- 1Sección Fisiología & Genética Bacterianas, Facultad de Ciencias, Montevideo, Uruguay
- 2Sección Bioquímica, Facultad de Ciencias, Montevideo, Uruguay
Cotrimoxazole, the combined formulation of sulfamethoxazole and trimethoprim, is one of the treatments of choice for several infectious diseases, particularly urinary tract infections. Both components of cotrimoxazole are synthetic antimicrobial drugs, and their combination was introduced into medical therapeutics about half a century ago. In Gram-negative bacteria, resistance to cotrimoxazole is widespread, being based on the acquisition of genes from the auxiliary genome that confer resistance to each of its antibacterial components. Starting from previous knowledge on the genotype of resistance to sulfamethoxazole in a collection of cotrimoxazole resistant uropathogenic Escherichia coli strains, this work focused on the identification of the genetic bases of the trimethoprim resistance of these same strains. Molecular techniques employed included PCR and Sanger sequencing of specific amplicons, conjugation experiments and NGS sequencing of the transferred plasmids. Mobile genetic elements conferring the trimethoprim resistance phenotype were identified and included integrons, transposons and single gene cassettes. Therefore, strains exhibited several ways to jointly resist both antibiotics, implying different levels of genetic linkage between genes conferring resistance to sulfamethoxazole (sul) and trimethoprim (dfrA). Two structures were particularly interesting because they represented a highly cohesive arrangements ensuring cotrimoxazole resistance. They both carried a single gene cassette, dfrA14 or dfrA1, integrated in two different points of a conserved cluster sul2-strA-strB, carried on transferable plasmids. The results suggest that the pressure exerted by cotrimoxazole on bacteria of our environment is still promoting the evolution toward increasingly compact gene arrangements, carried by mobile genetic elements that move them in the genome and also transfer them horizontally among bacteria.
1 Introduction
Cotrimoxazole (SXT), the combination of trimethoprim (TMP) and sulfamethoxazole (SMX), is widely used in the treatment of several infections, particularly those affecting the urinary tract. As an effective and inexpensive medication, it is on the list of the World Health Organization of essential medicines (World Health Organization, 2023). Its antibiotic components act as competitive inhibitors of two enzymes of the folic acid (Fol) synthesis pathway, and their action is enhanced when combined. Due to their mode of action, they are generically called antifolates. SMX belongs to the family of sulfonamides, which includes several compounds that inhibit dihydropteroate synthase, the first enzyme in the Fol pathway, and TMP inhibits dihydrofolate reductase, the third and last enzyme required to produce tetrahydrofolate. This latter compound is the active cofactor that performs the essential function of providing one-carbon units to many biosynthetic reactions in cells (Green and Matthews, 2007). Sulfonamides opened the antibiotic era, being the first antibacterial drugs used systemically in medicine, since the 1930s. TMP was developed and employed in the 1960s, and SXT came into use shortly after due to the observed improved effect of the combination of TMP and SMX (Huovinen, 1997).
Over time, resistance to SXT emerged and has steadily increased, limiting its clinical use. Resistance to this combination is due to the sum of resistance to both its components, and is particularly frequent among Gram-negative bacteria. It is mediated by genes belonging to the accessory gene pool encoding variants of the dihydropteroate synthase and dihydrofolate reductase that are resistant to SMX and TMP, respectively. Currently, four SMX resistance genes have been described (sul1, 2, 3, and 4) and several dozen for TMP resistance (dfrA numbered in the order in which they were described, and a dfrB series of eight variants) (Sköld, 2001; Alonso and Gready, 2006; Ambrose and Hall, 2021; de los Santos et al., 2021; Alcock et al., 2023).
In principle, Gram-negative strains resistant to SXT contain at least one sul gene and one dfr gene. These are usually associated with mobile genetic elements that enable them to move within the bacterial genome, such as integrons and transposons, and to be transferred horizontally between bacteria, typically being carried on conjugative plasmids. The sul and dfrA genes are significantly associated with class 1 and 2 integrons. These are genetic elements often referred to as “clinical integrons” because they confer resistance to multiple antibiotics and are commonly found in Gram-negative clinical isolates. These features distinguish them from other integrons found in environmental bacteria (Labbate et al., 2009).
Integrons consist of a conserved platform and a variable region. In the platform they encode a site-specific recombination system that captures and integrates free gene cassettes into an attachment site (attI) so that they become part of the integron and are expressed as an operon from a promoter also provided by the platform. Then, the variable region of an integron is the array of gene cassettes it contains. As free circular molecules, gene cassettes usually contain a single gene without a promoter, followed by its specific attachment site (attC). The integrase recombines the attC and the attI sites to operate the gene cassette integration. The mechanism described above implies that the first cassette found in an array was in fact the last one to be integrated. Also, since it is located immediately after the promoter, this cassette would be the one expressed at a higher level (Hall, 2012; Cury et al., 2016).
Class 1 integrons (Int1) are most prevalent in clinical isolates of Enterobacteriaceae and other Gram-negative families. They usually contain one or two genes in their variable region: a dfrA gene and/or an aadA gene, conferring TMP and streptomycin resistance, respectively. The presence of other gene cassettes has been repeatedly reported, but is less frequent (Domingues et al., 2015). In the Int1, the platform is divided by the variable region into two parts: (i) a 5′ conserved segment (5′ CS), containing the site-specific integrase gene intI1, the Pc promoter that directs transcription of the integrated gene cassettes, and the attachment site attI; and (ii) a 3′ conserved segment (3′ CS), containing a truncated gene for an export pump (qacEΔ) and the sul1 gene, conferring resistance to SMX (Figure 1A) (Labbate et al., 2009). As to the Pc promoter, several variants with different strengths have been recognized, being most frequent PcW (weak) and PcH1 (hybrid 1). In addition, some integrons contain a second promoter downstream of Pc, called P2, which usually accompanies the PcW variant, enhancing its strength (PcW-P2) (Poey and Laviña, 2014). It is worth mentioning that sul1, which is not a gene cassette, is the only resistance gene residing in the conserved platform of Int1s (Labbate et al., 2009). In a previous study we showed that even when sul1 is deleted, Int1+ strains remain resistant to SMX thanks to the presence of an unlinked sul2 gene or, less frequently, of a sul3 gene. This led us to propose that the presence of an SMX resistance gene would be a requirement for Int1+ strains (de los Santos et al., 2021). Therefore, in principle, every strain bearing an Int1 contains at least one sul gene, and in the vast majority, a dfrA gene in the variable region. The result is that all Int1+ strains are SMX resistant and most of them are also SXT resistant.
