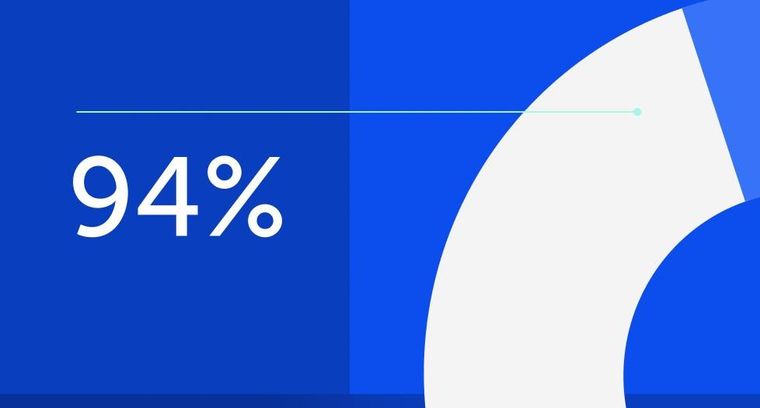
94% of researchers rate our articles as excellent or good
Learn more about the work of our research integrity team to safeguard the quality of each article we publish.
Find out more
ORIGINAL RESEARCH article
Front. Microbiol., 12 June 2024
Sec. Microbiotechnology
Volume 15 - 2024 | https://doi.org/10.3389/fmicb.2024.1389805
This article is part of the Research TopicNew Insight into the Roles of Microorganisms in Municipal and Environmental Engineering Technologies/Systems - Volume IIView all articles
Bacterial degradation mechanism for high chlorinated pentachlorobiphenyl (PentaCB) with worse biodegradability has not been fully elucidated, which could limit the full remediation of environments afflicted by the complex pollution of polychlorinated biphenyls (PCBs). In this research, a new PentaCB-degrading bacterium Microbacterium paraoxydans that has not been reported was obtained using enzymatic screening method. The characteristics of its intracellular enzymes, proteome and metabolome variation during PentaCB degradation were investigated systematically compared to non-PentaCB conditions. The findings indicate that the degradation rate of PentaCB (1 mg/L) could reach 23.9% within 4 hours and achieve complete degradation within 12 hours, with the mixture of intracellular enzymes being most effective at a pH of 6.0. During the biodegradation of PentaCB, the 12 up-regulated proteins characterized included ABC transporter PentaCB-binding protein, translocase protein TatA, and signal peptidase I (SPase I), indicating the presence of functional proteins for PentaCB degradation in both the cytoplasm and the outer surface of the cytoplasmic membrane. Furthermore, five differentially enriched metabolites were strongly associated with the aforementioned proteins, especially the up-regulated 1, 2, 4-benzenetriol which feeds into multiple degradation pathways of benzoate, chlorocyclohexane, chlorobenzene and aminobenzoate. These relevant results help to understand and speculate the complex mechanisms regarding PentaCB degradation by M. paraoxydans, which have both theoretical and practical implications for PCB bioremediation.
Industrialization and intensive use of chemical substance polychlorinated biphenyls (PCBs) are contributing to persistent and toxic environmental pollution (Furukawa and Fujihara, 2008; Megharaj et al., 2011). PCBs in submarine canyon sediments are several times higher than those in sediments from continental shelfs (Sevastyanov et al., 2020; Zhou et al., 2022). Removal of PCBs from contaminated environments has been primarily performed by incineration at high temperatures, which may entail additional energy consumption and greenhouse effect (Stella et al., 2017). Mild industrial conditions are beneficial for preventing modification of original heat-sensitive substrates and generation of adverse by-products (Ji et al., 2014). Biological PCBs clean-up methods have a considerable potential as a well-established and cost-effective strategy among methods employed for waste treatment and environmental remediation at ambient temperature (Šrédlová and Cajthaml, 2022). Microbial degradation of PCBs is highly strain-dependent (Furukawa and Matsumura, 1976; Han et al., 2023a) and screening for effective microbes is a time-consuming process, particularly when performed on a large scale. While several studies have examined bacteria that oxidatively degrade PCBs, encompassing both gram-negative and gram-positive bacteria (Furukawa and Fujihara, 2008; Megharaj et al., 2011), there still remains a pressing need for highly efficient screening methods and bacteria that can effectively degrade PCBs for practical application.
The majority of bacterial aromatic degradation is due to a series of enzymatic reactions, including the formation of diols, incorporation of oxygen atoms into the aromatic rings, and ring cleavage (Suenaga et al., 2009; Lee et al., 2016). Enzymes catalyze metabolic transformations under mild conditions with high catalytic rate and reaction selectivity, which could be realized in a shortened time (Ji et al., 2019). The screening speed of functional microbes could be much higher through bio-catalysis using enzymatic method. Moreover, bioconversion catalyzed by enzymes from microbes improves the bioavailability and the consequent biodegradation without prior desorption, which could more truly reflect the microbial degradation potential.
Commercial PCB mixtures are mostly tri- to hexachlorinated ones of 209 congeners (Furukawa and Fujihara, 2008). The di- and trichlorobiphenyls are the most readily degraded congeners (Gilbert and Crowley, 1998). Biodegradability decreases with increased number of chlorines, and PCB congeners with chlorine at position 2, 2′-(double ortho-substituted congeners) are poorly degraded (Furukawa and Fujihara, 2008). Wang et al. (2013) showed that the concentration of the most toxic dioxin-like PCBs (DL-PCBs) in surface soils ranged from 1.4 μg·kg−1 to 7.4 μg·kg−1 in Gudao town with chemical plants around, Dongying City, exceeding the Canadian soil environment quality guidelines. Tetrachlorobiphenyl (TetraCB) and pentachlorobiphenyl (PentaCB) were the major homologs, together accounting for more than 80% of the total DL-PCBs (Wang et al., 2013). Sanli et al. (2023) found that in industrial, urban and semi-rural areas of Bursa, Turkey, total PCBs content in spring surface soils reached 8.4~9.6 μg·kg−1, 2.9~4.1 μg·kg−1, 2.5~4.9 μg·kg−1, respectively, and was similarly dominated by TetraCBs and PentaCBs (Sanli et al., 2023). Previous studies about biodegradation of PentaCB are most involved in cometabolism with biphenyl or PCBs mixture (e.g., Aroclor mixtures) (Cvančarová et al., 2012). Bacterial degradation mechanism of PentaCB on protein and metabolite levels has not been fully elucidated. Incomplete biological information regarding the cellular responses restricts progress in the bioremediation process (Zhao and Poh, 2008).
