- 1Research Center, Beijing Hanmi Pharmaceutical Co., Ltd., Beijing, China
- 2Food & Biotech R&D Center, Coree Beijing Co., Ltd., Beijing, China
The global prevalence of obesity is rising year by year, which has become a public health problem worldwide. Many animal and clinical studies have shown that Lactiplantibacillus plantarum is considered an ideal probiotic and potential supplement for the treatment of obesity. In this study, we aimed to complete the genome sequence of L. plantarum HOM2217, which was isolated from human milk, and study its physiological characteristics and anti-obesity effects in 3T3-L1 cells and rats fed a high-fat diet (HFD) to determine its potential as a starter for functional food products. Whole-genome analysis demonstrated that HOM2217 contained a single circular chromosome of 3,267,529 bp with a GC content of 44.5% and one plasmid (62,350 bp) with a GC content of 38.5%. Compared to the reference strains, HOM2217 demonstrated superior tolerance to gastrointestinal conditions, higher adhesion to intestinal epithelial cell lines, potent antimicrobial activity against Enterobacter cloacae ATCC 13047, and effective cholesterol removal ability in vitro. Treatment with heat-killed HOM2217 significantly reduced lipid accumulation and intracellular triglyceride production in 3T3-L1 adipocytes. Daily treatment of HFD-fed rats with HOM2217 for 7 weeks decreased body weight, body weight gain, and body fat without changes in food intake. HOM2217 also significantly increased the serum high-density lipoprotein cholesterol (HDL-C) level, decreased the serum tumor necrosis factor (TNF-α) and increased short-chain fatty acid (SCFA) (formic acid, acetic acid, and butyric acid) levels in the cecum. Thus, HOM2217 could potentially prevent obesity in rats by inhibiting inflammatory responses and regulating lipid metabolism and SCFAs expression. Therefore, HOM2217 has potential as an alternative treatment for obesity.
1 Introduction
According to the World Health Organization, overweight and obesity are defined as abnormal or excessive fat accumulation that poses health risks. A body mass index (BMI) > 25 is considered overweight, and > 30 obese (WHO, 1997). In 2022, 2.5 billion adults aged 18 years and older were overweight, including over 890 million adults who were living with obesity (Phelps et al., 2024). Obesity has now reached epidemic proportions and it is estimated that by 2030, more than one billion adults worldwide will become obese (World Obesity Federation, 2022). Obesity is classified as a low-grade chronic and systemic inflammatory disease that results from complex interactions between genes and environmental factors and leads or relates to many diseases such as type 2 diabetes (Qin et al., 2020; Yu et al., 2022), nonalcoholic fatty liver disease (Quek et al., 2023), cardiovascular disease (Dwivedi et al., 2020; Opio et al., 2020; Mohammadian Khonsari et al., 2022), chronic kidney disease (Kjaergaard et al., 2022), thyroid disorders (Song et al., 2019), COVID-19 (Hussain et al., 2020; Raeisi et al., 2022), asthma (Scott et al., 2023), obstructive sleep apnea (Ghavami et al., 2023), stroke (Wang et al., 2022), osteoarthritis (Ortolan et al., 2021), and cancer (Petrelli et al., 2021; Lee et al., 2022). Moreover, it affects the quality of life. Currently, methods to treat obesity mainly include dietary regulation, exercise, drug administration, and bariatric surgery (American College of Cardiology/American Heart Association Task Force on Practice Guidelines, Obesity Expert Panel, 2013, 2014; Apovian et al., 2015; Garvey et al., 2016; Reid and Korner, 2022). Pharmacotherapy is indicated as an adjunct to a reduced-calorie diet and increased activity for long-term weight management (Yanovski and Yanovski, 2014; Apovian et al., 2015). However, some drugs may increase the incidence of gastroparesis (Wilding et al., 2021) and pulse rate (Pi-Sunyer et al., 2015) or attenuate the expected blood pressure reduction (Pi-Sunyer et al., 2015). Although Semaglutide, the latest breakthrough in anti-obesity medication, represents a significant advancement in obesity treatment, it also carries common side effects such as nausea, vomiting, diarrhea, constipation, injection site reactions, along with rare potential risks of thyroid tumors and pancreatitis (Wilding et al., 2021).
Obesity and its association with other chronic noncommunicable diseases are not only from genetic factors, eating habits, or lack of physical activity but also from the gut microbiota as an environmental factor (Alvarez-Arrano and Martin-Pelaez, 2021). Obesity is associated with perturbation of the gut microbiome (Ley et al., 2006), which affects metabolic function and energy homeostasis (Guirro et al., 2019) by affecting energy use, nutrient metabolism, inflammatory pathways, and the gut-brain axis (Rawat et al., 2023). Therefore, novel therapies targeting the gut microbiome, such as fecal microbiota transplantation and supplementation with probiotics and prebiotics, are potential treatments for obesity (Asadi et al., 2022). The consensus definition of probiotics is: “live microorganisms that, when administered in adequate amounts, confer a health benefit on the host” (Hill et al., 2014). Probiotics exert health effects via a variety of mechanisms, including the modulation of immune function, production of organic acids and antimicrobial compounds, interaction with resident microbiota, interfacing with the host, and improving gut barrier integrity and enzyme production (Sanders et al., 2019). Currently, members of the genera Lactobacillus and Bifidobacterium are primarily used.
Lactiplantibacillus plantarum, previously known as Lactobacillus plantarum, an important member of Lactobacillus, is a well-studied and versatile species with beneficial effects, such as promoting host immunity (Zhao et al., 2021), reducing atopic dermatitis (Prakoeswa et al., 2017), improving functional diarrhea (Jung et al., 2022) and irritable bowel syndrome symptom severity (Martoni et al., 2023), conferring antioxidant, anti-cholesterolemic and anti-glycemic effect (Oh et al., 2021; Stsepetova et al., 2023). It has a long tradition of safe use and enduring relationship with humans. In clinical studies, L. plantarum has been shown to have effective probiotic anti-obesity effects (Park et al., 2020; Rahayu et al., 2021; Sohn et al., 2023). However accumulating evidence indicates that probiotic effects may vary in a strain-and dose-specific manner (McFarland et al., 2018; Ji et al., 2019).
Therefore, in this study, we isolated the specific HOM2217 strain from human milk, analyzed its complete genome sequence, and evaluated its tolerance to gastrointestinal conditions (acid and bile), adhesion to the gastrointestinal epithelium, prevention of obesity, and cholesterol-lowering ability. We investigated its potential anti-obesity activity in 3T3-L1 cells and its effects on body weight, body fat, and serum lipid and cytokine concentrations in rats for the development of new functional food products.
2 Materials and methods
2.1 Bacterial strains and culture conditions
HOM2217 was isolated from human milk and deposited at the China General Microbiological Culture Collection Center (CGMCC; No. 25683). Enterobacter cloacae ATCC 13047, Lactocaseibacillus rhamnosus ATCC 53103 (GG), and L. plantarum ATCC 14917 were purchased from the American Type Culture Collection (ATCC) and used as reference strains for comparison of phenotypic properties. HOM2217, L. plantarum ATCC 14917, and L. rhamnosus ATCC 53103 (GG) were grown in de Man, Rogosa, and Sharpe (MRS) broth (OXOID, USA) and E. cloacae ATCC 13047 was grown in Tryptone Soya Broth (OXOID, USA) at 37°C for 20 h aerobically freshly before use. Lyophilized HOM2217 powder was used in animal experiments. First, it was fermented in a 30-L fermentation tank at 37°C at constant pH 5.5 and a stirring speed of 50 rpm for 20 h. Then, the cells were harvested by centrifugation at 10,000 × g, 4°C for 10 min, washed twice with sterile saline solution, resuspended in cytoprotectant, lyophilized for 48 h, and stored at −20°C until use. The lyophilized HOM2217 powder was freshly suspended in sterile saline solution daily for administration to animals.
2.2 Whole genome and comparative analysis
The HOM2217 genome was sequenced using the Illumina II and PacBio Sequel III platforms (Majorbio, Shanghai, China). The bacterial genome completion map was assembled de novo using Unicycler v0.4.8 (Wick et al., 2017) and error-corrected using Pilon v1.22. Gene coding sequences (CDS), tRNA, rRNA, and sRNA were predicted using Glimmer v3.02 (Delcher et al., 2007), GeneMarkS v4.3 (Besemer and Borodovsky, 2005), tRNAscan-SE v2.0 (Chan and Lowe, 2019), Barrnap v0.9, and Infernal v1.1.4, respectively. Genome visualization was performed using Circos v0.69.6 (Stothard and Wishart, 2005). The corresponding function annotation was completed by blasting genes against the Cluster of Orthologous Groups of proteins (COG) database (Jensen et al., 2008). The complete genome of HOM2217 was submitted to GenBank (GenBank accession number: CP152360.1).
For comparative genomic analysis, the genome sequences of nine L. plantarum strains [Dad-13 (GCA_023547165.1), LMT1-48 (CP033888.1), UBLP-40 (GCA_003692725.1), P-8 (CP005942.1), Q180 (CP073753.1), HAC01 (CP029349.1), EM (CP037429.1), 299v (GCA_001888735.1), and ATCC 14917 (GCA_000143745.1)] were obtained from the NCBI database and used as references. An orthologous gene set was built to identify the core genome and pan-genome sizes using OrthoMCL package v2.0.9 (Li et al., 2003). A maximum-likelihood (ML) tree with bootstrap values (1,000 replications) based on the concatenated nucleotide sequences of homologous single-copy genes was constructed using MEGA7 software (Kumar et al., 2016). The average nucleotide identity of the genome sequence between HOM2217 and other reference strains was evaluated using the orthologous average nucleotide identity (Ortho ANI) tool (Zhang et al., 2019).
2.3 Survival under simulated gastrointestinal tract conditions
Tolerance to gastrointestinal tract (GIT) conditions was evaluated using simulated gastric and intestinal juices based on the methods described by Kang et al. (2020) and Zhang et al. (2022), with some modifications. Briefly, 1 mL of the 20-h culture was harvested by centrifugation at 10,000 × g, 4°C for 10 min, and the cells were washed twice with phosphate buffer solution (PBS, Thermo Fisher, USA) to obtain a cell concentration of 1 × 108 CFU/mL. One milliliter of the cell suspensions was inoculated into 9 mL of simulated gastric juice composed of 10 g/L pepsin (Sigma-Aldrich, USA) with pH was adjusted to 3.0 and incubated at 37°C for 3 h. After incubation, the bacteria were centrifuged and resuspended in 10 mL of simulated small intestinal juice which was composed of 10 g/L trypsin (Sigma-Aldrich, USA), 3 g/L oxgall (Difco, USA) and 6.8 g/L KH2PO4 (Sigma-Aldrich, USA) with pH was adjusted to 6.8 and incubated at 37°C for 24 h sequentially. The GIT tolerance of cells was determined by viable cell counts on MRS agar plates. The percentage of bacterial survival was calculated using the following equation: survival rate (%) = final (log CFU/mL)/initial (log CFU/mL) × 100%.