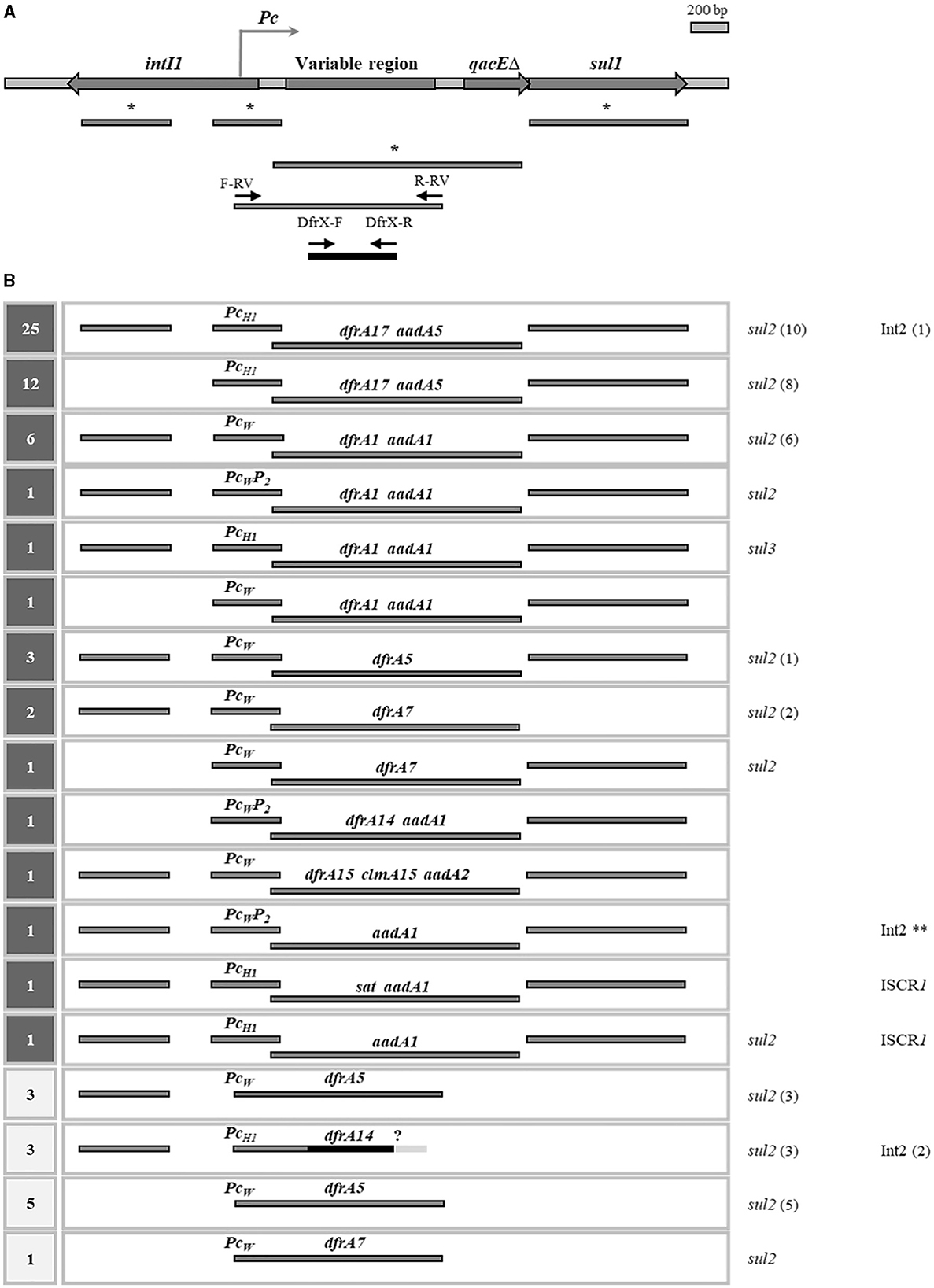
Figure 1. Class 1 integrons or its remnants in the cotrimoxazole resistant strains. (A) General structure of an Int1: genes are indicated with thick arrows; the genetic content of the variable region is not specified due to its variability. Below, bars indicate the generated amplicons to detect different parts of Int1 in previous (*) and present determinations. Small arrows, new primers used in this work. (B) Int1 structures detected in the 69 strains with Int1 sequences. At left, number of strains carrying each type of structure: in dark gray squares, previously determined; in clear squares, determined in this work. At right, presence of sul2, sul3, Int2, and ISCR1, with the number of strains in brackets. ?, unknown genetic content in the right end of the variable region. **, TMPR is encoded by the dfrA1 gene from the Int2. Previous determinations were described in Poey and Laviña (2014, 2018) and de los Santos et al. (2021).
Class 2 integrons (Int2) have a structure quite similar to that of Int1, but lack the 3′segment of the platform and have a nonsense mutation that inactivates the integrase gene intI2. Therefore, its structure is fixed and, in principle, its variable region is no longer variable. Nevertheless, it appears that the Int1 integrase would be able to trans-act on the Int2 attachment site, generating some variability; in fact, it is not uncommon to find both integrons 1 and 2 in the same strain. In its classical fixed structure, Int2 carries three antibiotic resistance genes in the form of gene cassettes: dfrA1, sat2, and aadA1, conferring resistance to TMP, streptothricin and streptomycin, respectively. This integron exemplifies very well the association of these types of elements with transposons. Int2 is located inside Tn7, so it moves while being carried by this transposon. Regarding its relationship with the resistance to antifolates, Int2 contains the dfrA1 gene cassette, and frequently resides in bacteria containing the sul2 gene elsewhere (Deng et al., 2015; de los Santos et al., 2021). Then, most Int2+ strains have a SXT resistant phenotype.
In this communication we address the genetic basis of cotrimoxazole resistance in a collection of 101 uropathogenic E. coli (UPEC) strains, gathering present with previous results (Poey and Laviña, 2014, 2018; de los Santos et al., 2021). These strains belong to a broader collection of 230 clinical isolates which had been gathered following an epidemiological design conducted in 2007–2009 in a hospital in Montevideo, Uruguay (Poey et al., 2012). Therefore, this collection is representative of the UPEC population circulating at that place and time. Since then, it has been studied from different points of view, including antibiotic resistance, presence of sul genes and of clinical integrons. Regarding antifolate resistance, more than half of the strains are resistant to one or both SMX and TMP, with a clear predominance of SXT resistance. These latter strains are 101, being the subject of interest of the present study. As to the genetic basis of their SXTR phenotype, they all contain at least one type of sul gene: sul1 (n = 27), sul2 (n = 46), sul1 and sul2 (n = 27), and sul1 and sul3 (n = 1), what explains their SMX resistance. Regarding the basis of their TMPR, we only had information on 54 strains, specifically those that contain an Int1 whose variable region could be amplified and sequenced, and which proved to have a dfrA gene cassette. These are: dfrA17 (n = 37), dfrA1 (n = 9), dfrA5 (n = 3), dfrA7 (n = 3), dfrA14 (n = 1), and dfrA15 (n = 1). In addition, the Pc promoter is also identified, thus completing the information on the central expression unit in these Int1s (Figures 1, 2) (Poey and Laviña, 2014, 2018; de los Santos et al., 2021). Therefore, the genetic basis of the SXTR of 54 strains had been previously elucidated and their TMPR proved to be due to dfrA gene cassettes included in class 1 integrons. In this work we devoted to complete the analysis of the genetic bases underlying TMPR in the 47 remaining SXTR strains and succeeded in identifying them in 40. Many were associated with Int1 remnants or with Int2, while others were found in other apparently less frequent genetic structures, including a novel gene arrangement.
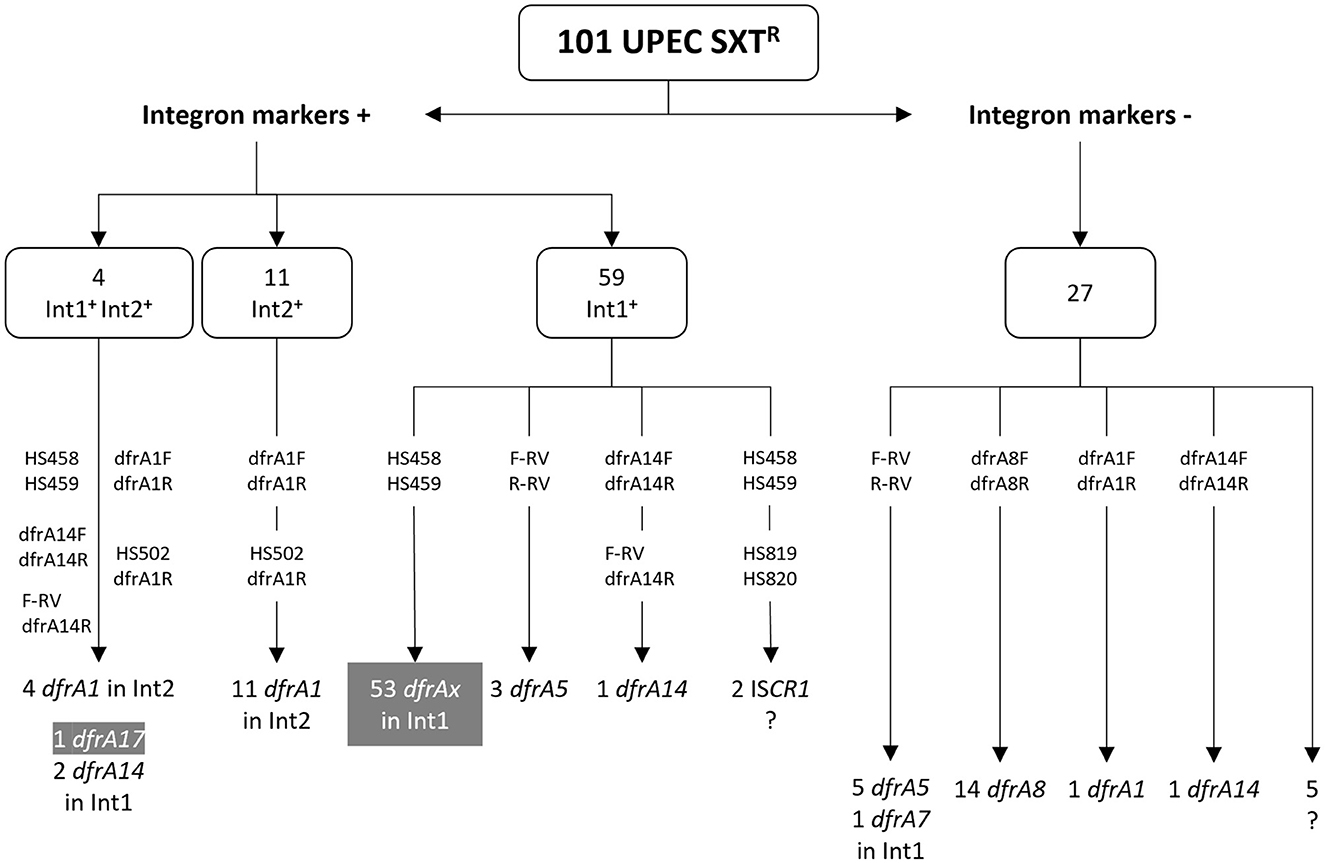
Figure 2. PCR amplifications for the detection of dfrA genes in the cotrimoxazole resistant strains. The number of strains with or without integron markers is shown above. Attached to vertical arrows, primer pairs that gave rise to amplicons. Below, type and number of dfrA genes identified; dfrAx generically designates several types of dfrA genes. In gray boxes, dfrA genes previously identified in amplicons with primer pair HS458 and HS459 (Poey and Laviña, 2018; de los Santos et al., 2021). ?, strains in which the gene responsible for the TMPR phenotype was not found.
