- 1School of Medicine and Pharmacy, Ocean University of China, Qingdao, China
- 2Women and Children Hospital, Qingdao University, Qingdao, China
- 3Qingdao Central Hospital, University of Health and Rehabilitation Sciences, Qingdao, China
- 4Department of Clinical and Toxicological Analysis, Faculty of Pharmaceutical Sciences, Universidade de São Paulo, São Paulo, Brazil
- 5Department of Statistics, Universidade Estadual de Maringá, Maringá, Paraná, Brazil
Background: The combination antimicrobial therapy consisting of amikacin, polymyxin-B, and sulbactam demonstrated in vitro synergy against multi-drug resistant Acinetobacter baumannii.
Objectives: The objectives were to predict drug disposition and extrapolate their efficacy in the blood, lung, heart, muscle and skin tissues using a physiologically-based pharmacokinetic (PBPK) modeling approach and to evaluate achievement of target pharmacodynamic (PD) indices against A. baumannii.
Methods: A PBPK model was initially developed for amikacin, polymyxin-B, and sulbactam in adult subjects, and then scaled to pediatrics, accounting for both renal and non-renal clearances. The simulated plasma and tissue drug exposures were compared to the observed data from humans and rats. Efficacy was inferred using joint probability of target attainment of target PD indices.
Results: The simulated plasma drug exposures in adults and pediatrics were within the 0.5 to 2 boundary of the mean fold error for the ratio between simulated and observed means. Simulated drug exposures in blood, skin, lung, and heart were consistent with reported penetration ratio between tissue and plasma drug exposure. In a virtual pediatric population from 2 to <18 years of age using pediatric dosing regimens, the interpretive breakpoints were achieved in 85–90% of the population.
Conclusion: The utility of PBPK to predict and simulate the amount of antibacterial drug exposure in tissue is a practical approach to overcome the difficulty of obtaining tissue drug concentrations in pediatric population. As combination therapy, amikacin/polymyxin-B/sulbactam drug concentrations in the tissues exhibited sufficient penetration to combat extremely drug resistant A. baumannii clinical isolates.
1 Introduction
The overuse of antibiotics has led to the emergence of multidrug-resistant (MDR) Acinetobacter baumannii, which made treatment of related infections increasingly difficult; consequently, antibiotic monotherapies are no longer effective in the clinic (Oo and Sy, 2020; Oo et al., 2023). With increasing incidences of MDR A. baumannii, polymyxins became the last-line antibiotic agent (Li and Nation, 2006; Li et al., 2006). Polymyxin-B combination with other antibiotics exhibited better efficacy against MDR A. baumannii than individual antibiotic monotherapies (Rao et al., 2016; Liu et al., 2023). Our laboratory previously evaluated the in vitro synergistic activities of polymyxin-B, amikacin and sulbactam combination therapy against 11 MDR A. baumannii and showed that this triple combination restricted the mutant selection window and reduced the opportunity for the bacteria to develop further resistance (Zhu et al., 2022a). Using metabolomic profiling, we further demonstrated that this triple antibiotic combination significantly disrupted cellular outer membrane structures, fatty acids, glycerophospholipids, nucleotide and peptide metabolic pathways in MDR A. baumannii (Zhu et al., 2023). Sulbactam and polymyxin-B disrupted the integrity and stability of the cell wall and outer membrane by affecting peptidoglycans and lipopolysaccharides (Zhang and Rock, 2008), allowing companion antibiotics such as amikacin to enter the bacteria cell and inhibit protein synthesis that leads to cell death (Taber et al., 1987; Kotra et al., 2000).
The interpretive criteria for antimicrobial susceptibility are based on drug exposures in the blood, as it is difficult to obtain drug exposure data in the tissues. A. baumannii infections in critically ill patients in intensive care unit are often due to pneumonia, endocarditis, skin and bloodstream infections (Sopirala et al., 2008; Lagana et al., 2015; Abdi et al., 2020); these infections often occur in tissues where drug concentrations are often lower than that in the blood. Physiologically-based pharmacokinetic (PBPK) models provide a non-invasive alternative to extrapolate drug efficacy in the infected tissues (Martins et al., 2020; Martins et al., 2021; Zhu et al., 2022b; Martins et al., 2023; Zhang et al., 2023) and provides the necessary tool to evaluate sufficiency of exposure in the target organs.
Even though the pharmacokinetics of polymyxin-B, amikacin, and sulbactam have been reported in pediatrics (Kafetzis et al., 1979; Schaad et al., 1986; Xu et al., 2022), drug exposure data were lacking in the above tissues. In this study, we developed robust PBPK models and linked them to an exposure-based pharmacodynamic (PD) assessment to explore the adequacy of drug exposure in these organs. The present study aimed to predict drug exposure in the blood, lung, skin, and heart using clinical dosing regimens of polymyxin-B, amikacin and sulbactam for combination therapy.
2 Materials and methods
2.1 Clinical data used for PBPK model development and evaluation
The model of sulbactam was developed and evaluated in our previous study (Zhu et al., 2022b); it was not thoroughly evaluated in the current study. The clinical data including pharmacokinetic profiles and exposure parameters of polymyxin-B and amikacin were obtained from search results in Web of Science and PubMed databases. The demographic information in the literature was summarized by gender, age, weight, renal function, dosing regimen and drug exposure. Chart extraction data tool, Web Plot Digitizer (version 4.5 https://automeris.io/wpd) was used to extract drug exposure data from the literature. Those extracted data were from critically ill patients and healthy volunteers, who received either single or multiple dosing regimens via intravenous bolus or infusion and were used to optimize key parameters of the PBPK model. Adult PBPK model was developed, qualified and then scaled to the pediatric population. The extracted data of pediatrics from the literature were used to validate the pediatric PBPK model.
2.2 The development of adult PBPK model
The PBPK software, PK-Sim® (Version 10.0; part of the Open Systems Pharmacology Suite, https://www.open-systems-pharmacology.org), was used to develop the adult model of polymyxin-B and amikacin. The standard distribution model assumes four sub-compartments per organ, which included compartments for blood cells, plasma, interstitial space, and intracellular space (Bischoff, 1986). Interstitial fluid in tissues is the medium for infection transmission, and also the medium for antibiotics to be distributed at the infection site (Segal et al., 1990); the tissue drug concentrations we assessed were taken from the interstitial fluid compartment. We used the parameter identification module to optimize and then select the partition coefficient method.
The physicochemical characteristics and physiological parameters of polymyxin-B and amikacin applied in model development were obtained from drug bank1 and the literature as listed in Table 1. Demographical information was applied in the model development including age, gender, and weight, in addition to dosing regimens and plasma concentration-time profiles. In order to ensure consistency of the simulated drug exposure distribution in the tissues with those reported in the literature, we adjusted the standard deviation of the partition coefficients by comparing the inter-individual variability of drug tissue exposure with that reported in the literature (Zhu et al., 2022b).
The clearance of polymyxin-B was minimally affected by renal function. Consequently, the dosing regimens of polymyxin-B were not adjusted based on renal function. Less than 1% unchanged polymyxin-B was recovered in human urine; due to tubular reabsorption, the net renal clearance was in the range of 0.00032–0.0039 mL/min/kg in humans (Zavascki et al., 2007; Zavascki et al., 2008; Manchandani et al., 2016). The specific mechanism for its metabolism is still not fully understood (Avedissian et al., 2019); the non-renal clearance parameter of the polymyxin-B model was set as its total clearance and then optimized in PK-Sim (Zavascki et al., 2008; Sandri et al., 2013a; Sandri et al., 2013b; Burkin et al., 2021; Xu et al., 2022). Biliary excretion could be one of the elimination routes, but the value was not provided in the literature (Manchandani et al., 2016); biliary elimination was optimized by the parameter identification module in PK-Sim.
Amikacin is the second most commonly used antibiotic in neonatal intensive care units (Spitzer et al., 2010). Like other aminoglycosides, amikacin is primarily eliminated by glomerular filtration; a high recovery rate of its unchanged form was detected in the urine (Lanao et al., 1981). The model assumed no hepatic metabolism and renal clearance is the primary route of elimination. The reported clearance of amikacin was used in the model (Vogelstein et al., 1977; Bauer et al., 1980; Lanao et al., 1981; Garraffo et al., 1990; Vanhaeverbeek et al., 1993; Mahmoudi et al., 2013). The physiological parameters of amikacin are listed in Table 1.
To account for changes in amikacin clearance in human population with renal insufficiency, the renal physiological parameters including renal blood flow, kidney volume, hematocrit, small intestinal transit and renal perfusion were adjusted accordingly (Malik et al., 2020).
The potential risk of drug–drug interactions was considered in combination antibiotic therapy. Amikacin and sulbactam have a high renal clearance rate, while polymyxin B is primarily reabsorbed in the renal tubular cells and cleared through non-renal pathways. These drugs are not metabolized by the liver and drug–drug interaction affecting their pharmacokinetics is unlikely. However, nephrotoxicity is a concern for the combination therapy. Wang et al. reported that the amikacin/polymyxin B combination did not lead to acute kidney injury during a 30-day treatment period (Wang et al., 2021). Furthermore, in vitro susceptibility results indicated that combining these three antibiotics significantly reduced the drug concentration required to combat multidrug-resistant A. baumannii, while monotherapy would likely result in therapeutic failure.
2.3 Adult PBPK model evaluation
The performance of the simulations for the two antibiotics was assessed via the mean fold error (MFE, Equation 1) (Biesdorf et al., 2019):
MFE was performed by comparing simulated to observed maximum drug concentration (Cmax) and the area under the concentration-time curve (AUC). The PBPK models for adults and pediatrics were accepted when the predicted to observed PK data were within 2-fold range (i.e., 0.5 ≤ MFE ≤ 2.0).
The observed Cmax and AUC were extracted from pharmacokinetic profiles reported in the literature. A virtual population containing 100 subjects with a 50:50 male-to-female ratio was established for the three antibiotics to simulate their blood and tissue drug concentrations.
2.4 Pediatric PBPK model development and evaluation
2.4.1 Physiological parameters in the pediatric population
In general, renal function is considered fully developed by 2 years of age. For polymyxin-B, the renal function does not affect its clearance whereas the clearances of amikacin and sulbactam are influenced by renal function. Given that the incidence of renal function impairment in pediatrics is low, a virtual pediatric population from 0 to 17 years was established assuming normal renal function. Both renal and non-renal eliminations were scaled by age-dependent maturation of organ weight.
Anatomic and physiological parameters for pediatrics such as organ volumes, and composition, blood flows, protein binding and maturation of elimination processes were used in the pediatric virtual population development. These parameters were summarized from previous studies and incorporated into PK-Sim’s ontogeny database (Poulin et al., 2001; Valentin, 2002; Ince et al., 2019). Weight-based dosing is used for pediatrics. The inter-individual variability of body weight by age and gender of pediatrics was compared to an age-matched polynomial function of body weight distribution in the literature (Sy et al., 2014). In addition, a method for protein binding prediction in small children was used to predict the unbound fraction of the antibiotics (McNamara and Alcorn, 2002).
Ince et al. (2021) established and validated a PBPK model for 10 small molecule compounds in adults, including amikacin and successfully extrapolated it to pediatric populations; however, they did not investigate drug exposure in the tissues. Claassen et al. (2015) incorporated factors such as renal and hepatic maturation (including individual hepatic enzyme development) into a PBPK model for premature infants, evaluating the model’s performance for amikacin and paracetamol. They compared the predicted plasma concentration-time profiles of the two drugs with observed in vivo data in the blood and simulated concentrations across a wide range of gestational and postnatal ages, providing reference information for clinical use in premature pediatric populations (Claassen et al., 2015). Darlow et al. (2024) established a PBPK model for amikacin and extended it to neonates to evaluate the achievement of pharmacodynamic targets. This model only predicted plasma drug concentration for 15 mg/kg q24h dosing in neonates aged 0–28 days.
