- 1School of Energy and Environment Science, Yunnan Normal University, Kunming, China
- 2Key Laboratory of Solar Heating and Cooling Technology of Yunnan Provincial Universities, Kunming, China
- 3Faculty of Modern Agricultural Engineering, Kunming University of Science and Technology, Kunming, China
Soil copper (Cu) pollution is a serious environmental risk in the Panax notoginseng planting area. However, the effect of Cu on soil microbial metabolism and nutrient cycling in this area remains unknown. Therefore, Biolog ECO-plate and enzyme stoichiometry methods were utilized in this study to investigate the impact of exogenous Cu (control: 0 mg·kg−1; Cu100: 100 mg·kg−1; Cu400: 400 mg·kg−1; and Cu600: 600 mg·kg−1) on the metabolic function of soil microbial and nutrient limitation in the P. notoginseng soil. The results indicated that Cu100 significantly increased soil organic carbon (SOC), total phosphorus (TP), soil C:N, microbial biomass carbon (MBC), and microbial biomass nitrogen (MBN) 9.89%, 15.65%, 17.91%, 61.87%, and 90.56% higher than the control, respectively. Moreover, the carbon source utilization ratio of carbohydrates, amino acids, and amphiphilic compounds of Cu100 also increased by 7.16%, 25.47%, and 84.68%, respectively, compared with the control. The activities of β-1,4-glucosidase, cellobiohyrolase, leucine amino peptidase, β-1,4-N-acetylglucosaminidase, and phosphatase significantly decreased with increasing Cu concentration. Soil enzyme stoichiometry showed that all treatments were limited by nitrogen (vector angle < 45°; 19.045–22.081). Cu600 led to the lowest carbon limitation (1.798) and highest carbon use efficiency (CUE:0.267). The PLS-SEM model also showed that MBC, MBN, MBP, and microbial diversity positively affected carbon and nitrogen limitation (0.654 and 0.424). Soil carbon, nitrogen, phosphorus, stoichiometric ratio, MBC, MBN, and MBP positively affected CUE (0.527 and 0.589). The microbial diversity index significantly negatively affected CUE (−1.490). Multiple linear stepwise regression analyses showed that CUE was mainly influenced by MBC, AP, C:P, and LAP. Thus, P. notoginseng soil can benefit soil microbial carbon and nitrogen limitations at low Cu concentrations. Clarifying the metabolic activity and nutritional status of microorganisms under Cu stress can provide some theoretical basis for realizing China's comprehensive and effective management and control policies for environmental risks from metals by 2035.
1 Introduction
Soil heavy metal pollution is one of the most serious environmental issues, exposing severe implications for human health, ecosystem function, and food security (Peng et al., 2022). In China, heavy metal contamination in basic farmland conservation area has been reported to exceed standards by as much as 16.1% (Wen et al., 2022). Wenshan, China, serves as a primary production area for Panax notoginseng and is also renowned for its mining activities (Li et al., 2014; Ou et al., 2016). Unfortunately, the soil in this region contains various heavy metals, including copper (Cu), cadmium (Cd), chromium (Cr), arsenic (As), and mercury (Hg), which often surpass China's soil environmental quality standards (Huang et al., 2023). The unregulated expansion of mining areas and the widespread use of heavy-metal pesticides have exacerbated heavy-metal pollution (Duan et al., 2015; Wu et al., 2022). Consequently, this situation poses critical threats to soil quality in such regions and the safety of P. notoginseng products bound for human consumption (Duan et al., 2015). Of those heavy metals, Cu pollution is in the soil of the highly coveted and irreplaceable planting regions (Zeng et al., 2009). Recent studies reveal that soil Cu content in the soil of P. notoginseng planting areas in Wenshan County ranges from 29.16 to 285.48 mg·kg−1 (Zu et al., 2017), which exceeds 49.9% of the standard limits set in the “Soil Environmental Quality Risk control standard for soil contamination of agricultural land (GB 15618, 2018)” of China, which specifies a limit of 100 mg·kg−1 for soil Cu (GB 15618, 2018).
Cu is an essential trace element for crop growth and microbial development (Gilbert, 1952). At optimal concentrations, it stimulates soil microbial growth and enhances the abundance of soil microbial communities (Mir et al., 2021). However, exceeding a certain Cu concentration threshold can negatively impact photosynthesis, microbial activities, enzyme function, and antioxidant systems, ultimately affecting crop growth and altering the abundance, structure, and function of microbial communities (Gong et al., 2019; Karimi et al., 2021).
Microorganisms play a crucial role in nutrient cycling and the transformation of organic matter within soil systems (Coonan et al., 2020; Philippot et al., 2023). Under heavy metal stress, soil microorganisms divert the energy used for community expansion to maintain cell function (Duan et al., 2022). In addition, the toxicity of heavy metals reduces the energy utilization efficiency of microbial metabolism, and more carbon sources are required to maintain microbial activity, thereby reducing microbial biomass (Graham and Haynes, 2005). Notably, soil Cu pollution inhibits enzyme activity, disrupting the cyclic transformation of major nutrients (Asensio et al., 2013). The roots of Panax notoginseng secrete various root exudates, including sugars, carboxylic acids, and amino acids, into the soil. These exudates can alter the balance of the P. notoginseng soil microbial community (Zhang et al., 2019; Zhao et al., 2023). Long-term cultivation of P. notoginseng has been observed to shift the dominant microbial community from bacteria to fungi (Zhang et al., 2019). Additionally, soil microorganisms are also affected by heavy metals in the soil. Research on heavy metal pollution in P. notoginseng planting soil primarily focuses on the present polluted status investigation, plant absorption and accumulation, and human health risk assessments. The specific impacts of Cu stress on nutrient cycling and carbon metabolism on P. notoginseng soil remain largely unexplored and warrant further investigation.
Recent studies have highlighted the importance of extracellular enzyme stoichiometry (C:N:P) as an effective measure for evaluating soil microbial energy and nutrient limitations (Kumar and Ghoshal, 2017; McGee et al., 2019). Furthermore, carbon use efficiency (CUE) provides valuable insights into variations in microbial abundance, the rate of soil carbon storage turnover, and the intensity and activity of microbial metabolism (Sinsabaugh et al., 2013; Mooshammer et al., 2017; Domeignoz-Horta et al., 2020). To address these gaps, our study utilizes BIOLOG technology to examine the carbon source utilization metabolic characteristics of soil microorganisms in P. notoginseng plantation soil under exogenous Cu stress. We also explore the effects of Cu on carbon, nitrogen, and phosphorus limitations in soil microbes. Ultimately, this research aims to establish a theoretical foundation for ecological risk assessment and microbial early warning systems related to Cu pollution in P. notoginseng plantation soil.
2 Materials and methods
2.1 Experimental site and soil sampling
The experimental soil was collected from the Miaoxiang Panax notoginseng Scientific Experimental Site in Wenshan Prefecture, Yunnan Province, China (23°31′44″N, 104°19′13′″E). The region experiences a subtropical monsoon climate with an average annual temperature of 16.6°C and annual cumulative rainfall of 1,111 mm. The soil in this study was yellowish-brown lateritic soil developed from tertiary igneous rocks. On June 5, 2022, soil samples were cored from plots where P. notoginseng had been planted for over 2 years. Three random plots, spaced 10 meters apart (each measuring 0.5 m × 0.5 m), were selected. The top 1 cm of surface soil was removed, and soil from 1 to 20 cm depth was cored. The collected soil samples were homogenized and sieved through a 2 mm mesh to remove impurities. A fraction of the sample was kept under cold storage at 4°C for further analysis of extracellular enzyme activity, microbial carbon source metabolism, microbial biomass carbon, nitrogen, and phosphorus. Another portion of the samples was used to evaluate the fundamental physicochemical properties after air drying. Soil bulk density samples were also collected, and each sampling site was repeated three times. The total Cu content of the background soil was 26.07 mg·kg−1.
