- 1Department of Microbiology, Immunobiology and Genetics, Max Perutz Labs, Center of Molecular Biology, Vienna Biocenter, University of Vienna, Vienna, Austria
- 2Vienna BioCenter PhD Program, a Doctoral School of the University of Vienna and the Medical University of Vienna, Max Perutz Labs, Vienna Biocenter, University of Vienna, Vienna, Austria
Bacteria employ small regulatory RNAs (sRNA) and/or RNA binding proteins (RBPs) to respond to environmental cues. In Enterobacteriaceae, the FinO-domain containing RBP ProQ associates with numerous sRNAs and mRNAs, impacts sRNA-mediated riboregulation or mRNA stability by binding to 5′- or 3′-untranslated regions as well as to internal stem loop structures. Global RNA-protein interaction studies and sequence comparisons identified a ProQ-like homolog (PA2582/ProQPae) in Pseudomonas aeruginosa (Pae). To address the function of ProQPae, at first a comparative transcriptome analysis of the Pae strains PAO1 and PAO1ΔproQ was performed. This study revealed more than 100 differentially abundant transcripts, affecting a variety of cellular functions. Among these transcripts were pprA and pprB, encoding the PprA/PprB two component system, psrA, encoding a transcriptional activator of pprB, and oprI, encoding the outer membrane protein OprI. RNA co-purification experiments with Strep-tagged Pae ProQ protein corroborated an association of ProQPae with these transcripts. In accordance with the up-regulation of the psrA, pprA, and pprB genes in strain PAO1ΔproQ a phenotypic analysis revealed an increased susceptibility toward the aminoglycosides tobramycin and gentamicin in biofilms. Conversely, the observed down-regulation of the oprI gene in PAO1ΔproQ could be reconciled with a decreased susceptibility toward the synthetic cationic antimicrobial peptide GW-Q6. Taken together, these studies revealed that ProQPae is an RBP that impacts antimicrobial resistance in Pae.
Introduction
The opportunistic pathogen Pseudomonas aeruginosa (Pae) is known to cause a variety of infections that are particularly harmful to immuno-compromised individuals (Beswick et al., 2020). A major obstacle in eradicating these infections is the high intrinsic resistance of Pae to a wide range of antibiotics (De Oliveira et al., 2020). In addition, the ability of Pae to form biofilms exacerbates antibiotic treatment, owing to restricted penetration and altered physiology (Maurice et al., 2018). Moreover, the metabolic versatility of Pae and the production of multiple virulence factors further augment the pathogenicity (Rojo, 2010; Azam and Khan, 2019). These traits are controlled by complex regulatory networks, employing sRNAs and RNA binding proteins (RBPs) (Sonnleitner et al., 2003, 2006; Mulcahy et al., 2008; Sonnleitner and Bläsi, 2014; Zhang et al., 2017; Pusic et al., 2018; Sonnleitner et al., 2020). The best characterized RBP in Pae is Hfq that assists sRNA-mediated riboregulation (reviewed in Vogel and Luisi, 2011; Kavita et al., 2018; Pusic et al., 2021), and serves together with the catabolite repression control protein Crc as a translational repressor of many transcripts encoding metabolic genes (Sonnleitner and Bläsi, 2014; Sonnleitner et al., 2018; Dendooven et al., 2023).
The FinO/ProQ family represents a diverse group of proteins that are widespread in α-, β-, and γ-Proteobacteria. It includes both specialized plasmid-encoded regulators, such as FinO, FopA and PcnR as well as chromosome encoded regulators like RocC and ProQ, the latter of which binds to sRNAs and mRNAs (reviewed in Glover et al., 2015; Olejniczak and Storz, 2017; Liao and Smirnov, 2023). In Escherichia coli and Salmonella enterica, ProQ was shown to associate with ~400 mRNAs and ~ 70 sRNAs (Smirnov et al., 2016; Holmqvist et al., 2018; Melamed et al., 2020). In E. coli, the deletion of proQ resulted in reduced levels of the proline and glycine betaine transporter ProP, and consequently in a reduced growth rate at high salt concentrations (Chaulk et al., 2011; Kerr et al., 2014). In addition, the proQ mutant strain was deficient in biofilm formation (Sheidy and Zielke, 2013), and showed a decrease in virulence (Wang et al., 2023). In S. enterica, ProQ modulates the expression of genes involved in motility and virulence (Westermann et al., 2019; Bergman et al., 2024), and impacts persister cell formation (Rizvanovic et al., 2022). Furthermore, ProQ affects the growth rate of S. enterica in full broth and minimal media with succinate as the sole C-source under microaerobic conditions (El Mouali et al., 2021), and was shown to be involved in sRNA-mediated riboregulation in this organism (Smirnov et al., 2017).
Structural and functional studies revealed that E. coli ProQ consists of the N-terminal FinO domain (NTD) (Smith et al., 2004; Gonzalez et al., 2017), which acts as an electrostatic scaffold for RNA binding (Pandey et al., 2020), and the C-terminal Tudor-like domain (CTD) (Ponting, 1997). Both domains are connected by an unstructured linker that is rich in positively charged amino acid (aa)-residues (Gonzalez et al., 2017). While the NTD is the principal RNA binding site with a preference for highly structured RNAs containing double-stranded regions (e.g., intrinsic terminators), the CTD has a broader RNA binding specificity (Pandey et al., 2020; Stein et al., 2020, 2023).
First hints that Pae protein PA2582 represents a ProQ-like FinO domain containing RBP came from sequence comparisons and a gradient profiling by sequencing (Grad-seq) approach performed with exponentially growing Pae O1 (Olejniczak and Storz, 2017; Gerovac et al., 2021). PA2582 displays a high sequence homology with the N-terminal FinO-domain of E. coli ProQ, including the majority of conserved residues important for RNA binding (Gonzalez et al., 2017; Gerovac et al., 2021). However, it lacks the C-terminal Tudor-like domain but contains a C-terminal extension of 36 aa (Liao and Smirnov, 2023).
In this study, we asked whether PA2582 has a regulatory role in Pae. A comparative RNASeq based transcriptome analysis of a PAO1 PA2582 deletion mutant and the corresponding wild-type revealed that PA2582 affects more than 100 genes, including the transcripts encoding the transcriptional regulator PsrA, the histidine kinase of the PprA/PprB two-component system (TCS) and the outer membrane protein OprI. Biofilms and liquid cultures of strain PAO1∆proQ showed an increased and decreased susceptibility toward the aminoglycosides tobramycin and gentamicin and the cationic peptide GW-Q6, respectively. In accordance with previous findings (de Bentzmann et al., 2012; Tseng et al., 2016), these observations can be reconciled with an increased expression of pprA/pprB and reduced transcript levels of oprI, respectively. RNA co-purification experiments with Strep-tagged PA2582 protein supported an interaction of the protein with these transcripts. Based on these observations and structural comparisons with ProQ homologs of Enterobacteriaceae, we propose the name ProQ for PA2582.
Materials and methods
Bacterial strains and growth conditions
Strains and plasmids used in this study are listed in Supplementary Table S1. If not indicated otherwise, the cultures were grown aerobically in LB (Lysogeny-broth) medium at 37°C (Miller, 1972). If required, Pae and E. coli were grown in the presence of 250 μg/mL carbenicillin or 50 μg/mL gentamicin and in the presence of 100 μg/mL ampicillin or 15 μg/mL of gentamicin, respectively. The genes controlled by the Ptac-promoter in plasmid pMMB67HE-derivatives were induced by addition of isopropyl β-D-1-thiogalactopyranoside (IPTG) (1 mM final concentration).
Construction of strains PAO1ΔproQ, PAO1-ProQFlag, PAO1-ProQStrep, and PAO1∆hfq∆proQ
To construct an in-frame deletion of Pae proQ and vectors for chromosomal integration of in-frame fusions of proQ to Strep-tag and Flag-tag encoding sequences, respectively, the following procedure was used. Two PCR products flanking the PAO1 proQ gene were obtained with chromosomal DNA of PAO1 as template. For the upstream fragment, 754 nucleotide (ΔproQ) and 1,285 nucleotide (proQStrep/proQFlag) long sequences were amplified using primer pairs D181/E181 (ΔproQ), D181/H181 (proQStrep) and D181/I181 (proQFlag), respectively. For the downstream fragment, a 770-nucleotide long sequence was amplified with the primer pair F181/G181. The resulting upstream and downstream fragments were then annealed and used as a template for a second overlapping PCR with oligonucleotides D181 and G181. The resulting PCR amplicons were cleaved with PstI and EcoRI and ligated into the corresponding sites of plasmid pEXG2. The generated plasmids pEXG2-ΔproQ, pEXG2-proQFlag and pEXG2-proQStrep were mobilized into PAO1 or PAO1∆hfq with the aid of the E. coli strain S17-1, and were finally chromosomally integrated through selection for gentamicin resistance. Excision of the vector by a second crossover event was achieved by selection for sucrose insensitive cells, as the pEXG2 vector encodes the Bacillus subtilis sacB gene, the product of which -levan sucrose- renders Pae sensitive to sucrose (Hmelo et al., 2015). The sequences of all mutagenic oligonucleotides used in this study are provided in Supplementary Table S2. All DNA manipulations were verified by DNA sequencing.
Construction of plasmids pMMB-proQStrep and pMMB-proQFlag
Chromosomal DNA of PAO1-ProQStrep and PAO1-ProQFlag were used as templates for PCR amplification together with oligonucleotides P185 and Q185 (Supplementary Table S2). The 663-base pairs (bp) and 657-bp long PCR products, encompassing the proQ gene abutted either to the Strep-tag or Flag-tag encoding sequence, were cleaved with PstI and EcoRI, and then ligated into the corresponding sites of plasmid pMMB67HE, resulting in plasmids pMMB-proQStrep and pMMB-proQFlag, respectively.
RNASeq
Total RNA was prepared from two biological replicates of strains PAO1 and PAO1ΔproQ grown in LB medium to an OD600 of 2.0. Then, 8 mL samples were withdrawn and total RNA was extracted using the hot phenol method (Leoni et al., 1996). The samples were treated with DNase I (TURBO™ DNase, Invitrogen), followed by phenol-chloroform-isoamyl alcohol (25:24:1) extraction and ethanol precipitation. Ribosomal RNA was depleted with the NEBNext rRNA Depletion Kit (Bacteria; New England BioLabs). The libraries were constructed using the NEBNext® Ultra™ Directional RNA Library Prep Kit for Illumina®. Hundred bp single end sequence reads were generated using the Illumina HiSeq 2000 platform at the in-house Next Generation Sequencing Facility (VBCF, Vienna, Austria1). Sequencing quality control of the raw reads was assessed using FastQC2 software and adaptor sequences were removed with Cutadapt (Martin, 2011). Mapping of the reads against the PAO1 reference genome (NCBI accession number NC_002516.2) was performed with Segemehl (Hoffmann et al., 2009) with default parameters. Reads per gene were counted using BEDTools (Quinlan and Hall, 2010) and the Refseq annotation of Pae (NC_002516.2). Differential gene expression analysis was performed with the DESeq2 R package (Love et al., 2014). All genes with a fold-change greater than 2 and a multiple testing adjusted p-values below 0.05 were considered to be modulated. The raw sequencing data were deposited in the European Nucleotide Archive (ENA) under accession number PRJEB73792.