In this study, an enzymatic screening approach (Appendix S1) was employed to acquire bacteria with high efficiency in degrading PentaCB. The most effective isolate, designated ECO-2, was identified as Microbacterium paraoxydans and officially preserved on June 1st, 2018, under the accession number 15836 at the China General Microbiological Culture Collection Center (CGMCC). Then, the enzymatic properties of Microbacterium paraoxydans, a bacterium with most significant degradative potential, were explored to elucidate the optimal conditions, addressing the limited information available on the PCBs degradation capacity in the genus of Microbacterium. Additionally, the cellular responses of M. paraoxydans to PentaCB exposure were examined at the proteome and metabolome levels. Tandem Mass Tag (TMT)-based quantitative proteomics and untargeted metabolomics were employed for a quantitative assessment, leading to the discovery of extensive changes in the proteome and metabolome profiles, providing new insights into how M. paraoxydans metabolically adapts throughout the biodegradation of PentaCB.
LB medium (Supplementary Table S1) was used for strain activation at 30°C and 150 rpm. Induction medium MSM (Supplementary Table S2, pH7.0) containing 1.0 mg·L−1 PCB101 (2, 2', 4, 5, 5'-Pentachlorobiphenyl; Anpel Company of Shanghai, China) which was one of the seven PCBs indicators of PCBs contamination status in soil and sediment specified by the United Nations GEMS/FOOD was used for PentaCB degrading enzymes production at 30°C and 150 rpm. Seed liquid was introduced into the induction medium and incubated. When the value of OD600 showed that the culture reached the end of the exponential phase, it was harvested by centrifugation at 8,000 rpm for 5 min under 4 °C. The cells were subsequently re-suspended in a 10 mM potassium phosphate buffer (4 mM DDT, pH7.0) and sonicated for 17 min at 320 W using a sonicator (SM-650D, Nanjing Shunma), with a sonication time of 3 s and quench time of 5 s (Ji et al., 2019). Following ultrasonic treatment, the suspension was centrifuged and the resulting supernatant was obtained as the PentaCB degrading enzymes. All experiments were performed in triplicate.
Microbacterium paraoxydans ECO-2, Arthrobacter phenanthrenivorans, Bacillus sp. ECO-12, Bacillus sp. ECO-1, Pseudomonas putida and Bacillus sp. ECO-10 are functional bacteria with biphenyl degrading ability which are screened and preserved in our laboratory. The preserved strains were removed from the −80°C refrigerator and activated using LB medium (Supplementary Table S1) for 12h at 30°C and 150rpm. Subsequently, seed liquids were introduced into the induction medium and incubated. When the value of OD600 showed that the culture reached the end of the exponential phase, they were harvested by centrifugation at 8,000 rpm for 5 min under 4 °C. Then the collected cells were re-suspended in a 10 mM potassium phosphate buffer (4 mM DDT, pH7.0). The volume of the buffer used was 20 times the mass of the cells. The cell lysing process, involving sonication at 320 W for 17 min with 2-s intervals of sonication and quenching, has been proven by response surface methodology to optimize enzyme activity. Following ultrasonic treatment, the suspension was centrifuged at 4°C and 8000 rpm for 20 min, and the resulting supernatant was PentaCB-degrading enzymes. All experiments were performed in triplicate.
Six enzymes were tested for PCB101 degradation rates. The reaction system consisted of 1.0 mg·L−1 PCB101, 2 mL PentaCB-degrading enzymes and PBS buffer (Supplementary Table S3) in a total reaction volume of 10 mL, control samples were devoid of these enzymes. After a reaction time of 12 h at 30°C and 150 rpm, the degradation rate of PentaCB was determined. All experiments were performed in triplicate.
The characteristics of intracellular enzyme from bacterium M. paraoxydans were measured. Each reaction setup operated individually with PentaCB-degrading enzymes at concentrations from 0.3 to 3.0 mg/L, enzymes concentrations were determined using BCA Protein Assay Kit (Beyotime, China), and pH values ranged from 4.0 to 8.0 in PBS buffer (Supplementary Table S3, various pH were adjusted using 1 mol/L HCl or NaOH). Control samples were devoid of these enzymes. The total volume of the reaction was 10 mL, and the reaction was carried out at 30°C and 150 rpm. At various intervals, samples were retrieved, and the remaining PentaCB concentrations were quantified via gas chromatography-mass spectrometry (GC-MS). Subsequently, the degradation rates were calculated based on these measurements.
Specifically, PentaCB in harvests was extracted using a Dionex 200 Accelerated Solvent Extraction (ASE) system and subjected to analysis using a GC–MS system (Varian, USA) consisting of 450-GC equipped with a Rxi-5Sil MS separation column (30 m × 0.25 mm × 0.25 μm) and 240-MS ion trap detector. The carrier gas, helium, was supplied at rate of 1 mL/min. A volume of 1 μl was injected with a splitless mode. The temperatures of injector, transfer line and ion trap were set at 280°C, 280°C and 220°C respectively. The oven temperature program was started at 100°C (held for 2 min) and then increased at 20°C/min to 230°C, at 2°C/min to 255°C and finally at 20°C/min to 300°C (held for 3 min). The MS detector used 70 eV for ionization. Qualitative analyses were performed by comparing retention times to those of real compounds and comparing mass spectra to plots in the NIST database. The PCB101 standard solution was prepared as five concentration gradients: 0.1, 0.5, 1.0, 2.0 and 5.0 mg·L−1, and a standard curve was prepared for quantification by peak area external standard method. The detection limit of this method was set at 0.02 mg·L−1. All experiments were performed in triplicate, and the presented results represent the mean values and standard deviations.
