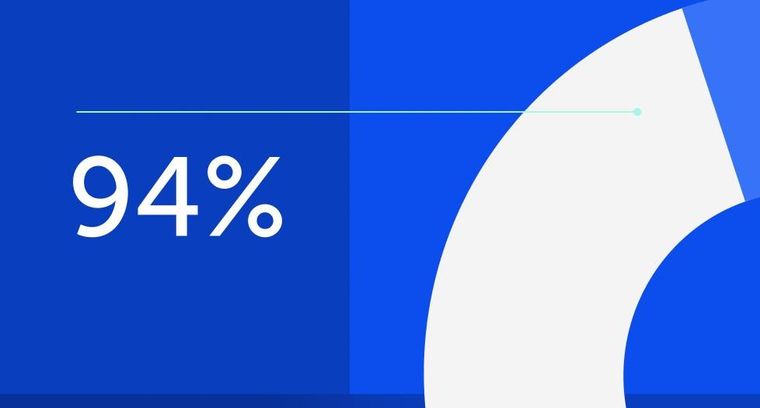
94% of researchers rate our articles as excellent or good
Learn more about the work of our research integrity team to safeguard the quality of each article we publish.
Find out more
ORIGINAL RESEARCH article
Front. Microbiol., 07 June 2024
Sec. Microbiological Chemistry and Geomicrobiology
Volume 15 - 2024 | https://doi.org/10.3389/fmicb.2024.1403279
This article is part of the Research TopicEnvironmental Remediation Strategies of New and Emerging Chemical ContaminantsView all 13 articles
Biodegradation was considered a promising and environmentally friendly method for treating environmental pollution caused by diuron. However, the mechanisms of biodegradation of diuron required further research. In this study, the degradation process of diuron by Achromobacter xylosoxidans SL-6 was systematically investigated. The results suggested that the antioxidant system of strain SL-6 was activated by adding diuron, thereby alleviating their oxidative stress response. In addition, degradation product analysis showed that diuron in strain SL-6 was mainly degraded by urea bridge cleavage, dehalogenation, deamination, and ring opening, and finally cis, cis-muconic acid was generated. The combined analysis of metabolomics and transcriptomics revealed the biodegradation and adaptation mechanism of strain SL-6 to diuron. Metabolomics analysis showed that after the strain SL-6 was exposed to diuron, metabolic pathways such as tricarboxylic acid cycle (cis, cis-muconic acid), glutathione metabolism (oxidized glutathione), and urea cycle (arginine) were reprogrammed in the cells. Furthermore, diuron could induce the production of membrane transport proteins in strain SL-6 cells and overexpress antioxidant enzyme genes, finally ultimately promoting the up-regulation of genes encoding amide hydrolases and dioxygenases, which was revealed by transcriptomics studies. This work enriched the biodegradation mechanism of phenylurea herbicides and provided guidance for the removal of diuron residues in the environment and promoting agriculture sustainable development.
Diuron (3-(3,4 dichlorophenyl)-1,1 dimethylurea) was a phenylurea herbicide that was extensively acted to Broadleaf weeds and grassy weeds in diverse crops fields such as cotton, fruit, and cereals (Li et al., 2021; Wang et al., 2022). Its function was to block the binding sites of plastid quinones in photosynthetic system II, and then destroy electron transport, thereby leading to weed death. However, most studies indicated that diuron was not easily volatile and photolytic in the soil, which could cause phytotoxicity and damage to subsequent sensitive crops, and induce environmental contamination of groundwater (Tandon and Pant, 2019). In addition, the ecological toxicity of diuron on non-target organisms was also reported, thereby raising community worry about its adverse impact on human health (Kao et al., 2019). Previous studies indicated that the diuron could cause endocrine and respiratory system disorders through its carcinogenic, mutagenic, and neurotoxic properties (Rocha et al., 2013; Huovinen et al., 2015; Behrens et al., 2016). Therefore, it is an urgent development for researchers to find a safe and effective method to manage the environmental pollution problem of diuron.
Currently, diuron could be degraded by means of physical adsorption, photocatalysis, chemistry, and microorganisms in the environment (Park and Jhung, 2020). Among them, microbial degradation was a safe and effective method to control organic contaminants from the natural environment (Bhatt et al., 2021). At present, only a few bacterial strains have been reported to degrade diuron, such as Neurospora intermedia (Wang et al., 2017), and Bacillus licheniformis (Singh and Singla, 2019). However, most of these bacterial isolates were unable to achieve complete mineralization of diuron and ended up producing only 1-(3,4-dichlorophenyl)-3-methyl urea (DCPMU), 1-(3,4-dichlorophenyl) urea (DCPU), or 3,4-dichloroaniline (3,4-DCA). In addition, previous research mainly focused on improving biodegradation efficiency, biodegradation characteristics, and identification of biodegradation products. There were relatively few reports on the resistance and transformation mechanism of microorganisms to diuron (Bhatt et al., 2021). Importantly, multi-omics technology provides new research ideas for elucidating the degradation mechanism of organic contaminants (Manzoni et al., 2018; Chunyan et al., 2023). Transcriptomics was used to explore key genes and degradation principles of microorganisms degrading pollutants (Filiatrault, 2011). Furthermore, metabolomics provided key information for us to further understand related metabolic pathways and their changes in the biodegradation process of aromatic compounds (Gao et al., 2021). Therefore, analyzing transcriptome and metabolome data could help us systematically understand the adaptation and biodegradation mechanisms of bacteria to diuron.
In this study, Achromobacter xylosoxidans SL-6 obtained in the early stage was used as the experimental object to investigate the biodegradation mechanism of diuron. Therefore, we combined toxicological evaluation, biodegradation product identification, metabolome analysis, and transcriptome analysis to reveal: (1) the adaptation mechanism of strain SL-6 to the oxidative stress caused by diuron; (2) the transport mechanism of strain SL-6 to diuron in cells; (3) the degradation mechanism of strain SL-6 to diuron in cells.
Diuron (analytically pure ≥97.0%) was provided by Shanghai McLean Biochemical Technology Co., Ltd. HPLC grade n-hexane and methanol were obtained from Thermo Fisher Scientific, United States. Mineral salt medium (0.2 g MgSO4·7H2O, 1 g (NH4)2 SO4, 1.5 g K2HPO4, 0.5 g NaCl, and 0.5 g KH2PO4 per litre of water; Sigma-Aldrich; purity ≥99%). Luria-Bertani (LB) medium (10.0 g tryptone, 5.0 g yeast extract, and 10.0 g NaCl per litre of water; Sigma-Aldrich; purity ≥99%). All chemicals were analytical reagent grade.
Previously, our research team has successfully discovered a strain that efficiently degrades diuron. The strain was isolated from the agricultural test site of Shihezi University, Shihezi City, Xinjiang Uygur Autonomous Region, China. The strain was identified and named Achromobacter xylosoxidans SL-6. Supplementary Figure S1 displays the colony morphology and evolutionary tree of the SL-6 strain. The results of the biological characteristics study of strain SL-6 are shown in Supplementary Figure S2. It was found that when the diuron dose reached 200 mg/L, the biodegradation efficiency of strain SL-6 was 93.1% within 5 days.