2.4 Adhesion ability to Caco-2 cells
Caco-2 cells were obtained from the cell bank of Chinese Academy of Sciences and grown in Dulbecco’s Modified Eagle Medium (DMEM, Thermo Fisher, USA) supplemented with 10% (v/v) fetal bovine serum (FBS, Corning, New Zealand) and antibiotics (100 U/mL penicillin, 100 μg/mL streptomycin, Thermo Fisher, USA) at 37°C in an atmosphere of 5% CO2 at constant humidity. The adherence assay was performed as described by Zhang et al. (2016) and Sophatha et al. (2020), with some modifications. Briefly, cells were seeded at approximately 2 × 105 cells/ml into 24-well cell culture plates (Corning, USA), and the medium was changed every 2 days until the cells were fully differentiated at 14 days. A fresh strain suspension (1 × 108 CFU/mL) which was suspended in 1 mL of DMEM with 10% (v/v) fetal bovine serum (FBS, Corning, New Zealand) without antibiotics, was added to the previously prepared Caco-2 cell monolayers. The cell mixtures were incubated at 37°C under 5% CO2 atmosphere for 2 h, and then each well was gently washed three times with PBS solution to remove non-adhering bacteria. The Caco-2 cells and adhering bacteria were disrupted using 0.1%TritonX-100 (Nanjing Jiancheng, China) and bacterial counts were performed on MRS agar media. Adhesion capacities were expressed as the percentage of bacteria that adhered to the total number of bacteria initially added.
2.5 Assessment of antimicrobial activity
The antimicrobial activity of HOM2217 against E. cloacae ATCC 13047 was performed as described by Choi et al. (2019) with some modifications. Briefly, the strain cultures were harvested by centrifugation at 10,000 × g, 4°C for 10 min and their Cell-free supernatants (CFS) were filtered through 0.22 μm membrane filter. CFS at different concentrations (0, 5, 10, 15, and 20%) were cocultured in the presence of E. cloacae ATCC 13047 (106 CFU/mL) at 37°C for 24 h. E. cloacae ATCC 13047 cells were used to evaluate the antimicrobial activity. The same concentrations of LGG cell-free supernatant were used as positive controls.
2.6 Assimilation of cholesterol
Cholesterol was evaluated using the procedure described by Albano et al. (2018) with some modifications. Briefly, freshly prepared MRS broth was supplemented with 0.30% ox gall. Water-soluble cholesterol (Cholesterol-PEG 600, Sigma, USA) was filter-sterilized and added to the broth at a final concentration of 120 μg/mL, inoculated with each strain, and incubated anaerobically at 37°C for 24 h. Following incubation, bacterial cells were harvested by centrifugation (10,000 × g, 10 min). The supernatant and uninoculated control MRS broth were assayed calorimetrically to determine cholesterol content using the o-phthalaldehyde method described by Shehata et al. (2016).
2.7 Assessment of the inhibitory effect on 3T3-L1 adipocytes
3T3-L1 cells were obtained from the cell bank of Peking Union Medical College (PUMC, China) and cell viability was evaluated using the CellTiter 96® Aqueous One Solution Cell Proliferation Assay kit (Promega, USA) (Park et al., 2011). 3T3-L1 cells were cultured as described by Jeung et al. (2018) with some modifications. Briefly, 3T3-L1 cells were seeded at a density of 2 × 105 cells/well in 24-well culture dishes until confluence was reached. Two days post-confluence (D0), the medium was replaced with differentiation cocktail medium (MDI: 0.5 mM 3-isobutyl-1-methylxanthine (IBMX; Sigma, USA), 1 μM dexamethasone (Dex; Sigma, USA), and 10 μg/mL insulin (Sigma, USA)) in DMEM supplemented with 10% FBS, and the cells were incubated for 4 days. On day four (D4), the medium was replaced with DMEM containing 10 μg/mL insulin (Sigma, USA) and the cells were incubated for 2 days. On day six (D6), the medium was replaced with DMEM and the cells were incubated for 2 days. Differentiation was completed on day eight (D8). Heat-killed strains (70°C, 30 min) were treated with the medium from D0 to D8. Lipid accumulation was quantified using Oil Red O (Nanjing Jiancheng, China) according to the manufacturer’s instructions. The cells were observed under an optical microscope. Oil Red O was quantified by extracting the dye with 100% isopropanol and measuring the absorbance at 500 nm using a microplate reader. TG and protein concentrations were measured using a commercial TG and TP assay kit (Nanjing Jiancheng, China) according to the manufacturer’s instructions.
2.8 Anti-obesity effect in diet-induced obese rats
The experimental animals comprised 70 males eight-week-old Sprague–Dawley rats purchased from Beijing HFK Bioscience Co., LTD (China). After 1 week of adaptation to solid formula feed (Beijing HFK Bioscience Co., LTD, China) at the Lab Animal Center of Beijing Union University, the animals were randomized according to their body weights and divided into two groups. Rats were fed either a high-fat diet (HFD; n = 60) or a normal diet (ND; n = 10) for 2 weeks. Energy from fat was 60 and 10% for the high-fat and normal diets, respectively. After 2 weeks of feeding, the rats fed a high-fat diet were sorted according to body weight gain, and one third of the rats that were obesity-resistant rats and exhibited lower weight gain were eliminated. Forty obesity-sensitive rats were randomly divided into four groups according to body weight: a model control group (HFD) and three treatment groups, high-fat diet with low dose HOM2217 (HFDL), high-fat diet with medium dose HOM2217 (HFDM), and high-fat diet with high dose HOM2217 (HFDH), with 10 rats in each group.
HOM2217 viable bacteria for animal administration were prepared by suspending the lyophilized bacterial powder in PBS. Clinical trials indicate that L. plantarum doses for humans range from 1 × 1010 to 2 × 1011 CFU/day, with a recommended low dose of 2.5 × 1010 CFU/day. In the study, rats in the HFDL, HFDM and HFDH groups received 2.5 × 109, 1.25 × 1010, and 2.5 × 1010 CFU/kg body weight, respectively, for 7 weeks, with doses converted from human levels using a 6.0-fold adjustment factor based on a 60 kg human weight (Kadooka et al., 2010; Michael et al., 2021; Nasiri et al., 2021; Oh et al., 2023).
The ND and HFD groups received the same volume of the vehicle. The well-being, food intake, and body weight of the animals were recorded weekly. The body weight gain was calculated. At the end of the experiment, the rats were fasted overnight and euthanized by CO2 asphyxiation. The body fat (epididymal and perirenal fat pads) was excised and weighed. Serum was separated from the blood by centrifugation at 1,200 × g for 10 min at 4°C and stored at −80°C until analyses. Serum TC, TG, HDL-C, and LDL-C levels were measured using a Cobas C311 Chemistry Fully Automatic Biochemistry Analyzer (Roche, Switzerland), according to the manufacturer’s protocols. Tumor necrosis factor-α (TNF-α) and interleukin 6 (IL-6) levels were determined using the rat ELISA Kit (RayBio, USA) according to the manufacturer’s instructions. Approximately 1,000 mg of cecal contents were removed, and the levels of SCFAs were determined by gas chromatography–mass spectrometry (GC–MS) (Agilent 8860, USA) using a previously described method (Li et al., 2020).
2.9 Statistical analysis
All in vitro experiments were repeated in triplicate. Experimental values are expressed as mean ± SD or mean ± SEM. Data were analyzed using either Student’s t-test or one-way analysis of variance (ANOVA), followed by Dunnett’s post hoc correction for multiple comparisons in GraphPad Prism (Version 9.0, GraphPad Software Inc., San Diego, CA, USA), and statistical significance was set at p < 0.05.
3 Results
3.1 General genome features of HOM2217
As shown in Figure 1A, the complete genome of HOM2217 contained a single circular chromosome of 3,267,529 bp with a GC content of 44.5%, and one plasmid (62,350 bp) with a GC content of 38.5%. A total of 2,741 genes were classified into COG functional categories (Figure 1B). Among these, a total of 295 genes (10.8%) were involved in carbohydrate transport and metabolism, 282 genes (10.3%) in transcription, 222 (8.1%) in amino acid transport and metabolism, 107 (3.9%) in lipid transport and metabolism, and 219 genes (8.0%) were involved in general function prediction. L. plantarum HOM2217 contains genes related to acid and bile tolerance, epithelial cell adhesion, immunomodulation, antibacterial activity, and lipid metabolism (Supplementary Table S1).
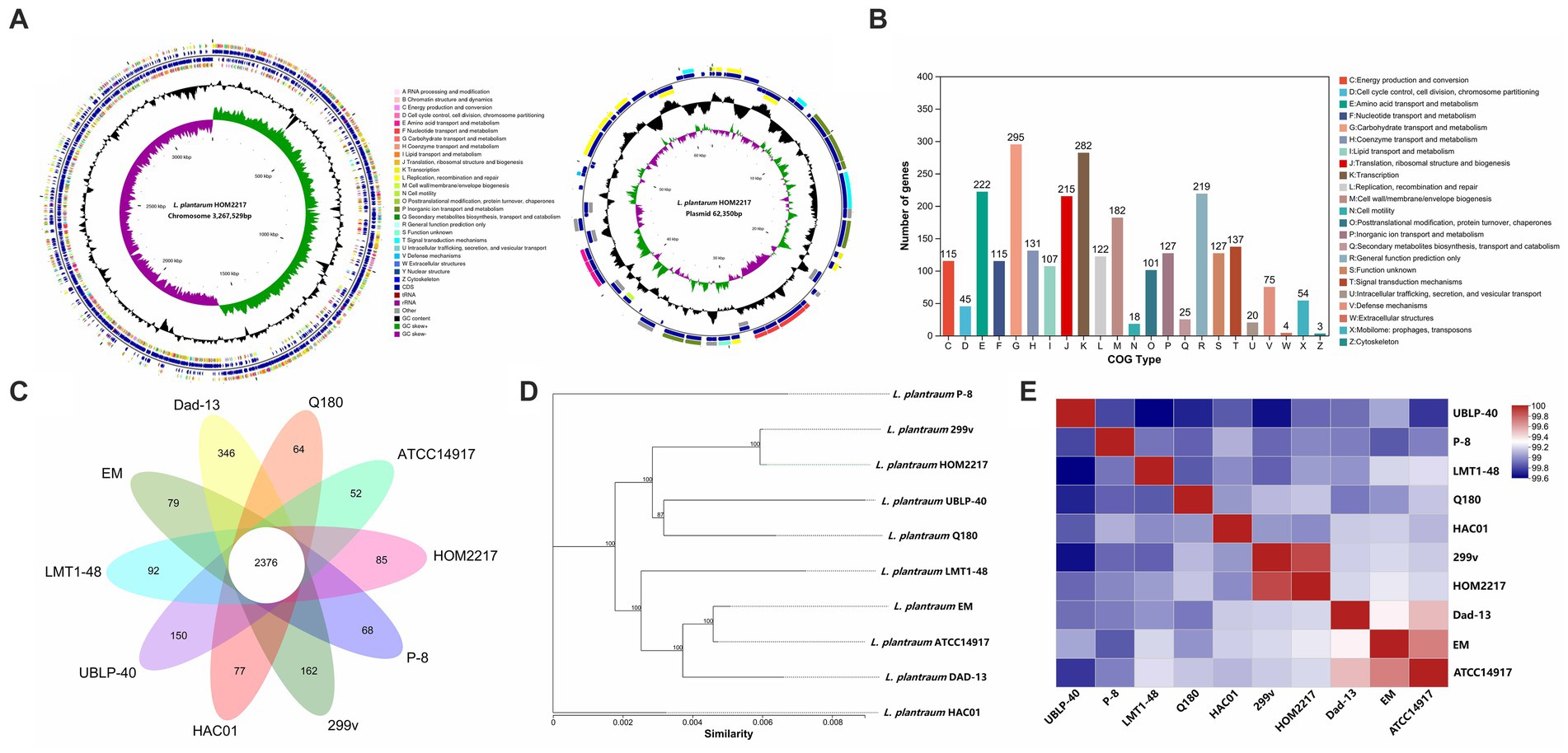
Figure 1. Genome features of HOM2217. (A) Circular genomic map of HOM2217. The circular map was generated using Circos and contains seven circles. Marked information is displayed from the outer circle to the innermost circle as follows: Genome size, CDSs on the forward stand, CDSs on the reverse stand, prophage regions, rRNA and tRNA, GC content, and GC skew. (B) Gene number of COG categories. (C) Numbers of orthologous gene families and unique genes among 10 L. plantarum strains. The Venn diagram shows the number of orthologous gene families of the core genome (center) and the numbers of unique genes of each genome. The different colors indicate different sampling areas of the strains as indicated. The orthologous gene families were determined using OrthoMCL software with an inflation value of 1.5. (D) Phylogenetic tree of 10 L. plantarum strains. The phylogenetic tree was built based on aligned concatenated sequences of single copy orthologous gene families. The bootstrap support value before each node represents the confidence degree of each branch. (E) Average nucleotide identity (ANI) tree analysis of 10 L. plantarum strains.
