- 1The Novo Nordisk Foundation Center for Biosustainability, Technical University of Denmark, DK-2800 Kgs, Lyngby, Denmark
- 2Department of Biochemistry and Systems Biology, Institute of Systems, Molecular and Integrative Biology, University of Liverpool, Liverpool, United Kingdom
- 3Department of Chemistry, Manchester Institute of Biotechnology, The University of Manchester, Manchester, United Kingdom
The exchange of small molecules between the cell and the environment happens through transporter proteins. Besides nutrients and native metabolic products, xenobiotic molecules are also transported, however it is not well understood which transporters are involved. In this study, by combining exo-metabolome screening in yeast with transporter characterization in Xenopus oocytes, we mapped the activity of 30 yeast transporters toward six small non-toxic substrates. Firstly, using LC–MS, we determined 385 compounds from a chemical library that were imported and exported by S. cerevisiae. Of the 385 compounds transported by yeast, we selected six compounds (viz. sn-glycero-3-phosphocholine, 2,5-furandicarboxylic acid, 2-methylpyrazine, cefadroxil, acrylic acid, 2-benzoxazolol) for characterization against 30 S. cerevisiae xenobiotic transport proteins expressed in Xenopus oocytes. The compounds were selected to represent a diverse set of chemicals with a broad interest in applied microbiology. Twenty transporters showed activity toward one or more of the compounds. The tested transporter proteins were mostly promiscuous in equilibrative transport (i.e., facilitated diffusion). The compounds 2,5-furandicarboxylic acid, 2-methylpyrazine, cefadroxil, and sn-glycero-3-phosphocholine were transported equilibratively by transporters that could transport up to three of the compounds. In contrast, the compounds acrylic acid and 2-benzoxazolol, were strictly transported by dedicated transporters. The prevalence of promiscuous equilibrative transporters of non-native substrates has significant implications for strain development in biotechnology and offers an explanation as to why transporter engineering has been a challenge in metabolic engineering. The method described here can be generally applied to study the transport of other small non-toxic molecules. The yeast transporter library is available at AddGene (ID 79999).
1 Introduction
Transporter proteins mediate the import or export of nutrients, metabolic products, and other small molecules through equilibrative and concentrative transport across the membranes. Paradoxically, despite their potential applications in biotechnology and human medicine, transporter proteins are among the least characterized protein categories (César-Razquin et al., 2015). Only a handful of the 341 S. cerevisiae 288C transporters listed in the TransportDB database (Berninsone and Hirschberg, 2000; Baek et al., 2016; Baldi et al., 2021; Belew et al., 2022) have been experimentally characterized for substrate specificity. Recently, efforts have been made to deorphanize transporter proteins in humans (Superti-Furga et al., 2020) and plants (Nour-Eldin et al., 2006, 2012; Payne et al., 2017; Otterbach et al., 2019).
It has been suggested that the export of xenobiotic molecules occurs by ATP-binding cassette (ABC) transporters. The import of xenobiotics was proposed to happen via nutrient carriers (Almeida et al., 2021). While ABC transporters are unidirectional, carriers are hypothesized to be bidirectional depending on the concentration gradient of the co-substrate for anti-porters and symporters (Alberts et al., 2002), or by the concentration of the compound on both sides of the membrane for uniporters (Alberts et al., 2002; Zhang and Han, 2016). While substrate specificity is paramount to the function of a transporter protein, the mechanism of transport is equally fundamental for the application of transporter proteins (Darbani et al., 2019).
The current go-to approaches for high-throughput characterization of transporters in yeast (Giaever et al., 2002) and human cell lines (Girardi et al., 2020) relies on phenotypes that are easy to assess. This bias favors substrates that exhibit cellular toxicity or are fluorescent, excluding many non-toxic, non-fluorescent xenobiotic compounds important in biotechnology or health context. Another strategy uses untargeted metabolomics to determine differential uptake in cell lines differing in their expression levels of the target of interest (Gründemann et al., 2005; Wright Muelas et al., 2020).
In this study, we sought to experimentally assign novel substrates to transporters from transporter superfamilies with known capacity for xenobiotic substrates. To do so, we initially performed a global identification of potential substrates for yeast transporters from a chemical library. We used human blood serum as a low-cost ‘chemical library’ (Gründemann et al., 2005; Dunn et al., 2011; Wright Muelas et al., 2020) as it contains a wide array of compounds, from metabolites to xenobiotics, stemming from traces of dietary compounds and drugs (Dunn et al., 2011; Psychogios et al., 2011; Wright Muelas et al., 2020). Human blood serum is an ideal mix for assessing substrate specificity across different chemical classes. We used the yeast S. cerevisiae, as the screening organism, as it is a well-studied eukaryotic model organism with well-defined transporter proteins (Kell et al., 2011). In a previous effort, we identified the human and fungal genomes as having the same fraction of active transporters (Darbani et al., 2018), arguably suggesting that the results found in yeast are broadly applicable to eukaryotes. Besides, yeast has an extraordinary ability to import and export compounds found in its environment and hence possesses a repository of transporters for xenobiotics and metabolites.
Next, to address the question of which yeast transporters could transport the selected compounds and their mode of transport, we generated a dedicated library of full-length mRNA encoding multidrug or complex metabolite transporters from yeast. The candidate transporters were expressed in Xenopus laevis oocytes and tested for activity toward the target compounds.
Xenopus oocytes have unique features, such as low background transport activity, high capacity for correct transporter protein synthesis, and low codon bias (Romero et al., 1998). The low level of endogenous transporters makes it possible to determine transport activity toward a given substrate by extracting the whole Xenopus oocyte and analyzing the content by LC–MS. This facilitates identification of transporters for non-electrogenic substrates, making the method more broadly applicable than analysis by electrophysiology, as electrophysiology requires the generation of an electrical current for the detection of transport activity. Utilizing a similar methodology, Xenopus oocytes have been used successfully to identify plant metabolite transporters (Nour-Eldin et al., 2012; Tal et al., 2016; Jørgensen et al., 2017; Wulff et al., 2019; Xu et al., 2023) and characterize transporters for the natural compounds resveratrol, succinic acid, and malic acid (Darbani et al., 2019), as well as metabolomic profiling for determination of substrate specificity of transporter proteins (Fairweather et al., 2021).
2 Materials and methods
2.1 Buffers and reagents
Sodium acrylate (Sigma-Aldrich, Søborg, Denmark), sn-Glycero-3-phosphocholine, >98% (Santa Cruz Biotechnology, Inc., Heidelberg, Germany), cefadroxil 95–105%, (VWR, Søborg, Denmark), 2-methylpyrazine (VWR, Søborg, Denmark), and 2-benzoxazolinone, ≥98.0% (VWR, Søborg, Denmark), 2,5-furandicarboxylic acid (TCI Europe N. V., Zwijndrecht, Belgium) were used in the study. Xenopus oocytes were incubated in either HEPES-based Kulori buffer (90 mM NaCl, 1 mM KCl, 1 mM MgCl2, 1 mM CaCl2, 5 mM HEPES pH 7.4) supplemented with gentamycin (100 μg/mL), or MES-based Kulori buffer (90 mM NaCl, 1 mM KCl, 1 mM MgCl2, 1 mM CaCl2, 5 mM MES pH 5.0).
2.2 Strains, media and growth conditions
Yeast knockout mutant strains with BY4741 (MATa; his3Δ1; leu2Δ0; met15Δ0; ura3Δ0) background were purchased from Open Biosystems (catalog # YSC1053). For Growth Profiler 960 (Enzyscreen B.V., CR9001) measurements, yeast pre-cultures were inoculated in 2 mL synthetic dextrose medium (SD –Trp) (20 g/L glucose, 7 g/L yeast nitrogen base, 2 g/L histidine, 3 g/L leucine, 2 g/L methionine, 2 g/L uracil) in 15 mL growth tubes and grown for 24 h at 30°C and 250 rpm. Optical density at 600 nm (OD600) was then measured and adjusted to 0.01, in a 24 deep-well plate (Enzyscreen B.V., CR1424d), containing 2 mL SD –Trp supplemented with the indicated concentrations of additives. The cultures were grown for 72 h at 30°C with 300 rpm agitation and measurements acquired every 20 min.
2.3 Exometabolome assay
Pooled human blood serum, 0.1 μm filtered was purchased from BioIVT (West Sussex, United Kingdom). S. cerevisiae strain CEN.PK113-7D (MATa URA3 HIS3 LEU2 TRP1 MAL2-8c SUC2) was a gift from Peter Kötter (Goethe University, Frankfurt/Main, Germany).
An overnight preculture of CEN.PK113-7D was grown in YPD overnight, resuspended in YPD to an OD600 = 2. At OD600 = 8, 1 mL of culture was taken and the cells were centrifuged at 3,000 × g for 5 min. The cells were washed, and resuspended in MilliQ to a final OD600 of 2. 1 mL of the yeast solution was centrifuged at 3,000 × g for 5 min, and resuspended in 200 μL pooled human blood serum, to a final OD600 of 10. The experiment was performed in duplicate, with two independent biological replicates. After 30 min of incubation at 30°C in a shaking incubator at 250 rpm, the cells were collected by centrifugation at 3,000 x g for 5 min and 200 μL of the supernatant was mixed with 700 μL of ice-cold methanol. Filtered pooled human blood serum was used as control. The mixtures of supernatant or serum in methanol were mixed by vortexing, and cleared for protein precipitate by centrifugation. The supernatant was aliquoted and dried in a centrifugal evaporator with no temperature application. Prior to LC–MS analysis, samples were resuspended in LC–MS grade water.
All analyses were performed using an Ultimate 300 LC system (Thermo Fisher Scientific) using a HypersilGold a C18 2.1 mm x 100 mm, 1.9 μm column (Thermo Fisher Scientific), coupled to an Orbitrap Fusion Tribrid Mass Spectrometer (Thermo Fisher Scientific). Parameters for LC elution gradient and parameters for data acquisition on the MS/MS system are described in Wright Muelas et al., (2020).
The raw data files were imported into Progenesis QI (Non-linear Dynamics, Newcastle upon Tyne, United Kingdom) for processing. Peak alignment, picking and compound identification were performed using the software. Basic descriptive analysis, as well as statistical analysis to calculate fold changes in metabolites of yeast incubated pooled human blood serum versus normal pooled human blood serum was done in R (version 3.5.1). A threshold of Log2 fold change of compound levels in yeast supernatant relative to serum >0.5 with a CV < 30% was used to identify consumed and secreted compounds.
2.4 DNA constructs
To express the transporter coding genes in oocytes, genes were cloned downstream of the T7 promoter in the USER compatible Xenopus expression vector pUSER016 (Nour-Eldin et al., 2006). The empty vector was digested by PacI and Nt.BbvCI (New England Biolabs). The transporter genes were amplified from genomic DNA of CEN.PK113-7D or BY4741, where indicated. The amplified DNA fragments were gel purified and together with the linearized plasmids incubated with USER enzyme (New England Biolabs) for 25 min at 37°C, followed by incubation at 25°C for 25 min. The reactions were transformed into chemically competent E. coli cells. All the cloned plasmids were verified by Sanger sequencing. See Supplementary Tables S1–S3 for the sequences of primers and a list of the plasmids constructed in this study (Supplementary Table S4).
2.5 Oocyte uptake assay
The selected transporters were cloned into expression vectors using USER cloning. The expression vector contained the T7 transcription initiation site and a poly-A tail and terminator. The full construct was amplified by PCR, and in vitro transcribed using the mMessage mMachine T7 transcription kit from Thermo Fisher Scientific. The quality of the generated RNA was analyzed using an Agilent 2,100 Bioanalyzer. Defollicated stage V-IV oocytes from Xenopus laevis were purchased from Ecocyte Bioscience (Dortmund, Germany). Individual oocytes were distributed into a 96 well plate with V-bottom containing Kulori buffer pH 7.4, and injected with 50 nL of a mix of 100 ng/μL RNA of GFP and 400 ng/μL RNA of a transporter of interest using a Roboinject 96 well multichannel injection system (Multi Channel Systems MCS GmbH, Reutlingen, Germany). Control oocytes were injected with 100 ng/μL RNA of GFP only. The oocytes incubated for 3 days at 18 °C.
