- 1Laboratory of mRNA and Cancer, Unit of Biomedical Research on Cancer, National Institute of Cancer (Instituto Nacional de Cancerología, INCan), Mexico City, Mexico
- 2Abteilung Mikrobiologie, Institut für Angewandte Biowissenschaften, Karlsruhe, Germany
- 3División de Biología Molecular, Instituto Potosino de Investigación Científica y Tecnológica A.C (IPICYT), San Luis Potosí, Mexico
- 4Institut für Biochemie und Molekulare Medizin (IBMM), Universität Bern, Bern, Switzerland
- 5Escuela de Medicina y Ciencias de la Salud, Tecnológico de Monterrey, Mexico City, Mexico
Introduction: Translation is a fundamental process of life. In eukaryotes, the elongation step of translation is highly conserved and is driven by eukaryotic translation elongation factors (eEF)1A and eEF2. A significant variation of the elongation is the activity of eukaryotic elongation factor (eEF) 3 in Saccharomyces cerevisiae encoded by the gene yeast elongation factor (YEF3) with orthologs in all fungal species, a few algae, and some protists. In S. cerevisiae, YEF3 is an essential gene and eEF3 plays a critical role in translation elongation, as it promotes binding of the ternary complex acylated-Transfer RNA (tRNA)—eEF1A—Guanosine-5'-triphosphate (GTP) to the aminoacyl (A) site of the ribosome, the release of uncharged tRNAs after peptide translocation, and ribosome recycling. Even though YEF3 was discovered more than 40 years ago, eEF3 has been characterized almost exclusively in S. cerevisiae.
Methods: We undertook an in vivo genetic approach to assess the functional conservation of eEF3 across phylogenetically distant fungal species.
Results: We found that eEF3 from Zygosaccharomyces rouxii and Candida glabrata (both belonging to phylum Ascomycota), Ustilago maydis (phylum Basidiomycota), and Gonapodya prolifera (phylum Monoblepharomycota), but not Aspergillus nidulans (phylum Ascomycota), supported the growth of S. cerevisiae lacking the endogenous YEF3 gene. We also proved that eEF3 is an essential gene in the ascomycetes C. glabrata and A. nidulans.
Discussion: Given that most existing knowledge on fungal translation has only been obtained from S. cerevisiae, our findings beyond this organism showed variability in the elongation process in Fungi. We also proved that eEF3 is essential in pathogenic fungi, opening the possibility of using eEF3 as a target to fight candidiasis.
Introduction
Translation plays a central role in gene expression in all forms of life (Hershey et al., 2019). The elongation step of translation consists of the decoding of a messenger ribonucleic acid's [[m]RNA] genetic information into protein by the ribosome and translation factors. In eukaryotes, this step requires the action of eukaryotic translation elongation factors (eEF) eEF1A and eEF2, as well as the nucleotide exchanger eEF1B (Dever et al., 2018). Elongation starts when an acylated-tRNA (also known as aminoacyl-tRNA, aa-tRNA) is brought to the ribosomal A site as part of the ternary complex aa-tRNA—eEF1A—GTP. Conformational changes in the ribosomal decoding center drive codon-anticodon pairing between aa-tRNAs and mRNA codons. Formation of the peptide bond is carried out at the ribosome peptidyl transferase center (PTC), between the incoming amino acid of the acylated-tRNA and the one positioned in the peptidyl (P) site (peptidyl-tRNA). Afterward, a peptide bond is formed, and eEF2 drives tRNA translocation from the A-P site to the P-E site so that the deacylated-tRNA is positioned at the exit (E) site and the peptidyl-tRNA is in the P site. GTP hydrolysis triggers eEF1A—GDP release, the uncharged tRNA leaves the exit (E) site, and the ribosome moves three nucleotides in the 5′-3′ direction to position the next codon at the empty A site. eEF1A is then recycled to its active form (eEF1A—GTP) by eEF1B. This cycle is repeated until an mRNA stop codon is placed at the free A site, which triggers peptide release from the ribosome to terminate translation. The ribosomal subunits dissociate, release the mRNA, and may enter into a new round of initiation (Choi et al., 2018; Dever et al., 2018).
A significant variation in translation elongation in eukaryotes is the requirement of eukaryotic elongation factor 3 (eEF3) in the budding yeast S. cerevisiae. During the elongation cycle, eEF3 facilitates (1) the release of deacylated-tRNAs from the ribosomal E site after peptide translocation (Ranjan et al., 2021); (2) binding of the ternary complex to the A site (Triana-Alonso et al., 1995; Andersen et al., 2006); and (3) plays a role in the recycling process, as it promotes the disassembly of post-termination complexes into their components (Kurata et al., 2010). YEF3, which encodes eEF3, is an essential gene in S. cerevisiae (Qin et al., 1990). The orthologs of YEF3, termed eEF3 genes, have been found in silico in all fungal species and some algae but not in animals, plants, or the vast majority of protists (Mateyak et al., 2016; Murina et al., 2019).
Despite its ubiquitous presence across the fungi kingdom and the fact that 48 years have passed since its discovery (Skogerson and Wakatama, 1976), YEF3 and eEF3 have been genetically, biochemically, and structurally characterized only in the yeast S. cerevisiae. The function of YEF3 orthologs in species other than S. cerevisiae has been scarcely studied, and it is not known whether the eEF3 gene is essential in different fungal species. In this study, we demonstrate that the function of YEF3 is partially conserved across phylogenetically distant fungi. We also show that eEF3 is an essential gene in Candida glabrata and Aspergillus nidulans, fungal species with enormous medical and research relevance.
Materials and methods
Databases search
We used 1,044 amino acids of the S. cerevisiae eEF3 protein to perform Basic local alignment search tool (BLAST) searches at: http://blast.ncbi.nlm.nih.gov/ and www.uniprot.org/blast/. eEF3 from the species selected for this study is listed in Table 1.
Genomic DNA extraction and plasmid construction
eEF3 DNA from Gonapodya prolifera and A. nidulans was in vitro synthesized with the preferential codon usage of S. cerevisiae into the pUC57-simple and pESC-TRP vectors, respectively, and purchased from GeneScript (Supplementary Table 1). Z. rouxii genomic DNA was purchased from the American Type Culture Collection (ATCC). Genomic DNA isolation from S. cerevisiae, C. glabrata, and Ustilago maydis was carried out using the Wizard Genomic DNA Purification Kit. The cloning for plasmid construction was achieved according to standard techniques (Sambrook and Russel, 1999).
An in-frame human influenza hemagglutinin (HA) protein tag sequence was introduced at the 3'-end of all eEF3 DNAs. Polymerase chain reaction (PCR)-amplified DNA bands were cloned into the pTZ57R/T vector to create the respective pTZ57-eEF3-HA constructs (Supplementary Table 2). Clones without artifactual mutations were further subcloned onto the p301-TRP1 vector to obtain the corresponding p301-eEF3-HA constructs. Recoded eEF3-HA DNAs (GeneScript) were subcloned from pUC57-simple onto the p301-TRP1 vector.
For pVT-U-YEF3 construction, S. cerevisiae YEF3 was PCR-amplified using genomic DNA as a template and cloned into the vector pVT-U (Vernet et al., 1987). To construct the plasmid pYC12-eEF3, C. glabrata eEF3 was PCR-amplified using genomic DNA as a template and cloned onto the vector pTZ57R/T. A DNA clone without artifactual mutations was further subcloned onto vector pYC12 (Yáñez-Carrillo et al., 2015). The C. glabrata eEF3 KO-cassette eEF3Δ::NATMX was created consisting of 1,000 nucleotides upstream (genomic left border, LB) of the eEF3 AUG start codon followed by the Nourseothricin resistance gene (NATMX) DNA and 1,000 nucleotides downstream of the eEF3 gene stop codon (genomic right border, RB; Supplementary Table 4). The eEF3 KO-cassette was further cloned into pTZ57R/T to create the plasmid pTZ57-cassette. The NATMX gene DNA was subcloned from the vector pAG25 (Goldstein and McCusker, 1999), and the different fragments of the C. glabrata eEF3 gene were PCR-amplified using genomic DNA and cloned onto the pTZ57R/T. All constructs were corroborated by Sanger sequencing. Plasmids are listed in Table 2.