The genomic features of the 10 L. plantarum reference strains with detailed WGS data are presented in Table 1. Each strain contained a circular chromosome and a different number of plasmids. The genome size of the reference strains was 3.2–3.6 Mp, the number of plasmids varied from 0 to 10, and the number of CDSs ranged from 2,893 to 3,127. A total of 2,350 genes were identified in the core genome, and 1,157 genes were identified as unique genes (Figure 1C). Among the unique genes, L. plantarum Dad-13 had the most (346) of unique genes, whereas L. plantarum ATCC 14917 had the fewest (52) unique genes. Eighty-five unique genes were identified in the HOM2217 genome, with 34 functionally annotated. A selection of these genes associated with probiotic functions is listed in Supplementary Table S2. To understand the evolutionary relationships among L. plantarum strains, ML and ANI trees were constructed (Figures 1D,E). HOM2217 showed a close relationship with the strain L. plantarum 299v, but was located on a relatively separate branch. ANI > 95% indicated the same species (Jain et al., 2018). The ANI values indicated that the 10 strains belonged to the same species (>99%), and L. plantarum 299v was the closest neighbor to HOM2217 (99.93% of the ANI value).
3.2 Survival under simulated gastrointestinal tract conditions
The initial viable cell density in the simulated gastric acid was approximately 1 × 108 CFU/mL. As shown in Figure 2A, HOM2217 had a significantly (p < 0.05) higher survival (100.39 ± 0.78%) in the simulated gastric fluid with pH of 3.0 for 3 h compared with that of L. rhamnosus GG (98.82 ± 0.46%). The final survival rates of HOM2217 following exposure to simulated intestinal fluid for 3 h and 24 h were 99.74 ± 1.15% and 99.95 ± 0.99%, respectively, which were significantly (p < 0.01) higher than those of L. rhamnosus GG.
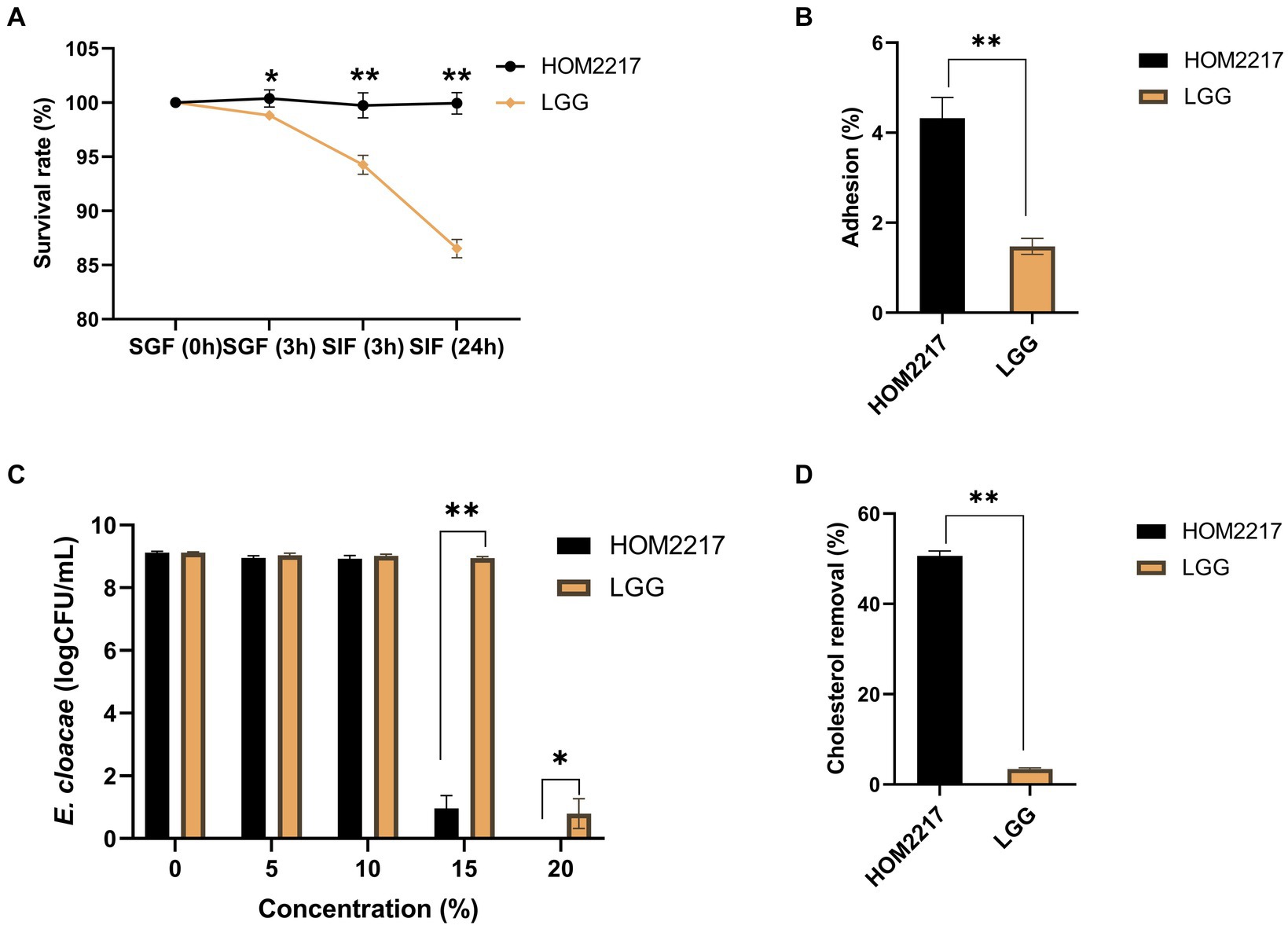
Figure 2. Characteristics of the HOM2217 strain. (A) The survival rate of HOM2217 under simulated gastrointestinal tract (GIT) conditions. SGF, simulated gastric fluid; SIF, simulated intestinal fluid. (B) Adhesion of HOM2217 to Caco-2 cells. (C) Antimicrobial activity of HOM2217 against the obesity-inducing bacterium Enterobacter cloacae. (D) Cholesterol removal (%) by HOM2217. L. rhamnosus GG was used as the control strain, error bars represent the mean ± SD (n = 3). *p < 0.05 and **p < 0.01.
3.3 Adhesion ability to Caco-2 cells
The adhesion of HOM2217 cells was expressed as the percentage of adhering isolates relative to the number of isolates added, as depicted in Figure 2B. The adhesion percentage of HOM2217 to Caco-2 cells after 2 h of cocultivation was 4.32 ± 0.46% which was significantly higher (p < 0.01) than that of L. rhamnosus GG.
3.4 Antimicrobial activity
Gram-negative Enterobacter cloacae strains were recently identified in obese mice and are considered pathogenic bacteria in the gut, resulting in obesity (Fei and Zhao, 2013). Therefore, a coculture experiment was performed to assess the antimicrobial activity of HOM2217 on E. cloacae ATCC 13047. The results are shown in Figure 2C. The number of E. cloacae only decreased by 0.16 and 0.19 orders of magnitude in the presence of 5 and 10% cell-free supernatants from HOM2217, respectively. However, the number of E. cloacae significantly decreased by 8.15 orders of magnitude when the 15% cell-free supernatant of HOM2217 was added. The growth of E. cloacae ATCC 13047 was completely inhibited when the cell-free supernatants of HOM2217 concentration was increased to 20%. The antimicrobial activity of HOM2217 against E. cloacae ATCC 13047 was significantly stronger (p < 0.05) than that of L. rhamnosus GG.
3.5 Assimilation of cholesterol
Bile tolerance and the ability to assimilate cholesterol are considered primary characteristics for the selection of potential probiotic strains with cholesterol-lowering effects. HOM2217 manifested superior ability (50.63 ± 1.08%) (Figure 2D) in the removal of cholesterol from the medium, and it was significantly (p < 0.01) higher than that of L. rhamnosus GG (3.42 ± 0.23%).
3.6 Assessment of the inhibitory effect on 3T3-L1 adipocytes
As shown in Figure 3A, the heat-killed strains exhibited no cytotoxicity against 3T3-L1 preadipocytes at concentrations of up to 1 × 109 thallus forming units (TFU/mL). Thus, in subsequent experiments, heat-killed strain treatments were performed at ≤1 × 109 TFU/mL. Heat-killed HOM2217 and L. rhamnosus GG were obtained by suspending the heat-killed cells in DPBS at 1 × 109, 1 × 108, and 1 × 107 TFU/mL and then were used to treat 3T3-L1 preadipocytes during the adipogenic phase. 3T3-L1 adipocytes stained with Oil Red O solution were observed under an optical microscope (Figure 3B), which showed that more lipid droplets were formed in cells treated with MDI only, and lipid accumulation was also significantly (p < 0.01) increased. An eight-day treatment with heat-killed strains of HOM2217 or L. rhamnosus GG inhibited lipid accumulation reduction in a dose-dependent manner (21.4 ± 6.97% and 9.37 ± 1.41% at 1 × 107 TFU/mL, 27.68 ± 4.10% and 13.93 ± 0.90% at 1 × 108 TFU/mL and 48.80 ± 2.92% and 19.89 ± 2.53% at 1 × 109 TFU/mL, respectively; Figure 3C). Based on the AdiopoRed assay, the addition of heat-killed strains of HOM2217 at 1 × 109, significantly decreased lipid accumulation by 48.80 ± 2.92%, which was significantly (p < 0.01) better than the effect of other treatments (Figure 3C). The TG content of differentiated 3T3-L1 cells was the most repressed by 1 × 109 TFU/mL of HOM2217 (Figure 3D). The degree of adipocyte differentiation inhibition was concentration-dependent on the heat-killed strain and was more pronounced with HOM2217 compared to L. rhamnosus GG, indicating that HOM2217 inhibits lipid accumulation in differentiated 3T3-L1 adipocytes.