The uptake assay was carried out as described in Nour-Eldin et al. (2006) and Wulff et al. (2019). After preincubation the oocytes were transferred to the assay solution containing Kulori and the compounds at a concentration of 2 mM each, with the pH of the solution adjusted to pH 5. The oocytes were incubated in the assay solution for 3 h at 18 °C. After washing with Kulori pH 7.4, pools of 10 oocytes were transferred to an Eppendorf tube, and the oocytes were disrupted by pipetting together with 50% ice cold MeOH. The oocyte mix was stored for min 2 h at −20°C, and the supernatant collected by centrifugation at 20,000 g for 15 min. The supernatant was further diluted with MilliQ to a final concentration of 29% MeOH before the samples were analyzed by LC–MS. LC–MS data was collected on EVOQ EliteTriple Quadrupole Mass Spectrometer system coupled with an Advance UHPLC pump (Bruker, Fremont, CA, United States). See Supplementary Table S5 for further information on the LC–MS method.
2.6 Detection of compounds
LC–MS data was collected on EVOQ EliteTriple Quadrupole Mass Spectrometer system coupled with an Advance UHPLC pump (Bruker, Fremont, CA, United States). Samples were kept cold in the CTC HTS PAL autosampler at a temperature of 5.0°C during the analysis. Injection volume was 1 μL, injected onto a Waters ACQUITY HSS T3 C18 UHPLC column (1.8 μm, 2.1 mm x 100 mm long). The column was held at a temperature of 35.0°C. The solvent system used was Solvent A “MilliQ water with 0.1% formic acid” and Solvent B “Acetonitrile with 0.1% formic acid.” The flow rate was 0.400 mL/min with an initial solvent composition of % A = 100, % B = 0 held until 0.50 min, the solvent composition was then changed following a linear gradient until it reached % A = 5.0 and % B = 95.0 at 1.00 min. This was held until 2.79 min when the solvent was returned to the initial conditions and the column was re-equilibrated until 4.00 min. The column eluent flowed directly into the Heated ESI probe of the MS which was held at 200°C and a voltage of 4,500 V. MRM Data was collected in positive ion mode. Other MS settings were as follows, Sheath Gas Flow Rate of 50 units, Nebulizer Gas Flow Rate of 50 units, Cone Gas Flow Rate of 20 units Cone Temp was 350°C, and collision gas pressure 1 mTorr.
2.7 Data analysis and bioinformatics
The exometabolome data was visualized using Umap, standard settings. We decided to use UMAP as it is often favored over PCA due to its ability to capture non-linear relationships, preserve both local and global structure in the data, produce more distinct clusters, and better separate different groups within the data. Significance was determined with Student’s t-test. P-levels >0.005 determines significance. Outliers were identified by the interquartile method. After the outliers were identified, they were removed and the statistical analysis redone. Blast was carried out using NCBI protein blast with standard settings. For blast searches in TCDB, Blosum62 was used as scoring matrix, and cut-off values were the standard settings. Data were visualized using UMAP, and analyzed using R and JMP. Phylogenetic tree was calculated using clustal Omega (Sievers et al., 2011) and visualized using iTOL (Letunic and Bork, 2007, 2021).
3 Results
3.1 Dedicated library of potential yeast xenobiotics transporters
For expression, we selected transporter proteins from secondary active transporter superfamilies known to transport drugs and natural compounds. Bacterial transporter proteins of the drug/metabolite transporter (DMT) superfamily transport natural products and toxins, like camphor, chloroquine, and ethidium bromide (Berninsone and Hirschberg, 2000; Caffaro and Hirschberg, 2006; Västermark et al., 2011). While yeast transporters of the DMT superfamily are largely uncharacterized, we hypothesized that they could include transporters of xenobiotic compounds. The multidrug/oligosaccharide/polysaccharide (MOP) superfamily includes the multi-antimicrobial extrusion (MATE) family, which consists of well-characterized xenobiotic efflux pumps across several organisms (Hvorup et al., 2003; Tanihara et al., 2007; Staud et al., 2013). At the same time, the two MATE transporters present in the yeast genome, YDR338C and Erc1 (Shiomi et al., 1995), have not previously been characterized for the uptake of non-toxic xenobiotic compounds. We also included transporters from the major facilitator superfamily (MFS), specifically the aromatic acid exporter (ArAE) family, and the yeast Equilibrative Nucleoside Transporters (ENT) family. We further reduced the list of transporters by omitting sugar transporters and other experimentally validated nutrient transporters. We also excluded the ABC transporters, as they tend to be exporters rather than importers, and the direction of transport is normally fixed via the internal hydrolysis of ATP.
The workflow of Xenopus oocyte uptake assay is described in Figure 1A. Briefly, the oocytes were injected with mRNA encoding the transporter of interest and mRNA encoding GFP and incubated for 3 days to allow protein expression. Fluorescence was used as a quality control to select for the oocytes that received mRNA and expressed the proteins, supposedly both GFP and transporter. The transporter expressing oocytes were incubated with the solution of several selected xenobiotic metabolites in pH 5 buffer. The low pH was chosen because the yeast S. cerevisiae is known to proliferate in an acidic environment. After incubation, the concentration of xenobiotic metabolites was determined in the buffer and in the oocytes by LC–MS.
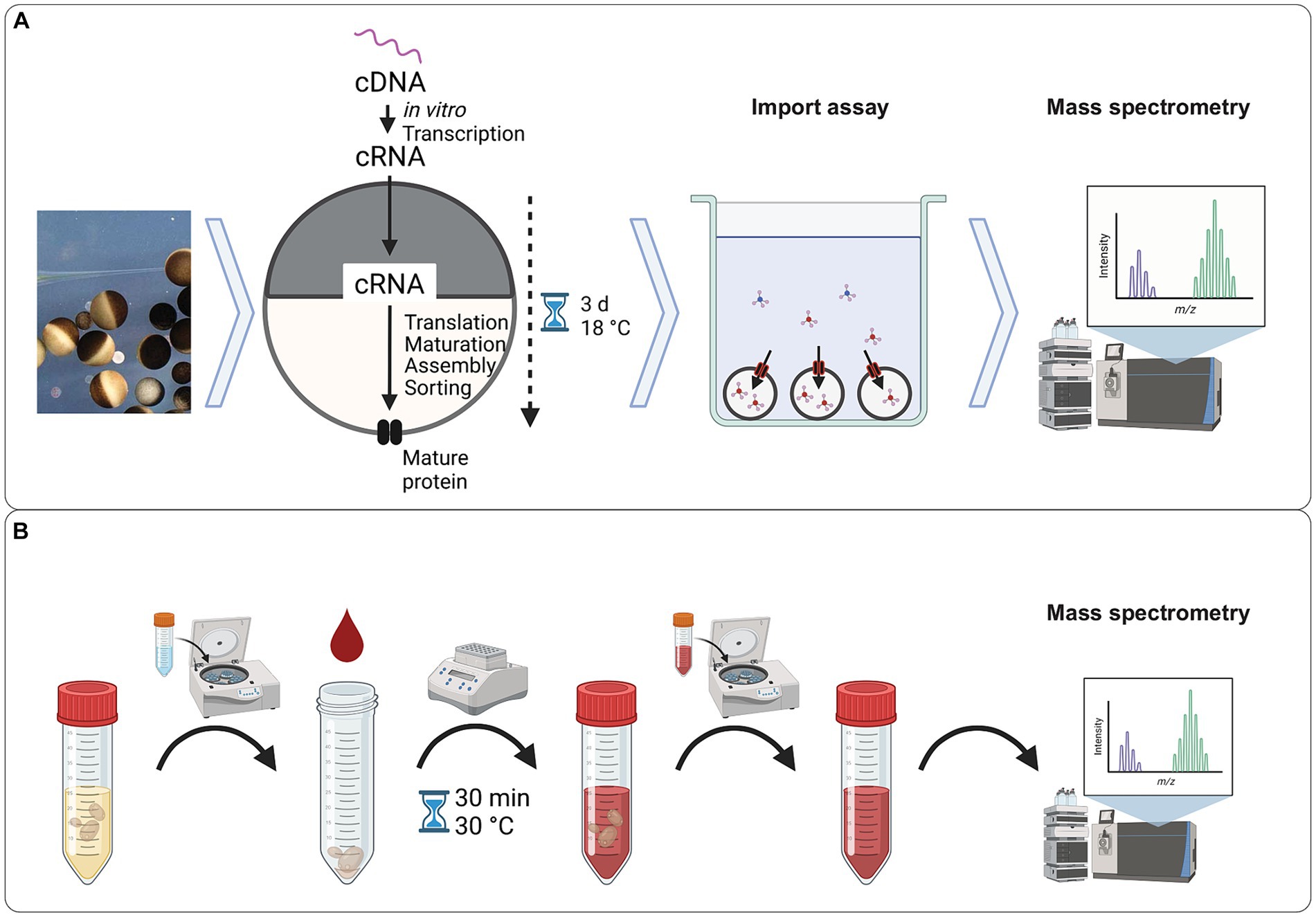
Figure 1. Experimental workflows. (A) Workflow of the uptake assay using Xenopus oocytes. Defolicated oocytes were injected with mRNA encoding the transporter of interest and mRNA encoding GFP. After 3 days of incubation at 18 °C, the fluorescence from the GFP was quantified, and oocytes with an active expression of GFP were selected for the uptake assay. Oocytes expressing the transporter of interest were exposed to a solution of the selected compounds in Kulori buffer at pH 5.0 for 3 h. After incubation, the oocytes were harvested, and the intracellular content was extracted and analyzed by LC–MS. (B) Workflow of the exometabolome assay. An overnight yeast culture was harvested by centrifugation, washed, and resuspended in human blood serum. After 30 min incubation with the human blood serum, the supernatant was harvested and subjected to analysis by LC–MS. Figure generated using BioRender.
3.2 Compounds transported by the yeast Saccharomyces cerevisiae
An initial screen to establish the small molecules that yeast cells can transport was conducted (Figure 1B). Yeast cells were incubated in human blood serum for 30 min, removed by centrifugation, and the supernatant analyzed on LC–MS. There was a concentration change (log2FC > 0.5) in 385 compounds (Supplementary Dataset S1 and Figure 2A). We observed a considerable variation in response across replicates in the yeast-treated samples compared with the untreated samples (Figure 2A), though not to the extent where the compound changed the direction of transport. The log2FC values in concentration compared to the untreated samples were predominantly negative (total of 221 compounds, 169 metabolites and 52 drugs/xenobiotics), indicating the import of compounds from the serum or the metabolism and degradation of compounds. On the other hand, the log2FC values were also positive for 164 compounds (97 metabolites and 67 drugs/xenobiotics), suggesting an increase in extracellular concentration due to the export or antiport of yeast metabolites or enzymatic activity on serum compounds (Figure 2B). The UMAP visualization showed a skewness, with compounds categorized as drugs present on the right-hand side of the U1 axis and compounds categorized as metabolites on the left (Figure 2C). This distinction in compound class has seemingly no influence on which compounds yeast interacts with, indicating that in our experiment, yeast had no substrate preference to drugs or metabolites.