Strain SL-6 was inoculated into MSM medium containing several doses of diuron (0, 50, 100, 200, 400, 600 mg/L) and cultured for 24 h and 48 h, respectively. After washing three times with PBS, the cells were performed at 4°C and 8,000 rpm to obtain bacterial cells. The reactive oxygen species (ROS) level, malondialdehyde (MDA) content, and lactate dehydrogenase (LDH) activity in strain SL-6 were measured, which was described in Supplementary Section SI-1.
Superoxide dismutase (SOD), catalase (CAT), and glutathione (GSH) were essential antioxidants for maintaining redox homeostasis in organisms (Staerck et al., 2017). The strain SL-6 was inoculated in several doses of diuron (0, 50, 100, 200, 400, 600 mg/L) and cultured for 24 h and 48 h, respectively. The cells were ultrasonically fragmented at 0°C for 15 min and centrifuged at 8,000 rpm to collect the supernatant. The activity of SOD, CAT, and the content of GSH in strain SL-6 cells was detected by the reagent kit (Jiancheng Bioengineering Institute, Nanjing, China).
The sample was extracted twice with n-hexane and the organic phase was combined. The organic solution was removed by the rotary evaporator and then redissolved with methanol. The degradation products of diuron were detected by GC-MS (Agilent 8,890-5977B, Agilent Technologies, CA, United States). The column temperature program, scan range, and the parameters of the mobile phase are shown in Supplementary Section SI-2.
To detect alterations in intracellular metabolites, we performed a nontargeted metabolomic analysis (Gika et al., 2019). Strain SL-6 cells grown in LB medium supplemented with 200 mg/L diuron were harvested. The appropriate amount of sample was added to 1,000 μL pre-cooled methanol/acetonitrile/water solution (2:2:1, v/v/v) and treated with a vortex oscillator for 30 s. After ultrasonication at 4°C for 25 min, it was placed at −20°C for 15 min. The samples were placed in a centrifuge at 4°C, 14,000 rpm for 15 min. The supernatant was dried in a vacuum concentrator. Then it was redissolved with 100 μL acetonitrile/water (1:1, v/v) solvent and vortexed for 30 s, and then placed in a 4°C, 14000 rpm centrifuge for 15 min. The extracted samples were detected by UPLC-Q-TOF/MS. The specific parameters of LC-MS/MS analysis were described in Supplementary Section SI-3.
The strain SL-6 was inoculated into MSM liquid medium containing diuron (200 mg/L) and without diuron by 10% inoculation volume, and three replicates were set up, respectively, (An et al., 2020). The cells were cultured in a shaker at 30°C and 180 rpm for 72 h. Centrifugation was performed at 4°C and 10,000 rpm for 10 min to collect bacterial sediment. Washed twice with PBS, frozen in liquid nitrogen for 5 min, and stored at −80°C. The transcriptome sequencing service of the test samples was carried out by Wuhan Kangce Technology Co., Ltd. (Wuhan, China). The specific process of transcriptome sequencing was described in Supplementary Section SI-4.
Statistical analysis was performed using IBM SPSS statistics software (version 26.0, IBM Corporation, New York, United States). The significance of the difference between the treatment group and the control group was compared by single factor analysis of variance and Duncan test. All data were expressed as the means ± standard deviation (SD) at the p < 0.05 level. Data visualization was performed using Origin 2023 software (OriginLab, Massachussets, United States).
It was reported diuron could induce lipid peroxidation of the cell membrane by generating ROS, thereby leading to oxidative stress injury (Wang et al., 2022). ROS, MDA, and LDH were common indicators of cellular oxidative damage (Wakeel et al., 2020). Therefore, the intracellular ROS, MDA content, and LDH activity of strain SL-6 during treatment with diuron at different initial concentrations were determined. The results indicated that the ROS level, MDA content, and LDH activity of strain SL-6 cells treated with diuron was enhanced in a concentration-dependent manner (Figure 1). In addition, after 600 mg/L diuron treatment for 48 h, the ROS level, MDA content, and LDH activity of cells were increased by 4.29, 3.89, and 1.80 times than that of the control group, respectively. This indicated that diuron could induce the production of ROS in strain SL-6 cells, leading to enhanced lipid peroxidation and ultimately causing cell damage of strain SL-6. Previous studies indicated that SOD, CAT, and GSH were the primary antioxidant active substances in cells and played an important role in scavenging excessive ROS to maintain cellular redox balance (Hernández et al., 2015). Therefore, the SOD, CAT activity, and GSH content in the cells exposed to diuron were measured (Supplementary Figure S3). The results showed that after treatment with 200 mg/L diuron, the SOD, CAT activity and GSH content of strain SL-6 cells increased by 80, 159, and 81%, respectively, compared with the control group. This indicated that the addition of low concentration of diuron (≤200 mg/L) could stimulate the increase of SOD, POD activity, and GSH content in strain SL-6 cells, and reduce the oxidative damage of cells by removing excessive ROS in strain SL-6 cells.
Figure 1. Effects of different concentrations of diuron on (A) ROS level (48 h), (B) MDA content, and (C) LDH activity of Achromobacter xylosoxidans SL-6 after treatment for 24 h and 48 h, respectively. Error bars represent standard deviation (p < 0.05).
Combined with previous research results, we found that strain SL-6 had the highest biodegradation efficiency to 200 mg/L diuron (Supplementary Figure S2D), and the addition of 200 mg/L diuron had a relatively small effect on the oxidative damage of strain SL-6 cells. Therefore, 200 mg/L was selected as the optimal application dose of diuron in subsequent experiments. At present, the study found that the use of reference mass spectral database is still one of the best approaches to annotate the structure of known metabolites (Vinaixa et al., 2016). A total of seven metabolites were identified according to the mass spectra information by GC/MS detection (Supplementary Figure S4), including demethylation products: DCPMU and DCPU (C8H8Cl2N2O and C7H5Cl2NO), amide bond hydrolysis product: 3,4-DCA (C6H5Cl2N), dechlorination products: 4-CA and aniline (C6H6ClN and C6H7N), and hydroxylation products: catechol and cis, cis-muconic acid (C6H6O2 and C6H6O4). The results confirmed that a series of continuous transformations of diuron occurred in the cells of strain SL-6, including demethylation, urea bridge cleavage, dehalogenation, deamination, hydroxylation, and ring-opening (Figure 2).