2.2 Experimental design
An incubation experiment including three exogenous Cu treatments (Cu100, Cu400, and Cu600) and a control was designed. Exogenous Cu was not added to the control. The CuSO4 solution was added to the soil to obtain total Cu concentrations of 100 mg·kg−1 for the Cu100 treatment, 400 mg·kg−1 for the Cu400 treatment, and 600 mg·kg−1 for the Cu600 treatment. The homogenized soil samples were placed in plastic pots and sealed using a fibrous membrane to ensure adequate ventilation while minimizing moisture evaporation. Incubation conditions were maintained at a constant temperature of 25°C and a consistent water content of 25%. The fibrous membrane on the top of the pots was removed after 7 days, and 1-year-old P. notoginseng seedlings were transplanted into the pots. Three seedlings were planted in each pot. Following a 30-day incubation period, the soil microbial properties were analyzed, and seedling survival rates were investigated.
2.3 Soil testing methods
Soil bulk density was determined using the ring knife method. Soil pH was measured using an electrode method with a soil-water ratio of 1:2.5 (Rayment and Higginson, 1992). The soil microbial biomass carbon and nitrogen content was also determined using the chloroform fumigation-potassium sulfate extraction method. The microbial biomass phosphorus was estimated using the chloroform fumigation-sodium bicarbonate extraction method. The SOC was quantified using the K2Cr2O7-FeSO4 titration method (Vance et al., 1987), while the total nitrogen was analyzed by H2SO4-HClO4 digestion followed by automated intermittent element analysis (AMS-Alliance) (Khan et al., 2001). The total phosphorus in the soil was measured utilizing the H2SO4-HClO4 digestion-molybdenum antimony anti-colorimetric method (Olsen, 1954). Furthermore, ammonium nitrogen and nitrate nitrogen were determined through the colorimetric method using a flow analyzer (SEAL Analytical) (Khan et al., 2001), while available phosphorus was estimated via the molybdenum antimony anti-colorimetric method (Olsen, 1954). The seedling survival rate of Panax notoginseng was at 75% of control, 87.5% of T1, 62.5% of T2, and 62.5% of T3. The results of the basic physical and chemical properties of the soil are shown in Table 1.
The BIOLOG-ECO plate method was employed to evaluate soil microbial carbon metabolism with 31 distinct carbon sources, each with three replicated samples. Samples of 10 g of soil were suspended in 90 ml of an aseptic of 0.85% NaCl solution and subjected to agitation at 180 rpm in a constant-temperature shaker for 30 min. After static incubation for 15 min, the supernatants were collected and subjected to subsequent continuous dilution procedures. A diluted solution that had been adjusted to a 10−3 times concentration was used to inoculate the BIOLOG-ECO microplates, which were then placed at 28°C for an incubation period of 10 days. The absorbance level (OD) was measured at 590 nm using a spectrophotometer (SPECTROstar Nano) every 24 h.
Soil extracellular enzyme activities were determined using fluorometric measurements (Bell et al., 2013), and the activities of five extracellular enzymes were measured. The enzyme species were β-1, 4-glucosidase (BG), cellobiohydrolase (CBH), leucine amino peptidase (LAP), β-1, 4-N-acetylglucosaminidase (NAG), and phosphatase (AP). The substrates were 4-methylumbelliferyl-β-D-glucoside, 4-methylumbelliferyl-β-D-cellobioside, L-leucine-7-amido-4-methylcoumarin hydrochloride, 4-methylumbelliferyl-N-acetyl-β-D-glucosaminide, and 4-methylumbelliferyl-phosphate, respectively. The fluorescence spectrophotometer was used to determine the excitation wavelength at 365 nm and the emission wavelength at 450 nm.
2.4 Statistical analysis
The average well color development (AWCD), which represents the capacity of culturable microbes to consume various substrates (carbon sources), is used to depict the metabolic activity of the soil microbial population. The AWCD was calculated by Equation (1) follows (Garland, 1996):
where AWCD means average well color development, is the absorbance at 590 nm of each reaction well, Ci represents the absorbance values of the 31 carbon-containing wells on the BIOLOG-ECO plate, and r is the comparable absorbance of the control well (water in the control well); negative (Ci-r) values were set to zero.
Soil microbial community richness index S is expressed by the number of reactive wells (absorbance value > 0.25, meaning the well's carbon source is absorbed and utilized, and the well is a reactive pore).
The Shannon-Wiener index (H) of the soil microbial community represents the functional diversity of the microbial community. The H parameter is given as follows Equation (2) (Garland, 1996):
where Pi = (Ci – r)/∑(Ci – r). Pi represents the ratio of the relative absorbance value of the i-th well on the BIOLOG-ECO plate to the sum of the average relative absorbance values across all wells on the plate.
The soil microbial community evenness index Pielou (E) is expressed as the ratio of the Shannon-Wiener index of the microbial community to the logarithm of the richness index. The Pielou (E) is given using Equation (3):
where S is the richness index.
The Simpson index (D) of soil microbial communities, also known as the dominance index, is a measure of concentration in terms of diversity. The Simpson index (D) can be calculated using Equation (4) as follows:
The EEA is expressed in nmol·(g·h)−1. However, the enzyme stoichiometry ratios of EEAC/N, EEAC/P, and EEAN/P are calculated using [(BG + CBH)/(NAG + LAP)], [(BG + CBH)/AP], and [(NAG + LAP)/AP], respectively. The vector lengths (carbon limitation) and angles (vector angle < 45° for nitrogen limitation and >45° for phosphorus limitation) were calculated for all treatments (Deng et al., 2019). The vector length is the square root of the sum of the squares of x and y (Equation 5). Where x represents the relative activity of C (BG and CBH) and P (AP) to acquire enzymes, and y represents the relative activity of C and N (NAG and LAP) to acquire enzymes. The vector length and angle of the enzyme stoichiometry ratio were used to quantify the extent of microbial carbon, nitrogen, or phosphorus limitation. A longer vector length indicates a greater degree of carbon limitation, while vector angles < 45° and >45° represent nitrogen and phosphorus limitation, respectively, with a stronger extent of limitation indicated by a larger deviation (Deng et al., 2019).
Where the vector angle is the arctangent of the straight line from the origin to point (x, y) and can be defined using Equation (6).
The carbon use efficiency (CUE) is given using Equation (7) (Sinsabaugh et al., 2013):
Where SC:N = BC:N/LC:N × 1/EEAC:N, SC:P = BC:P/LC:P × 1/EEAC:P, LC:N is the SOC: TN, LC:P is the SOC: TP, BC:N is the MBC: MBN, and BC:P is the MBC: MBP. KC:N and KC:P are the half-saturation constants of CUE based on the availability of C, N, and P. The model assumes that the maximum growth rate occurs when the proportion of absorbable nutrients supply matches the microbial biomass stoichiometry and the growth efficiency is proportional to the geometric mean ratio of N and P supply relative to C. It is assumed that all models' KC:N and KC:P are 0.5, and CUEmax is 0.6 (Sinsabaugh et al., 2013).