Phenotypic rescue of the YEF3 deletion in S. cerevisiae
Protocols for yeast growth and gene deletion were according to Nosek and Tomaska (2013) and Xiao (2014). All strains used in this study are listed in Table 3.
Phenotypic rescue assays were performed according to Altmann et al. (1989), consisting of S. cerevisiae endogenous YEF3 replacement by eEF3 from other species. We used the haploid S. cerevisiae strains ade2, trp1, leu2, his3, and ura3 to delete using homologous recombination the gene YEF3 encoding for eEF3 with the kanamycin resistance gene (KANMX) that confers resistance to the antibiotic kanamycin. The YEF3 function is rescued by the S. cerevisiae YEF3 DNA into the vector pVT-U (Vernet et al., 1987), giving rise to the strains ade2, leu2, trp1, ura3, his3, yef3Δ::KANMX, < pVT-U-YEF3>. This strain was transformed with the constructs p301-eEF3-HA or pESC-TRP-eEF3-HA expressing eEF3 DNA from different species (Table 1) under the Gal10 promoter. Cells were grown in plates with minimal medium (yeast nitrogen base) (S) containing 2% glucose (D) supplemented with adenine (2 mg/mL), histidine (20 mg/mL), and leucine (10 mg/mL) and incubated at 30°C for 3 days. Subsequently, individual colonies were randomly selected and plated on S containing 2% galactose (to switch on the Gal10 promoter) and supplemented with adenine (2 mg/mL), histidine (20 mg/mL), and leucine (10 mg/mL). Cells were plated on the same medium supplemented with 5-Fluoroorotic acid hydrate (5-FOA; 1 g/L) and uracil (10 mg/mL) to trigger the removal of < pVT-U-YEF3> and further incubated at 30°C for 5 days.
eEF3 deletion in C. glabrata
To delete C. glabrata eEF3, C. glabrata strain ura3Δ::Tn903NeoR (Cormack and Falkow, 1999) was transformed with the pYC12-eEF3 and plated onto a minimal medium (yeast nitrogen base + glucose, SD) supplemented with a dropout lacking uracil (casamino acids, CAA; Takara Bio Inc.) as an auxotrophic marker. The resulting yeast genotype was ura3Δ::Tn903NeoR < pYC12-eEF3>. To delete the C. glabrata eEF3 gene, the C. glabrata strain ura3Δ::Tn903NeoR<pYC12-eEF3> was transformed with 600 ng of the C. glabrata eEF3 KO-cassette (which contains NATMX gene that confers resistance to nouseothricin) and grown in Yeast extract peptone dextrose (YPD) medium supplemented with the antibiotic clonNAT (200 μg/mL) to select the C. glabrata strain ura3Δ::Tn903NeoR, eEF3Δ::NATMX < pYC12-eEF3>. Several isolated colonies growing in this medium were randomly selected, and their genomic DNA was isolated. The correct deletion of the eEF3 gene by the cassette insertion was further corroborated by PCR. We obtained five colonies (numbered 4–8) of the desired genotype. Colonies were plated onto a minimal medium (SD + CAA) + uracil (growth control) or minimal medium + 5-FOA + uracil to get rid of < pYC12-eEF3>.
eEF3 deletion in A. nidulans
A. nidulans was cultured on supplemented minimal medium (MM) using standard procedures described by Hill and Käfer (2001). For the deletion of eEF3, we followed the heterokaryon-rescue method as described by Osmani et al. (2006). The flanking regions of eEF3 Open reading frame (ORF) were amplified with specific primers (Supplementary Table 4). The genomic left border (LB) was amplified with primers eEF3-P1 and P3, and the genomic RB was amplified with primers P5 and P8. The Aspergillus fumigatus pyrG gene was used as an auxotrophic marker, and it was amplified from the plasmid pFN03 by using the primers pyrG-F and pyrG-R (Osmani et al., 2006). The three DNA fragments LB, RB, and pyrG were joined together with the PCR-fusion method using nested primers P2 and P7 (Szewczyk et al., 2006). The resulting PCR product was transformed as described previously in the pyrG− auxotrophic strain GR5 (Waring et al., 1989). Transformants were screened by PCR for the homologous integration event.
Microscopy
Bright field, differential interference contrast (DIC), and fluorescence images were taken at room temperature with an Axiophot Microscope (Zeiss), Germany. For GFP imaging, spores were incubated overnight on cover slides in 0.5 ml of liquid MM supplemented with 2% glycerol (de-repression of the alcA promoter). Images were taken the next day with a 63X immersion objective. For imaging of the KO-transformants, spores were grown in liquid or solid (0.8% agarose) MM supplemented with uracil and uridine (+UU) or without uracil and uridine (–UU). Images with the bright field 10X objective were taken directly from Petri dishes. Images were collected and analyzed with the Zen Software (Zeiss), Germany.
Immunoblotting
Yeast cells were grown to O.D. = 1, pelleted, and further lysed on a frozen mortar in 100 mM KCl, 20 mM HEPES-KOH pH 7.6, 0.2 mM EDTA, 10% Glicerol, 0.1% Tritón X-100, 7 mM β-Mercaptoetanol and an EDTA-free Proteases Inhibitor Cocktail (Roche), Swiss. Protein concentration was determined using the Bradford Reagent (BioRad), United States according to the manufacturer's instructions. A volume of 20 mg of total protein lysate was resolved on 8% SDS-polyacrylamide gels and either stained with Coomassie blue (loading control) or blotted onto nitrocellulose membranes. Membranes were proved with a monoclonal anti-HA-HRP antibody (Santa Cruz, sc-7392). The expected size of proteins is shown in Supplementary Table 3.
Results
eEF3 from phylogenetically distant species cross-complements YEF3 in S. cerevisiae
We analyzed whether the function of eEF3 is conserved in distant fungal species. We retrieved YEF3 ortholog sequences from the different databases and six species were selected from across four phyla, namely Z. rouxii, Candida glabrata, and A. nidulans (all three belonging to the phylum Ascomycota), U. maydis (belonging to the phylum Basidiomycota), and G. prolifera (belonging to the phylum Monoblepharomycota; Wijayawardene et al., 2020).
Through homologous recombination and plasmid shuffling experiments, we performed phenotypic rescue assays (Altmann et al., 1989), consisting of heterologous complementation of the S. cerevisiae YEF3 plasmid with the eEF3 DNA from the species mentioned above. We generated the haploid S. cerevisiae strain Δyef3::KANMX, < pVT-U-YEF3>, in which we deleted the YEF3 gene with the KANMX gene and introduced the constitutively expressed S. cerevisiae YEF3 DNA in the vector pVT-U (Figure 1A). This strain was afterward transformed with the eEF3 DNA from the ascomycetes C. glabrata and A. rouxii, which contain an in-frame HA tag in the p301 vector (Figure 1A). In the case of A. nidulans, the eEF3 DNA with an in-frame HA tag was cloned into the high-copy pESC-TRP vector (Figure 1A). Both vectors are under the inducible galactose (Gal10) promoter. To verify the eEF3-HA protein expression, we performed Western blotting analysis of total protein extracts using an anti-HA antibody (Figure 1B). Subsequently, S-gal plates were plated in S-gal medium supplemented with 5-FOA to expel the plasmid pVT-U-YEF3. Therefore, yeast growth depends solely on the expression of p301-eEF3-HA or pSEC-TRP-eEF3-HA in the case of A. nidulans. We observed that eEF3-HA DNA from C. glabrata, Z. rouxii, and S. cerevisiae (positive control), but not the empty vector, supported the growth of S. cerevisiae lacking the endogenous YEF3 gene (Figure 1C). Although the eEF3-HA cDNA from A. nidulans was cloned in a high-copy vector, eEF3-HA was barely expressed compared to the other HA-eEF3 (Figure 1B), and the low eEF3-HA synthesized was not able to support the growth of S. cerevisiae lacking the endogenous YEF3 gene. The growth curves of the strains that rescued the function of S. cerevisiae YEF3 showed similar growth patterns (Figure 1D).
