- Gomer Lab, Department of Biology, Texas A&M University, College Station, TX, United States
Mycobacterium tuberculosis (Mtb), the bacterium that causes tuberculosis (TB), can be difficult to treat because of drug tolerance. Increased intracellular polyphosphate (polyP) in Mtb enhances tolerance to antibiotics, and capsular polyP in Neisseria gonorrhoeae potentiates resistance to antimicrobials. The mechanism by which bacteria utilize polyP to adapt to antimicrobial pressure is not known. In this study, we found that Mtb adapts to the TB frontline antibiotic isoniazid (INH) by enhancing the accumulation of cellular, extracellular, and cell surface polyP. Gallein, a broad-spectrum inhibitor of the polyphosphate kinase that synthesizes polyP, prevents this INH-induced increase in extracellular and cell surface polyP levels. Gallein and INH work synergistically to attenuate Mtb's ability to grow in in vitro culture and within human macrophages. Mtb when exposed to INH, and in the presence of INH, gallein inhibits cell envelope formation in most but not all Mtb cells. Metabolomics indicated that INH or gallein have a modest impact on levels of Mtb metabolites, but when used in combination, they significantly reduce levels of metabolites involved in cell envelope synthesis and amino acid, carbohydrate, and nucleoside metabolism, revealing a synergistic effect. These data suggest that gallein represents a promising avenue to potentiate the treatment of TB.
Introduction
Tuberculosis (TB) remains a significant global public health challenge, and in 2022, the causative bacterium Mycobacterium tuberculosis (Mtb) was responsible for ~1.6 million deaths worldwide (Bagcchi, 2023). During infection, Mtb encounters a variety of stressors originating from the host, and in response, employs adaptive physiological mechanisms to endure these stresses, promoting persistence and both tolerance to antibiotics and the development of drug tolerance (McCune, 1956; Deb et al., 2009; Jain et al., 2016; Liu et al., 2016; Mehta et al., 2016). These complexities not only demand prolonged treatment regimens but also contribute to the emergence of drug-resistant Mtb strains (Bagcchi, 2023). Notably, resistance to the primary antibiotic, isoniazid (INH), is a prevalent form of monoresistance in Mtb, which is associated with treatment failures and the emergence of multidrug-resistant TB (Bagcchi, 2023). Mtb's resistance to the majority of antibiotics is attributed to the thickening of the cell envelope (Nguyen and Thompson, 2006; Silver, 2011), the activation of enzymes that modify antibiotics or their targets, and the action of efflux pumps (Jarlier and Nikaido, 1994; Nikaido, 1994). These mechanisms collectively reduce the efficacy of antibiotics.
Polyphosphate (polyP) is a chain of phosphate residues and is present in all kingdoms of life (Rao et al., 2009). PolyP metabolism has been linked to the virulence of pathogens such as Mtb, Burkholderia mallei, Pseudomonas aeruginosa, Salmonella enterica, and Shigella flexneri (Rashid et al., 2000; Kim et al., 2002; Tunpiboonsak et al., 2010; Chuang et al., 2013). A highly conserved bacterial enzyme, polyphosphate kinase (PPK), synthesizes polyP from ATP, while polyP levels are regulated by the action of exopolyphosphatase (PPX), an enzyme that removes terminal phosphate residues from a polyP chain (Kumble and Kornberg, 1995). The Mtb genome encodes two PPKs, PPK1 (Rv2984) and PPK2 (Rv3232c), as well as two PPXs, PPX1 (Rv0496) and PPX2 (Rv1026) (Cole et al., 1998).
Pathogenic bacteria lacking PPK or having reduced PPK levels exhibit defects in stress response, quorum sensing, growth, survival, and virulence (Rao et al., 1998; Rashid and Kornberg, 2000; Rashid et al., 2000; Chavez et al., 2004; Sureka et al., 2009; Zhang et al., 2010; Mookherjee et al., 2013; Manca et al., 2023). For instance, intracellular polyP is necessary for the survival of Mtb in host cells (Chavez et al., 2004; Sureka et al., 2007; Chuang et al., 2013; Singh et al., 2013), and deletion of PPK1 in M. smegmatis attenuates the survival of ingested M. smegmatis in human macrophages (Rijal et al., 2020). Conversely, increased intracellular polyP in Mtb causes increased tolerance to antibiotics (Thayil et al., 2011; Chuang et al., 2013, 2015; Singh et al., 2013). In addition to intracellular polyP, bacteria also have extracellular polyP. The pathogenic bacterium Neisseria gonorrhoeae has polyP in its capsule, and the polyP potentiates resistance to antimicrobials (Mookherjee et al., 2013; Manca et al., 2023). We observed that both Mtb and M. smegmatis accumulate extracellular polyP (Rijal et al., 2020). Treatment of Mtb-infected macrophages with a polyP-degrading recombinant exopolyphosphatase (ScPPX) reduced the Mtb burden in macrophages, suggesting that both intracellular and extracellular polyP potentiate the survival of Mtb in host cells (Rijal et al., 2020).
Given the absence of PPK enzymes in humans (Brown and Kornberg, 2004), bacterial PPKs could serve as potential targets for antituberculosis therapeutics. The small molecule gallein (Lillie et al., 1974) is a broad-spectrum PPK inhibitor (Neville et al., 2021; Roberge, 2021), and in this report, we find that gallein strongly potentiates the ability of INH to inhibit Mtb growth alone and in human macrophages.
Results
Gallein enhances the INH-mediated inhibition of Mtb growth
PPK1 and PPK2, enzymes which synthesize polyP, are both necessary for Mtb viability (Sureka et al., 2009; Jagannathan et al., 2010). Ellagic acid derivatives from a medicinal plant inhibit PPK1 in Pseudomonas aeruginosa (Sarabhai et al., 2015). Gallein, a small molecule with similarity to ellagic acid, was identified as a potent inhibitor of both PPK1 and PPK2 in P. aeruginosa (Neville et al., 2021). Gallein also inhibits Gβγ subunit signaling in mammalian cells (Smrcka, 2013; Sanz et al., 2017). To determine if gallein affects Mtb, cells were cultured in the presence of different concentrations of gallein. Gallein at 0.005, 0.05, or 0.5 μM did not significantly affect Mtb growth, as assessed by an increase in OD600 values (Supplementary Figures S1A–C), while 5 μM gallein slightly slowed growth, and 50 μM gallein inhibited growth by ~80% (Figures 1A, B). Given that we observed complete inhibition of Mtb growth with 100 μg/ml INH in our experimental setup, we opted to utilize 1 μg/ml INH for all our assays. This choice aligns with reports from other research groups, which showed tolerance and resistance of Mtb to concentrations of INH >1 μg/ml (Ojha et al., 2008; Flentie et al., 2019). In the absence of gallein, 1 μg/ml INH caused a partial but not complete reduction in Mtb growth (Supplementary Figure S1D), and this effect was potentiated by 5 and 50 μM gallein (Figures 1A, B). The addition of 0.005, 0.05, or 0.5 μM gallein did not significantly enhance the effect of 1 μg/ml INH (Supplementary Figures S1A–C). To determine if the reduced growth with INH and/or 5 and 50 μM gallein causes a permanent effect on Mtb, the day 14 cultures were washed and resuspended in medium without INH or gallein and the growth was monitored (Supplementary Figure S1E). Surprisingly, exposure to INH caused Mtb cells to grow faster and exposure to gallein caused a slight reduction in growth. Together, these data indicate that a 14 day exposure of Mtb to 1 μg/ml INH and 5 or 50 μM gallein is bacteriostatic but not bactericidal. However, the apparent decrease in Mtb growth in the presence of INH and/or 5 or 50 μM gallein might also be attributed to changes cell wall size/shape.
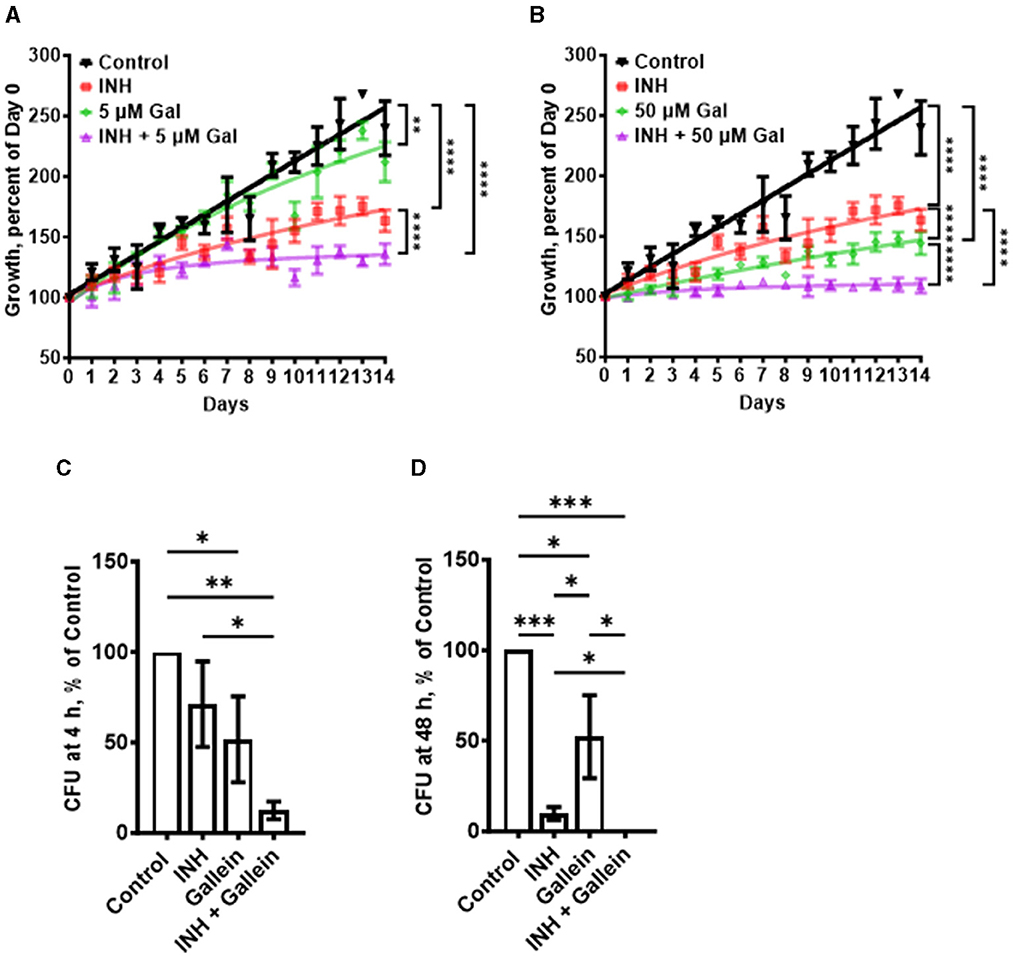
Figure 1. Gallein potentiates the ability of INH to inhibit Mtb growth both in in vitro culture and within macrophages. (A, B) Mtb cultures were grown for 14 days in the absence (Control) or presence of 1 μg/ml isoniazid (INH) and/or 5 μM (A) or 50 μM (B) gallein. The OD600 was measured daily, and growth was determined as a percentage of Day 0 OD600. (C, D) Viable ingested Mtb in macrophages, in the absence (Control) or presence of 1 μg/ml INH and/or 5 μM gallein, was determined as colony-forming units (CFU) at 4 h (C) and 48 h (D) after ingestion. CFU in the control was considered 100%. All values are mean ± SEM of three (A, B) and four (two females and two males) (C, D) independent experiments. *P < 0.05; **P < 0.01; ***P < 0.001, ****P < 0.0001 (two-way ANOVA with Dunnett's multiple comparisons test for A, B, and Mann-Whitney test for C, D).