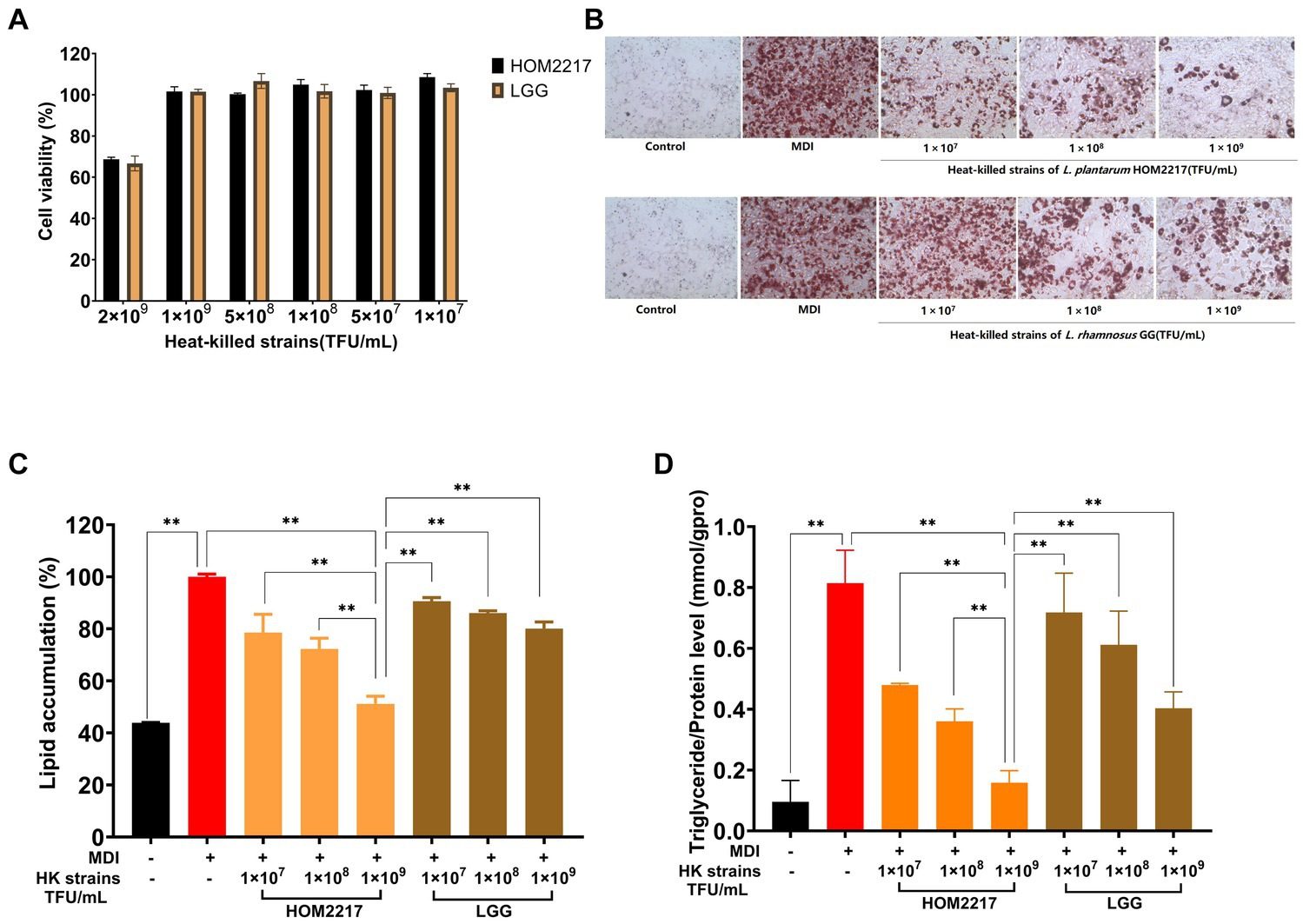
Figure 3. Effects of heat-killed strains of HOM2217 on lipid accumulation in 3T3-L1 adipocytes. (A) Cell viability, (B) Oil Red O staining, (C) Lipid accumulation, and (D) TG content. L. rhamnosus GG was used as the control strain. Error bars represent the mean ± SD (n = 3). *p < 0.05, **p < 0.01.
3.7 Anti-obesity effect in diet-induced obese rats
High-fat diet feeding induced significant (p < 0.01) body weight, body weight gain, and body fat throughout the study period compared to the ND (Figures 4A–C). The administration of high doses of HOM2217 induced lower body weight (p < 0.05), body weight gain (p < 0.01), and body fat (p < 0.05) compared to the HFD. However, the medium or low doses did not exhibit this effect. Interestingly, the reduction in body weight, body weight gain, and body fat in the HFDH group did not result from differences in food intake (Figure 4D).
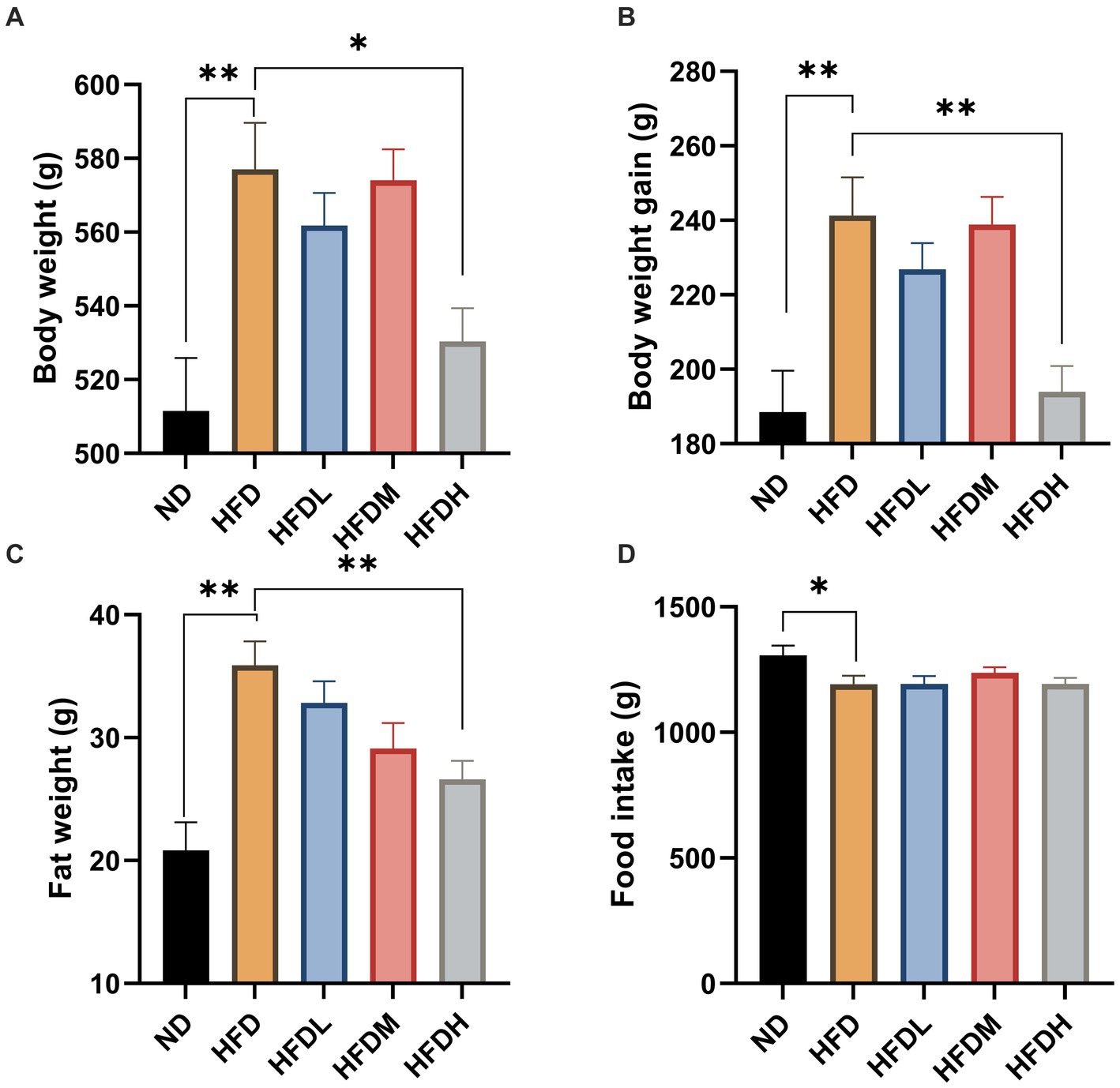
Figure 4. Anti-obesity effects of HOM2217 in high-fat diet-fed rats. (A) Body weight, (B) Body weight gain, (C) Fat weight, (D) Food intake. Error bars represent mean ± SEM (n = 10). *p < 0.05 and **p < 0.01. ND, normal diet; HFD, high-fat diet; HFDL, high-fat diet with low dose HOM2217 (2.5 × 109 CFU/kg body weight); HFDM, high-fat diet with medium dose HOM2217 (1.25 × 1010 CFU/kg body weight); HFDH, high-fat diet with high dose HOM2217 (2.5 × 1010 CFU/kg body weight).
As shown in Figure 5A, the serum total cholesterol concentrations were significantly higher in the HFC group than in the ND group (p < 0.01). However, there were no significant effects of HOM2217 supplementation on the TC concentration (p > 0.05). Serum TG levels were reduced by 17% in the HFDH group compared to those in the HFD group, but the difference was not significant owing to a large error (Figure 5B). Serum HDL-C concentrations were significantly increased both in the HFDM (p < 0.05) and HFDH groups (p < 0.01) compared to those in the HFD group (Figure 5C). There were no significant effects of HOM2217 supplementation on the LDL-C concentration (p < 0.05) (Figure 5D). As seen in Figures 5E,F, the serum cytokine indicators TNF-α and IL-6 were markedly higher in the HFD group than in the ND group (p < 0.01). Both the HFDM (p < 0.01) and HFDH groups (p < 0.01) had a significantly decreased serum TNF-α level but not serum IL-6 level.