Cells of M. paraoxydans (CGMCC 15836) were cultured at 30°C and 150 rpm for 72 h until the late stationary phase (OD600 = 0.5, Supplementary Figure S1) in 100 mL induction medium comprising PCB101, and subsequently harvested by centrifugation at 8,000 rpm for 5 min under 4 °C. The precipitation cells were then subjected to three rounds of washing with an equal volume of ice-cold 0.2 M sucrose, followed by a final wash with ice-cold methanol. Meanwhile, cells without induction by PCB101 were prepared as controls (CK) under identical conditions (Figure 1). Proteomics and metabolomics quantification were performed at Novogene Bioinformatics Technology Co., Ltd. (Beijing, China). Detailed sequencing procedures are presented in Appendices S2, S3.
Figure 1. Overview of the workflow. CK, Cells without induction; YD, Cells induced by PentaCB; CKU, The supernatant of cells without induction; YDU, The supernatant of cells induced by PentaCB.
Intracellular enzymes from the six biphenyl metabolic bacteria exhibited the capability to degrade PCB101 (Figure 2A). Notably, the intracellular enzymes from M. paraoxydans with the growth curve depicted in Supplementary Figure S1, demonstrated the highest efficiency, decomposing 100% of PCB101. Arthrobacter phenanthrenivorans and Bacillus sp. ECO-12 followed with degradation rates of 39.6% and 30.8%, respectively.
Figure 2. PentaCB-degrading capabilities by intracellular enzymes from biphenyl metabolic bacteria. (A) Enzymatic reaction with 1 mg·L−1 PCB101 for 12 h. (B) Enzymatic reaction under different concentrations of PCB101, enzyme dosages, pH levels and time courses.
Wang et al. (2017) applied an enzymatic method instead of the shaking culture one to analyze the microbial metabolism capability of petroleum hydrocarbons, and the testing time was substantially reduced. In our study, it was further verified that this means was more efficient in distinguishing functional bacteria capable of pollutant degradation, which was valuable for surveying environmental resources.
The characteristics of intracellular enzyme from bacterium M. paraoxydans were measured for the optimal operating conditions during PentaCB degradation. It was showed that PCB101 degradation rate decreased to 78.4% and 17.2% respectively following its progressively increased concentration (Figure 2B). The addition of enzyme enhanced the degradation efficiency. Similarly, reducing the enzyme dosage by half, the degradation rate of PCB101 maintained directly proportional to the enzyme content. Within 2 h and 4 h, the enzymatic degradation efficiency of PCB101 reached 12.5% and 23.9%, respectively. This indicates the potential to significantly shorten the high-throughput screening process for functional microbes. The optimal pH for enzyme activity was found to be 6.0, with stability observed over a broad pH range of 4.0 to 8.0.
Quantification of proteome changes under PentaCB stress were analyzed, resulting in a total of 885,055 matched spectra to known ones. Additionally, 17,460 unique peptides were generated, and a total of 2,231 proteins were detected.
To obtain more detailed functional information about the quantified and identified proteins, a Venn diagram was used to compare the Gene Ontology (GO), clusters of orthologous groups (COG), Kyoto encyclopedia of genes and genomes (KEGG), and InterPro (IPR) domain annotations (Figure 3A). Based on GO terms, a total of 1,354 proteins were annotated across three categories: biological process (BP), cellular component (CC), and moleculr function (MF). The most commonly annotated category under BP term was the oxidation-reduction process including 161 proteins. Under the CC term, 123 proteins were annotated as being associated with membranes. In the MF category, 185 proteins were annotated as ATP binding. Using the COG functional classification, a total of 2,026 proteins were annotated. The main functional classification was amino acid transport and metabolism, which involved 261 proteins. Furthermore, 1,955 proteins were annotated in different KEGG pathways. The metabolism pathway with 1,178 proteins identified had the highest number of annotated proteins. Simultaneously, 1,974 proteins were annotated with different IPR terms. The most common IPR-annotated proteins included the AAA+ ATPase domain (90 proteins), the ABC transporter-like domain (72 proteins), and the ABC transporter type 1 transmembrane domain MetI-like (40 proteins).
Figure 3. Insights from Proteomic Analysis of PentaCB Degradation by M. paraoxydans. (A) Venn diagram of function annotation based on enriched GO terms, GOG functional classification, KEGG pathways and IPR terms. (B) Volcano plot of differential proteins between YD and CK groups. Each dot represents one protein. Red dots represent the significantly up-regulated proteins and green dots represent the significantly down-regulated proteins. Gray dots represent no significantly differential proteins. (C) Clustering heat map of differential proteins. (D) PPI network. (E) KEGG enriched pathways of differential proteins (p < 0.05).
Here, there were 24 differential proteins detected in which 15 up-regulated and 9 down-regulated proteins were included (Figure 3B). Among the up-regulated proteins, 12 characterized proteins were identified, including carbohydrate ABC transporter substrate-binding protein, L-alanine-DL-glutamate epimerase, peptidase inhibitor I9, galactokinase, DNA-binding transcriptional regulators (Lrp family, PadR family, Lrp/AsnC family), putative adhesion proteins, Sec-independent protein translocase protein TatA, bifunctional protein GlmU, sugar phosphate permease, and signal peptidase I (SPase I) (Figure 3C). Based on MF category, these up-regulated proteins were involved in serine-type peptidase activity, galactokinase activity, sequence-specific DNA binding, and protein transporter activity. Besides, the differentially up-regulated proteins were enriched in pathways of protein export, amino sugar and nucleotide sugar metabolism, bacterial secretion system, galactose metabolism, and quorum sensing (Figures 3D, E).