In patients with tuberculosis, Mtb bacteria can be ingested by macrophages, and are able to survive inside the macrophages (Echeverría-Valencia, 2023). To determine if gallein affects the ability of Mtb to survive in macrophages, human macrophages, derived from circulating monocytes from healthy donors, were incubated with Mtb for 2 h, and the non-ingested Mtb were removed. The macrophages were then incubated with gallein and/or INH, and at 4 and 48 h after adding Mtb the macrophages were lysed with a detergent (0.1% Triton X-100) that does not kill Mtb, and the Supplementary Table S1). At both times, gallein decreased ingested Mtb viability compared to control, but the effects were similar at 4 and at 48 h (Figures 1C, D; Supplementary Table S1). One possibility for the stronger effect of gallein in vitro compared to the effect of gallein in macrophages is that compared to the concentration outside cells, the concentration of gallein inside a phagosome inside a macrophage is less, and in addition, the environment inside a phagosome is different from the environment in the in vitro experiment. In the presence of INH, gallein significantly decreased the viability of ingested Mtb, with no detected surviving bacteria at 48 h (Figures 1C, D; Supplementary Table S1). Together, these results suggest that for both free Mtb and Mtb in macrophages, gallein inhibits growth and enhances INH's ability to inhibit growth.
Isoniazid increases the accumulation of polyp
Increased intracellular polyP in Mtb causes increased tolerance to antibiotics (Thayil et al., 2011; Chuang et al., 2013, 2015; Singh et al., 2013). To determine if exposure of Mtb to INH potentiates accumulation of polyP (Thayil et al., 2011; Chuang et al., 2015), Mtb cells were cultured in the presence of INH. After exposure to INH for 21 days, the Mtb cells were fixed without permeabilizing the cells and then stained with the GFP-tagged polyP binding domain of Escherichia coli PPX (GFP-PPX) (Xie et al., 2019). For unknown reasons, the cells showed a wide range of staining intensities (Figures 2A, B). Although INH concentrations from 0.1 to 100 μg/ml increased the average amount of cell-surface polyP, 1 μg/ml INH had a stronger effect in increasing the amount of cell surface polyP (Figures 2A, B), which aligns with our previous decision to use 1 μg/ml INH for all of our assays. The apparently weaker effect of 10 and 100 μg/ml INH may be due to the higher concentrations of INH causing a general disruption in cell metabolism, including the ability to increase cell surface polyP. A 1 day exposure of Mtb to 1 μg/ml INH also increased cell-surface and total cellular polyP (Figures 2C–E). We previously observed that Mtb cells accumulate extracellular polyP (Rijal et al., 2020), and at 1 day INH also increased the accumulation of extracellular polyP (Figure 2F). At 5 and 14 days, 1 μg/ml INH significantly increased cell-surface, total cellular, and extracellular polyP (Figures 2G–L). At 14 days, Mtb controls had, using an arbitrary cutoff, 23% of cells with low levels of cell surface polyP, cells treated with gallein in the presence or absence of INH had 43% of cells with low levels of cell surface polyP, but none of the cells treated with INH had low levels of cell surface polyP (Supplementary Figure S2A). Together, these findings suggest that INH induces the accumulation of cellular, extracellular, and cell surface polyP.
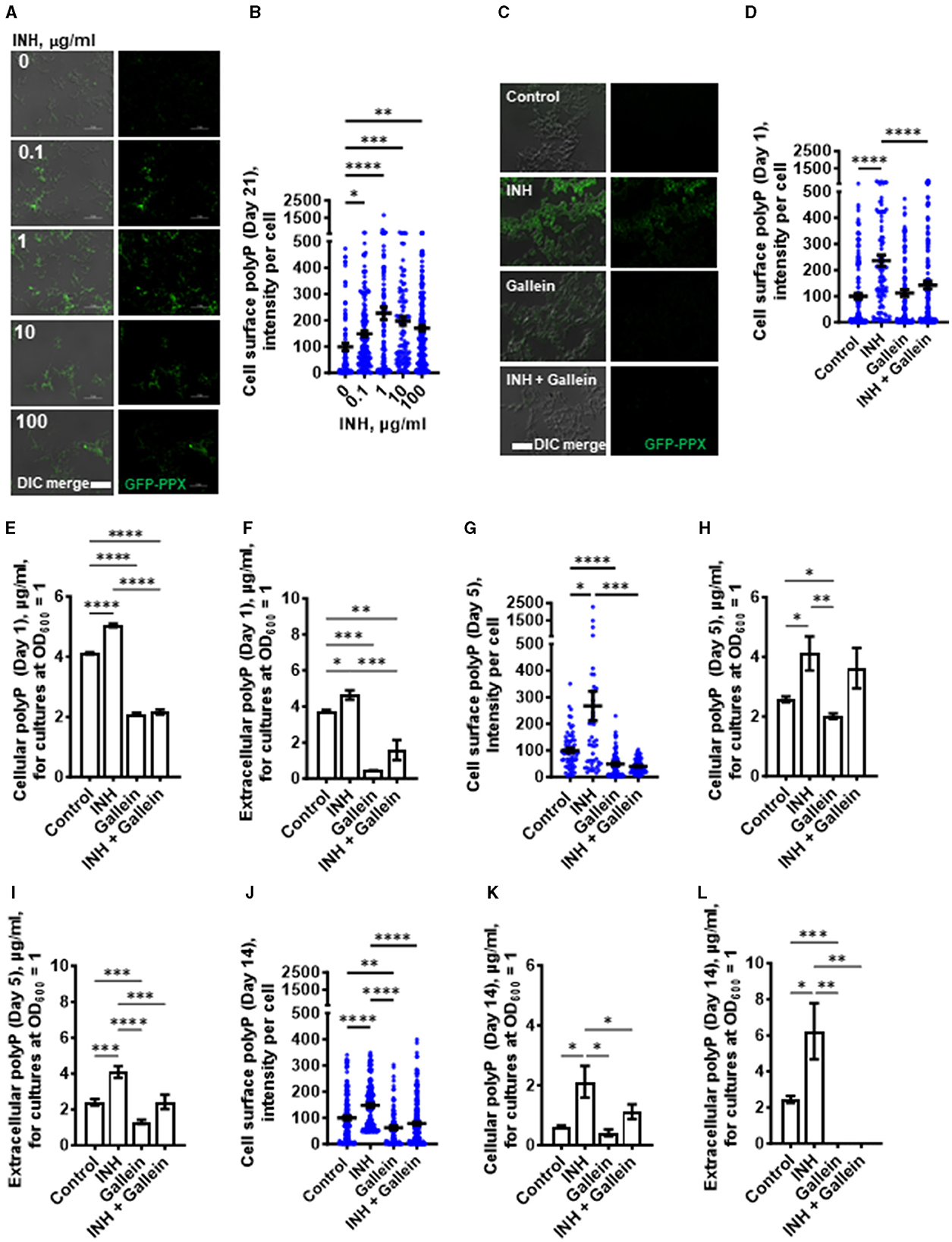
Figure 2. INH induces accumulation of Mtb cell surface, cellular, and extracellular polyP. (A) Mtb were cultured for 21 days in the presence of the indicated concentrations of INH and then stained with GFP-PPX. Differential interference contrast (DIC) merged with fluorescence are at the left, and fluorescence images are at the right. Representative images from at least three independent experiments are shown. Bars are 10 μm. (B) GFP-PPX staining intensity per cell, as a measure of Mtb cell surface polyP per cell, was determined from (A). Black bars are means. In (B, D, G, J), the staining intensities are in arbitrary units with the control averages set to 100. (C) DIC merge and fluorescence images of Mtb grown for 1 day in the absence or presence of 1 μg/ml INH and/or 5 μM gallein and stained with GFP-PPX are displayed. Representative images from at least three independent experiments are shown. Bar is 10 μm. (D) GFP-PPX staining intensity per cell, as a measure of Mtb cell surface polyP per cell, was determined from (C). Black bars are means. (E) Mtb were grown for 1 day in the absence or presence of 1 μg/ml INH and/or 5 μM gallein. The OD600 was measured, cells were lysed, and cellular polyP levels for cultures at OD600 = 1 were determined. (F) Mtb were grown for 1 day in the absence or presence of 1 μg/ml INH and/or 5 μM gallein. The OD600 was measured, and supernatant conditioned medium was collected. Extracellular polyP levels for cultures at OD600 = 1 were determined. (G–L) Mtb were grown for 5 days (G–I) or 14 days (J–L) in the absence or presence of 1 μg/ml INH and/or 5 μM gallein. Levels of cell surface polyP (G, J), cellular polyP (H, K), and extracellular polyP (I, L) were determined as described in (C–F). All values are mean ± SEM of three independent experiments. *P < 0.05; **P < 0.01; ***P < 0.001, ****P < 0.0001 (one-way ANOVA with Tukey's multiple comparisons test).