Metabolomics method was used to analyze the changes of intracellular metabolites of the strain SL-6 after diuron treatment. The results showed that 1,338 metabolites were detected and analyzed. Principal component analysis (PCA) revealed a clear distinction between the diuron treatment group and the control group on PC1, which represented 79.3% of the total variance (Supplementary Figure S5A). This indicated that the changes in endogenous metabolites of the degrading bacteria SL-6 cells were caused by diuron. Further analysis of metabolites was performed to clarify the differences in metabolites among the two groups. Among them, 388 were significantly up-regulated and 172 were significantly down-regulated (Supplementary Figure S5B). In addition, VIP score plots of relevant metabolites were obtained by PLS-DA (Supplementary Figure S5C). It is worth noting that the addition of 200 mg/L diuron had effects on a variety of metabolites related to diuron degradation or antioxidants by strain SL-6, such as DCPMU, DCPU, malic acid, arginine, eta-carotene, phosphatidylethanolamine, citric acid, and glutathione. Furthermore, KEGG pathways indicated that the metabolic pathways mainly affected by diuron treatment were the biosynthesis of amino acids, 2-oxocarboxylic acid metabolism, alanine, aspartate and glutamate metabolism, and arginine biosynthesis (Supplementary Figure S5D). The above results showed that strain SL-6 could efficiently degrade diuron by the diuron biodegradation pathway, the TCA cycle, glutathione metabolism, and the urea cycle (Figure 3).
Figure 3. Schematic diagram of the metabolic pathways of strain SL-6 cells exposed to 200 mg/L diuron. (A) Diuron degradation pathway. (B) TCA cycle. (C) Glutathione metabolism. (D) Urea cycle. Red represents upregulation. Gln, glutamine; Glu, glutamic acid; Orn, ornithine; Cit, citrulline; Arg-Suc, L-argininosuccinic acid; γ-Glu-Cys, γ-L-glutamyl-L-cysteine; DCPMU, 1-(3,4-dichlorophenyl)-3-methyl urea; DCPU, 1-(3,4-dichlorophenyl) urea; MA, cis, cis-muconic acid; CA, citric acid; ICA, isocitrate; PYR, pyruvate; 2-OG, 2-Oxopentanoic acid; Suc, succinic acid; Fum, fumarate; Mal, malic acid.
There were three degradation products involved in the biodegradation pathway of diuron, namely DCPMU, DCPU, and cis, cis-muconic acid (MA) (Figure 3A). The metabolites involved in the TCA cycle were divided into two categories: organic acids and amino acids (Figure 3B). Among them, the contents of succinyl coenzyme A, malic acid, and citric acid increased significantly, and their relative abundance increased by 32.15, 3.60, and 17.78%, respectively, compared with the control group (Supplementary Figure S6). The contents of arginine, glutamic acid, methionine, isoleucine, valine, proline, and threonine were up-regulated (Supplementary Figure S6). This suggested that the TCA cycle could provide energy and various nutrients required for the strain SL-6 to adapt and degrade diuron, and was the center of the degradation metabolic reaction. In addition, the levels of glutamic acid, oxoproline, glutathione, and oxidized glutathione (GSSH) involved in glutathione metabolism were increased, and their relative abundances increased by 12.45, 13.39, 20.43, and 29.72%, respectively, compared with the control group (Figure 3C; Supplementary Figure S6). At the same time, the content of arginine and arginine succinic acid involved in the urea cycle were significantly up-regulated, and their relative abundances increased by 24.59 and 36.53%, respectively, compared with the control group (Figure 3D; Supplementary Figure S6). This indicated that strain SL-6 could reduce the oxidative stress induced by diuron through glutathione metabolism and urea cycle pathway, enhance the detoxification ability of diuron cytotoxicity, and better serve the degradation of diuron.
Illumina MiSeq sequencing technology was used to research the differentially expressed genes during the degradation of diuron by strain SL-6, and the biodegradation mechanism of diuron was discussed. The PCA analysis showed that the diuron treatment group (200 mg/L) was significantly separated from the control group along PC1, which explained 42.84% of the total variance (Figure 4A). The results of the volcano plot showed that compared with the control group, the total number of differentially expressed genes (DEGs) after diuron induction was 1,057, of which 445 were up-regulated and 612 were down-regulated (Figure 4B). The 1,057 DEGs were annotated by the GO database and divided into three categories: molecular function, cellular component, and biological process (Figure 4C). In the molecular function classification, some up-regulated genes were annotated as oxidoreductase, dehydrogenase, cytochrome P450 and hydrolase activities (Ma et al., 2023). These DEGs involving the above GO entries might participate in the degradation of diuron by strain SL-6. The most important GO term in the classification of cellular components was ATPase-dependent transmembrane transport complex, followed by transmembrane transport protein complex, plasma membrane protein complex, and transport complex. These DEGs involved in the transmembrane transport of substances might help strain SL-6 transport diuron into cells. In the classification of biological processes, the up-regulated genes were mainly concentrated in carbon metabolism, nitrogen metabolism, fatty acid metabolism, and TCA cycle, while the down-regulated genes focused on cellular carbohydrate metabolism, glucan metabolism, polysaccharide metabolism, and glycogen metabolism (Qi et al., 2023). The above genes might participate in the energy metabolism process in strain SL-6 cells, providing energy to degrade and adapt to diuron. Similar results were observed for KEGG annotated pathway distribution. In KEGG pathway analysis, DEGs were mainly concentrated in multiple metabolic items, such as oxidative phosphorylation, glyoxylic acid and dicarboxylic acid metabolism, nitrogen metabolism, transmembrane transport, the TCA cycle, and glycolysis (Figure 5A).
Figure 4. (A) PCA analysis plot. (B) Volcano plot of all differentially expressed genes. (C) Significantly enriched GO terms in differentially expressed genes.
Figure 5. (A) KEGG secondary classification of differentially expressed genes. (B) Cluster heat map of differentially expressed genes related to transmembrane transport of substances, antioxidant reactions, and the action of diuron-degrading enzymes.
The degradation of diuron in strain SL-6 cells involved a variety of biological processes, including material transmembrane transport, antioxidant response, and the role of diuron degradation enzymes. Hierarchical clustering analysis was performed on DEGs related to the above biological processes (Figure 5B).
Cell transmembrane transport system: genes encoding transmembrane proteins, ATP-binding cassette (ABC), and major facilitator superfamily (MFS) transporters. According to the transcriptome results, we found that the expression of the genes (I6I48_RS07135 and livG) encoding Lipopolysaccharide export system ATP-binding protein LptB synthesis were significantly increased by 3.80-fold and 3.34-fold than that of the control group, respectively (Supplementary Table S1). The genes encoding outer membrane protein (I6I48_RS11310, adeC, and I6I48_RS00580) were up-regulated by 3.01-fold, 2.99-fold, and 3.19-fold than that of the control group, respectively (Supplementary Table S1). The expressions of nine ABC transporters (I6I48_RS03180, I6I48_RS10650, I6I48_RS28500, I6I48_RS29915, I6I48_RS19805, I6I48_RS18970, I6I48_RS23815 dctP, and pstS) and one MFS transporters (I6I48_RS22785) were significantly up-regulated (Supplementary Table S1). In summary, we speculate that the strain SL-6 cells had a transmembrane transport system capable of transporting exogenous organic substances, which could transport diuron into the cells to complete the next step of degradation.
