosome α subunit (thα), a major chaperone complex in Sulfolobales (Chaston et al., 2016; Baes et al., 2020), and the alba 5’-UTR originate from single gene transcripts (Cohen et al., 2016). Based on the Sulfolobus SD consensus sequence (GGUGA; Tolstrup et al., 2000) and the further potential of base-pairing between 5’-UTR sequence and aSD sequence, we proposed the presence of a 6- and 5-nucleotide long SD motif in the 5’-UTRs of saci_0330 and alba, respectively. The other three UTR sequences (thα, slaB, slaA) included SD motifs of 4 nts in length (Figure 4A). As before, the NcoI restriction and cloning site (5’ C’CATGG 3′) was maintained, i.e., the nucleotide identities at positions -2 and -1 (relative to the translation start codon) were changed to “CC” for all 5’-UTR sequences tested, while preserving the overall length of each 5’-UTR (Figure 4A; Supplementary Table S4). Screening was conducted as previously described using esterase Saci_1116-CtSS as reporter protein, and its production was evaluated by specific esterase activity, SDS-PAGE, and immunodetection (Figure 4).
The 5’-UTR screening revealed significantly increased reporter protein activity in the presence of all tested 5’-UTR sequences compared to the absence of a 5’-UTR (Figure 4). Activity-based results were confirmed through SDS-PAGE analysis and esterase immunodetection (Figure 4B; Supplementary Figure S6). Integration of different 5’-UTR sequences increased the amount of reporter protein to approximately 7.5–11% of the total protein compared to 3% without an inserted 5’-UTR (Supplementary Table S5). On average, the 5’-UTRs of saci_0330 and alba exhibited the highest production yield of active esterase, with a lower standard deviation for alba 5’-UTR (Figure 4B).
In addition to the esterase, we also investigated the impact of these 5’-UTRs on another reporter protein, β-galactosidase LacS from S. solfataricus (Supplementary Figures S7, S8) (Pisani et al., 1990). LacS was commonly employed as a reporter in various studies (e.g., Jonuscheit et al., 2003; Wagner et al., 2012), including promoter investigations (Berkner et al., 2007, 2010; Peng et al., 2012; van der Kolk et al., 2020). In contrast to esterase Saci_1116, LacS already exhibits substantial expression levels, both with the N- and C-terminal Twin-Strep tag, in the absence of a 5’-UTR (Supplementary Figure S7). Nevertheless, the addition of 5’-UTRs resulted in a slight increase in LacS production (Supplementary Figure S8). Specifically, the alba 5’-UTR led to a 1.6-fold increase in LacS production (Supplementary Figure S8). Remarkably, the effect of the different 5’-UTRs on the production of the two reporter proteins showed a consistent pattern, demonstrating the transferability of the observed 5’-UTR effects to different target proteins. Notably, the degree of increase in protein production was dependent on the target protein.
3.5 Application of the alba 5’-UTR optimized expression vector for protein production
Our previous experiments illustrated the impact of 5’-UTR-assisted protein production for two different target proteins. To further investigate the effectiveness of the newly modified expression vectors, we screened their impact for nine additional proteins. In several studies, the production of glycosyltransferases (GTs) has been reported as demanding (e.g., Breton et al., 2006; Cobucci-Ponzano et al., 2011a, 2011b; Mestrom et al., 2019). Accordingly, also GTs originating from S. acidocaldarius have proven difficult to express in both E. coli (Jachlewski, 2013) and S. acidocaldarius when using vectors lacking a 5’-UTR (this study). Thus, we utilized the new alba 5’-UTR modified expression vectors, designated as pBSaraFX-albaUTR-NtSS and -CtSS (Supplementary Figures S9, S10), for GT synthesis. We assessed the production of six GTs from S. acidocaldarius, both in absence and in presence of the alba 5’-UTR, respectively. For all GTs tested (Saci_1904, Saci_1909, Saci_1911, Saci_1915, Saci_1916, Saci_1921), the presence of alba 5’-UTR increased protein amounts significantly, as confirmed by immunodetection of the Twin-Strep-tagged target proteins in cell lysates (Figure 5, Supplementary Figure S11). As a proof of concept, the production and purification of GT Saci_1911 was analysed in more detail. In the case of this GT, its production was higher with a C-terminal compared to an N-terminal tag (Figure 5A). C-terminal tagged Saci_1911 was purified from crude extracts by affinity chromatography, and the different fractions were visualized by SDS-PAGE (Figures 5B,C). Notably, the amount of purified Saci_1911-CtSS increased when cells contained the alba 5’-UTR expression plasmid compared to expression in the absence of a 5’-UTR (Figure 5D). The alba 5’-UTR-modified expression system was further used for the synthesis of three more proteins, namely glycerol kinase Saci_2033 (Schmerling et al., 2024a), malto-oligosyltrehalose trehalohydrolase (TreZ) from S. solfataricus (SSO2093) (Kobayashi et al., 1996; Fang et al., 2006), and hydroxyacyl-CoA dehydratase Saci_1085 (Schmerling et al., 2024b). Similar to the results observed for LacS, the expression of Saci_2033 remained high in both the absence and presence of the alba 5’-UTR (Supplementary Figure S12A). TreZ production was clearly enhanced in the presence of alba 5’-UTR (Supplementary Figure S12B). In particular, the expression of the hydroxyacyl-CoA dehydratase Saci_1085 was largely improved by the use of the alba 5’-UTR-modified expression plasmid, as demonstrated by protein visualization in cell lysates (Figure 6A) and affinity chromatography fractions (Figure 6B). Notably, the synthesis of active Saci_1085 was unsuccessful in E. coli (pET15b, N-terminal His6-tag), whereas the homogenously expressed enzyme was soluble and active as reversible 3-hydroxyacyl-CoA dehydratase (Schmerling et al., 2024b).