2 Materials and methods
2.1 Bacterial strains and culture conditions
One hundred and one STXR UPEC isolates were considered for the analysis: 28 were isolated from pregnant women (PW) and 73 from children (CH). They belonged to a larger UPEC collection of 230 isolates from the Laboratory of Bacteriology of an obstetric and pediatric hospital in Montevideo, Uruguay (Centro Hospitalario Pereira Rossell). The isolates were collected in 2007 (PW) and 2008–2009 (CH) using the following inclusion criteria: they were all the E. coli isolates proceeding from different patients with urinary tract infection or that proved to be different when coming from the same patient. The 230 isolates were previously characterized for their phylogeny, virulence, antibiotic resistance and for the presence of class 1, 2, and 3 integrons, and were then considered as strains. Of the 101 SXTR strains, 63 contained an Int1 (by the criterion of being intI1+ and/or sul1+), 15 an Int2 (by the criterion of being intI2+), and four of them had both Int1 and Int2, and none had a class 3 integron (Poey et al., 2012; Poey and Laviña, 2014; de los Santos et al., 2021).
Strains were grown in LB medium at 37°C. Antibiotics were added to media at the following final concentrations: SMX, 800 μg/ml; TMP, 100 μg/ml; SXT, the combination of TMP, 100 μg/ml plus SMX, 500 μg/ml; nalidixic acid (NAL), 40 μg/ml, and kanamycin (KAN), 30 μg/ml.
2.2 PCR assays
The presence of genes encoding TMP resistance was searched for by PCR and primers designed are presented in Table 1. Primer pairs were used to amplify internal segments of some selected dfrA genes. Since our study was being performed on E. coli strains, the most common dfrA variants for this species were surveyed in the GenBank using the Blastn program (https://blast.ncbi.nlm.nih.gov/Blast.cgi) and, as queries, the dfrA sequences in CARD (Comprehensive Antibiotic Resistance Database) (https://card.mcmaster.ca/ontology/40753; Alcock et al., 2023). Nine dfrA variants were selected because they appeared in more than 15 releases as of June 2020. These genes and their frequencies of occurrence were: dfrA17 (461), dfrA12 (274), dfrA1 (165), dfrA7 (144), dfrA14 (111), dfrA5 (70), dfrA27 (41), dfrA15 (23), and dfrA8 (17). The presence of the uncommon genes dfrB (8 variants) was assayed with a generic pair of primers. In addition, primers F-RV and R-RV were designed to generate amplicons tightly adjusted to the Pc-gene cassettes region, which proved to be particularly useful when the Int1 was deleted for most of the 3'CS (Figure 1). Complex class 1 integrons were surveyed through the detection of the ISCR1 (Insertion Sequence Common Region associated to Int1) with primers described previously: HS819 (5′ GGGCCAGGTCTTGAGTATCG 3′) and HS820 (5′GCTTCGGCCATCACACC 3′), using an annealing temperature of 55°C and generating an expected product size of 522 bp (Márquez et al., 2008). The linkage between the intI2 and the dfrA1 genes in Int2+ strains was assayed with primers dfrA1-15R and HS502 (5′ GTAGCAAACGAGTGACGAAATG 3′) (Márquez et al., 2008), with an annealing temperature of 52°C and an expected product size of 1,619 bp.
PCR-amplifications were performed in a volume of 30 μl containing 1X buffer, 200 μM of each deoxynucleotide triphosphate, 200 nM of each primer, 2U of High Taq polymerase (BIORON), and 10 μl of cell lysate (prepared by the boil lysis method). The conditions used were: 2 min at 94°C, followed by 30 cycles of 94°C for 30 s, the annealing temperature for 30 s and extension at 72°C for 30 s or more, depending on the size of the amplicon, and a final extension step at 72°C for 2 min.
2.3 Conjugation and transformation experiments
Conjugation was performed essentially as described previously (Poey and Laviña, 2018). Several UPEC SXTR and sensitive to KAN and NAL were used as donors and an E. coli K12 derivative strain (hsdR::kan gyrA; KANR and NALR) as recipient. Transconjugants were selected on LB plates supplemented with TMP and KAN. In parallel, cultures of the parental strains were seeded separately on the same selective medium as controls. Some clones grown on the experimental plates were purified. To further confirm that they were true transconjugants, and not spontaneous KANR mutants of the donor, they were seeded on plates with NAL to corroborate their NALR phenotype. For transformation, the same E. coli K12 recipient strain was made competent using the standard protocol with calcium chloride (Sambrook et al., 1989). Transformants were selected on plates with trimethoprim.
2.4 DNA sequencing
All DNA sequencing was performed at Macrogen Inc. (Seoul, Korea). PCR-amplicons were sequenced by the Sanger method. Plasmids from five UPEC strains that had been transferred by conjugation to an E. coli K12 were extracted with the NucleoSpin Plasmid kit (Macherey-Nagel GmbH & Co. KG, Germany) and outsourced to Macrogen for sequencing. The library was prepared using a Nextera XT DNA Library Preparation kit (Illumina) and NGS was performed with an Illumina HiSeq X platform, obtaining paired-ended reads of 151 bp. Reads were quality-trimmed with Trimmomatic and cleaned with Bowtie2 to remove E. coli W3110 chromosome contaminating reads (GenBank: CP017979). The assembly was carried out with Unicycler within the BV-BRC server (https://www.bv-brc.org/) (Olson et al., 2023), and gene annotation was performed with Prokka 1.14.6 (Seemann, 2014).
2.5 GenBank accession numbers
Plasmid sequences have been submitted to GenBank under the following accession numbers: p31CH-1, draft assembly (contig of 91.664 nt) (PP566049); p61CH-1m, complete sequence of 6.799 nt (PP537156); p16CH, draft assembly (contig of 63.631 nt) (PP566048); p46CH-1, complete sequence of 73.233 nt (PP537157); and p47CH-1, complete sequence of 76.288 nt (PP566047).
3 Results
This study first concentrated on completing the characterization of the genetic basis of the TMPR in the 101 SXTR UPEC collection, i.e., in the remaining 47 strains for which we lacked this information. Whenever possible, we sought to identify the genes responsible for the TMPR phenotype as well as their genetic context. The final goal was to gather previous and present results to reach a general view on the genetics of cotrimoxazole resistance in E. coli and also on the mobile genetic elements involved.
The search for genes conferring TMPR first focused on strains containing class 1 or class 2 integrons, and then addressed the study of the remaining SXTR strains.
3.1 Genetics of resistance to trimethoprim in SXTR strains carrying class 1 and/or 2 integrons
A survey of class 1 and 2 integrons had previously been performed on the entire UPEC collection of 230 strains: Int1 was detected by the presence of the gene markers intI1 and/or sul1 in 66 isolates, and Int2 in 19 isolates by detecting the gene for its integrase intI2; four of them carried both integrons. This gave a total of 81 strains that were considered integron-positive, 74 of which were SXTR (91%): 59 Int1+ only, 11 Int2+ only, 4 with both Int1+ and Int2+ (Poey and Laviña, 2014; de los Santos et al., 2021). In 57 Int1+ SXTR strains, the variable region could be amplified with primers widely used by many authors and in 54 of them a dfrA variant was identified, thus accounting for the TMPR phenotype (Figures 1B, 2) (Poey and Laviña, 2014, 2018; de los Santos et al., 2021).