Antioxidant system: genes encoding catalase, peroxidase, glutathione metabolism, and redox homeostasis. According to the transcriptome of diuron-treated cells, the genes encoding peroxidase (I6I48_RS30485, I6I48_RS29650, and I6I48_RS11345) and catalase (I6I48_RS02815) were significantly up-regulated (Supplementary Table S2). The genes encoding glutathione S-transferase (I6I48_RS26100) and glutaredoxin (I6I48_RS17070) were up-regulated by 2.34-fold and 2.47-fold than that of the control group, respectively (Supplementary Table S2). Three cellular redox homeostasis-related genes (I6I48_RS18035, I6I48_RS30485, and I6I48_RS05390) were up-regulated by 2.05-fold, 2.48-fold, and 2.57-fold than that of the control group, respectively (Supplementary Table S2). Taken together, we speculate that there was an antioxidant system in the cells of strain SL-6 that could resist exogenous toxic substances and help the strain cells maintain normal survival, so as to ensure the degradation of diuron.
Diuron degradation system: genes encoding demethylase, amidase, amidohydrolase, dehalogenase, monooxygenase and dioxygenase. The results of transcriptome showed that the expression level of the cytochrome P450 (adhP) gene encoding xenobiotic metabolism increased by 2.73-fold than that of the control group (Supplementary Table S3), which might be involved in the demethylation reaction of strain SL-6 to diuron. The gene expressions of N-formylglutamate amidohydrolase (I6I48_RS16595), amidohydrolase family (I6I48_RS20465), amidohydrolase family protein (I6I48_RS04995), and N-acetylmuramyl-L-alanine amidase (I6I48_RS09150) were significantly up-regulated (Supplementary Table S3), indicating that they could be involved in the hydrolysis of amide bonds and act as hydrolases to catalyze the cleavage of urea bridges in this process. The gene expression of 4-chlorobenzoyl-CoA dehalogenase (I6I48_RS07180) was up-regulated by 2.20-fold than that of the control group (Supplementary Table S3), which might be related to the reductive dechlorination reaction during the biodegradation of diuron. The gene expression of nitrate monooxygenase (I6I48_RS21595) was up-regulated by 2.35-fold than that of the control group (Supplementary Table S3), which could be relevant to the deamination oxidation reaction of aniline during the biodegradation of diuron. The expression of genes encoding multiple dioxygenases was significantly up-regulated, including quercetin 2,3-dioxygenase (I6I48_RS17155), aromatic ring hydroxylating dioxygenase (I6I48_RS26030), homogentisate 1,2-dioxygenase (hmgA) and phytanoyl-CoA dioxygenase family protein (I6I48_RS04300) (Supplementary Table S3), indicating that they might be responsible for ring-opening cleavage. Therefore, we speculate that there was a diuron degradation system composed of genes encoding multiple degradation enzymes in the strain SL-6 cells, which helped the strain SL-6 cells degrade and utilize diuron.
The analysis of diuron degradation products provided information on the degradation pathway for the biodegradation mechanism of diuron by bacterial SL-6 cells. Previous studies indicated that microorganisms first generate DCPMU and DCPU by removing one or two methyl groups on diuron (Ellegaard-Jensen et al., 2013). This was followed by the hydrolysis of the amide bond, generating the metabolite 3,4-DCA, which was the common microbial diuron degradation product (Egea et al., 2017). According to previous reports, the biodegradation of 3,4-DCA mainly included two diverse reactions (dehalogenation and hydroxylation), followed by the formation of 4-chloroaniline (4-CA) and 1,2-dichlorobenzene (1,2-DCB) (Silambarasan et al., 2020). 4-CA was dechlorinated and hydroxylated to generate aniline and 4-chlorocatechol respectively, and then, aniline was deaminated to generate catechol (Mohlam et al., 2018; Li et al., 2022). Ultimately, ring-opening cleavage of catechol produced cis, cis-muconic acid (Shahryari et al., 2018; Yu et al., 2020). According to the seven degradation products identified by GC-MS, the degradation pathway of diuron in the cells of strain SL-6 was clarified. Mainly demethylation, urea bridge cleavage, dehalogenation, deamination and ring opening, which provided a basis for subsequent research on the biodegradation mechanism of diuron by strain SL-6.
Metabolomics was a science that reflects the physiological state of organisms by detecting changes in endogenous metabolites (Liang et al., 2021). Through metabolomic research, it was found that strain SL-6 requires the joint participation of the TCA cycle, glutathione metabolism, and urea cycle to adapt to and degrade diuron. As the center of metabolic pathways, the TCA cycle provided energy for other metabolic pathways in organisms (Liu et al., 2017; Ke et al., 2018). The degradation products of diuron could be utilized by SL-6 cells through the TCA cycle. Importantly, the TCA cycle could also produce organic acids to biosynthesize other amino acids (Arnold and Finley, 2023). These newly generated amino acids could participate in the regulation of energy metabolism, signaling pathways, and antioxidant defense responses in cells (Nesterov et al., 2020). The up-regulated arginine, glutamic acid, methionine, isoleucine, valine, proline, and threonine could directly or indirectly be involved in glycolysis, fat metabolism, pyruvate metabolism, and the TCA cycle, providing various nutrients for strain SL-6. Furthermore, Previous studies reported that glutathione was a common antioxidant in organisms and played an important role in maintaining cellular redox homeostasis, regulating cellular signals, and improving protein activity (Hasanuzzaman et al., 2017). Therefore, we speculated that the glutathione metabolic pathway could continuously provide antioxidants for SL-6 strain cells. The purpose was to remove the excessive ROS induced by diuron and maintain the redox homeostasis of SL-6 cells. Previous studies reported that arginine serves as an intermediate in the urea cycle, which could relieve ammonia poisoning and involved in the energy metabolism and antioxidant stress response of various microorganisms (Charlier and Bervoets, 2019; Hirose et al., 2021). Therefore, we speculate that the ammonia (NH3) removed during the degradation of diuron might eventually be excreted by the strain SL-6 cells via the urea cycle to achieve detoxification. Metabolomic analysis helped us to further expand our understanding of the mechanisms of degradation and adaptation of strain SL-6 to diuron.
Transcriptomics studies had shown that in order to fully degrade diuron, strain SL-6 cells might have cell transmembrane transport system, antioxidant system, and degradation system composed of a variety of different coding genes. Previous research had confirmed that most of the transmembrane transport of exogenous substances was mediated by the membrane transport system (Wu et al., 2023). Lipopolysaccharide, outer membrane protein, ABC, and MFS transporters were components of the cell transmembrane transport system. Studies had shown that lipopolysaccharide could adjust the hydrophobicity of bacterial cell surfaces and play an important role in the adsorption of organic contaminants (Okuda et al., 2016). Outer membrane proteins (OMPs) were structural proteins on the outer membrane of bacterial cells, which could participate in the passive and active transport of substances (Sun et al., 2022). It has been reported that ABC transporters and MFS transporters play important roles in cell transmembrane nutrient absorption (Mutanda et al., 2022). In addition, ABC and MFS transporters participated in the excretion of various toxic compounds within microbial cells and provided microorganisms with resistance to various compounds (Wilkens, 2015; Pasqua et al., 2021). We considered that diuron could enter the strain SL-6 cells through the cell transmembrane transport system to achieve multi-step degradation of diuron.