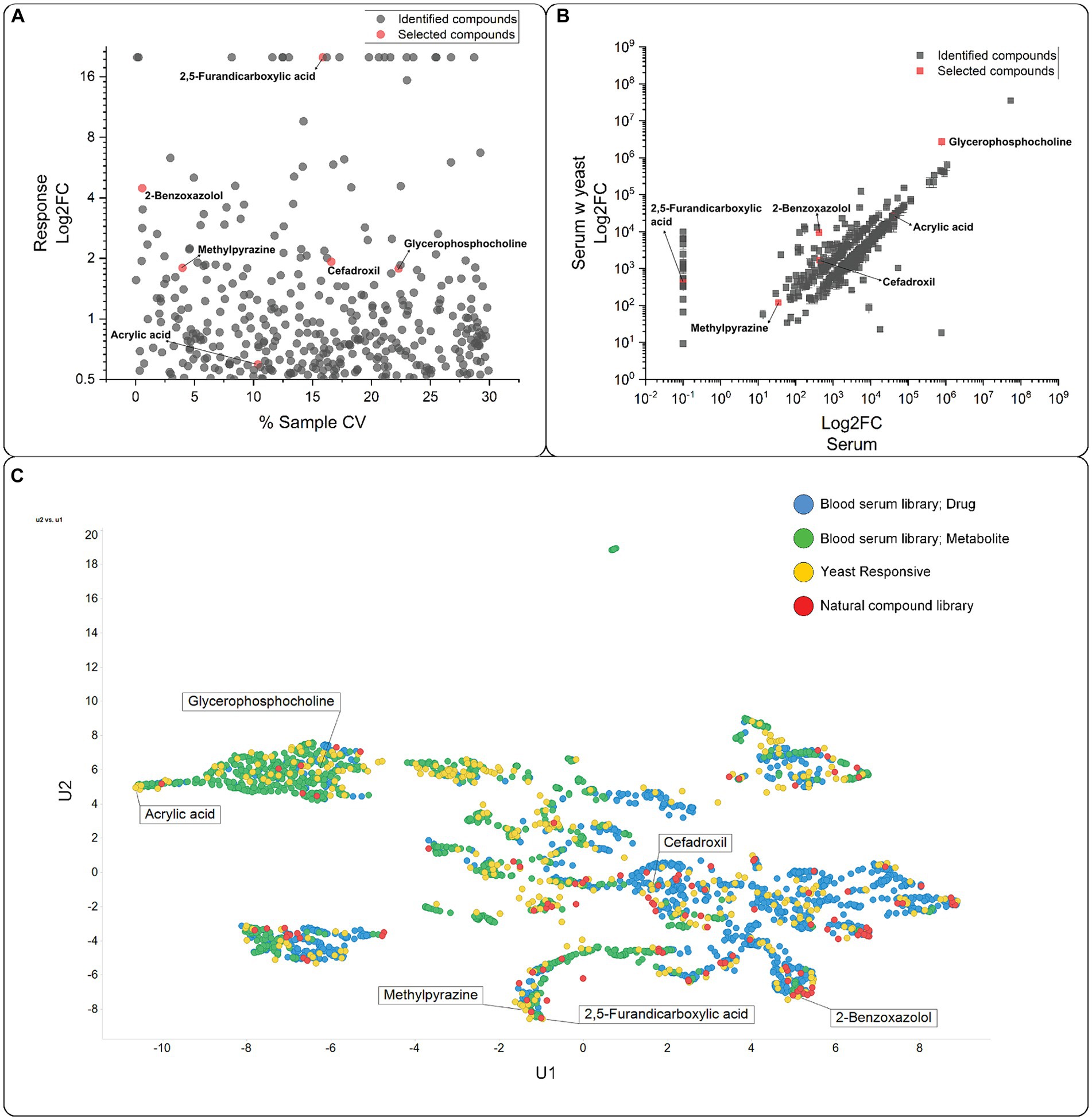
Figure 2. Compounds transported by yeast. (A) Variation observed between the repeats. CV, coefficient of variation. Compounds with a response below a log2FC = 0.5 were excluded. The selected compounds are highlighted in red. (B) The compounds identified in human blood serum were plotted against the compounds identified in the supernatant incubated with yeast. Compounds with a log2FC below 0.5 were excluded. Selected compounds are highlighted in red. (C) UMAP (McInnes et al., 2018a, 2018b) plot of the compounds with a log2FC above 0.5. Selected compounds are indicated with names. The compounds are grouped according to their classification in DrugBank 3.0 (https://www.drugbank.ca) and Recon 2 (Thiele et al., 2013) as detailed in O’Hagan and Kell (2017).
Subsequently, we overlaid the identified compounds with a log2FC > 0.5 from the exometabolome experiment data with a small natural compound library (O'Hagan and Kell, 2019) (Figure 2C). The overlay revealed that the small compounds categorized as drugs tend to have higher similarity to natural products, which was also previously described (O’Hagan and Kell, 2017), with most marketed drugs and natural products displaying a Tanimoto similarity of 0.7 or higher.
3.3 Selected compounds for characterization in transport assays in Xenopus laevis oocytes
The 385 compounds were evaluated for their commercial availability, solubility in water, and general commercial interest (see Supplementary Dataset S2). We then tested the top-ranking compounds for toxic effects on the oocytes and excluded compounds that resulted in an above-average death rate. Additionally, compounds not precisely detected by our MS method were also excluded. The six compounds selected for testing were sn-glycero-3-phosphocholine (GPC), 2,5-furandicarboxylic acid (FDC), 2-methylpyrazine (MPZ), cefadroxil (CFX), acrylic acid (ACA), and 2-benzoxazolol (BXZ). The compounds represent metabolites (GPC), compounds with natural origin (MPZ, BXZ, and FDC), and synthetic compounds (CFX and ACA). In the UMAP visualization, the six compounds are evenly distributed (Figure 2C), with MPZ and FDC being the most closely related compounds.
Both FDC and ACA are industrially relevant. FDC is one of the US Department of Energy’s top 12 compounds for establishing a biology-based chemical industry (Bozell and Petersen, 2010), while ACA is an essential monomer in the chemical industry. ACA is approved as a food contact material and acidity regulator (Silano et al., 2018). Both ACA and FDC are formed by excessive heating of food and enter the body via the diet. ACA and its precursor 3-hydropropionic acid have been produced in cell factories (Dishisha et al., 2015). CFX is a broad-spectrum antibiotic with several known transporters in humans [SLC15A1 (Ganapathy et al., 1995; Wenzel et al., 1996), SLC15A2 (Ganapathy et al., 1995; Terada et al., 1997; Ocheltree et al., 2004; Luckner and Brandsch, 2005), SLC22A5 (Ganapathy et al., 2000), SLC22A6 (Jariyawat, 2000; Jung et al., 2002; Takeda et al., 2002), SLC22A8 (Jung et al., 2002; Takeda et al., 2002)]. MPZ is a naturally occurring flavoring agent found in many foods, while BXZ is a compound naturally occurring in cereal and cereal products and rye seedlings. Lastly, GPC is a native metabolite in humans, yeast, plants, and Xenopus laevis.
3.4 Substrate identification
The six selected compounds were used to screen the transport activity of transporters expressed in Xenopus oocytes. For normalization, we used the intracellular concentration of compounds in control oocytes that expressed only GFP, but no yeast transporters.
A positive Log2FC is a measure of uptake activity compared to the control. Conversely, a negative Log2FC is a measure of export activity compared to the control (Figure 3A). The compounds were added in excess (2 mM), to ensure saturation for the transport protein was reached. Likewise, the incubation time (3 h) was chosen to ensure saturation. Export activity of transporters can be evaluated if the compound is imported into the GFP control by oocyte endogenous transporters or if the compound compromises the membrane of the oocytes and leaks in Figure 3B. Otherwise, export activity measurement would require injection of metabolites into oocytes, so called “export assay,” which was not performed in this study.
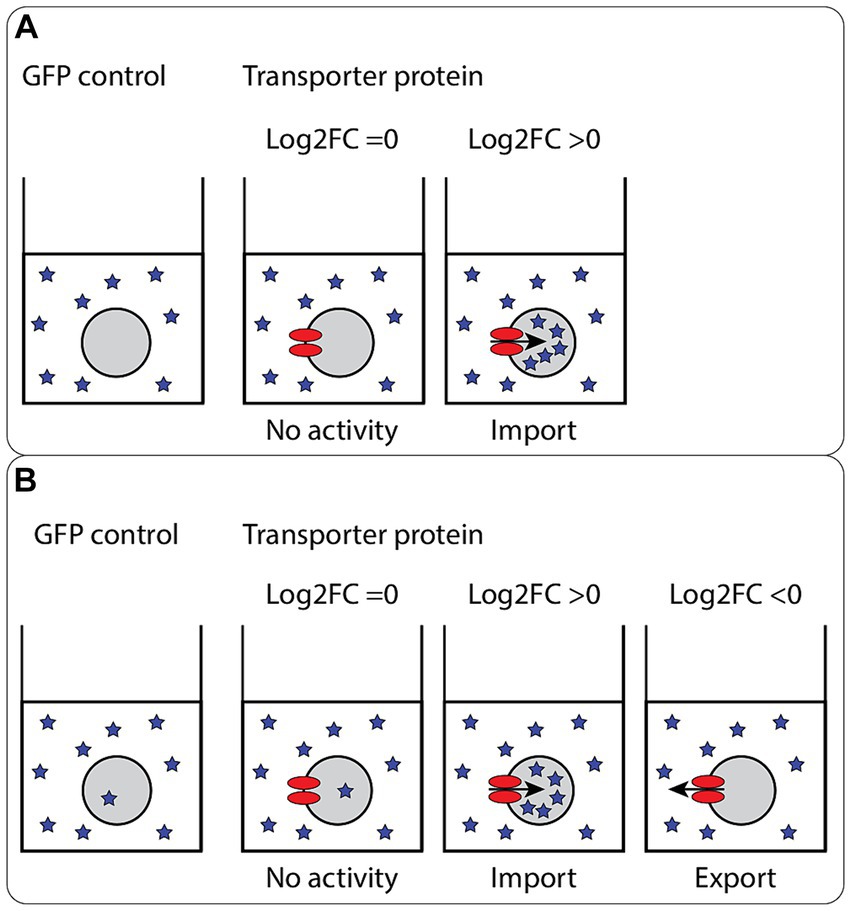
Figure 3. Principles of the oocyte import assay. Schematic representation of the oocyte import assay. For uptake, the intracellular concentration per oocyte is compared. (A) No or very little of the compounds are measured in the control oocytes. As no endogenous import activity is detected, only no activity (Log2FC = 0) or import activity (Log2FC > 0) can be detected for the expressed transporter proteins. The import can be categorized in two groups; concentrative import, where the intracellular concentration reached is higher than the concentration in the medium, and equilibrative import, where the intracellular concentration reached is similar or lower than the concentration found in the medium. (B) Some endogenous import activity is detected. With some endogenous import activity, export activity of the expressed transporter proteins can be measured (Log2FC < 0), in addition to no activity and import activity. In this scenario, two levels of import can also be reached; concentrative import and equilibrative import.
We identified transporter candidates with a significant response compared to the GFP control for all the tested compounds (Figure 4). Eight transporter candidates were identified for the three natural compounds, BXZ, MPZ, and FDC. For BXZ, the transporter proteins Rtc2, Thi74, and Ecm3 generated a significant response (Figure 4B). For MPZ, the transporter proteins Ypq2, YLR152C, and YNL095C generated a significant response (Figure 4F), and for FDC, the transporter proteins Thi74, YNL095C, Sly41, and Hut1 generated a significant response (Figure 4C). It is worth noting that Thi74 showed a negative Log2FC for BXZ, and Hut1 for FDC, suggesting Thi74 and Hut1 are exporters. All other transporters showed a positive Log2FC in intracellular concentration, suggesting the transporter proteins are importers. As prolonged incubation with BXZ and FDC did not result in increased mortality in oocytes, we do not have a reason to believe that BXZ or FDC compromised the oocyte membrane. The considerable variation between samples for Thi74 across the tested substrates could suggest difficulties with expressing Thi74 in oocytes.