One-way analysis of variance (ANOVA) was used with Duncan's test to evaluate significant differences (p < 0.05) in soil among different Cu concentrations. The final data were expressed as means ± standard deviation. Multiple linear stepwise regression analysis and model establishment were performed by SPSS 18.0. The absorbance values at 168 h of culturing period were selected to analyze carbon source metabolism characteristics and soil microbial diversity indices. Graphs were introduced by OriginPro2021. RDA analysis and corresponding graphs were conducted using Canoco5. Smartpls4 was employed to select a PLS-SEM model to assess further potential pathways involved in carbon-nitrogen limitation and carbon utilization efficiency.
3 Results
3.1 Effect of exogenous copper on basic physicochemical properties of soil
Table 2 presents the basic physical and chemical properties of the soil. Except for total nitrogen, Cu addition significantly impacted microbial biomass carbon (MBC), microbial biomass nitrogen (MBN), and microbial biomass phosphorus (MBP). Specifically, in the Cu100 treatment, soil organic carbon (SOC), total phosphorus (TP), MBC, MBN, and the C:N ratio were significantly higher than those in the control and other treatments (p < 0.05). However, the soil C:P and N:P ratios in Cu100 (39.95 ± 1.99 and 3.25 ± 0.24, respectively) were the lowest among all treatments. The highest soil C:P and N:P ratios were observed in the Cu600 treatment, with soil C:P ratios at 47.50 and N:P ratios at 4.34.
3.2 The effects of exogenous copper on soil microbial metabolic activity and carbon source utilization characteristics
The average well color development (AWCD) values for the control and Cu100 treatments increased rapidly after 24 h of cultivation (Figure 1A). However, the increase in the Cu100 treatment was lower than that in the control. In the Cu400 and Cu600 treatments, AWCD values increased rapidly after 72 h, and microbial utilization of carbon sources across all treatments stabilized after 168 h. Variance analysis revealed that the AWCD value of the control was significantly higher than that of other treatments starting from 72 h (p < 0.05), with increases of 53.6%, 352.7%, and 467.6% compared to Cu100, Cu400, and Cu600, respectively.
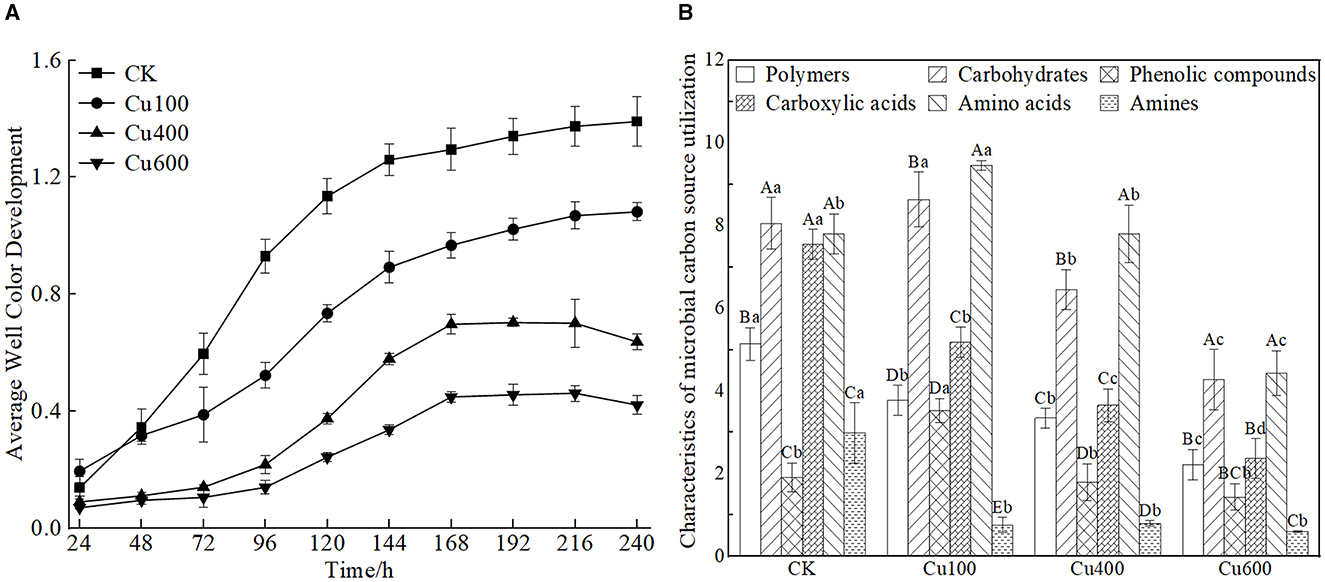
Figure 1. Changes of AWCD value of soil microbial carbon sources (A) and utilization characteristics of six types of carbon sources under Cu treatment (B). In (B), different uppercase letters indicate significant differences in different types of carbon sources in the same treatment group, and different lowercase letters indicate significant differences between groups of the same type of carbon sources (p < 0.05).
The relative utilization rate of different carbon sources by the soil microbial community in Cu-treated soil differed significantly from that in the control (Figure 1B). Overall, soil microbes exhibited the following trend in their ability to utilize major carbon sources: amino acids > carbohydrates > carboxylic acids > polymers > hydrophobic compounds > amines. The Cu100 treatment exhibited the highest utilization rates of carbohydrates (8.631 ± 0.663) and amino acids (9.457 ± 0.116), with increases of 7.16 and 25.5% compared to the control, respectively. Conversely, the utilization rate of hydrophobic compounds in the Cu100 treatment (3.533 ± 0.288) was significantly higher than that in other treatments (p < 0.05). Carboxylic acid and polymer carbon source utilization by soil microbes decreased significantly with increasing soil Cu concentration (p <0.05).
3.3 Effects of exogenous copper on microbial community structure diversity
The richness index (S) of soil microbes in the control (41.90) was significantly higher than that in the other treatments (Table 3). As exogenous Cu concentration increased, the richness index decreased significantly from 30.68 in the Cu100 to 13.06 in the Cu600 treatment. However, the Shannon-Wiener index did not differ significantly among treatments, with an average value of 3.13. The Simpson index of the Cu600 treatment (0.94) was lower than that of the control (0.96) and Cu100 treatments (0.96) but did not show a significant difference with that of Cu400. Pielou's evenness index (E) was significantly higher in the Cu400 and Cu600 treatments compared to the Control and Cu100 treatments (p < 0.05).
3.4 Effects of soil extracellular enzyme activity and enzymatic stoichiometry
The extracellular enzyme activity (EEAs) in the soil treated with exogenous Cu was significantly lower than that in the control (p < 0.05; Figure 2A). This decrease became more pronounced with increasing Cu concentration. Among all treatments, the Cu600 treatment exhibited the lowest EEAs, accounting for 0.88%−2.40% of the EEAs in the control. Notably, the activities of carbon acquisition enzymes (BG and CBH) and nitrogen acquisition enzymes (NAG and LAP) were higher than those of phosphorus acquisition enzymes (AP) in all treatments (Figure 2A).