At present, research has shown that there was an endogenous antioxidant system in organisms, which could counteract free radicals and neutralize oxidants to maintain cellular redox homeostasis (He et al., 2017). The common antioxidants include catalase, peroxidase, glutathione metabolism, and redox homeostasis genes. It was reported that catalase and peroxidase in cells participate in the scavenging of ROS and reduce cytotoxicity (Hasanuzzaman et al., 2017). Glutathione S-transferase and glutaredoxin mediate the reduction of glutathione and were involved in the detoxification of xenobiotics and oxidative stress response (Dasari et al., 2018; Chai and Mieyal, 2023). Furthermore, genes related to cellular redox homeostasis play an important role in maintaining the normal physiological activities of cells and responding to oxidative stress (He et al., 2017). We considered that the genes encoding catalase, peroxidase, glutathione metabolism, and redox homeostasis together constitute the antioxidant system, which could help the strain SL-6 better adapt to the toxicity of diuron and provide a healthy and stable cell environment for the degradation of diuron in the cell.
In addition, the biodegradation of diuron mainly involved demethylase, amidase, amidohydrolase, dehalogenase, monooxygenase, and dioxygenase, which played different roles in the biodegradation of diuron. Demethylation reaction: the DCPMU and DCPU generated by diuron degradation indicate that the enzyme catalyzes the sequential demethylation of diuron (Silva Moretto et al., 2019). Cytochrome P450 was proved to participate in the demethylation of diuron by Arthrobacter N2 (Giacomazzi and Cochet, 2004). It was also involved in the demethylation reaction of diuron in humans and other mammals (Abass et al., 2007; Uno et al., 2011). Amide bond hydrolysis reaction: the most important enzymatic reaction of diuron biodegradation was the hydrolysis and cleavage of the amide bond to produce the metabolite 3,4-DCA (Hussain et al., 2015; Silambarasan et al., 2020). It was found that amidohydrolases and amidases played a role in the hydrolysis of amide bonds during the biodegradation of phenylurea herbicides. Seven species were reported, including PuhA, PuhB, LibA, HylA, Phh, Mhh, and LahB (Dejonghe et al., 2003; Khurana et al., 2009; Bers et al., 2012; Zhang et al., 2018, 2020).
Dechlorination reaction: the literature states that the next step after the biodegradation of 3,4-DCA was the sequential dechlorination reaction, which resulted in the production of aniline (Hongsawat and Vangnai, 2011). Dehalogenases could catalyze the removal of halogen groups and then were used by many bacteria to degrade halogenated aromatic hydrocarbons (Ang et al., 2018; Pimviriyakul et al., 2020). Therefore, the gene encoding dehalogenase might be involved in the reductive dechlorination reaction during the biodegradation of diuron. Oxidative deamination reaction: it has been reported that aniline was converted into catechol by deamination and hydroxylation (Arora, 2015). Monooxygenases could catalyze the deamination and oxidation of aromatic nitrogen oxides to generate hydroxylated products (Paul et al., 2021). The gene encoding monooxygenase might be involved in the biodegradation of diuron by strain SL-6 and play a role in the deamination of aniline to catechol. Ring-opening reaction: catechol could be further degraded into cis, cis-muconic acid (MA) through the ortho-cleavage pathway (Liu et al., 2023). According to various studies, dioxygenase had the ability to catalyze the ring-opening cleavage reaction of catechol (Zhang et al., 2021). The generated cis, cis-muconic acid was ultimately metabolized through the TCA cycle (He et al., 2023). We considered that there was a diuron degradation system composed of genes encoding demethylase, amidase, amidohydrolase, dehalogenase, monooxygenase, and dioxygenase in strain SL-6 cells, which helped the strain SL-6 cells to degrade and utilize diuron step by step, and finally realize the complete mineralization of diuron.
In summary, the biodegradation and adaption mechanisms of Achromobacter xylosoxidans SL-6 to diuron were further revealed through toxicological assessment, identification of intermediate metabolites, metabolome, and transcriptome analysis. Toxicological evaluation results showed that diuron could activate the antioxidant system (superoxide dismutase, catalase, and glutathione) in the cells of strain SL-6, and relieve the oxidative damage induced by a large number of reactive oxygen species. The analysis of degradation metabolites indicated that the diuron underwent a sequence of reactions in strain SL-6 cells, urea bridge cleavage, dehalogenation, deamination oxidation, and ring-opening. Metabolomic analysis revealed that the degradation of diuron by strain SL-6 mainly involved four metabolic pathways: diuron biodegradation, tricarboxylic acid cycle, glutathione metabolism, and the urea cycle. Furthermore, transcriptome data analysis identified differentially expressed genes that participated in substance transmembrane transport, antioxidant response, and diuron degradation in strain SL-6 cells. Overall, our research results offered a new perspective on the biodegradation and adaptation mechanism of strain SL-6 to diuron and promoted the environmental remediation strategy to a deeper level.
The original contributions presented in the study are publicly available. This data can be found here: NCBI Sequence Read Archive (SRA) database, accession number: PRJNA1118467.
ZH: Data curation, Investigation, Software, Writing – original draft. CQ: Conceptualization, Writing – review & editing. HW: Conceptualization, Writing – review & editing. LS: Investigation, Writing – review & editing. CaW: Conceptualization, Funding acquisition, Methodology, Writing – review & editing. GZ: Resources, Supervision, Writing – review & editing. XH: Resources, Supervision, Writing – review & editing. ChW: Resources, Supervision, Writing – review & editing. TM: Resources, Supervision, Writing – review & editing. DY: Data curation, Project administration, Supervision, Writing – review & editing.
The author(s) declare that financial support was received for the research, authorship, and/or publication of this article. This work was financially supported by the National Natural Science Foundation of China (No. 3230688).
The authors declare that the research was conducted in the absence of any commercial or financial relationships that could be construed as a potential conflict of interest.
All claims expressed in this article are solely those of the authors and do not necessarily represent those of their affiliated organizations, or those of the publisher, the editors and the reviewers. Any product that may be evaluated in this article, or claim that may be made by its manufacturer, is not guaranteed or endorsed by the publisher.