Gallein prevents the INH-induced accumulation of extracellular and cell surface polyP
One possible mechanism for the effect of gallein on Mtb growth is that gallein, by inhibiting PPKs, decreases polyP levels. We measured cell surface, cellular and extracellular polyP levels in Mtb in the absence or presence of 1 μg/ml INH and/or 5 μM gallein. At 1 day, in the absence of INH, gallein did not decrease cell surface polyP, but did block the INH-induced increase in cell surface polyP, bringing the cell surface polyP levels to levels comparable to control cells (Figure 2D). Gallein decreased cellular polyP in both the absence and presence of INH (Figure 2E). Gallein also decreased extracellular polyP in both the absence and presence of INH (Figure 2F). At day 1, INH and gallein did not significantly affect levels of Mtb ppk1 and ppk2 mRNAs (Supplementary Figure S3A), but at day 5, INH and gallein significantly reduced the levels of Mtb ppk2 mRNAs (Supplementary Figure S3B), suggesting that the effects on polyP levels are mediated by changes in the levels of ppk2 mRNAs. At 5 days, gallein significantly decreased cell surface polyP in both the absence or in the presence of INH (Figure 2G). At 5 days, gallein decreased cellular polyP in the absence of INH (Figure 2H). Gallein also decreased extracellular polyP in both the absence and presence of INH (Figure 2I). In the presence of INH, gallein decreased extracellular polyP to levels comparable to control cells (Figure 2I). At 14 days, gallein significantly decreased cell surface polyP in both the absence or in the presence of INH (Figure 2J). At 14 days, gallein did not significantly decrease cellular polyP in the absence of INH (Figure 2K), but significantly decreased cellular polyP in the presence of INH (Figure 2K). Gallein decreased extracellular polyP in both the absence and presence of INH (Figure 2L). At 14 days, in the absence or presence of INH, gallein decreased extracellular polyP to an undetectable level (Figure 2L). At 14 days, in the presence of the combination of INH and gallein, cellular debris was visible, indicating cell death (Supplementary Figure S2B). For unknown reasons, in control cells, cellular and extracellular polyP levels tended to decrease with the age of the cultures. Together, these results suggest that INH increases total, cell surface, and extracellular polyP, and that gallein blocks this effect except for total polyP at day 5.
Gallein prevents INH-induced thickening of the Mtb cell envelope
When exposed to INH, Mtb undergoes cell envelope thickening and reduces cell envelope permeability as an adaptive response to withstand INH (Chuang et al., 2015). To investigate whether gallein can reverse the effects of INH on cell envelope morphology, Mtb was treated with 1 μg/ml INH and/or 5 μM gallein for 14 days, and the Mtb cell wall was visualized using transmission electron microscopy (TEM). As previously observed (Chuang et al., 2015), INH increased Mtb cell envelope thickness (Figures 3A, B). Gallein alone did not significantly affect cell envelope thickness. For cells exposed to both INH and gallein, the average cell envelope thickness was comparable to control cells, but there were two distinct populations of Mtb cells (Figures 3A, B). For 43.0 ± 2.6% (mean ± SEM, n = 3) of the Mtb in the presence of INH and gallein, there was a detectable cell envelope, while the remaining Mtb had no detectable cell envelope (Figures 3A, B). These results suggest that in the absence of INH, gallein does not inhibit growth by affecting cell envelope thickness, and that the combination of INH and gallein causes the formation of two populations of cells, one with a detectable cell envelope (which for many of these cells is thicker than that of control cells), and one with a compromised cell envelope.
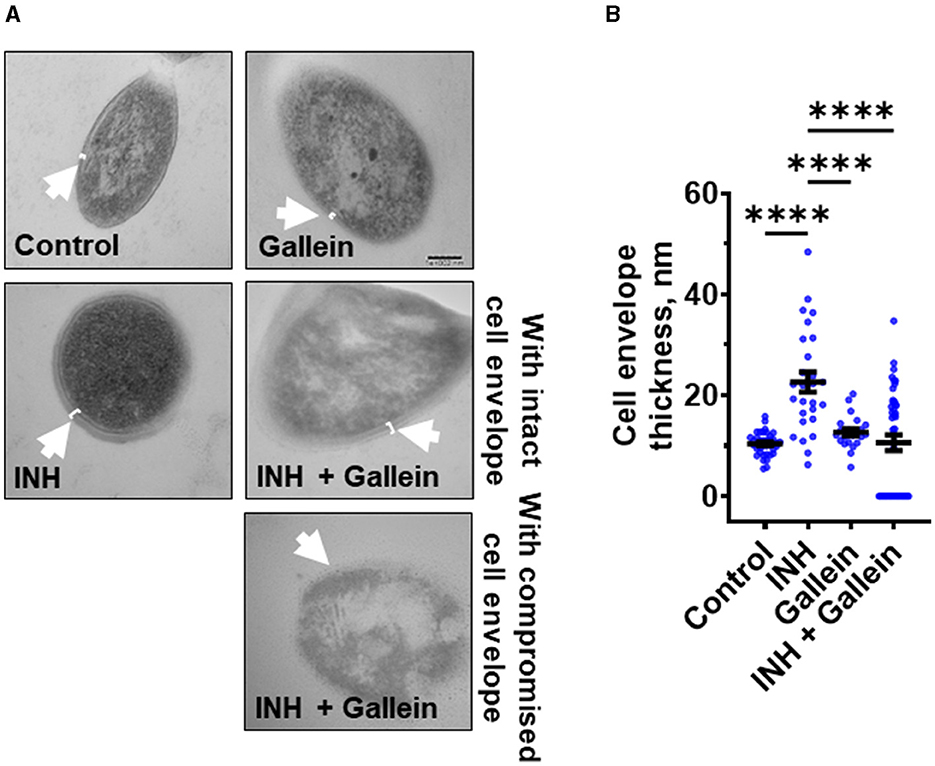
Figure 3. Gallein inhibits INH-induced Mtb cell envelope thickening. (A) Transmission electron microscopy images of Mtb treated without or with 1 μg/ml isoniazid (INH) and/or 5 μM gallein for 14 days. Representative images are from at least three independent experiments. The arrows indicate the cell envelope. Bar is 100 nm. (B) Quantification of cell envelope thickness. Values are mean ± SEM of at least 25 cells from three independent experiments. ****P < 0.0001 (one-way ANOVA with Tukey's multiple comparisons test).
Gallein and INH work synergistically to downregulate key metabolic pathways
To evaluate the impact of gallein on Mtb metabolism, Mtb were exposed to 1 μg/ml INH and/or 5 μM gallein for 24 h, then incubated with a viability dye for 12 h, and the resulting fluorescence signal was measured. The conversion of the non-fluorescent dye resazurin to the fluorescent resorufin product serves as a measure of metabolism (Cho et al., 2015; Lescat et al., 2019), and is also used to assess the susceptibility of Mtb to antimicrobial compounds (Parish and Stoker, 1998). In comparison to the control, Mtb exposed to INH showed increased metabolic activity (Supplementary Figure S4A). Gallein decreased metabolic activity in the presence or absence of INH (Supplementary Figure S4A).
Three clinically used tuberculosis drugs, namely INH, rifampicin (RIF), and streptomycin (STREP), induce a common pattern of metabolic alterations (Nandakumar et al., 2014). To determine how gallein affects metabolites, Mtb was exposed to 1 μg/ml INH and/or 5 μM gallein for 24 h. Mtb metabolites were extracted and subjected to untargeted metabolomics analysis, which identified a total of 119 metabolites. Partial least-squares discriminant analysis (PLS-DA) revealed a clear distinction between the Mtb populations treated with gallein and INH in comparison to the control. Component 2, responsible for the largest proportion of the total variance in metabolites (8.6%), placed the gallein and INH treated samples considerably apart from the control, gallein, or INH treated samples (Supplementary Figure S4B). This observation suggests that the metabolic changes induced by gallein and INH were more substantial than those caused by gallein or INH alone (Supplementary Figure S4B). A heatmap also indicated that although INH alone and gallein alone have some effect on metabolites, the combination of gallein and INH has a more profound effect (Figure 4A).
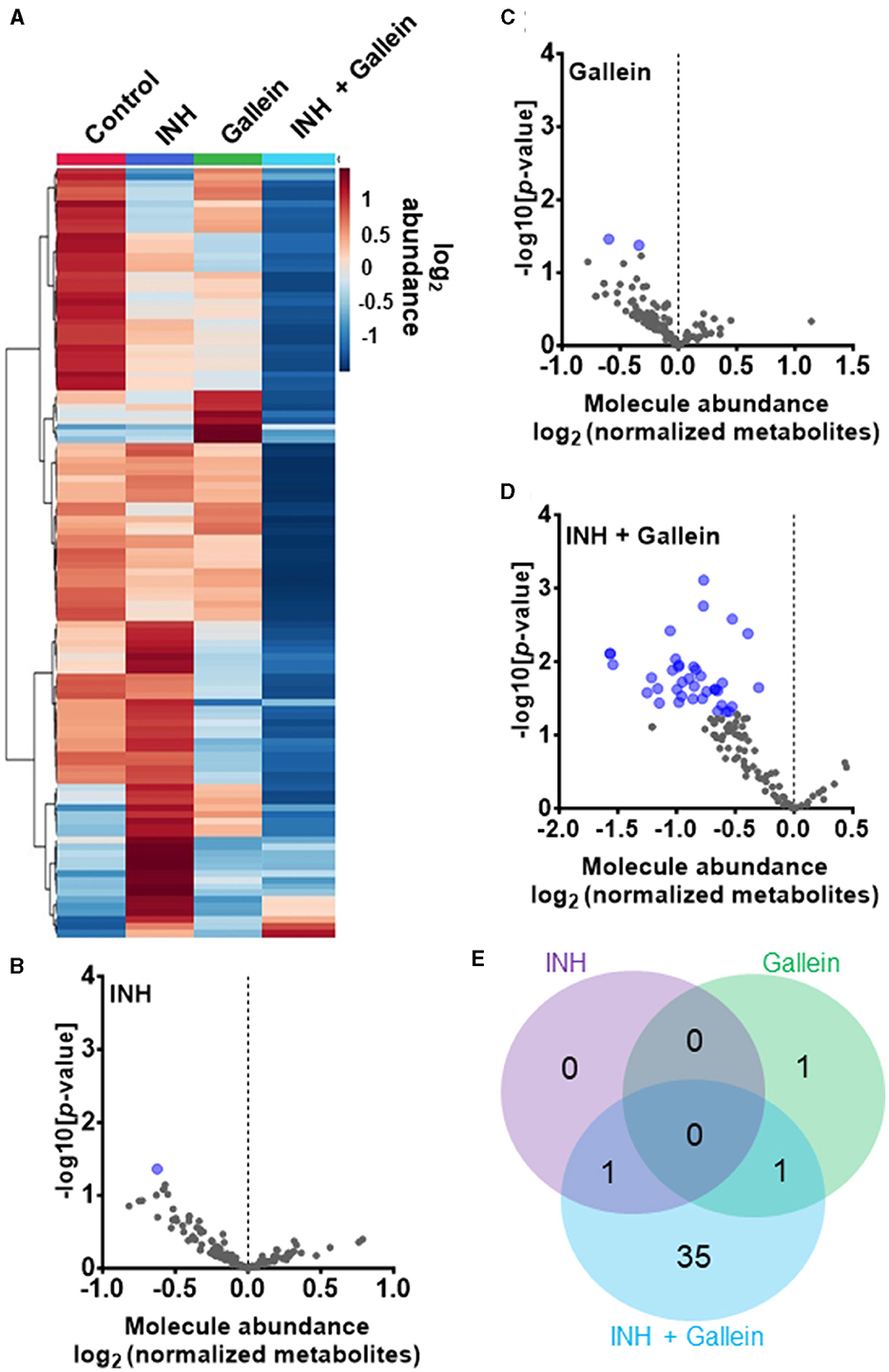
Figure 4. INH and gallein work synergistically to reduce metabolite levels. (A) Hierarchical clustering heatmap of 119 metabolites from Mtb treated with or without 1 μg/ml INH and/or 5 μM gallein for 24 h. Rows represent the mean abundance of individual metabolites detected in all three independent experiments, normalized to total protein content. The data were generated using the metabolomics data analysis tool MetaboAnalyst (https://www.metaboanalyst.ca/), and values are depicted on a log2 scale. (B–D) The abundance of individual metabolites detected in all three independent experiments was normalized to total protein content, and the mean fold change of normalized abundance of individual metabolites relative to the control on a log2 scale was plotted against the –log10 (p-value) to generate volcano plots. Blue dots indicate metabolites with relative levels having P < 0.05 (Student's t-test). (E) A Venn diagram depicts unique and common metabolites that were significantly reduced in (B–D). All values represent the mean ± SEM of three independent experiments.