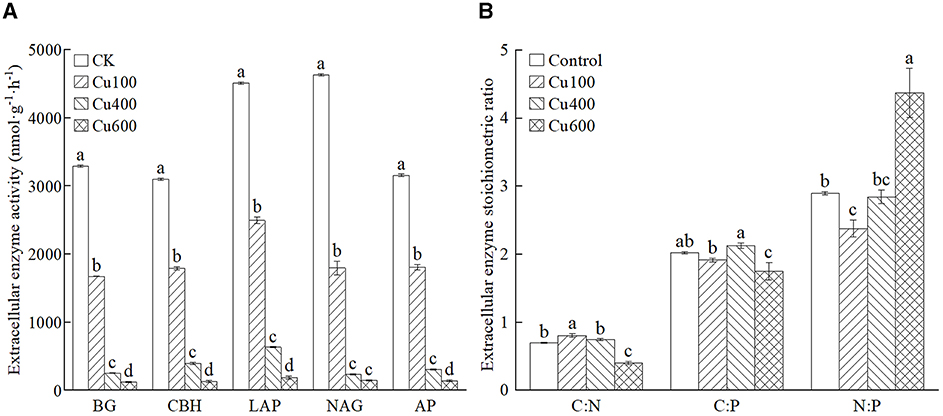
Figure 2. (A, B) Extracellular enzyme activity and stoichiometry ratio of different treatments. Different letters in the same column indicate significant differences between treatments using ANOVA at p < 0.05.
The C:N of extracellular enzymatic stoichiometry ratios in all treatments were in the range of 0.402–0.808, C:P was 1.753–2.127, and N:P was 2.377–4.374 (Figure 2B). Both C:P and N:P ratios exceeded 1 in all treatments. The addition of exogenous Cu led to variations in these ratios. Specifically, the Cu100 treatment promoted a significantly higher C:N ratio (0.878) compared to other treatments (p < 0.05). The Cu400 treatment exhibited the highest C:P ratio (2.127), significantly surpassing that of the Cu600 treatment (p < 0.05). Conversely, the Cu600 treatment resulted in a significantly higher N:P ratio (4.374) than other treatments.
3.5 Effects of ecological enzymatic stoichiometric vectors and carbon use efficiency
The vector length of the Cu400 treatment was significantly higher than that of the Cu100 and Cu600 treatment (p < 0.05). Specifically, Cu100 and Cu600 decreased by 2.94 and 16.10%, respectively, compared to the control; Cu400 increased by 5.23% compared to the control (Table 4). The enzyme stoichiometric vector analysis revealed that all treatments had vector angles <45°, indicating nitrogen limitation for soil microbes in P. notoginseng planting soil. Notably, the vector angle of the Cu100 treatment was significantly higher (15.9%) than that of the control treatment (p < 0.05). Conversely, the vector angle of the Cu600 treatment was significantly lower (32.0%) than that of the control treatment (p < 0.05). The carbon use efficiency value for treatments with exogenous Cu was higher by 0.021–0.058 than the control. Specifically, Cu100, Cu400, and Cu600 treatments exhibited CUE values 27.3%, 10.05%, and 27.8% higher than the control (p < 0.05), respectively.
3.6 RDA analysis of the effects of exogenous copper on soil microbial carbon and nitrogen limitation and carbon use efficiency
The RDA analysis was used to analyze the effects of exogenous Cu on soil microbial carbon and nitrogen limitation, as well as carbon use efficiency (Figure 3). The RDA1 and RDA2 accounted for 94.16% of the variation in extracellular enzyme activities (BG, CBH, LAP, NAG, and AP), CUE, and the other selected variations. The results showed the carbon limitation (vector length) was mainly and positively influenced by extracellular enzyme activity, pH, and MBP. The nitrogen limitation (vector angle) was mainly and positively influenced by extracellular enzyme activity, MBC, MBN, MBP, TP, C:N, pH, and SOC, but negatively affected by TN, C:P, and N:P. The CUE was negatively affected by pH, MBP, and EEA factors. Moreover, the CUE value was negatively correlated with carbon limitation and not with nitrogen limitation due to the right angle. In summary, the results of the RDA analysis indicated that environmental factors play a vital role in adjusting soil microbial functions and nutrient cycling processes in P. notoginseng planting soil. Specifically, EEAs, pH, MBP, TN, C:N, and N:P ratios contribute to differences in carbon and nitrogen limitation. Nevertheless, pH, MBP, and EEAs exhibit detrimental impacts on the CUE.
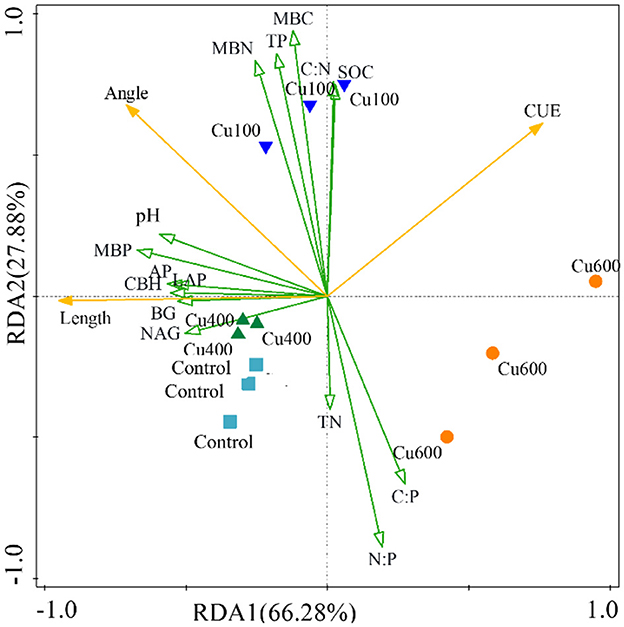
Figure 3. Redundant analysis of carbon and nitrogen limits and CUE by environmental factors under different treatments.
3.7 The effects of soil environmental factors on carbon and nitrogen limitations and CUE
Partial Least Squares Structural Equation Modeling (PLS-SEM) was used to further analyze the effects of soil microbial biomass, environmental factor variables, EEAs, and microbial diversity indices on soil carbon and nitrogen limitations, as well as CUE (Figure 4). The analysis demonstrated that MBC, MBN, MBP, and microbial diversity exhibited substantial positive contributions to carbon and nitrogen constraints with path coefficients of 0.654 and 0.424, respectively. In contrast, extracellular enzyme activity negatively affected carbon and nitrogen constraints (−0.448), while soil SOC, TN, TP, and their stoichiometric ratios exerted a minor negative effect on carbon and nitrogen constraints (−0.299). MBC, MBN, MBP, and soil SOC, TN, TP, and their stoichiometric ratios presented positive effects on CUE with the path coefficients 0.589 and 0.527, respectively. However, microbial diversity had a negative impact on CUE with a path coefficient of −1.490, and extracellular enzyme activity contributed to a slightly positive impact on CUE with a path coefficient of 0.234. Multiple linear stepwise regression analysis (Table 5) showed that MBC, AP, C:P, and LAP could explain 98.2% of the variation of CUE, Pielou index could only explain 29.6% of the variation of carbon limitation, and MBN could explain 62.9% of the variation of nitrogen limitation.