The Supplementary material for this article can be found online at: https://www.frontiersin.org/articles/10.3389/fmicb.2024.1403279/full#supplementary-material
Abass, K., Reponen, P., Turpeinen, M., Jalonen, J., and Pelkonen, O. (2007). Characterization of diuron N-demethylation by mammalian hepatic microsomes and cDNA-expressed human cytochrome P450 enzymes. Drug Metab. Dispos. 35, 1634–1641. doi: 10.1124/dmd.107.016295
An, X., Tian, C., Xu, J., Dong, F., Liu, X., Wu, X., et al. (2020). Characterization of hexaconazole-degrading strain Sphingobacterium multivorum and analysis of transcriptome for biodegradation mechanism. Sci. Total Environ. 722:137171. doi: 10.1016/j.scitotenv.2020.137171
Ang, T.-F., Maiangwa, J., Salleh, A. B., Normi, Y. M., and Leow, T. C. (2018). Dehalogenases: from improved performance to potential microbial dehalogenation applications. Molecules 23:1100. doi: 10.3390/molecules23051100
Arnold, P. K., and Finley, L. W. S. (2023). Regulation and function of the mammalian tricarboxylic acid cycle. J. Biol. Chem. 299:102838. doi: 10.1016/j.jbc.2022.102838
Arora, P. K. (2015). Bacterial degradation of monocyclic aromatic amines. Front. Microbiol. 6:820. doi: 10.3389/fmicb.2015.00820
Behrens, D., Rouxel, J., Burgeot, T., and Akcha, F. (2016). Comparative embryotoxicity and genotoxicity of the herbicide diuron and its metabolites in early life stages of Crassostrea gigas: implication of reactive oxygen species production. Aquat. Toxicol. 175, 249–259. doi: 10.1016/j.aquatox.2016.04.003
Bers, K., Sniegowski, K., De Mot, R., and Springael, D. (2012). Dynamics of the linuron hydrolase libA gene pool size in response to linuron application and environmental perturbations in agricultural soil and on-farm biopurification systems. Appl. Environ. Microbiol. 78, 2783–2789. doi: 10.1128/AEM.06991-11
Bhatt, P., Gangola, S., Bhandari, G., Zhang, W., Maithani, D., Mishra, S., et al. (2021). New insights into the degradation of synthetic pollutants in contaminated environments. Chemosphere 268:128827. doi: 10.1016/j.chemosphere.2020.128827
Chai, Y.-C., and Mieyal, J. J. (2023). Glutathione and glutaredoxin—key players in cellular redox homeostasis and signaling. Antioxidants 12:1553. doi: 10.3390/antiox12081553
Charlier, D., and Bervoets, I. (2019). Regulation of arginine biosynthesis, catabolism and transport in Escherichia coli. Amino Acids 51, 1103–1127. doi: 10.1007/s00726-019-02757-8
Chunyan, X., Qaria, M. A., Qi, X., and Daochen, Z. (2023). The role of microorganisms in petroleum degradation: current development and prospects. Sci. Total Environ. 865:161112. doi: 10.1016/j.scitotenv.2022.161112
Dasari, S., Ganjayi, M. S., Yellanurkonda, P., Basha, S., and Meriga, B. (2018). Role of glutathione S-transferases in detoxification of a polycyclic aromatic hydrocarbon, methylcholanthrene. Chem. Biol. Interact. 294, 81–90. doi: 10.1016/j.cbi.2018.08.023
Dejonghe, W., Berteloot, E., Goris, J., Boon, N., Crul, K., Maertens, S., et al. (2003). Synergistic degradation of linuron by a bacterial consortium and isolation of a single linuron-degrading Variovorax strain. Appl. Environ. Microbiol. 69, 1532–1541. doi: 10.1128/AEM.69.3.1532-1541.2003
Egea, T. C., da Silva, R., Boscolo, M., Rigonato, J., Monteiro, D. A., Grünig, D., et al. (2017). Diuron degradation by bacteria from soil of sugarcane crops. Heliyon 3:e00471. doi: 10.1016/j.heliyon.2017.e00471
Ellegaard-Jensen, L., Aamand, J., Kragelund, B. B., Johnsen, A. H., and Rosendahl, S. (2013). Strains of the soil fungus Mortierella show different degradation potentials for the phenylurea herbicide diuron. Biodegradation 24, 765–774. doi: 10.1007/s10532-013-9624-7
Filiatrault, M. J. (2011). Progress in prokaryotic transcriptomics. Curr. Opin. Microbiol. 14, 579–586. doi: 10.1016/j.mib.2011.07.023
Gao, J., Song, J., Ye, J., Duan, X., Dionysiou, D. D., Yadav, J. S., et al. (2021). Comparative toxicity reduction potential of UV/sodium percarbonate and UV/hydrogen peroxide treatments for bisphenol a in water: an integrated analysis using chemical, computational, biological, and metabolomic approaches. Water Res. 190:116755. doi: 10.1016/j.watres.2020.116755
Giacomazzi, S., and Cochet, N. (2004). Environmental impact of diuron transformation: a review. Chemosphere 56, 1021–1032. doi: 10.1016/j.chemosphere.2004.04.061
Gika, H., Virgiliou, C., Theodoridis, G., Plumb, R. S., and Wilson, I. D. (2019). Untargeted LC/MS-based metabolic phenotyping (metabonomics/metabolomics): the state of the art. J. Chromatogr. B 1117, 136–147. doi: 10.1016/j.jchromb.2019.04.009
Hasanuzzaman, M., Nahar, K., Anee, T. I., and Fujita, M. (2017). Glutathione in plants: biosynthesis and physiological role in environmental stress tolerance. Physiol. Mol. Biol. Plants 23, 249–268. doi: 10.1007/s12298-017-0422-2
He, L., He, T., Farrar, S., Ji, L., Liu, T., and Ma, X. (2017). Antioxidants maintain cellular redox homeostasis by elimination of reactive oxygen species. Cell. Physiol. Biochem. 44, 532–553. doi: 10.1159/000485089
He, S., Wang, W., Wang, W., Hu, H., Xu, P., and Tang, H. (2023). Microbial production of cis,cis-muconic acid from aromatic compounds in engineered Pseudomonas. Synth. Syst. Biotechnol. 8, 536–545. doi: 10.1016/j.synbio.2023.08.001
Hernández, L. E., Sobrino-Plata, J., Montero-Palmero, M. B., Carrasco-Gil, S., Flores-Cáceres, M. L., Ortega-Villasante, C., et al. (2015). Contribution of glutathione to the control of cellular redox homeostasis under toxic metal and metalloid stress. J. Exp. Bot. 66, 2901–2911. doi: 10.1093/jxb/erv063
Hirose, Y., Yamaguchi, M., Sumitomo, T., Nakata, M., Hanada, T., Okuzaki, D., et al. (2021). Streptococcus pyogenes upregulates arginine catabolism to exert its pathogenesis on the skin surface. Cell Rep. 34:108924. doi: 10.1016/j.celrep.2021.108924