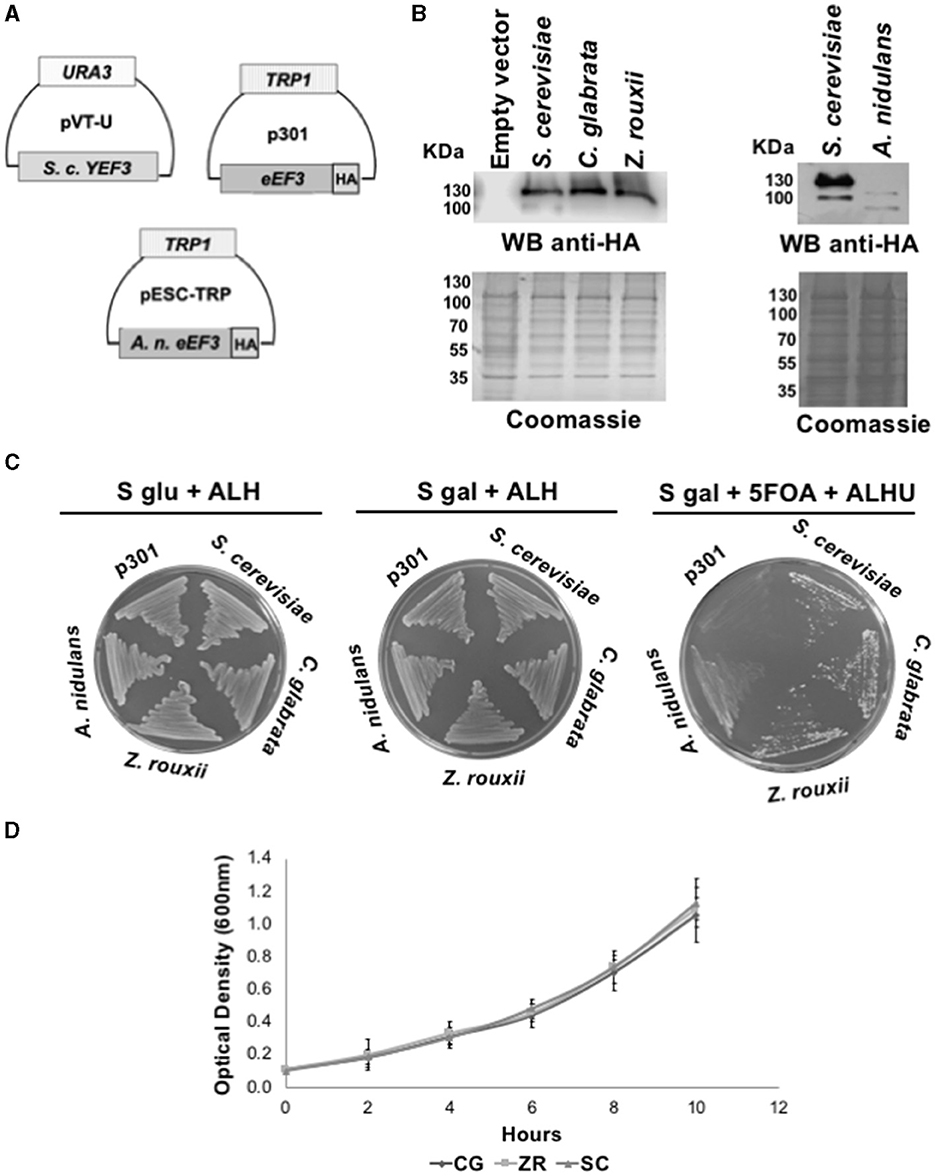
Figure 1. eEF3 from different Ascomycota species differentially complements S. cerevisiae YEF3. (A) The scheme of plasmids pVT-U-YEF3 containing the S. cerevisiae YEF3 (left), p301-eEF3-HA HA-tagged versions of eEF3 DNAs from different species used to perform the phenotypic rescues (right), and pSEC-TRP containing the A. nidulans eEF3-HA. (B) Western blotting analysis using an anti-HA antibody (up) and Coomassie blue stain of total protein extracted from different species (down). (C) Phenotypic rescue using eEF3 from Ascomycota species. Experiment controls: Empty vector (p301, negative control), S. cerevisiae (positive control). eEF3 DNAs from C. glabrata, Z. rouxii, and A. nidulans were tested. The media composition is shown. Plates S glu + ALH (p301-eEF3-HA or pSEC-TRP-eEF3-HA expression is off) and S gal + ALH (p301-eEF3-HA or pSEC-TRP-eEF3-HA expression is on) are growth controls. Plates S gal + ALHU + 5-FOA have expelled pVT-U-YEF3, and the growth relies only on p301-eEF3-HA or pSEC-TRP-eEF3-HA (Phenotypic rescue). (D) Growth curves of yeast cells expressing eEF3 from S. cerevisiae, C. glabrata, or Z. rouxii. CG, Candida glabrata; ZR, Z. rouxii; SC, Saccharomyces cerevisiae; A, Adenine; L, Leucine; H, Histidine; U, Uracil; 5-FOA, 5-fluoroorotic acid hydrate.
We next analyzed eEF3 from distant non-Ascomycota phyla using the same genetic approach and verified the expression of eEF3-HA proteins encoded by the plasmid p301-TRP1 (Figure 2A) by performing Western blotting experiments (Figure 2B). We observed that U. maydis and G. prolifera eEF3-HA also rescued the function of S. cerevisiae YEF3 (Figure 2C). The growth curves were similar for all the organisms that did complement (Figure 2D).
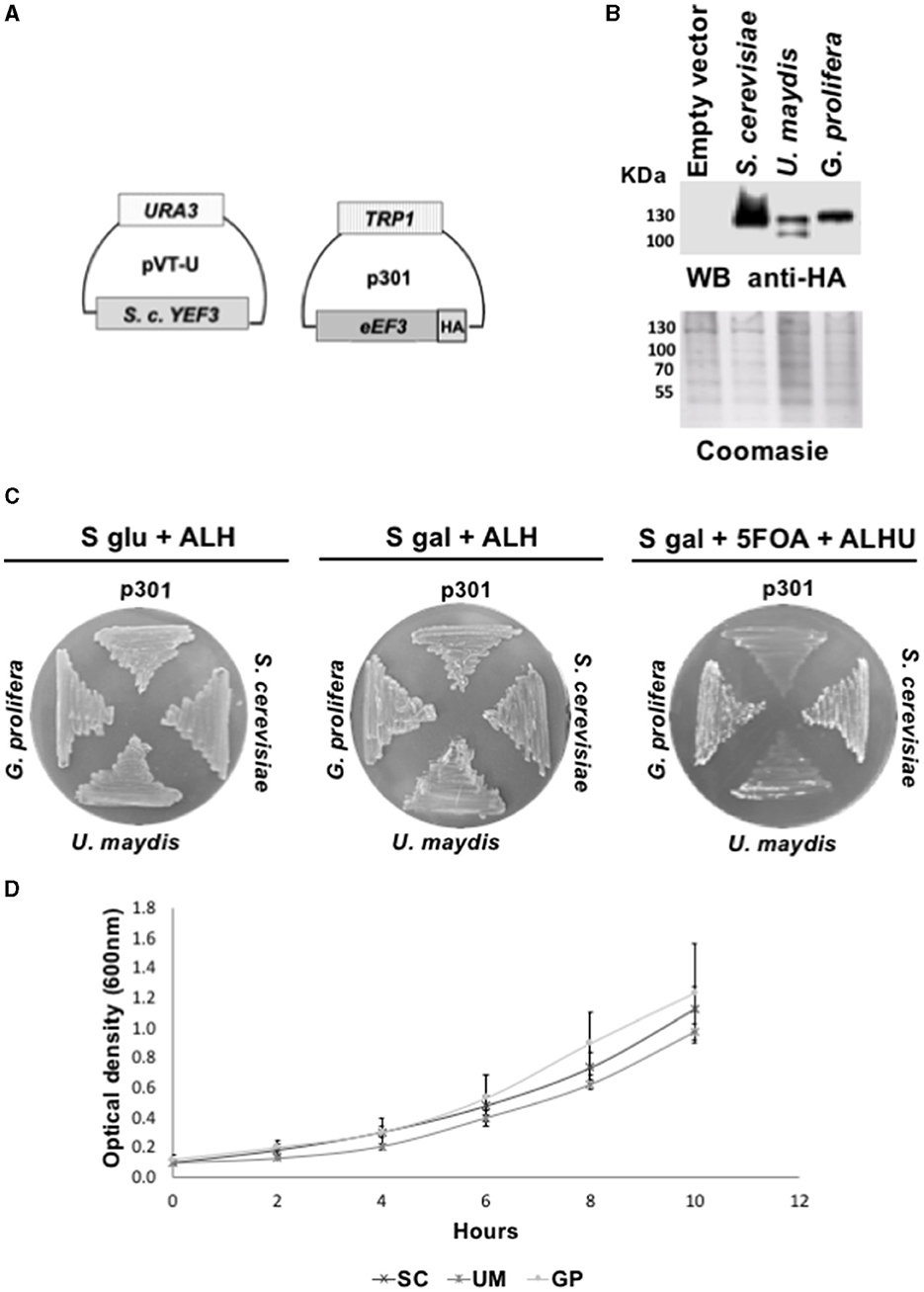
Figure 2. eEF3 from different phyla species differentially complements S. cerevisiae YEF3. (A) Scheme of plasmids pVT-U-YEF3 containing the S. cerevisiae YEF3 (left) and p301-eEF3-HA containing HA-tagged versions of eEF3 DNAs from different species used to perform the phenotypic rescues. (B) Western blotting analysis using an anti-HA antibody (up) and Coomassie blue stain of total protein extracted from different species (down). (C) Phenotypic rescue using eEF3 from species belonging to non-Ascomycota fungi. Experiment controls: Empty vector (p301, negative control), S. cerevisiae (positive control). eEF3 DNAs from the basidiomycete U. maydis and the monoblepharomycete G. prolifera were tested. The media composition is shown. Plates S glu + ALH (p301-eEF3-HA expression is off) and S gal + ALH (p301-eEF3-HA expression is on) are growth controls. Plates S gal + ALHU + 5-FOA have expelled pVT-U-YEF3, and the growth relies only on p301-eEF3-HA (Phenotypic rescue). (D) Growth curves of yeast cells expressing eEF3 from S. cerevisiae, U. maydis, or G. prolifera. UM, Ustilago maydis; GP, Gonapodya prolifera; SC, Saccharomyces cerevisiae; A, Adenine; L, Leucine; H, Histidine; U, Uracil; 5-FOA, 5-fluoroorotic acid hydrate.