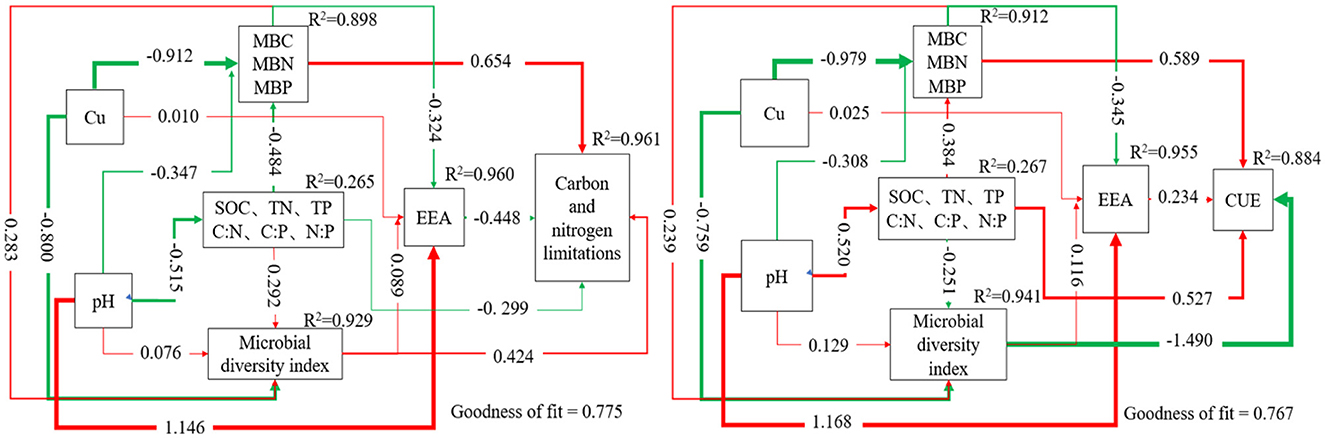
Figure 4. Partial least squares path modeling model. PLS-SEM path modeling shows the direct and indirect effects of Cu, soil physical and chemical properties, microbial biomass, microbial diversity, and extracellular enzymes on soil carbon and nitrogen limitation, CUE, and utilization of different types of carbon sources. The red and green lines represent positive and negative causal relationships. The numbers on the arrows indicate the standardized path coefficients; R2 represents the variance of the dependent variables explained by the model.
4 Discussion
4.1 Impact of exogenous copper on carbon metabolism
Previous studies have demonstrated that soil microbial communities experience reduced functional diversity when exposed to heavy metal contamination, such as that from mine tailings (Song et al., 2017). Additionally, the number of microbes utilizing carbon as an energy source decreases, along with their ability to efficiently use carbon substrates (Teng et al., 2008; Song et al., 2017). Our study aligns with these findings, as we observed decreased metabolic activity, reduced carbon source utilization capacity, and alterations in richness (S), Shannon index (H), and Simpson index (D) under Cu stress. Copper inhibits the ability of microorganisms to secrete extracellular enzymes, leading to a diminished ability to acquire essential resources. Consequently, this reduction in enzymatic activity results in decreased metabolic efficiency and carbon source utilization by microorganisms, as evidenced by ecological enzyme data. Specifically, the activities of BG, CBH, LAP, NAG, and AP were decreased with the increase of exogenous Cu content (Figure 2A). Compared with other carbon sources, the carbon source utilization of carbohydrates and amino acids was higher in each heavy metal treatment (Figure 1B). Moreover, the ability of microorganisms to use carbon sources was decreased in high Cu concentration (Cu600). This might be because the microbes need to consume more energy to maintain their normal life activities under heavy metal stress, leading to an increase in energy/carbon consumption, as reported by Xu et al. (2021) and Tao et al. (2022). Overall, heavy metal stress disrupts the diversity and carbon metabolism function of soil microbial communities (Song et al., 2017), resulting in a simplified microbial structure with a singular function (Teng et al., 2008; Wang et al., 2023). In soil with high concentrations of heavy metal pollution, certain resilient microbes become the dominant flora community (Teng et al., 2008; Qi et al., 2022).
4.2 Copper induces carbon and nitrogen limitation
Nitrogen limitation is widespread in terrestrial soils (Lebauer and Treseder, 2008). A lower soil C:N ratio will lead to microbes experiencing greater constraints from carbon availability than nitrogen availability, particularly when the ratio falls below 13–15 (Hodge et al., 2000; Cheng et al., 2019). Our study revealed that the C:N ratios of the selected P. notoginseng cultivation soils were all under 13 (as shown in Figure 2B and Table 4). Also, it was observed that the soil microbiota in P. notoginseng planting areas were concurrently limited by both carbon and nitrogen (as showed in Table 4). The microbial communities in the ecosystem may transit from N limitation (C saturation) to C limitation (N saturation) (Kopáček et al., 2013). Consequently, when nitrogen limitation is mitigated at low Cu concentrations (<100 mg·kg−1), carbon limitation is also alleviated (Allison et al., 2010) at 400 mg·kg−1 Cu concentration. The increasing costs of enzyme production reduce adaptation because those resources cannot be allocated to reproduction (Hall et al., 2018). Our study also found that the Pielou index is a major factor in carbon limitation, and MBN is a major factor in nitrogen limitation (Table 5) by multiple linear stepwise regression analysis. Previous studies have shown that the resource benefits of enzyme production can be invested in reproductive effort, thereby increasing microbial fitness, which is more advantageous for soil microbes in resisting Cu-induced stress (Qi et al., 2023). During the process of gene transcription and expression, soil microbes necessitated not only phosphorus but also nitrogen, which resulted in exacerbated nitrogen limitation. This result was proved by the “Evolutionary Economic Principle” by Allison in 2010 (Allison et al., 2010).
4.3 Exogenous copper effects on CUE of P. notoginseng planting soil
The Biogeochemical Equilibrium Model was used to explore the relationship between the effectiveness of environmental nutrients, EEAs, and microbial growth efficiency. On a global scale, the enzyme activity ratio for carbon, nitrogen, and phosphorus cycling is ~1:1:1 (Sinsabaugh et al., 2009). Further, Burns et al. (2013) proposed the “resource allocation theory,” which highlights the coupling relationship between resource effectiveness in soil and the relative activity of soil enzymes. The microbes will allocate more energy (C) and nutrients (N, P) to produce limited enzymatic resources as the environmental substrate is abundant and effective resources are scarce. In our study, the enzyme activity ratios related to soil carbon, nitrogen, and phosphorus cycling were ~1:1.5:1 (control), 1:1.2:1 (Cu100), 1:1.4:1 (Cu400), and 1:2.4:1 (Cu600). The average values of 40 major terrestrial soils in the globe were 1.41 for the enzyme C:N value, 0.62 for the C:P value, and 0.44 for the N:P value (Burns et al., 2013). Besides, the enzyme C:N values for the four treatments in our experiment were found below the average values of the 40 major terrestrial soils. The enzyme C:N values for all the treatments were estimated at 0.699 in the control, 0.808 in the Cu100, 0.748 in the Cu400, and 0.402 in the Cu600. It indicated that the microbial community in P. notoginseng plantation soil was limited by carbon and nitrogen.
The CUE may be relatively high when carbon availability (i.e., energy) is limited (Blagodatskaya and Kuzyakov, 2008). Furthermore, microbes may guide carbon into overflow respiration, leading to a decrease in CUE when nutrient limitation occurs (Manzoni et al., 2012; Sinsabaugh et al., 2013). Our study identified key factors influencing CUE, including MBC, AP, C:P ratio, and LAP. The production of extracellular enzymes by microbes requires carbon, which may lead to additional respiratory costs (Cotrufo et al., 2013; Moorhead et al., 2015), ultimately reducing CUE (Malik et al., 2018). In addition, temperature and soil moisture can alter microbial metabolism, affecting the balance between growth (μ) and assimilation rates, thus impacting CUE (Sinsabaugh et al., 2016). The decrease in CUE may result from the coincident limitation of the two elements (Waring et al., 2014; Malik et al., 2018). In our study, the P. notoginseng plantation soil selected was severely limited by carbon and nitrogen, leading to a decrease in CUE. Microbes may lower their CUE to buffer against environmental pressures because maintaining the microbe's condition requires more energy (Soares and Rousk, 2019). However, the soil microbial subjected to Cu stress increased CUE due to decreased microbial quantity. Environmental factors affect the ability of soil microbial communities to obtain energy and nutrients, as well as the availability of soil nutrient forms. These results are confirmed by Qi et al.'s (2016) study, which states that unstable carbon in the soil varies continuously with temperature, affecting the activity of EEAs by controlling soil microbial decomposition rates and mineralization processes. Our study found that CUE was mainly influenced by MBC, AP, C:P, and LAP from multiple linear stepwise regression analyses.