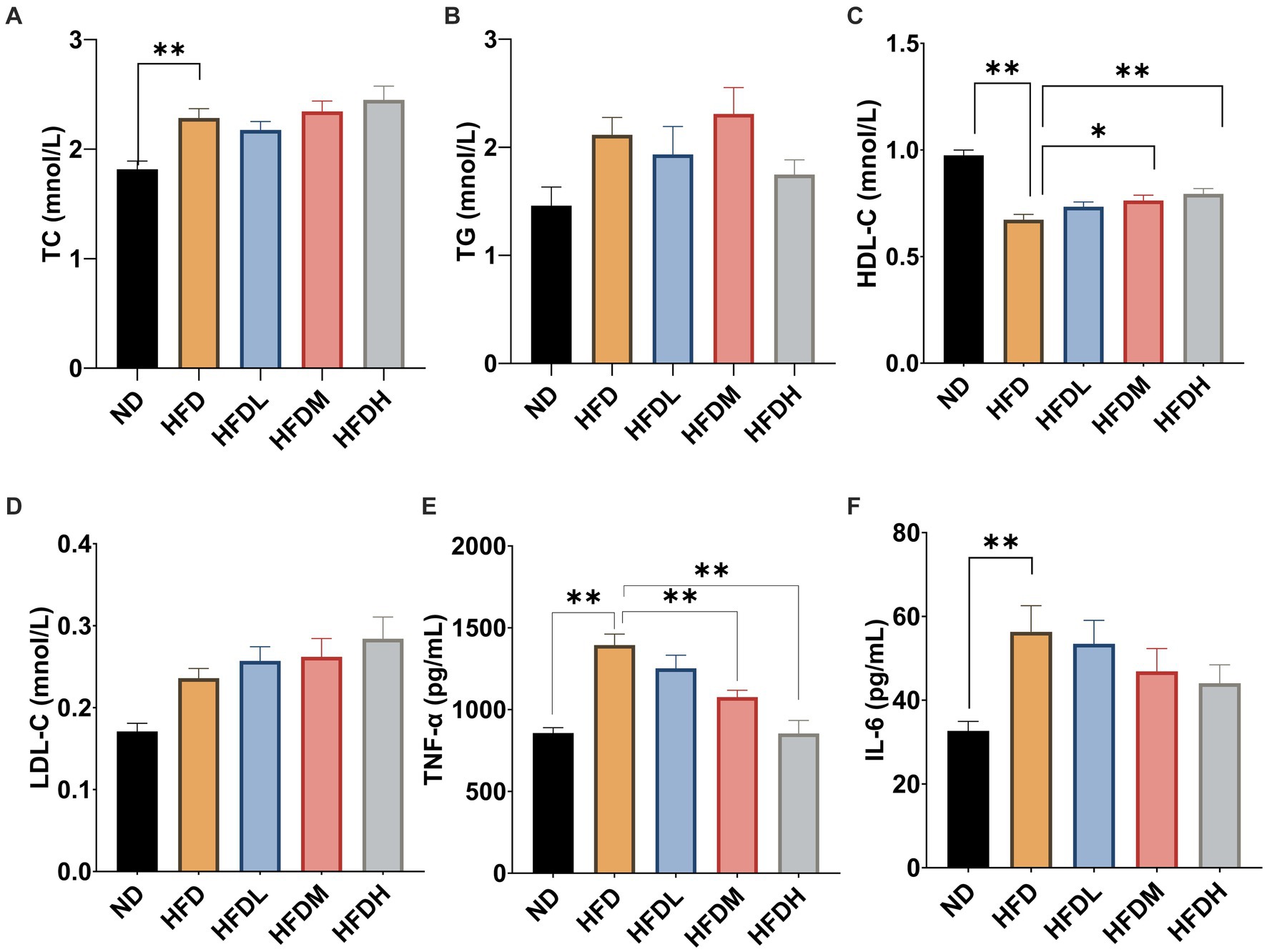
Figure 5. Effects of HOM2217 on blood lipid and cytokine levels in HFD-fed rats. (A) Serum total cholesterol (TC) level, (B) Serum triglyceride (TG) level, (C) Serum high-density lipoprotein cholesterol (HDL-C) level, (D) Serum low-density lipoprotein cholesterol (LDL-C) level, (E) Serum tumor necrosis factor (TNF-α) level, and (F) Serum interleukin 6 (IL-6) level. Error bars represent mean ± SEM (n = 10). *p < 0.05, **p < 0.01. ND, normal diet; HFD, high-fat diet; HFDL, high-fat diet with low dose HOM2217 (2.5 × 109 CFU/kg body weight); HFDM, high-fat diet with medium dose HOM2217 (1.25 × 1010 CFU/kg body weight); HFDH, high-fat diet with high dose HOM2217 (2.5 × 1010 CFU/kg body weight).
As shown in Figure 6, the concentrations of formic acid (p < 0.01), acetic acid (p < 0.05), propionic acid (p < 0.01), and butyric acid (p < 0.05) were significantly lower in the HFD group than in ND group. Supplementation with a high dose of HOM2217 significantly (p < 0.01) increased the concentrations of formic acid, acetic acid, and butyric acid, but did not significantly change the concentrations of propionic acid. The medium concentration of HOM2217 also significantly increased formic acid concentrations compared with the HFD group (p < 0.05) (Figure 6A).
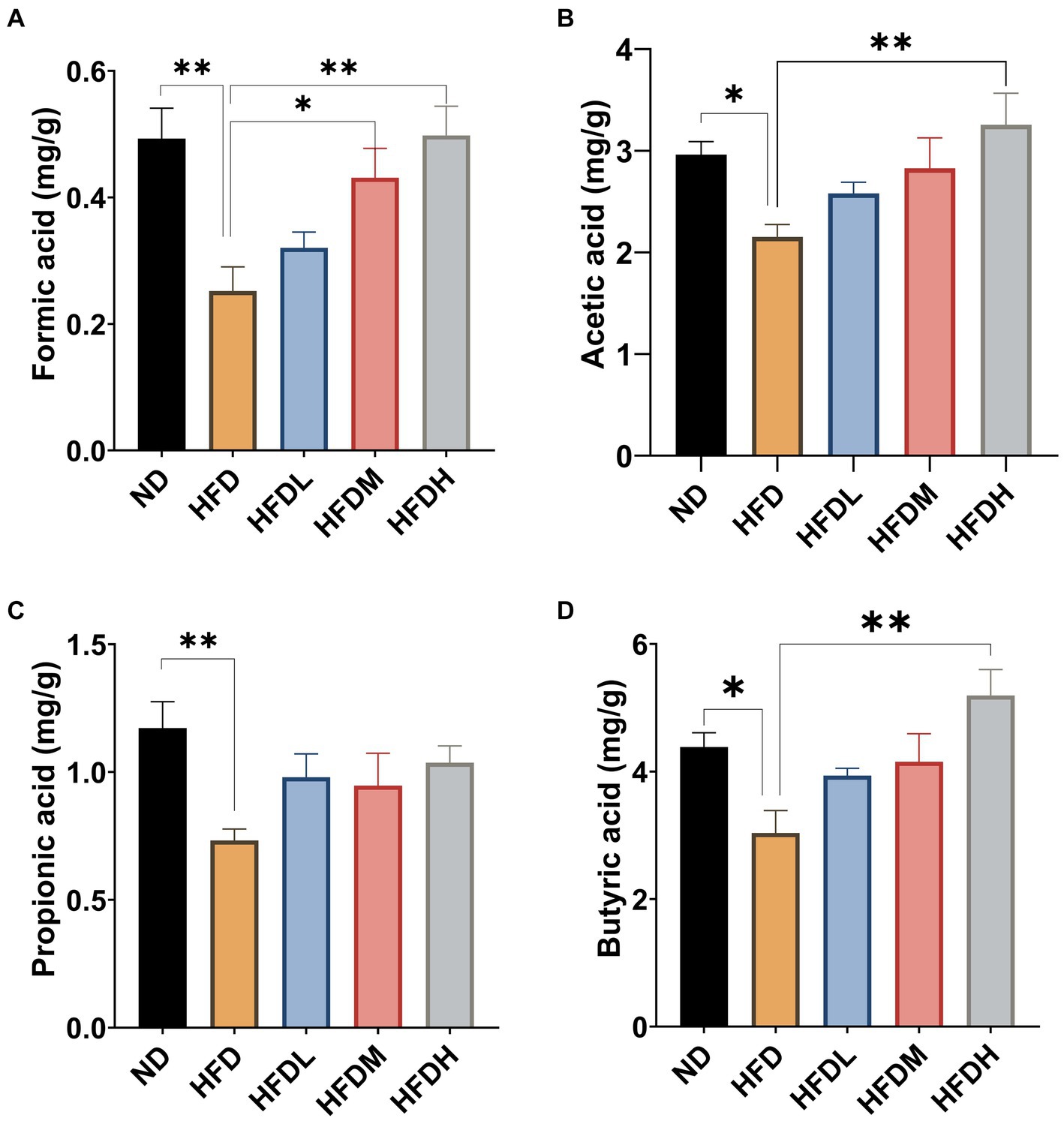
Figure 6. Effects of HOM2217 on cecum SCFAs concentrations in HFD-fed rats. (A) Formic acid, (B) Acetic acid, (C) Propionic acid, and (D) Butyric acid. Error bars represent mean ± SEM (n = 10). *p < 0.05, **p < 0.01. ND, normal diet; HFD, high-fat diet; HFDL, high-fat diet with low dose HOM2217 (2.5 × 109 CFU/kg body weight); HFDM, high-fat diet with medium dose HOM2217 (1.25 × 1010 CFU/kg body weight); HFDH, high-fat diet with high dose HOM2217 (2.5 × 1010 CFU/kg body weight).
4 Discussion
Obesity poses a major public health problem in all age groups worldwide; therefore, there is an urgent need to implement obesity prevention programs and identify new therapeutic and intervention targets. Previous study has demonstrated that several probiotics, used alone or in symbiotic mixtures, exert anti-obesity effects through species-and strain-specific mechanisms. In particular, L. rhamnosus GG supplementation 1 month before the expected delivery and subsequent treatment of the baby for a further 6 months lowered weight gain (age range 1–4 years) (Luoto et al., 2010). L. rhamnosus GG warrants consideration as a therapeutic tool to treat hypertransaminasemia in children with hepatopathic obesity who are noncompliant with lifestyle interventions (Vajro et al., 2011). L. rhamnosus GG inhibits fat accumulation in the liver by phosphorylating of adenosine monophosphate kinase (AMPK) (Zhang et al., 2015). A randomized, double-blind, placebo-controlled trial showed that the administration of L. plantarum LMT1-48 decreased body weight, abdominal visceral fat area, insulin resistance, and leptin levels in overweight subjects by regulating the gut microbiota (Sohn et al., 2023). In addition, L. plantarum Dad-13 significantly decreased body weight and BMI (p < 0.05) by decreasing the abundance of Firmicutes and increasing that of Bacteroidetes (particularly Prevotella) (Rahayu et al., 2021).
In this study, we isolated a novel strain from human milk, HOM2217, and analyzed its whole-genome sequence. The results showed that HOM2217 cells were identified as L. plantarum. L. plantarum is generally recognized as safe based on the long history of human consumption of Lactobacilli in food. It has been generally recognized as safe (GRAS) by the US Food and Drug Administration (FDA) and is included in the list of qualified presumptions of safety (QPS), recommended by the European Food Safety Authority (EFSA). To verify the probiotic characteristics of L. plantarum HOM2217 at the genomic level, genes related to probiotic properties were annotated using COG database. Based on published literature data, they reveal probiotic marker genes (PMG) which related to their characteristics (Chintakovid et al., 2024; Isaac et al., 2024). The proposed genes may represent strain-specific traits enhancing probiotic potential. The HOM2217 strain carries various probiotic marker genes, including four bsh genes (bsh1, bsh2, bsh3, and bsh4). Notably, bsh1 is primarily responsible for Bsh activity against pancreatin and low gastric pH and plays a role in lowering serum cholesterol levels (Lambert et al., 2008). This finding indicated its potential anti-obesity functions at genomic levels. Comparison with other L. plantarum strains revealed unique genes in HOM2217. For instance, the katE gene and its product, catalase, are crucial for the bacterium’s resistance to oxidative damage and its survival across various environmental conditions, including during infections and in the presence of host immune responses (Sun et al., 2022). In addition, our ANI tree results revealed that L. plantarum 299v and HOM2217 shared the highest similarity. As described, L. plantarum 299v is one of the most documented L. plantarum strains in the world, described in over 170 scientific publications, of which more than 60 are human clinical studies. It is safe, does not confer antibiotic resistance, and has been shown to provide relief of symptoms for those suffering from IBS and IBD and to mitigate iron deficiency (Nordstrom et al., 2021). In addition, Bouaziz et al. (2021) reported that the administration of L. plantarum 299v was useful in the treatment of obesity and metabolic syndrome in rabbits.