Metabolic profiling was performed to gain insights into the efficient degradation mechanism of PentaCB by M. paraoxydans. A total of 283 metabolites were identified and screened for differential accumulation. The top five differential metabolites distinguishing YD from CK (Figure 4) were determined based on VIP (variable importance in projection) score > 1.0, absolute value of log2 (fold change) > 1.2, and p <0.05. Four metabolites including 1, 2, 4-benzenetriol (C6H6O3), octadecanol, malonamide, citraconic acid (C5H6O4) showed significant up-regulation while hexadecane exhibited significant down-regulation (Figures 4B, C). Differential metabolites were enriched in pathways of benzoate degradation, chlorocyclohexane and chlorobenzene degradation, and aminobenzoate degradation (Figure 4D). The metabolic pathways were significantly enhanced in cells induced by PentaCB. The enriched pathways of benzoate degradation and aminobenzoate degradation were observed only in induced cells samples.
Figure 4. Insights from Metabolomic Analysis of PentaCB Degradation by M. paraoxydans. (A) Venn diagram for multigroup comparison of differential metabolites. (B) Volcano plot of differential metabolites between YD and CK groups. Each dot represents one metabolite. Red dots represent the significantly up-regulated metabolites and green dots represent the significantly down-regulated metabolites. Gray dots represent no significantly differential metabolites. The dot size represents the value of the VIP score. (C) Clustering heat map of differential metabolites. (D) KEGG enriched pathway of differential metabolite 1, 2, 4-benzenetriol. It reacts with oxygen to produce 2-maleylacetate. This reaction (R03891) is a constituent of the chlorocyclohexane and chlorobenzene degradation pathway (rn00361). CK, Cells without induction; CKU, The supernatant of cells without induction; YD, Cells induced by PentaCB; YDU, The supernatant of cells induced by PentaCB.
Through a combined analysis of differential proteome and metabolome, it was found that the 5 most influential metabolites were strongly associated with the 24 differentially abundant proteins that distinguished YD from CK during degradation process of PentaCB (Figure 5).
Figure 5. Correlation between differential proteins and metabolites during PentaCB Degradation by M. paraoxydans. The redder the color, the stronger the positive correlation, and the bluer the color, the stronger the negative correlation.
Several genera with reported PCBs-degrading ability include Rhodococcus, Bacillus, Pseudomonas, Achromobacter, and Burkholderia (Vasilyeva and Strijakova, 2007; Field and Sierra-Alvarez, 2008; Stella et al., 2017; Han et al., 2023b). Gilbert and Crowley (1997) observed that resting cells of carvone-induced Arthrobacter sp. B1B degraded 26 major components of Aroclor 1242, including 14 ± 6% of PCB101 within 15 h, and noted that the more highly chlorinated congeners (such as tetra- and pentachlorinated ones) were transformed at a slower rate compared to the di- and trichlorobiphenyls. There were also 14 bacteria strains co-metabolizing biphenyl and PCBs isolated from environmental samples, belonging to the genera Pseudomonas and Rhodococcus (Takahito et al., 2016). And Pseudomonas extremaustralis ADA-5 can utilize decachlorobiphenyl (DCB) as the unique carbon source and degrade 9.75% DCB within 336 h (López et al., 2021). In previous studies, the white-rot fungi Pleurotus ostreatus was the most efficient known organism in PCBs degradation, decomposing 99.6% of low-chlorinated biphenyls (commercial mixture Delor 103 with 2.6% PentaCB) after 42 days and having the capability of breaking down penta- and hexachlorinated biphenyls (Cvančarová et al., 2012). For contaminated soil, P. ostreatus achieved a 50.5% removal of PCBs from the rhizosphere of dumpsite soils after 12 weeks of treatment, however, significant PCBs concentration reduction was not observed within the first 6 weeks of incubation in highly contaminated soil. Both P. ostreatus and Irpex lacteus were capable of efficiently degrading a wide range of aromatic organopollutants through the secretion of oxidative enzymes with low substrate specificity in the process of PCBs biotransformation (Stella et al., 2017). Resuscitating viable but non-culturable (VBNC) bacteria could provide huge candidates for obtaining high-efficient PCBs degraders. Lin et al. (2022) utilized resuscitation-promoting factor (Rpf) to screen a resuscitated strain Streptococcus sp. SPC0 from PCBs (tri- and tetrachlorinated ones) contaminated soil and found that it degraded 88.6% of total PCBs within 84 h. Han et al. (2023b) used the similar methods to screen a strain Bacillus sp. LS1 under aerobic condition that degraded 59.6% and 50.1% of total PCBs (tri- and tetrachlorinated ones) with the concentrations of 10 mg/L and 20 mg/L within 84 h, respectively Table 1 summarizes the above PCBs-degrading strains and their capabilities. Compared with previous research, we used an efficient enzymatic screening method in this research, which directly acted on pollutants by extracting intracellular enzymes, and omitted the step of transferring pollutants from extracellular to intracellular, which greatly saved time. By using this method, we report the species M. paraoxydans exhibiting efficient ability of PentaCB degradation for the first time to our knowledge. M. paraoxydans and its intracellular enzymes showed the application value in the remediation of highly toxic and poorly degradable PCBs pollutants.
Functional enzymes of prokaryotic bacteria involved in organopollutants degradation were mainly intracellular (Kamei et al., 2006a). The intake process of pollutants was considered as a rate-limiting step. For example, the uptake of long-chain alkanes is the first step in its utilization by many gram-negative bacteria (Liu et al., 2023). Proteomic quantitative results based on iTRAQ showed that 123 proteins of Novosphingobium sp.ES2-1 were significantly upregulated under 17β-estradiol (E2) induction, two of which were associated with the ABC transport system (Li et al., 2024). In our study, it was also found that the expression of carbohydrate ABC transporter substrate-binding protein involved in transmembrane transport was up-regulated during PentaCB degradation.
Besides, as it was known, ABC transporters primarily utilized ATP binding and hydrolysis to transport different substrates into cells (Jiang et al., 2021). The transmembrane transport of fluoranthene in B. cereus required transmembrane proteins and energy (Jiang et al., 2021). In our study, GO and IPR functional analysis also showed transmembrane transport involved energy consumption in M. paraoxydans for PentaCB degradation, which could be categorized into active transport.