The enzymes that obtain nutrients (N and P) are equally important for the effect of CUE. Heavy metals affect the metabolic function of soil microbes by changing the rate of decomposition and diminishing the ability of microbes to secrete extracellular enzymes (Gong et al., 2019). Therefore, their ability to acquire energy (C) and nutrients (N) from the external environment is reduced (Duan et al., 2022). According to Allison's theory (Allison et al., 2010), the increased production costs for enzymes reduced adaptability because these resources cannot be allocated to reproduction. The positive impact of microbial biomass on carbon and nitrogen limitation might be because soil microbes do not produce more extracellular enzymes, which can obtain energy (C) and nutrients (N and P) when subjected to Cu stress (Manzoni et al., 2012; Burns et al., 2013; Tao et al., 2022). This is detrimental to producing microbial Cu resistance genes to resist external stress. The increase in microbial carbon/nitrogen ratio influenced the increase of CUE (Sinsabaugh et al., 2013). The allocation of resources for nitrogen acquisition by microbes reduced CUE (Cleveland and Liptzin, 2007), which led to a positive effect from MBC, MBN, and MBP, while microbial diversity had a negative effect on CUE.
5 Conclusions
The current study revealed that soil microbes under high Cu concentration reduced metabolic activity and the diversity indices of the microbial community; however, the low Cu concentration has a stimulating effect on microbial mass and diversity (such as the Shannon and Simpson indices).
The enzyme stoichiometry analysis revealed that microbes in PNG planting soils were both subjected to carbon and nitrogen limitations. In Cu-treated soils, limitations of carbon and nitrogen were ameliorated at low concentrations (<100 mg·kg−1). Whereas high concentrations (up to 600 mg·kg−1) presented more pronounced carbon and nitrogen restrictions than the control. Under elevated Cu stress conditions, the capacity of soil microbes to obtain energy (C) and nutrients (N) was substantially impeded, consequently reducing microbial diversity. Environmental factors, including pH, MBP, and TP, significantly influence the metabolism and EEAs of soil microbial communities. Heavy metal stress can also reduce EEAs, as the increased production cost of enzymes reduces adaptability to the growth environment. PLS-SEM pathway analysis showed that soil carbon and nitrogen limitation is aggravated by soil microbial mass and microbial diversity (0.654 and 0.424), and soil element and stoichiometric ratios and EEAs relieve soil carbon and nitrogen limitation (0.527 and 0.589). Soil element stoichiometry and microbial mass had a positive effect on CUE (−1.490).
Data availability statement
The data supporting the conclusions of this article will be made available by the authors, without undue reservation. Requests to access the datasets should be directed to: ZmFuZ2xpbmcuZmFuJiN4MDAwNDA7eW5udS5lZHUuY24=.
Author contributions
TW: Software, Writing – original draft, Writing – review & editing. XW: Methodology, Writing – review & editing. TH: Writing – review & editing. XM: Resources, Writing – review & editing. HY: Writing – review & editing. ZT: Resources, Supervision, Writing – review & editing. FF: Resources, Supervision, Writing – review & editing. YH: Resources, Supervision, Writing – review & editing.
Funding
The author(s) declare financial support was received for the research, authorship, and/or publication of this article. This work was supported by the National Natural Science Foundation of China (42107256), the General Program of National Steering Committee of Agricultural Graduate Education (2021-NYYB-51), Yunnan Fundamental Research Projects (202201AT070036, 202201AU070147, and 202301AT070460), and High-level talent introduction project of Yunnan Normal University (ZX22020102).
Conflict of interest
The authors declare that the research was conducted in the absence of any commercial or financial relationships that could be construed as a potential conflict of interest.
Publisher's note
All claims expressed in this article are solely those of the authors and do not necessarily represent those of their affiliated organizations, or those of the publisher, the editors and the reviewers. Any product that may be evaluated in this article, or claim that may be made by its manufacturer, is not guaranteed or endorsed by the publisher.
Supplementary material
The Supplementary Material for this article can be found online at: https://www.frontiersin.org/articles/10.3389/fmicb.2024.1390921/full#supplementary-material
References
Allison, S. D., Weintraub, M. N., Gartner, T. B., and Waldrop, M. P. (2010). “Evolutionary-economic principles as regulators of soil enzyme production and ecosystem function,” in Soil Enzymology, eds. S. D. Allison, M. N. Weintraub, T. B. Gartner, and M. P. Waldrop (Berlin: Springer), 229–243. doi: 10.1007/978-3-642-14225-3_12
Asensio, V., Covelo, E. F., and Kandeler, E. (2013). Soil management of copper mine tailing soils–sludge amendment and tree vegetation could improve biological soil quality. Sci. Total Environ. 456–457, 82–90. doi: 10.1016/j.scitotenv.2013.03.061
Bell, C. W., Fricks, B. E., Rocca, J. D., Steinweg, J. M., Mcmahon, S. K., Wallenstein, M. D., et al. (2013). High-throughput fluorometric measurement of potential soil extracellular enzyme activities. J. Vis. Exp. 81:e50961. doi: 10.3791/50961-v
Blagodatskaya, E., and Kuzyakov, Y. (2008). Mechanisms of real and apparent priming effects and their dependence on soil microbial biomass and community structure: critical review. Biol. Fertil. Soils 45, 115–131. doi: 10.1007/s00374-008-0334-y
Burns, R., Deforest, J., Marxsen, J., Sinsabaugh, R., Stromberger, M., Wallenstein, M., et al. (2013). Soil enzymes in a changing environment: current knowledge and future directions. Soil Biol. Biochem. 58, 216–234. doi: 10.1016/j.soilbio.2012.11.009
Cheng, Y., Wang, J., Wang, J., Zhao, X., Chang, S., Cai, Z., et al. (2019). Nitrogen deposition differentially affects soil gross nitrogen transformations in organic and mineral horizons. Earth-Sci. Rev. 201:103033. doi: 10.1016/j.earscirev.2019.103033
Cleveland, C. C., and Liptzin, D. (2007). C:N:P stoichiometry in soil: is there a “Redfield ratio” for the microbial biomass? Biogeochemistry 85, 235–252. doi: 10.1007/s10533-007-9132-0
Coonan, E. C., Kirkby, C. A., Kirkegaard, J. A., Amidy, M. R., Strong, C. L., Richardson, A. E., et al. (2020). Microorganisms and nutrient stoichiometry as mediators of soil organic matter dynamics. Nutr. Cycling Agroecosyst. 117, 273–298. doi: 10.1007/s10705-020-10076-8
Cotrufo, M. F., Wallenstein, M. D., Boot, C. M., Denef, K., and Paul, E. (2013). The microbial efficiency-matrix stabilization (MEMS) framework integrates plant litter decomposition with soil organic matter stabilization: do labile plant inputs form stable soil organic matter? Glob. Chang. Biol. 19, 988–995. doi: 10.1111/gcb.12113
Deng, L., Peng, C., Huang, C., Wang, K., Liu, Q., Yulin, L., et al. (2019). Drivers of soil microbial metabolic limitation changes along a vegetation restoration gradient on the Loess Plateau, China. Geoderma 353, 188–200. doi: 10.1016/j.geoderma.2019.06.037
Domeignoz-Horta, L. A., Pold, G., Liu, X. A., Frey, S. D., Melillo, J. M., Deangelis, K. M., et al. (2020). Microbial diversity drives carbon use efficiency in a model soil. Nat. Commun. 11:3684. doi: 10.1038/s41467-020-17502-z
Duan, C., Wang, Y., Wang, Q., Ju, W., Zhang, Z., Cui, Y., et al. (2022). Microbial metabolic limitation of rhizosphere under heavy metal stress: evidence from soil ecoenzymatic stoichiometry. Environ. Pollut. 300:118978. doi: 10.1016/j.envpol.2022.118978
Duan, Zhang, G., Rong, L., Fang, H., He, D., and Feng, D. (2015). Spatial distribution and environmental factors of catchment-scale soil heavy metal contamination in the dry-hot valley of Upper Red River in southwestern China. Catena 135, 59–69. doi: 10.1016/j.catena.2015.07.006
Garland, J. L. (1996). Analytical approaches to the characterization of samples of microbial communities using patterns of potential C source utilization. Soil Biol. Biochem. 28, 213–221. doi: 10.1016/0038-0717(95)00112-3
GB 15618 (2018). Soil Environmental Quality: Risk Control Standard for Soil Contamination of Agricultural Land. Beijing: Ministry of Ecology and Environment China (in Chinese).