Reverse transcription quantitative polymerase chain reactions
For the real time reverse transcription quantitative polymerase chain reaction (RT-qPCR), PAO1 and PAO1ΔproQ were grown in LB medium to an OD600 of 2.0. RNA extraction was carried out as described above for the RNASeq analysis. Then, cDNA was synthesized from 1 μg of DNA-free RNA using random hexamer primers (Promega) and SuperScript III reverse transcriptase (Thermo Fisher) as specified by the manufacturer. The real-time RT-qPCR was performed with 5 × HOT FIREPol EvaGreen® qPCR Mix Plus (no ROX) (Medibena), 25 ng cDNA, and 250 nM of each primer. For all reactions including the DNA standards and the negative control (no template), two biological replicates and three technical replicates were generated. The PCR was performed with specific oligonucleotides for psrA (G196/H196), pprA (A195/B195), pprB (U194/V194), and cupE1 (S194/T194) (Supplementary Table S2). The transcript levels of the rpoD gene obtained with the primer pair Q117/R117 (Supplementary Table S2) were used for normalization of the signals for RT-qPCR as described by Lee et al. (2012). Fold-changes in the psrA, pprA, pprB and cupE1 mRNA levels were calculated as previously described (Pfaffl, 2001).
Co-purification of mRNAs with ProQPae
PAO1∆proQ harboring either plasmid pMMB67HE (mock control) or pMMB-proQStrep were grown in 1 L LB medium to an OD600 of 2.0. The expression of the plasmid encoded proQStrep gene controlled by the Ptac promoter was induced throughout growth with IPTG (1 mM final concentration). The harvested cells were washed with buffer W (100 mM Tris/HCl pH 7.0, 150 mM NaCl, 1 mM EDTA) and further processed by resuspending them in buffer W containing 20 μg/mL lysozyme and 2 mM β-mercaptoethanol. Lysis was accomplished using a single cycle in a cell disruptor (Constant Systems Ltd.) with the pressure set at 1.9 kPa. After lysis, 1 mM phenylmethylsulfonyl fluoride (PMSF) was added. ProQ-Strep was further purified using Strep-Tactin® Sepharose following the protocol provided by the manufacturer (IBA). The RNA bound to ProQ-Strep was subjected to phenol-chloroform extraction and ethanol precipitation. The mock control was treated in the same way as the ProQ-Strep containing samples. For RT-PCR, cDNA was synthesized from 1 μg of DNA-free RNA that was bound to ProQ-Strep as well as from an equivalent volume of the mock control using random hexamer primers (Promega) and SuperScript III reverse transcriptase (Thermo Fisher) as specified by the manufacturer. For RT-PCR, 1 μL of a 1:10 dilution of the RT reaction or the corresponding amount of RNA (5 ng) without addition of reverse transcriptase (negative control) or an equivalent volume of the mock control with and without reverse transcriptase were used with Go-Taq Master Mix (Promega) and 30 cycles of PCR. The PCR was performed with specific oligonucleotides for psrA (G196/H196), pprA (A195/B195), pprB (U194/V194), and cupE1 (S194/T194) (Supplementary Table S2). The experiment was performed with two biological replicates.
Northern-blot analysis
The transcript levels of oprI were determined by Northern-blotting employing 10 μg of total RNA or 4 μg RNA bound to ProQ-Strep. The RNA samples were denatured for 5 min at 65°C in loading buffer containing 50% formamide, separated on 6% polyacrylamide gels containing 8 M urea, and then transferred to nylon membranes by electroblotting. The RNAs were cross-linked to the membrane by exposure to UV light and then hybridized with an oprI-specific 32P-labeled oligonucleotide (I84; Supplementary Table S2) at 55°C overnight. A 5S rRNA-specific oligonucleotide (I26; Supplementary Table S2) was used to detect 5S rRNA (loading control). The signals were visualized using a PhosphorImager (Molecular Dynamics).
Determination of the minimal bactericidal concentration of tobramycin and gentamicin for biofilms grown on glass beads
The MBCs of tobramycin and gentamicin were determined by growing PAO1 and PAO1ΔproQ biofilms on glass beads (Konrat et al., 2016). One autoclaved 4 mm glass bead (ROBU® Glasfilter-Geräte GmbH, Hattert, Germany) was placed into each well of a 48 well microtiter plate. The overnight cultures grown in LB medium were diluted to an OD600 of 0.05 in LB medium and dispensed into the bead-containing 48 well microplates (1 mL per well). The plate was then placed into a moisture box and incubated at 37°C for 24 h at 120 rpm on an orbital shaker. After 24 h, the liquid culture was removed, and the beads were washed twice with 1x PBS to remove loosely attached bacteria. Fresh medium was added with or without serial dilutions of the respective antibiotic (tobramycin: 3.0-12.0 µg/ml). Gentamicin: 3.0–48.0 μg/mL and incubated for additional 20 h at 37°C at 120 rpm on an orbital shaker. Subsequently, the beads were washed twice with 1x PBS and placed in a 2 mL microcentrifuge tube containing 1 mL of fresh 1x PBS. The samples were vortexed for 30 s, sonicated in an ultrasonic bath at 35 kHz for 20 min at 25°C, and vortexed again for 30 s. The bacterial suspensions were serially diluted in 1x PBS and spotted onto LB agar plates. After 24 h of incubation at 37°C, the biofilm cells were quantified as CFU/bead.
Antimicrobial activity of the GW-Q6 peptide against PAO1 and PAO1ΔproQ
The GW-Q6 peptide (GIKIAKKAITIAKKIAKIYW) was synthesized by ProteoGenix SAS (Schiltigheim, France) with more than 95% purity, and its molecular size was verified by mass spectrometry. The antimicrobial activity was tested as described by Tseng et al. (2016) with the following modifications. PAO1, PAO1ΔproQ, PAO1(pMMB67HE), PAO1ΔproQ(pMMB67HE), and PAO1ΔproQ(pMMB-proQFlag) were grown aerobically in LB medium to an OD600 of 2.0. The expression of proQFlag in strain PAO1ΔproQ(pMMB-proQFlag) was induced with IPTG (1 mM final concentration) 30 min before reaching an OD600 of 2.0. Cultures of PAO1(pMMB67HE) and PAO1ΔproQ(pMMB67HE) were treated with the same concentration of IPTG. Subsequently, approximately 104 cells were either left untreated or treated with a sub-inhibitory concentration (0.1 μM) of the GW-Q6 peptide for 1.5 h at 37°C. Then, serial dilutions were plated on LB agar plates and the corresponding CFU/ml were determined after overnight growth at 37°C. The values of the untreated cells were set to 100% and the percentage of cell survival for the treated cells were calculated accordingly.
Determination of OprI levels
PAO1, PAO1ΔproQ, PA14, and PA14ΔoprI strains were grown in 25 mL LB medium to an OD600 of 2.0. Then, the cells were harvested by centrifugation at 5,000 × g for 10 min at 4°C, resuspended in 5 mL 10 mM HEPES buffer (pH 7.8) and lysed by sonication. Cell debris were removed by centrifugation at 20,000 × g for 30 min. The cleared lysates were collected and centrifuged at 200,000 × g for 1 h at 4°C. The supernatants, which represents the cytoplasmic fraction, were discarded. The pellets, which contained the total membrane protein fraction, were dissolved in 500 μL of 10 mM HEPES buffer (pH 7.8) supplemented with N-lauryl sarcosine (final concentration 0.7%). The samples were incubated for 30 min at room temperature, and subsequently centrifuged at 200,000 × g for 2 h at 4°C. The resulting supernatants contained the inner membrane (IM) proteins, whereas the pellet fraction comprised the outer membrane (OM) proteins. The OM protein fractions were resuspended in 500 μL of 10 mM HEPES buffer (pH 7.8) containing 2% Triton-X100. The concentration of the OM proteins was assessed with the Pierce™ BCA Protein Assay Kit (Thermo Fisher). 15 μg of OM proteins were boiled in Laemmli-buffer (125 mM Tris/HCl pH 6.8, 3% (w/v) SDS, 10% (v/v) glycerol, 5% (v/v) β-mercaptoethanol and 0.0025% (w/v) Bromophenol blue), and separated on a 15.3% Tricine-SDS-polyacrylamide gel containing 4 M urea as described by Schägger (2006). The proteins were visualized by Coomassie Brilliant Blue R 250 staining and the protein levels were quantified with ImageQuantTL software.
Statistical analysis
Unless indicated otherwise, all experiments were performed in duplicate with two biological replicates. All statistical analyses were performed using GraphPad Prism 8. Except for the antimicrobial activity assay of the synthetic peptide GW-Q6, the statistical analyses were performed with a two-tailed distributed Student’s t-test, ns (non-significant); ∗p < 0.05, ∗∗p < 0.01, and ∗∗∗p ≤ 0.001. Due to the multiple comparisons for the antimicrobial activity assay of the synthetic peptide GW-Q6, the results were statistically analyzed by one-way ANOVA with the Tukey’s post hoc test. ns (non-significant); ∗p < 0.05, ∗∗p < 0.01, and ∗∗∗p < 0.001 were considered as statistically significant.
Results
PA2582 is a ProQ-like protein
To gain initial information whether PA2582 is a ProQ-like protein, the structures predicted by AlphaFold of PA2582 and the ProQ homologs of E. coli (ProQEco) and S. enterica (ProQSen), the NMR structure of the N-terminal FinO-domain of ProQEco and the crystal structure of ProQ (NMB1681) of Neisseria meningitidis (ProQNme) (Chaulk et al., 2010; Gonzalez et al., 2017; Jumper et al., 2021; Varadi et al., 2024) were superimposed (Figure 2). As anticipated from previous sequence comparisons (Gerovac et al., 2021; Liao and Smirnov, 2023), the moiety of PA2582 comprising amino acids 40–122 (α-helix 2 to 5; Supplementary Figure S1A) has a high structural similarity with the FinO-domain of all proteins (Figure 1). The α-helix 6 consists of one long helix and ends in a long unstructured stretch, comparable to ProQ of E. coli and S. enterica, but lacking the Tudor-like domain. On the other hand, the 31 aa extension at the N-terminus forms an α-helix connected to the FinO-domain with a seemingly unstructured linker. A N-terminal α-helix is also present in ProQNme, which however is devoid of the linker (Figure 1). As described for other ProQ homologs (Olejniczak and Storz, 2017), the electrostatic surface potential of PA2582 shows that parts of α-helix 1 and the inner side of the U-shape protein region formed by the linker between α-helix 1 and the FinO domain, the FinO domain itself and the α-helix 6 are positively charged, which could suggest an involvement in RNA binding (Supplementary Figure S1B). In addition, the conserved residues Y70 and R80 (E. coli numbering) that form the main RNA-binding site on this conserved concave face of ProQEco (Stein et al., 2023) are present in PA2582 (corresponding to Y101 and R111 in PA2582). The third essential residue for RNA binding, R58 (E. coli numbering) most likely corresponds to K89 in ProQPae, thus retaining the positive charge (Stein et al., 2023; Supplementary Figure S1A). As PA2582 shows significant structural similarities to known ProQ homologs, we henceforward term this protein ProQPae.