The amikacin PBPK model developed in the current study provided an in-depth evaluation of drug penetration in various tissues (blood, lung, heart, and skin) under dosing regimens of 7.5 mg/kg q12h and 15 mg/kg q12h. Compared to previous models, this model offers a more detailed prediction of drug distribution in tissues, providing new insights into drug distribution in critical infection sites such as the lungs and heart.
The current model shares foundational physiological modeling frameworks with existing PBPK models, including drug distribution between organs and metabolic pathways. These are fundamental building blocks of PBPK modeling and are thoroughly utilized in this study.
2.4.2 Pediatric dosing regimens
The recommended IV dosing regimens of polymyxin-B were 1.5–2.5 mg/kg/day for both adults and pediatrics (FDA, 2011). A loading dose and higher maintenance dose of up to 3 mg/kg/day were often used in clinical practice when treating MDR bacterial infection. When MIC was less than 0.5 mg/L, the dosage of 1.5–3.0 mg/kg/day could achieve over 90% probability of target attainments (PTA) in pediatric patient (Wang et al., 2022).
Nephrotoxicity is the main factor to consider when amikacin is administered to patients. The recommended dosing regimen of amikacin for patients with normal renal function is 15 mg/kg/day once-daily or divided in two or three equal doses (Perez-Blanco et al., 2021). At this dose, the trough concentration is maintained at <10 μg/mL in majority of the patients to minimize toxicity. EUCAST has recently changed antimicrobial susceptibility endpoints and increased dosing recommendation up to 30 mg/kg/day (EUCAST, 2020a); no increased toxicity for amikacin administered at higher doses (25–30 mg/kg/day) than the standard 15 mg/kg/day has been shown in specific populations including severe sepsis and critically ill patients (Galvez et al., 2011; de Montmollin et al., 2014; Kato et al., 2017; Perez-Blanco et al., 2021; Frost et al., 2023).
The dosing regimen of sulbactam in pediatrics was utilized according to our previous study (Zhu et al., 2022a). Both amikacin and sulbactam in pediatrics were grouped by pediatric body weight assuming normal renal function. Table 2 shows the dosing regimens for polymyxin-B, amikacin and sulbactam used in the pediatric population.
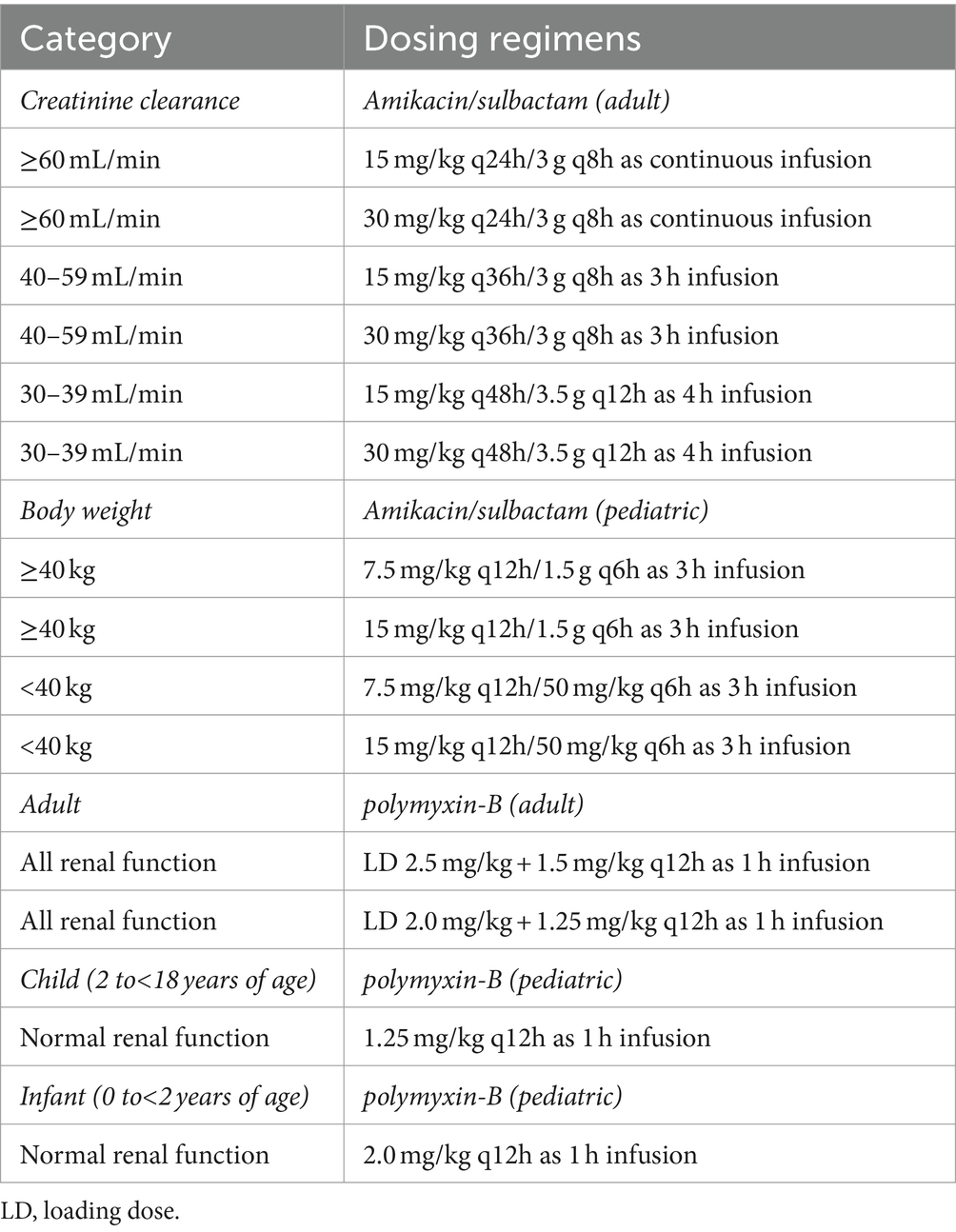
Table 2. Dosing regimens of amikacin/sulbactam/polymyxin-B used in simulation by creatinine clearance and by body weight categories.
2.5 Tissue drug concentrations and penetration rates
Drug concentrations in tissues including blood, lung, heart and skin for the three antibiotics were simulated and predict by PBPK model in both pediatric and adult populations. The penetration rate was determined by comparing the Cmax and AUC of each tissue to that in the blood. To ensure the accuracy of our simulation results, we compared the results with those reported in the literature.
2.6 Pharmacodynamic indices and probability of target attainment
For antibiotic pharmacodynamics (PD), the effectiveness of a drug is closely related to its exposure at the infection site. Antibiotics are generally classified into three categories: time-dependent, concentration-dependent, and time-dependent with a long post-antibiotic effect (PAE) (Sy et al., 2016).
The bactericidal effect of time-dependent antibiotics depends on the duration of time the free drug concentration exceeds the minimum inhibitory concentration (MIC) of the bacteria. The PD index for these antibiotics is the percentage of time the drug concentration remains above MIC during the dosing interval (fT > MIC). The PD index of sulbactam is 40% fT > MIC against lung infection model of A. baumannii (Yokoyama et al., 2015). The effectiveness of these antibiotics relies on maintaining an effective concentration for a sufficient period to ensure antibacterial activity.
For time-dependent antibiotics with a long PAE, the PD index is the ratio of the free drug exposure over 24 h to MIC (fAUC0–24/MIC). The killing effect of polymyxin B is associated with fAUC0–24/MIC index. An fAUC/MIC range of 8.2–42.1 was associated with a 2 log kill in the lung infection model (Dudhani et al., 2010; Sandri et al., 2013a; Zhu et al., 2022b). Thus, fAUC/MIC ≥8.2 was selected as the PD index of polymyxin B against A. baumannii.
The antibacterial effect of concentration-dependent antibiotics is related to the level of the free drug concentration. The higher the concentration, the better the bactericidal effect. The PD index for these drugs can be the peak drug concentration (Cmax) to MIC ratio (fCmax/MIC) or fAUC0–24/MIC. For amikacin, the fCmax/MIC target value should be at least 8 for a satisfactory therapeutic effect, and fAUC/MIC ≥80 is also recommended (Moore et al., 1987; Rybak et al., 1999; Darlow et al., 2024).
In the case of monotherapy, the probability of target attainment (PTA) was defined as the proportion of the simulated concentration-time profiles that could achieve the target indices. For combination therapy, we have previously proposed that the joint PTA be the minimum of the individual PTAs of the antibiotics in the combination. A sufficient probability of success is when the MICs of each drug in the combination are associated with ≥90% PTA (Menegucci et al., 2019; Martins et al., 2021). The PD index and PTA analyses were carried out using user-defined functions in R (version 4.1.2).
3 Results
3.1 PBPK model qualification in adult and pediatric populations
The PBPK model development and evaluation for polymyxin-B, amikacin and sulbactam were sourced from 5, 10, and 4 reports containing 5, 11, and 6 populations, respectively; 17, 285, and 70 observations of polymyxin-B, amikacin and sulbactam PK profiles, respectively, from adults and pediatrics were utilized for model verification (Supplementary Tables S1–S3). The disease statuses of patients used in the development of PBPK models are listed in Supplementary Table S4. The collection of PK data used for PBPK model development came from diverse medical conditions. The observed data extracted from the literature for polymyxin-B, amikacin and sulbactam were within the 95% prediction interval of the corresponding simulations (Supplementary Figure S1). The two key exposure parameters, Cmax and AUC, for adult and pediatric simulation were all within the MFE boundary of 0.5 to 2.0 compared to observed data (Figure 1).
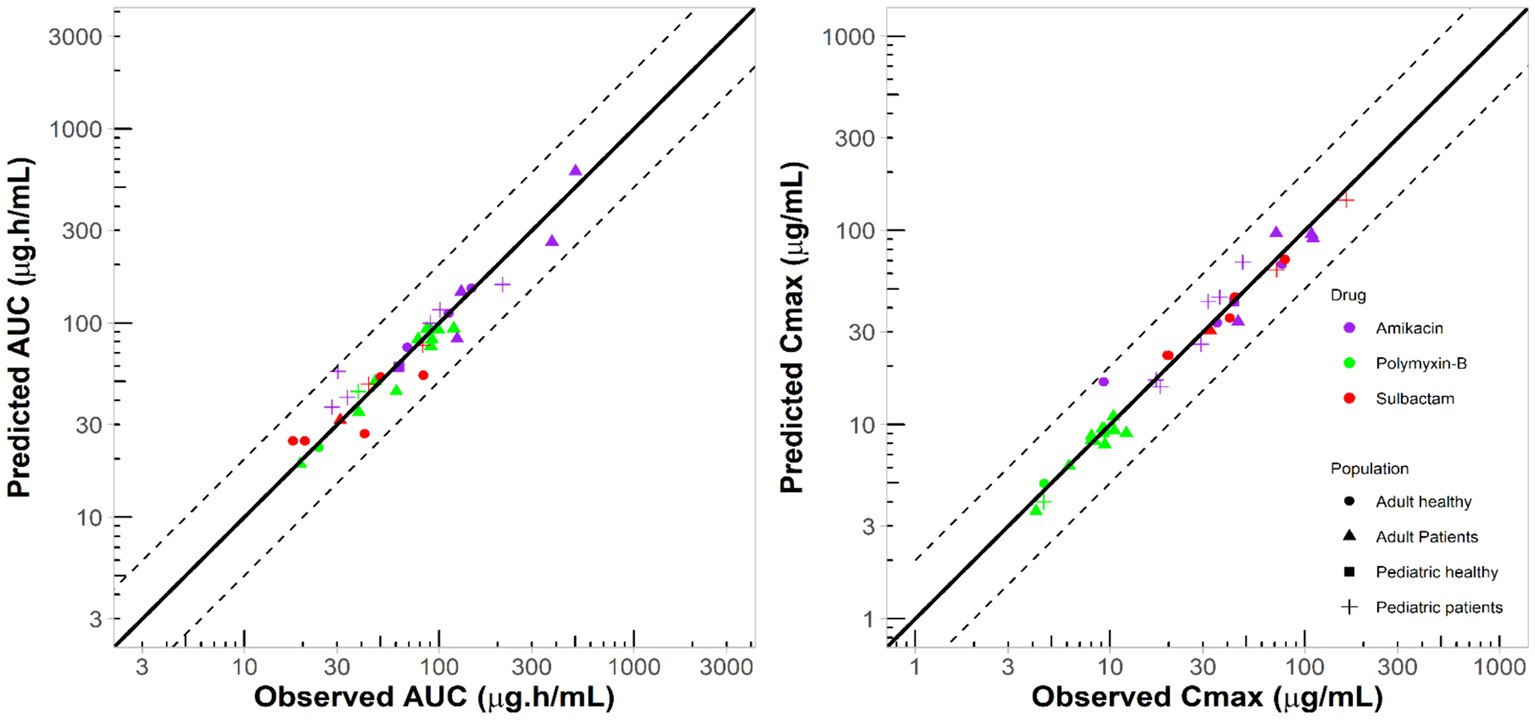
Figure 1. Comparison between simulated and observed exposure parameters from several studies in the literature for different populations. Solid lines represent line of unity; dashed lines represent two-fold difference.