Gilbert, F. A. (1952). Copper in nutrition. Adv. Agron. 4, 147–177. doi: 10.1016/S0065-2113(08)60308-9
Gong, Q., Wang, L., Dai, T., Zhou, J., Kang, Q., Chen, H., et al. (2019). Effects of copper on the growth, antioxidant enzymes and photosynthesis of spinach seedlings. Ecotoxicol. Environ. Saf. 171, 771–780. doi: 10.1016/j.ecoenv.2019.01.016
Graham, M. H., and Haynes, R. J. (2005). Organic matter accumulation and fertilizer-induced acidification interact to affect soil microbial and enzyme activity on a long-term sugarcane management experiment. Biol. Fertil. Soils 41, 249–256. doi: 10.1007/s00374-005-0830-2
Hall, E. K., Bernhardt, E. S., Bier, R. L., Bradford, M. A., Boot, C. M., Cotner, J. B., et al. (2018). Understanding how microbiomes influence the systems they inhabit. Nat. Microbiol. 3, 977–982. doi: 10.1038/s41564-018-0201-z
Hodge, A., Robinson, D., and Fitter, A. (2000). Are microorganisms more effective than plants at competing for nitrogen? Trends Plant Sci. 5, 304–308. doi: 10.1016/S1360-1385(00)01656-3
Huang, Z., Shen, Z., Liu, C., Shi, H., He, S., Long, G., et al. (2023). Characteristics of heavy metal accumulation and risk assessment in understory Panax notoginseng planting system. Environ. Geochem. Health 45, 9029–9040. doi: 10.1007/s10653-022-01392-9
Karimi, B., Masson, V., Guilland, C., Leroy, E., Pellegrinelli, S., Giboulot, E., et al. (2021). Ecotoxicity of copper input and accumulation for soil biodiversity in vineyards. Environ. Chem. Lett. 19, 2013–2030. doi: 10.1007/s10311-020-01155-x
Khan, S., Mulvaney, R., and Hoeft, R. (2001). A simple soil test for detecting sites that are nonresponsive to nitrogen fertilization. Soil Sci. Soc. Am. J. 65:118978. doi: 10.2136/sssaj2001.1751
Kopáček, J., Cosby, B. J., Evans, C. D., Hruška, J., Moldan, F., Oulehle, F., et al. (2013). Nitrogen, organic carbon and sulphur cycling in terrestrial ecosystems: linking nitrogen saturation to carbon limitation of soil microbial processes. Biogeochemistry 115, 33–51. doi: 10.1007/s10533-013-9892-7
Kumar, C., and Ghoshal, N. (2017). Impact of land-use change on soil microbial community composition and organic carbon content in the dry tropics of India. Pedosphere 27, 974–977. doi: 10.1016/S1002-0160(17)60404-1
Lebauer, D. S., and Treseder, K. K. (2008). Nitrogen limitation of net primary productivity in terrestrial ecosystems is globally distributed. Ecology 89, 371–379. doi: 10.1890/06-2057.1
Li, Z., Ma, Z., Van Der Kuijp, T. J., Yuan, Z., and Huang, L. (2014). A review of soil heavy metal pollution from mines in China: pollution and health risk assessment. Sci. Total Environ. 468–469, 843–853. doi: 10.1016/j.scitotenv.2013.08.090
Malik, A. A., Puissant, J., Buckeridge, K. M., Goodall, T., Jehmlich, N., Chowdhury, S., et al. (2018). Land use driven change in soil pH affects microbial carbon cycling processes. Nat. Commun. 9:3591. doi: 10.1038/s41467-018-05980-1
Manzoni, S., Taylor, P., Richter, A., Porporato, A., and Agren, G. I. (2012). Environmental and stoichiometric controls on microbial carbon-use efficiency in soils. New Phytol. 196, 79–91. doi: 10.1111/j.1469-8137.2012.04225.x
McGee, K. M., Eaton, W. D., Shokralla, S., and Hajibabaei, M. (2019). Determinants of soil bacterial and fungal community composition toward carbon-use efficiency across primary and secondary forests in a Costa Rican conservation area. Microb. Ecol. 77, 148–167. doi: 10.1007/s00248-018-1206-0
Mir, A. R., Pichtel, J., and Hayat, S. (2021). Copper: uptake, toxicity and tolerance in plants and management of Cu-contaminated soil. Biometals 34, 737–759. doi: 10.1007/s10534-021-00306-z
Moorhead, D., Sinsabaugh, R., Hill, B., and Weintraub, M. (2015). Vector analysis of ecoenzyme activities reveal constraints on coupled C, N and P dynamics. Soil Biol. Biochem. 93, 1–7. doi: 10.1016/j.soilbio.2015.10.019
Mooshammer, M., Hofhansl, F., Frank, A. H., Wanek, W., Hämmerle, I., Leitner, S., et al. (2017). Decoupling of microbial carbon, nitrogen, and phosphorus cycling in response to extreme temperature events. Sci. Adv. 3:e1602781. doi: 10.1126/sciadv.1602781
Olsen, S. R. (1954). Estimation of Available Phosphorus in Soils by Extraction with Sodium Bicarbonate. Washington, DC: US Department of Agriculture.