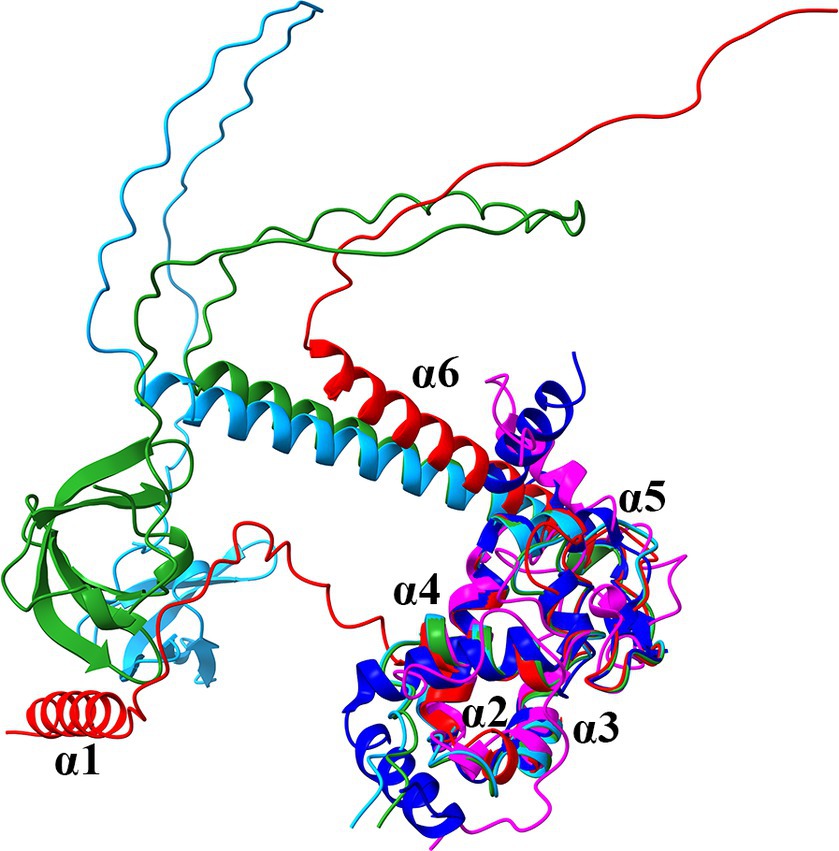
Figure 1. Superposition of the ribbon diagrams of the predicted AlphaFold structures of ProQPae (Q9I0Q4; red), the ProQ homologs of E. coli (P45577; light blue) and S. enterica (A0A3Y9V7K5; green), the N-terminal ProQ NMR structure of E. coli (PDB ID: 5NB9; magenta) and the crystal structure of ProQNme from N. meningitidis (PDB ID: 3 MW6; dark blue).
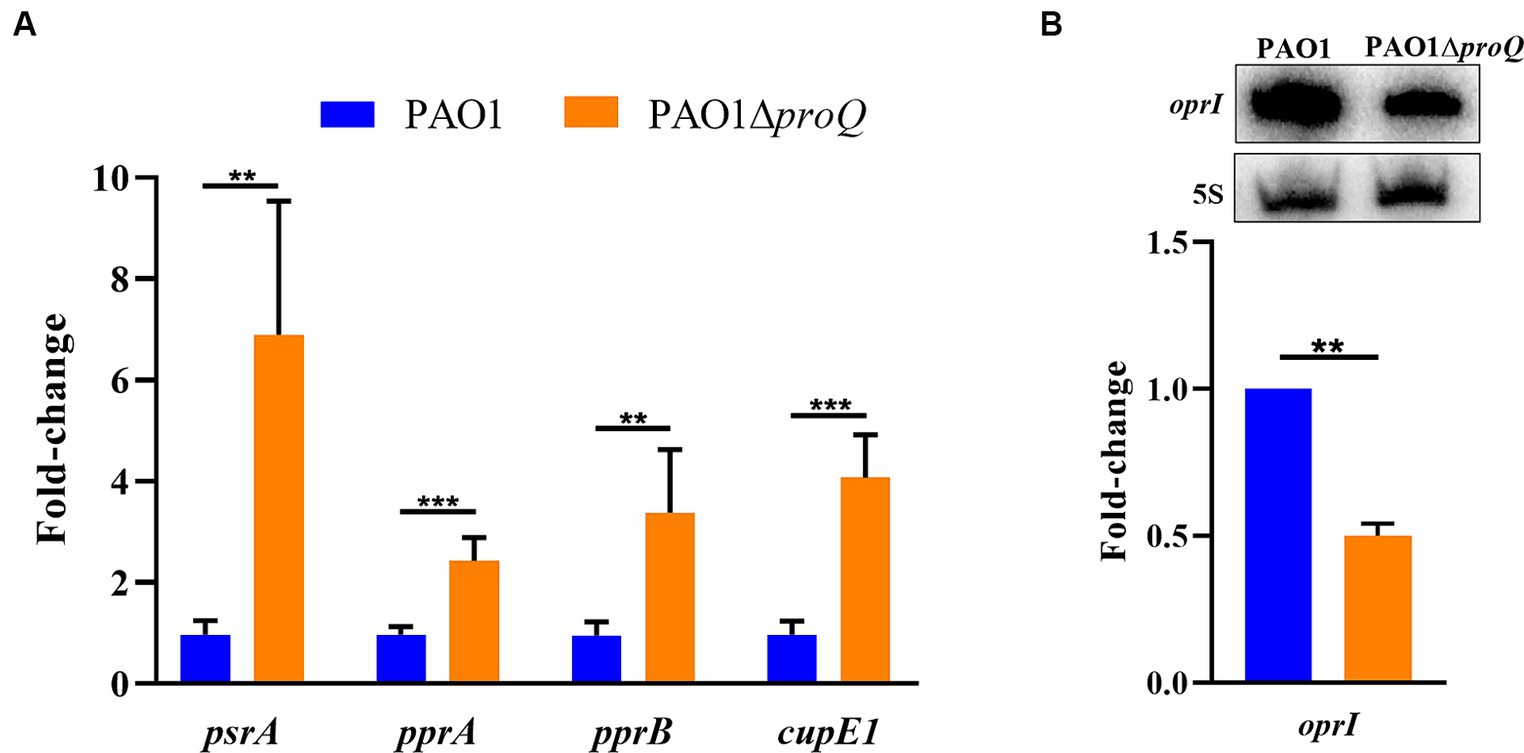
Figure 2. ProQPae affects the transcript levels of psrA, pprA, pprB, and cupE1. (A) Total RNA was purified from cultures of PAO1 and PAO1ΔproQ after growth in LB medium to an OD600 of 2.0. The RT-qPCR was carried out with three technical replicates derived from two biological replicates and is given as fold-change relative to PAO1. Error bars represent standard deviations from two biological replicates, each performed in triplicate. Significance was evaluated using a two-tailed Student’s t-test and indicated as follows: ∗∗p ≤ 0.01 and ∗∗∗p ≤ 0.001. (B) The Northern-blot signals for oprI mRNA were normalized to the signals of 5S rRNA (loading control). A representative picture of a Northern-blot is shown on top. Bottom, graphical representation of the data. The error bar represents the standard deviation from two biological replicates.
ProQPae is not involved in osmoregulation, oxidative stress response, motility and biofilm formation
In E. coli and S. enterica, ProQ is known to play a role in a wide range of biological processes including osmotic stress responses, motility and biofilm formation (Sheidy and Zielke, 2013; Kerr et al., 2014). ProQ of N. meningitidis, which lacks the Tudor-like domain like ProQPae, was shown to interact with RNAs and to protect transcripts from degradation. In addition, ProQNme is important to survive oxidative stress caused by H2O2 exposure (Bauriedl et al., 2020). To examine whether ProQPae is as well involved in these processes, we first tested whether ProQPae is important to cope with osmotic stress by growing PAO1 and PAO1ΔproQ on microtiter plates in LB medium containing either 0.17 M NaCl (no salt stress), 0.8 M (high salt) or 1.0 M NaCl (osmotic stress). As shown in Supplementary Figure S2A, we observed no growth difference between the two strains in response to high salt concentrations. Similarly, as observed for the growth in LB medium in microtiter plates without salt stress (Supplementary Figure S2A), we noted no growth difference between the two strains grown aerobically in Erlenmeyer flasks (not shown). Second, to test whether ProQPae plays a role in oxidative stress, the growth inhibition zones for PAO1 and PAO1∆proQ were determined in the presence of filter disks containing 10 μL of 30% H2O2. As shown in Supplementary Figure S2B, no difference in the diameter of the inhibition zones was observed. Third, PAO1 and PAO1ΔproQ showed the same swimming, swarming and twitching motility (Supplementary Figure S2C), and displayed no significant difference in the production of static biofilm after 24 h (Supplementary Figure S2D). In N. meningitidis deletion of hfq but not proQ reduced growth. However, inactivation of proQ in the hfq mutant led to further retardation of growth, suggesting that ProQ in N. meningitis is complementary but not fully exchangeable with Hfq (Bauriedl et al., 2020). To test whether this growth behavior holds for Pae, the growth of PAO1, PAO1Δhfq, PAO1ΔproQ, and PAO1ΔhfqΔproQ was monitored on microtiter plates in LB medium. Again, there was no significant difference in growth between PAO1Δhfq and PAO1ΔhfqΔproQ, and only the Hfq deficiency resulted in a growth defect (Supplementary Figure S2E).
ProQPae affects the abundance of transcripts encoding diverse proteins
To obtain information on the potential role of ProQPae as a gene expression regulator, a comparative RNASeq based transcriptome analysis was performed with strains PAO1 and PAO1ΔproQ. Prior to this study, we tested whether ProQPae is constitutively produced throughout growth. The protein levels were determined in strain PAO1-ProQFlag, carrying the chromosomally encoded ProQPae protein fused in frame to a C-terminal Flag-tag. The strain PAO1-ProQFlag was grown aerobically in LB medium and protein samples were collected at an OD600 of 1.0 (exponential phase), 2.0 (early stationary phase) and 3.0 (stationary phase), respectively. As shown in Supplementary Figure S3, the ProQFlag levels did not significantly change throughout growth.
The samples for the RNASeq analysis were withdrawn at an OD600 of 2.0 after aerobic growth of both strains in LB medium. This cell density was chosen with the rationale that regulatory RBPs are known to be employed to cope with stress, which increases by entering stationary phase. For differential gene expression analysis and interpretation, only annotated genes deposited in the Pseudomonas genome database (Winsor et al., 2016) were considered for comparison, while the Benjamin-Hochberg adjusted p-values (padj) (Benjamini and Hochberg, 1995) of 0.05 was set as a threshold for significance. Only transcripts with a fold-change equal to or greater than ±2 were considered differentially expressed. When compared with PAO1, a total of 161 transcripts were differentially abundant in PAO1∆proQ, with 63 and 97 genes being up- and down-regulated, respectively (Supplementary Table S3).
The functional classes representing the majority of down-regulated genes are related to translational and post-translational modification, whereas chaperone proteins were found to be mostly up-regulated in the absence of ProQPae. Furthermore, ProQPae apparently impacts gene functions encoding transcriptional regulators as well as functions involved in fatty acid-, phospholipid-, energy-, central intermediary-, carbon- and amino acid- metabolism. Interestingly, functions involved in transport of small molecules, protein secretion/export apparatus, membrane proteins and cell wall/lipopolysaccharide/capsule synthesis were also found to be differentially expressed (Supplementary Figure S4). On the other hand, none of the annotated Pae sRNAs (Winsor et al., 2016) were affected by the absence of ProQPae (Supplementary Table S3).