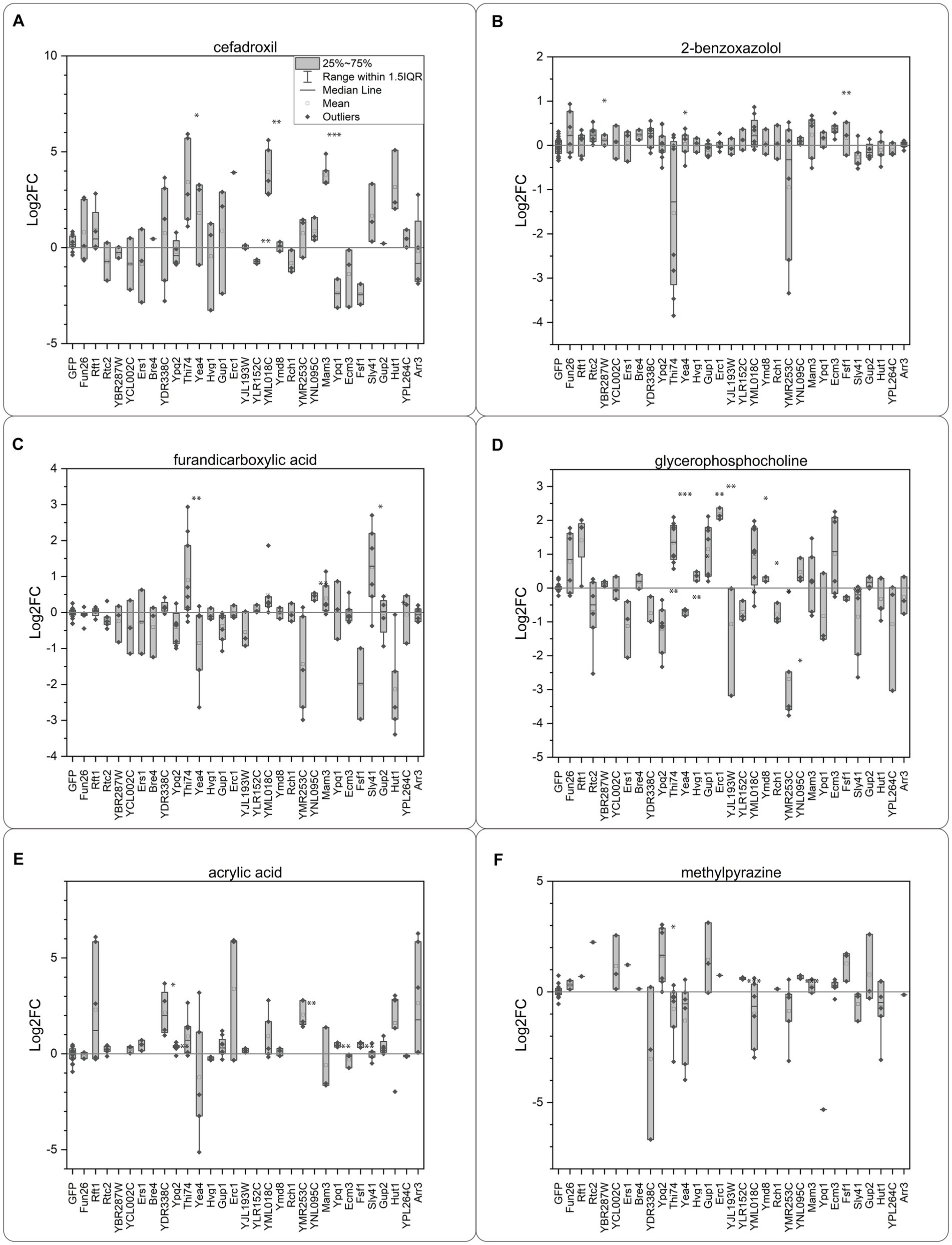
Figure 4. Oocyte import assay. Each compound was added at an extracellular concentration of 2 mM at pH 5.0. (A) Cefadroxil, (B) 2-benzoxazolol, (C) 2,5-furandicarboxylic acid, (D) sn-glycero-3-phosphocholine, (E) acrylic acid, (F) 2-methylpyrazine. The response was calculated as fold change compared to the response of the GFP injected control oocytes. Each data point consists of data from 7 to 10 oocytes in triplicates and each experiment was repeated on several occasions. The intracellular concentration in the single oocytes was calculated depending on the number of oocytes used in the individual experiment and used for calculating the Log2 fold change compared to the GFP control. Each point is a replicate based on extraction data from 10 oocytes. Significance was tested with a two-sided Students t-test, for transporters having an intracellular concentration higher than the GFP expressing controls. Significance levels indicated in the graphs are: *p ≤ 0.05, **p ≤ 0.01, ***p ≤ 0.001.
For the synthetic compounds ACA and CFX, we identified nine transporters. The transporter proteins YDR338C, Ypq2, YMR253C, Ypq1, and Fsf1, showed a significant response for ACA (Figure 4E). For CFX, we identified both proteins in the paralog pair Thi74 and YML018C as candidates, together with the transporter proteins Mam3 and YLR152C (Figure 4A).
While all the compounds mentioned were identified as substrates for between 2 and 4 transporters, the metabolite GPC was the substrate of 12 transporters (Figure 4D). We identified both paralogs Thi74 and YML018C as GPC transporter candidates, but only Gup1 from the paralog pair Gup1/Gup2. Other identified GPC candidates were Ypq2, Yea4, Hvg1, Erc1, YLR152C, Ymd8, Rch1, YMR253C, and Fsf1. Ypq2, Yea4, YLR152C, and Fsf1 all showed a negative Log2FC in intracellular concentration, suggesting the transporter proteins are exporters.
3.5 Transporter proteins allow the passage of xenobiotics in both a concentrative and equilibrative manner
To describe the thermodynamic nature of the transport mechanism of the identified transporter proteins acting as importers [i.e., equilibrative vs. concentrative (Kell and Oliver, 2014)], we compared the normalized intracellular concentration of the compounds found in the oocyte with the extracellular concentration found in the medium (Figure 5A). As the compounds were added in excess, and the volume of the medium was significantly higher than the volume of the oocytes, we assumed that the concentration found in the medium was constant for all experiments. A Log2FC > 0 suggests a concentrative mode of transport, indicating that the internal concentration within the oocytes surpasses that found in the medium. Conversely, a Log2FC < 0 indicates an equilibrative mode of transport, where the internal concentration within the oocyte does not surpass that found in the medium. The comparison revealed that only the transporters of BXZ (Ecm3, Rtc2) and ACA (Fsf1, YDR338C, YMR253C, Ypq1, and Ypq2) import their substrates in a concentrative manner (Log2FC > 0) (Figure 5B), while CFX, FDC, GPC, and MPZ as substrates are effectively imported in an equilibrative manner (Log2FC < 0) (Supplementary Table S5). However, when the GFP expressing control is taken into consideration, it becomes apparent that the transporters of BXZ and ACA have a systemic bias. For BXZ and ACA, the GFP control has a Log2FC > 0. This indicates uptake of the compounds by an endogenous transporter. While subtracting the effect from endogenous transporters suggests YDR338C and YMR253C transport ACA concentratively, further experiments are needed to verify the results.
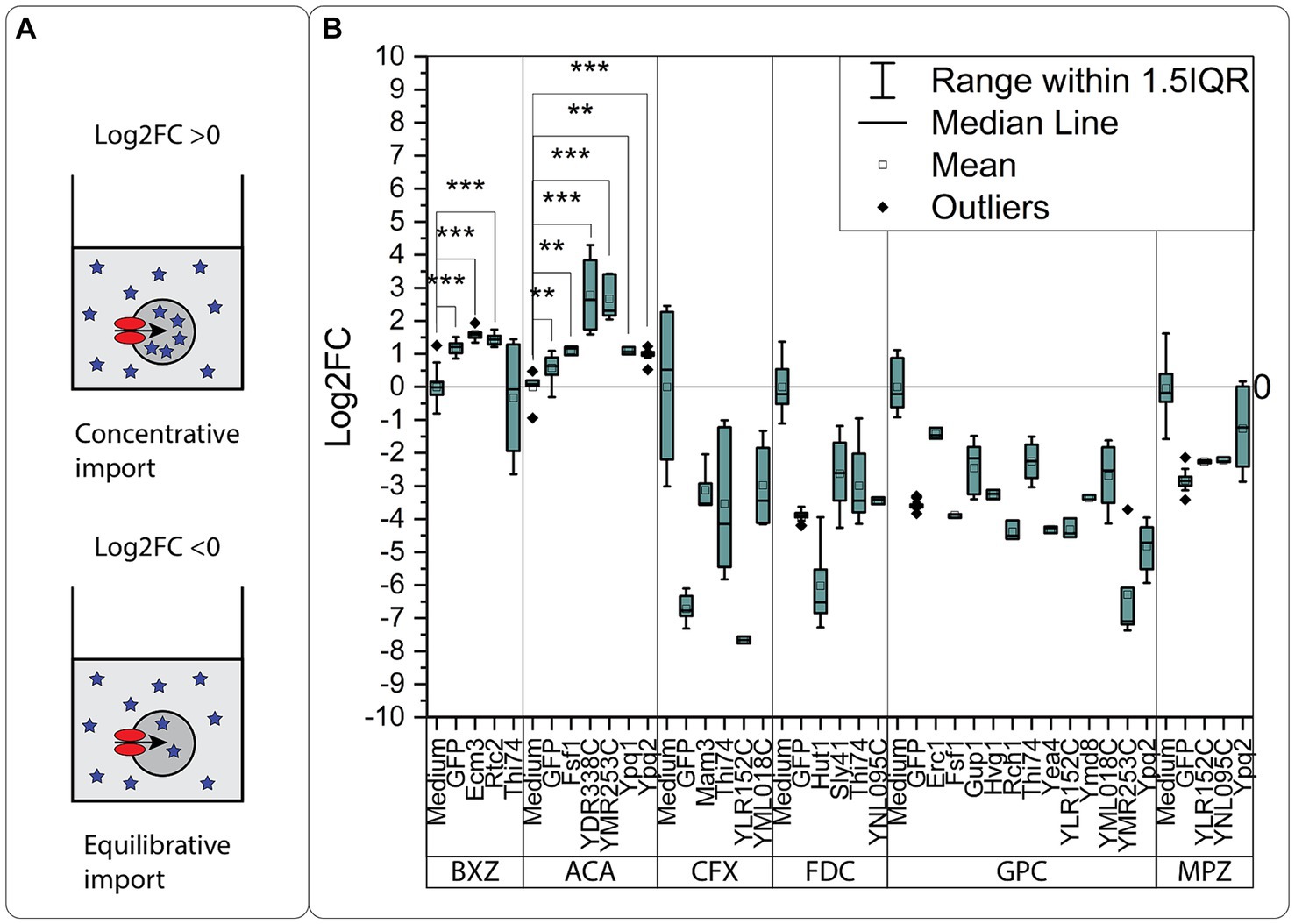
Figure 5. The mode of transport. (A) Schematic representation of the comparison with the medium concentration. A Log2FC > 0 indicates a concentrative mode of transport, as the internal concentration in the oocytes exceeds the concentration found in the medium. A Log2FC < 0 indicates an equilibrative mode of transport, as the internal concentration found in the oocyte does not exceed the concentration found in the medium. (B) The significant transporters identified in the oocyte uptake assay are plotted together with the relative concentration found in the medium. All values are calculated as Log2FC compared to the extracellular concentration found in the medium. Significance was tested with a one-sided Students t-test, for transporters having an intracellular concentration higher than the medium. Each point is a replicate based on extraction data from 10 oocytes. Significance levels indicated in the graphs are: *p ≤ 0.05, **p ≤ 0.01, ***p ≤ 0.001.
In the oocyte uptake assay, we identified GPC as the substrate for the highest number of transporters (12). The comparison with the concentration found in the medium revealed that all transporters transported GPC equilibratively. We previously observed that GPC could enter the oocyte through an unknown mechanism, possibly through leakage or through an endogenous transporter. We rule out the possibility that the oocytes are compromised in general by GPC, as we did not detect all six compounds intracellularly in the GFP-expressing control oocytes.
If GPC is excluded, all concentrative transporters but Ypq2 transported only one compound (ACA), strictly in a concentrative manner. The remaining equilibrative transporters transported from one substrate (Sly41/FDC) up to three substrates for Thi74 (BXZ, CFX, FDC). The concentrative nature of the ACA transporters would suggest they are either primary or secondary active transporters, able kinetically and thermodynamically to transport their substrate in a uni-directional way under the conditions tested.
Examining the direction of transport found in the data from the exometabolite assay, ACA was found to be effectively imported rather than exported. Our results suggest that ACA, in general, is imported into the cell by the transporters YMR253C, YDR338C, Fsf1, Ypq2, and Ypq1, where YMR253C and YDR338C potentially transport ACA in a concentrative manner. When further assessing the oocyte uptake data, we identified Thi74 as an exporter of BXZ rather than an importer. In the exometabolite assay, we had identified BXZ to be effectively exported, meaning either Ecm3 and/or Rtc2 transports BXZ in a bi-directional manner, or other transporters exist, including Thi74. For CFX, FDC, and MPZ, all three compounds were found to be effectively exported in the exometabolome assay. In the oocyte uptake assay, we had a higher extracellular concentration, identifying solely equilibrative transport for CFX, FDC and MPZ.