As previously observed using a variety of INH concentrations (Rohde and Sorci, 2020), 1 μg/ml INH significantly reduced the levels of nicotinamide adenine dinucleotide (NAD+) (Figure 4B; Table 1). Other workers also found that 6.4 μg/ml INH alters levels of many metabolites (Nandakumar et al., 2014). We observed that gallein significantly reduced the levels of deoxythymidine diphosphate (dTDP) and biotin (Figure 4C; Table 1). The combination of gallein and INH significantly reduced the levels of 37 metabolites (Figure 4D; Table 1) including NAD+ and dTDP but not biotin (Figure 4E). These pathways are detailed in Table 2 and include nucleoside and nucleotide biosynthesis and degradation, carrier, cofactor, and vitamin biosynthesis, amino acid biosynthesis and degradation, carbohydrate biosynthesis and degradation, aminoacyl tRNA charging, cell structure biosynthesis, metabolic regulator biosynthesis, fermentation, and fatty acid and lipid degradation pathways. Gallein, INH, or the combination of gallein and INH, did not significantly upregulate any detectable metabolite. These data indicate that gallein and INH work synergistically to impact the majority of key metabolic pathways, contributing to the inhibition of Mtb growth.
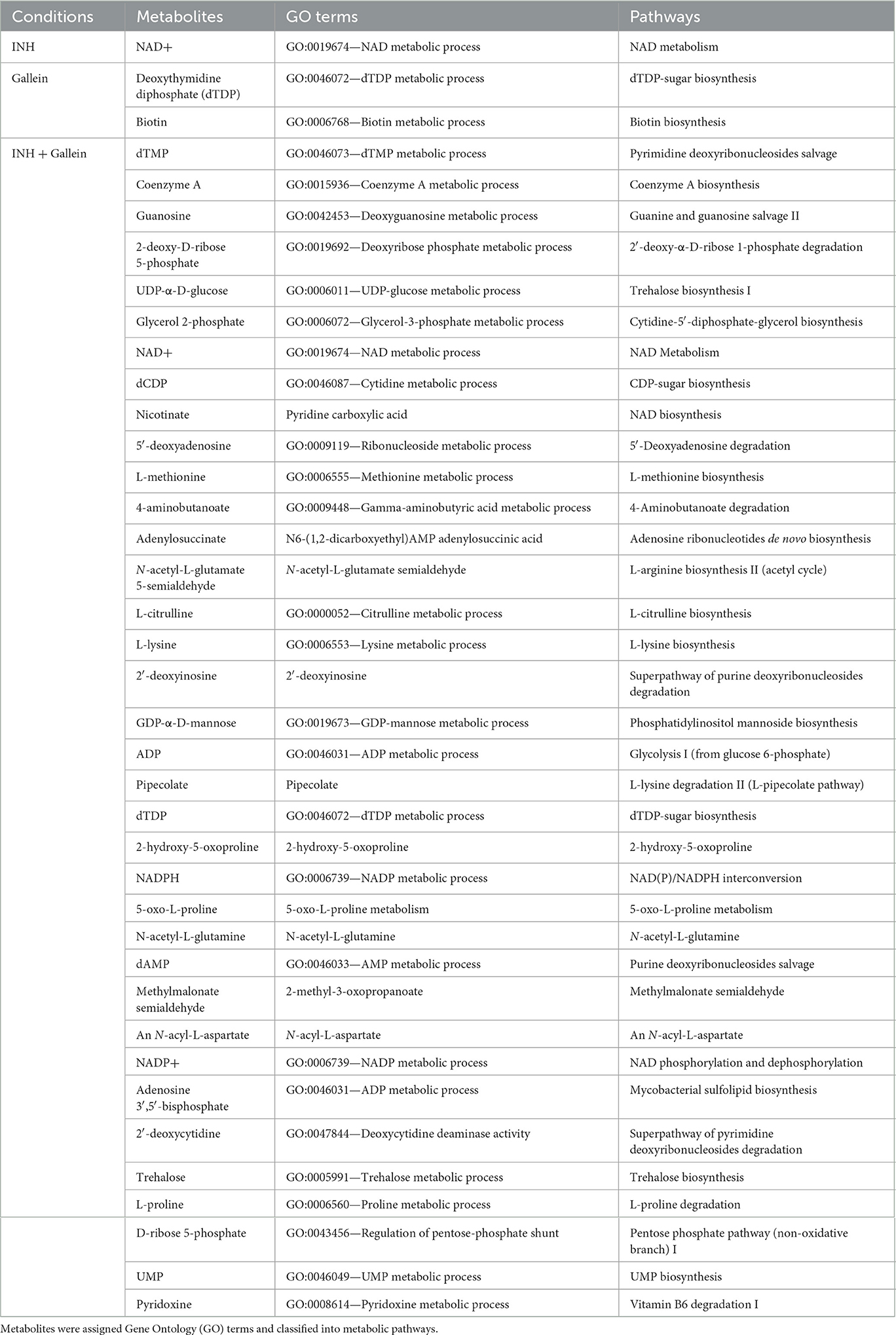
Table 1. Metabolites significantly altered in Mtb treated with INH and/or gallein, as compared to the control.
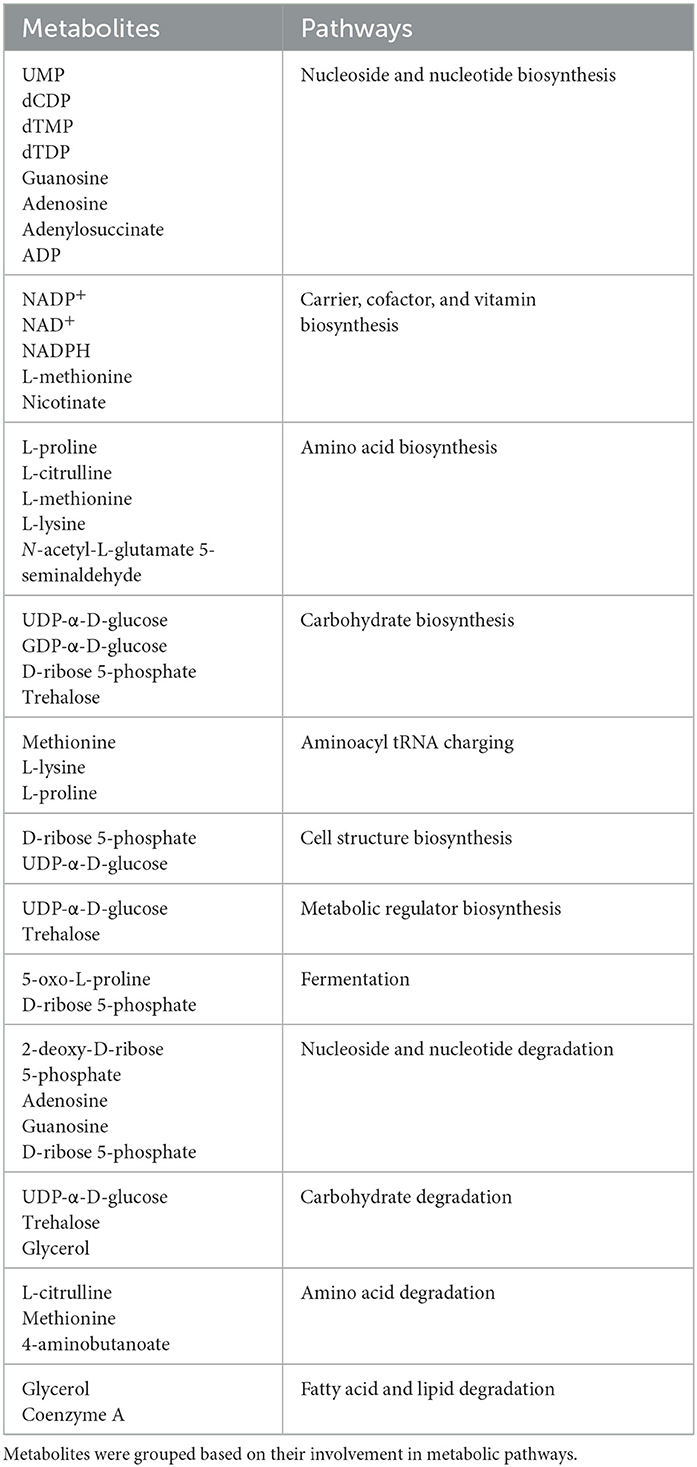
Table 2. Characterization of metabolites significantly altered in Mtb treated with the combination of INH and gallein compared to control.
Discussion
Bacteria resist antibiotics by activating drug efflux pumps and/or enzymes which modify the antibiotic or its target (Nguyen and Thompson, 2006). Cellular metabolic rearrangements can also cause antibiotic resistance (Morris et al., 2005; Smith and Romesberg, 2007; Allison et al., 2011; Baek et al., 2011; Nguyen et al., 2011; Nandakumar et al., 2014). In this report, we show that the antibiotic INH causes Mtb to increase the accumulation of cell surface, cellular, and extracellular polyP. Gallein, a bacterial PPK 1 and 2 inhibitor, inhibits Mtb cell surface, cellular, and extracellular polyP accumulation in both the presence and absence of INH, and in the presence of INH inhibits Mtb cell envelope formation in some but not all Mtb cells. Both INH and gallein have modest effects on metabolite levels, but the combination of INH and gallein strongly reduces levels of metabolites in several metabolic pathways. Possibly as a consequence of the effects of gallein on polyP levels, cell envelope formation, and metabolites, gallein inhibits Mtb growth in both in vitro culture and in human macrophages, and strongly potentiates INH inhibition of Mtb growth in in vitro culture and in human macrophages (Figure 5).