Majority proteins in the cytosol of gram-positive bacteria were exported through the two systems, the general secretion (Sec) system or the twin-arginine translocation (Tat) system (Sargent et al., 1998; Berks et al., 2000; Palmer and Berks, 2012). The latter was known for its ability to export completely folded proteins across the cytoplasmic membrane (Freudl, 2013). In this study, as one of the integral membrane proteins of Tat translocase, the expression of Sec-independent translocase protein TatA was differentially up-regulated (Supplementary Figure S2) and enriched in the pathway of protein export and bacterial secretion. Arauzo-Aguilera et al. (2023) reported recently protein YebF could be exported to the periplasm at high level through Tat pathway.
Moreover, another differentially up-regulated protein, the unique serine endoprotease SPase I, was essential for bacterial viability (Szałaj et al., 2023) and required by bacterial protein translocation pathways (Sec or Tat) for releasing translocated preproteins from the membrane during their transport from the cytoplasmic site of synthesis to extracytoplasmic locations (Auclair et al., 2012). In the case of M. paraoxydans, carbohydrate ABC transporter substrate-binding protein, TatA and SPase I (Supplementary Figure S2) were together up-regulated, indicating that bacterial enzymes involved in PentaCB degradation were distributed both in the cytoplasm and at the outer face of the cytoplasmic membrane.
Additionally, the differentially up-regulated proteins from M. paraoxydans under PCB101 stress were enriched in one pathway of quorum sensing, which suggested it played a role in promoting PentaCB degradation. As reported, gram-positive bacterium typically employed quorum sensing communication circuits mediated by processed oligopeptides to regulate various physiological activities, including symbiosis, movement, and biofilm formation (Alonso-Echanove et al., 2001; Jiang et al., 2021). At the same time, another up-regulated protein in our study, the bifunctional protein GlmU, was reported to be related to biofilm production in E. coli, Staphylococcus epidermidis, and Staphylococcus aureus (Burton et al., 2006; Suman et al., 2011; Sharma et al., 2016). GlmU was also found critical for biofilm formation in Mycobacterium smegmatis under alkylating stress (Somma et al., 2019).
Bacteria responses to environmental changes were also analyzed by metabolomics here (Eguchi et al., 2017; Zhou and Hu, 2017). Previous studies on PCBs bioremediation by white-rot fungi P. ostreatus and I. lacteus detected transformation products such as hydroxylated and methoxylated PCBs, chlorobenzoates, and chlorobenzyl alcohols (Stella et al., 2017). However, in this study, chlorinated derivatives of hydroxy- and methoxy-biphenyls were not detected among the differential metabolites. Instead, pathways related to chlorocyclohexane and chlorobenzene degradation, benzoate degradation, and aminobenzoate degradation were enriched.
The accumulation of intermediate PCBs metabolites was found to be minimal during degradation processes (Cvančarová et al., 2012). While previous research detected methoxylated metabolites but no hydroxylated compounds (Kamei et al., 2006b), this study identified a significantly up-regulated metabolite, 1, 2, 4-benzenetriol, which is a benzene metabolite (Sommers and Schiestl, 2006). Additionally, citraconic acid, another up-regulated metabolite, is associated with pyrimidine, purine, glutathione, and cysteine and methionine metabolism (Eguchi et al., 2017). Glutathione levels have been shown to decrease during PCBs treatment (Lai et al., 2010; Nomiyama et al., 2023; Zarerad et al., 2023), and PCBs metabolites can react with glutathione to form hydroquinone adducts of PCBs (Amaro et al., 1996). The increased octadecanol may enhance the hydrolytic action of phospholipases, which played a critical role in cell proliferation and metastasis (Foster and Xu, 2003; Bedia et al., 2015). On the other hand, hexadecane, which was significantly down-regulated, is used as a reaction medium for catalytic hydrodechlorination (Murena and Schioppa, 2000). These findings suggest that M. paraoxydans has the inherent ability to degrade PentaCB. The differential metabolites observed in M. paraoxydans cell samples, rather than the supernatant, indicate the cellular response to PentaCB stress, involving aromatic moiety decomposition, hydrodechlorination, and cell proliferation and metastasis.
In this research, an efficient enzymatic screening method helped to obtain a new PentaCB-degrading bacterium M. paraoxydans. Further, proteomic and metabolomic sequencing and deep analyses were employed to investigate the aerobic bacterial degradation mechanism of PentaCB. Figure 6 summarized the mechanism. The differential proteins and metabolites together revealed that PentaCB transport into cytoplasm required transmembrane proteins such as ABC transporter substrate-binding protein and energy expenditure. And functional bacterial proteins for PentaCB degradation were distributed both in the cytoplasm and outer surface of cytoplasmic membrane. Protein export, bacterial secretion, biofilm formation and quorum sensing also played crucial roles simultaneously during bacterial degradation of PentaCB (Supplementary Figure S2). These findings will contribute to a deeper understanding of the internal regulatory networks underlying the biodegradation mechanism of PentaCB.
The datasets presented in this study can be found in online repositories. The names of the repository/repositories and accession number(s) can be found in the article/Supplementary material.
LJ: Writing – original draft, Writing – review & editing. XC: Writing – original draft. LW: Writing – review & editing. XF: Writing – review & editing. WL: Writing – review & editing. LZ: Writing – review & editing. QL: Writing – review & editing. YX: Writing – review & editing. ZY: Writing – review & editing. YG: Writing – original draft, Writing – review & editing. FY: Writing – original draft, Writing – review & editing.
The author(s) declare that financial support was received for the research, authorship, and/or publication of this article. This work was supported by the National Key Research and Development Program of China (2023YFD1902701), the Shandong Provincial Key Research and Development Program, China (2018GSF117004), the National Natural Science Foundation of China (62102065), the Research Project (Youth Fund) of Shandong Academy of Sciences (2018QN0018), the Special Fund for Clinical Research of Shandong Provincial Medical Association (YXH2019ZX016), the Central-guided Local Science and Technology Development Fund (YDZX2023057), Joint Funds for the Innovation of Science and Technology, Fujian Province (2022J05055), and Fujian Medical University Research Foundation of Talented Scholars (XRCZX2022003).