Overall, we found the equilibrative transport of compounds to be a widespread phenomenon among the tested transporters and more common than concentrative transport. Moreover, we found that the ability to transport a compound concentratively was not restricted to a specific compound class, though assessing a larger chemical library is needed to investigate the phenomenon further.
3.6 Effect of transporter deletions in Saccharomyces cerevisiae
We further tested the effect of the identified transporters from the oocyte screen in vivo by growing S. cerevisiae strains harboring deletions of the single genes in medium with the supplementation of one of the six selected compounds. The strains were grown at the LC50 concentration of the compounds, to allow both improvements in growth and growth inhibitions to be detectible (the data forming the basis for the LC50 calculations is available in Supplementary Table S6). We were not able to obtain a growth defect approximating to 50% for the reference BY4741 strain grown in SD-Trp supplemented with GPC or CFX. Therefore, we omitted the two compounds from the final growth experiment. We also omitted FDC from the final growth experiment, as the compounds precipitated at the calculated LC50, which biased the results. Using the calculated LC50 concentrations for ACA, BXZ and MPZ, strains harboring deletions of the identified transporters were cultivated in SD-Trp with and without the compounds in a growth profiler, the growth curves can be found in Supplementary Figures S1–S4. Figure 6 shows the calculated maximum OD (Max OD) and the maximum specific growth rate (μmax) using the growth curves For acrylic acid, the strain harboring a deletion of FSF1 (fsf1∆), showed a decrease in μmax rate when grown in SD-Trp (Figure 6A). The decrease in growth rate was rescued in the presence of acrylic acid. In addition, there was no difference in maximum OD for the ACA transporters deletion strains in SD-Trp, while when grown in the presence of ACA, all the ACA transporters deletion strains had a significantly lower maximum OD compared to the wildtype strain (Figure 6A).
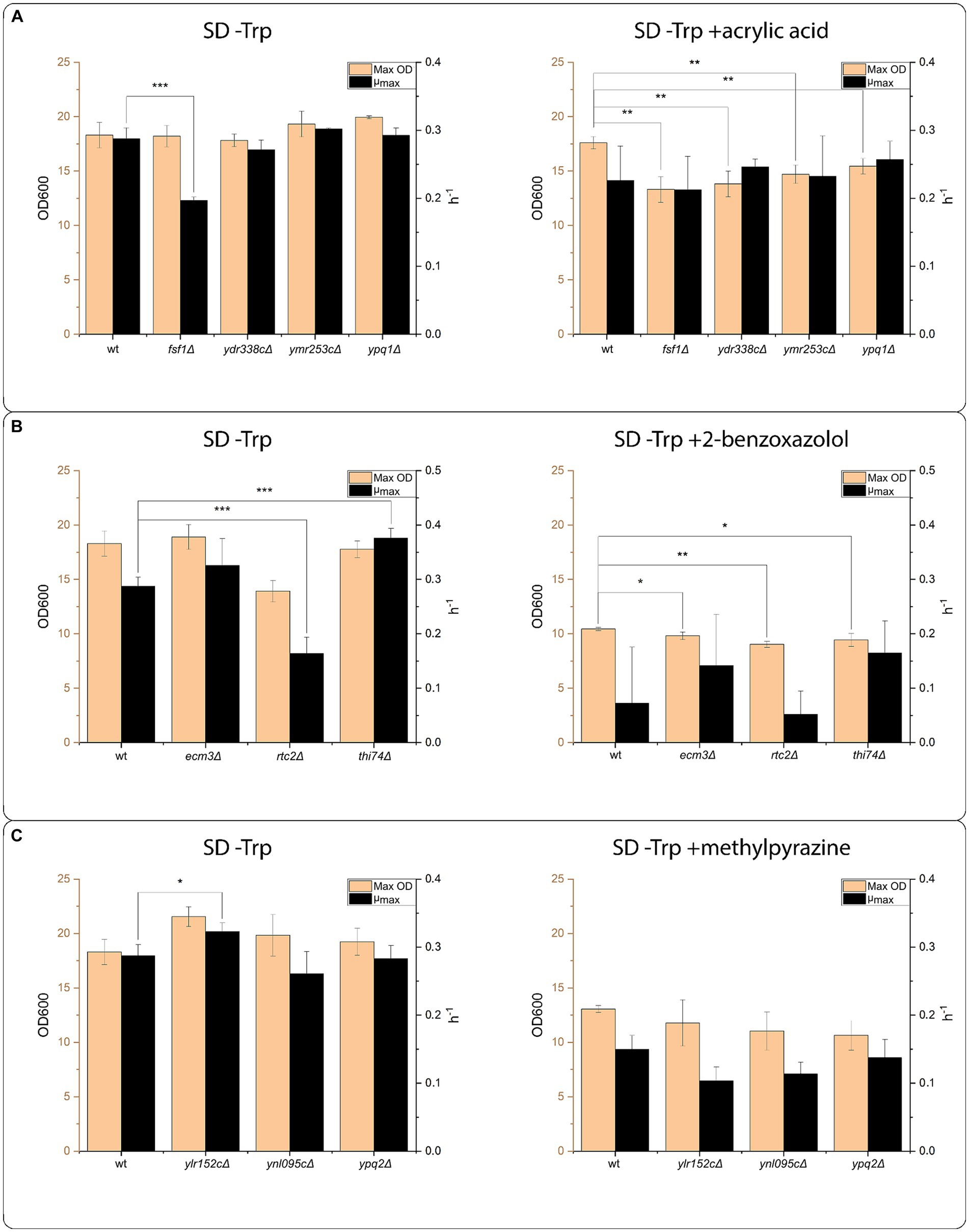
Figure 6. Transporter mutant assays. Growth assay of strains with the transporter proteins identified in the screening effort. From the growth curves generated in the growth profiler, maximum OD reached (Max OD), and maximum specific growth rate (μmax) were calculated. All strains were grown in the medium composition SD –Trp, and in SD –Trp supplemented with the indicated compounds at LC50 concentration. The LC50 concentration was established in a pre-experiment using the wildtype BY4741 strain. (A) Growth assay of strains harboring deletions of transporters genes identified in the screening effort as transporter proteins having transport activity toward acrylic acid. The assay was conducted with 2.45 mM acrylic acid. (B) Growth assay of strains harboring deletions of transporters genes identified in the screening effort as transporter proteins having transport activity toward 2-benzoxazolol. The assay was conducted with 6.44 mM 2-benzoxazolol. (C) Growth assay of strains harboring deletions of transporters genes identified in the screening effort as transporter proteins having transport activity toward methylpyrazine. The assay was conducted with 155.15 mM methylpyrazine. Significance levels indicated in the graphs are: *p ≤ 0.05, **p ≤ 0.01, ***p ≤ 0.001.
For BXZ transporters, rtc2∆ mutant had lower μmax and thi74∆ had higher μmax in SD-Trp than the reference strain (Figure 6B). In the presence of BXZ, neither of the three strains tested had a significant difference in μmax, while all tested strains had a significant decrease in maximum OD. For MPZ transporters, only YLR152C∆ exhibited a growth phenotype (Figure 6C). YLR152C∆ showed a significant increase in μmax compared to the reference when grown on SD-Trp, while this μmax was not significantly different from the reference when grown in the presence of MPZ (Figure 6C).
3.7 Sequence-function relationship
For transporters, divergent evolution is overwhelmingly the most common means of evolution, i.e., similar sequences evolve to transport different substrates (Saier, 1994; Wang et al., 2016; Jørgensen et al., 2017). To look into the conservation of substrate specificity among the identified transporters, we generated a phylogenetic tree as well as assessed their transporter classification for indications of a potential co-substrate.
Among the chosen transporters were a few pairs of paralogs with a considerable sequence similarity: Thi74/YML018C, Rtc2/Ypq1, Gup1/Gup2, Ecm3/YNL095C (Figure 7). For Thi74 and YML018C, we identified CFX and GPC as shared substrates, while FDC and BXZ were unique to Thi74. Both Rtc2/Ypq1 and Gup1/Gup2 failed to show an overlap in the substrate. The lack of functional overlap for the paralog pairs could suggest evolutional diversification through relatively few mutations. Indeed, paralog transporters have been previously shown to have different substrate specificities (Ramaswamy et al., 2017; Jiang et al., 2023). Consequently, the substrate of a transporter protein is hard to predict based on the amino acid sequence alone. There was no clear correlation between sequence similarity and substrate specificity in our study.
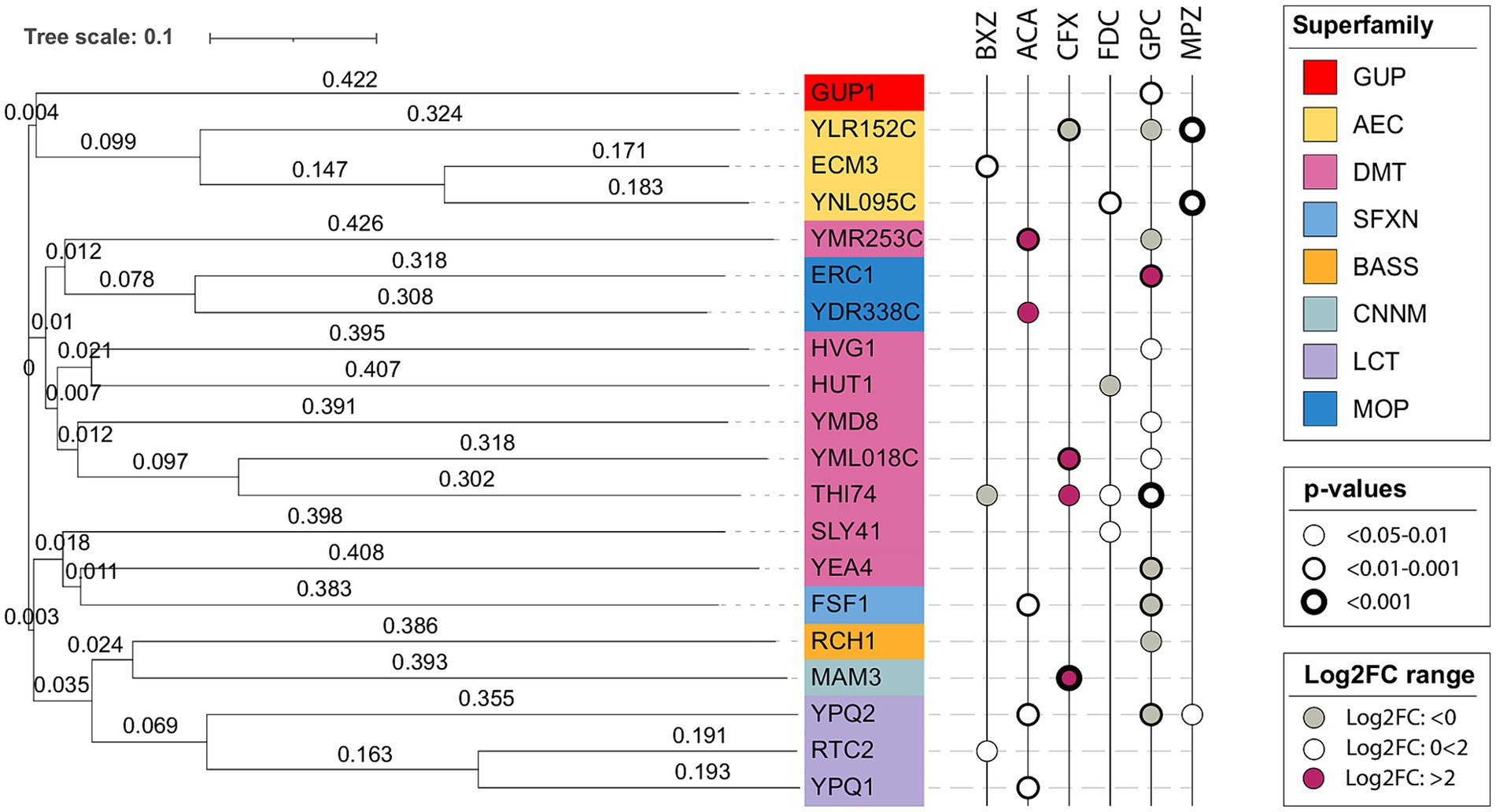
Figure 7. Phylogenetic tree of tested transporters with identified substrates. The tree was generated with Clustal Omega (Sievers et al., 2011) version 1.2.4 using the standard parameters. The generated phylogenetic tree was visualized with iTOL (Letunic and Bork, 2007, 2021). The transporter proteins are color-coded according to superfamily. The transporter superfamilies are abbreviated as follows: the glycerol uptake (GUP) superfamily, the auxin efflux carrier (AEC) family from the bile/arsenite/riboflavin transporter (BART) superfamily, drug/metabolite transporter (DMT) superfamily, the sideroflexin (SFXN) family, the bile acid: Na+ symporter (BASS) family, the cyclin M Mg2+ exporter (CNNM) family, the lysosomal cystine transporter (LCT) family, the multidrug/oligosaccharidyl-lipid/polysaccharide (MOP) flippase superfamily.