To achieve an anti-obesity effect in the intestine, probiotic bacteria should have the ability to pass through the upper parts of the gastrointestinal tract and adhere to intestinal epithelial cells, which helps them resist fluctuations in their intestinal levels and inhibit the attachment of pathogenic bacteria via competitive adhesion throughout the intestine. In this study, HOM2217 showed high tolerance to in vitro gastrointestinal conditions and exhibited high adhesive abilities to intestinal epithelial cell lines. Enterobacteriaceae, which are present in higher levels in obese subjects compared to non-obese subjects (Karlsson et al., 2012), can be used as an in vitro simulator of obesity. In this study, HOM2217 showed antimicrobial properties against E. cloacae ATCC 13047, indicating that HOM2217 may have a strong anti-obesity effect. Albano (Albano et al., 2018) tested the cholesterol removal capacity of seven Lactobacillus casei strains, 27 Lactobacillus paracasei ssp. paracasei strains, five Lactococcus lactis ssp. lactis strains, 15 L. plantarum strains, one Enterococcus lactis strain, and one Enterococcus faecium strain and reported that the lowest reduction in cholesterol was induced by Lactococcus lactis ssp. Lactis, whereas L. plantarum (26.1 ± 14.2%) and Lactobacillus paracasei ssp. paracasei (26.0 ± 10.4%) induced the highest decrease. In this study, HOM2217 showed a high cholesterol removal ability (50.63 ± 1.08%). Obesity is related to the differentiation, expansion, and lipid accumulation of adipocytes, and the degree of obesity is closely related to the degree of differentiation of preadipocytes into adipocytes and the enlargement of adipocytes in adipose tissues. HOM2217 significantly decreased TG content and lipid accumulation in fully differentiated 3T3-L1 adipocytes, reflecting its anti-obesity potential. Several studies have also found that L. plantarum species had an anti-obesity effect in 3T3-L1 cells, demonstrating that the inhibition of adipogenesis and lipid accumulation exerted by functional bacterial strains in mouse preadipocyte cells were mediated by the suppression of key adipogenic transcription factors (Park et al., 2011; Park et al., 2014; Jeung et al., 2018).
Our in vitro results indicated that treatment with heat-killed HOM2217 reduced lipid accumulation in adipocytes, prompting us to explore the anti-obesity effects of live HOM2217 in vivo. The consumption of a HFD is a key obesity-inducing factor, and long-term ingestion of HFD causes an increase in body fat in mammals. Therefore, in the present study, we investigated the anti-obesity effects of HOM2217 at three different doses in HFD-induced obese rats. We found that only high dose of HOM2217 exhibited anti-obesity effects against HFD-induced obesity, as demonstrated by the decreased body weight, body weight gain, and body fat without changes in food intake, which was in line with previous reports showing that only high dose of L. plantarum SKO-001 decreased the body weight and body fat in mice (Choi et al., 2023). The HDL-C level was higher than that of the HFD control group after supplementation with high and medium doses of HOM2217, indicating that HOM2217 helps regulate lipid metabolism in rats by increasing HDL-C levels. Recently, attention to inflammation in the intestine has been increasing since it was revealed that HFD induces inflammation by altering the gut microbiome and increasing intestinal permeability and may be a causative factor in the onset of obesity (Kim et al., 2012). We found that HOM2217 decreased serum TNF-α levels, which was supported by previous reports that L. plantarum ATG-K2 reduced the expression levels of inflammatory cytokines such as TNF-α and NF-κB in the small intestine of high-fat and high-fructose diet-fed rats (Park et al., 2021). Studies have also demonstrated that probiotics that produce short-chain fatty acids (SCFA) exhibit anti-obesity properties by regulating lipid and glucose metabolism, reducing adipocyte size, lowering cholesterol, and regulating leptin levels (den Besten et al., 2013). HOM2217 increased SCFA levels (formic, acetic, and butyric acids) in the cecum, which is consistent with the findings of Lee et al. (2021), who also found that gut microbiome modulation by L. plantarum ATG-K2 to increase SCFAs may serve as potential mechanism to alleviate obesity (Lee et al., 2021). However, due to the limitations of SCFAs produced by L. plantarum, further studies are needed to identify the effects of HOM2217 on the gut microbiota.
In summary, we sequenced the complete genome of HOM2217 isolated from human milk, studied its physiological characteristics, and investigated its anti-obesity effects in 3T3-L1 cells and HFD-fed rats to determine its potential as a starter for functional food products. Based on the complete genome sequence, the strain was named HOM2217, and was closest to L. plantarum 299v. The HOM2217 strain demonstrated greater tolerance to in vitro gastrointestinal conditions, higher adhesion to intestinal epithelial cell lines, superior antimicrobial activity against E. cloacae ATCC 13047, and more effective cholesterol removal compared to L. rhamnosus GG. In the present study, we found that HOM2217 exhibited anti-obesity effects in 3T3-L1 adipocytes and HFD-fed obese rats, which were accompanied by the inhibition of the inflammatory response and regulation of lipid metabolism and SCFAs levels. Hence, our results provide valuable insights into the potential of L. plantarum HOM2217 for suppressing obesity in vitro and in rats fed a high-fat diet. Taken together, these findings suggest that HOM2217 may has beneficial effects in ameliorating obesity and offer novel therapeutic strategies for obesity.
Data availability statement
The datasets presented in this study can be found in online repositories. The names of the repository/repositories and accession number(s) can be found in the article/Supplementary material.
Ethics statement
The animal study was approved by the Ethics Committee of the Health Food Function Testing Center, College of Applied Arts and Science, Beijing Union University. The study was conducted in accordance with the local legislation and institutional requirements.
Author contributions
TW: Writing – original draft, Writing – review & editing, Conceptualization, Data curation, Formal analysis, Investigation, Methodology, Project administration, Software, Supervision, Validation, Visualization. XZ: Data curation, Project administration, Writing – review & editing. LF: Data curation, Project administration, Writing – review & editing. YZ: Data curation, Project administration, Writing – review & editing. ZZ: Data curation, Project administration, Writing – review & editing. ZC: Data curation, Project administration, Writing – review & editing. YX: Supervision, Validation, Writing – review & editing. SL: Supervision, Validation, Writing – review & editing. CL: Funding acquisition, Project administration, Resources, Writing – review & editing. SZ: Conceptualization, Data curation, Formal analysis, Investigation, Methodology, Project administration, Software, Supervision, Validation, Visualization, Writing – review & editing.
Funding
The author(s) declare financial support was received for the research, authorship, and/or publication of this article. This study was supported by Beijing Hanmi pharmaceutical Co., Ltd. (RD113) and Coree Beijing Co., Ltd. (RD06).
Conflict of interest
TW, XZ, LF, YZ, ZZ, and ZC were employed by Beijing Hanmi Pharmaceutical Co., Ltd. YX, SL, CL, and SZ were employed by Coree Beijing Co., Ltd.
Publisher’s note
All claims expressed in this article are solely those of the authors and do not necessarily represent those of their affiliated organizations, or those of the publisher, the editors and the reviewers. Any product that may be evaluated in this article, or claim that may be made by its manufacturer, is not guaranteed or endorsed by the publisher.
Supplementary material
The Supplementary material for this article can be found online at: https://www.frontiersin.org/articles/10.3389/fmicb.2024.1436378/full#supplementary-material
References
Albano, C., Morandi, S., Silvetti, T., Casiraghi, M. C., Manini, F., and Brasca, M. (2018). Lactic acid bacteria with cholesterol-lowering properties for dairy applications: in vitro and in situ activity. J. Dairy Sci. 101, 10807–10818. doi: 10.3168/jds.2018-15096
Alvarez-Arrano, V., and Martin-Pelaez, S. (2021). Effects of probiotics and synbiotics on weight loss in subjects with overweight or obesity: a systematic review. Nutrients 13:3627. doi: 10.3390/nu13103627
American College of Cardiology/American Heart Association Task Force on Practice Guidelines, Obesity Expert Panel, 2013 (2014). Expert panel report: guidelines (2013) for the management of overweight and obesity in adults. Obesity 22, S41–S410. doi: 10.1002/oby.20660
Apovian, C. M., Aronne, L. J., Bessesen, D. H., McDonnell, M. E., Murad, M. H., Pagotto, U., et al. (2015). Pharmacological management of obesity: an endocrine society clinical practice guideline. J. Clin. Endocrinol. Metab. 100, 342–362. doi: 10.1210/jc.2014-3415
Asadi, A., Shadab Mehr, N., Mohamadi, M. H., Shokri, F., Heidary, M., Sadeghifard, N., et al. (2022). Obesity and gut-microbiota-brain axis: A narrative review. J. Clin. Lab. Anal. 36:e24420. doi: 10.1002/jcla.24420
Besemer, J., and Borodovsky, M. (2005). GeneMark: web software for gene finding in prokaryotes, eukaryotes and viruses. Nucleic Acids Res. 33, W451–W454. doi: 10.1093/nar/gki487
Bouaziz, A., Dib, A. L., Lakhdara, N., Kadja, L., Espigares, E., Moreno, E., et al. (2021). Study of probiotic effects of Bifidobacterium animalis subsp. lactis BB-12 and Lactobacillus plantarum 299v strains on biochemical and morphometric parameters of rabbits after obesity induction. Biology 10:131. doi: 10.3390/biology10020131
Chan, P. P., and Lowe, T. M. (2019). tRNAscan-SE: searching for tRNA genes in genomic sequences. Methods Mol. Biol. 1962, 1–14. doi: 10.1007/978-1-4939-9173-0_1