3.2 Tissue exposures
We compared the PBPK model to the population PK model for adult dosing regimen by renal function and evaluated the interindividual variability in pharmacokinetic profiles (Supplementary Figures S2, S3). The interstitial fluid compartment is the site where we simulated representative tissue drug concentrations. The penetration rates (computed as AUC ratio or Cmax ratio for tissue/plasma) from the simulation and the literature were compared to evaluate the accuracy of the model.
The penetration ratios of amikacin in heart, lung and skin were 1.10–1.24, 0.49–0.58, and 0.41–0.46, respectively (Tables 3, 4). The cardiac partition coefficient was adjusted to reflect higher exposure of amikacin in the heart. The literature reported values were as follows: heart tricuspid, 1.15–1.27; lung alveolar, 0.50; skin blister fluid, 0.54 (Lanao et al., 1983; Najmeddin et al., 2020; Shin et al., 2024). Sulbactam penetration rates in the heart, lung and skin were 0.50–0.51, 0.58–0.59 and 0.28–0.31, respectively (Tables 3, 4). The pulmonary penetration ratio was 52% following IV administration in healthy adult subjects (Rodvold et al., 2018). For other organs, the penetration ratios of sulbactam were not reported for humans.
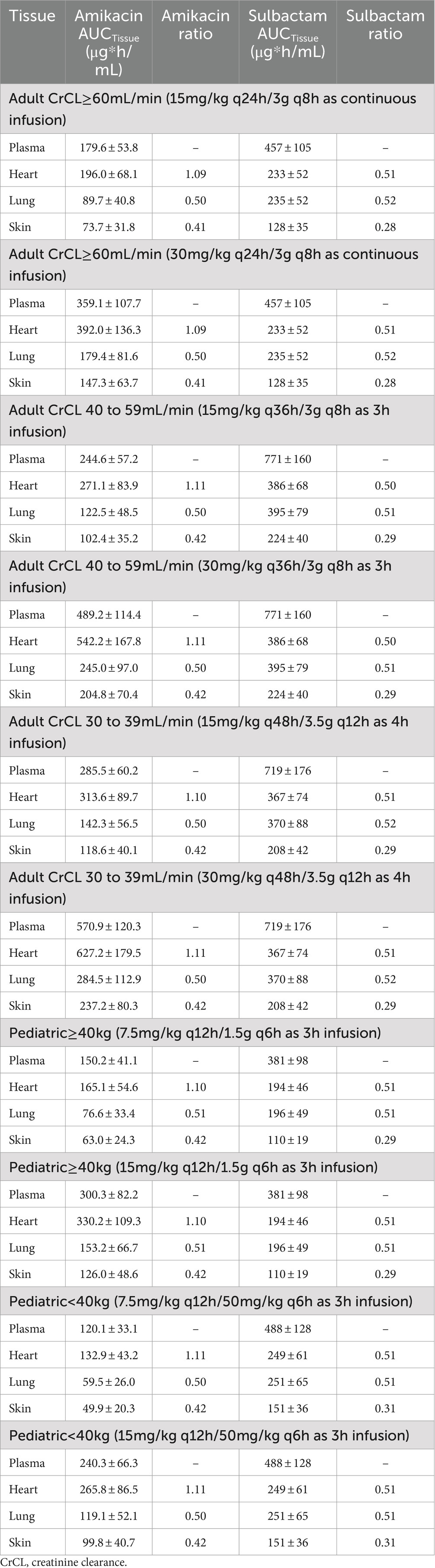
Table 3. Simulated steady-state AUC0–24h in various tissues and AUC ratio comparing tissue to plasma exposure of amikacin and sulbactam in adults and pediatrics 2 to <18 years of age.
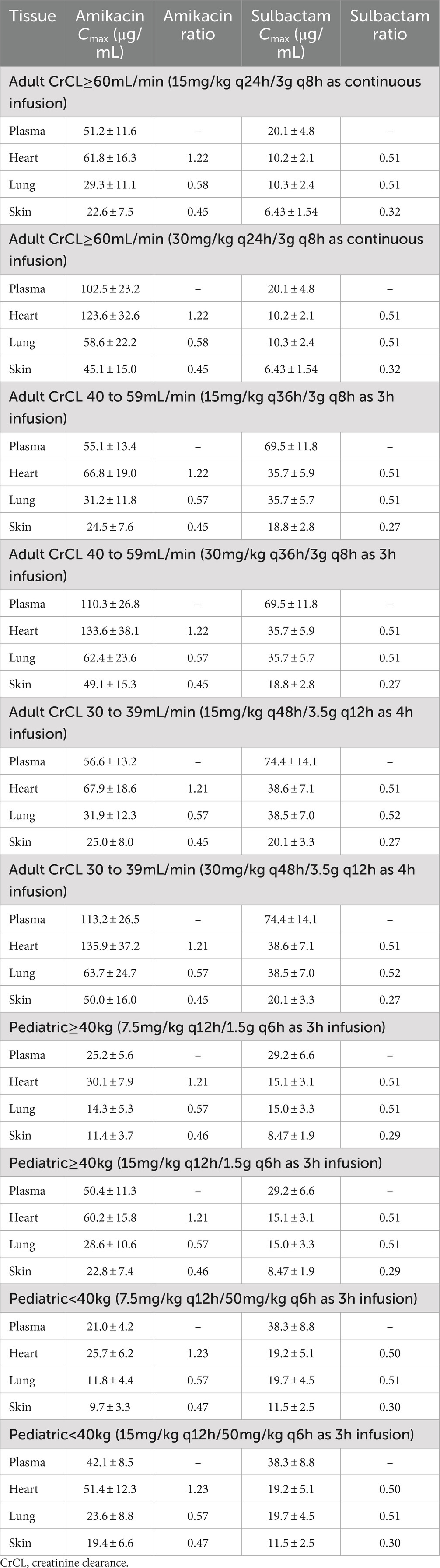
Table 4. Simulated steady-state Cmax in various tissues and Cmax ratio comparing tissue to plasma exposure of amikacin and sulbactam in adults and pediatrics 2 to <18 years of age.
For polymyxin-B, these values were 0.980–1.05, 2.80–2.96, and 1.46–1.53 (Tables 5, 6). The penetration ratios of polymyxin-B in the heart and lungs were 1.03–1.12 and 1.93–3.38 in the rat model, respectively (Manchandani et al., 2016).
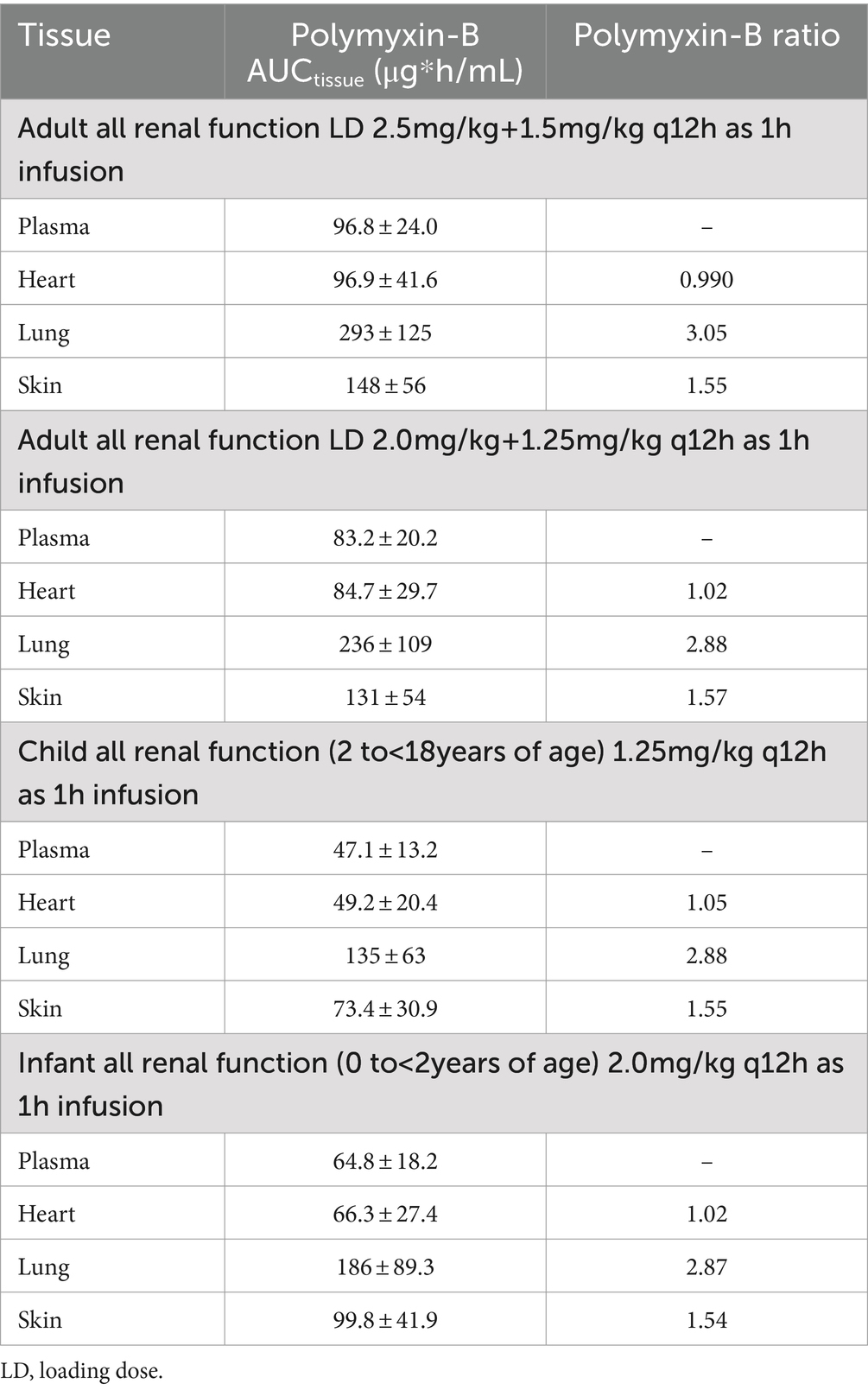
Table 5. Simulated steady-state AUC0–24h in various tissues and AUC ratio comparing tissue to plasma exposure of polymyxin-B in adults and pediatrics 2 to <18 years of age.