From here on, the results correspond to the present work. We first analyzed the three Int1+ strains in which there was no dfrA gene in the variable region. One of them also contained an Int2, which provided a dfrA1 gene (see below). The other two strains contained complex integrons, as an ISCR1 was PCR-detected in them; due to the many different arrangements that can be found in such complex Int1 elements and that they usually contain dfrA genes of infrequent types, no further efforts were made to identify the genes responsible for the TMPR phenotype of these two strains (Figures 1B, 2) (Toleman et al., 2006).
It still remained to identify the TMPR determinants in six Int1+ strains whose variable region had not been able to be amplified. They all lacked sul1, indicating that they were deleted for all or part of the integron's 3' conserved region. Then, the primer pair F-RV and R-RV, adjusted to the Pc-variable region, was used, and in three cases an amplicon was generated and then sequenced. The three strains had the same gene content: a PcW promoter and dfrA5 as a unique gene cassette (Figures 1B, 2). In the remaining three strains, the search continued using primer pairs internal to the dfrA genes and thus it was found that they contained a dfrA14 variant. Then, its association with an Int1 platform was confirmed by amplifying with primers F-RV and dfrA14-R, and a dfrA14 cassette preceded by a PcH1 promoter was found (Figures 1B, 2).
In relation to the Int2+ strains, the dfrA1 gene was searched for using a pair of primers internal to this gene. As expected, the 15 SXTR strains carrying an Int2 were positive for dfrA1, including the four cases in which the Int2 coexisted with Int1. The linkage of dfrA1 with the intI2 gene was confirmed by PCR in all the Int2+ strains.
It was considered that the remaining 27 SXTR strains, although being negative for the integron markers (intI1, sul1, and intI2), might still retain remnants of an integron with gene cassettes encoding resistance to TMP. It should be remembered that all of them owed their resistance to SMX to a sul2 gene. First, the 27 strains were PCR-assayed for the Int1 variable region employing primers tightly adjusted to the Pc-gene cassettes region. There was a successful amplification in six of them and, indeed, the amplicons contained dfrA gene cassettes: five a PcW followed by dfrA5, as a unique gene, and one a similar content, but with dfrA7. Therefore, although these six strains had been cataloged as Int1−, they ultimately resulted to keep the central part of an Int1 (Figures 1B, 2).
In sum, with the exception of the two ISCR1-containing strains, the SXTR phenotype could be completely explained by the presence of well-defined dfrA genes contained in the variable region of class 1 or 2 integrons in 78 strains.
3.2 Genetics of resistance to trimethoprim in strains without integrons
In this part of the work, we specifically focused on identifying the genes responsible for TMPR in the remaining 21 SXTR UPEC strains. PCR-reactions were assayed with primers internal to the dfrA genes, and in 16 of them a dfrA gene could be identified: dfrA1 in one, dfrA14 in another, and dfrA8 in 14 (Figure 2). With this approach, the genetic context of the genes remained unknown. As to dfrA8, it is known that it is not related to an integron but to a compound transposon (Tn5091). In this structure, dfrA8 appears together with another gene, sbcD, encoding a subunit of a DNA repair exonuclease, both genes being flanked by IS26 elements (Sundström et al., 1995).
To gain more insight into the genetics of these 21 remaining SXTR strains, conjugation experiments were designed to attempt to transfer their TMPR determinants to a laboratory E. coli K12 strain. Due to the resistance profile of the UPEC strains, this approach could be applied to 20 of them. In five crossings, TMPR transconjugants grew, so that the determinants of this phenotype were located in transferable plasmids of the corresponding five UPEC strains. Their dfrA genes had already been identified by internal primers (1 dfrA1, 1 dfrA14, and 3 dfrA8), but, as already mentioned, their genetic context remained unknown. The plasmid content of the five transconjugants was extracted: each of them had a large plasmid, but one also contained a small one. This latter was transformed into competent E. coli K12 cells and TMPR clones grew, thus proving that the small plasmid, which was called p61CH-1m, was responsible for that phenotype and had been mobilized during conjugation by an accompanying large conjugative plasmid. The five plasmids, each carrying a dfrA gene, were then sequenced: p61CH-1m (dfrA1), p31CH-1 (dfrA14), and the remaining three, p16CH, p46CH-1 and p47CH-1 (dfrA8). Their names are the same as those of the UPEC strains, and in the case of p61CH-1m, the “m” was added to indicate that it was mobilizable.
The dfrA14 gene in the large conjugative plasmid p31CH-1 (>91 kb) was a single gene cassette that was located in a two-gene locus conferring streptomycin resistance, strA strB-also named APH(3′')-Ib and APH(6)-Id, respectively- interrupting the strA gene. In fact, this same strA'-dfrA14-'strA structure had already been found in small, globally disseminated plasmids from strains of several bacterial genera, recovered in many countries. It was repeatedly found in the larger genetic cluster sul2-strA'-dfrA14-'strA-strB (Ojo et al., 2002; Anantham and Hall, 2012; Miranda et al., 2016). In the last years, more GenBank entries have appeared containing this gene arrangement and a few corresponded to large, possibly conjugative plasmids. The comparative analysis of this region of p31CH-1 with that of selected plasmids from the data bank is presented in Figure 3A. The comparison included a large plasmid, pME20 (from an E. coli isolated from a wastewater treatment plant in France), which presented the highest homology with p31CH-1 through its entire length but lacked the dfrA14 cassette insertion, and two small plasmids, almost identical to each other except for the presence (pCERC1) or absence (p9123) of the dfrA14 cassette. In addition, both large plasmids p31CH-1 and pME20 shared a transposon Tn3 interrupting the strB gene exactly at the same position, a marker that points to their close relationship. Therefore, the dfrA14 insertion into the strA gene of plasmid p31CH-1 was found to be widespread among small plasmids of several enterobacterial strains, always integrating the sul2-strA'-dfrA14-'strA-strB genetic arrangement, a locus that confers the phenotype of SXT resistance.
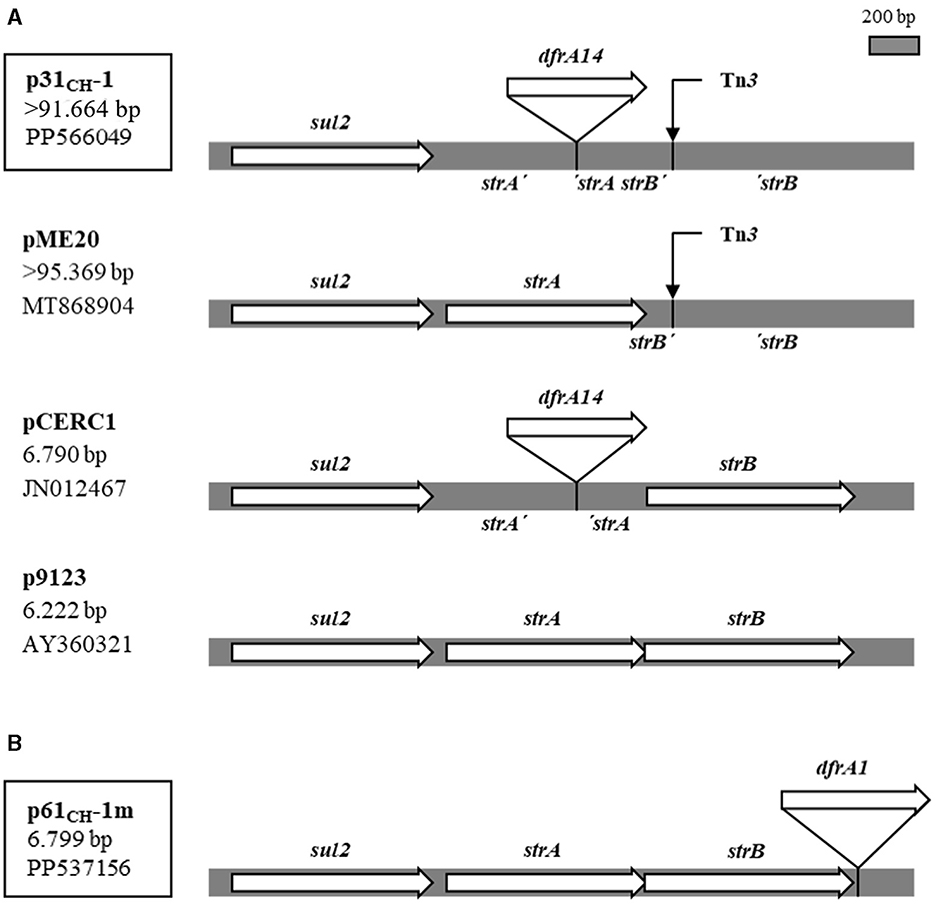
Figure 3. Single dfrA gene-cassettes inserted into the sul2 strA strB locus in plasmids p31CH-1 and p61CH-1m. (A) Structure of the locus in p31CH-1 compared with the same region in large and small plasmids with and without the dfrA14 gene cassette insertion. The site of a Tn3 insertion (4.957 bp) is indicated with a thin arrow. (B) The same locus with the dfrA1 gene cassette insertion in p61CH-1m. At left, plasmids name, size and GenBank accession number. Plasmids from this work are boxed.