Chintakovid, N., Singkhamanan, K., Yaikhan, T., Nokchan, N., Wonglapsuwan, M., Jitpakdee, J., et al. (2024). Probiogenomic analysis of Lactiplantibacillus plantarum SPS109: A potential GABA-producing and cholesterol-lowering probiotic strain. Heliyon 10:e33823. doi: 10.1016/j.heliyon.2024.e33823
Choi, W. J., Dong, H. J., Jeong, H. U., Jung, H. H., Kim, Y. H., and Kim, T. H. (2019). Antiobesity effects of Lactobacillus plantarum LMT1-48 accompanied by inhibition of Enterobacter cloacae in the intestine of diet-induced obese mice. J. Med. Food 22, 560–566. doi: 10.1089/jmf.2018.4329
Choi, M. J., Yu, H., Kim, J. I., Seo, H., Kim, J. G., Kim, S. K., et al. (2023). Anti-obesity effects of Lactiplantibacillus plantarum SKO-001 in high-fat diet-induced obese mice. Eur. J. Nutr. 62, 1611–1622. doi: 10.1007/s00394-023-03096-x
Delcher, A. L., Bratke, K. A., Powers, E. C., and Salzberg, S. L. (2007). Identifying bacterial genes and endosymbiont DNA with glimmer. Bioinformatics 23, 673–679. doi: 10.1093/bioinformatics/btm009
den Besten, G., van Eunen, K., Groen, A. K., Venema, K., Reijngoud, D. J., and Bakker, B. M. (2013). The role of short-chain fatty acids in the interplay between diet, gut microbiota, and host energy metabolism. J. Lipid Res. 54, 2325–2340. doi: 10.1194/jlr.R036012
Dwivedi, A. K., Dubey, P., Cistola, D. P., and Reddy, S. Y. (2020). Association between obesity and cardiovascular outcomes: updated evidence from Meta-analysis studies. Curr. Cardiol. Rep. 22:25. doi: 10.1007/s11886-020-1273-y
Fei, N., and Zhao, L. (2013). An opportunistic pathogen isolated from the gut of an obese human causes obesity in germfree mice. ISME J. 7, 880–884. doi: 10.1038/ismej.2012.153
Garvey, W. T., Mechanick, J. I., Brett, E. M., Garber, A. J., Hurley, D. L., Jastreboff, A. M., et al. (2016). American Association of Clinical Endocrinologists and American College of endocrinology comprehensive clinical practice guidelines for medical Care of Patients with obesity. Endocr. Pract. 22, 1–203. doi: 10.4158/EP161365.GL
Ghavami, T., Kazeminia, M., Ahmadi, N., and Rajati, F. (2023). Global prevalence of obstructive sleep apnea in the elderly and related factors: a systematic review and meta-analysis study. J. Perianesth. Nurs. 38, 865–875. doi: 10.1016/j.jopan.2023.01.018
Guirro, M., Costa, A., Gual-Grau, A., Herrero, P., Torrell, H., Canela, N., et al. (2019). Effects from diet-induced gut microbiota dysbiosis and obesity can be ameliorated by fecal microbiota transplantation: a multiomics approach. PLoS One 14:e0218143. doi: 10.1371/journal.pone.0218143
Hill, C., Guarner, F., Reid, G., Gibson, G. R., Merenstein, D. J., Pot, B., et al. (2014). Expert consensus document. The International Scientific Association for Probiotics and Prebiotics consensus statement on the scope and appropriate use of the term probiotic. Nat. Rev. Gastroenterol. Hepatol. 11, 506–514. doi: 10.1038/nrgastro.2014.66
Hussain, A., Mahawar, K., Xia, Z., Yang, W., and El-Hasani, S. (2020). Obesity and mortality of COVID-19. Meta-analysis. Obes. Res. Clin. Pract. 14, 295–300. doi: 10.1016/j.orcp.2020.07.002
Isaac, S. L., Abdul Malek, A. Z., Hazif, N. S., Roslan, F. S., Mohd Hashim, A., Song, A. A., et al. (2024). Genome mining of Lactiplantibacillus plantarum PA21: insights into its antimicrobial potential. BMC Genomics 25:571. doi: 10.1186/s12864-024-10451-7
Jain, C., Rodriguez, R. L., Phillippy, A. M., Konstantinidis, K. T., and Aluru, S. (2018). High throughput ANI analysis of 90K prokaryotic genomes reveals clear species boundaries. Nat. Commun. 9:5114. doi: 10.1038/s41467-018-07641-9
Jensen, L. J., Julien, P., Kuhn, M., von Mering, C., Muller, J., Doerks, T., et al. (2008). eggNOG: automated construction and annotation of orthologous groups of genes. Nucleic Acids Res. 36, D250–D254. doi: 10.1093/nar/gkm796
Jeung, W. H., Shim, J. J., Woo, S. W., Sim, J. H., and Lee, J. L. (2018). Lactobacillus curvatus HY7601 and Lactobacillus plantarum KY1032 cell extracts inhibit Adipogenesis in 3T3-L1 and HepG2 cells. J. Med. Food 21, 876–886. doi: 10.1089/jmf.2017.4157
Ji, Y., Chung, Y. M., Park, S., Jeong, D., Kim, B., and Holzapfel, W. H. (2019). Dose-dependent and strain-dependent anti-obesity effects of Lactobacillus sakei in a diet induced obese murine model. PeerJ 7:e6651. doi: 10.7717/peerj.6651
Jung, M., Jung, S., Kim, N., Ahn, H., Yun, H., and Kim, K. N. (2022). A randomized, double-blind, placebo-controlled trial to assess the efficacy and safety of Lactiplantibacillus plantarum CJLP243 in patients with functional diarrhea and high fecal calprotectin levels. Nutrients 14:389. doi: 10.3390/nu14020389
Kadooka, Y., Sato, M., Imaizumi, K., Ogawa, A., Ikuyama, K., Akai, Y., et al. (2010). Regulation of abdominal adiposity by probiotics (Lactobacillus gasseri SBT2055) in adults with obese tendencies in a randomized controlled trial. Eur. J. Clin. Nutr. 64, 636–643. doi: 10.1038/ejcn.2010.19
Kang, W., Pan, L., Peng, C., Dong, L., Cao, S., Cheng, H., et al. (2020). Isolation and characterization of lactic acid bacteria from human milk. J. Dairy Sci. 103, 9980–9991. doi: 10.3168/jds.2020-18704
Karlsson, C. L., Onnerfalt, J., Xu, J., Molin, G., Ahrne, S., and Thorngren-Jerneck, K. (2012). The microbiota of the gut in preschool children with normal and excessive body weight. Obesity 20, 2257–2261. doi: 10.1038/oby.2012.110
Kim, K. A., Gu, W., Lee, I. A., Joh, E. H., and Kim, D. H. (2012). High fat diet-induced gut microbiota exacerbates inflammation and obesity in mice via the TLR4 signaling pathway. PLoS One 7:e47713. doi: 10.1371/journal.pone.0047713
Kjaergaard, A. D., Teumer, A., Witte, D. R., Stanzick, K. J., Winkler, T. W., Burgess, S., et al. (2022). Obesity and kidney function: a two-sample Mendelian randomization study. Clin. Chem. 68, 461–472. doi: 10.1093/clinchem/hvab249
Kumar, S., Stecher, G., and Tamura, K. (2016). MEGA7: molecular evolutionary genetics analysis version 7.0 for bigger datasets. Mol. Biol. Evol. 33, 1870–1874. doi: 10.1093/molbev/msw054
Lambert, J. M., Bongers, R. S., de Vos, W. M., and Kleerebezem, M. (2008). Functional analysis of four bile salt hydrolase and penicillin acylase family members in Lactobacillus plantarum WCFS1. Appl. Environ. Microbiol. 74, 4719–4726. doi: 10.1128/aem.00137-08
Lee, S., Jang, J., Abe, S. K., Rahman, S., Saito, E., Islam, R., et al. (2022). Association between body mass index and oesophageal cancer mortality: a pooled analysis of prospective cohort studies with >800 000 individuals in the Asia cohort consortium. Int. J. Epidemiol. 51, 1190–1203. doi: 10.1093/ije/dyac023
Lee, Y. S., Park, E. J., Park, G. S., Ko, S. H., Park, J., Lee, Y. K., et al. (2021). Lactiplantibacillusplantarum ATG-K2 exerts an anti-obesity effect in high-fat diet-induced obese mice by modulating the gut microbiome. Int. J. Mol. Sci. 22:12665. doi: 10.3390/ijms222312665
Ley, R. E., Turnbaugh, P. J., Klein, S., and Gordon, J. I. (2006). Microbial ecology: human gut microbes associated with obesity. Nature 444, 1022–1023. doi: 10.1038/4441022a
Li, H., Liu, F., Lu, J., Shi, J., Guan, J., Yan, F., et al. (2020). Probiotic mixture of Lactobacillus plantarum strains improves lipid metabolism and gut microbiota structure in high fat diet-fed mice. Front. Microbiol. 11:512. doi: 10.3389/fmicb.2020.00512
Li, L., Stoeckert, C. J. Jr., and Roos, D. S. (2003). OrthoMCL: identification of ortholog groups for eukaryotic genomes. Genome Res. 13, 2178–2189. doi: 10.1101/gr.1224503
Luoto, R., Kalliomaki, M., Laitinen, K., and Isolauri, E. (2010). The impact of perinatal probiotic intervention on the development of overweight and obesity: follow-up study from birth to 10 years. Int. J. Obes. 34, 1531–1537. doi: 10.1038/ijo.2010.50
Martoni, C. J., Srivastava, S., Damholt, A., and Leyer, G. J. (2023). Efficacy and dose response of Lactiplantibacillus plantarum in diarrhea-predominant irritable bowel syndrome. World J. Gastroenterol. 29, 4451–4465. doi: 10.3748/wjg.v29.i28.4451
McFarland, L. V., Evans, C. T., and Goldstein, E. J. C. (2018). Strain-specificity and disease-specificity of probiotic efficacy: a systematic review and meta-analysis. Front. Med. 5:124. doi: 10.3389/fmed.2018.00124
Michael, D. R., Davies, T. S., Jack, A. A., Masetti, G., Marchesi, J. R., Wang, D., et al. (2021). Daily supplementation with the Lab4P probiotic consortium induces significant weight loss in overweight adults. Sci. Rep. 11:5. doi: 10.1038/s41598-020-78285-3
Mohammadian Khonsari, N., Khashayar, P., Shahrestanaki, E., Kelishadi, R., Mohammadpoor Nami, S., Heidari-Beni, M., et al. (2022). Normal weight obesity and cardiometabolic risk factors: a systematic review and meta-analysis. Front. Endocrinol. 13:857930. doi: 10.3389/fendo.2022.857930
Nasiri, G., Bastani, A., Haji-Aghamohammadi, A. A., Nooshabadi, M. R., Shahmirzalou, P., and Haghighian, H. K. (2021). Effects of probiotic and alpha-lipoic acid supplements, separately or in combination on the anthropometric indicators and maintenance of weight in overweight individuals. Clin. Nutr. ESPEN 41, 242–248. doi: 10.1016/j.clnesp.2020.12.007
Nordstrom, E. A., Teixeira, C., Montelius, C., Jeppsson, B., and Larsson, N. (2021). Lactiplantibacillus plantarum 299v (LP299V((R))): three decades of research. Benef. Microbes 12, 441–465. doi: 10.3920/BM2020.0191
Oh, S. J., Cho, Y. G., Kim, D. H., and Hwang, Y. H. (2023). Effect of Lactobacillus sakei OK67 in reducing body and visceral fat in lifestyle-modified overweight individuals: a 12-week, randomized, double-blind, placebo-controlled clinical trial. Nutrients 15:3074. doi: 10.3390/nu15133074
Oh, M. R., Jang, H. Y., Lee, S. Y., Jung, S. J., Chae, S. W., Lee, S. O., et al. (2021). Lactobacillus plantarum HAC01 supplementation improves glycemic control in prediabetic subjects: a randomized, double-blind, placebo-controlled trial. Nutrients 13:2337. doi: 10.3390/nu13072337
Opio, J., Croker, E., Odongo, G. S., Attia, J., Wynne, K., and McEvoy, M. (2020). Metabolically healthy overweight/obesity are associated with increased risk of cardiovascular disease in adults, even in the absence of metabolic risk factors: a systematic review and meta-analysis of prospective cohort studies. Obes. Rev. 21:e13127. doi: 10.1111/obr.13127