The authors declare that the research was conducted in the absence of any commercial or financial relationships that could be construed as a potential conflict of interest.
All claims expressed in this article are solely those of the authors and do not necessarily represent those of their affiliated organizations, or those of the publisher, the editors and the reviewers. Any product that may be evaluated in this article, or claim that may be made by its manufacturer, is not guaranteed or endorsed by the publisher.
The Supplementary Material for this article can be found online at: https://www.frontiersin.org/articles/10.3389/fmicb.2024.1389805/full#supplementary-material
Alonso-Echanove, J., Shah, S. S., Valenti, A. J., Dirrigl, S. N., Carson, L. A., Arduino, M. J., et al. (2001). Nosocomial outbreak of Microbacterium species bacteremia among cancer patients. J. Infect. Dis. 184, 754–760. doi: 10.1086/323080
Amaro, A. R., Oakley, G. G., Bauer, U., Spielmann, H. P., and Robertson, L. W. (1996). Metabolic activation of PCBs to quinones: reactivity toward nitrogen and sulfur nucleophiles and influence of superoxide dismutase. Chem. Res. Toxicol. 9, 623–629. doi: 10.1021/tx950117e
Arauzo-Aguilera, K., Saaranen, M. J., Robinson, C., and Ruddock, L. W. (2023). Highly efficient export of a disulfide-bonded protein to the periplasm and medium by the Tat pathway using CyDisCo in Escherichia coli. Microbiol. Open. 12:e1350. doi: 10.1002/mbo3.1350
Auclair, S. M., Bhanu, M. K., and Kendall, D. A. (2012). Signal peptidase I: cleaving the way to mature proteins. Protein Sci. 21, 13–25. doi: 10.1002/pro.757
Bedia, C., Dalmau, N., Jaumot, J., and Tauler, R. (2015). Phenotypic malignant changes and untargeted lipidomic analysis of long-term exposed prostate cancer cells to endocrine disruptors. Environ. Res. 140, 18–31. doi: 10.1016/j.envres.2015.03.014
Berks, B. C., Sargent, F., and Palmer, T. (2000). The Tat protein export pathway. Mol. Microbiol. 35, 260–274. doi: 10.1046/j.1365-2958.2000.01719.x
Burton, E., Gawande, P. V., Yakandawala, N., LoVetri, K., Zhanel, G. G., Romeo, T., et al. (2006). Antibiofilm activity of GlmU enzyme inhibitors against catheter-associated uropathogens. Antimicrob. Agents Chemother. 50, 1835–1840. doi: 10.1128/AAC.50.5.1835-1840.2006
Cvančarová, M., Kresinová, Z., Filipová, A., Covino, S., and Cajthaml, T. (2012). Biodegradation of PCBs by ligninolytic fungi and characterization of the degradation products. Chemosphere 88, 1317–1323. doi: 10.1016/j.chemosphere.2012.03.107
Eguchi, A., Sakurai, K., and Watanabe, M. (2017). Chisato Mori. Exploration of potential biomarkers and related biological pathways for PCB exposure in maternal and cord serum: a pilot birth cohort study in Chiba, Japan. Environ. Int. 102, 157–164. doi: 10.1016/j.envint.2017.02.011
Field, J. A., and Sierra-Alvarez, R. (2008). Microbial transformation and degradation of polychlorinated biphenyls. Environ. Pollut. 155, 1–12. doi: 10.1016/j.envpol.2007.10.016
Foster, D. A., and Xu, L. (2003). Phospholipase D in cell proliferation and cancer. Mol. Cancer Res. 1, 789–800. Available online at: https://pubmed.ncbi.nlm.nih.gov/14517341/
Freudl, R. (2013). Leaving home ain't easy: protein export systems in Gram-positive bacteria. Res. Microbiol. 164, 664–674. doi: 10.1016/j.resmic.2013.03.014
Furukawa, K., and Fujihara, H. (2008). Microbial degradation of polychlorinated biphenyls: biochemical and molecular features. J. Biosci. Bioeng. 105, 433–449. doi: 10.1263/jbb.105.433
Furukawa, K., and Matsumura, F. (1976). Microbial metabolism of polychlorinated biphenyls. Studies on the relative degradability of polychlorinated biphenyl components by Alcaligenes sp. J. Agric. Food Chem. 24, 251–256. doi: 10.1021/jf60204a002
Gilbert, E. S., and Crowley, D. E. (1997). Plant compounds that induce polychlorinated biphenyl biodegradation by Arthrobacter sp. strain B1B. Appl. Environ. Microbiol. 63, 1933–1938. doi: 10.1128/aem.63.5.1933-1938.1997
Gilbert, E. S., and Crowley, D. E. (1998). Repeated application of carvone-induced bacteria to enhance biodegradation of polychlorinated biphenyl in soil. Appl. Microbiol. Biotechnol. 50, 489–494. doi: 10.1007/s002530051325
Han, Z., Hou, A. Q., Cai, X. L., Xie, M. Q., Sun, F. Q., Shen, C. F., et al. (2023a). Unlocking the potential of resuscitation-promoting factor for enhancing anaerobic microbial dechlorination of polychlorinated biphenyls. Sci. Total Environ. 897:165440. doi: 10.1016/j.scitotenv.2023.165440
Han, Z., Lin, Q. H., Zhang, S. S., Zhou, X. R., Li, S., Sun, F. Q., et al. (2023b). High PCBs mineralization capability of a resuscitated strain Bacillus sp. LS1 and its survival in PCB-contaminated soil. Sci. Total Environ. 856:159224. doi: 10.1016/j.scitotenv.2022.159224
Ji, L., Fu, X. W., Wang, M. T., Xu, C., Chen, G. H., Song, F. Y., et al. (2019). Enzyme cocktail containing NADH regeneration system for efficient bioremediation of oil sludge contamination. Chemosphere 233, 132–139. doi: 10.1016/j.chemosphere.2019.05.253