The mobilizable plasmid containing a dfrA1 gene, p61CH-1m, was completely sequenced and found to be small, of 6.799 bp. The dfrA1 gene was recognized as a single gene-cassette not linked to any integron. Instead, it was inserted 21 bp downstream of a strB gene, in a cluster sul2-strA-strB-dfrA1 and being read in the same direction as the other genes in the arrangement (Figure 3B). In a Blastn search, four plasmids appeared with dfrA1 in the same context: two of them, proceeding from E. coli isolates from racehorses in Hong Kong, were quasi-identical to p61P-1m, and the other two were larger plasmids from strains of the Acinetobacter genus isolated in China. Many other GenBank entries corresponded to plasmids very similar to p61P-1m, but all lacked the dfrA1 gene. Therefore, plasmid 61CH-1m contained a novel gene arrangement conferring SXT resistance. Regarding its condition of mobilizable, a putative oriT sequence was identified in p61P-1m (gggtttcggggcgcagccctgaaccagtcatgtagcgctagcggagtgtatactggctta), which was of the ColE1 type (Francia et al., 2004).
The three remaining plasmids that were sequenced, p16CH, p46CH-1, and p47CH-1, were all conjugative and quite large. They were highly homologous to each other and all contained a dfrA8 gene into the transposon Tn5091. The three plasmids had the same point of insertion of the transposon, close to a blaTEM gene, and quite near this latter there was a sul2-strA-strB locus. As to the remaining 11 strains with dfrA8, it seems reasonable to assume that they all belonged to the same transposon structure. Tn5091 was first described in 1995 (Sundström et al., 1995) and since then dfrA8 has been detected in many surveys performed in different parts of the world. When searching in the GenBank with Blastn, using the sequences of dfrA8 and of Tn5091 as queries, matches coincided, indicating that dfrA8 is in fact a conserved constituent of this transposon. In addition, it was observed that Tn5091 was generally located in large plasmids.
In our search for the genetic bases of the TMPR in the 21 SXTR strains considered here, we failed to find them in five strains. Three further possibilities were then assayed. First, considering that the five strains were sensitive to streptomycin and sul2+, we investigated the possible presence of an untested dfrA gene cassette insertion within a strA gene, as was the case in plasmid p31CH-1. The search was performed by PCR with primers annealing to the beginning and end of strA so as to detect if an insertion had occurred between them (Table 1). The result was that there was no amplification at all, unlike other strains from the collection that were strA+ (band of 857 bp) or to a strain with p31CH-1 (band of 1,425 bp). Other possible determinants of a TMPR phenotype could be the rare dfrB genes (8 variants), which are Int1-associated gene cassettes; this was assayed with a generic pair of primers and no amplicon was generated (Table 1). Finally, we considered that a mutation in the chromosomal gene folA, encoding the dihydrofolate reductase of the Fol pathway, could be responsible for the TMPR phenotype. Then, the folA gene and its upstream sequences were amplified and sequenced, but no changes appeared relative to the wild type genotype (Table 1).
In sum, considering previous and present results on the 101 SXTR UPEC strains, it was found that 78 owed their phenotype of TMPR to a dfrA gene cassette contained in a class 1 or 2 integron, two to dfrA single gene cassettes, and 14 to the dfrA8 gene carried in a transposon (Figure 4). Curiously, no strain contained the dfrA27 gene cassette nor the highly frequent dfrA12 one.
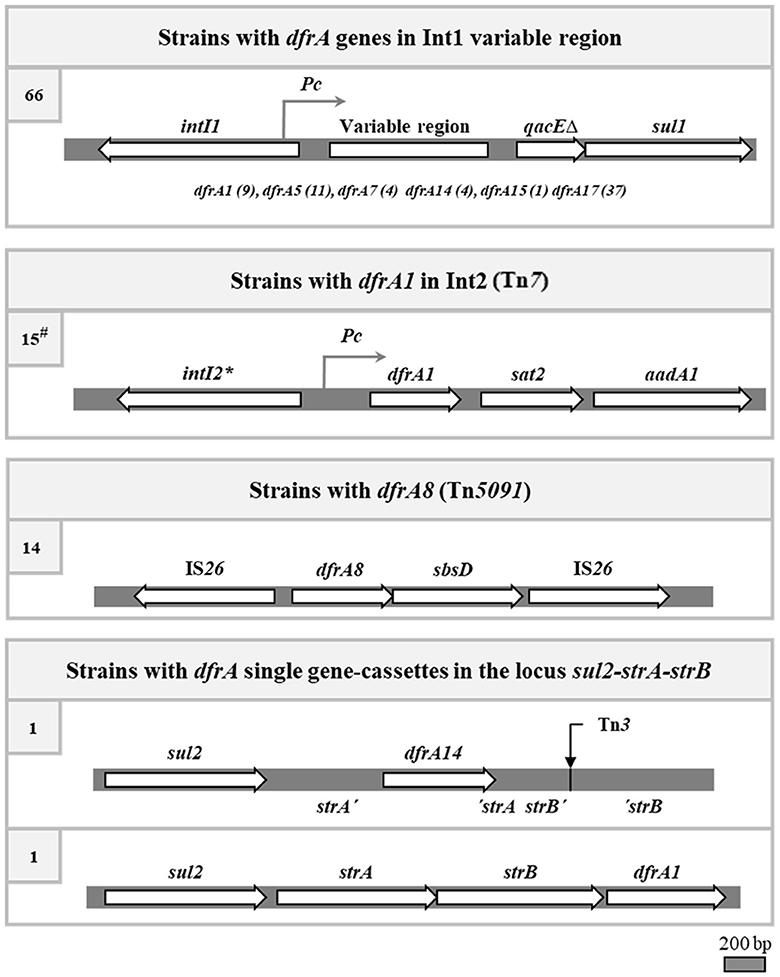
Figure 4. The four identified genetic contexts responsible for the trimethoprim resistance in the SXTR strains. The genetic maps of the canonical integrons and transposons are depicted, although some strains may have deletions of parts of them. *, stop codon inactivating the intI2 gene. At left, number of strains carrying each type of structure. #, four of the 15 strains with an Int2 also have an Int1, and in one of them the dfrA1 gene of Int2 is the only responsible for the TMPR of the strain.
4 Discussion
In this work we present the genetic bases of cotrimoxazole resistance in a collection of 101 uropathogenic E. coli strains. The study integrates previous and present results to provide the most complete understanding possible of the joint resistance to both antifolate components of the SXT formulation. In addition, considering the systematic strategy followed to collect the strains, the view provided here acquires epidemiological significance.
A generalized observation is that resistance to antifolates is strongly related to the presence of clinical integrons in many Gram-negative isolates from specimens of infected material. SXT is one of the treatments of choice for urinary tract infections, which are most frequently caused by E. coli strains, so testing for SXT resistance in UPEC isolates is routinely performed in the clinics. In a recent article, a 100% association was found between the phenotype of SXT resistance and the detection of class 1, 2, and 3 integrase genes when PCR reactions were applied directly to urine samples (Elias et al., 2022). In the present work we also found an important, albeit lower association, around 80%, between SXTR and class 1 and 2 integrons. To reach this figure we had to survey many portions of integrons, particularly of Int1, in a significant number of UPEC isolates.
Int1 or remnants of it were thoroughly searched for and were found in 69 of the 101 SXTR strains. In all but three, a dfrA gene cassette was found in the variable region, which explained the TMPR phenotype of the strains (Figure 1). Although more than 40 dfrA variants have been described (Ambrose and Hall, 2021; Alcock et al., 2023), only six were found in the Int1 sequences of our SXTR collection, and these were among the most frequently found in multiple surveys worldwide. As observed by other authors, the most commonly found cassette arrays were dfrA17-aadA5 and dfrA1-aadA1, while dfrA5 and dfrA7 appeared as unique cassettes (Figure 1) (Domingues et al., 2015). One of the strains that lacked a dfrA gene in its Int1 variable region also had an Int2, which provided its dfrA1 gene. The other two were positive for the ISCR1 element, a marker of a complex Int1 structure that contains non-cassette resistance genes outside the Int1 variable region, dfrA among them. These latter dfrA genes are quite unusual and are specifically found in ISCR1 complex integrons, e.g., dfrA10, 11, 19, among others (Toleman et al., 2006; Ambrose and Hall, 2021). It seems very likely that the two ISCR1+ strains in our collection possess one of these dfrA genes.