eEF3 is an essential gene in C. glabrata and A. nidulans
Genetic experiments demonstrated that YEF3 is an essential gene in S. cerevisiae (Qin et al., 1990). However, whether the YEF3 orthologs are essential in other species has not been investigated. To address this question, we performed chromosomal recombination and plasmid shuffling experiments to delete eEF3 with a KO-cassette with a selection marker. Its homologous integration disrupts the original gene, which is substituted by the resistance cassette.
For C. glabrata, we constructed the eEF3 KO-cassette eEF3Δ::NATMX, consisting of the NATMX marker that confers resistance to the antibiotic cloNAT, flanked by regions of the eEF3 gene (Figure 3A, upper). Simultaneously, the C. glabrata eEF3-HA DNA in the vector pYC12, which constitutively expresses eEF3, was introduced in cells of the strain ura3Δ::Tn903NeoR, eEF3Δ::NATMX to generate the strain ura3Δ::Tn903NeoR, eEF3Δ::NATMX, < pYC12-eEF3-HA> in which we can control the lack of eEF3. To corroborate the correct homologous integration of the eEF3 KO-cassette, we amplified a fragment of DNA that spans from within NATMX to the adjacent region of this cassette. We found the integration of the cassette in several independent transformants, except in the wild-type cells (Figure 3A, lower).
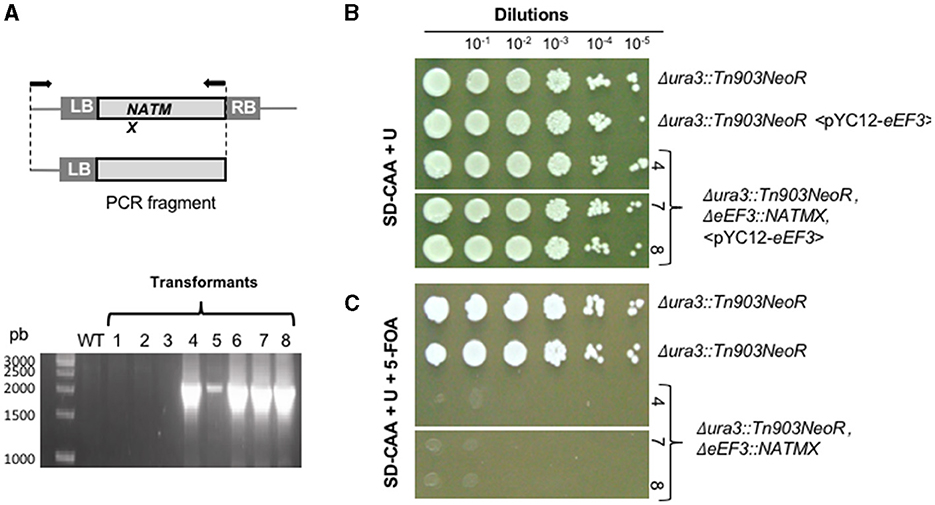
Figure 3. eEF3 is an essential gene in C. glabrata. [(A), upper] eEF3 KO-cassette was used to delete the endogenous eEF3 gene. LB, genomic left border; RB, genomic right border. A scheme of the amplified fragment of the eEF3 KO-cassette integrated onto the eEF3 locus is depicted. Arrows indicate the position of the used primers. (Lower) PCR analysis of independent KO-transformants (lanes 1–8). (B, C) KO-strains that integrated the eEF3 KO-cassette. (B) Growth controls. (C) Lethality tests. The presence of 5-FOA triggers the expulsion of the pCY12-eEF3 plasmid. The pYC12-eEF3 loss leads to lethality phenotypes only in the strain Δura3::Tn903NeoR, ΔeEF3::NATMX lacking endogenous eEF3 gene. The independent transformants 4, 7, and 8 were tested, obtaining identical results. Genotypes in all cases are described. SD-CAA, minimal medium containing casamino acids; U, uracil; 5-FOA, 5-fluoroorotic acid hydrate.
We next performed a growth assay in the presence of 5-FOA that was selected for cells that had lost the pYC12-eEF3 plasmid. Serial dilutions at a 1:10 ratio were plated onto MM supplemented with uracil (growth control; Figure 3B) and on MM containing uracil and 5-FOA (Figure 3C). Since endogenous eEF3 is deleted and the plasmid pYC12-eEF3 was expelled in the presence of 5-FOA, the absence of growth of ura3Δ::Tn903NeoR, eEF3Δ::NATMX cells in the latter medium indicates that eEF3 is an essential gene in C. glabrata (Figure 3C). We obtained identical results in three independent colonies in which a successful deletion of endogenous eEF3 was carried out.
We also assessed whether eEF3 of A. nidulans is essential. To address this, we next performed the heterokaryon rescue technique (Osmani et al., 2006) that allows the production of polynucleated protoplasts with two genetically distinct types of nuclei, i.e., one possesses the deleted gene and the other contains the wild-type allele. For this aim, we constructed an eEF3 KO-cassette that consisted of the A. fumigatus pyrG+ gene as an auxotrophic marker and flanking regions of the eEF3 open reading frame. We transformed the pyrG−− auxotrophic strain GR5 caused by the pyrG89 mutation that requires UU to grow (Waring et al., 1989) with the eEF3 KO-cassette that complements the UU auxotrophy. In this method, the homologous integration event at the eEF3 locus occurs in only one of the nuclei, and a multinucleated heterokaryon strain is created containing both wild-type and eEF3Δ nuclei. Thus, the heterokaryon grows on selective minimal media lacking (–UU) and on non-selective minimal media (+UU). The conidia are mononucleated, and the GR5 grows on +UU but not on –UU minimal media. In contrast, conidia containing the eEF3 KO-cassette can grow in selective –UU minimal media too. If the eEF3 cassette is integrated at the eEF3 locus and the deletion is lethal, the spores of the transformed conidia cannot grow in any medium.
To verify the homologous integration of the selection marker, we amplified a fragment of DNA that spans from pyrG to the adjacent region of the eEF3 KO-cassette (Figure 4A). We found homologous integration of the eEF3 KO-cassette in all the tested transformants except transformant 3. As expected, no amplification was obtained in the wild-type cells (negative control). We observed that transformant numbers 4, 7, and 9 did grow on +UU but not on –UU (Figure 4B) media, indicating that only the wild-type conidia germinated on +UU. Spores were further cultivated overnight in liquid selective –UU or non-selective +UU minimal media. Microscopic analyses showed that the eEF3Δ spores germinated in selective minimal media –UU and produced up to three germination tubes, but they could not grow further (Figure 4B inset and Figure 4C). The growth of transformants 1, 2, 5, 6, and 8 can be explained by an additional heterologous integration event of the selection marker. We conclude that eEF3 is an essential gene in A. nidulans.