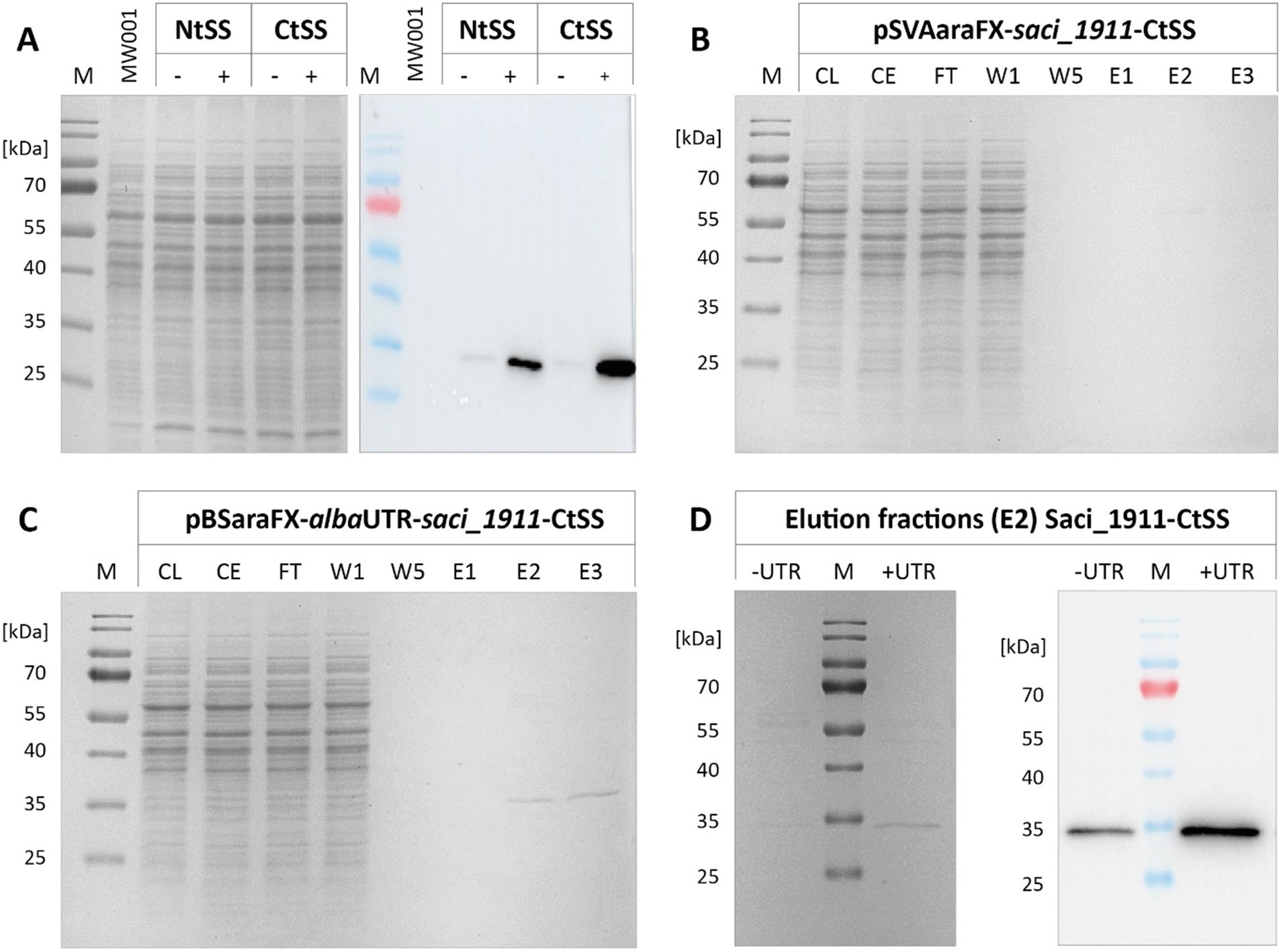
Figure 5. Effect of the alba 5’-UTR on the homologous production of glycosyltransferase Saci_1911. (A) SDS-PAGE and immunodetection of Twin-Strep-tagged Saci_1911 in cell lysates from S. acidocaldarius expressing saci_1911 without (−) and with alba 5’-UTR (+), and with an N-terminal (Nt) or C-terminal (Ct) Twin-Strep tag (SS). S. acidocaldarius MW001 without plasmid served as a reference (MW001). (B,C) SDS-PAGE gels of Saci_1911-CtSS affinity chromatography fractions after plasmid-based expression in S. acidocaldarius without (B) and with alba 5’-UTR (C). (D) SDS-PAGE and immunodetection of Saci_1911-CtSS in affinity chromatography elution fractions (E2). Expression cultures were harvested at OD600nm values of 0.8. Protein was purified from crude extracts using the Strep-Tactin®XT 4Flow® resin following the manufacturer’s protocol. M: marker; CL: cell lysate; CE: crude extract; FT: flow-through; W: wash fraction; E: elution fraction, 20 μL each. Theoretical molecular weight of Saci_1911-CtSS: 33.8 kDa.
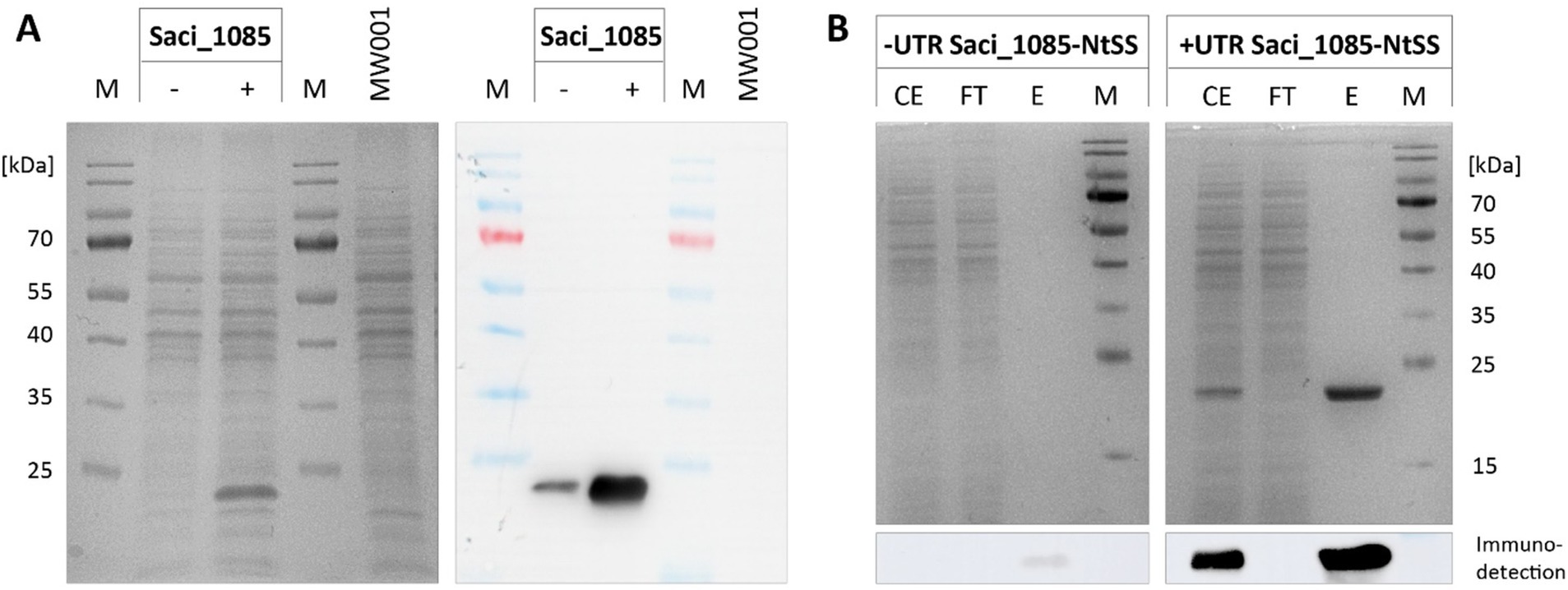
Figure 6. Effect of the alba 5’-UTR on the production of hydroxyacyl-CoA dehydratase Saci_1085-NtSS. (A) SDS-PAGE and immunodetection of Saci_1085-NtSS in cell lysates from S. acidocaldarius expressing saci_1085 without (−) and with alba 5’-UTR (+) and with an N-terminal Twin-Strep tag (NtSS). S. acidocaldarius MW001 without plasmid served as a reference (MW001). (B) SDS-PAGE of Saci_1085-NtSS affinity chromatography fractions. Protein was purified using Strep-Tactin®XT 4Flow® resin. The relevant image section of Saci_1085-NtSS immunodetection using Strep-Tactin-HRP conjugate is presented below. M: marker; CE: crude extract; FT: flow-through; E: pooled elution fractions; 2 μg protein for +UTR. Theoretical molecular weight of Saci_1085-NtSS: 22.5 kDa.