Two apparently abundant dfrA variants, as found in databases and in multiple reports, are dfrA12 and dfrA27. However, these were not found in our collection, a result that raises the question of whether there could be differences in gene prevalence between geographic regions and time of strain collection. This topic has been addressed by some groups with varying results. In a survey of integrons and genes conferring SXTR performed on UPEC strains from Europe and Canada in 1999 and 2000, no regional dependence was observed (Blahna et al., 2006). On the contrary, other authors found differences in the prevalence of dfrA variants and of gene cassette arrays in surveys conducted in other regions as well as in follow-up studies over several years (Yu et al., 2003; Su et al., 2006; Dahmen et al., 2010; Manyahi et al., 2017). Perhaps the dfrA set of variants found in our study may indeed reveal a certain bias with respect to other parts of the world and to the time period in which the UPEC isolates were collected (2007–2009).
An important focus in this work was on the Int1 central expression unit, composed of a Pc promoter and the gene cassettes under its transcriptional influence. A close relationship was found between these elements: the most frequent cassette array, dfrA17-aadA5, was invariably under the PcH1 promoter, while PcW alone or reinforced by P2 generally preceded the other types of arrays (Figure 1). In fact, the number of different combinations of all these central elements was quite limited. The Int1 central expression unit analyzed here was found in canonical integrons, with their entire structure (40), but also in remnants lacking the intI1 gene from the 5′ CS (15), or lacking sul1 from the 3′ CS (8), or lacking both (6). Considering these results and our previous analyses on the whole UPEC collection, it should be noted that no strain was found with only the 5′ CS and only one just conserved the 3' CS (qacEΔ-sul1) (Poey and Laviña, 2014, 2018; de los Santos et al., 2021). This suggests that the Int1 variable region would paradoxically be the most “conserved region” of the integron, being particularly selected over time.
Gene cassettes are the mobile genetic elements that characterize integrons, and each is a gene followed by its cognate attC site (Patridge et al., 2009; Hall, 2012; Cury et al., 2016). In most cases, they can be simply recognized as such when located in the variable region of an integron, but they must be analyzed at the sequence level when they are outside their normal integration site. This is what happened with two of the SXTR strains in our collection, whose dfrA genes could only be detected by PCR using primers internal to these genes, being one dfrA14 and the other dfrA1 (Figure 3). They were carried by plasmids that could be transferred by conjugation to an E. coli K12 and then were sequenced. Both dfrA genes proved to be true gene cassettes, each one with its specific attC site, but located outside an integron; that is, they were single gene cassettes.
The dfrA14 cassette, carried by a large conjugative plasmid, was inserted within a strA gene. This same location has been found repeatedly in small plasmids from different bacterial genera in many countries, and the gene arrangement described is sul2-strA'-dfrA14-'strA-strB. The question arose as to how it had been transferred horizontally since it was carried by small plasmids that apparently could not be mobilized by conjugation (Ojo et al., 2002; Anantham and Hall, 2012; Miranda et al., 2016). However, the same insertion was also detected in E. coli isolates from food animals in Lithuania and could be transferred by conjugation, although the plasmid containing it was not further analyzed (Šeputiene et al., 2010). In the present work, a variant of the described arrangement was found in a conjugative plasmid, p31CH-1, which included the addition of a Tn3 insertion in strB: sul2-strA'-dfrA14-'strA-strB'-Tn3-'strB. As shown in Figure 3A, small and large plasmids contain the region of reference, with or without the dfrA14 insertion, but both large plasmids share the same Tn3 insertion. In fact, p31CH-1 and pME20 are highly homologous to each other in all their extension, and their main difference is the presence or not of the dfrA14 gene cassette into strA. Therefore, pME20 would be representative of a p31CH-1 precursor, and their comparison indicates that p31CH-1 could have acquired the dfrA14 insertion by exchange of a DNA segment, for example with a small plasmid carrying the sul2-strA'-dfrA14-'strA-strB arrangement. This would most likely be operated by homologous recombination occurring in sequences flanking the dfrA14 cassette in a situation in which both the large conjugative plasmid and a small plasmid with the cluster sul2-strA'-dfrA14-'strA-strB coexisted in the same bacterium. Then, the presence of the arrangement in conjugative plasmids explains its horizontal transfer between strains, species and related genera. Curiously, the UPEC carrying p31CH-1 was isolated from a child with urinary tract infection in 2008, a date that coincides with an outbreak of shigellosis in Chile caused by a strain of Shigella sonnei carrying the same sul2-strA'-dfrA14-'strA-strB arrangement in a small plasmid (Miranda et al., 2016). At this point, it should be recognized that this genetic arrangement is undoubtedly successful given its wide dissemination among bacteria all over the world, and would represent a gene combination different from integrons that confers resistance to cotrimoxazole.
As to the single dfrA1 gene cassette in the small mobilizable plasmid p61CH-1m, it was found to be included in the arrangement sul2-strA-strB-dfrA1, being inserted very close to the end of strB, but not interrupting any gene (Figure 3B). Therefore, dfrA1 must be expressed together with strA and strB as an operon. In this case, only four entries in GenBank presented this gene cluster and, notably, two plasmids from racehorses in Hong Kong (in releases dated 2022) were quasi-identical to p61CH-1m in extension and content, a fact that could be related to the export of this type of horses from our region to Hong Kong. Apparently, the genetic arrangement sul2-strA-strB-dfrA1 in p61CH-1m has not been widely spread, so that it appears to be a relatively new gene arrangement conferring SXT resistance. In fact, it could be considered that this locus might have been formed in the very context in which the UPEC carrying this plasmid was isolated from the infected urine of a child. Although the isolate was collected some 15 years ago, it should be kept in mind that the horizontal transfer of the arrangement would depend on the chance of coexistence of the mobilizable small plasmid carrying it with a compatible conjugative one, and this requirement could reduce the probability of its spreading. In this sense, a putative ColE1 type oriT sequence was detected in p61CH-1m. This would make it susceptible to mobilization by conjugative plasmids belonging to several incompatibility groups. For example, these include members of the broad host range group IncP, which is known to spread antibiotic resistance genes among Gram-negative bacteria (Francia et al., 2004; Popowska and Krawczyk-Balska, 2013). Therefore, it could be expected that the wide dissemination of the sul2-strA-strB-dfrA1 locus found in p61CH-1m is only a matter of time.
As to the 15 strains bearing an Int2, they all contributed to the SXTR with a dfrA1 gene, in accordance with their fixed structure. Therefore, summing up the 78 strains with dfrA genes inside class 1 and 2 integrons, the two strains with complex Int1 containing an ISCR1, plus two with the single gene cassettes dfrA1 and dfrA14, there were still a good number missing to reach the total of 101 SXTR strains. Of them, 14 resulted to have a dfrA8 gene, known to be part of the composite transposon Tn5091, as corroborated in the three sequenced dfrA8-carrying plasmids. These also contained a sul2 gene, which together with the dfrA8 gene conferred the SXTR phenotype. It should also be mentioned that in the three plasmids sul2 was found in a locus sul2-strA-strB, a few kilobases apart from the Tn5091.
In sum, in this work we elucidated the genetic basis of TMP resistance in 40 of the 47 SXTR strains of the collection that remained to be analyzed. Combining these results with previous findings, four main genetic contexts responsible for the TMPR phenotype were identified: class 1 integrons, class 2 integrons, transposon Tn5091, and dfrA single gene-cassettes integrated into the sul2-strA-strB locus (Figure 4). These data were then correlated with the already known sul content of the strains, so that the genetic basis of the cotrimoxazole resistance were determined in 94 of the 101 SXTR strains of the collection (Poey and Laviña, 2018; de los Santos et al., 2021). In these strains, the genetic linkage between the sul and dfrA genes varied depending on the genetic element involved. The loosest linkage would be that of the Int2 with sul2: Tn7, which contains the Int2, is most frequently located in the chromosome at its attachment site and we found no reference of its proximity to a sul gene (Peters, 2014). Similarly, dfrA8, contained in Tn5091, would not be closely linked to a sul gene, although the 14 strains carrying it were positive for sul2. The three plasmids dfrA8+ that were sequenced in this work contained the entire transposon and, quite near it, a sul2 gene included in the sul2 strA strB locus; therefore, sul2 and dfrA8 were in the same replicon and had been co-transferred by conjugation. Class 1 integrons and the two sul2 strA strB derivative structures containing dfrA gene cassettes exhibited the most compact arrangements bringing together the two types of antifolate resistance genes. It could be thought that the evolutionary trend is toward the co-carriage of sul and dfrA genes in the same mobile genetic element so that they can be transferred together to new cellular hosts. The pressure exerted by the clinical use of cotrimoxazole should have strongly influenced in this direction. Since the use of sulfonamides preceded that of TMP, the insertion of dfrA genes close to a preexisting sul-containing genetic structure must have been selectively favored. The two most commonly found sul genes are sul1, which is part of class 1 integrons, and sul2, which is often found adjacent to the strA strB genes (Yau et al., 2010; de los Santos et al., 2021). These are precisely the most compact structures encoding SXT resistance.