As a means of further validating our results, we sought to verify the presence of co-substrates for the transporter families. The presence of a co-substrate would indicate whether there is a known source of electrochemical power and hence would suggest the transporter can transport substrates concentratively. Assessing the identified transporters’ TCDB classification (Saier, 2006; Saier et al., 2016) did not give any further insight. All transporters, except Mam3, belong to the pores transporter class, including both symporters, uniporters, and antiporters (Supplementary Table S7), giving no additional information concerning potential co-substrates.
4 Discussion
We applied a workflow combining exometabolome analysis and Xenopus expression to map substrate-transporter relationships for six metabolites and 30 yeast transporters. The fact that we could identify transporters for all selected compounds was surprising considering that we only tested 30 transporters, which comprises only a tenth of all the yeast transporters. Of the 20 transporter proteins identified in this screen, Sly41, YML018C, Mam3, and Ecm3 have not previously been assigned a substrate experimentally.
In transporter mutant assays in S. cerevisiae we observed a growth phenotype for most of the strains, which further strengths the screening approach using Xenopus oocytes. We did however not observe a growth phenotype for all the tested transporters. The lack of growth phenotype could be due to the localization of the transporter. If the transporter and the compound are not localized within the same cellular compartment, their interaction (transport) cannot occur, and consequently, observable effects may not manifest. However, it does not exclude the transporter protein from having activity against the compound. The observed growth phenotype of the transporter deletion strains indicates the transporters are involved in resistance toward ACA, BXZ and MPZ (though only Ylr152c) at the tested concentration. The observed alleviation of the slower growth rate for FSF1∆ in the presence of ACA, and RTC2∆ for BXZ could imply a potential association of Fsf1 and Rtc2 with the import or availability of these compounds. Fsf1, identified as a mitochondrial protein (Reinders et al., 2006), may likely contribute to the availability of ACA at its intramitochondrial target, where it may exert its toxic effects, rather than being directly involved in its import into the cell. Similarly, Rtc2, proposed as a probable vacuolar protein (Jézégou et al., 2012), aligns with a potential role in facilitating the availability of BXZ in the cytoplasm rather than its direct import. However, the exact relationship between the function and the subcellular localization of these transporters warrants further investigation for a comprehensive understanding.
The significant decrease in maximum OD when the strains are grown in the presence of the compound is in line with a function in export of the compound, or making the compound unavailable at the site of action. This is the case for Ydr338c, ymr253c and Ypq1, for ACA, and Ecm3, Rtc2 and Thi74 for BXZ. For THI74∆ on BXZ and YLR152C∆ on MPZ, the μmax was significantly higher than the wildtype when grown on SD-Trp, while when grown in the presence of the appropriate compound, the μmax was not significantly different. Thi74 is a mitochondrial carrier (Mojzita and Hohmann, 2006) which corresponds well with a function in making BXZ unavailable at the site of action. While Thi74 exhibited bi-directional transport depending on the substrate in the oocyte experiments, similar activities have been observed in other transporters (Lai, 2013). Ylr152c is a protein of unknown function meaning the observed decrease in μmax in the presence of the compounds could suggest Ylr152c is involved in exporting MPZ, or making MPZ unavailable at the site of action.
While we identified a range of transporter proteins for the tested substrates, we only found six transporter proteins to transport their substrates with a Log2FC above two. This suggests there are many solute carriers with the capacity to transport xenobiotics from many different chemical classes when the compounds are present in high concentrations, while the capacity to transport the compounds at an efficiency above Log2FC is much rarer. The presence of multiple promiscuous transporter proteins in yeast allows the passage of a diverse range of compounds found in the environment. Thereby the yeast cell can ‘sample’ its environment without energy expenditure in the form of ATP or electrochemical gradients. As the transport is equilibrative, the compounds will not accumulate inside the cell. In case of toxicity, the yeast possesses a variety of ATP-driven ABC transporters for efficient multidrug efflux (Egner and Kuchler, 1996; Ortiz et al., 1997; Prasad and Goffeau, 2012; Liu et al., 2023).
The GPC was the most utilized substrate, with 12 transporter proteins showing activity toward GPC. However, more validation of these results is needed because GPC may have impacted the oocyte membrane or had some other secondary effect. Phospholipids, such as GPC, have been shown to alter the function of specific transporter proteins (Stieger et al., 2021). In this context, alternative in vitro assays, particularly those employing liposomes and solid-supported membrane-based electrophysiology, represent invaluable tools for comprehensive transporter characterization (Groeneveld et al., 2010; Bazzone et al., 2016, 2017). These methodologies facilitate in-depth investigations into transport mechanisms, substrate specificity, pH dependency, and other critical parameters. Such systems hold promise for further validating or exploring perplexing findings observed with challenging-to-express transporters in oocytes, such as Thi74, and confirming differential substrate specificities observed in transporter paralogs. Furthermore, they could provide avenues to explore aspects such as the absence of structural similarity between our identified and already known substrates and the lack of clear relationships between transporter families and metabolite structures. The prevalence of promiscuous equilibrative transporters has significant implications for strain development in biotechnology. Transporter engineering has shown notable improvements in yeast cell factories for various compounds such as resveratrol (Pan et al., 2008; Vos et al., 2015), squalene (Liu et al., 2023), muconic acid (Pereira et al., 2019; Wang et al., 2021), and betaxanthin (Wang et al., 2021). Several reviews provide additional insights into this progress [see Kell et al. (2015), Jezierska and Van Bogaert (2017), Kell (2018), van der Hoek and Borodina (2020), and Belew et al. (2022)]. However, in certain cases, transporter engineering did not achieve the desired outcomes. For example, with ergothioneine (van der Hoek et al., 2019, 2022) and lactic acid (Fox et al., 2000; Branduardi et al., 2006; Pacheco et al., 2012; Baek et al., 2016; Baldi et al., 2021). The lack of consistent success with transport engineering could be explained by sufficient non-specific secretion of the products by multiple native transporters through equilibrative mechanism, as the product concentration inside the producing cells would typically be higher than the extracellular concentration. The effective extracellular concentration of the product can also be decreased by precipitation or by biphasic fermentation, thus maintaining the product concentration gradient that promotes secretion even when high amounts of product are present in the medium. Resveratrol and muconic acid have low solubility in water and readily precipitate during the process. Squalene is a water-insoluble terpenoid that requires a biphasic fermentation to maintain high titers (Liu et al., 2023). Notably though, if transport can be changed to happen with higher energetic efficiency, then significant improvements in the cell factories by transporter engineering can be achieved. The production of L-glutamic acid (used as taste enhancer monosodium glutamate) was achieved in the 50s’ by corynebacteria when process conditions were discovered that activated a mechanosensitive channel for glutamate export (Nakamura et al., 2007; Sano, 2009). Another example is the discovery of Schizosaccharomyces pombe malic acid transporter MAE1 which significantly improved the secretion of dicarboxylic acids in yeast cell factories (Jansen et al., 2012). It was later found that this transporter was likely a voltage-dependent slow-anion channel operating with high energy efficiency, without the use of proton or Na+ motive force (Darbani et al., 2019).
In summary, our study provides insights into high transporter promiscuity when it comes to equilibrative transport. This promiscuity has implications both for drug development and for biotechnology and calls for further studies of the phenomenon.
Data availability statement
The original contributions presented in the study are included in the article/Supplementary material, further inquiries can be directed to the corresponding author.
Ethics statement
Ethical approval was not required for the studies on animals in accordance with the local legislation and institutional requirements because only commercially available established cell lines were used.
Author contributions
IM-H: Writing – original draft, Writing – review & editing, Conceptualization, Formal analysis, Investigation, Visualization. JS-S: Writing – review & editing, Investigation. SH: Investigation, Writing – review & editing. JD: Formal analysis, Writing – review & editing. HC: Formal analysis, Writing – review & editing. MW: Formal analysis, Writing – review & editing. SO’H: Visualization, Writing – review & editing. DK: Conceptualization, Funding acquisition, Supervision, Writing – review & editing. IB: Conceptualization, Funding acquisition, Supervision, Writing – review & editing.
Funding
The author(s) declare financial support was received for the research, authorship, and/or publication of this article. This project has received funding from the European Research Council under the European Union’s Horizon 2020 research and innovation program (YEAST-TRANS project, Grant Agreement No. 757384) and from the Novo Nordisk Foundation (Grant Agreement No. NNF20CC0035580, NNF20OC0060809, and NNF21OC0072559). DK and MW also thank the BBSRC (grant BB/P009042/1) for financial support.
Acknowledgments
IM-H thanks Behrooz Darbani for technical assistance and advice with Roboinject.
Conflict of interest
The authors declare that the research was conducted in the absence of any commercial or financial relationships that could be construed as a potential conflict of interest.
Publisher’s note
All claims expressed in this article are solely those of the authors and do not necessarily represent those of their affiliated organizations, or those of the publisher, the editors and the reviewers. Any product that may be evaluated in this article, or claim that may be made by its manufacturer, is not guaranteed or endorsed by the publisher.
Supplementary material
The Supplementary material for this article can be found online at: https://www.frontiersin.org/articles/10.3389/fmicb.2024.1376653/full#supplementary-material
References
Alberts, B., Johnson, A., Lewis, J., Raff, M., Roberts, K., and Walter, P. (2002) ‘Carrier proteins and active membrane transport’, in molecular biology of the cell. 4th. New York, Garland Science.
Almeida, L. D., Silva, A. S. F., Mota, D. C., Vasconcelos, A. A., Camargo, A. P., Pires, G. S., et al. (2021). Yeast double transporter gene deletion library for identification of xenobiotic carriers in low or high throughput. MBio 12:e03221. doi: 10.1128/mbio.03221-21
Baek, S.-H., Kwon, E. Y., Kim, S. Y., and Hahn, J. S. (2016). GSF2 deletion increases lactic acid production by alleviating glucose repression in Saccharomyces cerevisiae. Sci. Rep. 6:34812. doi: 10.1038/srep34812
Baldi, N., de Valk, S. C., Sousa-Silva, M., Casal, M., Soares-Silva, I., and Mans, R. (2021). Evolutionary engineering reveals amino acid substitutions in Ato2 and Ato3 that allow improved growth of Saccharomyces cerevisiae on lactic acid. FEMS Yeast Res. 21:foab033. doi: 10.1093/femsyr/foab033
Bazzone, A., Barthmes, M., and Fendler, K. (2017). “SSM-based electrophysiology for transporter research” in Methods in enzymology (Elsevier), 31–83.