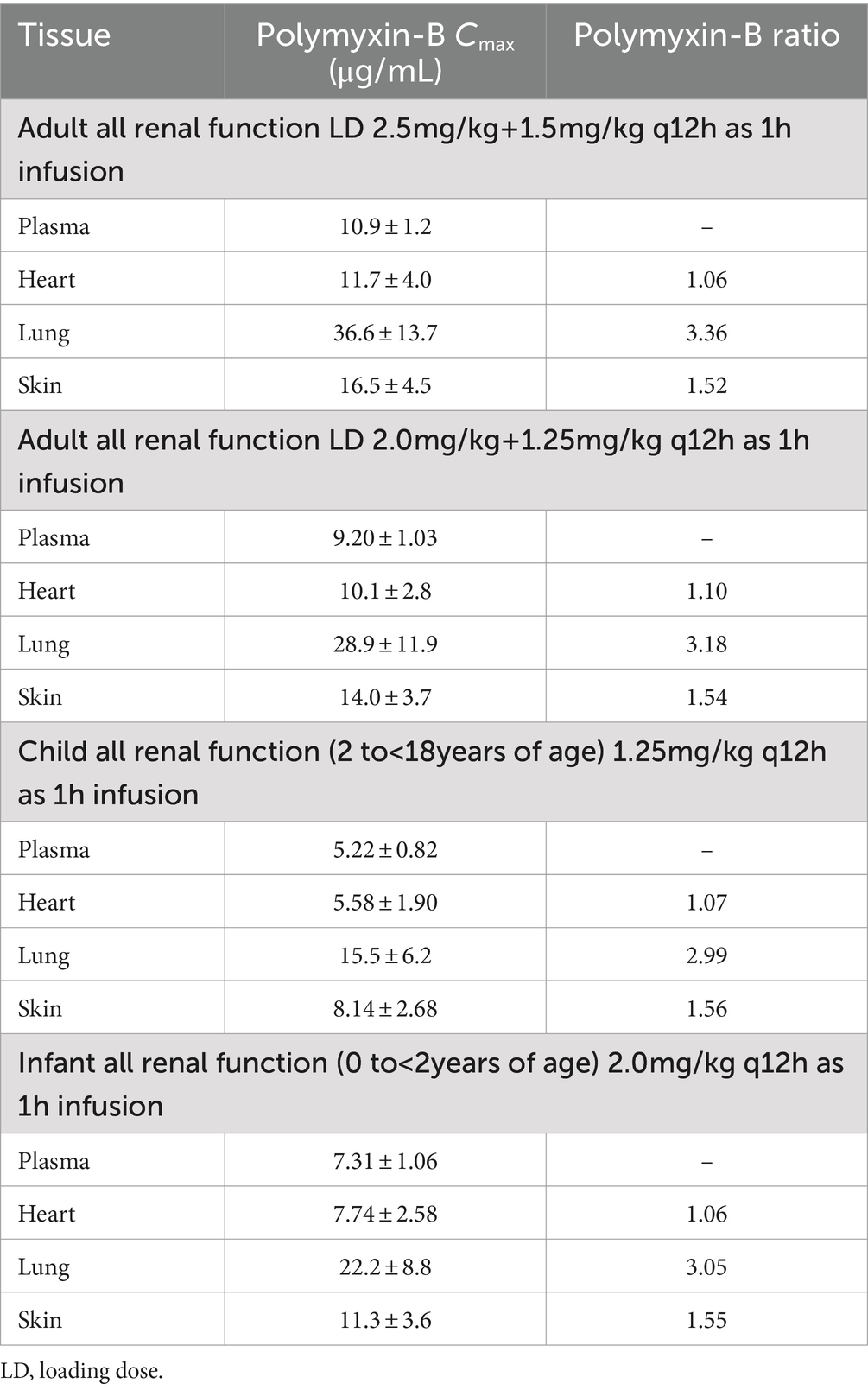
Table 6. Simulated steady-state Cmax in various tissues and Cmax ratio comparing tissue to plasma exposure of polymyxin-B in adults and pediatrics 2 to <18 years of age.
The standard deviation of the partition coefficients of tissue/plasma for polymyxin-B and amikacin in PK-Sim were adjusted and the resulting coefficient of variation (CV) of tissue Cmax and AUC were also compared to that reported in the literature. For amikacin, the CV% of simulated Cmax in the blood ranged from 20.6 to 23.7%, which was consistent with the reported CV% or Cmax of 23.0% (Najmeddin et al., 2020). Since amikacin exposure in the heart mirrors that in the blood, the CV% in the heart tissue ranged from 24.1 to 28.3%, similar to those in the blood. In the lung and skin, the CV% ranged from 37.2 to 38.6% and 30.9 to 34.3%, respectively. These values were also consistent with the reported CV% or Cmax, which were 38.6 and 38.8%, respectively in the same tissues (Lanao et al., 1983; Bayer et al., 1988). There are no reported exposure parameters of polymyxin-B in human tissues or body fluids other than the blood. Instead, we compared the CV values to those reported in the rat; the CV of polymyxin-B in the rat’s heart and lung were 31.6–51.2% and 30.8–75.3%, respectively (Manchandani et al., 2016). The corresponding CV values from our PBPK simulations in humans were 31.3–33.6% and 33.0–42.1% for the same respective organs. As for sulbactam, the variability due to PBPK simulation in the lung was 14.7–22.9%, which is close to the corresponding CV of 29.6% from the literature (Rodvold et al., 2018).
3.3 Probability of target attainment (PTA) for pediatric dosing regimens
The interpretive criteria for polymyxin-B against A. baumannii are the following: intermediate, MIC ≤2 μg/mL; and resistant, MIC ≥4 μg/mL (Satlin et al., 2020; CLSI, 2024). We assumed that the target pharmacodynamic index for polymyxin-B (i.e., fAUC/MIC≥8.2) is the same for the blood and other tissues. The IV administration of polymyxin-B in the pediatric population was simulated to evaluate drug concentrations in pediatric tissues. The results in Figure 2 showed that in the blood, dosing regimens consisting of a loading dose (LD) 2.5 mg/kg plus maintenance dose (MD) 1.5 mg/kg q12h in adults and 2 mg/kg q12h in pediatrics (0 to <2 years old) were able to achieve more than 90% PTA for an MIC of ≤4 μg/mL whereas LD 2.0 + 1.25 mg/kg q12h in adults and 1.25 mg/kg q12h in pediatric (2 to <18 years old) achieved similar results for ≤2 μg/mL MIC. In cardiac tissues, all dosing regimens achieved more than 90% PTA at ≤4 μg/mL MIC, and pediatric (0 to <2 years old) 2 mg/kg q12h achieved more than 90% PTA at ≤8 μg/mL MIC. In the lung, an adequate coverage (PTA ≥85%) was achieved at ≤4 μg/mL MIC for all listed regimens. In the skin, all dosing regiments achieved >85% PTA at ≤8 μg/mL MIC. Polymyxin-B adult and pediatric dosing regimens have comparable exposures.
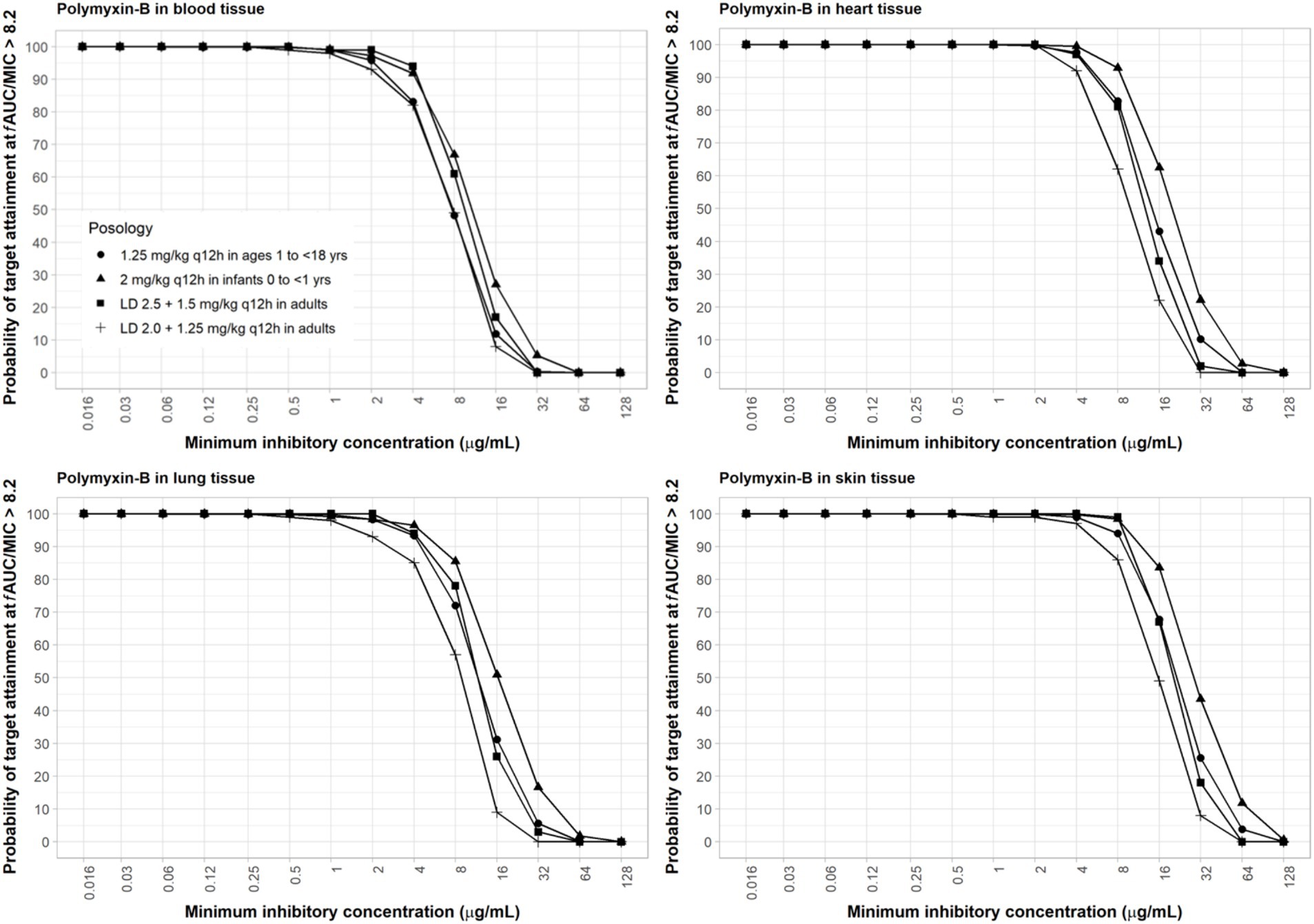
Figure 2. Probability of target attainment of polymyxin-B PD index of fAUC/MIC of at least 8.2 in the blood, lung, heart, and skin for adult and pediatric dosing regimens.
Amikacin approved dosing regimen is 15 mg/kg once-daily or 7.5 mg/kg twice-daily. A revised dosing of 25–30 mg/kg once daily has been recommended by EUCAST, given the risk of sub-therapeutic drug concentrations with the 15 mg/kg once daily dosing against pathogens with high MIC value (4–16 mg/L) (EUCAST, 2020a). EUCAST susceptibility breakpoints are 8 mg/L and 16 mg/L for Enterobacterales and Pseudomonas sp., respectively (EUCAST, 2020b). Both the approved and revised dosing regimens were evaluated. PBPK simulations of both adult and pediatric dosing regimens indicated that drug exposure was highest in the heart, followed by the blood, lungs, and skin. A reason for a slightly higher exposure of amikacin in the heart compared to plasma is that lower blood flow and pressure that are usually present in the cardiac tricuspid valve tissue compared to the aorta can result in longer blood retention time, resulting in a higher drug exposure (McColm and Ryan, 1985; Bayer et al., 1988).