Taking into account that antifolates are synthetic, resistance to them should have appeared in clinical isolates after the introduction of these drugs in antimicrobial treatments, i.e., after some 80 years ago for sulfonamides and 50 for TMP. In this sense, there is a collection of historical strains (1917–1954) -the Murray collection- of several hundred isolates of mainly Enterobacteriaceae, including many clinical species that are today enriched in antibiotic resistance genes. This collection is being analyzed by some groups in the world and, significantly, no traces of clinical integrons or of antifolate resistance genes have been found (Baker et al., 2015; Sütterlin et al., 2020). Therefore, it can be presumed that antifolate resistance is a relatively recent phenomenon, at least among clinical Gram-negative bacteria, and that it may still be actively evolving. In particular, the pressure exerted by the intensive administration of cotrimoxazole must have been strong enough to select for clones bearing both sul and dfr genes, which predominate over those carrying only one of these genes (de los Santos et al., 2021). As previously mentioned, sul genes integrate or accompany clinical integrons and dfrA genes are mostly located inside them, so that these mobile genetic elements could have reached their current structure and bacterial hosts in the last decades. For instance, dfrA genes are almost always in the first position in integron arrays, which indicates that these genes were the last to be acquired. It should be remembered that soon after the introduction of TMP into medical practice, descriptions appeared of enterobacterial clinical isolates, particularly from infected urines, that were highly resistant to TMP. In one of them, only 3 years after TMP became available for general use in medicine, R factors (resistance plasmids) encoding resistance to SXT were detected through transferring them to an E. coli K12 by conjugation (Fleming et al., 1972). Most probably, these plasmids carried already formed integrons.
In our collection, we found that class 1 integrons, endowed with the resource of a site-specific recombination system, were the main responsible for SXTR, but we also found that there were other elements contributing to this phenotype. Transposons, represented by Tn7, which contains an already fixed class 2 integron, and Tn5091, carrying the dfrA8 gene, both equally conferred TMP resistance to a good number of additional SXTR strains, while coexisting with an unlinked sul2 gene. Two further genetic structures were detected, involving dfrA single gene cassettes that associated with a specific locus formed by the sul2 strA strB genes, which may represent the beginnings of well-structured new genetic elements encoding SXT resistance. The recent origin of both arrangements can be deduced from the very limited distribution of one of them (sul2- strA-strB-dfrA1), and from the persistence of the intact sequence of strA, turned into a pseudogene by the insertion of a dfrA14 gene, in the other structure.
Finally, some of all these genetic structures conferring antifolate resistance could be horizontally transferred by conjugation, conjugative mobilization and even transduction, as shown previously (Poey and Laviña, 2018) and in the present communication. In sum, the idea emerges that the genetic structures conferring SXT resistance in Gram-negative bacteria are constantly evolving by recombination, transposition and horizontal gene transfer.
Data availability statement
The datasets presented in this study can be found in online repositories. The data has been deposited at https://www.ncbi.nlm.nih.gov/ with accession numbers: PP566047–PP566049.
Ethics statement
Ethical approval was not required for the study involving humans in accordance with the local legislation and institutional requirements. Written informed consent to participate in this study was not required from the participants or the participants' legal guardians/next of kin in accordance with the national legislation and the institutional requirements.
Author contributions
MP: Conceptualization, Investigation, Methodology, Supervision, Writing – review & editing. ES: Conceptualization, Investigation, Methodology, Writing – review & editing. DA: Investigation, Writing – review & editing. CG-L: Data curation, Investigation, Methodology, Software, Writing – review & editing. ML: Writing – original draft, Writing – review & editing.
Funding
The author(s) declare that financial support was received for the research, authorship, and/or publication of this article. This work was supported by Programa de Desarrollo de las Ciencias Básicas, Uruguay.
Conflict of interest
The authors declare that the research was conducted in the absence of any commercial or financial relationships that could be construed as a potential conflict of interest.
Publisher's note
All claims expressed in this article are solely those of the authors and do not necessarily represent those of their affiliated organizations, or those of the publisher, the editors and the reviewers. Any product that may be evaluated in this article, or claim that may be made by its manufacturer, is not guaranteed or endorsed by the publisher.
References
Alcock, B. P., Huynh, W., Chalil, R., Smith, K. W., Raphenya, A. R., Wlodarski, M. A., et al. (2023). CARD 2023: expanded curation, support for machine learning, and resistome prediction at the Comprehensive Antibiotic Resistance Database. Nucleic Acids Res. 51, D690–D699. doi: 10.1093/nar/gkac920
Alonso, H., and Gready, J. E. (2006). Integron-sequestered dihydrofolate reductase: a recently redeployed enzyme. Trends Microb. 14, 236–242. doi: 10.1016/j.tim.2006.03.003
Ambrose, S. J., and Hall, R. M. (2021). dfrA trimethoprim resistance genes found in Gram-negative bacteria: compilation and unambiguous numbering. J. Antimicrob. Chemother. 76, 2748–2756. doi: 10.1093/jac/dkab212
Anantham, S., and Hall, R. M. (2012). pCERC1, a small, globally disseminated plasmid carrying the dfrA14 cassette in the strA gene of the sul2-strA-strB gene cluster. Microb. Drug Resist. 18, 364–371. doi: 10.1089/mdr.2012.0008
Baker, K. S., Burnett, E., McGregor, H., Deheer-Graham, A., Boinett, C., Langridge, G. C., et al. (2015). The Murray collection of pre-antibiotic era Enterobacteriaceae: a unique research resource. Gen. Med. 7:97. doi: 10.1186/s13073-015-0222-7
Blahna, M. T., Zalewski, C. A., Reuer, J., Kahlmeter, G., Foxman, B., and Marrs, C. F. (2006). The role of horizontal gene transfer in the spread of trimethoprim-sulfamethoxazole resistance among uropathogenic Escherichia coli in Europe and Canada. J. Antimicrob. Chemother. 57, 666–672. doi: 10.1093/jac/dkl020
Cury, J., Jov,é, T., Touchon, M., Néron, B., and Rocha, E. P. C. (2016). Identification and analysis of integrons and cassette arrays in bacterial genomes. Nucleic Acids Res. 44, 4539–4550. doi: 10.1093/nar/gkw319
Dahmen, S., Mansour, W., Boujaafar, N., Arlet, G., and Bouallègue, O. (2010). Distribution of Cotrimoxazole Resistance Genes Associated with Class 1 Integrons in Clinical Isolates of Enterobacteriaceae in a University Hospital in Tunisia. Microb. Drug Resist. 16, 43–47. doi: 10.1089/mdr.2009.0091
de los Santos, E., Laviña, M., and Poey, M. E. (2021). Strict relationship between class 1 integrons and resistance to sulfamethoxazole in Escherichia coli. Microb. Pathog. 161:105206. doi: 10.1016/j.micpath.2021.105206
Deng, Y., Bao, X., Ji, L., Chen, L., Liu, J., Miao, J., et al. (2015). Resistance integrons: class 1, 2 and 3 integrons. Ann. Clin. Microbiol. Antimicrob. 14:45. doi: 10.1186/s12941-015-0100-6
Domingues, S., da Silva, G. J., and Nielsen, K. M. (2015). Global dissemination patterns of common gene cassette arrays in class 1 integrons. Microbiology 161, 1313–1337. doi: 10.1099/mic.0.000099