ProQPae alters the abundance of genes linked to antimicrobial resistance
As Pae is notorious for its high resistance against clinically used antibiotics, we focused in the follow-up studies on differentially regulated genes implicated in antibiotic susceptibility and membrane permeability. The most up-regulated gene in PAO1ΔproQ when compared to PAO1 was psrA (fold-change of 6.4) (Table 1; Supplementary Table S3). The expression of psrA is increased in the presence of cationic antimicrobial peptides (AMPs) (Gooderham et al., 2008). Moreover, a psrA mutant showed enhanced susceptibility to the AMPs polymyxin B and indolicidin, which correlated with an OM that was more easily permeabilized by these AMPs in the psrA mutant when compared with the wild-type strain. In addition, PsrA functions as a global regulator influencing biofilm formation, type III secretion, adhesion, and swarming motility. It acts as an autogenous repressor and activator of rpoS expression (Kojic et al., 2005; Gooderham et al., 2008). Furthermore, a previous microarray analysis revealed PsrA as a positive regulator of pprB expression encoding the response regulator of the PprA/PprB TCS (Gooderham et al., 2008). Overexpression of pprB resulted in increased susceptibility to aminoglycosides and hyper-biofilm formation that also led to increased susceptibility to tobramycin (Wang et al., 2003; de Bentzmann et al., 2012). When compared with PAO1, the transcript abundance of pprB was 1.7-fold increased in PAO1ΔproQ, and thus below the set threshold level. However, the corresponding two-component sensor kinase encoding gene pprA was 2.59-fold increased (Table 1; Supplementary Table S3). In addition, the transcript PA4294, forming an operon together with pprA, as well as the bapA and cupE1 genes that are known to be controlled by PprA/PprB, were up-regulated in the absence of ProQ (Table 1; Supplementary Table S3). Furthermore, the transcript encoding the envelope stress response regulator PA4596 (EsrC) was more than 2-fold up-regulated in the absence of ProQPae (Table 1; Supplementary Table S3). The expression of esrC is induced under envelope stress conditions. Together with the transcriptional regulator NfxB, it functions as a second repressor of the MexCD-OprJ multi drug resistance operon (Purssell et al., 2015; Lorusso et al., 2022). The MexCD-OprJ efflux system is mainly associated with the resistance to fluoroquinolones but can also extrude other antimicrobial agents (Lorusso et al., 2022). In addition, the transcript PA3584 (glpD), encoding the glycerol-3-phosphate-dehydrogenase, was 4.66-fold upregulated in the absence of ProQPae. A deletion of glpD resulted in increased persister cell formation after exposure to ofloxacin (Shuman et al., 2018).
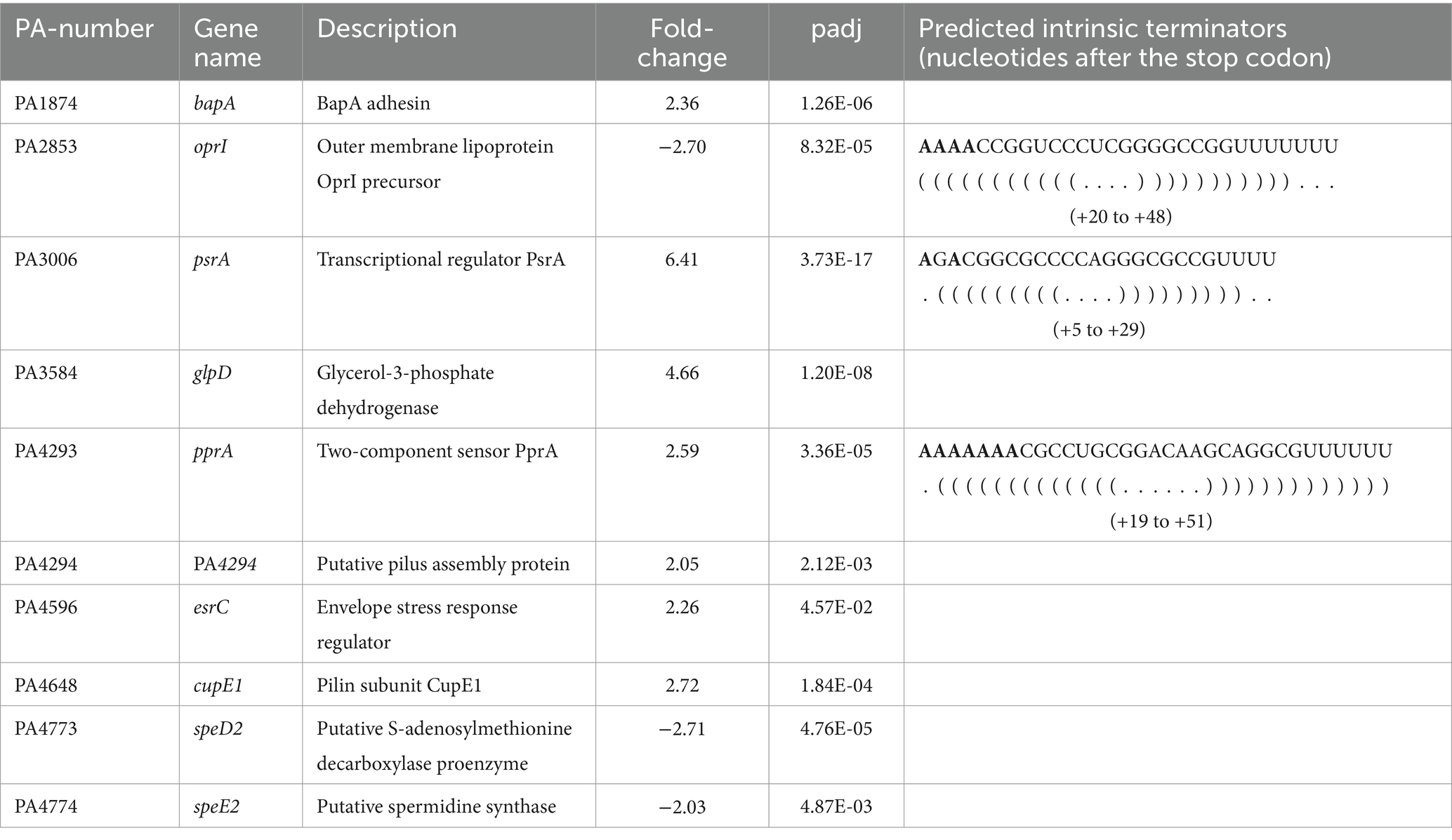
Table 1. Selection of genes related to antimicrobial resistance that were differently expressed in PAO1ΔproQ versus PAO1.
The oprI gene, encoding the major OM protein I (OprI) was 2.7-fold down-regulated in the absence of ProQPae (Table 1; Supplementary Table S3). OprI recruits and affects the susceptibility to α-helical AMPs, like GW-Q6 (Lin et al., 2010; Chang et al., 2015; Tseng et al., 2016). In addition, two transcripts involved in the synthesis of the polyamine spermidine, PA4773 (speD2) and PA4774 (speE2), showed reduced abundance in PAO1ΔproQ when compared with the wild type (Table 1; Supplementary Table S3). Spermidine contributes to polymyxin susceptibility by interacting with divalent cation-binding sites of lipopolysaccharides (LPS), which renders them inaccessible for polymyxin binding (Johnson et al., 2012). Moreover, inactivation of the gene speE2 alters the outer membrane permeability barrier to polymyxin B, CP10A, and gentamicin (Johnson et al., 2012). Taken together, ProQPae modulates the expression of genes involved in intrinsic and adaptive antibiotic resistance, primarily influencing functions related to membrane composition and permeability.
ProQPae interacts with transcripts encoding functions linked to antimicrobial resistance
To verify the RNASeq data, we next confirmed in strain PAO1ΔproQ the increased transcript levels of psrA, pprA, pprB, and cupE1 by RT-qPCR (Figure 2A) and the decreased transcript level of oprI by Northern-blotting (Figure 2B).
To gain further information on the potential interaction of ProQPae with these transcripts, we first inspected the data of the recently published Grad-seq analysis of Pae (Gerovac et al., 2021). This study investigated the interactions between RNA molecules and protein complexes at a global level. Native cellular lysates including RNA-protein complexes were partitioned on a glycerol gradient, fractionized and analyzed by sequencing and mass spectrometry (Gerovac et al., 2021). The majority of the ProQPae protein was found in fractions 3–11, which included the oprI (fractions 3 and 4), psrA (fractions 3–8), pprA (fractions 4 and 5), pprB (fraction 4) and cupE1 (fractions 4 and 7) transcripts (Supplementary Table S4; Gerovac et al., 2021). This re-assessment indicated that ProQPae may indeed associate with these transcripts. Moreover, in E. coli and S. enterica several RNA ligands of ProQ have A-rich motifs at the 5′-side of intrinsic terminator hairpins (Stein et al., 2020). Therefore, we asked whether the identified putative ProQ targets (Table 1) possess such sequences. As shown in Table 1, at least for oprI, psrA and pprA such intrinsic terminator sequences are present.
To show that ProQPae associates with the oprI, psrA, and pprA mRNAs, we next analyzed RNAs that were bound to Strep-tagged ProQPae protein. PAO1ΔproQ harboring plasmid pMMB-proQStrep was grown in LB medium to an OD600 of 2.0 and the RNAs bound to ProQPae-Strep were purified as described in Materials and Methods. Unspecific binding to the affinity matrix was controlled by a mock purification using strain PAO1ΔproQ harboring the parental plasmid pMMB67HE. After electrophoretic separation and ethidium bromide staining RNA was only visible in the ProQ-Strep derived sample but not in the mock control (Supplementary Figure S5). The presence of oprI was confirmed by Northern-blotting (Figure 3A) and that of psrA by RT-PCR (Figure 3B). For pprA, we only observed a weak signal by RT-PCR, questioning whether pprA is a direct target of ProQPae. In addition, the presence of pprB and cupE1 mRNAs was confirmed in the ProQ-Strep derived sample. None of these five mRNAs were detected by RT-PCR in the samples of the mock control. Taken together, these studies strongly indicated that ProQPae can act as an RBP.
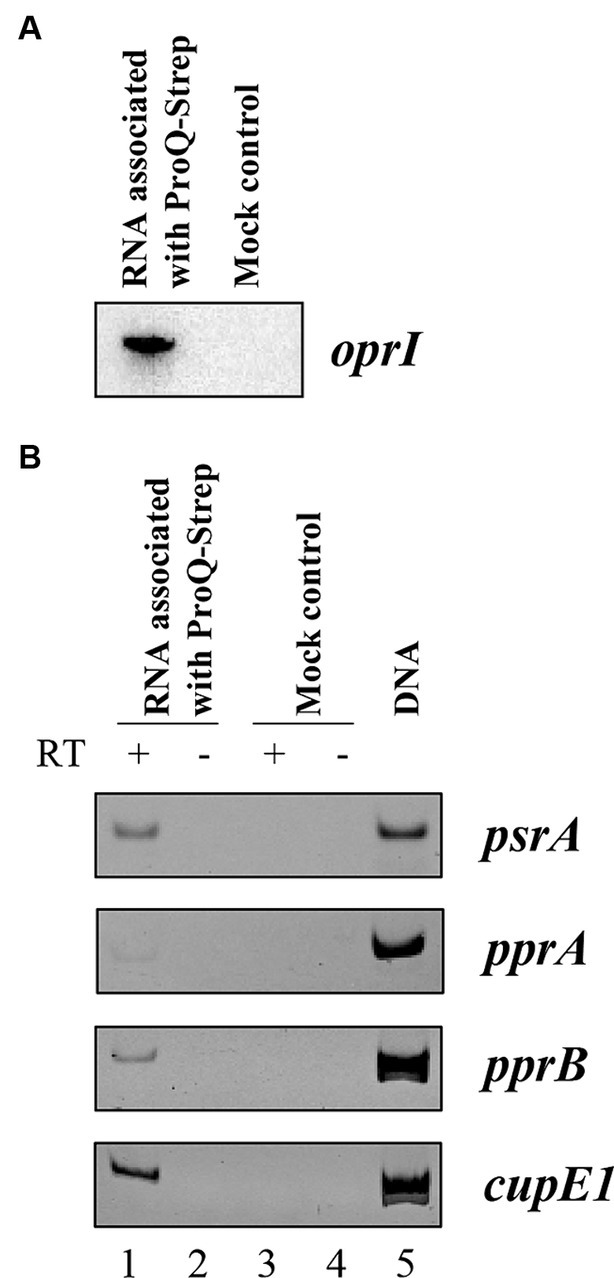
Figure 3. Co-purification of oprI, psrA, pprA, pprB, and cupE1 mRNAs with Strep-tagged ProQPae. Aliquots of the RNA samples associated with ProQPae-Strep were used for Northern-blotting to detect oprI mRNA (A), and for RT-PCR to detect psrA, pprA, pprB, and cupE1 mRNAs (B). PAO1∆proQ(pMMB-proQStrep) and PAO1∆proQ(pMMB67HE) (mock control) were grown in LB medium to an OD600 of 2.0. The RNA was isolated by phenol-chloroform extraction after ProQ-Strep protein purification by affinity chromatography using the Strep-Tactin® resin. The corresponding eluates of the mock control were obtained under the same conditions. PCR reactions with the RNA as template without reverse transcriptase reaction were used as negative control (lanes 2 and 4). Genomic DNA served as template for the positive control (lane 5).