For amikacin, two relevant PD target indices are fCmax/MIC ≥8 and fAUC/MIC ≥80. The fAUC/MIC target at 80–90 is believed to be a more robust alternative and may be more suitable for critically ill patients with high bacterial burden infections such as nosocomial pneumonia (Bland et al., 2018; De winter et al., 2018; Perez-Blanco et al., 2021). Due to differences in dosing frequencies of amikacin between adults with renal insufficiencies and pediatrics, we used these two target PD indices for amikacin in our computation of PTA for a more thorough assessment. With the high dose of amikacin, at least 90% PTA was achieved in blood with an MIC of ≤16 μg/mL using ≥8 fCmax/MIC (Supplementary Figure S5) and ≤4 μg/mL using ≥80 fAUC/MIC (Figure 3). The 15 mg/kg adult dosing regimens only attained half the MIC of the high dose (Supplementary Figures S6, S7). The 15 mg/kg twice-daily in pediatrics resulted in similar PTA as that of the adult of the same total daily dose using target AUC PD index (Figure 3) but lower PTA if PD index based on Cmax was used (Supplementary Figure S6), since the twice-daily regimen resulted in only half the Cmax as the once-daily regimen with the same total daily dose. As amikacin exposure in the lung and skin is lower than that in the blood, sufficient coverage can be achieved at ≤2 and ≤1 μg/mL MIC in the lung and skin, respectively (Figure 3) for the adult high dose with the AUC PD index. With the Cmax PD index, sufficient coverage can be achieved at a two-fold higher MIC values. Due to low permeability of amikacin in the skin, skin infections are difficult to treat with intravenous amikacin administration.
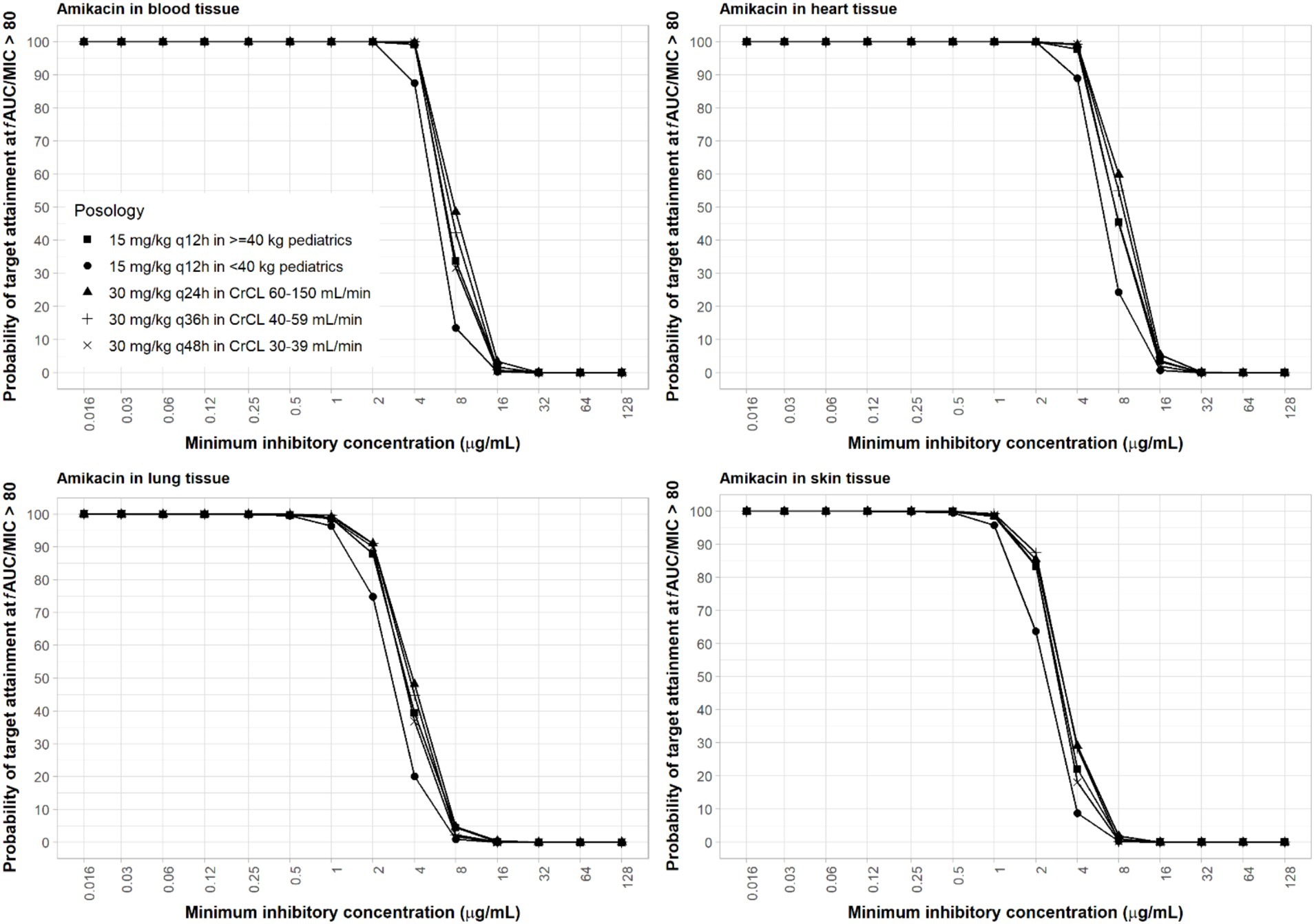
Figure 3. Probability of target attainment of amikacin PD index of fAUC/MIC ratio of at least 80 in the blood, lung, heart, and skin for dosing regimens in pediatrics (15 mg/kg) and adults (30 mg/kg).
The interpretive criteria for Acinetobacter spp. susceptibility to sulbactam treatment is ≤4 μg/mL for susceptible and 8 μg/mL for intermediate (CLSI, 2024). Sulbactam pediatric regimen of 40 mg/kg q6h as 3-h infusion was selected for a body weight <40 kg. Previous studies have shown that sulbactam adult regimens against A. baumannii at 8 μg/mL MIC, using 40% fT > MIC as PD index, can reach ≥90% PTA (Yokoyama et al., 2015). The calculations assumed a plasma protein binding of 5% and no protein binding in other tissues (Yokoyama et al., 2014). Sulbactam was able to achieve ≥90% PTA in all age groups of pediatric patients with an MIC of ≤8 μg/mL using the administration schedule listed in Table 2. At 4 μg/mL MIC, coverage in the lung, skin, and heart were adequate based on the proposed dosing regimens of sulbactam (Figure 4).
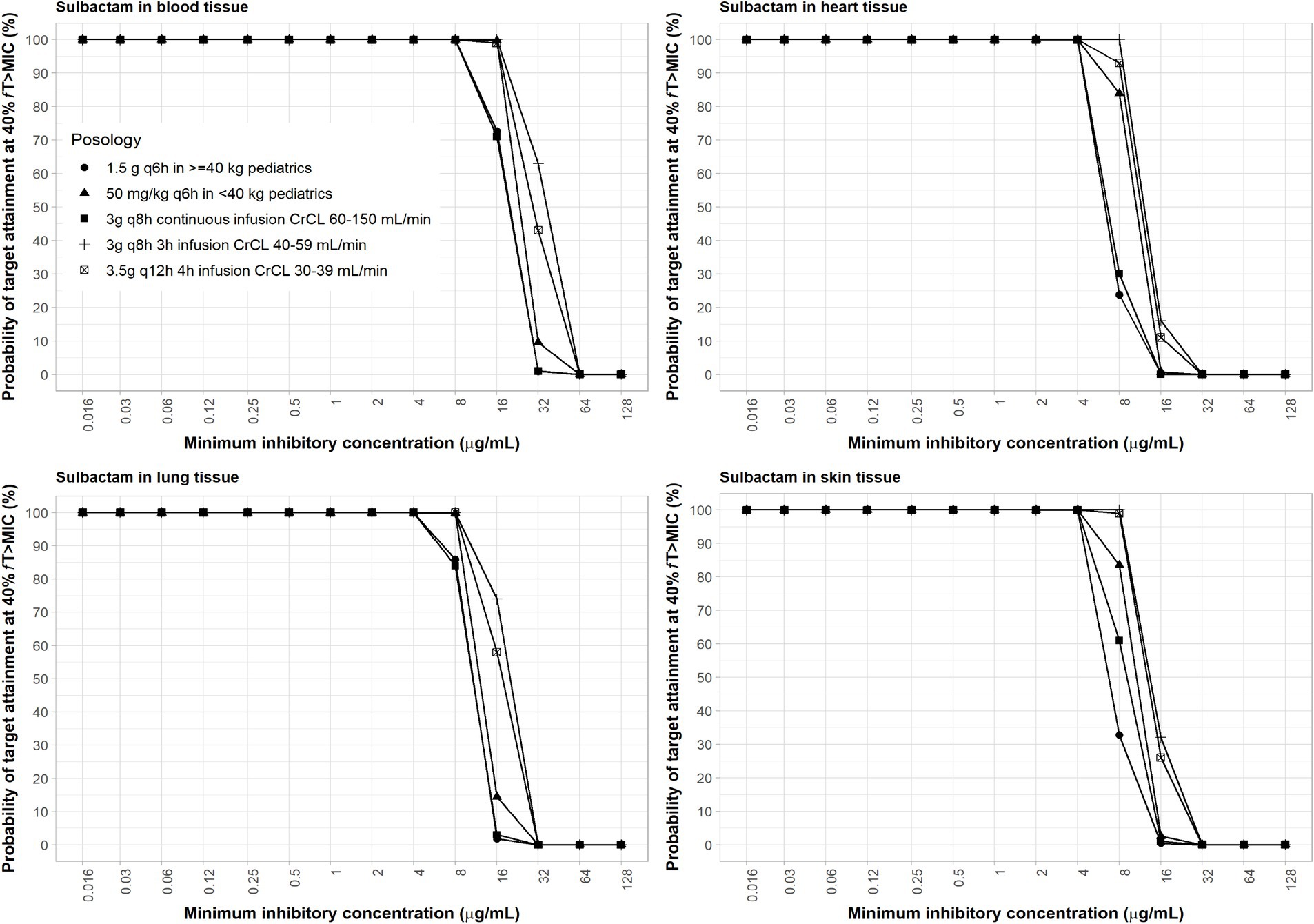
Figure 4. Probability of target attainment of sulbactam PD index of fT > MIC of at least 60% in the blood, lung, heart, and skin for adult and pediatric dosing regimens.
In the lung, the MIC values for amikacin, polymyxin-B and sulbactam combination to sufficiently achieve satisfactory joint PTA are 2/4/4 μg/mL.
4 Discussion
The predicted antibiotic exposures using PBPK models and target achievement in major tissues associated with common infections including the heart, lungs, and skin can be used to infer efficacy at the site of infection. Often actual drug exposures in these commonly infected sites are lacking, especially drug concentrations in pediatric tissues and organs; pediatric data are more difficult to obtain than in adults (Kafetzis et al., 1979; Schaad et al., 1986; Xu et al., 2022). We established and validated PBPK models in adults and extrapolated them to the pediatric population to evaluate the therapeutic effects of these antibiotics at the sites of infection. The application of the PBPK model to determine site-specific drug concentrations assumes that PD targets and thresholds associated with microbial outcomes are appropriate not only for their assessment in the blood, but also in the infected tissues.
The clinical efficacy of antimicrobial combination is often inferred from case reports. The combination of polymyxin-B and amikacin has been shown to be effective against blood infections caused by Klebsiella pneumoniae and offered a survival benefit (Cleary et al., 1979). This combination therapy has not been studied in a randomized clinical trial. Polymyxin-B combined with cefoperazone/sulbactam successfully cured posterior ventriculitis caused by extensively resistant A. baumannii in a child. In another case, a patient presented with pneumonia-related multiple organ dysfunction syndrome due to MDR P. aeruginosa and A. baumannii was successfully treated with polymyxin-B/amikacin combination (Wang et al., 2021; Yang et al., 2021).