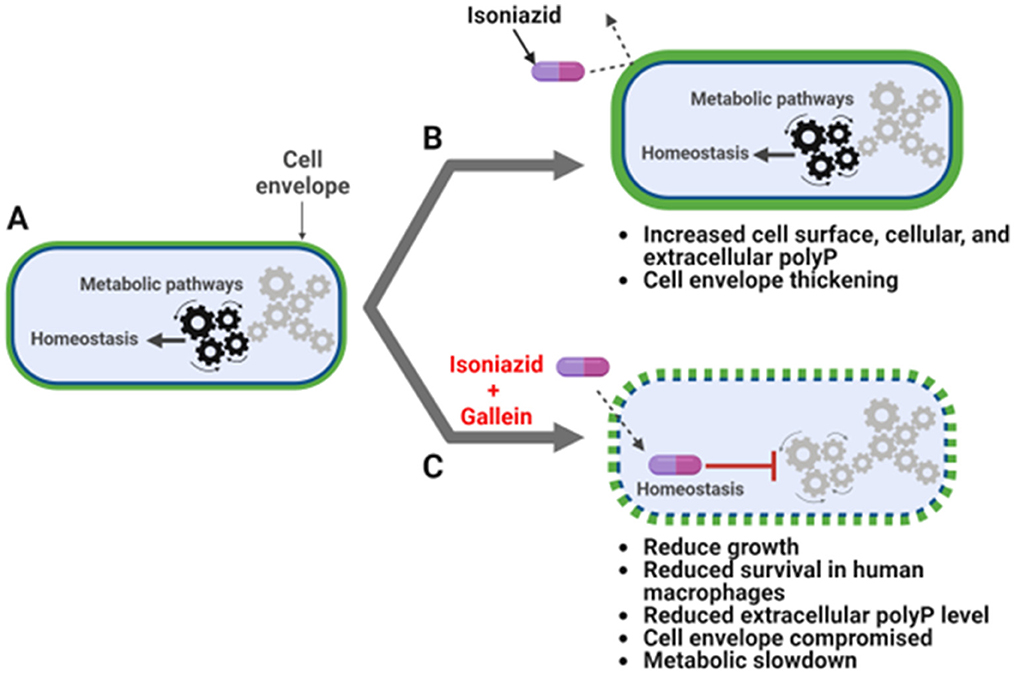
Figure 5. INH and gallein synergistically alter cellular homeostasis. (A) Mtb under no antibiotic pressure maintain homeostasis. (B) Under isoniazid (INH) pressure, Mtb grow slowly in in vitro culture, and increase the accumulation of cell surface, cellular, and extracellular polyP, as well as thickness of the cell envelope. (C) When exposed to both INH and gallein, Mtb exhibit decreased metabolic activity, decreased cell surface and extracellular polyP, and decreased cell envelope formation and integrity in many cells, resulting in very slow growth in both in vitro culture and within macrophages. The pathway diagram was created using BioRender.com.
We observed a significant increase in cellular, extracellular, and cell surface polyP in response to INH. Compared to log-phase cells, stationary phase Mtb have more polyP and are more resistant to killing by INH (Herbert et al., 1996; Rijal et al., 2020), and capsular polyP accumulation protects N. gonorrhoeae from antibiotics (Mookherjee et al., 2013; Manca et al., 2023). Combining these observations, one possibility is that Mtb increase polyP in response to INH as a protective measure. The antibiotic rifampicin causes Mtb to thicken its capsular outer layer and increase the net negative charge of the cell surface to reduce rifampicin permeability (Sebastian et al., 2020). It is possible that the INH-induced increase in the accumulation of the highly negatively charged polyP on the cell surface might similarly increase the net negative charge of the cell surface, reducing permeability to INH.
At 5 μM, gallein inhibits extracellular polyP levels in Mtb and inhibits INH-induced increases in cell surface and extracellular polyP. Gallein treatment of P. aeruginosa required concentrations exceeding 25 μM to reduce intracellular polyP accumulation, and 100 μM to mimic the effects of PPK deletion (Neville et al., 2021). Our findings suggest that gallein exhibits a greater effectiveness on Mtb compared to P. aeruginosa.
Mtb can differentiate into heterogeneous populations, such as non-replicating persisters and growing bacteria with the capacity to become persisters (Zhang, 2007). Mtb treated with INH causes a rapid killing of cells followed by a reduction in the killing rate as the number of persister cells increases (Van den Bergh et al., 2017; Vilchèze and Jacobs, 2019). In Clostridiodes difficile, a gram-positive bacteria, some cells in a population stochastically become antibiotic tolerant persisters (Álvarez et al., 2018). C. difficile forms metabolically dormant spores that resist antibiotic pressure and persist in the host (Deakin et al., 2012). Two distinct morphotypes of C. difficile spores exist, one with thick outer surface layer and the other with thin outer surface layer (Pizarro-Guajardo et al., 2016a,b). Multi-drug resistant Mtb have thicker cell envelopes (Schami et al., 2023). INH caused many, but not all, Mtb cells to have thickened cell envelopes. In response to INH and gallein, Mtb formed two distinct populations of cells: one with cell envelope thicknesses comparable to INH-treated cells, and the other with no discernible cell envelope. One possibility is that under combined INH and gallein pressure, either stochastic differentiation or asymmetric cell division gives rise to ~43% of cells with an intact cell envelope that have the potential to become slow growing persisters, and ~57% of cells with compromised cell envelope that eventually die, and this might be the reason we observed no net increase in Mtb cell growth in the presence of 50 μM gallein and INH.
For control Mtb cells, there was a large variation in cell surface polyP levels, and INH increased both the average cell surface polyP levels and cell envelope thickness. At day 14, although there was a clear bimodal distribution of cell envelope thicknesses in the presence of INH and gallein, there was no observable bimodal distribution of cell-surface polyP levels. This suggests that there is not a strict correlation between cell-surface polyP level and cell envelope thickness, and that the effect of combined INH and gallein on cell wall thicknesses may be due to effects on additional pathways in addition to cell surface polyP.
While 5 μM gallein enhances INH's ability to inhibit Mtb growth in both in vitro culture and within macrophages, the extracellular and cell surface polyP levels from cells treated with either gallein alone or the combination of gallein and INH were similar. This implies that gallein, by reducing polyP levels, may lead to Mtb envelope destabilization, rendering it susceptible to INH in both in vitro culture and human macrophages. In addition, the effect of gallein and/or gallein with INH on cell viability does not correlate with the effects of these compounds on cellular, extracellular, or cell-surface polyP accumulation. Although polyP levels seemed comparable in cells treated with gallein alone or in combination with INH, the synergistic action of gallein and INH may influence additional pathways to curb Mtb growth.
An INH-NAD adduct inhibits Enoyl acyl carrier protein reductase (InhA), a key enzyme involved in mycolic acid biosynthesis in Mtb (Quemard et al., 1995; Rawat et al., 2003). Mtb residing in macrophages have been shown to increase levels of NADH to prevent INH-mediated inhibition of InhA (Bhat et al., 2016). It is possible that the increase in NADH levels in response to INH may have led to the conversion of the non-fluorescent blue dye resazurin into the fluorescent pink product resorufin (Rampersad, 2012), which may not necessarily indicate increased metabolic activity in Mtb treated with INH.
Mtb uses the glucose disaccharide trehalose as a core component of cell surface glycolipids important for virulence, and during the transition into a non-replicating persister phase, it metabolizes trehalose to generate ATP (Elbein et al., 2003; Kalscheuer and Koliwer-Brandl, 2014; Koliwer-Brandl et al., 2016). Deletion of PPK1 in Mtb perturbs key metabolites involved in trehalose metabolism (Chugh et al., 2024). Although gallein alone did not significantly alter trehalose levels, the combination of INH and gallein reduced the levels of trehalose and components necessary for trehalose biosynthesis, such as UDP-α D-glucose (De Smet et al., 2000), and mimics effects of PPK1 deletion (Chugh et al., 2024), suggesting that the combination of INH and gallein compromises trehalose-dependent cell-surface glycolipid, and thus cell envelope, formation as we observed under TEM, and by decreasing trehalose levels, reduces this source of energy to prevent persister formation.
Loss of PPK1 in Mtb reduces levels of glucose 6-phosphate, the pentose phosphate pathway component ribose-5-phosphate, and tricarboxylic acid cycle components such as citric acid, succinate, fumarate, and malate (Chugh et al., 2024). These components of central carbon metabolism are important for amino acid biosynthesis (Umbarger, 1978; Chugh et al., 2024). Reduced levels of metabolites from carbohydrate and amino acid biosynthesis pathways in Mtb treated with the combination of INH and gallein suggests that these perturbation in central carbon and amino acid metabolism mimics PPK1 loss in Mtb (Chugh et al., 2024).
Increased polyP accumulation in Mtb increases levels of pyrophosphate, NAD+, NADH, nicotinamide, malate, succinate, 2-methyl citrate, acetyl-CoA, and metabolites belonging to arginine metabolism such as arginine, citrulline, and ornithine (Chuang et al., 2016; Chugh et al., 2024). Reduced levels of NAD+ and nicotinate from carrier, cofactor, and vitamin biosynthesis in Mtb treated with INH alone or the combination of INH and gallein indicate that INH alone or combination of INH and gallein perturbs polyP accumulation. Since few metabolites from carrier, cofactor, and vitamin biosynthesis pathway were altered compared to Mtb with increased polyP accumulation as a result of loss of PPX, it is also possible that INH and gallein affect some components of the pathway that do not involve the polyP pathway. Increased polyP accumulation reduces the nucleoside and nucleotide biosynthesis pathway components such as guanosine (Chugh et al., 2024), which suggests that reduced nucleoside and nucleotide biosynthesis pathway components in Mtb treated with the combination of INH and gallein might also be independent of the polyP pathway.
Gallein significantly reduced the levels of biotin. Mtb relies on biotin synthesis for its survival during infection, and the disruption of the biotin biosynthesis pathway results in cell death rather than growth arrest (Dey et al., 2010). This suggests that gallein may inhibit Mtb growth by promoting cell death rather than merely inhibiting growth. The combination of gallein and INH resulted in decreased levels of metabolites associated with several key metabolic pathways. It is plausible that the synergistic action of INH and gallein on Mtb growth is not solely due to the reduced integrity of the cell envelope, but also to the disruption of other metabolic pathways.
In conclusion, the combination of INH and gallein affects several aspects of Mtb physiology, and gallein thus potentiates INH antibiotic effects on Mtb. Because they are not present in mammalian cells (Brown and Kornberg, 2004), PPKs are attractive target for suppressing Mtb growth and elimination.