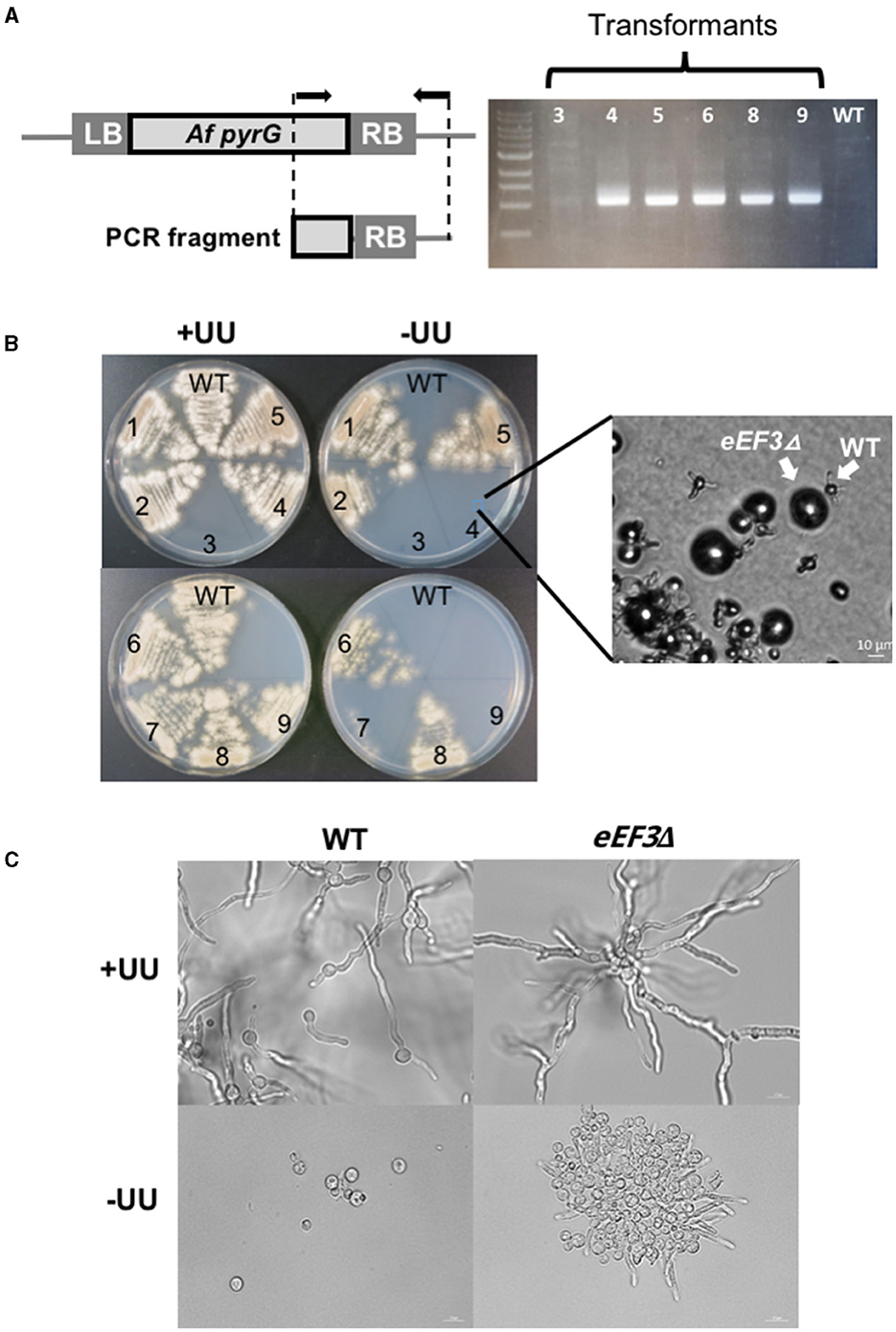
Figure 4. eEF3 is an essential gene in A. nidulans. (A) PCR analysis of the KO-strains. A scheme of the amplified A. fumigatus (Af) fragment of the KO-cassette integrated onto the eEF3 locus is depicted. Arrows indicate the position of the used primers. LB, genomic left border; RB, genomic right border (RB). (B) Spores of the wild-type strain (GR5) and several transformants with the integrated eEF3 KO-cassette were streaked out onto minimal medium (MM) plates with (+UU) or without (–UU) uracil and uridine. Inset: a microscopic picture of the spores taken directly from the Petri dish. (C) DIC microscopic pictures of the wild-type strain and transformant number 4. Spores were cultivated O/N on liquid MM with (+UU) or without (–UU) uracil and uridine at 28°C.
Discussion
In this study, we demonstrated that eEF3 from the fungal species Z. rouxii, C. glabrata, U. maydis, and G. prolifera supports the growth of S. cerevisiae lacking endogenous YEF3. We could not determine whether or not A. nidulans eEF3 complements the lack of eEF3 in S. cerevisiae. The low expression level of A. nidulans eEF3 that we systematically observed might be the result of DNA recombination, protein degradation, or both, as the protein might be harmful to S. cerevisiae. eEF3 from other Ascomycota species, including Schizosaccharomices pombe (Mateyak et al., 2018) and Candida albicans (Di Domenico et al., 1992; Myers et al., 1992), and the basidiomycete yeast Cryptococcus neoformans (Blakely et al., 2001), also cross-complement S. cerevisiae YEF3. eEF3 from a fungus-like species, namely the parasitic oomycete Phytophthora infestans, also cross-complements S. cerevisiae YEF3 (Mateyak et al., 2018).
Recent in silico studies have discovered eEF3 orthologs in various non-fungal lineages, such as green and red algae, choanoflagellates, heterokonts, dinoflagellates, cryptophytes, and oomycetes (Mateyak et al., 2016; Murina et al., 2019), and in viruses infecting the algae Chlorella (Yamada et al., 1993) and Phaeocystis (Murina et al., 2019). It would be interesting to investigate whether algal, protists, and viral eEF3 orthologs are involved in translation.
Rational design of fungicides: eEF3 in the spotlight
The biotechnological and clinical importance of fungal infections has been dramatically growing. However, the control of fungal pathogens is currently limited due to the small number of effective fungicides and the lack of suitable targets (Kim et al., 2020). Here, we have shown that eEF3 is an essential gene in C. glabrata and A. nidulans, two ascomycetes with strong medical and research relevance, respectively (Turner and Butler, 2014; Glöckner and Cornely, 2015; Park et al., 2017; Chen et al., 2021; Hernandez-Ramirez et al., 2021). This is the first study showing the essentiality of the eEF3 gene beyond S. cerevisae. Since no plant or mammalian eEF3 ortholog exists, our observations open for the first time the possibility of using rationally designed drugs to target eEF3 to fight human fungal infections by these species, as well as other infections by harmful fungal species (Sturtevant, 2002). These drugs would be an alternative to the current antifungal drugs, which are based on azoles that disrupt cell walls but display inefficient results overall.
ATP-binding cassette (ABC)-ATPases and the origin of eEF3
The evolutionary origin of eEF3 is unknown. The existence of eEF3 orthologs in fungi, algae, some protists, and a few viruses suggests that the last eukaryotic common ancestor (LECA) possessed an eEF3 gene that was later lost in some taxonomic lineages, including metazoans, land plants, and different protists (Mateyak et al., 2016; Murina et al., 2019). Accordingly, S. cerevisiae eEF1A and eEF2 alone support translation with purified rat liver ribosomes in vitro. In contrast, rat liver eEF1A and eEF2 require yeast eEF3 to drive translation with yeast ribosomes (Skogerson and Engelhardt, 1977), suggesting that mammalian ribosomes evolved to catalyze protein synthesis in the absence of eEF3 (Mateyak et al., 2016), therefore losing the eEF3 gene.