ProQPae affects tobramycin and gentamicin susceptibility in biofilms
According to the studies presented above, ProQPae binds to and affects the abundance of the transcript psrA (Table 1; Figures 2A, 3B; Table 1; Supplementary Table S3). The lack of PsrA resulted in an increased membrane permeability and susceptibility to AMPs (Gooderham et al., 2008). To test whether ProQPae affects the susceptibility to AMPs, we determined the minimal inhibitory concentration of colistin in PAO1 and PAO1ΔproQ grown in LB medium. However, PAO1 and PAO1ΔproQ showed no difference in their susceptibility toward colistin (not shown).
As mentioned above, PsrA was identified as a positive regulator of pprB (Gooderham et al., 2008), and overexpression of pprB resulted in an increased susceptibility to tobramycin under biofilm growth conditions (de Bentzmann et al., 2012). As the transcript levels of psrA, pprB and pprA were elevated in the absence of ProQPae (Figure 2A), we next asked whether ProQ impacts the sensitivity toward aminoglycosides in biofilms by determining the MBC of biofilm cells for tobramycin and gentamicin in PAO1 and PAO1ΔproQ. Biofilms were formed on 4 mm glass beads for 24 h in LB medium and then treated with different concentrations of tobramycin and gentamicin for 20 h. Survivor cells on beads were quantified by counting the CFUs. PAO1ΔproQ biofilms were more susceptible to tobramycin (Figure 4) and gentamicin (Supplementary Figure S6) than PAO1 biofilms. However, despite the elevated levels of pprB in PAO1∆proQ and at variance with de Bentzmann et al. (2012), we did not observe an increased susceptibility toward tobramycin of planktonically growing PAO1∆proQ (not shown). This might be explained by the overexpression of pprB from a Ptac promoter (de Bentzmann et al., 2012), when compared to the rather moderately increased levels of pprB in PAO1∆proQ (Table 1).
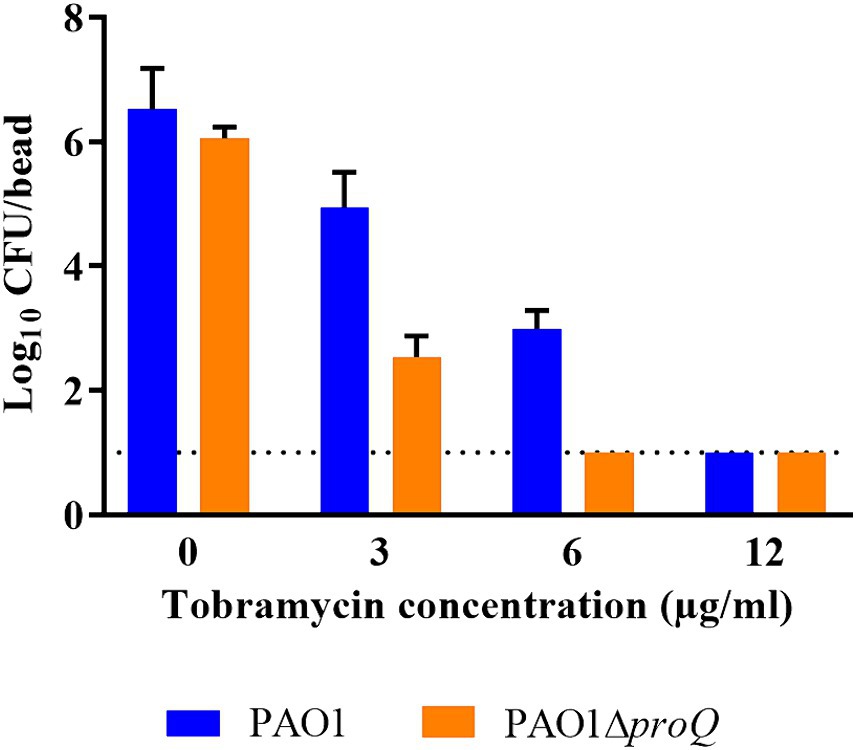
Figure 4. Determination of the minimal bactericidal concentration (MBC) of tobramycin in biofilms. Biofilms of PAO1 and PAO1ΔproQ were grown at 37°C on 4 mm glass beads submerged in LB medium as described in Materials and Methods. Biofilm formation is displayed as the logarithm of the CFU per glass bead (Log10 CFU/bead). Whenever the CFU count was zero, the value “1” (dashed line) was assigned. The error bars represent standard deviations from two biological replicates.
Increased susceptibility to sub-inhibitory concentration of GW-Q6 in the absence of ProQPae
The highly abundant OM lipoprotein OprI is targeted by naturally derived cationic AMPs such as SMAP-29, LL37 and human RNase7 in Pae (Lin et al., 2010). However, naturally occurring AMPs have low bioavailability and are prone to degradation (Moncla et al., 2011; Torcato et al., 2013). Therefore, synthetic AMPs were developed as a promising alternative strategy to combat multidrug-resistant pathogens (Chou et al., 2008; Lima et al., 2021). One of these newly designed cationic α-helical peptides, the synthetic AMP GW-Q6, has been shown to exert bactericidal activity in Pae by targeting OprI (Tseng et al., 2016). The transcript levels of oprI were 2.7-fold decreased in the absence of ProQPae (Table 1; Supplementary Table S3) suggesting that PAO1ΔproQ might exhibit increased resistance toward GW-Q6. To test this hypothesis, both strains were grown in LB medium to an OD600 of 2.0 and treated with a sub-inhibitory concentration of GW-Q6 for 1.5 h at 37°C (Figure 5A). The addition of the peptide reduced the survival of the wild-type strain by approximately 60%, while the lack of ProQ rendered the cells more resistant to the peptide, showing only a slight decrease in cell viability. Complementation of proQ through ectopic expression of the plasmid borne proQFlag gene in strain PAO1ΔproQ(pMMB-proQFlag) resulted again in increased sensitivity, whereas the presence of the empty vector pMMB67HE in PAO1 and PAO1ΔproQ showed the same susceptibility as the respective strains without plasmid (Figure 5A).
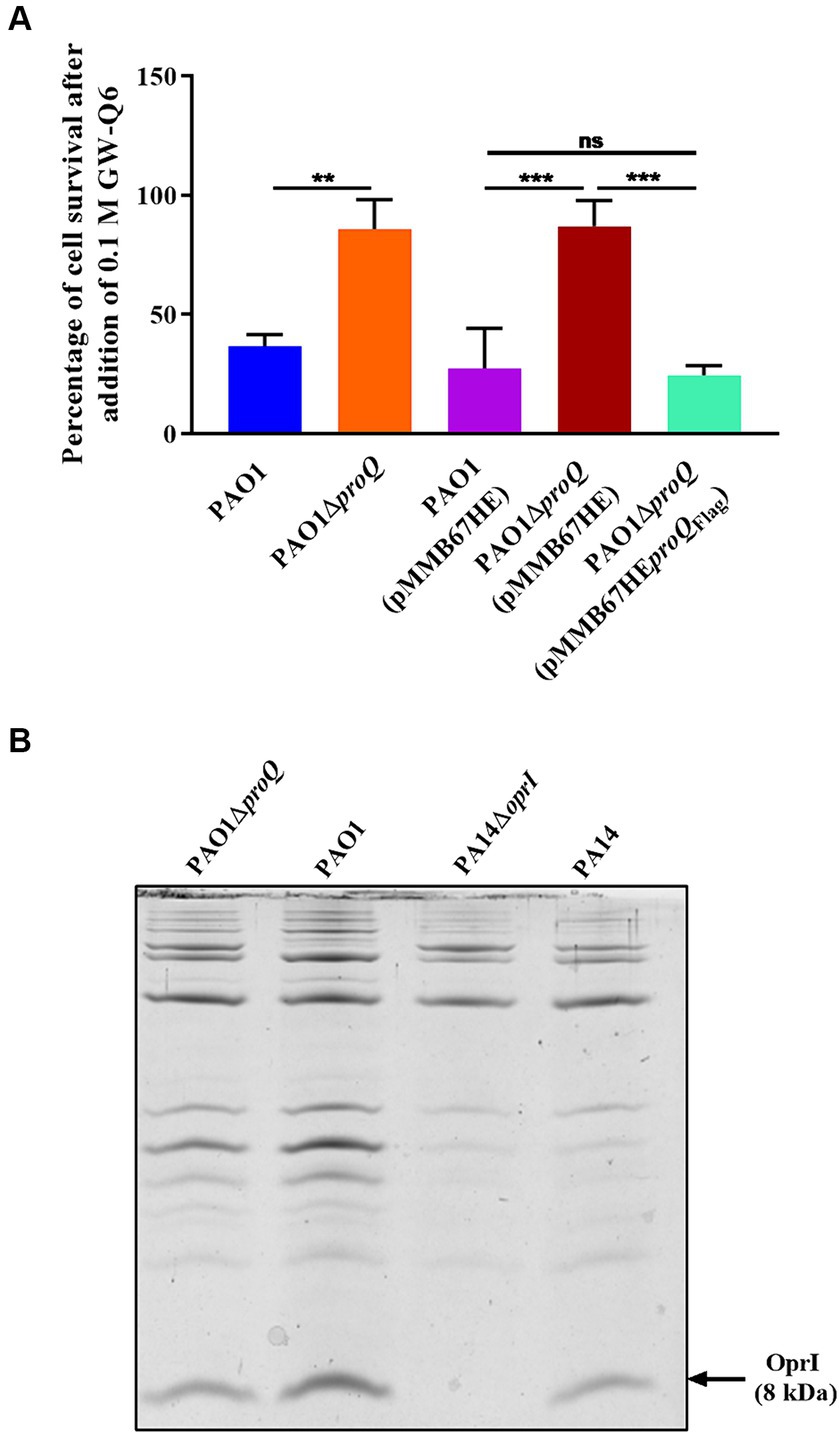
Figure 5. (A) Survival after exposure to the synthetic cationic AMP GW-Q6. PAO1 (blue), PAO1ΔproQ (orange), PAO1(pMMB67HE) (magenta), PAO1ΔproQ(pMMB67HE) (red) and PAO1ΔproQ(pMMB-proQFlag) (cyan) were grown aerobically in LB medium to an OD600 of 2.0. Then, the cultures were diluted to approximately 104 cells and treated with 0.1 μM of GW-Q6 peptide for 1.5 h. The percentage of cell survival was determined as described in Materials and Methods. The error bars represent standard deviations of two independent experiments. Statistical significance was determined using one-way ANOVA with the Tukey’s post hoc test. ns (non-significant), ∗∗p < 0.01 and ∗∗∗p < 0.001. (B) Determination of OprI protein levels. The strains PAO1, PAO1ΔproQ, PA14ΔoprI and PA14 were grown in LB medium. At an OD600 of 2.0, the cells were harvested, and the OM proteins were purified. The OM proteins were loaded on a 15.3% Tricine-SDS-polyacrylamide gel containing urea and stained with Coomassie Brilliant Blue. The arrow marks the position of the OprI protein.