Materials and methods
Cell culture
Human peripheral blood was collected from healthy volunteers who gave written consent, and with specific approval from the Texas A&M University human subjects institutional review board. Peripheral blood mononuclear cells (PBMCs) were purified as previously described (Pilling et al., 2009). The PBMCs were cultured in RBCSG (RPMI-1640, # 15-040-CV, Corning, Corning, NY) containing 10% bovine calf serum [VWR Life Science Seradigm, Radnor, PA) and 2 mM L-glutamine (Lonza)], and where indicated containing 25 ng/mL human granulocyte-macrophage colony-stimulating factor (GM-CSF) ((# 572903, Biolegend, San Diego, CA) at 37°C in a humidified chamber with 5% CO2 in 96-well, tissue-culture-treated, polystyrene plates (type 353072, Corning) with 2 × 105 cells in 200 μL in each well. At day 7, loosely adhered cells were removed by gentle pipetting and removing the medium, and fresh RBCSG containing GM-CSF (as described above) was added to the cells to a final volume of 200 μL per well, and Mtb survival assays were performed as described below.
The attenuated (mc-ΔleuDΔpanCD) Biosafety Level-2 strain of Mtb, which is a derivative of the H37Rv strain (Sampson et al., 2004), was a gift from Dr. Jim Sacchettini, Texas A&M University, College Station, TX and is referred to as Mtb in this paper. This strain was cultured according to the methods described in Larsen et al. (2007), Qi et al. (2013), and Rock et al. (2017) in Middlebrook 7H9 broth [Becton, Dickinson and Company (BD), Sparks, MD] containing 0.5% glycerol (VWR), 0.05% Tween 80 (MP Biomedicals, Solon, OH), Middlebrook Oleic ADC Enrichment (BD), 50 μg/mL leucine (VWR), and 50 μg/mL pantothenate (Beantown Chemical, Hudson, NH) either in a rotator (10 rpm) or on plates with 7H10 agar (BD) and the above additives. All cultures were incubated at 37°C in a humidified incubator. These supplemented cultures are hereafter referred to as 7H9S or 7H10S.
Mtb growth assay
Mtb was grown in 24-well plates (type 353047, Corning). Each well was filled with 1 ml of 7H9S media, either containing no isoniazid (INH) or supplemented with 0.1, 1, 10, or 100 μg/ml INH (prepared from a 50 mg/ml stock in water, Cat#I3377, Sigma, Livonia, MI). Mtb samples obtained from log-phase liquid culture, as described above, were washed twice with 10 ml of 7H9S by centrifugation at 4,000 × g for 10 min. The optical density at 600 nm (OD600) was measured in a well of a 96-well, tissue-culture-treated, polystyrene plate (type 353072, Corning) at 600 nM with a Synergy Mx monochromator microplate reader (BioTek, Winooski, VT). The Mtb were then resuspended in an appropriate volume of 7H9S to achieve a final OD600 of 1. Ten microliters of Mtb with an OD600 of 1 were added to each well to reach a final OD600 of 0.01 in 1 ml. The plates were subsequently incubated in a container with humidity provided by wet paper towels at 37 °C in a humidified incubator. On the 21st day, the Mtb culture was gently resuspended, and 100 μl of the cells were transferred to a 96-well, tissue culture-treated plate (# 353072, Corning). The OD600 was measured using a microplate reader. Given that we observed complete inhibition of Mtb growth with 100 μg/ml INH in our experimental setup, we opted to utilize 1 μg/ml INH for all our assays. This choice aligns with previous reports from other research groups, which had documented the tolerance and resistance of Mtb to concentrations of INH >1 μg/ml (Ojha et al., 2008; Flentie et al., 2019).
To investigate the impact of gallein (3′,4′,5′,6′-Tetrahydroxyspiro[isobenzofuran-1(3H),9′-(9H)xanthen]-3-one) (Cat#3090, Tocris, Minneapolis, MN) and/or INH on Mtb growth, a Mtb culture plate was prepared as described method. However, in this case, each well of a type 353046, 6-well, tissue culture-treated plate (Corning) contained a final OD600 of 0.01 in 5 ml. Mtb was incubated with gallein at concentrations of 0.005, 0.05, 0.5, 5, or 50 μM and/or 1 μg/ml INH. A 50 mM gallein stock in DMSO (VWR) was diluted to 5 mM in 7H9S and further serially diluted in 7H9S to obtain lower concentrations. The control well contained 7H9S with DMSO, which was similarly serially diluted in 7H9S, as was done for gallein. The OD600 of the cells was measured daily for 14 days, and the Mtb growth curves were generated as a percentage of the OD600 on day 0.
To determine the viability of Mtb cells pre-exposed to INH and/or gallein for 14 days, 200 μl of cells from above experiments were washed twice with 1 ml of 7H9S, resuspended in 200 μl of 7H9S without INH and gallein 96-well plates (# 353072, Corning), and the OD600 of the cells was measured daily for 14 days, and the Mtb growth curves were generated as a percentage of the OD600 on day 0.
Bacterial survival assay
To determine the effect of INH and/or Gallein on the survival of Mtb in human macrophages, human macrophages (from blood monocytes cultured with GM-CSF for 6 days were mixed with Mtb following (Rijal et al., 2020), in the absence or in the presence of 1 μg/ml INH and/or 5 μM Gallein. At day 7, after isolating monocytes from donor blood, after removing loosely adhered cells as described above, 200 μL RBCSGLP (RBCSG containing 50 μg/mL leucine and 50 μg/mL pantothenate) were added to macrophages in each well in 96-well, tissue-culture-treated, polystyrene plates (# 353072, Corning) and incubated for 30 min at 37°C. Meanwhile, 1 mL of Mtb from a log phase culture was washed twice with RBCSGLP without GM-CSF by centrifugation at 12,000 × g for 2 min in a microcentrifuge tube, resuspended in 1 mL of RBCSGLP, and the OD600 of 100 μl of the culture in a well in a 96-well plate (# 353072 Corning) was measured as above. Two-hundred microliter of RBCSGLP was used as a blank. The bacteria were diluted to an OD600 of 0.5 (~107 Mtb/mL) in RBCSGLP. Mtb (~1 μl) was added to macrophages in each well such that there were ~5 bacteria per macrophage, considering ~20% of the blood monocytes converted to macrophages in the presence of GM-CSF (Cui et al., 2021). The bacteria-macrophage co-culture plate was centrifuged at 500 × g for 3 min with a Multifuge X1R Refrigerated Centrifuge (Thermo Scientific, Waltham, MA) to synchronize phagocytosis of the bacteria, and incubated for 2 h at 37°C. The supernatant medium was removed by gentle pipetting and was discarded. Two-hundred microliter of PBS warmed to 37°C was added to the co-culture in each well, cells were gently washed to remove un-ingested extracellular bacteria, the PBS was removed, and 200 μL of RBCSGLP with GMCSF in the absence or in the presence of 1 μg/ml INH and/or 5 μM gallein was added to the cells. After 2 h, cells were washed twice with PBS as above. Two-hundred microliter of RBCSGLP with GMCSF in the absence or in the presence of 1 μg/ml INH and/or 5 μM gallein was then added to the cells. After 4 and/or 48 h of infection, macrophages were washed as above with PBS, the PBS was removed, and cells were lysed using 100 μL 0.1% Triton X-100 (Alfa Aesar, Ward Hill, MA) in PBS for 5 min at room temperature by gentle pipetting, and 10 and 100 μL of the lysates were plated onto agar plates (as described above for Mtb culture). The Mtb containing agar plates were incubated for 3–4 weeks or until the Mtb colonies appeared. Bacterial colonies obtained from plating 10 and 100 μL lysates were manually counted, the number of viable ingested bacterial colonies per 10 and 100 μL lysates was calculated, and the number of viable ingested bacteria colony forming units (cfu) per ml of lysate was then calculated, which corresponds to the number of viable ingested bacteria in ~2 × 105 macrophages. To calculate the percent of control, cfu/ml of the control was considered 100%.
PolyP assays, RNA extraction, and quantitative reverse transcription PCR
Log phase Mtb cultures were prepared similarly to the growth assays in 6-well, tissue culture-treated plates (# 353046, Corning), with the exception that 5 μM gallein and/or 1 μg/ml INH were used. Plates were incubated in a container humidified with wet paper towels at 37°C for 1, 5, or 14 days. After incubation, 100 μl of cells were transferred to a 96-well, black/clear, tissue-culture-treated, glass-bottom plate (# 353219, Corning) for imaging as described below. The remaining cells were transferred to a 15-ml conical tube, harvested by centrifugation at 4,000 × g for 10 min, and 4.5 ml of the supernatant was transferred to a new 15-ml conical tube for extracellular polyP measurement. Cell pellets were further processed for RNA extraction, following the procedure outlined below. PolyP levels in the supernatant were assessed by adding 25 μg/ml of DAPI (Biolegend) (from a stock of 2 mg/ml) and measuring fluorescence at 415 nm excitation and 550 nm emission, as previously described (Aschar-Sobbi et al., 2008). PolyP standards (Sodium Polyphosphates, Glassy, Spectrum, New Brunswick, NJ) at concentrations of 0, 0.5, 1, 10, 100, 200, and 500 μg/ml were prepared in 7H9S. The polyP content was normalized to the total protein content, which was determined from the cell lysates as described below.
For RNA extraction, cell pellets obtained from the treatments described above were resuspended in 250 μL of GITC lysis buffer (containing 4 M guanidine isothiocyanate and 50 mM Tris-HCl at pH 7). The cells were lysed by incubating at 95°C for 10 min. Ten microliters of the lysates were used to determine the total protein content using a Bradford assay. The remaining lysates were used to extract RNA using an RNA extraction kit (Zymo Research, Irvine, CA). Complementary DNA (cDNA) was synthesized from 2 μg of RNA using the Maxima H Minus First Strand cDNA Synthesis kit (Thermo Scientific). Quantitative PCR was performed using SYBR GreenER™ qPCR SuperMix Universal reagent (Thermo Scientific), following the manufacturer's instructions using a QuantStudio (TM) 6 Flex thermal cycler (Thermo Scientific). The levels of Mtb's ppk1 and ppk2 mRNAs were determined using the gene-specific primers listed in Supplementary Table S2.