Hongsawat, P., and Vangnai, A. S. (2011). Biodegradation pathways of chloroanilines by Acinetobacter baylyi strain GFJ2. J. Hazard. Mater. 186, 1300–1307. doi: 10.1016/j.jhazmat.2010.12.002
Huovinen, M., Loikkanen, J., Naarala, J., and Vähäkangas, K. (2015). Toxicity of diuron in human cancer cells. Toxicol. In Vitro 29, 1577–1586. doi: 10.1016/j.tiv.2015.06.013
Hussain, S., Arshad, M., Springael, D., SøRensen, S. R., Bending, G. D., Devers-Lamrani, M., et al. (2015). Abiotic and biotic processes governing the fate of phenylurea herbicides in soils: a review. Crit. Rev. Environ. Sci. Technol. 45, 1947–1998. doi: 10.1080/10643389.2014.1001141
Kao, C. M., Ou, W.-J., Lin, H.-D., Eva, A. W., Wang, T.-L., and Chen, S. C. (2019). Toxicity of diuron in HepG2 cells and zebrafish embryos. Ecotoxicol. Environ. Saf. 172, 432–438. doi: 10.1016/j.ecoenv.2019.01.036
Ke, M., Qu, Q., Peijnenburg, W. J. G. M., Li, X., Zhang, M., Zhang, Z., et al. (2018). Phytotoxic effects of silver nanoparticles and silver ions to Arabidopsis thaliana as revealed by analysis of molecular responses and of metabolic pathways. Sci. Total Environ. 644, 1070–1079. doi: 10.1016/j.scitotenv.2018.07.061
Khurana, J. L., Jackson, C. J., Scott, C., Pandey, G., Horne, I., Russell, R. J., et al. (2009). Characterization of the phenylurea hydrolases a and B: founding members of a novel amidohydrolase subgroup. Biochem. J. 418, 431–441. doi: 10.1042/bj20081488
Li, C., Sun, Y., Sun, G., Zang, H., Sun, S., Zhao, X., et al. (2022). An amidase and a novel phenol hydroxylase catalyze the degradation of the antibacterial agent triclocarban by Rhodococcus rhodochrous. J. Hazard. Mater. 430:128444. doi: 10.1016/j.jhazmat.2022.128444
Li, J., Zhang, W., Lin, Z., Huang, Y., Bhatt, P., and Chen, S. (2021). Emerging strategies for the bioremediation of the phenylurea herbicide diuron. Front. Microbiol. 12:686509. doi: 10.3389/fmicb.2021.686509
Liang, Y., Zhang, H., and Cai, Z. (2021). New insights into the cellular mechanism of triclosan-induced dermal toxicity from a combined metabolomic and lipidomic approach. Sci. Total Environ. 757:143976. doi: 10.1016/j.scitotenv.2020.143976
Liu, S., Guo, C., Dang, Z., and Liang, X. (2017). Comparative proteomics reveal the mechanism of Tween80 enhanced phenanthrene biodegradation by Sphingomonas sp. GY2B. Ecotoxicol. Environ. Saf. 137, 256–264. doi: 10.1016/j.ecoenv.2016.12.015
Liu, Y., Wei, F., Xu, R., Cheng, T., and Ma, Y. (2023). Insights into the binding interaction of catechol 1,2-dioxygenase with catechol in Achromobacter xylosoxidans DN002. Appl. Biochem. Biotechnol. 195, 298–313. doi: 10.1007/s12010-022-04129-7
Ma, Q., Han, X., Song, J., Wang, J., Li, Q., Parales, R. E., et al. (2023). Characterization of a new chlorimuron-ethyl-degrading strain Cedecea sp. LAM2020 and biodegradation pathway revealed by multiomics analysis. J. Hazard. Mater. 443:130197. doi: 10.1016/j.jhazmat.2022.130197
Manzoni, C., Kia, D. A., Vandrovcova, J., Hardy, J., Wood, N. W., Lewis, P. A., et al. (2018). Genome, transcriptome and proteome: the rise of omics data and their integration in biomedical sciences. Brief. Bioinform. 19, 286–302. doi: 10.1093/bib/bbw114
Mohlam, F., Bakeer, W., El-Gebaly, E., and Amin, M. (2018). Molecular characterization of aniline biodegradation by some bacterial isolates having unexpressed catechol 2,3-dioxygenase gene. J. Pure Appl. Microbiol. 12, 2027–2039. doi: 10.22207/JPAM.12.4.39
Mutanda, I., Sun, J., Jiang, J., and Zhu, D. (2022). Bacterial membrane transporter systems for aromatic compounds: regulation, engineering, and biotechnological applications. Biotechnol. Adv. 59:107952. doi: 10.1016/j.biotechadv.2022.107952
Nesterov, S. V., Yaguzhinsky, L. S., Podoprigora, G. I., and Nartsissov, Y. R. (2020). Amino acids as regulators of cell metabolism. Biochemistry 85, 393–408. doi: 10.1134/S000629792004001X
Okuda, S., Sherman, D. J., Silhavy, T. J., Ruiz, N., and Kahne, D. (2016). Lipopolysaccharide transport and assembly at the outer membrane: the PEZ model. Nat. Rev. Microbiol. 14, 337–345. doi: 10.1038/nrmicro.2016.25
Park, J. M., and Jhung, S. H. (2020). Polyaniline-derived carbons: remarkable adsorbents to remove atrazine and diuron herbicides from water. J. Hazard. Mater. 396:122624. doi: 10.1016/j.jhazmat.2020.122624
Pasqua, M., Bonaccorsi di Patti, M. C., Fanelli, G., Utsumi, R., Eguchi, Y., Trirocco, R., et al. (2021). Host-bacterial pathogen communication: the wily role of the multidrug efflux pumps of the MFS family. Front. Mol. Biosci. 8:723274. doi: 10.3389/fmolb.2021.723274
Paul, C. E., Eggerichs, D., Westphal, A. H., Tischler, D., and van Berkel, W. J. H. (2021). Flavoprotein monooxygenases: versatile biocatalysts. Biotechnol. Adv. 51:107712. doi: 10.1016/j.biotechadv.2021.107712
Pimviriyakul, P., Wongnate, T., Tinikul, R., and Chaiyen, P. (2020). Microbial degradation of halogenated aromatics: molecular mechanisms and enzymatic reactions. Microb. Biotechnol. 13, 67–86. doi: 10.1111/1751-7915.13488
Qi, X., Zhu, M., Yuan, Y., Rong, X., Dang, Z., and Yin, H. (2023). Integrated toxicology, metabolomics, and transcriptomics analyses reveal the biodegradation and adaptation mechanisms to BDE-47 in Pseudomonas plecoglossicida. Chem. Eng. J. 454:140412. doi: 10.1016/j.cej.2022.140412
Rocha, M. S. D., Arnold, L. L., Dodmane, P. R., Pennington, K. L., Qiu, F., Camargo, J. L. V. D., et al. (2013). Diuron metabolites and urothelial cytotoxicity: in vivo, in vitro and molecular approaches. Toxicology 314, 238–246. doi: 10.1016/j.tox.2013.10.005