In summary, we observed a noticeable positive impact of the alba 5’-UTR on protein expression in nine out of eleven cases. For the remaining two proteins, LacS and Saci_2033, production levels were already high without the 5’-UTR and remained high in its presence. Altogether, these results strongly demonstrate the efficacy of the 5’-UTR modified expression vector and highlight its potential for enhancing protein production across various targets.
3.6 Impact of Shine-Dalgarno (SD) motif on protein production
As previously noted, the native alba 5’-UTR contains a 5 nt long SD motif. This prompted us to investigate the relevance of this motif for efficient protein production. Considering the aSD sequence found in S. acidocaldarius 16S rRNA, the SD motif “5’ UGAGGUGA 3′” would perfectly complement the aSD over a length of 8 nucleotides (Ma et al., 2002; Schmitt et al., 2020; Wen et al., 2021). To explore the potential enhancement of protein production by extending the SD motif, we constructed alba 5’-UTR variants containing 8-nucleotide long SD motifs. The first variant, designated “8SDmut” was generated by site-directed mutagenesis, altering nucleotides neighboring the native 5 nt long SD motif while maintaining the overall UTR length (Figure 7A). The second variant, named “8SDins,” was generated by the insertion of two nucleotides to create an 8-nucleotide long SD motif, thereby increasing the total length of the 5’-UTR. Additionally, the native 5 nt long SD motif was completely mutated to generate a 5’-UTR lacking the SD motif (“noSD” variant) (Figure 7A). Throughout these modifications, the overall GC content of the 5’-UTR was kept constant at 50%. Esterase Saci_1116-CtSS was utilized as reporter protein and its production was assessed by enzymatic activity, SDS-PAGE, and immunodetection (Figure 7B).
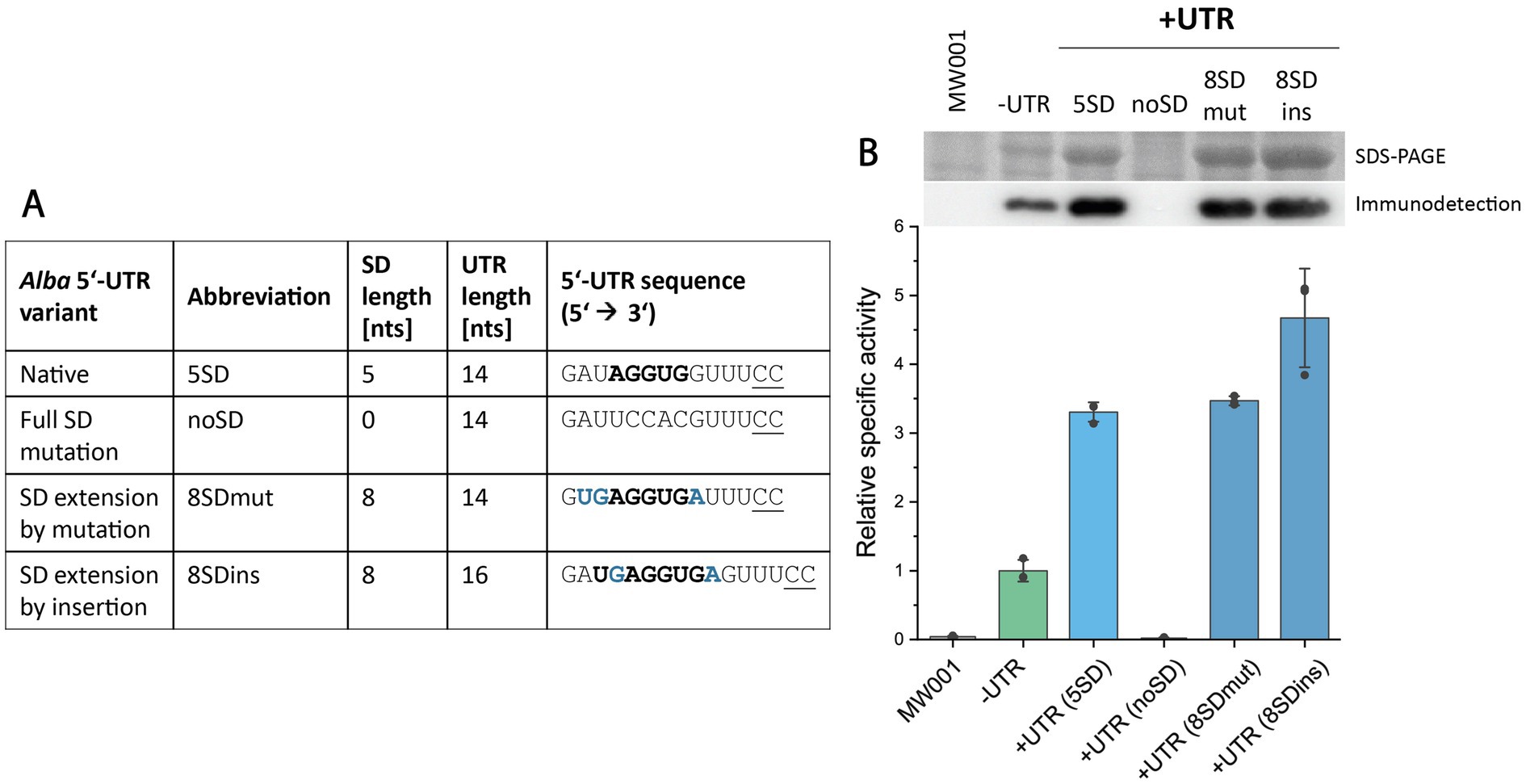
Figure 7. Effect of SD motif sequence alterations within the alba 5’-UTR on esterase production. (A) Overview of tested alba 5’-UTR-SD variants, including SD lengths, UTR lengths, and 5’-UTR nucleotide sequences. (B) Esterase activity in cell lysate samples from S. acidocaldarius MW001 expression cultures was determined using the continuous pNPA assay. Error bars indicate the standard deviation of three biological replicates (n = 3), shown as data points. Sections of the respective SDS-PAGE analysis of cell lysates (upper row) and Saci_1116-CtSS immunodetection (lower row) are shown (uncropped images in Supplementary Figure S13). Cultures were harvested at OD600nm values of 0.6–0.8 (log phase). S. acidocaldarius MW001 without plasmid served as a reference (MW001).
Strikingly, the absence of the SD motif within the alba 5’-UTR resulted in a complete cessation of protein production, as confirmed by enzymatic measurements and protein visualization (Figure 7, Supplementary Figure S13). One of the 8-nucleotide long SD variants, “8SDins,” facilitated a further increase in protein production compared to the native alba 5’-UTR with a 5-nucleotide long SD motif. In contrast, the variant “8SDmut,” generated through nucleotide mutagenesis, displayed protein production levels comparable to those of the native alba 5’-UTR (Figure 7B). These results suggest that the sole extension of the SD motif does not account for the observed increase in protein production, indicating the presence of complex effects at the sequence or potentially transcript level. Importantly, our findings underscore the crucial role of the SD motif within the alba 5’-UTR in allowing protein synthesis.