Fluorescence microscopy
To determine the localization of polyP in Mtb, 100 μl of Mtb cells from the growth assay on Day 21 or the growth assay on Days 1, 5, or 14 were transferred to a 96-well, black/clear, tissue-culture-treated, glass-bottom plate (# 353219, Corning) or cells smears were prepared on Superfrost micro glass slides (Cat#48311-703, VWR) as described previously (Pilling et al., 1989). The cells were fixed with 4% (wt/vol) paraformaldehyde (Cat#19210, Electron Microscopy Sciences, Hatfield, PA) in PBS for 10 min. After fixation, the cells were washed two times with 300 μl of PBS, blocked with 1 mg/ml BSA (Thermo Scientific) in PBS, and then stained with 10 μg/ml of GFP-PPX (provided generously by Dr. Ursula Jacob from the University of Michigan) in PBS/0.1% Tween 20 (PBST; Fisher Scientific) (Xie et al., 2019). Following staining, the Mtb cells were washed three times with PBST, and 200 μl of PBS was added to the well, or coverslips were mounted on slides with smears with Vectashield hard set mounting medium (Cat# H-1800, Vector Labs, Burlingame, CA) and left to dry overnight in darkness. Images of Mtb were captured using a 100 × oil-immersion objective on a Nikon Eclipse Ti2 (Nikon, Kyoto, Japan), and image deconvolution was performed using the Richardson–Lucy algorithm (Laasmaa et al., 2011) in NIS-Elements AR software (Nikon). The integrated fluorescence density was measured in randomly selected individual cells manually using the freehand selection feature in Fiji (ImageJ) (Schindelin et al., 2012).
Transmission electron microscopy
Mtb cells treated with 5 μM gallein and/or 1 μg/ml INH for 14 days were prepared as described for the growth assays above. A volume of 100 μl of cells in 7H9S was fixed by adding an equal volume of 2 × fixative, which contained 84 mM NaH2PO4, 68 mM NaOH, 4% paraformaldehyde (Cat#19210, Electron Microscopy Sciences), and 1% glutaraldehyde (Cat#0875, VWR). The samples were gently rocked for 1 h and then stored at 4°C. Sample preparation for TEM imaging was performed by the Texas A&M University Microscopy and Imaging Center Core Facility's staff (RRID: SCR_022128). Briefly, on the following day, the fixed samples were collected by centrifugation for 5 min at 14,000 × g. Subsequently, they were postfixed and stained for 2 h with 1% osmium tetroxide in 0.05 M HEPES at pH 7.4. The samples were then collected by centrifugation and washed with water five times, and dehydrated with acetone according to the following protocol: 15 min in 30, 50, 70, and 90% acetone each, followed by three changes of 100% acetone, each lasting 30 min. During the final wash step, a minimal amount of acetone was retained, just enough to cover the pellets, to prevent rehydration of the samples. Subsequently, the samples were infiltrated with modified Spurr's resin (Quetol ERL 4221 resin; Electron Microscopy Sciences; RT 14300) in a Pelco Biowave processor (Ted Pella, Inc., Redding, CA). The process included 1:1 acetone-resin for 10 min at 200 W (no vacuum), 1:1 acetone-resin for 5 min at 200 W (vacuum at 20 inches Hg, with vacuum cycles involving open sample container caps), and 1:2 acetone-resin for 5 min at 200 W (vacuum at 20 inches Hg). This was followed by four cycles of 100% resin for 5 min each at 200 W (vacuum at 20 inches Hg). The resin was then removed, and the sample fragments were transferred to BEEM conical-tip capsules that were prefilled with a small amount of fresh resin. More resin was added to fill the capsules, and they were left to stand upright for 30 min to ensure that the samples sank to the bottom. The samples were polymerized at 65°C for 48 h in an oven and then left at room temperature (RT) for an additional 24 h before sectioning. Sections of 70–80 nm thickness were obtained using a Leica UC/FC7 ultramicrotome (Leica Microsystems), deposited onto 300-mesh copper grids, and stained with uranyl acetate-lead citrate. Grids were imaged using a JEOL 1200 EX TEM operating at 100 kV. To avoid discrepancies, the cell envelope thickness of distinct cells found in each grid was measured using ImageJ at random places on the envelope. Cells with no intact cell envelope were scored as having a thickness of 0 nm. Cells in clumps were not scored for cell envelope thickness. Values determined for individual cells were plotted.
Metabolic activity assay
Cell proliferation and viability in Mtb can be assessed by incubating the cells with resazurin reagent for 6–12 h, and monitoring the color change from blue to purple (Franzblau et al., 1998). Metabolically active cells transform the non-fluorescent blue dye (resazurin) into a fluorescent pink product (resorufin), while inactive cells rapidly lose their metabolic capacity and, consequently, do not generate a fluorescent signal (Lescat et al., 2019). Mtb from the log phase culture were prepared as previously described for the proliferation assay, with 100 μl of cells being prepared per well in 96-well, tissue culture-treated plate (# 353072, Corning). Mtb was treated with 5 μM gallein and/or 1 μg/ml INH, and the plate containing the Mtb was incubated at 37 °C for 24 h. Cells were then incubated with prewarmed Deep Blue Cell Viability resazurin dye (Biolegend) to a final concentration of 10% in each well for 12 h (Franzblau et al., 1998). The fluorescence signal was then measured using a microplate reader following the manufacturer's protocol.
Metabolomics
Mtb cells from log-phase cultures were prepared as described for the growth assays, with the exception that 10 ml of culture was prepared. The Mtb cells were treated with 5 μM gallein and/or 1 μg/ml INH. After 24 h, 10 ml of the culture was collected by centrifugation at 4,000 × g for 10 min at 4°C. The cells were then washed twice with 10 ml of chilled (0–4°C) phosphate-buffered saline (PBS) to prevent metabolite contamination from the culture media. The cells were resuspended in 10 ml of PBS (Mackay et al., 2015), and 100 μl of this suspension was transferred to a 96-well plate to measure the OD600. The remaining cell suspension was centrifuged again at 4,000 × g for 10 min at 4°C to collect the cell pellets for metabolite extraction. After the final wash, excess PBS was carefully removed from all the samples. The day before the assay, 10 ml of extraction solvent [acetonitrile (BDH83639.400, VWR): methanol (BDH20864.400, VWR):water (40:40:20)] was prepared and stored at −70°C. On the day of the experiment, heavy amino acid standards (Metabolomics amino acid mix standard, Cat# MSK-A2-1.2, Cambridge Isotope Laboratories, Tewksbury, MA) were added as a spike to the extraction solvent to 5 μM final concentration. To halt bacterial metabolism, the Mtb pellets were immediately suspended in 200 μl of spiked extraction solvent that had been pre-cooled on dry ice (Nandakumar et al., 2014). Mtb disruption was achieved using a Mini-beadbeater-16 (BioSpec Products, Bartlesville, OK). The Mtb samples (200 μl) were placed in 2 ml Polypropylene Microvials (Cat#10832, BioSpec Products) containing approximately 70 μl of 0.1 mm Zirconia/Silica beads (Cat# 11079101z, BioSpec Products). Bead-beating was performed in three cycles of 1 min at 3,450 oscillations/min, with 2 min of cooling on ice between cycles (Mehra and Philips, 2014). Subsequently, the microvials containing the disrupted cells and zirconium beads were incubated at −20°C for 20 min. They were then clarified by centrifugation at 8,000 × g for 15 min at 4 degrees Celsius. The resulting supernatant was filtered through a 0.22 μm filter (Cat# UFC30GV25, Merck Millipore, Cork, IRL) into 0.2 ml glass Stepvial inserts (Cat# 200 238, ThermoScientific). These step vials were placed inside 0.25 ml polypropylene vials with polypropylene caps with PTFE/silicone septa (Cat# 200 410, ThermoScientific). The pellets containing beads were resuspended in 100 μl of Radioimmunoprecipitation assay buffer (RIPA) (Cat#89900, Thermo Scientific) containing 1X protease and phosphatase inhibitor cocktail (Cat#1861281, Thermo Scientific), incubated on ice for 15 min, clarified by centrifugation at 10,000 × g for 5 min at 4°C, and 25 μl of the supernatant was used to determine the protein amount following the manufacturer's instructions using a Pierce BCA Protein Assay Kit (Cat#23225, Thermo Scientific). A vial containing a quality control mixture pool of 12.5 μl from each sample (150 μl total) was prepared along with the other samples. These vials were sealed with Parafilm and stored at −80°C before the untargeted metabolomics analysis (Mackay et al., 2015). Samples were analyzed with a Shimadzu high-performance liquid chromatography (HPLC) (Nexera X2 LC-30AD, Kyoto, Japan) coupled to a Sciex TripleTOF 6600 high-resolution mass spectrometer (HRMS) for the separation and detection of various classes of metabolites in the samples using an untargeted metabolomics approach at the UTSW metabolomics core facility (https://www.utsouthwestern.edu/research/core-facilities/metabolomics/). Metabolites detected in at least three biological replicates were considered for further analysis. The peak area of metabolites was normalized to the total protein content determined from Mtb lysates, and pathway enrichment analysis was conducted using the online analytical tool Metaboanalyst (www.metaboanalyst.ca) and the BioCyc Database, employing the Mtb H37Rv reference genome (Karp et al., 2019).
Statistical analysis
Statistical analyses were performed using Prism 10 (GraphPad Software, Boston, MA) or Microsoft Excel. P < 0.05 was considered significant.
Conclusions
Mycobacterium tuberculosis (Mtb) is the causative agent of tuberculosis (TB), which is responsible for more deaths than any other infectious disease. The alarming prevalence of drug-tolerant Mtb strains has further exacerbated this global health crisis. Some pathogenic bacteria such as Mtb appear to increase levels of polyphosphate as a defense against antibiotics. We found that gallein, a small molecule inhibitor of bacterial polyphosphate kinases, strongly potentiates the ability of the frontline anti-tuberculosis drug isoniazid (INH) to inhibit the growth of Mtb both alone and in human macrophages. This has unveiled vulnerabilities in Mtb that could be strategically leveraged to reverse INH tolerance.
Data availability statement
The original contributions presented in the study are included in the article/Supplementary material, further inquiries can be directed to the corresponding authors.
Ethics statement
The studies involving humans were approved by Texas A&M University human subjects Institutional Review Board. The studies were conducted in accordance with the local legislation and institutional requirements. The participants provided their written informed consent to participate in this study.
Author contributions
RR: Conceptualization, Formal analysis, Investigation, Validation, Writing—original draft, Writing—review & editing. RG: Conceptualization, Funding acquisition, Supervision, Validation, Writing—review & editing.
Funding
The author(s) declare that financial support was received for the research, authorship, and/or publication of this article. This work was supported by National Institutes of Health grant GM139486.
Conflict of interest
RR and RG are inventors on a patent application for the use of gallein for the treatment of tuberculosis.
The author(s) declared that they were an editorial board member of Frontiers, at the time of submission. This had no impact on the peer review process and the final decision.
Publisher's note
All claims expressed in this article are solely those of the authors and do not necessarily represent those of their affiliated organizations, or those of the publisher, the editors and the reviewers. Any product that may be evaluated in this article, or claim that may be made by its manufacturer, is not guaranteed or endorsed by the publisher.