Ji, L., Yang, J., Fan, H., Yang, Y., Li, B., Yu, X., et al. (2014). Synergy of crude enzyme cocktail from cold-adapted Cladosporium cladosporioides Ch2-2 with commercial xylanase achieving high sugars yield at low cost. Biotechnol. Biofuels. 7:130. doi: 10.1186/s13068-014-0130-x
Jiang, R., Wu, X. X., Xiao, Y. Q., Kong, D. K., Li, Y., Wang, H. Q., et al. (2021). Tween 20 regulate the function and structure of transmembrane proteins of Bacillus cereus: Promoting transmembrane transport of fluoranthene. J. Hazard. Mater 403, 123707–123717. doi: 10.1016/j.jhazmat.2020.123707
Kamei, I., Kogura, R., and Kondo, R. (2006a). Metabolism of 4,4′-dichlorobiphenyl by white-rot fungi Phanerochaete chrysosporium and Phanerochaete sp. MZ142. Appl. Microbiol. Biotechnol. 72, 566–575. doi: 10.1007/s00253-005-0303-4
Kamei, I., Sonoki, S., Haraguchi, K., and Kondo, R. (2006b). Fungal bioconversion of toxic polychlorinated biphenyls by white-rot fungus, Phlebia brevispora. Appl. Microbiol. Biotechnol. 73, 932–940. doi: 10.1007/s00253-006-0529-9
Lai, I., Chai, Y., Simmons, D., Luthe, G., Coleman, M. C., Spitz, D., et al. (2010). Acute toxicity of 3,3′,4,4′,5-pentachlorobiphenyl (PCB 126) in male Sprague-Dawley rats: effects on hepatic oxidative stress, glutathione andmetals status. Environ. Int. 36, 918–923. doi: 10.1016/j.envint.2009.11.002
Lee, S. Y., Sekhon, S. S., Ban, Y. H., Ahn, J. Y., Ko, J. H., Lee, L., et al. (2016). Proteomic analysis of polycyclic aromatic hydrocarbons (PAHs) degradation and detoxification in Sphingobium chungbukense DJ77. J. Microbiol. Biotechnol. 26, 1943–1950. doi: 10.4014/jmb.1606.06005
Li, S. Y., Yang, W., Mo, J. J., Wang, Y. B., Lu, C., Gao, Y. Z., et al. (2024). Adaptive responses and metabolic strategies of Novosphingobium sp. ES2-1–17β-estradiol analyzed through integration of genomic and proteomic approaches. J. Hazard. Mater. 461:132543. doi: 10.1016/j.jhazmat.2023.132543
Lin, Q. H., Zhou, X. R., Zhang, S. S., Gao, J. L., Xie, M. Q., Tao, L. Q., et al. (2022). Oxidative dehalogenation and mineralization of polychlorinated biphenyls by a resuscitated strain Streptococcus sp. SPC0. Environ. Res. 207:112648. doi: 10.1016/j.envres.2021.112648
Liu, J., Chen, S., Zhao, B., Li, G. Q., and Ma, T. (2023). A Novel FadL homolog, altl, mediates transport of long-chain alkanes and fatty acids in acinetobacter venetianus RAG-1. Appl. Environ. Microbiol. 88:e0129422. doi: 10.1128/aem.01294-22
López, M. A. G., Zenteno-Rojas, A., Martinez-Romero, E., Rincón-Molina, C. I., Vences-Guzmán, M. A., Ruíz-Valdiviezo, V. M., et al. (2021). Biodegradation and Bioaccumulation of Decachlorobiphenyl (DCB) by Native Strain Pseudomonas extremaustralis ADA-5. Water Air Soil Pollut. 232:192. doi: 10.1007/s11270-021-05122-2
Megharaj, M., Ramakrishnan, B., Venkateswarlu, K., Sethunathan, N., and Naidu, R. (2011). Bioremediation approaches for organic pollutants: a critical perspective. Environ. Int. 37, 1362–1375. doi: 10.1016/j.envint.2011.06.003
Murena, F., and Schioppa, E. (2000). Kinetic analysis of catalytic hydrodechlorination process of polychlorinated biphenyls (PCBs). Appl. Catal. B. 27, 257–267. doi: 10.1016/S0926-3373(00)00157-0
Nomiyama, K., Yamamoto, Y., Eguchi, A., Nishikawa, H., Mizukawa, H., Yokoyama, N., et al. (2023). Health impact assessment of pet cats caused by organohalogen contaminants by serum metabolomics and thyroid hormone analysis. Sci. Total Environ. 842:156490. doi: 10.1016/j.scitotenv.2022.156490
Palmer, T., and Berks, B. (2012). The twin-arginine translocation (Tat) protein export pathway. Nat. Rev. Microbiol. 10, 483–496. doi: 10.1038/nrmicro2814
Sanli, G. E., Erkul, S. N., and Tasdemir, Y. (2023). Spatio-temporal variations, fugacity fractions and air-soil exchanges of PCBs in industrial, urban and semi-rural sites. Polycycl. Aromat. Compd. 44, 1019–1036. doi: 10.1080/10406638.2023.2185267
Sargent, F., Bogsch, E. G., Stanley, N. R., Wexler, M., Robinson, C., Berks, B. C., et al. (1998). Overlapping functions of components of a bacterial Sec-independent protein export pathway. EMBO J. 17, 3640–3650. doi: 10.1093/emboj/17.13.3640
Sevastyanov, V. S., Kuznetsova, O. V., Fedulov, V. S., Fedulova, V. Y., Dushenko, N. V., Naimushin, S. G., et al. (2020). Accumulation of organic matter, heavy metals, and rare-earth elements in marine sediment at different distance from the Indigirka River Delta. Geochem. Int. 58, 1313–1320. doi: 10.1134/S0016702920120046
Sharma, R., Lambu, M. R., Jamwa, U., Rani, C., Chib, R., Wazir, P., et al. (2016). Escherichia coli N-Acetylglucosamine-1-Phosphate-Uridyltransferase/Glucosamine-1-Phosphate-Acetyltransferase (GlmU) inhibitory activity of terreic acid isolated from Aspergillus terreus. SLAS Discov. 21, 342–353. doi: 10.1177/1087057115625308
Somma, A. D., Caterino, M., Soni, V., Agarwal, M., Pasquale, P. D., Zanetti, S., et al. (2019). The bifunctional protein GlmU is a key factor in biofilm formation induced by alkylating stress in Mycobacterium smegmatis. Res. Microbiol. 170, 171–181. doi: 10.1016/j.resmic.2019.03.002