4 Discussion
In this study, we examined the impact of 5’-UTR sequence insertions into S. acidocaldarius Para expression plasmids on the production of different (reporter) proteins. Our findings elucidate that the integration of SD motif-containing 5’-UTRs from proteins with high cellular abundance significantly enhanced plasmid-based protein synthesis for the majority of tested target proteins.
The utilization of 5’-UTRs and RBS, i.e., preserved Shine-Dalgarno sequences, is prevalent in bacterial expression vectors, such as pBAD, pET, pMAL, pQE, and pGEX plasmids for heterologous expression in E. coli, or pHT and pBSMul1 expression plasmids in Bacillus subtilis (Brockmeier et al., 2006; Yang et al., 2021). Consequently, the influence of bacterial 5’-UTR sequence elements, i.e., SD motifs or spacer sequences, has been extensively investigated in several bacterial organisms over the last decades (e.g., Wu and Janssen, 1997; Sakai et al., 2001; Khosa et al., 2018; Volkenborn et al., 2020). In contrast, 5’-UTRs have been less considered and studied as a relevant feature for gene overexpression in Archaea. However, this disparity is likely related to the fact that, unlike bacteria, many common archaeal model organisms, including Haloarchaea, Thermoproteales, and Sulfolobales, primarily generate leaderless gene transcripts (e.g., 72% for H. volcanii (Schmitt et al., 2020), or 69% for S. solfataricus (Wurtzel et al., 2010); Slupska et al., 2001; Brenneis et al., 2007; Kramer et al., 2014). Consequently, 5’-UTRs were considered less relevant for protein synthesis and as a feature of archaeal expression vectors. However, some existing archaeal expression vectors do generate leadered mRNA transcripts for gene overexpression, facilitated by the presence of an extended “promoter” sequence that leads to the generation of a 5’-UTR. For instance, the sequence of the heat-inducible tf55 promoter in S. solfataricus expression vectors generates an 18 nt long 5’-UTR harbouring a putative 4 nt long SD motif (Jonuscheit et al., 2003). The existing sugar-inducible S. acidocaldarius expression vectors featuring Para from saci_2122 and Pmal (maltose) from saci_1165 generate leaderless transcripts, as determined by available RNA sequencing data of corresponding gene transcripts from the genome (Cohen et al., 2016). However, in the case of Pxyl (promoter from saci_1938) (van der Kolk et al., 2020), a leadered transcript devoid of an SD motif is generated (34 nts, Cohen et al., 2016).
Here, we introduced SD motif-containing 5’-UTR sequences from genes encoding highly abundant proteins into the Para S. acidocaldarius expression vector. All five tested 5’-UTR insertions demonstrated enhanced production of esterase Saci_1116, showing a two- to four-fold increase. Notably, the use of alba (saci_1322) 5’-UTR, encoding the most abundant protein in S. acidocaldarius according to full proteome data (Schmerling et al., 2024a), resulted in a four-fold increase in the yield of soluble, active esterase. In agreement, in Methanococcus maripaludis the replacement of a wild-type 5’-UTR by 5’-UTRs from highly expressed genes (e.g., slp (S-layer protein) and hmmA (histone A)) led to increased protein production (Akinyemi et al., 2021). Thus, both in M. maripaludis and in S. acidocaldarius the utilization of 5’-UTRs originating from highly expressed genes resulted in higher plasmid-based target protein production.
In contrast, tested native 5’-UTRs from H. volcanii (hlr (hoxA-like transcriptional regulator) and hp (conserved hypothetical protein)) without an SD motif reduced reporter protein amounts by approximately two-fold when compared to leaderless transcripts (Brenneis and Soppa, 2009). However, two out of four artificial 5’-UTR sequences (20 nts, random sequence, no SD) increased reporter activity by factors of two and five, respectively, in H. volcanii (Brenneis et al., 2007; Hering et al., 2009). Furthermore, 5’-UTR sequences from genes involved in gas vesicle formation in Halobacterium salinarum and a short 5′-terminal nucleotide sequence (4 nts) from heat shock protein 70 (hsp70) of Natrinema sp. J7 decreased reporter production in H. volcanii (Born and Pfeifer, 2019; Chen et al., 2015) (compare Supplementary Tables S6, S7 for literature review). Notably, modifications to the nucleotide identities at positions “-1” and “-2” within the short hsp70 5’-UTR revealed varied effects on protein production in H. volcanii (Chen et al., 2015), hence providing a more complex picture.
In S. islandicus, mutating the two nucleotides upstream of the translation start codon (from “AU” to “CC”) within the 6 nt long 5’-UTR of ParaS (Peng et al., 2009) reduced protein levels by approximately 45%, attributed to a 50% decrease in transcript levels but a 30% increase in translational efficiency (Ao et al., 2013). Notably, 5’-UTRs were previously discussed to increase the relative promoter strength of the araS promoter in S. solfataricus by acting as initiator element that can contribute to promoter strength (Peng et al., 2009; Ao et al., 2013). In our study, we also made changes to the two nucleotides immediately upstream of the translation start codon within the alba 5’-UTR to retain the NcoI cloning site. However, we did not observe a significant effect of this modification on esterase production. Thus, the impact of the nucleotide identities directly upstream of the translation start codon is likely unique to the overall 5’-UTR sequence and its effects on different regulatory levels, such as transcription initiation, transcript stability or translation initiation, rendering it rather complex to predict. Further investigations, including the determination of transcript levels and stabilities, will help to shed more light into these aspects in the future.
Occasionally, the relevance of the SD (RBS) sequence on protein production has been investigated for some 5’-UTRs in Archaea (Supplementary Table S7). Concerning Sulfolobales, previous studies have investigated SD mutations or insertions in S. solfataricus and S. islandicus, respectively. For S. solfataricus, the substitution of two nucleotides of the SD motifs of ORF104 or ORF143 of a bicistronic mRNA (8 and 7 nt long SD motifs, respectively) entirely abolished their translation in a cell-free in vitro translational system (Condó et al., 1999). Consistent with this finding, we observed no protein production upon complete mutagenesis of the SD motif within the alba 5’-UTR in S. acidocaldarius. The insertion of 14 nts, including an 8 nt long SD motif, into an existing 6 nt long 5’-UTR sequence in ParaS expression vectors led to a three-fold increase in reporter activity (β-galactosidase) in S. islandicus (Peng et al., 2012; Peng et al., 2017). Similarly, we found that the insertion of two additional nucleotides to the alba 5’-UTR, thereby extending the length of the SD motif and the overall UTR length, increased the yield of esterase. In Thermococcus kodakarensis, mutations to the second nucleotide of a 6-nucleotide SD motif significantly decreased protein production (Santangelo et al., 2008). However, in H. volcanii, effects of SD motif modifications in different 5’-UTR sequences were more ambiguous (Kramer et al., 2014; Sartorius-Neef and Pfeifer, 2004) (compare Supplementary Table S7). Concluding, the relevance of SD motifs within archaeal 5’-UTRs remains uncertain, emphasizing the need to further investigate their impact on protein expression at the different regulatory levels.