Although there is no ATPase associated with translation elongation in mammals or plants, which is driven by the GTPases eEF1A and eEF2, some ABC ATPases play key roles in translation. This is the case of ABCE1, which interacts with release factors and drives the recycling step of translation in eukaryotes. In S. cerevisiae, GCN20 mediates ribosome-associated eIF2alpha kinase GCN2 during translation elongation under amino acid starvation (Vazquez de Aldana et al., 1995; Marton et al., 1997) and ARB1 controls ribosome biogenesis (Dong et al., 2005). In mammals, ABC50 interacts with eIF2 and associates with the ribosome to promote translation initiation (Tyzack et al., 2000). In bacteria, ABCF proteins bind the ribosome upon exposure to antibiotics. Etta occupies the ribosomal E site to displace drugs from the ribosome, and both VgaA and MsrE reset peptidyl transferase activity in response to antibiotic treatment (Gerovac and Tampé, 2019). Altogether, these phenomena show that ABC ATPases involved in translation are a common theme in life, supporting the notion that LECA might have possessed eEF3 activity that was lost later in evolution in some lineages.
Evolutionary diversification of the elongation factors
The existence of eEF3 in the fungal kingdom and some protists demonstrates the diversity of factors catalyzing elongation in eukaryotes. Genome-wide studies have revealed that many unicellular lineages lack eEF1A and instead possess a related factor termed the elongation factor-like (EFL) protein, with the residues critical for eEF1A function conserved. EFL genes exist in widespread taxa, including green and red algae, some fungi, diatoms, euglenozoans, dinoflagellates, and other protists (Keeling and Inagaki, 2004; Noble et al., 2007; Kamikawa et al., 2008, 2010, 2011; Cocquyt et al., 2009; Gile et al., 2009a,b; Sakaguchi et al., 2009; Atkinson et al., 2014; Mikhailov et al., 2014). Interestingly, eEF1A and EFL genes display mutually exclusive distributions across taxa. Thus, eEF1A is not universally present in eukaryotes, and it is assumed that eEF1A and EFL are functionally equivalent (Keeling and Inagaki, 2004; Noble et al., 2007; Kamikawa et al., 2008, 2010, 2011; Cocquyt et al., 2009; Gile et al., 2009a,b; Sakaguchi et al., 2009; Mikhailov et al., 2014). Moreover, Murina et al. showed that the taxonomic distribution of eEF3 across protists is similar to that of EFL, i.e., it is present in EFL-possessing lineages and is absent in eEF1A-containing species (Murina et al., 2019).
Selenium is a trace element present in selenoproteins in the form of the amino acid selenocysteine (Sec). Most selenoproteins are enzymes containing Sec at the active site. Sec is encoded by the stop codon UGA, which is recoded and incorporated into polypeptides by the action of the Sec-specific elongation factor (eEFSec) and a specific tRNA (tRNA-Sec) as part of the Sec machinery (Labunskyy et al., 2014). Selenoproteins and the Sec machinery are essential for vertebrates and are commonly found in most algae (except red algae; Mariotti et al., 2015; Castellano, 2019; Liang et al., 2019; Jiang et al., 2020) but are missing in most insects and land plants. Selenoproteins are highly scattered throughout protists, which include many lineages lacking selenoproteins (Mariotti et al., 2015; Castellano, 2019). Sec was also lost at the root of Dikarya, which comprises the fungal phyla Ascomycota and Basidiomycota (Castellano, 2019; Mariotti et al., 2019). The Sec machinery genes were identified in nine fungal species belonging to three early-branching fungal groups, namely Chytridiomycota, Zoopagomycota, and Mucoromycota (Castellano, 2019; Mariotti et al., 2019).
Overall, the studies on eEF3, eEF1A, EFL, and eEFSec have unveiled a significant diversification of the translation elongation factors across eukaryotes.
Conclusion
The investigation of eEF3 across species within the fungal kingdom is crucial, not only because it shows variability in the translational process but also because of its potential pharmaceutical and genetic research implications. Discoveries in this area not only contribute to the understanding of fundamental factors necessary for translation but also illuminate the processes by which such knowledge can be harnessed for the development of more effective products and technologies. Given that a significant portion of existing knowledge has only been obtained from S. cerevisiae, our study beyond this model organism revealed that eEF3 is essential in pathogenic fungi. This discovery holds promise for a breakthrough in antifungal research as this protein, absent in mammals and plants, could serve as a remarkably specific target.
Data availability statement
The datasets presented in this study can be found in online repositories. The names of the repository/repositories and accession number(s) can be found in the article/Supplementary material.
Author contributions
GM: Conceptualization, Data curation, Formal analysis, Investigation, Methodology, Software, Validation, Writing – review & editing, Supervision, Writing – original draft. AG: Conceptualization, Data curation, Formal analysis, Investigation, Methodology, Software, Validation, Writing – review & editing. SH: Conceptualization, Data curation, Formal analysis, Investigation, Methodology, Validation, Writing – review & editing. IC: Data curation, Methodology, Resources, Validation, Writing – review & editing. MA: Data curation, Formal analysis, Investigation, Methodology, Resources, Supervision, Validation, Writing – review & editing. RF: Data curation, Formal analysis, Investigation, Methodology, Resources, Supervision, Validation, Writing – review & editing. GH: Conceptualization, Data curation, Formal analysis, Funding acquisition, Investigation, Methodology, Project administration, Resources, Supervision, Validation, Visualization, Writing – original draft, Writing – review & editing.
Funding
The author(s) declare financial support was received for the research, authorship, and/or publication of this article. GH was supported by the intramural funding program of the National Institute of Cancer (Instituto Nacional de Cancerología, Mexico). CONAHCyT Ph. D. fellowships were awarded to AG (No. 436200) and GM (No. 749487). The study on A. nidulans was supported by Deutsche Forschungsgemeinschaft (DFG) Fi459/19-2. The C. glabrata study was funded in part by CONAHCYT Grant No. CF-2019-610281 to IC.
Acknowledgments
We thank Aysha H. Osmani and Hans Trachsel for plasmids. This study is part of the AG and GM curricula in the Ph.D. Program in Biological Sciences (AG) and Ph.D. Program in Biochemical Sciences (GM; Doctorado en Ciencias Biológicas y Doctorado en Ciencias Bioquímicas), respectively, UNAM, Mexico. We also thank the reviewers for their insightful criticism and valuable comments on the manuscript.
Conflict of interest
The C. glabrata strain ura3Δ::KANMX, eEF3Δ::NATMX<pYC12-eEF3> is claimed by the patent MX/a/2019/000406, application file MX/E/2019/001890, 10th January of 2019, property of Instituto Nacional de Cancerología, Mexico.
GH declared that he was an editorial board member of Frontiers, at the time of submission. This had no impact on the peer review process and the final decision.
The remaining authors declare that the research was conducted in the absence of any commercial or financial relationships that could be construed as a potential conflict of interest.
Publisher's note
All claims expressed in this article are solely those of the authors and do not necessarily represent those of their affiliated organizations, or those of the publisher, the editors and the reviewers. Any product that may be evaluated in this article, or claim that may be made by its manufacturer, is not guaranteed or endorsed by the publisher.