Elias, P., Barraud, O., Hamel, C. E., Chainier, D., Dallochio, A., Grélaud, C., et al. (2022). Integron detection for prediction of trimethoprim/sulfamethoxazole susceptibility in children with Enterobacterales urinary tract infections. J. Antimicrob. Chemother. 77, 767–770. doi: 10.1093/jac/dkab431
Fleming, M. P., Datta, N., and Grüneberg, R. N. (1972). Trimethoprim Resistance determined by R Factors. Br. Med. J. 1, 726–728. doi: 10.1136/bmj.1.5802.726
Francia, M. A., Varsaki, A., Garcillán-Barcia, M. P., Latorre, A., Drainas, C., and de la Cruz, F. (2004). A classification scheme for mobilization regions of bacterial plasmids. FEMS Microbiol. Rev. 28, 79–100. doi: 10.1016/j.femsre.2003.09.001
Green, J. M., and Matthews, R. G. (2007). Folate biosynthesis, reduction, and polyglutamylation and the interconversion of folate derivatives. EcoSal Plus 2:2. doi: 10.1128/ecosalplus.3.6.3.6
Hall, R. M. (2012). Integrons and gene cassettes: hotspots of diversity in bacterial genomes. Ann. N.Y. Acad. Sci. 1267, 71–78. doi: 10.1111/j.1749-6632.2012.06588.x
Huovinen, P. (1997). Increases in rates of resistance to trimethoprim. Clin. Infect. Dis. 24, S63–S66. doi: 10.1093/clinids/24.Supplement_1.S63
Labbate, M., Case, R. J., and Stokes, H. W. (2009). The integron/gene cassette system: an active player in bacterial adaptation. Methods Mol. Biol. 532, 103–125. doi: 10.1007/978-1-60327-853-9_6
Manyahi, J., Tellevik, M. G., Ndugulile, F., Moyo, S. J., Langeland, N., and Blomberg, B. (2017). Molecular characterization of cotrimoxazole resistance genes and their associated integrons in clinical isolates of gram-negative bacteria from tanzania. Microb. Drug Resist. 23, 37–43. doi: 10.1089/mdr.2016.0074
Márquez, C., Labbate, M., Raymond, C., Fernández, J., Gestal, A. M., Holley, M., et al. (2008). Urinary tract infections in a south american population: dynamic spread of class 1 integrons and multidrug resistance by homologous and site-specific recombination. J. Clin. Microbiol. 46, 3417–3425. doi: 10.1128/JCM.00835-08
Miranda, A., Ávila, B., Díaz, P., Rivas, L., Bravo, K., Astudillo, J., et al. (2016). Emergence of plasmid-borne dfrA14 trimethoprim resistance gene in Shigella sonnei. Front. Cell. Infect. Microbiol. 6:77. doi: 10.3389/fcimb.2016.00077
Ojo, K. K., Kehrenberg, C., Schwarz, S., and Odelola, H. A. (2002). Identification of a complete dfrA14 gene cassette integrated at a secondary site in a resistance plasmid of uropathogenic Escherichia coli from Nigeria. Antimicrob. Agents Chemother. 46, 2054–2055. doi: 10.1128/AAC.46.6.2054-2055.2002
Olson, R. D., Assaf, R., Brettin, T., Conrad, N., Cucinell, C., Davis, J. J., et al. (2023). Introducing the bacterial and viral bioinformatics resource center (BV-BRC): a resource combining PATRIC, IRD and ViPR. Nucleic Acids Res. 51, D678–D689. doi: 10.1093/nar/gkac1003
Patridge, S. R., Tsafnat, G., Coiera, E., and Iredell, J. R. (2009). Gene cassettes and cassette arrays in mobile resistance integrons. FEMS Microbiol. Rev. 33, 757–784. doi: 10.1111/j.1574-6976.2009.00175.x
Peters, J. E. (2014). Tn7. Microbiol. Spectr. 2, 647–667. doi: 10.1128/microbiolspec.MDNA3-0010-2014
Poey, M. E., Albini, M., Saona, G., and Laviña, M. (2012). Virulence profiles in uropathogenic Escherichia coli isolated from pregnant women and children with urinary tract abnormalities. Microb. Pathog. 52, 292–301. doi: 10.1016/j.micpath.2012.02.006
Poey, M. E., and Laviña, M. (2014). Integrons in uropathogenic Escherichia coli and their relationship with phylogeny and virulence. Microb. Pathog. 77, 73–77. doi: 10.1016/j.micpath.2014.11.002
Poey, M. E., and Laviña, M. (2018). Horizontal transfer of class 1 integrons from uropathogenic Escherichia coli to E. coli K12. Microb. Pathog. 117, 16–22. doi: 10.1016/j.micpath.2018.02.006
Popowska, M., and Krawczyk-Balska, A. (2013). Broad-host-range IncP-1 plasmids and their resistance potential. Front. Microbiol. 4:44. doi: 10.3389/fmicb.2013.00044
Sambrook, J., Fritsch, E. F., and Manitis, T. (1989). Molecular Cloning: A Laboratory Manual, second ed. New York: Cold Spring Harbor Laboratory Press.
Seemann, T. (2014). Prokka: rapid prokaryotic genome annotation. Bioinformatics 30, 2068–2069. doi: 10.1093/bioinformatics/btu153
Šeputiene, V., Povilonis, J., RuŽauskas, M., Pavilonis, A., and SuŽiedeliene, E. (2010). Prevalence of trimethoprim resistance genes in Escherichia coli isolates of human and animal origin in Lithuania. J. Med. Microbiol. 59, 315–322. doi: 10.1099/jmm.0.015008-0
Sköld, O. (2001). Resistance to trimethoprim and sulfonamides. Vet. Res. 32, 261–273. doi: 10.1051/vetres:2001123
Su, J., Shi, L., Yang, L., Xiao, Z., Li, X., and Yamasaki, S. (2006). Analysis of integrons in clinical isolates of Escherichia coli in China during the last six years. FEMS Microbiol. Lett. 254, 75–80. doi: 10.1111/j.1574-6968.2005.00025.x
Sundström, L., Jansson, C., Bremer, K., Heikkilä, E., Olsson-Liljequist, B., and Sköld, O. (1995). A new dhfrVIII trimethoprim-resistance gene, flanked by IS26, whose product is remote from other dihydrofolate reductases in parsimony analysis. Gene 154, 7–14. doi: 10.1016/0378-1119(94)00905-8
Sütterlin, S., Bray, J. E., Maiden, M. C. J., and Tano, E. (2020). Distribution of class 1 integrons in historic and contemporary collections of human pathogenic Escherichia coli. PLoS ONE 15:e0233315. doi: 10.1371/journal.pone.0233315
Toleman, M. A., Bennett, P. M., and Walsh, T. R. (2006). ISCR elements: novel gene-capturing systems of the 21st Century? Microbiol. Mol. Biol. Rev. 70, 296–316. doi: 10.1128/MMBR.00048-05
World Health Organization (2023). Model List of Essential Medicines (20th List). Available online at: https://www.who.int/publications/i/item/WHO-MHP-HPS-EML-2023.02
Yau, S., Liu, X., Djordjevic, S. P., and Hall, R. M. (2010). RSF1010-like plasmids in Australian Salmonella enterica serovar Typhimurium and origin of their sul2-strA-strB antibiotic resistance gene cluster. Microb. Drug Resist. 16, 249–252. doi: 10.1089/mdr.2010.0033
Yu, H. S., Lee, J. C., Kang, H. Y., Ro, D. W., Chung, J. Y., Jeong, Y. S., et al. (2003). Changes in gene cassettes of class 1 integrons among Escherichia coli isolates from urine specimens collected in Korea during the last two decades. J. Clin. Microbiol. 41, 5429–5433. doi: 10.1128/JCM.41.12.5429-5433.2003
Keywords: antibiotic resistance, trimethoprim, cotrimoxazole, integrons, gene cassettes, transposons, plasmid transfer, Escherichia coli
Citation: Poey ME, de los Santos E, Aznarez D, García-Laviña CX and Laviña M (2024) Genetics of resistance to trimethoprim in cotrimoxazole resistant uropathogenic Escherichia coli: integrons, transposons, and single gene cassettes. Front. Microbiol. 15:1395953. doi: 10.3389/fmicb.2024.1395953
Received: 04 March 2024; Accepted: 27 May 2024;
Published: 12 June 2024.
Edited by:
Jean-Philippe Rasigade, Université Claude Bernard Lyon 1, FranceReviewed by:
Natacha Lenuzza, Institut National de la Santé et de la Recherche Médicale (INSERM), FranceCatherine M. Logue, University of Georgia, United States
Saskia Camille Flament Simon, University of Santiago de Compostela, Spain
Copyright © 2024 Poey, de los Santos, Aznarez, García-Laviña and Laviña. This is an open-access article distributed under the terms of the Creative Commons Attribution License (CC BY). The use, distribution or reproduction in other forums is permitted, provided the original author(s) and the copyright owner(s) are credited and that the original publication in this journal is cited, in accordance with accepted academic practice. No use, distribution or reproduction is permitted which does not comply with these terms.
*Correspondence: Magela Laviña, bWFnZWxhQGZjaWVuLmVkdS51eQ==