Altogether, reports on the influence of 5’-UTRs in Archaea suggest that, compared to leaderless transcripts, 5’-UTRs do not consistently enhance protein production. Multiple factors may contribute to the differential effects of 5’-UTR sequences on protein production efficiency, including complex regulatory mechanisms at the posttranscriptional level (e.g., secondary structures, transcript stability), as well as alterations to the ribosome docking site for translation initiation. This complexity likely applies to S. acidocaldarius as well; however, our preselection of 5’-UTR sequences from genes with high protein abundance probably precluded the appearance of 5’-UTRs that might reduce protein production.
5 Conclusion
For many archaeal genes, heterologous expression in mesophilic bacterial strains, such as E. coli, is effective, with established straightforward purification protocols, including heat precipitation for thermophilic proteins. However, there are numerous examples where attempts to express archaeal proteins in mesophilic bacterial hosts fail. Thus, archaeal expression systems are needed for synthesizing demanding archaeal proteins, often with biotechnological potential, that cannot be efficiently produced in bacterial hosts. Our study showcases the optimization of plasmid-based protein production through the incorporation of SD motif-containing 5’-UTR sequences in the archaeal expression host S. acidocaldarius. The integration of all tested 5′-terminal sequences into Para expression vectors led to a significant enhancement in protein yield, exemplified by the substantial increase in the production of esterase Saci_1116. Notably, among the five different 5′-terminal sequences examined, the 5’-UTR of the Alba-encoding gene saci_1322 was found to be highly efficient and reliable. The alba 5’-UTR containing expression vector proved to be effective for the synthesis of different Sulfolobales target proteins. Overall, the application of 5’-UTR-optimized expression vectors holds promise for facilitating the synthesis of other challenging thermostable proteins in the future.
Data availability statement
The original contributions presented in the study are included in the article/Supplementary material, further inquiries can be directed to the corresponding authors. Nucleotide sequences and the vector DNA are available to the scientific community upon request.
Author contributions
LK: Conceptualization, Formal analysis, Writing – original draft, Writing – review & editing, Data curation, Investigation, Methodology, Visualization. AW: Data curation, Formal analysis, Investigation, Methodology, Writing – original draft. CS: Investigation, Writing – review & editing, Visualization. TB: Formal analysis, Writing – review & editing. JK: Formal analysis, Writing – review & editing. CB: Supervision, Writing – review & editing. BS: Conceptualization, Formal analysis, Supervision, Writing – review & editing, Funding acquisition, Project administration, Validation.
Funding
The author(s) declare that financial support was received for the research, authorship, and/or publication of this article. This work received funding from the German Research Foundation (DFG SI 642/13-1) (ArchaeaEPS -Archaeal biofilms: Composition of extracellular polymeric substances, exopolysaccharide synthesis and transport in Sulfolobus acidocaldarius) and the Federal Ministry of Education and Research (BS, BMBF 031B0848A; JK, BMBF 031B0848B; HotAcidFACTORY -"Sulfolobus acidocaldarius as novel thermoacidophilic bio-factory").
Acknowledgments
SIA preprint of this article was published on March 2, 2024 on bioRxiv (Kuschmierz et al., 2024; doi: 10.1101/2024.03.01.582787). OpenAI (2024) ChatGPT (version 3.5) [Large language model] was applied for language optimization. We acknowledge support by the Open Access Publication Fund of the University of Duisburg-Essen. We would like to express our sincere gratitude to Sabrina Ninck and Markus Kaiser for providing proteomics information and Malin Kretzschmar and Semira Gertchen for their valuable experimental contributions to this research project.
Conflict of interest
The authors declare that the research was conducted in the absence of any commercial or financial relationships that could be construed as a potential conflict of interest.
Publisher’s note
All claims expressed in this article are solely those of the authors and do not necessarily represent those of their affiliated organizations, or those of the publisher, the editors and the reviewers. Any product that may be evaluated in this article, or claim that may be made by its manufacturer, is not guaranteed or endorsed by the publisher.
Supplementary material
The Supplementary material for this article can be found online at: https://www.frontiersin.org/articles/10.3389/fmicb.2024.1443342/full#supplementary-material
References
Akinyemi, T. S., Shao, N., Lyu, Z., Drake, I. J., Liu, Y., and Whitman, W. B. (2021). Tuning gene expression by phosphate in the methanogenic archaeon Methanococcus maripaludis. ACS Synth. Biol. 10, 3028–3039. doi: 10.1021/acssynbio.1c00322
Ao, X., Li, Y., Wang, F., Feng, M., Lin, Y., Zhao, S., et al. (2013). The Sulfolobus initiator element is an important contributor to promoter strength. J. Bacteriol. 195, 5216–5222. doi: 10.1128/JB.00768-13
Babski, J., Haas, K. A., Nather-Schindler, D., Pfeiffer, F., Forstner, K. U., Hammelmann, M., et al. (2016). Genome-wide identification of transcriptional start sites in the haloarchaeon Haloferax volcanii based on differential RNA-Seq (dRNA-Seq). BMC Genomics 17:629. doi: 10.1186/s12864-016-2920-y
Baes, R., Lemmens, L., Mignon, K., Carlier, M., and Peeters, E. (2020). Defining heat shock response for the thermoacidophilic model crenarchaeon Sulfolobus acidocaldarius. Extremophiles 24, 681–692. doi: 10.1007/s00792-020-01184-y