Supplementary material
The Supplementary Material for this article can be found online at: https://www.frontiersin.org/articles/10.3389/fmicb.2024.1438900/full#supplementary-material
References
Altmann, M., Müller, P. P., Pelletier, J., Sonenberg, N., and Trachsel, H. (1989). A mammalian translation initiation factor can substitute for its yeast homologue in vivo. J. Biol. Chem. 264, 12145–12147. doi: 10.1016/S0021-9258(18)63833-5
Andersen, C. B., Becker, T., Blau, M., Anand, M., Halic, M., Balar, B., et al. (2006). Structure of eEF3 and the mechanism of transfer RNA release from the E-site. Nature 443, 663–668. doi: 10.1038/nature05126
Atkinson, G. C., Kuzmenko, A., Chicherin, I., Soosaar, A., Tenson, T., Carr, M., et al. (2014). An evolutionary ratchet leading to loss of elongation factors in eukaryotes. BMC Evol. Biol. 14:35. doi: 10.1186/1471-2148-14-35
Blakely, G., Hekman, J., Chakraburtty, K., and Williamson, P. R. (2001). Evolutionary divergence of an elongation factor 3 from Cryptococcus neoformans. J. Bacteriol. 183, 2241–2248. doi: 10.1128/JB.183.7.2241-2248.2001
Castellano, S. (2019). Selenium strikes back at fungi. Nat. Microbiol. 4, 726–727. doi: 10.1038/s41564-019-0434-5
Chen, A., Mao, X., Sun, Q., Wei, Z., Li, J., You, Y., et al. (2021). Alternaria mycotoxins: an overview of toxicity, metabolism, and analysis in food. J. Agricult. Food Chem. 69, 7817–7830. doi: 10.1021/acs.jafc.1c03007
Choi, J., Grosely, R., Prabhakar, A., Lapointe, C. P., Wang, J., and Puglisi, J. D. (2018). How messenger RNA and nascent chain sequences regulate translation elongation. Annu. Rev. Biochem. 87, 421–449. doi: 10.1146/annurev-biochem-060815-014818
Cocquyt, E., Verbruggen, H., Leliaert, F., Zechman, F. W., Sabbe, K., and De Clerck, O. (2009). Gain and loss of elongation factor genes in green algae. BMC Evol. Biol. 9:39. doi: 10.1186/1471-2148-9-39
Cormack, B. P., and Falkow, S. (1999). Efficient homologous and illegitimate recombination in the opportunistic yeast pathogen Candida glabrata. Genetics 151, 979–987. doi: 10.1093/genetics/151.3.979
Dever, T. E., Dinman, J. D., and Green, R. (2018). Translation elongation and recoding in eukaryotes. Cold Spring Harb. Persp. Biol. 10:a032649. doi: 10.1101/cshperspect.a032649
Di Domenico, B. J., Lupisella, J., Sandbaken, M., and Chakraburtty, K. (1992). Isolation and sequence analysis of the gene encoding translation elongation factor 3 from Candida albicans. Yeast 8, 337–352. doi: 10.1002/yea.320080502
Dong, J., Lai, R., Jennings, J. L., Link, A. J., and Hinnebusch, A. G. (2005). The novel ATP-binding cassette protein ARB1 is a shuttling factor that stimulates 40S and 60S ribosome biogenesis. Mol. Cell. Biol. 25, 9859–9873. doi: 10.1128/MCB.25.22.9859-9873.2005
Efimov, V. P., Zhang, J., and Xiang, X. (2006). CLIP-170 homologue and NUDE play overlapping roles in NUDF localization in Aspergillus nidulans. Mol. Biol. Cell, 17, 2021–2034. doi: 10.1091/mbc.e05-11-1084
Gerovac, M., and Tampé, R. (2019). Control of mRNA translation by versatile ATP-driven machines. Trends Biochem. Sci. 44, 167–180. doi: 10.1016/j.tibs.2018.11.003
Gile, G. H., Faktorová, D., Castlejohn, C. A., Burger, G., Lang, B. F., Farmer, M. A., et al. (2009a). Distribution and phylogeny of EFL and EF-1alpha in Euglenozoa suggest ancestral co-occurrence followed by differential loss. PLoS ONE 4:e5162. doi: 10.1371/journal.pone.0005162
Gile, G. H., Novis, P. M., Cragg, D. S., Zuccarello, G. C., and Keeling, P. J. (2009b). The distribution of Elongation Factor-1 Alpha (EF-1alpha), Elongation Factor-Like (EFL), and a non-canonical genetic code in the ulvophyceae: discrete genetic characters support a consistent phylogenetic framework. J. Eukaryotic Microbiol. 56, 367–372. doi: 10.1111/j.1550-7408.2009.00411.x
Glöckner, A., and Cornely, O. A. (2015). Candida glabrata–unique features and challenges in the clinical management of invasive infections. Mycoses 58, 445–450. doi: 10.1111/myc.12348
Goldstein, A. L., and McCusker, J. H. (1999). Three new dominant drug resistance cassettes for gene disruption in Saccharomyces cerevisiae. Yeast 15, 1541–1553. doi: 10.1002/(SICI)1097-0061(199910)15:14<1541::AID-YEA476>3.0.CO;2-K
Hernandez-Ramirez, G., Barber, D., Tome-Amat, J., Garrido-Arandia, M., and Diaz-Perales, A. (2021). Alternaria as an inducer of allergic sensitization. J. Fungi 7:838. doi: 10.3390/jof7100838
Hershey, J. W. B., Sonenberg, N., and Mathews, M. B. (2019). Principles of translational control. Cold Spring Harb. Persp. Biol. 11:a032607. doi: 10.1101/cshperspect.a032607
Hill, T. W., and Käfer, E. (2001). Improved protocols for Aspergillus minimal medium: trace element and minimal medium salt stock solutions. Fungal Genetics Rep. 48, 20–21. doi: 10.4148/1941-4765.1173
Jiang, L., Lu, Y., Zheng, L., Li, G., Chen, L., Zhang, M., et al. (2020). The algal selenoproteomes. BMC Genom. 21:699. doi: 10.1186/s12864-020-07101-z
Kamikawa, R., Inagaki, Y., and Sako, Y. (2008). Direct phylogenetic evidence for lateral transfer of elongation factor-like gene. Proc. Natl. Acad. Sci. U. S. A. 105, 6965–6969. doi: 10.1073/pnas.0711084105
Kamikawa, R., Sakaguchi, M., Matsumoto, T., Hashimoto, T., and Inagaki, Y. (2010). Rooting for the root of elongation factor-like protein phylogeny. Mol. Phylogen. Evol. 56, 1082–1088. doi: 10.1016/j.ympev.2010.04.040
Kamikawa, R., Yabuki, A., Nakayama, T., Ishida, K., Hashimoto, T., and Inagaki, Y. (2011). Cercozoa comprises both EF-1α-containing and EFL-containing members. Eur. J. Protistol. 47, 24–28. doi: 10.1016/j.ejop.2010.08.002
Keeling, P. J., and Inagaki, Y. (2004). A class of eukaryotic GTPase with a punctate distribution suggesting multiple functional replacements of translation elongation factor 1alpha. Proc. Natl. Acad. Sci. U. S. A. 101, 15380–15385. doi: 10.1073/pnas.0404505101
Kim, J. H., Cheng, L. W., Chan, K. L., Tam, C. C., Mahoney, N., Friedman, M., et al. (2020). Antifungal drug repurposing. Antibiotics 9:812. doi: 10.3390/antibiotics9110812
Kurata, S., Nielsen, K. H., Mitchell, S. F., Lorsch, J. R., Kaji, A., and Kaji, H. (2010). Ribosome recycling step in yeast cytoplasmic protein synthesis is catalyzed by eEF3 and ATP. Proc. Natl. Acad. Sci. U. S. A. 107, 10854–10859. doi: 10.1073/pnas.1006247107
Labunskyy, V. M., Hatfield, D. L., and Gladyshev, V. N. (2014). Selenoproteins: molecular pathways and physiological roles. Physiol. Rev. 94, 739–777. doi: 10.1152/physrev.00039.2013
Liang, H., Wei, T., Xu, Y., Li, L., Kumar Sahu, S., Wang, H., et al. (2019). Phylogenomics provides new insights into gains and losses of selenoproteins among Archaeplastida. Int. J. Mol. Sci. 20:3020. doi: 10.3390/ijms20123020
Mariotti, M., Salinas, G., Gabaldón, T., and Gladyshev, V. N. (2019). Utilization of selenocysteine in early-branching fungal phyla. Nat. Microbiol. 4, 759–765. doi: 10.1038/s41564-018-0354-9
Mariotti, M., Santesmasses, D., Capella-Gutierrez, S., Mateo, A., Arnan, C., Johnson, R., et al. (2015). Evolution of selenophosphate synthetases: emergence and relocation of function through independent duplications and recurrent subfunctionalization. Genome Res. 25, 1256–1267. doi: 10.1101/gr.190538.115
Marton, M. J., Vazquez de Aldana, C. R., Qiu, H., Chakraburtty, K., and Hinnebusch, A. G. (1997). Evidence that GCN1 and GCN20, translational regulators of GCN4, function on elongating ribosomes in activation of eIF2alpha kinase GCN2. Mol. Cell. Biol. 17, 4474–4489. doi: 10.1128/MCB.17.8.4474
Mateyak, M., Sasikumar, A. N., Dunaway, S., and Kinzy, T. (2016). “The unique evolutionary distribution of translation elongation factor 3,” in Evolution of the Protein Synthesis Machinery and Its Regulation, eds G. Hernández and R. Jagus (Cham: Springer), 313–326.