Ortolan, A., Lorenzin, M., Felicetti, M., and Ramonda, R. (2021). Do obesity and overweight influence disease activity measures in axial Spondyloarthritis? A systematic review and Meta-analysis. Arthritis Care Res. 73, 1815–1825. doi: 10.1002/acr.24416
Park, D. Y., Ahn, Y. T., Huh, C. S., Jeon, S. M., and Choi, M. S. (2011). The inhibitory effect of Lactobacillus plantarum KY1032 cell extract on the adipogenesis of 3T3-L1 cells. J. Med. Food 14, 670–675. doi: 10.1089/jmf.2010.1355
Park, S. Y., Cho, S. A., Kim, S. H., and Lim, S. D. (2014). Physiological characteristics and anti-obesity effect of Lactobacillus plantarum Q180 isolated from feces. Korean J. Food Sci. Anim. Resour. 34, 647–655. doi: 10.5851/kosfa.2014.34.5.647
Park, Y. E., Kim, M. S., Shim, K. W., Kim, Y. I., Chu, J., Kim, B. K., et al. (2020). Effects of Lactobacillus plantarum Q180 on postprandial lipid levels and intestinal environment: A double-blind, randomized, placebo-controlled, parallel trial. Nutrients 12:255. doi: 10.3390/nu12010255
Park, M., Park, E. J., Kim, S. H., and Lee, H. J. (2021). Lactobacillus plantarum ATG-K2 and ATG-K6 ameliorates high-fat with high-fructose induced intestinal inflammation. Int. J. Mol. Sci. 22:4444. doi: 10.3390/ijms22094444
Petrelli, F., Cortellini, A., Indini, A., Tomasello, G., Ghidini, M., Nigro, O., et al. (2021). Association of obesity with survival outcomes in patients with cancer: a systematic review and meta-analysis. JAMA Netw. Open 4:e213520. doi: 10.1001/jamanetworkopen.2021.3520
Phelps, N. H., Singleton, R. K., Zhou, B., Heap, R. A., Mishra, A., Bennett, J. E., et al. (2024). Worldwide trends in underweight and obesity from 1990 to 2022: a pooled analysis of 3663 population-representative studies with 222 million children, adolescents, and adults. Lancet 403, 1027–1050. doi: 10.1016/s0140-6736(23)02750-2
Pi-Sunyer, X., Astrup, A., Fujioka, K., Greenway, F., Halpern, A., Krempf, M., et al. (2015). A randomized, controlled trial of 3.0 mg of Liraglutide in weight management. N. Engl. J. Med. 373, 11–22. doi: 10.1056/NEJMoa1411892
Prakoeswa, C. R. S., Herwanto, N., Prameswari, R., Astari, L., Sawitri, S., Hidayati, A. N., et al. (2017). Lactobacillus plantarum IS-10506 supplementation reduced SCORAD in children with atopic dermatitis. Benef Microbes 8, 833–840. doi: 10.3920/BM2017.0011
Qin, P., Li, Q., Zhao, Y., Chen, Q., Sun, X., Liu, Y., et al. (2020). Sugar and artificially sweetened beverages and risk of obesity, type 2 diabetes mellitus, hypertension, and all-cause mortality: a dose-response meta-analysis of prospective cohort studies. Eur. J. Epidemiol. 35, 655–671. doi: 10.1007/s10654-020-00655-y
Quek, J., Chan, K. E., Wong, Z. Y., Tan, C., Tan, B., Lim, W. H., et al. (2023). Global prevalence of non-alcoholic fatty liver disease and non-alcoholic steatohepatitis in the overweight and obese population: a systematic review and meta-analysis. Lancet Gastroenterol. Hepatol. 8, 20–30. doi: 10.1016/S2468-1253(22)00317-X
Raeisi, T., Mozaffari, H., Sepehri, N., Darand, M., Razi, B., Garousi, N., et al. (2022). The negative impact of obesity on the occurrence and prognosis of the 2019 novel coronavirus (COVID-19) disease: a systematic review and meta-analysis. Eat. Weight Disord. 27, 893–911. doi: 10.1007/s40519-021-01269-3
Rahayu, E. S., Mariyatun, M., Putri Manurung, N. E., Hasan, P. N., Therdtatha, P., Mishima, R., et al. (2021). Effect of probiotic Lactobacillus plantarum Dad-13 powder consumption on the gut microbiota and intestinal health of overweight adults. World J. Gastroenterol. 27, 107–128. doi: 10.3748/wjg.v27.i1.107
Rawat, S. S., Narain, N. P., Marathe, S. M., Sonawale, S. B., and Veligandla, K. C. (2023). Early-life antibiotics and childhood obesity: yeast probiotics as a strategy to modulate gut microbiota. Cureus 15:e36795. doi: 10.7759/cureus.36795
Reid, T. J., and Korner, J. (2022). Medical and surgical treatment of obesity. Med. Clin. North Am. 106, 837–852. doi: 10.1016/j.mcna.2022.03.002
Sanders, M. E., Merenstein, D. J., Reid, G., Gibson, G. R., and Rastall, R. A. (2019). Probiotics and prebiotics in intestinal health and disease: from biology to the clinic. Nat. Rev. Gastroenterol. Hepatol. 16, 605–616. doi: 10.1038/s41575-019-0173-3
Scott, H. A., Ng, S. H., McLoughlin, R. F., Valkenborghs, S. R., Nair, P., Brown, A. C., et al. (2023). Effect of obesity on airway and systemic inflammation in adults with asthma: a systematic review and meta-analysis. Thorax 78, 957–965. doi: 10.1136/thorax-2022-219268
Shehata, M. G., El Sohaimy, S. A., El-Sahn, M. A., and Youssef, M. M. (2016). Screening of isolated potential probiotic lactic acid bacteria for cholesterol lowering property and bile salt hydrolase activity. Ann. Agric. Sci. 61, 65–75. doi: 10.1016/j.aoas.2016.03.001
Sohn, M., Jung, H., Lee, W. S., Kim, T. H., and Lim, S. (2023). Effect of Lactobacillus plantarum LMT1-48 on body fat in overweight subjects: a randomized, double-blind, placebo-controlled trial. Diabetes Metab. J. 47, 92–103. doi: 10.4093/dmj.2021.0370
Song, R. H., Wang, B., Yao, Q. M., Li, Q., Jia, X., and Zhang, J. A. (2019). The impact of obesity on thyroid autoimmunity and dysfunction: a systematic review and meta-analysis. Front. Immunol. 10:2349. doi: 10.3389/fimmu.2019.02349
Sophatha, B., Piwat, S., and Teanpaisan, R. (2020). Adhesion, anti-adhesion and aggregation properties relating to surface charges of selected Lactobacillus strains: study in Caco-2 and H357 cells. Arch. Microbiol. 202, 1349–1357. doi: 10.1007/s00203-020-01846-7
Stothard, P., and Wishart, D. S. (2005). Circular genome visualization and exploration using CGView. Bioinformatics 21, 537–539. doi: 10.1093/bioinformatics/bti054
Stsepetova, J., Ratsep, M., Gerulis, O., Joesaar, A., Mikelsaar, M., and Songisepp, E. (2023). Impact of Lactiplantibacillus plantarum Inducia on metabolic and antioxidative response in cholesterol and BMI variable indices: randomised, double-blind, placebo-controlled trials. Benef. Microbes 14, 1–15. doi: 10.3920/BM2022.0030
Sun, Y., Zhang, S., Li, H., Zhu, J., Liu, Z., Hu, X., et al. (2022). Assessments of probiotic potentials of Lactiplantibacillus plantarum strains isolated from Chinese traditional fermented food: phenotypic and genomic analysis. Front. Microbiol. 13:895132. doi: 10.3389/fmicb.2022.895132
Vajro, P., Mandato, C., Licenziati, M. R., Franzese, A., Vitale, D. F., Lenta, S., et al. (2011). Effects of Lactobacillus rhamnosus strain GG in pediatric obesity-related liver disease. J. Pediatr. Gastroenterol. Nutr. 52, 740–743. doi: 10.1097/MPG.0b013e31821f9b85
Wang, X., Huang, Y., Chen, Y., Yang, T., Su, W., Chen, X., et al. (2022). The relationship between body mass index and stroke: a systemic review and meta-analysis. J. Neurol. 269, 6279–6289. doi: 10.1007/s00415-022-11318-1
WHO (1997). Obesity and overweight. Available at: http://www.https://www.who.int/health-topics/obesity#tab=tab_1
Wick, R. R., Judd, L. M., Gorrie, C. L., and Holt, K. E. (2017). Unicycler: resolving bacterial genome assemblies from short and long sequencing reads. PLoS Comput. Biol. 13:e1005595. doi: 10.1371/journal.pcbi.1005595
Wilding, J. P. H., Batterham, R. L., Calanna, S., Davies, M., Van Gaal, L. F., Lingvay, I., et al. (2021). Once-weekly semaglutide in adults with overweight or obesity. N. Engl. J. Med. 384, 989–1002. doi: 10.1056/NEJMoa2032183
World Obesity Federation (2022). World_Obesity_Atlas_2022. Available at: https://s3-eu-west-1.amazonaws.com/wof-files/World_Obesity_Atlas_2022.pdf
Yanovski, S. Z., and Yanovski, J. A. (2014). Long-term drug treatment for obesity: a systematic and clinical review. JAMA 311, 74–86. doi: 10.1001/jama.2013.281361
Yu, H. J., Ho, M., Liu, X., Yang, J., Chau, P. H., and Fong, D. Y. T. (2022). Association of weight status and the risks of diabetes in adults: a systematic review and meta-analysis of prospective cohort studies. Int. J. Obes. 46, 1101–1113. doi: 10.1038/s41366-022-01096-1
Zhang, Z., Man, C., Sun, L., Yang, X., Li, M., Zhang, W., et al. (2019). Short communication: complete genome sequence of Lactobacillus plantarum J26, a probiotic strain with immunomodulatory activity. J. Dairy Sci. 102, 10838–10844. doi: 10.3168/jds.2019-16593
Zhang, Z., Tao, X., Shah, N. P., and Wei, H. (2016). Antagonistics against pathogenic Bacillus cereus in milk fermentation by Lactobacillus plantarum ZDY2013 and its anti-adhesion effect on Caco-2 cells against pathogens. J. Dairy Sci. 99, 2666–2674. doi: 10.3168/jds.2015-10587
Zhang, M., Wang, C., Wang, C., Zhao, H., Zhao, C., Chen, Y., et al. (2015). Enhanced AMPK phosphorylation contributes to the beneficial effects of Lactobacillus rhamnosus GG supernatant on chronic-alcohol-induced fatty liver disease. J. Nutr. Biochem. 26, 337–344. doi: 10.1016/j.jnutbio.2014.10.016
Zhang, S., Wang, T., Zhang, D., Wang, X., Zhang, Z., Lim, C., et al. (2022). Probiotic characterization of Lactiplantibacillus plantarum HOM3204 and its restoration effect on antibiotic-induced dysbiosis in mice. Lett. Appl. Microbiol. 74, 949–958. doi: 10.1111/lam.13683
Keywords: Lactiplantibacillus plantarum, complete genome sequence, 3T3-L1 cells, anti-obesity effect, high-fat diet
Citation: Wang T, Zhang X, Fan L, Zhao Y, Zhang Z, Cao Z, Xu Y, Lee S, Lim C and Zhang S (2024) Complete genome sequence and anti-obesity potential of Lactiplantibacillus plantarum HOM2217 in 3T3-L1 cells and high-fat diet-fed rats. Front. Microbiol. 15:1436378. doi: 10.3389/fmicb.2024.1436378
Edited by:
Alex Galanis, Democritus University of Thrace, GreeceReviewed by:
Konstantinos Papadimitriou, Agricultural University of Athens, GreeceMyeong Soo Park, BIFIDO Co., Ltd, Republic of Korea
Copyright © 2024 Wang, Zhang, Fan, Zhao, Zhang, Cao, Xu, Lee, Lim and Zhang. This is an open-access article distributed under the terms of the Creative Commons Attribution License (CC BY). The use, distribution or reproduction in other forums is permitted, provided the original author(s) and the copyright owner(s) are credited and that the original publication in this journal is cited, in accordance with accepted academic practice. No use, distribution or reproduction is permitted which does not comply with these terms.
*Correspondence: Shiqi Zhang, emhhbmdzaGlxaTFAYmpoYW5taS5jb20uY24=