Shahryari, S., Zahiri, H. S., Haghbeen, K., Adrian, L., and Noghabi, K. A. (2018). High phenol degradation capacity of a newly characterized Acinetobacter sp. SA01: bacterial cell viability and membrane impairment in respect to the phenol toxicity. Ecotoxicol. Environ. Saf. 164, 455–466. doi: 10.1016/j.ecoenv.2018.08.051
Silambarasan, S., Logeswari, P., Ruiz, A., Cornejo, P., and Kannan, V. R. (2020). Influence of plant beneficial Stenotrophomonas rhizophila strain CASB3 on the degradation of diuron-contaminated saline soil and improvement of Lactuca sativa growth. Environ. Sci. Pollut. Res. 27, 35195–35207. doi: 10.1007/s11356-020-09722-z
Silva Moretto, J. A., Rueda Furlan, J. P., Tonelli Fernandes, A. F., Bauermeister, A., Lopes, N. P., and Stehling, E. G. (2019). Alternative biodegradation pathway of the herbicide diuron. Int. Biodeterior. Biodegradation 143:104716. doi: 10.1016/j.ibiod.2019.06.004
Singh, A. K., and Singla, P. (2019). Biodegradation of diuron by endophytic Bacillus licheniformis strain SDS12 and its application in reducing diuron toxicity for green algae. Environ. Sci. Pollut. Res. 26, 26972–26981. doi: 10.1007/s11356-019-05922-4
Staerck, C., Gastebois, A., Vandeputte, P., Calenda, A., Larcher, G., Gillmann, L., et al. (2017). Microbial antioxidant defense enzymes. Microb. Pathog. 110, 56–65. doi: 10.1016/j.micpath.2017.06.015
Sun, J., Rutherford, S. T., Silhavy, T. J., and Huang, K. C. (2022). Physical properties of the bacterial outer membrane. Nat. Rev. Microbiol. 20, 236–248. doi: 10.1038/s41579-021-00638-0
Tandon, S., and Pant, R. (2019). Kinetics of diuron under aerobic condition and residue analysis in sugarcane under subtropical field conditions. Environ. Technol. 40, 86–93. doi: 10.1080/09593330.2017.1380709
Uno, T., Kaji, S., Goto, T., Imaishi, H., Nakamura, M., Kanamaru, K., et al. (2011). Metabolism of the herbicides chlorotoluron, diuron, linuron, simazine, and atrazine by CYP1A9 and CYP1C1 from Japanese eel (Anguilla japonica). Pestic. Biochem. Physiol. 101, 93–102. doi: 10.1016/j.pestbp.2011.08.005
Vinaixa, M., Schymanski, E. L., Neumann, S., Navarro, M., Salek, R. M., and Yanes, O. (2016). Mass spectral databases for LC/MS- and GC/MS-based metabolomics: state of the field and future prospects. Trends Analyt Chem 78, 23–35. doi: 10.1016/j.trac.2015.09.005
Wakeel, A., Xu, M., and Gan, Y. (2020). Chromium-induced reactive oxygen species accumulation by altering the enzymatic antioxidant system and associated cytotoxic, genotoxic, ultrastructural, and photosynthetic changes in plants. Int. J. Mol. Sci. 21:728. doi: 10.3390/ijms21030728
Wang, Y., Li, H., Feng, G., Du, L., and Zeng, D. (2017). Biodegradation of diuron by an endophytic fungus Neurospora intermedia DP8-1 isolated from sugarcane and its potential for remediating diuron-contaminated soils. PLoS One 12:e0182556. doi: 10.1371/journal.pone.0182556
Wang, X. D., Zhang, C. Y., Yuan, Y., Hua, Y. F., Asami, T., Qin, Y., et al. (2022). Molecular responses and degradation mechanisms of the herbicide diuron in rice crops. J. Agric. Food Chem. 70, 14352–14366. doi: 10.1021/acs.jafc.2c05142
Wilkens, S. (2015). Structure and mechanism of ABC transporters. F1000Prime Rep. 7:14. doi: 10.12703/P7-14
Wu, J., Zhao, R., Zhao, L., Xu, Q., Lv, J., and Ma, F. (2023). Sorption of petroleum hydrocarbons before transmembrane transport and the structure, mechanisms and functional regulation of microbial membrane transport systems. J. Hazard. Mater. 441:129963. doi: 10.1016/j.jhazmat.2022.129963
Yu, H., Wang, L., Lin, Y., Liu, W., Tuyiringire, D., Jiao, Y., et al. (2020). Complete metabolic study by dibutyl phthalate degrading Pseudomonas sp. DNB-S1. Ecotoxicol. Environ. Saf. 194:110378. doi: 10.1016/j.ecoenv.2020.110378
Zhang, C., Chen, H., Xue, G., Liu, Y., Chen, S., and Jia, C. (2021). A critical review of the aniline transformation fate in azo dye wastewater treatment. J. Clean. Prod. 321:128971. doi: 10.1016/j.jclepro.2021.128971
Zhang, L., Hang, P., Hu, Q., Chen, X.-L., Zhou, X.-Y., Chen, K., et al. (2018). Degradation of phenylurea herbicides by a novel bacterial consortium containing synergistically catabolic species and functionally complementary hydrolases. J. Agric. Food Chem. 66, 12479–12489. doi: 10.1021/acs.jafc.8b03703
Keywords: Achromobacter xylosoxidans, degradation, bioremediation, diuron, multiomics
Citation: Hu Z, Qian C, Wang H, Sun L, Wu C, Zhang G, Han X, Wang C, Ma T and Yang D (2024) Comprehensive toxicological, metabolomic, and transcriptomic analysis of the biodegradation and adaptation mechanism by Achromobacter xylosoxidans SL-6 to diuron. Front. Microbiol. 15:1403279. doi: 10.3389/fmicb.2024.1403279
Received: 19 March 2024; Accepted: 21 May 2024;
Published: 07 June 2024.
Edited by:
Gaurav Pant, Graphic Era University, IndiaCopyright © 2024 Hu, Qian, Wang, Sun, Wu, Zhang, Han, Wang, Ma and Yang. This is an open-access article distributed under the terms of the Creative Commons Attribution License (CC BY). The use, distribution or reproduction in other forums is permitted, provided the original author(s) and the copyright owner(s) are credited and that the original publication in this journal is cited, in accordance with accepted academic practice. No use, distribution or reproduction is permitted which does not comply with these terms.
*Correspondence: Desong Yang, eWRzX2FnckBzaHp1LmVkdS5jbg==
Disclaimer: All claims expressed in this article are solely those of the authors and do not necessarily represent those of their affiliated organizations, or those of the publisher, the editors and the reviewers. Any product that may be evaluated in this article or claim that may be made by its manufacturer is not guaranteed or endorsed by the publisher.
Research integrity at Frontiers
Learn more about the work of our research integrity team to safeguard the quality of each article we publish.