Berkner, S., Grogan, D., Albers, S. V., and Lipps, G. (2007). Small multicopy, non-integrative shuttle vectors based on the plasmid pRN1 for Sulfolobus acidocaldarius and Sulfolobus solfataricus, model organisms of the (cren-)archaea. Nucleic Acids Res. 35:e88. doi: 10.1093/nar/gkm449
Berkner, S., Wlodkowski, A., Albers, S. V., and Lipps, G. (2010). Inducible and constitutive promoters for genetic systems in Sulfolobus acidocaldarius. Extremophiles 14, 249–259. doi: 10.1007/s00792-010-0304-9
Born, J., and Pfeifer, F. (2019). Improved GFP variants to study gene expression in haloarchaea. Front. Microbiol. 10:1200. doi: 10.3389/fmicb.2019.01200
Brenneis, M., Hering, O., Lange, C., and Soppa, J. (2007). Experimental characterization of cis-acting elements important for translation and transcription in halophilic archaea. PLoS Genet. 3:e229. doi: 10.1371/journal.pgen.0030229
Brenneis, M., and Soppa, J. (2009). Regulation of translation in haloarchaea: 5′- and 3'-UTRs are essential and have to functionally interact in vivo. PLoS One 4:e4484. doi: 10.1371/journal.pone.0004484
Breton, C., Snajdrova, L., Jeanneau, C., Koca, J., and Imberty, A. (2006). Structures and mechanisms of glycosyltransferases. Glycobiology 16, 29R–37R. doi: 10.1093/glycob/cwj016
Brock, T. D., Brock, K. M., Belly, R. T., and Weiss, R. L. (1972). Sulfolobus: a new genus of sulfur-oxidizing bacteria living at low pH and high temperature. Arch. Mikrobiol. 84, 54–68. doi: 10.1007/BF00408082
Brockmeier, U., Wendorff, M., and Eggert, T. (2006). Versatile expression and secretion vectors for Bacillus subtilis. Curr. Microbiol. 52, 143–148. doi: 10.1007/s00284-005-0231-7
Cajili, M. K. M., and Prieto, E. I. (2022). Interplay between Alba and Cren 7 regulates chromatin compaction in Sulfolobus solfataricus. Biomol. Ther. 12:481. doi: 10.3390/biom12040481
Chaston, J. J., Smits, C., Aragao, D., Wong, A. S., Ahsan, B., Sandin, S., et al. (2016). Structural and functional insights into the evolution and stress adaptation of type II chaperonins. Structure 24, 364–374. doi: 10.1016/j.str.2015.12.016
Chen, H., Bjerknes, M., Kumar, R., and Jay, E. (1994). Determination of the optimal aligned spacing between the Shine-Dalgarno sequence and the translation initiation codon of Escherichia coli mRNAs. Nucleic Acids Res. 22, 4953–4957. doi: 10.1093/nar/22.23.4953
Chen, L., Brugger, K., Skovgaard, M., Redder, P., She, Q., Torarinsson, E., et al. (2005). The genome of Sulfolobus acidocaldarius, a model organism of the Crenarchaeota. J. Bacteriol. 187, 4992–4999. doi: 10.1128/JB.187.14.4992-4999.2005
Chen, W., Yang, G., He, Y., Zhang, S., Chen, H., Shen, P., et al. (2015). Nucleotides flanking the start codon in hsp70 mRNAs with very short 5’-UTRs greatly affect gene expression in haloarchaea. PLoS One 10:e0138473. doi: 10.1371/journal.pone.0138473
Cobucci-Ponzano, B., Rossi, M., and Moracci, M. (2011a). “Carbohydrate-active enzymes from hyperthermophiles: biochemistry and applications” in Extremophiles handbook. ed. K. Horikoshi (Tokyo: Springer), 427–441.
Cobucci-Ponzano, B., Strazzulli, A., Rossi, M., and Moracci, M. (2011b). Glycosynthases in biocatalysis. Adv. Synth. Catal. 353, 2284–2300. doi: 10.1002/adsc.201100461
Cohen, O., Doron, S., Wurtzel, O., Dar, D., Edelheit, S., Karunker, I., et al. (2016). Comparative transcriptomics across the prokaryotic tree of life. Nucleic Acids Res. 44, W46–W53. doi: 10.1093/nar/gkw394
Condó, I., Clammaruconi, A., Benelli, D., Ruggero, D., and Londei, P. (1999). Cis-acting signals controlling translational initiation in the thermophilic archaeon Sulfolobus solfataricus. Mol. Microbiol. 34, 377–384. doi: 10.1046/j.1365-2958.1999.01615.x
de Lise, F., Iacono, R., Moracci, M., Strazzulli, A., and Cobucci-Ponzano, B. (2023). Archaea as a model system for molecular biology and biotechnology. Biomol. Ther. 13:114. doi: 10.3390/biom13010114
Edelheit, O., Hanukoglu, A., and Hanukoglu, I. (2009). Simple and efficient site-directed mutagenesis using two single-primer reactions in parallel to generate mutants for protein structure-function studies. BMC Biotechnol. 9:61. doi: 10.1186/1472-6750-9-61
Eichler, J., and Adams, M. W. (2005). Posttranslational protein modification in Archaea. Microbiol. Mol. Biol. Rev. 69, 393–425. doi: 10.1128/MMBR.69.3.393-425.2005
Esser, D., Hoffmann, L., Pham, T. K., Bräsen, C., Qiu, W., Wright, P. C., et al. (2016). Protein phosphorylation and its role in archaeal signal transduction. FEMS Microbiol. Rev. 40, 625–647. doi: 10.1093/femsre/fuw020
Esser, D., Kouril, T., Zaparty, M., Sierocinski, P., Chan, P. P., Lowe, T., et al. (2011). Functional curation of the Sulfolobus solfataricus P 2 and S. acidocaldarius 98-3 complete genome sequences. Extremophiles 15, 711–712. doi: 10.1007/s00792-011-0392-1
Fang, T. Y., Tseng, W. C., Guo, M. S., Shih, T. Y., and Hung, X. G. (2006). Expression, purification, and characterization of the maltooligosyltrehalose trehalohydrolase from the thermophilic archaeon Sulfolobus solfataricus ATCC 35092. J. Agric. Food Chem. 54, 7105–7112. doi: 10.1021/jf061318z
Geertsma, E. R., and Dutzler, R. (2011). A versatile and efficient high-throughput cloning tool for structural biology. Biochemistry 50, 3272–3278. doi: 10.1021/bi200178z
Gomes-Filho, J. V., Daume, M., and Randau, L. (2018). Unique archaeal small RNAs. Annu. Rev. Genet. 52, 465–487. doi: 10.1146/annurev-genet-120417-031300
Hering, O., Brenneis, M., Beer, J., Suess, B., and Soppa, J. (2009). A novel mechanism for translation initiation operates in haloarchaea. Mol. Microbiol. 71, 1451–1463. doi: 10.1111/j.1365-2958.2009.06615.x
Jachlewski, S. (2013). Biofilm formation and EPS analysis of the thermoacidophilic archaeon Sulfolobus acidocaldarius. Dissertation, University of Duisburg-Essen. urn:nbn:de:hbz:464-20131211-102054-5.