Sommers, C. H., and Schiestl, R. H. (2006). Effect of benzene and its closed ring metabolites on intrachromosomal recombination in Saccharomyces cerevisiae. Mutat. Res. Fund Mol. M. 593, 1–8. doi: 10.1016/j.mrfmmm.2005.06.026
Šrédlová, K., and Cajthaml, T. (2022). Recent advances in PCB removal from historically contaminated environmental matrices. Chemosphere 287:132096. doi: 10.1016/j.chemosphere.2021.132096
Stella, T., Covino, S., Cvančarová, M., Filipová, A., Petruccioli, M., D'Annibale, A., et al. (2017). Bioremediation of long-term PCB-contaminated soil by white-rot fungi. J. Hazard. Mater. 324, 701–710. doi: 10.1016/j.jhazmat.2016.11.044
Suenaga, H., Koyama, Y., Miyakoshi, M., Miyazaki, R., Yano, H., Sota, M., et al. (2009). Novel organization of aromatic degradation pathway genes in a microbial community as revealed by metagenomic analysis. ISME J. 3, 1335–1348. doi: 10.1038/ismej.2009.76
Suman, E., D'souza, S. J., Jacob, P., Sushruth, M. R., and Kotian, M. S. (2011). Anti-biofilm and anti-adherence activity of Glm-U inhibitors. Indian J. Med. Sci. 65, 387–392. doi: 10.4103/0019-5359.108954
Szałaj, N., Benediktsdottir, A., Rusin, D., Karlén, A., Mowbray, S. L., and Wieckowska, A. (2023). Bacterial type I signal peptidase inhibitors - Optimized hits from nature. Eur. J. Med. Chem. 238:114490. doi: 10.1016/j.ejmech.2022.114490
Takahito, W., Hidehiko, F., Jun, H., Hikaru, S., and Nobutada, K. (2016). Use of biphenyl/polychlorinated biphenyl-degrading bacteria for the production of useful aromatic compounds. Sust. Humanosphere Bullet. Res. Inst. Sust. Human. Kyoto Univ. 12, 5–5. Available online at: https://repository.kulib.kyoto-u.ac.jp/dspace/handle/2433/225929
Vasilyeva, G. K., and Strijakova, E. R. (2007). Bioremediation of soils and sediments contaminated by polychlorinated biphenyls. Microbiology 76, 639–653. doi: 10.1134/S002626170706001X
Wang, D. G., Cui, Z. J., Fu, X. W., Yin, Y. Q., and Xu, H. Y. (2013). Characteristics of dioxin-like polychlorinated biphenyls contamination in soils of Gudao region in Dongying. Environ. Sci. 34, 2416–2421. Available online at: https://pubmed.ncbi.nlm.nih.gov/23947064/
Wang, S., Guo, S. H., Wang, J. N., Li, F. M., Wu, B., Zhang, Q., et al. (2017). Rapid screening and construction of high-efficient petroleum-degrading microflora through enzyme activity assay. Chin. J. Appl. Ecol. 28, 1932–1940. doi: 10.13287/j.1001-9332.201706.024
Zarerad, E., Niksalehi, K., Armandeh, M., Sani, M. A., Ataei, M., Mousavi, T., et al. (2023). Polychlorinated biphenyls: a review of recent updates on food safety and environmental monitoring, health and toxicological implications, and analysis. Mini Rev. Med. Chem. 23, 1390–1411. doi: 10.2174/1389557523666221213091445
Zhao, B., and Poh, C. L. (2008). Insights into environmental bioremediation by microorganisms through functional genomics and proteomics. Proteomics 8, 874–881. doi: 10.1002/pmic.200701005
Zhou, Q., Wang, S., Liu, J., Hu, X., Liu, Y., He, Y., et al. (2022). Geological evolution of offshore pollution and its long-term potential impacts on marine ecosystems. Geosci. Front. 13:101427. doi: 10.1016/j.gsf.2022.101427
Keywords: enzymatic screening, pentachlorobiphenyl degradation, Microbacterium paraoxydans, proteome, metabolome
Citation: Ji L, Chang X, Wang L, Fu X, Lai W, Zheng L, Li Q, Xing Y, Yang Z, Guan Y and Yang F (2024) Insights into the biodegradation of pentachlorobiphenyl by Microbacterium paraoxydans: proteomic and metabolomic studies. Front. Microbiol. 15:1389805. doi: 10.3389/fmicb.2024.1389805
Received: 22 February 2024; Accepted: 28 May 2024;
Published: 12 June 2024.
Edited by:
Xiaochen Chen, Fuzhou University, ChinaReviewed by:
Yongchao Yin, Northeastern University, United StatesCopyright © 2024 Ji, Chang, Wang, Fu, Lai, Zheng, Li, Xing, Yang, Guan and Yang. This is an open-access article distributed under the terms of the Creative Commons Attribution License (CC BY). The use, distribution or reproduction in other forums is permitted, provided the original author(s) and the copyright owner(s) are credited and that the original publication in this journal is cited, in accordance with accepted academic practice. No use, distribution or reproduction is permitted which does not comply with these terms.
*Correspondence: Lei Ji, amlsZWkuMTAxMEAxNjMuY29t; Yuyao Guan, Z3VhbnhpYW95YW8wODE1QDE2My5jb20=; Fenglong Yang, eWFuZ2Zlbmdsb25nQGZqbXUuZWR1LmNu
†These authors share first authorship
Disclaimer: All claims expressed in this article are solely those of the authors and do not necessarily represent those of their affiliated organizations, or those of the publisher, the editors and the reviewers. Any product that may be evaluated in this article or claim that may be made by its manufacturer is not guaranteed or endorsed by the publisher.
Research integrity at Frontiers
Learn more about the work of our research integrity team to safeguard the quality of each article we publish.