To confirm that the increased survival of PAO1ΔproQ strain was indeed due to a diminished amount of OprI protein, the OM proteins of PAO1 and PAO1ΔproQ were purified as described in Materials and Methods and separated on a Tris-Tricine-Urea-PAGE gel followed by Coomassie Brilliant Blue staining (Figure 5B). To unambiguously identify the 8 kDa OprI protein, the OM proteins of PA14 and of the PA14ΔoprI strain (grown under the same experimental conditions) were loaded as a positive and a negative control, respectively. The absence of ProQPae in strain PAO1ΔproQ resulted in an approximately 55% reduction of the OprI protein levels (Figure 5B).
Discussion
The comparative transcriptome analysis clearly indicated that ProQPae acts – like its enterobacterial counterparts—as a regulator in Pae (Table 1; Supplementary Table S3). However, when compared with E. coli and S. enterica, the regulation of specific cellular functions by ProQPae seems to vary. In E. coli and S. enterica, ProQ is involved in osmoregulation, motility, and biofilm formation (Sheidy and Zielke, 2013; Kerr et al., 2014; Westermann et al., 2019). None of these processes seem to be affected by ProQPae (Supplementary Figure S2). Furthermore, some functions related to ProQNme (e.g., response to oxidative stress) were not affected by ProQPae (Supplementary Figure S2). Rather, ProQPae appears to control gene functions important for membrane integrity/permeability and antibiotic resistance in Pae.
We provided evidence that ProQPae affects antimicrobial susceptibility most likely through modulating the transcript levels of psrA, pprA, pprB, and oprI. There is only little information on FinO/ProQ-family proteins being involved in regulation of resistance functions. In most cases, the underlying mechanism seems to be related to the FinO/ProQ-family protein-mediated regulation of conjugation or replication of plasmids that contain antibiotic resistance genes (Dempsey, 1987; Gerovac et al., 2020; Yang et al., 2021). In S. enterica, ProQSen is involved in persister cell formation (Rizvanovic et al., 2022). ProQSen was shown to activate genes required for flagellum synthesis as well as genes of the pathogenicity island 2 (SPI-2), encoding a type III secretion system being important for intracellular survival. The enhanced expression of these genes causes an energetic burden, resulting in growth arrest of a subset of cells that are able to survive treatment with lethal concentrations of different antibiotics (Rizvanovic et al., 2022). We did not observe any difference in the swimming and swarming behavior of PAO1 and PAO1∆proQ (Supplementary Figure S2C), which are flagellum dependent (Henrichsen, 1972; Köhler et al., 2000). Thus, it seems rather unlikely that ProQPae affects persister cell formation by modulating flagellum biosynthesis (Supplementary Figure S2C).
PsrA was previously shown to be a positive regulator of type III secretion in a mucoid strain of Pae grown in complex medium (Shen et al., 2006). However, Gooderham et al. (2008) showed that PsrA is a negative regulator of type III secretion in the non-mucoid strain PAO1, and that a psrA mutant did not affect cytotoxicity toward epithelial cells, which is partially dependent on type III secretion. To our knowledge there is no evidence that PsrA is involved in persister cell formation in strain PAO1. It therefore remains elusive whether ProQPae can affect persister cell formation through modulation of the psrA transcript levels.
PsrA can act as a positive regulator of pprB. As shown in Figure 4 and Supplementary Figure S6, PAO1∆proQ showed an increased susceptibility toward tobramycin and gentamicin in biofilms, which can be reconciled with the elevated transcript levels of psrA, pprA and pprB (Figure 2A). The activation of the PprA/PprB TCS by PsrA results in an increased membrane permeability, which in turn leads to an increased sensitivity to tobramycin that is prevalent during biofilm conditions (de Bentzmann et al., 2012).
The activation of PprA/PprB in the absence of ProQPae is also in agreement with the increased expression of cupE1 and bapA (Table 1; Figure 2A), which are known to be under positive control of the TCS (Bernard et al., 2009; Giraud et al., 2011; de Bentzmann et al., 2012). As the psrA and pprB mRNAs interact with ProQ-Strep (Figure 3B), it is likely that ProQPae regulate pprB directly by binding to its mRNA and indirectly by modulating PsrA-mediated transcriptional regulation of pprB. Moreover, in the absence of ProQPae a reduced abundance of the speD2 and speE2 genes was observed (Table 1). These functions are involved in spermidine biosynthesis and might also contribute to tobramycin resistance by altering the membrane permeability (Johnson et al., 2012; Wilton et al., 2015). In any case, the reduced abundance of these transcripts in the absence of ProQPae would be in accord with the observation that a deletion of speE2 resulted in an increased aminoglycoside susceptibility in the presence of extracellular DNA, which contributes to biofilm formation (Whitchurch et al., 2002; Wilton et al., 2015).
The small major OM protein OprI plays a critical role in maintaining the integrity of the OM and serves as receptor for cationic α-helical AMPs such as the synthetic peptide GW-Q6 (Mizuno and Kageyama, 1979; Lin et al., 2010; Tseng et al., 2016). Binding of GW-Q6 to OprI causes a depolarization of the membrane and increases the membrane permeability (Tseng et al., 2016). Here, we have shown that the absence of ProQPae resulted in reduced oprI transcript levels (Figure 2B), and consequently OprI protein (Figure 5B). These results are consistent with the finding that the PAO1∆proQ strain displays an increased resistance toward sub-inhibitory concentration of the cationic AMP GW-Q6 (Figure 5A).
The co-purification studies with Strep-tagged ProQPae indicate that the protein associates with the psrA, pprB, cupE1 and oprI transcripts (Figure 3B). However, it remains to be shown how the protein affects their transcript abundance. For some ProQ homologs, it has been suggested that the protein binds to the 3′-ends of mRNAs and stabilizes these transcripts (Holmqvist et al., 2018; Bauriedl et al., 2020; Gulliver et al., 2022; Bergman et al., 2024). Alternatively or in addition, ProQPae might be involved in sRNA-mediated regulation of these genes. This was shown for RaiZ-mediated regulation of hupU mRNA in S. enterica. Here, ProQSen stabilizes the sRNA RaiZ and facilitates duplex formation between RaiZ and hupU, which results in translational repression (Smirnov et al., 2017). In turn, the lack of translation is known to destabilize transcripts (Deana and Belasco, 2005; Kaberdin and Bläsi, 2006).
We did not observe any sRNA transcript to be affected by ProQPae (Supplementary Table S3). However, as only a limited number of sRNAs are annotated in the Pae genome database (Winsor et al., 2016), we cannot exclude that as yet unknown sRNAs are concerned or that the function but not the stability of the sRNAs are affected by ProQPae.
In summary, this study provided evidence that ProQPae can act as an RBP and regulator of antibiotic resistance determinants. However, whether ProQPae affects the susceptibility to antibiotics in a positive or negative manner seems to vary with the antibiotic class (e.g., AMPs or aminoglycosides). Hence, a better understanding of the underlying molecular mechanism(s) by which ProQPae regulates the respective mRNAs might offer novel strategies to counteract antibiotic resistance of Pae.
Data availability statement
The datasets presented in this study can be found in online repositories. The names of the repository/repositories and accession number(s) can be found in the article/Supplementary material.
Author contributions
AC: Writing – review & editing, Writing – original draft, Investigation, Formal analysis, Data curation, Conceptualization. AR: Writing – review & editing, Investigation, Formal analysis, Data curation. IM: Writing – review & editing, Conceptualization. UB: Writing – review & editing, Writing – original draft, Funding acquisition, Formal analysis, Conceptualization. ES: Writing – review & editing, Writing – original draft, Investigation, Funding acquisition, Formal analysis, Data curation, Conceptualization.
Funding
The author(s) declare that financial support was received for the research, authorship, and/or publication of this article. The work was supported by the Austrian Science Fund (www.fwf.ac.at/en) through project P33617-B (UB and ES). AC was supported through the FWF funded doctoral program RNA-Biology W-1207.
Acknowledgments
We thank Drs Laura Wicke and Jörg Vogel, University of Würzburg, Germany, for providing details on their Grad-seq analysis of Pae prior to publication, and Beatrice Krennmayr and Sabine Bechter for technical assistance.
Conflict of interest
The authors declare that the research was conducted in the absence of any commercial or financial relationships that could be construed as a potential conflict of interest.
Publisher’s note
All claims expressed in this article are solely those of the authors and do not necessarily represent those of their affiliated organizations, or those of the publisher, the editors and the reviewers. Any product that may be evaluated in this article, or claim that may be made by its manufacturer, is not guaranteed or endorsed by the publisher.