Jarrell, K. F., Ding, Y., Meyer, B. H., Albers, S. V., Kaminski, L., and Eichler, J. (2014). N-linked glycosylation in Archaea: a structural, functional, and genetic analysis. Microbiol. Mol. Biol. Rev. 78, 304–341. doi: 10.1128/MMBR.00052-13
Jonuscheit, M., Martusewitsch, E., Stedman, K. M., and Schleper, C. (2003). A reporter gene system for the hyperthermophilic archaeon Sulfolobus solfataricus based on a selectable and integrative shuttle vector. Mol. Microbiol. 48, 1241–1252. doi: 10.1046/j.1365-2958.2003.03509.x
Khosa, S., Scholz, R., Schwarz, C., Trilling, M., Hengel, H., Jaeger, K.-E., et al. (2018). An A/U-rich enhancer region is required for high-level protein secretion through the HlyA type I secretion system. Appl. Environ. Microbiol. 84, e01163–e01117. doi: 10.1128/AEM.01163-17
Kobayashi, K., Kato, M., Miura, Y., Kettoku, M., Komeda, T., and Iwamatsu, A. (1996). Gene cloning and expression of new trehalose-producing enzymes from the hyperthermophilic archaeum Sulfolobus solfataricus KM1. Biosci. Biotechnol. Biochem. 60, 1882–1885. doi: 10.1271/bbb.60.1882
Kramer, P., Gabel, K., Pfeiffer, F., and Soppa, J. (2014). Haloferax volcanii, a prokaryotic species that does not use the Shine Dalgarno mechanism for translation initiation at 5'-UTRs. PLoS One 9:e94979. doi: 10.1371/journal.pone.0094979
Kuschmierz, L., Wagner, A., Busche, T., Kalinowski, J., Bräsen, C., and Siebers, B. (2024). 5′-untranslated region sequences enhance plasmid-based protein production in Sulfolobus acidocaldarius. BioRxiv. 2024.03.01.582787. doi: 10.1101/2024.03.01.582787
Laurens, N., Driessen, R. P., Heller, I., Vorselen, D., Noom, M. C., Hol, F. J., et al. (2012). Alba shapes the archaeal genome using a delicate balance of bridging and stiffening the DNA. Nat. Commun. 3:1328. doi: 10.1038/ncomms2330
Lewis, A. M., Recalde, A., Bräsen, C., Counts, J. A., Nussbaum, P., Bost, J., et al. (2021). The biology of thermoacidophilic archaea from the order Sulfolobales. FEMS Microbiol. Rev. 45:fuaa063. doi: 10.1093/femsre/fuaa063
Liebeton, K., Lengefeld, J., and Eck, J. (2014). The nucleotide composition of the spacer sequence influences the expression yield of heterologously expressed genes in Bacillus subtilis. J. Biotechnol. 191, 214–220. doi: 10.1016/j.jbiotec.2014.06.027
Lipps, G. (2004). The replication protein of the Sulfolobus islandicus plasmid pRN1. Biochem. Soc. Trans. 32, 240–244. doi: 10.1042/bst0320240
Ma, J., Campbell, A., and Karlin, S. (2002). Correlations between Shine-Dalgarno sequences and gene features such as predicted expression levels and operon structures. J. Bacteriol. 184, 5733–5745. doi: 10.1128/jb.184.20.5733-5745.2002
Mai, V. Q., Chen, X., Hong, R., and Huang, L. (1998). Small abundant DNA binding proteins from the thermoacidophilic archaeon Sulfolobus shibatae constrain negative DNA supercoils. J. Bacteriol. 180, 2560–2563. doi: 10.1128/JB.180.9.2560-2563.1998
Martínez-Espinosa, R. M. (2019). Heterologous and homologous expression of proteins from haloarchaea: denitrification as case of study. Int. J. Mol. Sci. 21:82. doi: 10.3390/ijms21010082
Mestrom, L., Przypis, M., Kowalczykiewicz, D., Pollender, A., Kumpf, A., Marsden, S. R., et al. (2019). Leloir glycosyltransferases in applied biocatalysis: a multidisciplinary approach. Int. J. Mol. Sci. 20:263. doi: 10.3390/ijms20215263
Peng, N., Deng, L., Mei, Y., Jiang, D., Hu, Y., Awayez, M., et al. (2012). A synthetic arabinose-inducible promoter confers high levels of recombinant protein expression in hyperthermophilic archaeon Sulfolobus islandicus. Appl. Environ. Microbiol. 78, 5630–5637. doi: 10.1128/AEM.00855-12
Peng, N., Han, W., Li, Y., Liang, Y., and She, Q. (2017). Genetic technologies for extremely thermophilic microorganisms of Sulfolobus, the only genetically tractable genus of crenarchaea. Sci. China Life Sci. 60, 370–385. doi: 10.1007/s11427-016-0355-8
Peng, N., Xia, Q., Chen, Z., Liang, Y. X., and She, Q. (2009). An upstream activation element exerting differential transcriptional activation on an archaeal promoter. Mol. Microbiol. 74, 928–939. doi: 10.1111/j.1365-2958.2009.06908.x
Pisani, F. M., Rella, R., Raia, C. A., Rozzo, C., Nucci, R., Gambacorta, A., et al. (1990). Thermostable beta-galactosidase from the archaebacterium Sulfolobus solfataricus. J. Biochem. 187, 321–328. doi: 10.1111/j.1432-1033.1990.tb15308.x
Reeve, B., Hargest, T., Gilbert, C., and Ellis, T. (2014). Predicting translation initiation rates for designing synthetic biology. Front. Bioeng. Biotechnol. 2:1. doi: 10.3389/fbioe.2014.00001
Ren, G.-X., Guo, X.-P., and Sun, Y.-C. (2017). Regulatory 3′ untranslated regions of bacterial mRNAs. Front. Microbiol. 8:1276. doi: 10.3389/fmicb.2017.01276
Rosano, G. L., and Ceccarelli, E. A. (2014). Recombinant protein expression in Escherichia coli: advances and challenges. Front. Microbiol. 5:172. doi: 10.3389/fmicb.2014.00172
Sakai, H., Imamura, C., Osada, Y., Saito, R., Washio, T., and Tomita, M. (2001). Correlation between Shine-Dalgarno sequence conservation and codon usage of bacterial genes. J. Mol. Evol. 52, 164–170. doi: 10.1007/s002390010145
Santangelo, T. J., Cubonova, L., and Reeve, J. N. (2008). Shuttle vector expression in Thermococcus kodakaraensis: contributions of cis elements to protein synthesis in a hyperthermophilic archaeon. Appl. Environ. Microbiol. 74, 3099–3104. doi: 10.1128/AEM.00305-08
Sartorius-Neef, S., and Pfeifer, F. (2004). In vivo studies on putative Shine-Dalgarno sequences of the halophilic archaeon Halobacterium salinarum. Mol. Microbiol. 51, 579–588. doi: 10.1046/j.1365-2958.2003.03858.x
Schmerling, C., Schroeder, C., Zhou, X., Busche, T., Kalinowski, J., Montero, L., et al. (2024a). Glycerol degradation in the thermoacidophilic crenarchaeon Sulfolobus acidocaldarius involves an unusual glycerol-3-phosphate dehydrogenase. BioRxiv. 2024.02.29.582781. doi: 10.1101/2024.02.29.582781
Schmerling, C., Zhou, X., Goers, P. E., Koestlbacher, S., and Kessenbrock, T. (2024b). De novo synthesis of fatty acids in Archaea via an archaeal fatty acid synthase complex. BioRxiv. 2024.07.05.601840. doi: 10.1101/2024.07.05.601840
Schmitt, E., Coureux, P. D., Kazan, R., Bourgeois, G., Lazennec-Schurdevin, C., and Mechulam, Y. (2020). Recent advances in archaeal translation initiation. Front. Microbiol. 11:584152. doi: 10.3389/fmicb.2020.584152
Schocke, L., Bräsen, C., and Siebers, B. (2019). Thermoacidophilic Sulfolobus species as source for extremozymes and as novel archaeal platform organisms. Curr. Opin. Biotechnol. 59, 71–77. doi: 10.1016/j.copbio.2019.02.012