Ou, X., Wang, L., Guo, L., Cui, X., Liu, D., Yang, Y., et al. (2016). Soil-plant metal relations in Panax notoginseng: an ecosystem health risk assessment. Int. J. Environ. Res. Public Health 13:1089. doi: 10.3390/ijerph13111089
Peng, J.-Y., Zhang, S., Han, Y., Bate, B., Ke, H., Chen, Y., et al. (2022). Soil heavy metal pollution of industrial legacies in China and health risk assessment. Sci. Total Environ. 816:151632. doi: 10.1016/j.scitotenv.2021.151632
Philippot, L., Chenu, C., Kappler, A., Rillig, M. C., and Fierer, N. (2023). The interplay between microbial communities and soil properties. Nat. Rev. Microbiol. 22, 226–239. doi: 10.1038/s41579-023-00980-5
Qi, Q., Hu, C., Lin, J., Wang, X., Tang, C., Dai, Z., et al. (2022). Contamination with multiple heavy metals decreases microbial diversity and favors generalists as the keystones in microbial occurrence networks. Environ. Pollut. 306:119406. doi: 10.1016/j.envpol.2022.119406
Qi, R., Li, J., Lin, Z., Li, Z., Li, Y., Yang, X., et al. (2016). Temperature effects on soil organic carbon, soil labile organic carbon fractions, and soil enzyme activities under long-term fertilization regimes. Appl. Soil Ecol. 102, 36–45. doi: 10.1016/j.apsoil.2016.02.004
Qi, S., Wang, G., Li, W., and Zhou, S. (2023). Exploring the competitive dynamic enzyme allocation scheme through enzyme cost minimization. ISME Commun. 3:121. doi: 10.1038/s43705-023-00331-8
Rayment, G. E., and Higginson, F. R. (1992). Australian Laboratory Handbook of Soil and waTer Chemical Methods. Inkata Press. Available online at: https://www.nhbs.com/australian-soil-and-land-survey-book
Sinsabaugh, R., Turner, B., Talbot, J., Waring, B., Powers, J., Kuske, C., et al. (2016). Stoichiometry of microbial carbon use efficiency in soils. Ecol. Monogr. 86, 172–189. doi: 10.1890/15-2110.1
Sinsabaugh, R. L., Hill, B. H., and Follstad Shah, J. J. (2009). Ecoenzymatic stoichiometry of microbial organic nutrient acquisition in soil and sediment. Nature 462, 795–798. doi: 10.1038/nature08632
Sinsabaugh, R. L., Manzoni, S., Moorhead, D. L., and Richter, A. (2013). Carbon use efficiency of microbial communities: stoichiometry, methodology and modelling. Ecol. Lett. 16, 930–939. doi: 10.1111/ele.12113
Soares, M., and Rousk, J. (2019). Microbial growth and carbon use efficiency in soil: links to fungal-bacterial dominance, SOC-quality and stoichiometry. Soil Biol. Biochem. 131, 195–205. doi: 10.1016/j.soilbio.2019.01.010
Song, F. M., Ge, H. G., Li, C., Zhang, X. C., and Zhen, L. S. (2017). Heavy metals pollution in iron tailing soil and its effects on microbial communities metabolism and enzyme activities. J. Mines Metals Fuels 65, 319–327. doi: 10.18311/jmmf/2017/27012
Tao, Y., Shen, L., Han, S., Li, Z., Cui, Y., Lin, Y., et al. (2022). Metagenomic study of carbon metabolism in black soil microbial communities under lead-lanthanum stress. J. Hazard. Mater. 446, 130666–130666. doi: 10.1016/j.jhazmat.2022.130666
Teng, Y., Luo, Y.-M., Huang, C.-Y., Long, J., Li, Z.-G., Christie, P., et al. (2008). Tolerance of grasses to heavy metals and microbial functional diversity in soils contaminated with copper mine tailings1. Pedosphere 18, 363–370. doi: 10.1016/S1002-0160(08)60026-0
Vance, E. D., Brookes, P. C., and Jenkinson, D. (1987). An extraction method for measuring soil microbial biomass c. Soil Biol. Biochem. 19, 703–707. doi: 10.1016/0038-0717(87)90052-6
Wang, X., Dai, Z., Jiahui, L., Zhao, H., Yu, H., Ma, B., et al. (2023). Heavy metal contamination collapses trophic interactions in the soil microbial food web via bottom-up regulation. Soil Biol. Biochem. 184:109058. doi: 10.1016/j.soilbio.2023.109058
Waring, B. G., Weintraub, S. R., and Sinsabaugh, R. L. (2014). Ecoenzymatic stoichiometry of microbial nutrient acquisition in tropical soils. Biogeochemistry 117, 101–113. doi: 10.1007/s10533-013-9849-x
Wen, M., Ma, Z., Gingerich, D. B., Zhao, X., and Zhao, D. (2022). Heavy metals in agricultural soil in China: a systematic review and meta-analysis. Eco Environ. Health 1, 219–228. doi: 10.1016/j.eehl.2022.10.004
Wu, Y., Li, X., Yu, L., Wang, T., Wang, J., Liu, T., et al. (2022). Review of soil heavy metal pollution in China: spatial distribution, primary sources, and remediation alternatives. Resour. Conserv. Recycl. 181:106261. doi: 10.1016/j.resconrec.2022.106261
Xu, M., Cui, Y., Beiyuan, J., Wang, X., Duan, C., Fang, L., et al. (2021). Heavy metal pollution increases soil microbial carbon limitation: evidence from ecological enzyme stoichiometry. Soil Ecol. Lett. 3, 230–241. doi: 10.1007/s42832-021-0094-2
Zeng, H. C., Zhang, W. B., Feng, G. Q., Liu, Y. Z., Ma, N., and Yang, J. Z. (2009). The Cu, Pb, Cd, and Zn Content and pollution evaluation of Panax notoginseng cultivated soil in Wenshan. Chin. Tradit. Patent Med. 31, 317–320.
Zhang, Y., Zheng, Y., Xia, P., Xun, L., and Liang, Z. (2019). Impact of continuous Panax notoginseng plantation on soil microbial and biochemical properties. Sci. Rep. 9:13205. doi: 10.1038/s41598-019-49625-9
Zhao, L., Wang, Z., Kong, L., Zhao, Z., Geng, B., Gu, S., et al. (2023). Risk assessment of soil heavy metals in mining activity areas: a case study in Eastern Shandong Province, China. Environ. Earth Sci. 82:513. doi: 10.1007/s12665-023-11204-7
Keywords: exogenous copper, Panax notoginseng, carbon source utilization, extracellular enzyme activities, carbon use efficiency
Citation: Wang T, Wang X, Hadibi T, Ma X, Yao H, Tang Z, Fan F and Huang Y (2024) Effects of exogenous copper on microbial metabolic function and carbon use efficiency of Panax notoginseng planting soil. Front. Microbiol. 15:1390921. doi: 10.3389/fmicb.2024.1390921
Received: 24 February 2024; Accepted: 20 June 2024;
Published: 10 July 2024.
Edited by:
Min Luo, Fuzhou University, ChinaReviewed by:
Dengzhou Gao, Fujian Normal University, ChinaXianbiao Lin, Ocean University of China, China
Jianfei Sun, Nanning Normal University, China
Copyright © 2024 Wang, Wang, Hadibi, Ma, Yao, Tang, Fan and Huang. This is an open-access article distributed under the terms of the Creative Commons Attribution License (CC BY). The use, distribution or reproduction in other forums is permitted, provided the original author(s) and the copyright owner(s) are credited and that the original publication in this journal is cited, in accordance with accepted academic practice. No use, distribution or reproduction is permitted which does not comply with these terms.
*Correspondence: Fangling Fan, ZmFuZ2xpbmcuZmFuJiN4MDAwNDA7eW5udS5lZHUuY24=; Yizong Huang, eWl6b25naHVhbmcmI3gwMDA0MDsxMjYuY29t