Bazzone, A., Madej, M. G., Kaback, H. R., and Fendler, K. (2016). pH regulation of Electrogenic sugar/H+ symport in MFS sugar permeases. PLoS One 11, 11:e0156392. doi: 10.1371/journal.pone.0156392
Belew, Z. M., Poborsky, M., Nour-Eldin, H. H., and Halkier, B. A. (2022). Transport engineering in microbial cell factories producing plant-specialized metabolites. Curr. Opin. Green Sustain. Chem. 33:100576. doi: 10.1016/j.cogsc.2021.100576
Berninsone, P. M., and Hirschberg, C. B. (2000). Nucleotide sugar transporters of the Golgi apparatus. Curr. Opin. Struct. Biol. 10, 542–547. doi: 10.1016/S0959-440X(00)00128-7
Bozell, J. J., and Petersen, G. R. (2010). Technology development for the production of biobased products from biorefinery carbohydrates—the US Department of Energy’s “top 10” revisited. Green Chem. 12:539. doi: 10.1039/b922014c
Branduardi, P., Sauer, M., de Gioia, L., Zampella, G., Valli, M., Mattanovich, D., et al. (2006). Lactate production yield from engineered yeasts is dependent from the host background, the lactate dehydrogenase source and the lactate export. Microb. Cell Fact. 5:4. doi: 10.1186/1475-2859-5-4
Caffaro, C. E., and Hirschberg, C. B. (2006). Nucleotide sugar transporters of the Golgi apparatus: from basic science to diseases. Acc. Chem. Res. 39, 805–812. doi: 10.1021/ar0400239
César-Razquin, A., Snijder, B., Frappier-Brinton, T., Isserlin, R., Gyimesi, G., Bai, X., et al. (2015). A call for systematic research on solute carriers. Cell 162, 478–487. doi: 10.1016/j.cell.2015.07.022
Darbani, B., Kell, D. B., and Borodina, I. (2018). Energetic evolution of cellular Transportomes. BMC Genomics 19:418. doi: 10.1186/s12864-018-4816-5
Darbani, B., Stovicek, V., van der Hoek, S. A., and Borodina, I. (2019). Engineering energetically efficient transport of dicarboxylic acids in yeast Saccharomyces cerevisiae. Proc. Natl. Acad. Sci. 116, 19415–19420. doi: 10.1073/pnas.1900287116
Dishisha, T., Pyo, S.-H., and Hatti-Kaul, R. (2015). Bio-based 3-hydroxypropionic- and acrylic acid production from biodiesel glycerol via integrated microbial and chemical catalysis. Microb. Cell Fact. 14:200. doi: 10.1186/s12934-015-0388-0
Dunn, W. B., Broadhurst, D., Begley, P., Zelena, E., Francis-McIntyre, S., Anderson, N., et al. (2011). Procedures for large-scale metabolic profiling of serum and plasma using gas chromatography and liquid chromatography coupled to mass spectrometry. Nat. Protoc. 6, 1060–1083. doi: 10.1038/nprot.2011.335
Egner, R., and Kuchler, K. (1996). The yeast multidrug transporter Pdr5 of the plasma membrane is ubiquitinated prior to endocytosis and degradation in the vacuole. FEBS Lett. 378, 177–181. doi: 10.1016/0014-5793(95)01450-0
Fairweather, S. J., Okada, S., Gauthier-Coles, G., Javed, K., Bröer, A., and Bröer, S. (2021). A GC-MS/single-cell method to evaluate membrane transporter substrate specificity and signaling. Front. Mol. Biosci. 8:646574. doi: 10.3389/fmolb.2021.646574
Fox, J. E. M., Meredith, D., and Halestrap, A. P. (2000). Characterisation of human monocarboxylate transporter 4 substantiates its role in lactic acid efflux from skeletal muscle. J. Physiol. 529, 285–293. doi: 10.1111/j.1469-7793.2000.00285.x
Ganapathy, M. E., Brandsch, M., Prasad, P. D., Ganapathy, V., and Leibach, F. H. (1995). Differential recognition of β-lactam antibiotics by intestinal and renal peptide transporters, PEPT 1 and PEPT 2. J. Biol. Chem. 270, 25672–25677. doi: 10.1074/jbc.270.43.25672
Ganapathy, M. E., Huang, W., Rajan, D. P., Carter, A. L., Sugawara, M., Iseki, K., et al. (2000). β-Lactam antibiotics as substrates for OCTN2, an organic cation/carnitine transporter. J. Biol. Chem. 275, 1699–1707. doi: 10.1074/jbc.275.3.1699
Giaever, G., Chu, A. M., Ni, L., Connelly, C., Riles, L., Véronneau, S., et al. (2002). Functional profiling of the Saccharomyces cerevisiae genome. Nature 418, 387–391. doi: 10.1038/nature00935
Girardi, E., César-Razquin, A., Lindinger, S., Papakostas, K., Konecka, J., Hemmerich, J., et al. (2020). A widespread role for SLC transmembrane transporters in resistance to cytotoxic drugs. Nat. Chem. Biol. 16, 469–478. doi: 10.1038/s41589-020-0483-3
Groeneveld, M., Detert Oude Weme, R. G. J., Duurkens, R. H., and Slotboom, D. J. (2010). Biochemical characterization of the C4 -dicarboxylate transporter DctA from Bacillus subtilis. J. Bacteriol. 192, 2900–2907. doi: 10.1128/JB.00136-10
Gründemann, D., Harlfinger, S., Golz, S., Geerts, A., Lazar, A., Berkels, R., et al. (2005). Discovery of the ergothioneine transporter. Proc. Natl. Acad. Sci. 102, 5256–5261. doi: 10.1073/pnas.0408624102
Hvorup, R. N., Winnen, B., Chang, A. B., Jiang, Y., Zhou, X. F., and Saier, M. H. Jr. (2003). The multidrug/oligosaccharidyl-lipid/polysaccharide (MOP) exporter superfamily. Eur. J. Biochem. 270, 799–813. doi: 10.1046/j.1432-1033.2003.03418.x
Jansen, M. L. A., et al. (2012) Dicarboxylic acid production process. Available at: https://patents.google.com/patent/WO2012038390A1/en (Accessed: January 15, 2024).
Jariyawat, S. (2000). The interaction and transport of β-lactam antibiotics with the cloned rat renal organic anion transporter 1. J-Stage 4, 653–654. doi: 10.11434/kyorinmed.31.653
Jézégou, A., Llinares, E., Anne, C., Kieffer-Jaquinod, S., O’Regan, S., Aupetit, J., et al. (2012). Heptahelical protein PQLC2 is a lysosomal cationic amino acid exporter underlying the action of cysteamine in cystinosis therapy. Proc. Natl. Acad. Sci. 109, E3434–E3443. doi: 10.1073/pnas.1211198109
Jezierska, S., and Van Bogaert, I. N. A. (2017). Crossing boundaries: the importance of cellular membranes in industrial biotechnology. J. Ind. Microbiol. Biotechnol. 44, 721–733. doi: 10.1007/s10295-016-1858-z
Jiang, Y., Li, Z., Sui, D., Sharma, G., Wang, T., MacRenaris, K., et al. (2023). Rational engineering of an elevator-type metal transporter ZIP8 reveals a conditional selectivity filter critically involved in determining substrate specificity. Commun. Biol. 6:778. doi: 10.1038/s42003-023-05146-w
Jørgensen, M. E., Xu, D., Crocoll, C., Ernst, H. A., Ramírez, D., Motawia, M. S., et al. (2017). Origin and evolution of transporter substrate specificity within the NPF family. eLife 6:e19466. doi: 10.7554/eLife.19466
Jung, K. Y., Takeda, M., Shimoda, M., Narikawa, S., Tojo, A., Kim, D. K., et al. (2002). Involvement of rat organic anion transporter 3 (rOAT3) in cephaloridine-induced nephrotoxicity: in comparison with rOAT1. Life Sci. 70, 1861–1874. doi: 10.1016/S0024-3205(02)01500-X
Kell, D. B. (2018) Control of metabolite efflux in microbial cell factories, In Fermentation microbiology and biotechnology, fourth edition. 1st. 4th. eds. E. M. T. El-Mansi, J. Nielsen, D. Mousdale, and R. P. Carlson. Boca Raton: Taylor & Francis, 2018: CRC Press.
Kell, D. B., Dobson, P. D., and Oliver, S. G. (2011). Pharmaceutical drug transport: the issues and the implications that it is essentially carrier-mediated only. Drug Discov. Today 16, 704–714. doi: 10.1016/j.drudis.2011.05.010
Kell, D. B., and Oliver, S. G. (2014). How drugs get into cells: tested and testable predictions to help discriminate between transporter-mediated uptake and lipoidal bilayer diffusion. Front. Pharmacol. 5:231. doi: 10.3389/fphar.2014.00231
Kell, D. B., Swainston, N., Pir, P., and Oliver, S. G. (2015). Membrane transporter engineering in industrial biotechnology and whole cell biocatalysis. Trends Biotechnol. 33, 237–246. doi: 10.1016/j.tibtech.2015.02.001
Lai, Y. (2013). “Organic anion, organic cation and zwitterion transporters of the SLC22 and SLC47 superfamily (OATs, OCTs, OCTNs and MATEs)” in Transporters in drug discovery and development. (Sawston, United Kingdom: Elsevier), 455–631.
Letunic, I., and Bork, P. (2007). Interactive tree of life (iTOL): an online tool for phylogenetic tree display and annotation. Bioinformatics 23, 127–128. doi: 10.1093/bioinformatics/btl529
Letunic, I., and Bork, P. (2021). Interactive tree of life (iTOL) v5: an online tool for phylogenetic tree display and annotation. Nucleic Acids Res. 49, W293–W296. doi: 10.1093/nar/gkab301
Liu, J., Wang, X., Jin, K., Liu, Y., Li, J., du, G., et al. (2023). In silico prediction and Mining of Exporters for secretory bioproduction of Terpenoids in Saccharomyces cerevisiae. ACS Synth. Biol. 12, 863–876. doi: 10.1021/acssynbio.2c00673
Luckner, P., and Brandsch, M. (2005). Interaction of 31 β-lactam antibiotics with the H+/peptide symporter PEPT2: analysis of affinity constants and comparison with PEPT1. Eur. J. Pharm. Biopharm. 59, 17–24. doi: 10.1016/j.ejpb.2004.07.008
McInnes, L., Healy, J., and Melville, J. (2018a). UMAP: Uniform manifold approximation and projection for dimension reduction. arXiv. doi: 10.48550/ARXIV.1802.03426
McInnes, L., Healy, J., Saul, N., and Großberger, L. (2018b). UMAP: uniform manifold approximation and projection. J. Open Source Softw. 3:861. doi: 10.21105/joss.00861
Mojzita, D., and Hohmann, S. (2006). Pdc2 coordinates expression of the THI regulon in the yeast Saccharomyces cerevisiae. Mol. Gen. Genomics. 276, 147–161. doi: 10.1007/s00438-006-0130-z
Nakamura, J., Hirano, S., Ito, H., and Wachi, M. (2007). Mutations of the Corynebacterium glutamicum NCgl1221 gene, encoding a mechanosensitive channel homolog, induce L-glutamic acid production. Appl. Environ. Microbiol. 73, 4491–4498. doi: 10.1128/AEM.02446-06
Nour-Eldin, H. H., Andersen, T. G., Burow, M., Madsen, S. R., Jørgensen, M. E., Olsen, C. E., et al. (2012). NRT/PTR transporters are essential for translocation of glucosinolate defence compounds to seeds. Nature 488, 531–534. doi: 10.1038/nature11285
Nour-Eldin, H. H., Hansen, B. G., Nørholm, M. H. H., Jensen, J. K., and Halkier, B. A. (2006). Advancing uracil-excision based cloning towards an ideal technique for cloning PCR fragments. Nucleic Acids Res. 34:e122. doi: 10.1093/nar/gkl635
Nour-Eldin, H. H., Nørholm, M. H., and Halkier, B. A. (2006). Screening for plant transporter function by expressing a normalized Arabidopsis full-length cDNA library in Xenopus oocytes. Plant Methods 2:17. doi: 10.1186/1746-4811-2-17
O’Hagan, S., and Kell, D. B. (2017). Consensus rank orderings of molecular fingerprints illustrate the most genuine similarities between marketed drugs and small endogenous human metabolites, but highlight exogenous natural products as the most important “natural” drug transporter substrates. ADMET 5:85. doi: 10.5599/admet.5.2.376
Ocheltree, S. M., Shen, H., Hu, Y., Xiang, J., Keep, R. F., and Smith, D. E. (2004). Mechanisms of Cefadroxil uptake in the choroid plexus: studies in wild-type and PEPT2 knockout mice. J. Pharmacol. Exp. Ther. 308, 462–467. doi: 10.1124/jpet.103.060400
O'Hagan, S., and Kell, D. B. (2019). Generation of a small library of natural products designed to cover chemical space inexpensively. Pharm Front 1:e190005. doi: 10.20900/pf20190005
Ortiz, D. F., Pierre, M. V. S., Abdulmessih, A., and Arias, I. M. (1997). A yeast ATP-binding cassette-type protein mediating ATP-dependent bile acid transport. J. Biol. Chem. 272, 15358–15365. doi: 10.1074/jbc.272.24.15358
Otterbach, S. L., Yang, T., Kato, L., Janfelt, C., and Geu-Flores, F. (2019). Quinolizidine alkaloids are transported to seeds of bitter narrow-leafed lupin. J. Exp. Bot. 70, 5799–5808. doi: 10.1093/jxb/erz334
Pacheco, A., Talaia, G., Sá-Pessoa, J., Bessa, D., Gonçalves, M. J., Moreira, R., et al. (2012). Lactic acid production in Saccharomyces cerevisiae is modulated by expression of the monocarboxylate transporters Jen1 and Ady2. FEMS Yeast Res. 12, 375–381. doi: 10.1111/j.1567-1364.2012.00790.x
Pan, Z., Agarwal, A. K., Xu, T., Feng, Q., Baerson, S. R., Duke, S. O., et al. (2008). Identification of molecular pathways affected by pterostilbene, a natural dimethylether analog of resveratrol. BMC Med. Genet. 1:7. doi: 10.1186/1755-8794-1-7
Payne, R. M. E., Xu, D., Foureau, E., Teto Carqueijeiro, M. I. S., Oudin, A., Bernonville, T. D., et al. (2017). An NPF transporter exports a central monoterpene indole alkaloid intermediate from the vacuole. Nat. Plants 3:16208. doi: 10.1038/nplants.2016.208
Pereira, R., Wei, Y., Mohamed, E., Radi, M., Malina, C., Herrgård, M. J., et al. (2019). Adaptive laboratory evolution of tolerance to dicarboxylic acids in Saccharomyces cerevisiae. Metab. Eng. 56, 130–141. doi: 10.1016/j.ymben.2019.09.008
Prasad, R., and Goffeau, A. (2012). Yeast ATP-binding cassette transporters conferring multidrug resistance. Ann. Rev. Microbiol. 66, 39–63. doi: 10.1146/annurev-micro-092611-150111
Psychogios, N., Hau, D. D., Peng, J., Guo, A. C., Mandal, R., Bouatra, S., et al. (2011). The human serum metabolome. PLoS One 6:e16957. doi: 10.1371/journal.pone.0016957
Ramaswamy, V. K., Vargiu, A. V., Malloci, G., Dreier, J., and Ruggerone, P. (2017). Molecular rationale behind the differential substrate specificity of bacterial RND multi-drug transporters. Sci. Rep. 7:8075. doi: 10.1038/s41598-017-08747-8
Reinders, J., Zahedi, R. P., Pfanner, N., Meisinger, C., and Sickmann, A. (2006). Toward the complete yeast mitochondrial proteome: multidimensional separation techniques for mitochondrial proteomics. J. Proteome Res. 5, 1543–1554. doi: 10.1021/pr050477f
Romero, M. F., Kanai, Y., Gunshin, H., and Hediger, M. A. (1998). “[2] expression cloning using Xenopus laevis oocytes” in Methods in enzymology. (San Diego, CA: Elsevier), 17–52.