The combination strategy can sensitize MDR microorganisms to the drug concentrations produced using the approved dosing regimens. In our previous survey of extremely drug-resistant A. baumannii clinical isolates, the MIC50 and MIC90 for amikacin/polymyxin-B/sulbactam combination were 1/4/4 and 8/4/4 μg/mL, respectively (Zhu et al., 2022a). Based on the PTA evaluation for this combination in the blood, lung, heart and skin, sufficient coverage can be achieved for the MIC50 of the surveyed collection. Amikacin dosing regimens, on the other hand, would not provide sufficient coverage against MIC90 of the collection based on the stricter PD index using fAUC/MIC >80. However, the fCmax/MIC >8 criteria can be achieved with the high dose of 30 mg/kg/day in the blood, heart and lung, but not the skin.
Our analysis indicated that drug exposures for this combination in the tissues or organs evaluated were effective against at least 50% of extremely drug resistant A. baumannii clinical isolates. The inference for clinical efficacy using PTA did not include the antibacterial effect of human immunity. In the in vivo infection model used to define target PD indices, neutropenia was induced in mice prior to infecting the animal (Sy and Derendorf, 2014). The methodology to derive PD indices reflects the worst-case scenario in immunocompromised individuals. The immune system will play an important role against MDR infections because it does not distinguish between resistant and sensitive bacteria (Rayner et al., 2021).
The variability in antibiotic permeability across different tissues led to differences in the PTA results. Amikacin, as an aminoglycoside, is a hydrophilic molecule with low tissue permeability. Passive diffusion across endothelial cells of capillaries requires drugs to be lipophilic, which may result in low amikacin exposure in the lungs and skin (Honeybourne, 1994; Najmeddin et al., 2020). However, amikacin is commonly used in the clinic for the treatment of acute exacerbations in patients with cystic fibrosis (Kiem and Schentag, 2008), which may be attributed to inflammation-induced lung endothelial damage, affecting alveolar epithelial permeability, thereby enhancing amikacin penetration and distribution into the lungs (Lamer et al., 1993). Although it can achieve high therapeutic concentrations in the lungs, amikacin clearance from the lungs is affected by its exchange in the blood.
Polymyxin B is a cationic polypeptide antibiotic, and its large molecular characteristics restrict its distribution, metabolism, and excretion after intravenous injection (Avedissian et al., 2019). Our predictions for lung exposure were consistent with the observed drug concentrations reported (Manchandani et al., 2016). The 6-h sample of polymyxin in the lung tissues was approximately 2-fold the serum drug concentration, indicating accumulation of polymyxin in the lung over time (Manchandani et al., 2016), whereas polymyxin exposure in the epithelial lining fluid in mice was previously shown to be lower than that in the serum (He et al., 2010). There is a high degree of variability in the literature on polymyxin lung penetration.
The current guidelines for polymyxins recommends combination therapy, since it is not possible to increase the daily doses beyond the recommended limit of 2.5 mg/kg loading dose and 1.5 mg/kg q12h maintenance dose (Tsuji et al., 2019). Lung infection model indicated lower efficacy than thigh infection model (Cheah et al., 2015). With increasing polymyxin resistance, the strategy to optimize polymyxin therapeutics could also include inhalation, in the case of lung infection (Zhu et al., 2022a).
While our PBPK model offers valuable insights into the pharmacokinetics of drug combinations in pediatric patients, particularly in predicting drug concentrations across various tissues, including the lungs, we must acknowledge the inherent uncertainties and limitations of these predictions. The complexity and variability of lung physiology, the heterogeneity in drug distribution, and the lack of comprehensive clinical data to fully validate these predictions present many challenges toward an accurate prediction of lung polymyxin concentrations.
Dosing guideline of antibiotics in pediatrics should be based on antimicrobial susceptibility determination. We do not recommend deviating from the recommended clinical dosages. Of the three antibiotics, only amikacin has a standard dose and a high dose, due to recent changes in consensus guidelines. Decision to use combination therapy should be based on whether the MIC for the combination can be sufficiently covered by the joint PTA.
Strategies for antibiotic combination use need to consider whether there is a potential risk of an enhanced toxicity. Consequently, the duration of treatment could be limited by adverse events. The polymyxin-B/amikacin/sulbactam triple combination has the potential for nephrotoxicity and other adverse effects. Polymyxin-B undergoes renal reabsorption through tubular cells while amikacin and sulbactam are primarily eliminated by the kidney. We chose polymyxin-B and sulbactam to be combined with amikacin because the renal liability of polymyxin-B is considerably less compared to colistin (Zavascki and Nation, 2017). The addition of sulbactam to the combination is based on a matched cohort study showing low potential of sulbactam to induce acute kidney injury when used as a partnering β-lactamase inhibitor to piperacillin (Rutter and Burgess, 2017). While amikacin is known to cause nephrotoxicity (Kaynar et al., 2007), the amikacin/polymyxin-B combination in a case report did not result in acute kidney injury even though this combination was administered for the duration of 30 days; the patient’s follow-up serum creatinine was 75 μmol/L which indicated no evidence of acute renal impairment (Wang et al., 2021).
Several limitations of the current approach are identified. The lack of actual tissue drug concentration data in human tissues limits our ability to verify the simulation of drug concentration. The complexity of organ tissue structure also affects the accuracy of drug concentration simulation; the microanatomy of tissue can lead to concentration gradients between compartments. The tissue drug concentration simulated in this study comes from interstitial space and may not necessarily represent the microspace where bacteria proliferate.
In summary, this study explored the use of PBPK models to predict drug exposure in several potential sites of infection in the pediatric population, and assessed whether exposure could achieve the desired target achievement rate. The results of the study have yet to be confirmed in clinical trials. Antibiotic combination offers a potential treatment option against tissue infections caused by drug-resistant bacteria, which are increasingly threatening human health. At a time when new antibiotics are scarce, effective antibiotic combination therapy has practical implications for addressing the pressing problem of drug resistance. PBPK and other modeling methods to predict and simulate the amount of antibacterial drug exposure in tissue is a practical approach to overcome the difficulty of obtaining tissue drug concentrations in pediatric population.
Data availability statement
The original contributions presented in the study are included in the article/Supplementary material, further inquiries can be directed to the corresponding authors.
Ethics statement
Ethical approval was not required for the study involving humans in accordance with the local legislation and institutional requirements. Written informed consent to participate in this study was not required from the participants or the participants’ legal guardians/next of kin in accordance with the national legislation and the institutional requirements.
Author contributions
MW: Writing – original draft, Writing – review & editing, Investigation, Methodology, Software. KF: Writing – review & editing, Writing – original draft, Methodology. XW: Writing – review & editing, Investigation. CL: Writing – review & editing. SZ: Writing – original draft, Data curation. FM: Writing – review & editing, Conceptualization, Investigation. MYu: Writing – original draft, Writing – review & editing. ZL: Writing – original draft, Writing – review & editing, Supervision. MYa: Writing – review & editing, Supervision. SS: Writing – original draft, Writing – review & editing, Supervision.
Funding
The author(s) declare that financial support was received for the research, authorship, and/or publication of this article. This work was supported by grant from Shandong Provincial Natural Science Foundation (ZR2019BC025).
Conflict of interest
The authors declare that the research was conducted in the absence of any commercial or financial relationships that could be construed as a potential conflict of interest.
Publisher’s note
All claims expressed in this article are solely those of the authors and do not necessarily represent those of their affiliated organizations, or those of the publisher, the editors and the reviewers. Any product that may be evaluated in this article, or claim that may be made by its manufacturer, is not guaranteed or endorsed by the publisher.
Supplementary material
The Supplementary material for this article can be found online at: https://www.frontiersin.org/articles/10.3389/fmicb.2024.1435906/full#supplementary-material
Footnotes
References
Abdi, S. N., Ghotaslou, R., Ganbarov, K., Mobed, A., Tanomand, A., Yousefi, M., et al. (2020). Acinetobacter baumannii efflux pumps and antibiotic resistance. Infect. Drug Resist. 13, 423–434. doi: 10.2147/IDR.S228089
Avedissian, S. N., Liu, J., Rhodes, N. J., Lee, A., Pais, G. M., Hauser, A. R., et al. (2019). A review of the clinical pharmacokinetics of polymyxin B. Antibiotics 8:31. doi: 10.3390/antibiotics8010031
Bauer, L. A., Blouin, R. A., Griffen, W. O., Record, K. E., and Bell, R. M. (1980). Amikacin pharmacokinetics in morbidly obese patients. Am. J. Hosp. Pharm. 37, 519–522. doi: 10.1093/ajhp/37.4.519
Bayer, A. S., Crowell, D. J., Yih, J., Bradley, D. W., and Norman, D. C. (1988). Comparative pharmacokinetics and pharmacodynamics of amikacin and ceftazidime in tricuspid and aortic vegetations in experimental Pseudomonas endocarditis. J. Infect. Dis. 158, 355–359. doi: 10.1093/infdis/158.2.355
Biesdorf, C., Martins, F. S., Sy, S. K. B., and Diniz, A. (2019). Physiologically-based pharmacokinetics of ziprasidone in pregnant women. Br. J. Clin. Pharmacol. 85, 914–923. doi: 10.1111/bcp.13872
Bischoff, K. B. (1986). Physiological pharmacokinetics. Bull. Math. Biol. 48, 309–322. doi: 10.1007/BF02459684
Bland, C. M., Pai, M. P., and Lodise, T. P. (2018). Reappraisal of contemporary pharmacokinetic and pharmacodynamic principles for informing aminoglycoside dosing. Pharmacotherapy 38, 1229–1238. doi: 10.1002/phar.2193
Burkin, M. A., Galvidis, I. A., Surovoy, Y. A., Plyushchenko, I. V., Rodin, I. A., and Tsarenko, S. V. (2021). Development of ELISA formats for polymyxin B monitoring in serum of critically ill patients. J. Pharm. Biomed. Anal. 204:114275. doi: 10.1016/j.jpba.2021.114275
Cheah, S. E., Wang, J., Nguyen, V. T., Turnidge, J. D., Li, J., and Nation, R. L. (2015). New pharmacokinetic/pharmacodynamic studies of systemically administered colistin against Pseudomonas aeruginosa and Acinetobacter baumannii in mouse thigh and lung infection models: smaller response in lung infection. J. Antimicrob. Chemother. 70, 3291–3297. doi: 10.1093/jac/dkv267
Claassen, K., Thelen, K., Coboeken, K., Gaub, T., Lippert, J., Allegaert, K., et al. (2015). Development of a physiologically-based pharmacokinetic model for preterm neonates: evaluation with in vivo data. Curr. Pharm. Des. 21, 5688–5698. doi: 10.2174/1381612821666150901110533
Cleary, T. G., Pickering, L. K., Kramer, W. G., Culbert, S., Frankel, L. S., and Kohl, S. (1979). Amikacin pharmacokinetics in pediatric patients with malignancy. Antimicrob. Agents Chemother. 16, 829–832. doi: 10.1128/AAC.16.6.829
CLSI (2024). “Performance standards for antimicrobial susceptibility testing” in CLSI document M100. 34th ed (Wayne, PA: Clinical and Laboratory Standards Institute).