Mateyak, M. K., Pupek, J. K., Garino, A. E., Knapp, M. C., Colmer, S. F., Kinzy, T. G., et al. (2018). Demonstration of translation elongation factor 3 activity from a non-fungal species, Phytophthora infestans. PLoS ONE 13:e0190524. doi: 10.1371/journal.pone.0190524
Mikhailov, K. V., Janouškovec, J., Tikhonenkov, D. V., Mirzaeva, G. S., Diakin, A. Y., Simdyanov, T. G., et al. (2014). A complex distribution of elongation family GTPases EF1A and EFL in basal alveolate lineages. Genome Biol. Evol. 6, 2361–2367. doi: 10.1093/gbe/evu186
Murina, V., Kasari, M., Takada, H., Hinnu, M., Saha, C. K., Grimshaw, J. W., et al. (2019). ABCF ATPases involved in protein synthesis, ribosome assembly and antibiotic resistance: structural and functional diversification across the tree of life. J. Mol. Biol. 431, 3568–3590. doi: 10.1016/j.jmb.2018.12.013
Myers, K. K., Fonzi, W. A., and Sypherd, P. S. (1992). Isolation and sequence analysis of the gene for translation elongation factor 3 from Candida albicans. Nucleic Acids Res. 20, 1705-1710. doi: 10.1093/nar/20.7.1705
Nayak, T., Szewczyk, E., Oakley, C. E., Osmani, A., Ukil, L., Murray, S. L., et al. (2006). A versatile and efficient gene-targeting system for Aspergillus nidulans. Genetics 172, 1557–1566. doi: 10.1534/genetics.105.052563
Noble, G. P., Rogers, M. B., and Keeling, P. J. (2007). Complex distribution of EFL and EF-1alpha proteins in the green algal lineage. BMC Evol. Biol. 7:82. doi: 10.1186/1471-2148-7-82
Nosek, J., and Tomaska, L. (2013). Laboratory protocols in molecular and cell biology of yeast, 1st Edn. Bratislava: CreateSpace Independent Publishing Platform.
Osmani, A. H., Oakley, B. R., and Osmani, S. A. (2006). Identification and analysis of essential Aspergillus nidulans genes using the heterokaryon rescue technique. Nat. Protocols 1, 2517–2526. doi: 10.1038/nprot.2006.406
Park, H. S., Jun, S. C., Han, K. H., Hong, S. B., and Yu, J. H. (2017). Diversity, application, and synthetic biology of industrially important Aspergillus fungi. Adv. Appl. Microbiol. 100, 161–202. doi: 10.1016/bs.aambs.2017.03.001
Qin, S. L., Xie, A. G., Bonato, M. C., and McLaughlin, C. S. (1990). Sequence analysis of the translational elongation factor 3 from Saccharomyces cerevisiae. J. Biol. Chem. 265, 1903–1912. doi: 10.1016/S0021-9258(19)39916-8
Ranjan, N., Pochopien, A. A., Chih-Chien Wu, C., Beckert, B., Blanchet, S., Green, R., et al. (2021). Yeast translation elongation factor eEF3 promotes late stages of tRNA translocation. EMBO J. 40:e106449. doi: 10.15252/embj.2020106449
Sakaguchi, M., Takishita, K., Matsumoto, T., Hashimoto, T., and Inagaki, Y. (2009). Tracing back EFL gene evolution in the cryptomonads-haptophytes assemblage: separate origins of EFL genes in haptophytes, photosynthetic cryptomonads, and goniomonads. Gene 441, 126–131. doi: 10.1016/j.gene.2008.05.010
Sambrook, J., and Russel, D. W. (1999). Molecular Cloning: a Laboratory Manual. Cold Spring Harbor, NY: Cold Spring Harbor Laboratory Press.
Skogerson, L., and Engelhardt, D. (1977). Dissimilarity in protein chain elongation factor requirements between yeast and rat liver ribosomes. J. Biol. Chem. 252, 1471–1475. doi: 10.1016/S0021-9258(17)40680-6
Skogerson, L., and Wakatama, E. (1976). A ribosome-dependent GTPase from yeast distinct from elongation factor 2. Proc. Natl. Acad. Sci. U. S. A. 73, 73–76. doi: 10.1073/pnas.73.1.73
Sturtevant, J. (2002). Translation elongation-3-like factors: are they rational antifungal targets? Expert Op. Therap. Targets 6, 545–553. doi: 10.1517/14728222.6.5.545
Szewczyk, E., Nayak, T., Oakley, C. E., Edgerton, H., Xiong, Y., Taheri-Talesh, N., et al. (2006). Fusion PCR and gene targeting in Aspergillus nidulans. Nat. Protocols 1, 3111–3120. doi: 10.1038/nprot.2006.405
Triana-Alonso, F. J., Chakraburtty, K., and Nierhaus, K. H. (1995). The elongation factor 3 unique in higher fungi and essential for protein biosynthesis is an E site factor. J. Biol. Chem. 270, 20473–20478. doi: 10.1074/jbc.270.35.20473
Turner, S. A., and Butler, G. (2014). The Candida pathogenic species complex. Cold Spring Harb. Perspect. Med. 4:a019778. doi: 10.1101/cshperspect.a019778
Tyzack, J. K., Wang, X., Belsham, G. J., and Proud, C. G. (2000). ABC50 interacts with eukaryotic initiation factor 2 and associates with the ribosome in an ATP-dependent manner. J. Biol. Chem. 275, 34131–34139. doi: 10.1074/jbc.M002868200
Vazquez de Aldana, C. R., Marton, M. J., and Hinnebusch, A. G. (1995). GCN20, a novel ATP binding cassette protein, and GCN1 reside in a complex that mediates activation of the eIF-2 alpha kinase GCN2 in amino acid-starved cells. EMBO J. 14, 3184–3199. doi: 10.1002/j.1460-2075.1995.tb07321.x
Vernet, T., Dignard, D., and Thomas, D. Y. (1987). A family of yeast expression vectors containing the phage f1 intergenic region. Gene 52, 225–233. doi: 10.1016/0378-1119(87)90049-7
Waring, R. B., May, G. S., and Morris, N. R. (1989). Characterization of an inducible expression system in Aspergillus nidulans using alcA and tubulin-coding genes. Gene 79, 119–130. doi: 10.1016/0378-1119(89)90097-8
Wijayawardene, N. N., Hyde, K. D., Al-Ani, L. K. T., Tedersoo, L., Haelewaters, D., Rajeshkumar, K., et al. (2020). Outline of Fungi and fungus-like taxa. Mycosphere 11, 1060–1456. doi: 10.5943/mycosphere/11/1/8
Yamada, T., Fukuda, T., Tamura, K., Furukawa, S., and Songsri, P. (1993). Expression of the gene encoding a translational elongation factor 3 homolog of Chlorella virus CVK2. Virology 197, 742–750. doi: 10.1006/viro.1993.1650
Keywords: YEF3, eEF3, translation elongation, translation evolution, fungal translation, RNA metabolism
Citation: Maldonado G, García A, Herrero S, Castaño I, Altmann M, Fischer R and Hernández G (2024) The gene YEF3 function encoding translation elongation factor eEF3 is partially conserved across fungi. Front. Microbiol. 15:1438900. doi: 10.3389/fmicb.2024.1438900
Received: 26 May 2024; Accepted: 30 July 2024;
Published: 23 August 2024.
Edited by:
Christoph Engl, Queen Mary University of London, United KingdomReviewed by:
Vigyasa Singh, University of Arizona, United StatesPablo Smircich, Instituto de Investigaciones Biológicas Clemente Estable (IIBCE), Uruguay
Copyright © 2024 Maldonado, García, Herrero, Castaño, Altmann, Fischer and Hernández. This is an open-access article distributed under the terms of the Creative Commons Attribution License (CC BY). The use, distribution or reproduction in other forums is permitted, provided the original author(s) and the copyright owner(s) are credited and that the original publication in this journal is cited, in accordance with accepted academic practice. No use, distribution or reproduction is permitted which does not comply with these terms.
*Correspondence: Greco Hernández, Z2hlcm5hbmRlenJAaW5jYW4uZWR1Lm14; Z3JlY28uaGVybmFuZGV6QHRlYy5teA==
†These authors have contributed equally to this work