Shine, J., and Dalgarno, L. (1975). Terminal-sequence analysis of bacterial ribosomal RNA. Correlation between the 3′-terminal-polypyrimidine sequence of 16-S RNA and translational specificity of the ribosome. Eur. J. Biochem. 57, 221–230. doi: 10.1111/j.1432-1033.1975.tb02294.x
Slupska, M. M., King, A. G., Fitz-Gibbon, S., Besemer, J., Borodovsky, M., and Miller, J. H. (2001). Leaderless transcripts of the crenarchaeal hyperthermophile Pyrobaculum aerophilum. J. Mol. Biol. 309, 347–360. doi: 10.1006/jmbi.2001.4669
Sobek, H., and Görisch, H. (1988). Purification and characterization of a heat-stable esterase from the thermoacidophilic archaebacterium Sulfolobus acidocaldarius. Biochem. J. 250, 453–458. doi: 10.1042/bj2500453
Sobek, H., and Görisch, H. (1989). Further kinetic and molecular characterization of an extremely heat-stable carboxylesterase from the thermoacidophilic archaebacterium Sulfolobus acidocaldarius. Biochem. J. 261, 993–998. doi: 10.1042/bj2610993
Srivastava, A., Gogoi, P., Deka, B., Goswami, S., and Kanaujia, S. P. (2016). In silico analysis of 5′-UTRs highlights the prevalence of Shine-Dalgarno and leaderless-dependent mechanisms of translation initiation in bacteria and archaea, respectively. J. Theor. Biol. 402, 54–61. doi: 10.1016/j.jtbi.2016.05.005
Stracke, C., Meyer, B. H., Hagemann, A., Jo, E., Lee, A., Albers, S. V., et al. (2020). Salt stress response of Sulfolobus acidocaldarius involves complex trehalose metabolism utilizing a novel trehalose-6-phosphate synthase (TPS)/trehalose-6-phosphate phosphatase (TPP) pathway. Appl. Environ. Microbiol. 86:1565. doi: 10.1128/AEM.01565-20
Straub, C. T., Counts, J. A., Nguyen, D. M. N., Wu, C. H., Zeldes, B. M., Crosby, J. R., et al. (2018). Biotechnology of extremely thermophilic archaea. FEMS Microbiol. Rev. 42, 543–578. doi: 10.1093/femsre/fuy012
Tolstrup, N., Sensen, C. W., Garrett, R. A., and Clausen, I. G. (2000). Two different and highly organized mechanisms of translation initiation in the archaeon Sulfolobus solfataricus. Extremophiles 4, 175–179. doi: 10.1007/s007920070032
Torarinsson, E., Klenk, H. P., and Garrett, R. A. (2005). Divergent transcriptional and translational signals in Archaea. Envion Microbiol 7, 47–54. doi: 10.1111/j.1462-2920.2004.00674.x
van der Kolk, N., Wagner, A., Wagner, M., Wassmer, B., Siebers, B., and Albers, S. V. (2020). Identification of XylR, the activator of arabinose/xylose inducible regulon in Sulfolobus acidocaldarius and its application for homologous protein expression. Front. Microbiol. 11:1066. doi: 10.3389/fmicb.2020.01066
Veith, A., Klingl, A., Zolghadr, B., Lauber, K., Mentele, R., Lottspeich, F., et al. (2009). Acidianus, Sulfolobus and Metallosphaera surface layers: structure, composition and gene expression. Mol. Microbiol. 73, 58–72. doi: 10.1111/j.1365-2958.2009.06746.x
Vellanoweth, R. L., and Rabinowitz, J. C. (1992). The influence of ribosome-binding-site elements on translational efficiency in Bacillus subtilis and Escherichia coli in vivo. Mol. Microbiol. 6, 1105–1114. doi: 10.1111/j.1365-2958.1992.tb01548.x
Volkenborn, K., Kuschmierz, L., Benz, N., Lenz, P., Knapp, A., and Jaeger, K. E. (2020). The length of ribosomal binding site spacer sequence controls the production yield for intracellular and secreted proteins by Bacillus subtilis. Microb. Cell Factories 19:154. doi: 10.1186/s12934-020-01404-2
Wagner, M., van Wolferen, M., Wagner, A., Lassak, K., Meyer, B. H., Reimann, J., et al. (2012). Versatile genetic tool box for the Crenarchaeote Sulfolobus acidocaldarius. Front. Microbiol. 3:214. doi: 10.3389/fmicb.2012.00214
Wagner, M., Wagner, A., Ma, X., Kort, J. C., Ghosh, A., Rauch, B., et al. (2014). Investigation of the malE promoter and MalR, a positive regulator of the maltose regulon, for an improved expression system in Sulfolobus acidocaldarius. Appl. Environ. Microbiol. 80, 1072–1081. doi: 10.1128/AEM.03050-13
Wardleworth, B. N., Russell, R. J., Bell, S. D., Taylor, G. L., and White, M. F. (2002). Structure of Alba: an archaeal chromatin protein modulated by acetylation. EMBO J. 21, 4654–4662. doi: 10.1093/emboj/cdf465
Wen, J. D., Kuo, S. T., and Chou, H. D. (2021). The diversity of Shine-Dalgarno sequences sheds light on the evolution of translation initiation. RNA Biol. 18, 1489–1500. doi: 10.1080/15476286.2020.1861406
Wu, C. J., and Janssen, G. R. (1997). Expression of a streptomycete leaderless mRNA encoding chloramphenicol acetyltransferase in Escherichia coli. J. Bacteriol. 179, 6824–6830. doi: 10.1128/jb.179.21.6824-6830.1997
Wurtzel, O., Sapra, R., Chen, F., Zhu, Y., Simmons, B. A., and Sorek, R. (2010). A single-base resolution map of an archaeal transcriptome. Genome Res. 20, 133–141. doi: 10.1101/gr.100396.109
Yang, H., Qu, J., Zou, W., Shen, W., and Chen, X. (2021). An overview and future prospects of recombinant protein production in Bacillus subtilis. Appl. Microbiol. Biotechnol. 105, 6607–6626. doi: 10.1007/s00253-021-11533-2
Zor, T., and Selinger, Z. (1996). Linearization of the Bradford protein assay increases its sensitivity: theoretical and experimental studies. Anal. Biochem. 236, 302–308. doi: 10.1006/abio.1996.0171
Keywords: Protein expression, Archaea, Sulfolobus acidocaldarius, 5’-untranslated region, Shine-Dalgarno
Citation: Kuschmierz L, Wagner A, Schmerling C, Busche T, Kalinowski J, Bräsen C and Siebers B (2024) 5′-untranslated region sequences enhance plasmid-based protein production in Sulfolobus acidocaldarius. Front. Microbiol. 15:1443342. doi: 10.3389/fmicb.2024.1443342
Edited by:
Qunxin She, Shandong University, ChinaReviewed by:
Fabian Blombach, University College London, United KingdomQihong Huang, Shandong University, China
Copyright © 2024 Kuschmierz, Wagner, Schmerling, Busche, Kalinowski, Bräsen and Siebers. This is an open-access article distributed under the terms of the Creative Commons Attribution License (CC BY). The use, distribution or reproduction in other forums is permitted, provided the original author(s) and the copyright owner(s) are credited and that the original publication in this journal is cited, in accordance with accepted academic practice. No use, distribution or reproduction is permitted which does not comply with these terms.
*Correspondence: Laura Kuschmierz, bGF1cmEua3VzY2htaWVyekB1bmktZHVlLmRl; Bettina Siebers, YmV0dGluYS5zaWViZXJzQHVuaS1kdWUuZGU=