Darlow, C. A., Parrott, N., Peck, R. W., and Hope, W. (2024). Development and application of neonatal physiology-based pharmacokinetic models of amikacin and fosfomycin to assess pharmacodynamic target attainment. CPT Pharmacometrics Syst. Pharmacol. 13, 464–475. doi: 10.1002/psp4.13097
De Montmollin, E., Bouadma, L., Gault, N., Mourvillier, B., Mariotte, E., Chemam, S., et al. (2014). Predictors of insufficient amikacin peak concentration in critically ill patients receiving a 25 mg/kg total body weight regimen. Intensive Care Med. 40, 998–1005. doi: 10.1007/s00134-014-3276-x
De winter, S., Wauters, J., Meersseman, W., Verhaegen, J., Van Wijngaerden, E., Peetermans, W., et al. (2018). Higher versus standard amikacin single dose in emergency department patients with severe sepsis and septic shock: a randomised controlled trial. Int. J. Antimicrob. Agents 51, 562–570. doi: 10.1016/j.ijantimicag.2017.11.009
Dudhani, R. V., Turnidge, J. D., Nation, R. L., and Li, J. (2010). fAUC/MIC is the most predictive pharmacokinetic/pharmacodynamic index of colistin against Acinetobacter baumannii in murine thigh and lung infection models. J. Antimicrob. Chemother. 65, 1984–1990. doi: 10.1093/jac/dkq226
EUCAST (2020a). European committee on antimicrobial susceptibility testing (EUCAST) 2020 amikacin: rationale for the EUCAST clinical breakpoints, version 2.0. Available at: https://www.eucast.org/fileadmin/src/media/PDFs/EUCAST_files/Rationale_documents/Amikacin_rationale_1.2_0906.pdf (accessed on April, 24, 2024)
EUCAST (2020b). European committee on antimicrobial susceptibility testing: clinical breakpoints and dosing of antibiotics version 11.0, [updated on 6.1.21]. Available at: https://eucast.org/clinical_breakpoints/ (accessed April 24, 2024)
FDA (2011). U.S. Food and Drug Administration, Rockville, MD. Polymyxin B (package insert). Available at: https://www.accessdata.fda.gov/drugsatfda_docs/label/2012/060716Orig1s020Lbl.pdf (accessed December 23, 2021)
Frost, K. J., Hamilton, R. A., Hughes, S., Jamieson, C., Rafferty, P., Troise, O., et al. (2023). Systematic review of high-dose amikacin regimens for the treatment of gram-negative infections based on EUCAST dosing recommendations. Eur. J. Hosp. Pharm. 30, 189–195. doi: 10.1136/ejhpharm-2022-003421
Galvez, R., Luengo, C., Cornejo, R., Kosche, J., Romero, C., Tobar, E., et al. (2011). Higher than recommended amikacin loading doses achieve pharmacokinetic targets without associated toxicity. Int. J. Antimicrob. Agents 38, 146–151. doi: 10.1016/j.ijantimicag.2011.03.022
Gamba, G., Contreras, A. M., Cortes, J., Nares, F., Santiago, Y., Espinosa, A., et al. (1990). Hypoalbuminemia as a risk factor for amikacin nephrotoxicity. Rev. Investig. Clin. 42, 204–209
Garraffo, R., Drugeon, H. B., Dellamonica, P., Bernard, E., and Lapalus, P. (1990). Determination of optimal dosage regimen for amikacin in healthy volunteers by study of pharmacokinetics and bactericidal activity. Antimicrob. Agents Chemother. 34, 614–621. doi: 10.1128/AAC.34.4.614
He, J., Ledesma, K. R., Lam, W. Y., Figueroa, D. A., Lim, T. P., Chow, D. S., et al. (2010). Variability of polymyxin B major components in commercial formulations. Int. J. Antimicrob. Agents 35, 308–310. doi: 10.1016/j.ijantimicag.2009.11.005
Honeybourne, D. (1994). Antibiotic penetration into lung tissues. Thorax 49, 104–106. doi: 10.1136/thx.49.2.104
Ince, I., Dallmann, A., Frechen, S., Coboeken, K., Niederalt, C., Wendl, T., et al. (2021). Predictive performance of physiology-based pharmacokinetic dose estimates for pediatric trials: evaluation with 10 Bayer small-molecule compounds in children. J. Clin. Pharmacol. 61 Suppl 1, S70–S82. doi: 10.1002/jcph.1869
Ince, I., Solodenko, J., Frechen, S., Dallmann, A., Niederalt, C., Schlender, J., et al. (2019). Predictive pediatric modeling and simulation using ontogeny information. J. Clin. Pharmacol. 59, S95–S103. doi: 10.1002/jcph.1497
Kafetzis, D. A., Sinaniotis, C. A., Papadatos, C. J., and Kosmidis, J. (1979). Pharmacokinetics of amikacin in infants and pre-school children. Acta Paediatr. Scand. 68, 419–422. doi: 10.1111/j.1651-2227.1979.tb05030.x
Kato, H., Hagihara, M., Hirai, J., Sakanashi, D., Suematsu, H., Nishiyama, N., et al. (2017). Evaluation of amikacin pharmacokinetics and pharmacodynamics for optimal initial dosing regimen. Drugs R D 17, 177–187. doi: 10.1007/s40268-016-0165-5
Kaynar, K., Gul, S., Ersoz, S., Ozdemir, F., Ulusoy, H., and Ulusoy, S. (2007). Amikacin-induced nephropathy: is there any protective way? Ren. Fail. 29, 23–27. doi: 10.1080/08860220601039072
Kiem, S., and Schentag, J. J. (2008). Interpretation of antibiotic concentration ratios measured in epithelial lining fluid. Antimicrob. Agents Chemother. 52, 24–36. doi: 10.1128/AAC.00133-06
Kotra, L. P., Haddad, J., and Mobashery, S. (2000). Aminoglycosides: perspectives on mechanisms of action and resistance and strategies to counter resistance. Antimicrob. Agents Chemother. 44, 3249–3256. doi: 10.1128/AAC.44.12.3249-3256.2000
Lagana, P., Melcarne, L., and Delia, S. (2015). Acinetobacter baumannii and endocarditis, rare complication but important clinical relevance. Int. J. Cardiol. 187, 678–679. doi: 10.1016/j.ijcard.2015.04.019
Lamer, C., De Beco, V., Soler, P., Calvat, S., Fagon, J. Y., Dombret, M. C., et al. (1993). Analysis of vancomycin entry into pulmonary lining fluid by bronchoalveolar lavage in critically ill patients. Antimicrob. Agents Chemother. 37, 281–286. doi: 10.1128/AAC.37.2.281
Lanao, J. M., Dominguez-Gil, A. A., Dominguez-Gil, A., Malaga, S., Crespo, M., and Santos, F. (1981). Pharmacokinetics of amikacin in children with normal and impaired renal function. Kidney Int. 20, 115–121. doi: 10.1038/ki.1981.112
Lanao, J. M., Navarro, A. S., Dominguez-Gil, A., Tabernero, J. M., Rodriguez, I. C., and Gonzalez Lopez, A. (1983). Amikacin concentrations in serum and blister fluid in healthy volunteers and in patients with renal impairment. J. Antimicrob. Chemother. 12, 481–488. doi: 10.1093/jac/12.5.481
Li, J., and Nation, R. L. (2006). Old polymyxins are back: is resistance close? Clin. Infect. Dis. 43, 663–664. doi: 10.1086/506571
Li, J., Nation, R. L., Turnidge, J. D., Milne, R. W., Coulthard, K., Rayner, C. R., et al. (2006). Colistin: the re-emerging antibiotic for multidrug-resistant gram-negative bacterial infections. Lancet Infect. Dis. 6, 589–601. doi: 10.1016/S1473-3099(06)70580-1
Liu, H., Hu, D., Wang, D., Wu, H., Pan, Y., Chen, X., et al. (2023). In vitro analysis of synergistic combination of polymyxin B with 12 other antibiotics against MDR Acinetobacter baumannii isolated from a Chinese tertiary hospital. J. Antibiot. 76, 20–26. doi: 10.1038/s41429-022-00573-z
Mahmoudi, L., Mohammadpour, A. H., Ahmadi, A., Niknam, R., and Mojtahedzadeh, M. (2013). Influence of sepsis on higher daily dose of amikacin pharmacokinetics in critically ill patients. Eur. Rev. Med. Pharmacol. Sci. 17, 285–291
Malik, P. R. V., Yeung, C. H. T., Ismaeil, S., Advani, U., Djie, S., and Edginton, A. N. (2020). A physiological approach to pharmacokinetics in chronic kidney disease. J. Clin. Pharmacol. 60, S52–S62. doi: 10.1002/jcph.1713
Manchandani, P., Zhou, J., Ledesma, K. R., Truong, L. D., Chow, D. S., Eriksen, J. L., et al. (2016). Characterization of polymyxin B biodistribution and disposition in an animal model. Antimicrob. Agents Chemother. 60, 1029–1034. doi: 10.1128/AAC.02445-15
Martins, F. S., Martins, J. E. S., Severino, P., Annaert, P., and Sy, S. K. B. (2023). Physiologically based pharmacokinetic modelling to inform combination dosing regimens of ceftaroline and daptomycin in special populations. Br. J. Clin. Pharmacol. 89, 2726–2738. doi: 10.1111/bcp.15731
Martins, F. S., Sy, S. K. B., Fonseca, M. J. V., and De Freitas, O. (2020). Pharmacokinetics, pharmacodynamics and dermal distribution of 5-methoxypsoralen based on a physiologically based pharmacokinetic model to support phytotherapy using Brosimum gaudichaudii. Planta Med. 86, 276–283. doi: 10.1055/a-1087-8374
Martins, F. S., Zhu, P., Heinrichs, M. T., and Sy, S. K. B. (2021). Physiologically based pharmacokinetic-pharmacodynamic evaluation of meropenem plus fosfomycin in paediatrics. Br. J. Clin. Pharmacol. 87, 1012–1023. doi: 10.1111/bcp.14456
Mccolm, A. A., and Ryan, D. M. (1985). Comparative pharmacokinetics of ceftazidime in fibrin clots and cardiac vegetations in rabbits with Staphylococcus aureus endocarditis. Antimicrob. Agents Chemother. 27, 925–927. doi: 10.1128/AAC.27.6.925
Mcnamara, P. J., and Alcorn, J. (2002). Protein binding predictions in infants. AAPS PharmSci 4, 19–26. doi: 10.1208/ps040104
Menegucci, T. C., Fedrigo, N. H., Lodi, F. G., Albiero, J., Nishiyama, S. A. B., Mazucheli, J., et al. (2019). Pharmacodynamic effects of sulbactam/meropenem/polymyxin-B combination against extremely drug resistant Acinetobacter baumannii using checkerboard information. Microb. Drug Resist. 25, 1266–1274. doi: 10.1089/mdr.2018.0283
Moore, R. D., Lietman, P. S., and Smith, C. R. (1987). Clinical response to aminoglycoside therapy: importance of the ratio of peak concentration to minimal inhibitory concentration. J. Infect. Dis. 155, 93–99. doi: 10.1093/infdis/155.1.93
Najmeddin, F., Shahrami, B., Azadbakht, S., Dianatkhah, M., Rouini, M. R., Najafi, A., et al. (2020). Evaluation of epithelial lining fluid concentration of amikacin in critically ill patients with ventilator-associated pneumonia. J. Intensive Care Med. 35, 400–404. doi: 10.1177/0885066618754784
Oo, C., and Sy, S. K. B. (2020). Learning and augmenting natural processes: potential means of combating antimicrobial resistance from a drug R&D perspective. Drug Discov. Today 25, 1–3. doi: 10.1016/j.drudis.2019.07.012
Oo, C., Zhang, X., and Sy, S. K. B. (2023). Evaluating the status of antibiotic approvals and readiness to combat antimicrobial resistance: what else can we do better? Drug Discov. Today 28:103674. doi: 10.1016/j.drudis.2023.103674
Perez-Blanco, J. S., Saez Fernandez, E. M., Calvo, M. V., Lanao, J. M., and Martin-Suarez, A. (2021). Evaluation of current amikacin dosing recommendations and development of an interactive nomogram: the role of albumin. Pharmaceutics 13:264. doi: 10.3390/pharmaceutics13020264