Saier, M. H. (1994). Convergence and divergence in the evolution of transport proteins. BioEssays 16, 23–29. doi: 10.1002/bies.950160104
Saier, M. H. (2006). TCDB: the transporter classification database for membrane transport protein analyses and information. Nucleic Acids Res. 34, D181–D186. doi: 10.1093/nar/gkj001
Saier, M. H., Reddy, V. S., Tsu, B. V., Ahmed, M. S., Li, C., and Moreno-Hagelsieb, G. (2016). The transporter classification database (TCDB): recent advances. Nucleic Acids Res. 44, D372–D379. doi: 10.1093/nar/gkv1103
Sano, C. (2009). History of glutamate production. Am. J. Clin. Nutr. 90, 728S–732S. doi: 10.3945/ajcn.2009.27462F
Shiomi, N., Fukuda, H., Murata, K., and Kimura, A. (1995). Improvement of S-adenosylmethionine production by integration of the ethionine-resistance gene into chromosomes of the yeast Saccharomyces cerevisiae. Appl. Microbiol. Biotechnol. 42, 730–733. doi: 10.1007/BF00171953
Sievers, F., Wilm, A., Dineen, D., Gibson, T. J., Karplus, K., Li, W., et al. (2011). Fast, scalable generation of high-quality protein multiple sequence alignments using Clustal omega. Mol. Syst. Biol. 7:539. doi: 10.1038/msb.2011.75
Silano, V., Barat Baviera, J. M., Bolognesi, C., Brüschweiler, B. J., Chesson, A., Cocconcelli, P. S., et al. (2018). Safety assessment of the active substance polyacrylic acid, sodium salt, cross-linked, for use in active food contact materials. EFSA J. 16:e05448. doi: 10.2903/j.efsa.2018.5448
Staud, F., Cerveny, L., Ahmadimoghaddam, D., and Ceckova, M. (2013). Multidrug and toxin extrusion proteins (MATE/SLC47); role in pharmacokinetics. Int. J. Biochem. Cell Biol. 45, 2007–2011. doi: 10.1016/j.biocel.2013.06.022
Stieger, B., Steiger, J., and Locher, K. P. (2021). Membrane lipids and transporter function. Biochim. Biophys. Acta Mol. Basis Dis. 1867:166079. doi: 10.1016/j.bbadis.2021.166079
Superti-Furga, G., Lackner, D., Wiedmer, T., Ingles-Prieto, A., Barbosa, B., Girardi, E., et al. (2020). The RESOLUTE consortium: unlocking SLC transporters for drug discovery. Nat. Rev. Drug Discov. 19, 429–430. doi: 10.1038/d41573-020-00056-6
Takeda, M., Babu, E., Narikawa, S., and Endou, H. (2002). Interaction of human organic anion transporters with various cephalosporin antibiotics. Eur. J. Pharmacol. 438, 137–142. doi: 10.1016/S0014-2999(02)01306-7
Tal, I., Zhang, Y., Jørgensen, M. E., Pisanty, O., Barbosa, I. C. R., Zourelidou, M., et al. (2016). The Arabidopsis NPF3 protein is a GA transporter. Nat. Commun. 7:11486. doi: 10.1038/ncomms11486
Tanihara, Y., Masuda, S., Sato, T., Katsura, T., Ogawa, O., and Inui, K. I. (2007). Substrate specificity of MATE1 and MATE2-K, human multidrug and toxin extrusions/H+-organic cation antiporters. Biochem. Pharmacol. 74, 359–371. doi: 10.1016/j.bcp.2007.04.010
Terada, T., Saito, H., Mukai, M., and Inui, K. I. (1997). Recognition of β-lactam antibiotics by rat peptide transporters, PEPT1 and PEPT2, in LLC-PK 1 cells. Am. J. Physiol. Renal Physiol. 273, F706–F711. doi: 10.1152/ajprenal.1997.273.5.F706
Thiele, I., Swainston, N., Fleming, R. M. T., Hoppe, A., Sahoo, S., Aurich, M. K., et al. (2013). A community-driven global reconstruction of human metabolism. Nat. Biotechnol. 31, 419–425. doi: 10.1038/nbt.2488
van der Hoek, S. A., and Borodina, I. (2020). Transporter engineering in microbial cell factories: the ins, the outs, and the in-betweens. Curr. Opin. Biotechnol. 66, 186–194. doi: 10.1016/j.copbio.2020.08.002
van der Hoek, S. A., Darbani, B., Zugaj, K. E., Prabhala, B. K., Biron, M. B., Randelovic, M., et al. (2019). Engineering the yeast Saccharomyces cerevisiae for the production of L-(+)-Ergothioneine. Front. Bioeng. Biotechnol. 7:262. doi: 10.3389/fbioe.2019.00262
van der Hoek, S. A., Rusnák, M., Wang, G., Stanchev, L. D., de Fátima Alves, L., Jessop-Fabre, M. M., et al. (2022). Engineering precursor supply for the high-level production of ergothioneine in Saccharomyces cerevisiae. Metab. Eng. 70, 129–142. doi: 10.1016/j.ymben.2022.01.012
Västermark, Å., Almén, M. S., Simmen, M. W., Fredriksson, R., and Schiöth, H. B. (2011). Functional specialization in nucleotide sugar transporters occurred through differentiation of the gene cluster EamA (DUF6) before the radiation of Viridiplantae. BMC Evol. Biol. 11:123. doi: 10.1186/1471-2148-11-123
Vos, T., de la Torre Cortés, P., van Gulik, W. M., Pronk, J. T., and Daran-Lapujade, P. (2015). Growth-rate dependency of de novo resveratrol production in chemostat cultures of an engineered Saccharomyces cerevisiae strain. Microb. Cell Factories 14:133. doi: 10.1186/s12934-015-0321-6
Wang, G., Møller-Hansen, I., Babaei, M., D'Ambrosio, V., Christensen, H. B., Darbani, B., et al. (2021). Transportome-wide engineering of Saccharomyces cerevisiae. Metab. Eng. 64, 52–63. doi: 10.1016/j.ymben.2021.01.007
Wang, W., Zhou, H., Ma, B., Owiti, A., Korban, S. S., and Han, Y. (2016). Divergent evolutionary pattern of sugar transporter genes is associated with the difference in sugar accumulation between grasses and eudicots. Sci. Rep. 6:29153. doi: 10.1038/srep29153
Wenzel, U., Gebert, I., Weintraut, H., Weber, W. M., Clauss, W., and Daniel, H. (1996). Transport characteristics of differently charged cephalosporin antibiotics in oocytes expressing the cloned intestinal peptide transporter PepT1 and in human intestinal Caco-2 cells. J. Pharmacol. Exp. Ther. 277, 831–839.
Wright Muelas, M., Roberts, I., Mughal, F., O’Hagan, S., Day, P. J., and Kell, D. B. (2020). An untargeted metabolomics strategy to measure differences in metabolite uptake and excretion by mammalian cell lines. Metabolomics 16:107. doi: 10.1007/s11306-020-01725-8
Wulff, N., Ernst, H. A., Jørgensen, M. E., Lambertz, S., Maierhofer, T., Belew, Z. M., et al. (2019). An optimized screen reduces the number of GA transporters and provides insights into nitrate transporter 1/peptide transporter family substrate determinants. Front. Plant Sci. 10:1106. doi: 10.3389/fpls.2019.01106
Xu, D., Sanden, N. C. H., Hansen, L. L., Belew, Z. M., Madsen, S. R., Meyer, L., et al. (2023). Export of defensive glucosinolates is key for their accumulation in seeds. Nature 617, 132–138. doi: 10.1038/s41586-023-05969-x
Keywords: small molecule transporters, xenobiotic substrates, metabolite transport, cell factories, transporter protein, exometabolome, metabolic engineering, substrate specificity
Citation: Møller-Hansen I, Sáez-Sáez J, van der Hoek SA, Dyekjær JD, Christensen HB, Wright Muelas M, O’Hagan S, Kell DB and Borodina I (2024) Deorphanizing solute carriers in Saccharomyces cerevisiae for secondary uptake of xenobiotic compounds. Front. Microbiol. 15:1376653. doi: 10.3389/fmicb.2024.1376653
Edited by:
Mingtao Huang, South China University of Technology, ChinaReviewed by:
Gennaro Agrimi, University of Bari Aldo Moro, ItalyFu-Xing Niu, Guangxi University of Science and Technology, China
Copyright © 2024 Møller-Hansen, Sáez-Sáez, van der Hoek, Dyekjær, Christensen, Wright Muelas, O’Hagan, Kell and Borodina. This is an open-access article distributed under the terms of the Creative Commons Attribution License (CC BY). The use, distribution or reproduction in other forums is permitted, provided the original author(s) and the copyright owner(s) are credited and that the original publication in this journal is cited, in accordance with accepted academic practice. No use, distribution or reproduction is permitted which does not comply with these terms.
*Correspondence: Irina Borodina, aXJib0BiaW9zdXN0YWluLmR0dS5kaw==