Supplementary material
The Supplementary material for this article can be found online at: https://www.frontiersin.org/articles/10.3389/fmicb.2024.1422742/full#supplementary-material
Footnotes
References
Azam, M. W., and Khan, A. U. (2019). Updates on the pathogenicity status of Pseudomonas aeruginosa. Drug Discov. Today 24, 350–359. doi: 10.1016/j.drudis.2018.07.003
Bauriedl, S., Gerovac, M., Heidrich, N., Bischler, T., Barquist, L., Vogel, J., et al. (2020). The minimal meningococcal ProQ protein has an intrinsic capacity for structure-based global RNA recognition. Nat. Commun. 11:2823. doi: 10.1038/S41467-020-16650-6
Benjamini, Y., and Hochberg, Y. (1995). Controlling the false discovery rate: a practical and powerful approach to multiple testing. J. R. Stat. Soc. Ser. B 57, 289–300. doi: 10.1111/j.2517-6161.1995.tb02031.x
Bergman, S., Andresen, L., Kjellin, J., Martinez Burgo, Y., Geiser, P., Baars, S., et al. (2024). ProQ-dependent activation of Salmonella virulence genes mediated by post-transcriptional control of PhoP synthesis. mSphere 9:e0001824. doi: 10.1128/msphere.00018-24
Bernard, C. S., Bordi, C., Termine, E., Filloux, A., and De Bentzmann, S. (2009). Organization and PprB-dependent control of the Pseudomonas aeruginosa tad locus, involved in Flp pilus biology. J. Bacteriol. 191, 1961–1973. doi: 10.1128/JB.01330-08
Beswick, E., Amich, J., and Gago, S. (2020). Factoring in the complexity of the cystic fibrosis lung to understand aspergillus fumigatus and Pseudomonas aeruginosa interactions. Pathogens 9, 1–19. doi: 10.3390/pathogens9080639
Chang, T. W., Wang, C. F., Huang, H. J., Wang, I., Hsu, S. T., and Liao, Y. D. (2015). Key residues of outer membrane protein OprI involved in hexamer formation and bacterial susceptibility to cationic antimicrobial peptides. Antimicrob. Agents Chemother. 59, 6210–6222. doi: 10.1128/AAC.01406-15
Chaulk, S., Lu, J., Tan, K., Arthur, D. C., Edwards, R. A., Frost, L. S., et al. (2010). N. Meningitidis 1681 is a member of the FinO family of RNA chaperones. RNA Biol. 7, 812–819. doi: 10.4161/rna.7.6.13688
Chaulk, S., Smith-Frieday, M. N., Arthur, D. C., Culham, D. E., Edwards, R. A., Soo, P., et al. (2011). ProQ is an RNA chaperone that controls ProP levels in Escherichia coli. Biochemistry 50, 3095–3106. doi: 10.1021/BI101683A
Chou, H. T., Kuo, T. Y., Chiang, J. C., Pei, M. J., Yang, W. T., Yu, H. C., et al. (2008). Design and synthesis of cationic antimicrobial peptides with improved activity and selectivity against Vibrio spp. Int. J. Antimicrob. Agents 32, 130–138. doi: 10.1016/j.ijantimicag.2008.04.003
de Bentzmann, S., Giraud, C., Bernard, C. S., Calderon, V., Ewald, F., Plésiat, P., et al. (2012). Unique biofilm signature, drug susceptibility and decreased virulence in Drosophila through the Pseudomonas aeruginosa two-component system PprAB. PLoS Pathog. 8:e1003052. doi: 10.1371/journal.ppat.1003052
De Oliveira, D. M. P., Forde, B. M., Kidd, T. J., Harris, P. N. A., Schembri, M. A., Beatson, S. A., et al. (2020). Antimicrobial resistance in ESKAPE pathogens. Clin. Microbiol. Rev. 33, e00181–e00119. doi: 10.1128/CMR.00181-19
Deana, A., and Belasco, J. G. (2005). Lost in translation: the influence of ribosomes on bacterial mRNA decay. Genes Dev. 19, 2526–2533. doi: 10.1101/gad.1348805
Dempsey, W. B. (1987). Transcript analysis of the plasmid R100 traJ and finP genes. Mol. Gen. Genet. 209, 533–544. doi: 10.1007/BF00331160
Dendooven, T., Sonnleitner, E., Bläsi, U., and Luisi, B. F. (2023). Translational regulation by Hfq-Crc assemblies emerges from polymorphic ribonucleoprotein folding. EMBO J. 42:e111129. doi: 10.15252/embj.2022111129
El Mouali, Y., Ponath, F., Scharrer, V., Wenner, N., Hinton, J. C. D., and Vogel, J. (2021). Scanning mutagenesis of RNA-binding protein ProQ reveals a quality control role for the Lon protease. RNA 27, 1512–1527. doi: 10.1261/RNA.078954.121/-/DC1
Gerovac, M., El Mouali, Y., Kuper, J., Kisker, C., Barquist, L., and Vogel, J. (2020). Global discovery of bacterial RNA-binding proteins by RNase-sensitive gradient profiles reports a new FinO domain protein. RNA 26, 1448–1463. doi: 10.1261/rna.076992.120
Gerovac, M., Wicke, L., Chihara, K., Schneider, C., Lavigne, R., and Vogel, J. (2021). A grad-seq view of RNA and protein complexes in Pseudomonas aeruginosa under standard and bacteriophage predation conditions. MBio 12, 1–26. doi: 10.1128/mBio.03454-20
Giraud, C., Bernard, C. S., Calderon, V., Yang, L., Filloux, A., Molin, S., et al. (2011). The PprA-PprB two-component system activates CupE, the first non-archetypal Pseudomonas aeruginosa chaperone-usher pathway system assembling fimbriae. Environ. Microbiol. 13, 666–683. doi: 10.1111/J.1462-2920.2010.02372.X
Glover, J. N. M., Chaulk, S. G., Edwards, R. A., Arthur, D., Lu, J., and Frost, L. S. (2015). The FinO family of bacterial RNA chaperones. Plasmid 78, 79–87. doi: 10.1016/j.plasmid.2014.07.003
Gonzalez, G. M., Hardwick, S. W., Maslen, S. L., Mark Skehel, J., Holmqvist, E., Vogel, J., et al. (2017). Structure of the Escherichia coli ProQ RNA-binding protein. RNA 23, 696–711. doi: 10.1261/rna.060343.116
Gooderham, W. J., Bains, M., McPhee, J. B., Wiegand, I., and Hancock, R. E. W. (2008). Induction by cationic antimicrobial peptides and involvement in intrinsic polymyxin and antimicrobial peptide resistance, biofilm formation, and swarming motility of PsrA in Pseudomonas aeruginosa. J. Bacteriol. 190, 5624–5634. doi: 10.1128/JB.00594-08
Gulliver, E. L., Sy, B. M., Wong, J. L., Deveson Lucas, D. S., Powell, D. R., Harper, M., et al. (2022). The role and targets of the RNA-binding protein ProQ in the gram-negative bacterial pathogen Pasteurella multocida. J. Bacteriol. 204:e0059221. doi: 10.1128/jb.00592-21
Henrichsen, J. (1972). Bacterial surface translocation: a survey and a classification. Bacteriol. Rev. 36, 478–503. doi: 10.1128/br.36.4.478-503.1972
Hmelo, L. R., Borlee, B. R., Almblad, H., Love, M. E., Randall, T. E., Tseng, B. S., et al. (2015). Precision-engineering the Pseudomonas aeruginosa genome with two-step allelic exchange. Nat. Protoc. 10, 1820–1841. doi: 10.1038/nprot.2015.115
Hoffmann, S., Otto, C., Kurtz, S., Sharma, C. M., Khaitovich, P., Vogel, J., et al. (2009). Fast mapping of short sequences with mismatches, insertions and deletions using index structures. PLoS Comput. Biol. 5, e1000502–e1000510. doi: 10.1371/journal.pcbi.1000502
Holmqvist, E., Li, L., Bischler, T., Barquist, L., and Vogel, J. (2018). Global maps of ProQ binding in vivo reveal target recognition via RNA structure and stability control at mRNA 3′ ends. Mol. Cell 70, 971–982.e6. doi: 10.1016/j.molcel.2018.04.017
Johnson, L., Mulcahy, H., Kanevets, U., Shi, Y., and Lewenza, S. (2012). Surface-localized spermidine protects the Pseudomonas aeruginosa outer membrane from antibiotic treatment and oxidative stress. J. Bacteriol. 194, 813–826. doi: 10.1128/JB.05230-11
Jumper, J., Evans, R., Pritzel, A., Green, T., Figurnov, M., Ronneberger, O., et al. (2021). Highly accurate protein structure prediction with AlphaFold. Nature 596, 583–589. doi: 10.1038/s41586-021-03819-2
Kaberdin, V. R., and Bläsi, U. (2006). Translation initiation and the fate of bacterial mRNAs. FEMS Microbiol. Rev. 30, 967–979. doi: 10.1111/j.1574-6976.2006.00043.x
Kavita, K., de Mets, F., and Gottesman, S. (2018). New aspects of RNA-based regulation by Hfq and its partner sRNAs. Curr. Opin. Microbiol. 42, 53–61. doi: 10.1016/j.mib.2017.10.014
Kerr, C. H., Culham, D. E., Marom, D., and Wood, J. M. (2014). Salinity-dependent impacts of ProQ, Prc, and Spr deficiencies on Escherichia coli cell structure. J. Bacteriol. 196, 1286–1296. doi: 10.1128/JB.00827-13
Köhler, T., Curty, L. K., Barja, F., van Delden, C., and Pechère, J. C. (2000). Swarming of Pseudomonas aeruginosa is dependent on cell-to-cell signaling and requires flagella and pili. J. Bacteriol. 182, 5990–5996. doi: 10.1128/JB.182.21.5990-5996.2000
Kojic, M., Jovcic, B., Vindigni, A., Odreman, F., and Venturi, V. (2005). Novel target genes of PsrA transcriptional regulator of Pseudomonas aeruginosa. FEMS Microbiol. Lett. 246, 175–181. doi: 10.1016/j.femsle.2005.04.003
Konrat, K., Schwebke, I., Laue, M., Dittmann, C., Levin, K., Andrich, R., et al. (2016). The bead assay for biofilms: a quick, easy and robust method for testing disinfectants. PLoS One 11:e0157663. doi: 10.1371/JOURNAL.PONE.0157663
Lee, K. M., Go, J., Yoon, M. Y., Park, Y., Kim, S. C., Yong, D. E., et al. (2012). Vitamin B12-mediated restoration of defective anaerobic growth leads to reduced biofilm formation in Pseudomonas aeruginosa. Infect. Immun. 80, 1639–1649. doi: 10.1128/IAI.06161-11
Leoni, L., Ciervo, A., Orsi, N., and Visca, P. (1996). Iron-regulated transcription of the pvdA gene in Pseudomonas aeruginosa: effect of Fur and PvdS on promoter activity. J. Bacteriol. 178, 2299–2313. doi: 10.1128/JB.178.8.2299-2313.1996
Liao, Z., and Smirnov, A. (2023). FinO/ProQ-family proteins: an evolutionary perspective. Biosci. Rep. 43:20220313. doi: 10.1042/BSR20220313
Lima, P. G., Oliveira, J. T. A., Amaral, J. L., Freitas, C. D. T., and Souza, P. F. N. (2021). Synthetic antimicrobial peptides: characteristics, design, and potential as alternative molecules to overcome microbial resistance. Life Sci. 278:119647. doi: 10.1016/j.lfs.2021.119647
Lin, Y. M., Wu, S. J., Chang, T. W., Wang, C. F., Suen, C. S., Hwang, M. J., et al. (2010). Outer membrane protein I of Pseudomonas aeruginosa is a target of cationic antimicrobial peptide/protein. J. Biol. Chem. 285, 8985–8994. doi: 10.1074/jbc.M109.078725
Lorusso, A. B., Carrara, J. A., Barroso, C. D. N., Tuon, F. F., and Faoro, H. (2022). Role of efflux pumps on antimicrobial resistance in Pseudomonas aeruginosa. Int. J. Mol. Sci. 23:15779. doi: 10.3390/ijms232415779
Love, M. I., Huber, W., and Anders, S. (2014). Moderated estimation of fold change and dispersion for RNA-seq data with DESeq2. Genome Biol. 15:550. doi: 10.1186/s13059-014-0550-8
Martin, M. (2011). Cutadapt removes adapter sequences from high-throughput sequencing reads. EMBnet.journal 17:10. doi: 10.14806/ej.17.1.200