Poulin, P., Schoenlein, K., and Theil, F. P. (2001). Prediction of adipose tissue: plasma partition coefficients for structurally unrelated drugs. J. Pharm. Sci. 90, 436–447. doi: 10.1002/1520-6017(200104)90:4<436::AID-JPS1002>3.0.CO;2-P
Rao, G. G., Ly, N. S., Bulitta, J. B., Soon, R. L., San Roman, M. D., Holden, P. N., et al. (2016). Polymyxin B in combination with doripenem against heteroresistant Acinetobacter baumannii: pharmacodynamics of new dosing strategies. J. Antimicrob. Chemother. 71, 3148–3156. doi: 10.1093/jac/dkw293
Rayner, C. R., Smith, P. F., Andes, D., Andrews, K., Derendorf, H., Friberg, L. E., et al. (2021). Model-informed drug development for anti-infectives: state of the art and future. Clin. Pharmacol. Ther. 109, 867–891. doi: 10.1002/cpt.2198
Rodvold, K. A., Gotfried, M. H., Isaacs, R. D., O'donnell, J. P., and Stone, E. (2018). Plasma and intrapulmonary concentrations of ETX2514 and sulbactam following intravenous administration of ETX2514SUL to healthy adult subjects. Antimicrob. Agents Chemother. 62:e01089-18. doi: 10.1128/AAC.01089-18
Rutter, W. C., and Burgess, D. S. (2017). Acute kidney injury in patients treated with IV beta-lactam/beta-lactamase inhibitor combinations. Pharmacotherapy 37, 593–598. doi: 10.1002/phar.1918
Rybak, M. J., Abate, B. J., Kang, S. L., Ruffing, M. J., Lerner, S. A., and Drusano, G. L. (1999). Prospective evaluation of the effect of an aminoglycoside dosing regimen on rates of observed nephrotoxicity and ototoxicity. Antimicrob. Agents Chemother. 43, 1549–1555. doi: 10.1128/AAC.43.7.1549
Sandri, A. M., Landersdorfer, C. B., Jacob, J., Boniatti, M. M., Dalarosa, M. G., Falci, D. R., et al. (2013a). Population pharmacokinetics of intravenous polymyxin B in critically ill patients: implications for selection of dosage regimens. Clin. Infect. Dis. 57, 524–531. doi: 10.1093/cid/cit334
Sandri, A. M., Landersdorfer, C. B., Jacob, J., Boniatti, M. M., Dalarosa, M. G., Falci, D. R., et al. (2013b). Pharmacokinetics of polymyxin B in patients on continuous venovenous haemodialysis. J. Antimicrob. Chemother. 68, 674–677. doi: 10.1093/jac/dks437
Satlin, M. J., Lewis, J. S., Weinstein, M. P., Patel, J., Humphries, R. M., Kahlmeter, G., et al. (2020). Clinical and laboratory standards institute and European committee on antimicrobial susceptibility testing position statements on polymyxin B and colistin clinical breakpoints. Clin. Infect. Dis. 71, e523–e529. doi: 10.1093/cid/ciaa121
Schaad, U. B., Guenin, K., and Straehl, P. (1986). Single-dose pharmacokinetics of intravenous sulbactam in pediatric patients. Rev. Infect. Dis. 8, S512–S517. doi: 10.1093/clinids/8.Supplement_5.S512
Segal, J. L., Brunnemann, S. R., and Eltorai, I. M. (1990). Pharmacokinetics of amikacin in serum and in tissue contiguous with pressure sores in humans with spinal cord injury. Antimicrob. Agents Chemother. 34, 1422–1428. doi: 10.1128/AAC.34.7.1422
Shin, E., Zhang, Y., Zhou, J., Lang, Y., Sayed, A. R. M., Werkman, C., et al. (2024). Improved characterization of aminoglycoside penetration into human lung epithelial lining fluid via population pharmacokinetics. Antimicrob. Agents Chemother. 68:e0139323. doi: 10.1128/aac.01393-23
Sopirala, M. M., Pope-Harman, A., Nunley, D. R., Moffatt-Bruce, S., Ross, P., and Martin, S. I. (2008). Multidrug-resistant Acinetobacter baumannii pneumonia in lung transplant recipients. J. Heart Lung Transplant. 27, 804–807. doi: 10.1016/j.healun.2008.03.023
Spitzer, A. R., Ellsbury, D. L., Handler, D., and Clark, R. H. (2010). The Pediatrix BabySteps® data warehouse and the Pediatrix QualitySteps improvement project system—tools for “meaningful use” in continuous quality improvement. Clin. Perinatol. 37, 49–70. doi: 10.1016/j.clp.2010.01.016
Sy, S. K., Asin-Prieto, E., Derendorf, H., and Samara, E. (2014). Predicting pediatric age-matched weight and body mass index. AAPS J. 16, 1372–1379. doi: 10.1208/s12248-014-9657-9
Sy, S. K., and Derendorf, H. (2014). “Pharmacometrics in bacterial infections” in Applied pharmacometrics. eds. S. Schmidt and H. Derendorf. 1st ed (New York: springer), 229–258.
Sy, S. K., Zhuang, L., and Derendorf, H. (2016). Pharmacokinetics and pharmacodynamics in antibiotic dose optimization. Expert Opin. Drug Metab. Toxicol. 12, 93–114. doi: 10.1517/17425255.2016.1123250
Taber, H. W., Mueller, J. P., Miller, P. F., and Arrow, A. S. (1987). Bacterial uptake of aminoglycoside antibiotics. Microbiol. Rev. 51, 439–457. doi: 10.1128/mr.51.4.439-457.1987
Tsuji, B. T., Pogue, J. M., Zavascki, A. P., Paul, M., Daikos, G. L., Forrest, A., et al. (2019). International consensus guidelines for the optimal use of the polymyxins: endorsed by the American College of Clinical Pharmacy (ACCP), European Society of Clinical Microbiology and Infectious Diseases (ESCMID), Infectious Diseases Society of America (IDSA), International Society for Anti-infective Pharmacology (ISAP), Society of Critical Care Medicine (SCCM), and Society of Infectious Diseases Pharmacists (SIDP). Pharmacotherapy 39, 10–39. doi: 10.1002/phar.2209
Valentin, J. (2002). Basic anatomical and physiological data for use in radiological protection: reference values: ICRP publication 89. Ann. ICRP 32, 1–277. doi: 10.1016/S0146-6453(03)00002-2
Vanhaeverbeek, M., Siska, G., and Herchuelz, A. (1993). Pharmacokinetics of once-daily amikacin in elderly patients. J. Antimicrob. Chemother. 31, 185–187. doi: 10.1093/jac/31.1.185
Vogelstein, B., Kowarski, A., and Lietman, P. S. (1977). The pharmacokinetics of amikacin in children. J. Pediatr. 91, 333–339. doi: 10.1016/S0022-3476(77)80847-0
Wang, P. L., Liu, P., Zhang, Q. W., Yuan, W. H., Wang, D., Zhang, X. J., et al. (2022). Population pharmacokinetics and clinical outcomes of polymyxin B in paediatric patients with multidrug-resistant gram-negative bacterial infections. J. Antimicrob. Chemother. 77, 3000–3008. doi: 10.1093/jac/dkac265
Wang, J., Yun, L., Zhao, H., and Li, X. (2021). Combination therapy of polymyxin B and amikacin for community-acquired Pseudomonas aeruginosa pneumonia with MODS in a previously healthy patient: a case report. Infect Drug Resist 14, 2895–2900. doi: 10.2147/IDR.S312601
Xu, C., Liu, X., Cui, Y., Huang, X., Wang, Y., Fan, Y., et al. (2022). Case report: therapeutic drug monitoring of polymyxin B during continuous renal replacement therapy in two pediatric patients: do not underestimate extracorporeal clearance. Front. Pharmacol. 13:822981. doi: 10.3389/fphar.2022.822981
Yang, R., Li, F., Mao, W. W., Wei, X., Liu, X. Z., Guo, X. W., et al. (2021). Successful treatment of postneurosurgical ventriculitis caused by extensively drug-resistant in a child: case report. Jundishapur J Microbiol 14:e118114. doi: 10.5812/jjm.11811
Yokoyama, Y., Matsumoto, K., Ikawa, K., Watanabe, E., Morikawa, N., and Takeda, Y. (2015). Population pharmacokinetic-pharmacodynamic target attainment analysis of sulbactam in patients with impaired renal function: dosing considerations for Acinetobacter baumannii infections. J. Infect. Chemother. 21, 284–289. doi: 10.1016/j.jiac.2014.12.005
Yokoyama, Y., Matsumoto, K., Ikawa, K., Watanabe, E., Shigemi, A., Umezaki, Y., et al. (2014). Pharmacokinetic/pharmacodynamic evaluation of sulbactam against Acinetobacter baumannii in in vitro and murine thigh and lung infection models. Int. J. Antimicrob. Agents 43, 547–552. doi: 10.1016/j.ijantimicag.2014.02.012
Zavascki, A. P., Goldani, L. Z., Cao, G., Superti, S. V., Lutz, L., Barth, A. L., et al. (2008). Pharmacokinetics of intravenous polymyxin B in critically ill patients. Clin. Infect. Dis. 47, 1298–1304. doi: 10.1086/592577
Zavascki, A. P., Goldani, L. Z., Li, J., and Nation, R. L. (2007). Polymyxin B for the treatment of multidrug-resistant pathogens: a critical review. J. Antimicrob. Chemother. 60, 1206–1215. doi: 10.1093/jac/dkm357
Zavascki, A. P., and Nation, R. L. (2017). Nephrotoxicity of polymyxins: is there any difference between colistimethate and polymyxin B? Antimicrob. Agents Chemother. 61:e02319-16. doi: 10.1128/AAC.02319-16
Zhang, Y. M., and Rock, C. O. (2008). Membrane lipid homeostasis in bacteria. Nature Rev Microbiol 6, 222–233. doi: 10.1038/nrmicro1839
Zhang, J., Song, C., Wu, M., Yue, J., Zhu, S., Zhu, P., et al. (2023). Physiologically-based pharmacokinetic modeling to inform dosing regimens and routes of administration of rifampicin and colistin combination against Acinetobacter baumannii. Eur. J. Pharm. Sci. 185:106443. doi: 10.1016/j.ejps.2023.106443
Zhu, S., Song, C., Zhang, J., Diao, S., Heinrichs, T. M., Martins, F. S., et al. (2022a). Effects of amikacin, polymyxin-B, and sulbactam combination on the pharmacodynamic indices of mutant selection against multi-drug resistant Acinetobacter baumannii. Front. Microbiol. 13:1013939. doi: 10.3389/fmicb.2022.1013939
Zhu, S., Yue, J., Wang, X., Zhang, J., Yu, M., Zhan, Y., et al. (2023). Metabolomics revealed mechanism for the synergistic effect of sulbactam, polymyxin-B and amikacin combination against Acinetobacter baumannii. Front. Microbiol. 14:1217270. doi: 10.3389/fmicb.2023.1217270
Keywords: antibiotic combination, tissue exposure, physiologically-based pharmacokinetic, pharmacodynamic index, multidrug resistance (MDR)
Citation: Wu M, Feng K, Wu X, Liu C, Zhu S, Martins FS, Yu M, Lv Z, Yan M and Sy SKB (2024) Prediction of tissue exposures of polymyxin-B, amikacin and sulbactam using physiologically-based pharmacokinetic modeling. Front. Microbiol. 15:1435906. doi: 10.3389/fmicb.2024.1435906
Edited by:
Giancarlo Ceccarelli, Sapienza University of Rome, ItalyReviewed by:
Deok Yong Yoon, Novartis, United StatesSasikala Muthusamy, Brigham and Women’s Hospital and Harvard Medical School, United States
Copyright © 2024 Wu, Feng, Wu, Liu, Zhu, Martins, Yu, Lv, Yan and Sy. This is an open-access article distributed under the terms of the Creative Commons Attribution License (CC BY). The use, distribution or reproduction in other forums is permitted, provided the original author(s) and the copyright owner(s) are credited and that the original publication in this journal is cited, in accordance with accepted academic practice. No use, distribution or reproduction is permitted which does not comply with these terms.
*Correspondence: Zhihua Lv, bHZ6aGlodWFAb3VjLmVkdS5jbg==; Meixing Yan, bWVpeGluZ0AxNjMuY29t; Sherwin K. B. Sy, c2hlcndpbi5rZW5uZXRoLnN5QGdtYWlsLmNvbQ==
†These authors have contributed equally to this work and share first authorship