Maurice, N. M., Bedi, B., and Sadikot, R. T. (2018). Pseudomonas aeruginosa biofilms: host response and clinical implications in lung infections. Am. J. Respir. Cell Mol. Biol. 58, 428–439. doi: 10.1165/rcmb.2017-0321TR
Melamed, S., Adams, P. P., Zhang, A., Zhang, H., and Storz, G. (2020). RNA-RNA interactomes of ProQ and Hfq reveal overlapping and competing roles. Mol. Cell 77, 411–425.e7. doi: 10.1016/j.molcel.2019.10.022
Mizuno, T., and Kageyama, M. (1979). Isolation of characterization of a major outer membrane protein of Pseudomonas aeruginosa. Evidence for the occurrence of a lipoprotein. J. Biochem. 85, 115–122. doi: 10.1093/oxfordjournals.jbchem.a132300
Moncla, B. J., Pryke, K., Rohan, L. C., and Graebing, P. W. (2011). Degradation of naturally occurring and engineered antimicrobial peptides by proteases. Adv. Biosci. Biotechnol. 2, 404–408. doi: 10.4236/abb.2011.26059
Mulcahy, H., O'Callaghan, J., O'Grady, E. P., Maciá, M. D., Borrell, N., Gómez, C., et al. (2008). Pseudomonas aeruginosa RsmA plays an important role during murine infection by influencing colonization, virulence, persistence, and pulmonary inflammation. Infect. Immun. 76, 632–638. doi: 10.1128/IAI.01132-07
Olejniczak, M., and Storz, G. (2017). ProQ/FinO-domain proteins: another ubiquitous family of RNA matchmakers? Mol. Microbiol. 104, 905–915. doi: 10.1111/MMI.13679
Pandey, S., Gravel, C. M., Stockert, O. M., Wang, C. D., Hegner, C. L., LeBlanc, H., et al. (2020). Genetic identification of the functional surface for RNA binding by Escherichia coli ProQ. Nucleic Acids Res. 48, 4507–4520. doi: 10.1093/NAR/GKAA144
Pfaffl, M. W. (2001). A new mathematical model for relative quantification in real-time RT–PCR. Nucleic Acids Res. 29:e45, 45e–445e. doi: 10.1093/NAR/29.9.E45
Ponting, C. P. (1997). Tudor domains in proteins that interact with RNA. Trends Biochem. Sci. 22, 51–52. doi: 10.1016/S0968-0004(96)30049-2
Purssell, A., Fruci, M., Mikalauskas, A., Gilmour, C., and Poole, K. (2015). EsrC, an envelope stress-regulated repressor of the mexCD-oprJ multidrug efflux operon in Pseudomonas aeruginosa. Environ. Microbiol. 17, 186–198. doi: 10.1111/1462-2920.12602
Pusic, P., Sonnleitner, E., and Bläsi, U. (2021). Specific and global RNA regulators in Pseudomonas aeruginosa. Int. J. Mol. Sci. 22:8632. doi: 10.3390/IJMS22168632
Pusic, P., Sonnleitner, E., Krennmayr, B., Heitzinger, D. A., Wolfinger, M. T., Resch, A., et al. (2018). Harnessing metabolic regulation to increase Hfq-dependent antibiotic susceptibility in Pseudomonas aeruginosa. Front. Microbiol. 9:2709. doi: 10.3389/fmicb.2018.02709
Quinlan, A. R., and Hall, I. M. (2010). BEDTools: a flexible suite of utilities for comparing genomic features. Bioinformatics 26, 841–842. doi: 10.1093/bioinformatics/btq033
Rizvanovic, A., Michaux, C., Panza, M., Iloglu, Z., Helaine, S., Wagner, G. E. H., et al. (2022). The RNA-binding protein ProQ promotes antibiotic persistence in Salmonella. MBio 13:e0289122. doi: 10.1128/mbio.02891-22
Rojo, F. (2010). Carbon catabolite repression in Pseudomonas: optimizing metabolic versatility and interactions with the environment. FEMS Microbiol. Rev. 34, 658–684. doi: 10.1111/j.1574-6976.2010.00218.x
Sheidy, D. T., and Zielke, R. A. (2013). Analysis and expansion of the role of the Escherichia coli protein ProQ. PLoS One 8:79656. doi: 10.1371/JOURNAL.PONE.0079656
Shen, D. K., Filopon, D., Kuhn, L., Polack, B., and Toussaint, B. (2006). PsrA is a positive transcriptional regulator of the type III secretion system in Pseudomonas aeruginosa. Infect. Immun. 74, 1121–1129. doi: 10.1128/IAI.74.2.1121-1129.2006
Shuman, J., Giles, T. X., Carroll, L., Tabata, K., Powers, A., Suh, S. J., et al. (2018). Transcriptome analysis of a Pseudomonas aeruginosa sn-glycerol-3-phosphate dehydrogenase mutant reveals a disruption in bioenergetics. Microbiology (United Kingdom) 164, 551–562. doi: 10.1099/mic.0.000646
Smirnov, A., Förstner, K. U., Holmqvist, E., Otto, A., Günster, R., Becher, D., et al. (2016). Grad-seq guides the discovery of ProQ as a major small RNA-binding protein. Proc. Natl. Acad. Sci. USA 113, 11591–11596. doi: 10.1073/pnas.1609981113
Smirnov, A., Wang, C., Drewry, L. L., and Vogel, J. (2017). Molecular mechanism of mRNA repression in trans by a ProQ-dependent small RNA. EMBO J. 36, 1029–1045. doi: 10.15252/embj.201696127
Smith, M. N., Crane, R. A., Keates, R. A., and Wood, J. M. (2004). Overexpression, purification, and characterization of ProQ, a posttranslational regulator for osmoregulatory transporter ProP of Escherichia coli. Biochemistry 43, 12979–12989. doi: 10.1021/bi048561g
Sonnleitner, E., and Bläsi, U. (2014). Regulation of Hfq by the RNA CrcZ in Pseudomonas aeruginosa carbon catabolite repression. PLoS Genet. 10:e1004440. doi: 10.1371/journal.pgen.1004440
Sonnleitner, E., Hagens, S., Rosenau, F., Wilhelm, S., Habel, A., Jäger, K. E., et al. (2003). Reduced virulence of a hfq mutant of Pseudomonas aeruginosa O1. Microb. Pathog. 35, 217–228. doi: 10.1016/s0882-4010(03)00149-9
Sonnleitner, E., Pusic, P., Wolfinger, M. T., and Bläsi, U. (2020). Distinctive regulation of carbapenem susceptibility in Pseudomonas aeruginosa by Hfq. Front. Microbiol. 11:1001. doi: 10.3389/fmicb.2020.01001
Sonnleitner, E., Schuster, M., Sorger-Domenigg, T., Greenberg, E. P., and Bläsi, U. (2006). Hfq-dependent alterations of the transcriptome profile and effects on quorum sensing in Pseudomonas aeruginosa. Mol. Microbiol. 59, 1542–1558. doi: 10.1111/j.1365-2958.2006.05032.x
Sonnleitner, E., Wulf, A., Campagne, S., Pei, X. Y., Wolfinger, M. T., Forlani, G., et al. (2018). Interplay between the catabolite repression control protein Crc, Hfq and RNA in Hfq-dependent translational regulation in Pseudomonas aeruginosa. Nucleic Acids Res. 46, 1470–1485. doi: 10.1093/nar/gkx1245
Stein, E. M., Kwiatkowska, J., Basczok, M. M., Gravel, C. M., Berry, K. E., and Olejniczak, M. (2020). Determinants of RNA recognition by the FinO domain of the Escherichia coli ProQ protein. Nucleic Acids Res. 48, 7502–7519. doi: 10.1093/nar/gkaa497
Stein, E. M., Wang, S., Dailey, K. G., Gravel, C. M., Wang, S., Olejniczak, M., et al. (2023). Biochemical and genetic dissection of the RNA-binding surface of the FinO domain of Escherichia coli ProQ. RNA 29, 1772–1791. doi: 10.1261/rna.079697.123
Torcato, I. M., Huang, Y. H., Franquelim, H. G., Gaspar, D., Craik, D. J., Castanho, M. A., et al. (2013). Design and characterization of novel antimicrobial peptides, R-BP100 and RW-BP100, with activity against gram-negative and gram-positive bacteria. Biochim. Biophys. Acta 1828, 944–955. doi: 10.1016/j.bbamem.2012.12.002
Tseng, T. S., Wang, S. H., Chang, T. W., Wei, H. M., Wang, Y. J., Tsai, K. C., et al. (2016). Sarkosyl-induced helical structure of an antimicrobial peptide GW-Q6 plays an essential role in the binding of surface receptor OprI in Pseudomonas aeruginosa. PLoS One 11:e0164597. doi: 10.1371/journal.pone.0164597
Varadi, M., Bertoni, D., Magana, P., Paramval, U., Pidruchna, I., Radhakrishnan, M., et al. (2024). AlphaFold protein structure database in 2024: providing structure coverage for over 214 million protein sequences. Nucleic Acids Res. 52, D368–D375. doi: 10.1093/nar/gkad1011
Vogel, J., and Luisi, B. F. (2011). Hfq and its constellation of RNA. Nat. Rev. Microbiol. 9, 578–589. doi: 10.1038/nrmicro2615
Wang, Z., Chen, R., Xia, F., Jiang, M., Zhu, D., Zhang, Y., et al. (2023). ProQ binding to small RNA RyfA promotes virulence and biofilm formation in avian pathogenic Escherichia coli. Vet. Res. 54:109. doi: 10.1186/s13567-023-01241-2
Wang, Y., Ha, U., Zeng, L., and Jin, S. (2003). Regulation of membrane permeability by a two-component regulatory system in Pseudomonas aeruginosa. Antimicrob. Agents Chemother. 47, 95–101. doi: 10.1128/AAC.47.1.95-101.2003
Westermann, A. J., Venturini, E., Sellin, M. E., Förstner, K. U., Hardt, W. D., and Vogel, J. (2019). The major RNA-binding protein ProQ impacts virulence gene expression in Salmonella enterica serovar typhimurium. MBio 10, e02504–e02518. doi: 10.1128/mBio.02504-18
Whitchurch, C. B., Tolker-Nielsen, T., Ragas, P. C., and Mattick, J. S. (2002). Extracellular DNA required for bacterial biofilm formation. Science 295:1487. doi: 10.1126/science.295.5559.1487
Wilton, M., Charron-Mazenod, L., Moore, R., and Lewenza, S. (2015). Extracellular DNA acidifies biofilms and induces aminoglycoside resistance in Pseudomonas aeruginosa. Antimicrob. Agents Chemother. 60, 544–553. doi: 10.1128/AAC.01650-15
Winsor, G. L., Griffiths, E. J., Lo, R., Dhillon, B. K., Shay, J. A., and Brinkman, F. S. L. (2016). Enhanced annotations and features for comparing thousands of Pseudomonas genomes in the Pseudomonas genome database. Nucleic Acids Res. 44, D646–D653. doi: 10.1093/nar/gkv1227
Yang, J., Wang, H. H., Lu, Y., Yi, L. X., Deng, Y., Lv, L., et al. (2021). A ProQ/FinO family protein involved in plasmid copy number control favours fitness of bacteria carrying mcr-1-bearing IncI2 plasmids. Nucleic Acids Res. 49, 3981–3996. doi: 10.1093/nar/gkab149
Keywords: RNA-binding protein, ProQ, Pseudomonas aeruginosa, antibiotic resistance, aminoglycosides, antimicrobial peptides
Citation: Cianciulli Sesso A, Resch A, Moll I, Bläsi U and Sonnleitner E (2024) The FinO/ProQ-like protein PA2582 impacts antimicrobial resistance in Pseudomonas aeruginosa. Front. Microbiol. 15:1422742. doi: 10.3389/fmicb.2024.1422742
Edited by:
Guangshun Wang, University of Nebraska Medical Center, United StatesReviewed by:
Zhongjie Li, Henan University of Science and Technology, ChinaMohammad Tahir Siddiqui, Indian Institute of Technology Delhi, India
Copyright © 2024 Cianciulli Sesso, Resch, Moll, Bläsi and Sonnleitner. This is an open-access article distributed under the terms of the Creative Commons Attribution License (CC BY). The use, distribution or reproduction in other forums is permitted, provided the original author(s) and the copyright owner(s) are credited and that the original publication in this journal is cited, in accordance with accepted academic practice. No use, distribution or reproduction is permitted which does not comply with these terms.
*Correspondence: Udo Bläsi, dWRvLmJsYWVzaUB1bml2aWUuYWMuYXQ=; Elisabeth Sonnleitner, ZWxpc2FiZXRoLnNvbm5sZWl0bmVyQHVuaXZpZS5hYy5hdA==