Supplementary material
The Supplementary Material for this article can be found online at: https://www.frontiersin.org/articles/10.3389/fmicb.2024.1369763/full#supplementary-material
References
Allison, K. R., Brynildsen, M. P., and Collins, J. J. (2011). Metabolite-enabled eradication of bacterial persisters by aminoglycosides. Nature 473, 216–220. doi: 10.1038/nature10069
Álvarez, R., Inostroza, O., Garavaglia, M., Minton, N. P., Paredes-Sabja, D., Gil, F., et al. (2018). Effect of antibiotic treatment on the formation of non-spore Clostridium difficile persister-like cells. J. Antimicrob. Chemother. 73, 2396–2399. doi: 10.1093/jac/dky186
Aschar-Sobbi, R., Abramov, A. Y., Diao, C., Kargacin, M. E., Kargacin, G. J., French, R. J., et al. (2008). High sensitivity, quantitative measurements of polyphosphate using a new DAPI-based approach. J. Fluoresc. 18, 859–866. doi: 10.1007/s10895-008-0315-4
Baek, S. H., Li, A. H., and Sassetti, C. M. (2011). Metabolic regulation of mycobacterial growth and antibiotic sensitivity. PLoS Biol. 9:e1001065. doi: 10.1371/journal.pbio.1001065
Bagcchi, S. (2023). WHO's global tuberculosis report 2022. Lancet Microbe 4:e20. doi: 10.1016/S2666-5247(22)00359-7
Bhat, S. A., Iqbal, I. K., and Kumar, A. (2016). Imaging the NADH:NAD(+) homeostasis for understanding the metabolic response of mycobacterium to physiologically relevant stresses. Front. Cell. Infect. Microbiol. 6:145. doi: 10.3389/fcimb.2016.00145
Brown, M. R., and Kornberg, A. (2004). Inorganic polyphosphate in the origin and survival of species. Proc. Natl. Acad. Sci. U. S. A. 101, 16085–16087. doi: 10.1073/pnas.0406909101
Chavez, F. P., Lunsdorf, H., and Jerez, C. A. (2004). Growth of polychlorinated-biphenyl-degrading bacteria in the presence of biphenyl and chlorobiphenyls generates oxidative stress and massive accumulation of inorganic polyphosphate. Appl. Environ. Microbiol. 70, 3064–3072. doi: 10.1128/AEM.70.5.3064-3072.2004
Cho, S., Lee, H. S., and Franzblau, S. (2015). Microplate Alamar Blue Assay (MABA) and Low Oxygen Recovery Assay (LORA) for Mycobacterium tuberculosis. Methods Mol. Biol. 1285, 281–292. doi: 10.1007/978-1-4939-2450-9_17
Chuang, Y. M., Bandyopadhyay, N., Rifat, D., Rubin, H., Bader, J. S., Karakousis, P. C., et al. (2015). Deficiency of the novel exopolyphosphatase Rv1026/PPX2 leads to metabolic downshift and altered cell wall permeability in Mycobacterium tuberculosis. MBio 6:e02428. doi: 10.1128/mBio.02428-14
Chuang, Y. M., Belchis, D. A., and Karakousis, P. C. (2013). The polyphosphate kinase gene ppk2 is required for Mycobacterium tuberculosis inorganic polyphosphate regulation and virulence. MBio 4, e00039–e00013. doi: 10.1128/mBio.00039-13
Chuang, Y. M., Dutta, N. K., Hung, C. F., Wu, T. C., Rubin, H., Karakousis, P. C., et al. (2016). Stringent response factors PPX1 and PPK2 play an important role in Mycobacterium tuberculosis metabolism, biofilm formation, and sensitivity to isoniazid in vivo. Antimicrob. Agents Chemother. 60, 6460–6470. doi: 10.1128/AAC.01139-16
Chugh, S., Tiwari, P., Suri, C., Gupta, S. K., Singh, P., Bouzeyen, R., et al. (2024). Polyphosphate kinase-1 regulates bacterial and host metabolic pathways involved in pathogenesis of Mycobacterium tuberculosis. Proc. Natl. Acad. Sci. U. S. A. 121:e2309664121. doi: 10.1073/pnas.2309664121
Cole, S. T., Brosch, R., Parkhill, J., Garnier, T., Churcher, C., Harris, D., et al. (1998). Deciphering the biology of Mycobacterium tuberculosis from the complete genome sequence. Nature 393, 537–544. doi: 10.1038/31159
Cui, C., Schoenfelt, K. Q., Becker, K. M., and Becker, L. (2021). Isolation of polymorphonuclear neutrophils and monocytes from a single sample of human peripheral blood. STAR Protoc. 2:100845. doi: 10.1016/j.xpro.2021.100845
De Smet, K. A. L., Weston, A., Brown, I. N., Young, D. B., and Robertson, B. D. (2000). Three pathways for trehalose biosynthesis in mycobacteria. Microbiology 146, 199–208. doi: 10.1099/00221287-146-1-199
Deakin, L. J., Clare, S., Fagan, R. P., Dawson, L. F., Pickard, D. J., West, M. R., et al. (2012). The Clostridium difficile spo0A gene is a persistence and transmission factor. Infect. Immun. 80, 2704–2711. doi: 10.1128/IAI.00147-12
Deb, C., Lee, C. M., Dubey, V. S., Daniel, J., Abomoelak, B., Sirakova, T. D., et al. (2009). novel in vitro multiple-stress dormancy model for Mycobacterium tuberculosis generates a lipid-loaded, drug-tolerant, dormant pathogen. PLoS ONE 4:e6077. doi: 10.1371/journal.pone.0006077
Dey, S., Lane, J. M., Lee, R. E., Rubin, E. J., and Sacchettini, J. C. (2010). Structural characterization of the Mycobacterium tuberculosis biotin biosynthesis enzymes 7,8-diaminopelargonic acid synthase and dethiobiotin synthetase. Biochemistry 49, 6746–6760. doi: 10.1021/bi902097j
Echeverría-Valencia, G. (2023). “Phagocytosis of Mycobacterium tuberculosis: a narrative of the uptaking and survival,” in Phagocytosis - Main Key of Immune System, eds A. Seyyed Shamsadin, and N. Entezar Mehrabi (Rijeka: IntechOpen), Ch. 3.
Elbein, A. D., Pan, Y., Pastuszak, I., and Carroll, D. (2003). New insights on trehalose: a multifunctional molecule. Glycobiology 13, 17R−27R. doi: 10.1093/glycob/cwg047
Flentie, K., Harrison, G. A., Tukenmez, H., Livny, J., Good, J. A. D., Sarkar, S., et al. (2019). Chemical disarming of isoniazid resistance in Mycobacterium tuberculosis. Proc. Natl. Acad. Sci. U. S. A. 116, 10510–10517. doi: 10.1073/pnas.1818009116
Franzblau, S. G., Witzig, R. S., McLaughlin, J. C., Torres, P., Madico, G., Hernandez, A., et al. (1998). Rapid, low-technology MIC determination with clinical Mycobacterium tuberculosis isolates by using the microplate Alamar Blue assay. J. Clin. Microbiol. 36, 362–366. doi: 10.1128/JCM.36.2.362-366.1998
Herbert, D., Paramasivan, C., Venkatesan, P., Kubendiran, G., Prabhakar, R., Mitchison, D., et al. (1996). Bactericidal action of ofloxacin, sulbactam-ampicillin, rifampin, and isoniazid on logarithmic-and stationary-phase cultures of Mycobacterium tuberculosis. Antimicrob. Agents Chemother. 40, 2296–2299. doi: 10.1128/AAC.40.10.2296
Jagannathan, V., Kaur, P., and Datta, S. (2010). Polyphosphate kinase from M. tuberculosis: an interconnect between the genetic and biochemical role. PLoS ONE 5:e14336. doi: 10.1371/journal.pone.0014336
Jain, P., Weinrick, B., Kalivoda, E., Yang, H., Munsamy, V., Vilcheze, C., et al. (2016). Dual-reporter mycobacteriophages (U2DRMs) reveal preexisting Mycobacterium tuberculosis persistent cells in human sputum. MBio 7, 01023–01016. doi: 10.1128/mBio.01023-16
Jarlier, V., and Nikaido, H. (1994). Mycobacterial cell wall: structure and role in natural resistance to antibiotics. FEMS Microbiol. Lett. 123, 11–18. doi: 10.1111/j.1574-6968.1994.tb07194.x
Kalscheuer, R., and Koliwer-Brandl, H. (2014). Genetics of mycobacterial trehalose metabolism. Microbiol. Spectr. doi: 10.1128/microbiolspec.MGM2-0002-2013
Karp, P. D., Billington, R., Caspi, R., Fulcher, C. A., Latendresse, M., Kothari, A., et al. (2019). The BioCyc collection of microbial genomes and metabolic pathways. Brief. Bioinformat. 20, 1085–1093. doi: 10.1093/bib/bbx085
Kim, K. S., Rao, N. N., Fraley, C. D., and Kornberg, A. (2002). Inorganic polyphosphate is essential for long-term survival and virulence factors in Shigella and Salmonella spp. Proc. Natl. Acad. Sci. U. S. A. 99, 7675–7680. doi: 10.1073/pnas.112210499
Koliwer-Brandl, H., Syson, K., van de Weerd, R., Chandra, G., Appelmelk, B., Alber, M., et al. (2016). Metabolic network for the biosynthesis of intra-and extracellular α-glucans required for virulence of Mycobacterium tuberculosis. PLoS Pathog. 12:e1005768. doi: 10.1371/journal.ppat.1005768
Kumble, K. D., and Kornberg, A. (1995). Inorganic polyphosphate in mammalian cells and tissues. J. Biol. Chem. 270, 5818–5822. doi: 10.1074/jbc.270.11.5818
Laasmaa, M., Vendelin, M., and Peterson, P. (2011). Application of regularized Richardson-Lucy algorithm for deconvolution of confocal microscopy images. J. Microsc. 243, 124–140. doi: 10.1111/j.1365-2818.2011.03486.x
Larsen, M. H., Biermann, K., Tandberg, S., Hsu, T., and Jacobs, W. R. Jr. (2007). Genetic manipulation of Mycobacterium tuberculosis. Curr. Protoc. Microbiol. Chapter 10:Unit 10A 2. doi: 10.1002/9780471729259.mc10a02s6
Lescat, M., Poirel, L., Tinguely, C., and Nordmann, P. A. (2019). Resazurin reduction-based assay for rapid detection of polymyxin resistance in Acinetobacter baumannii and Pseudomonas aeruginosa. J. Clin. Microbiol. 57:e01563-18. doi: 10.1128/JCM.01563-18
Lillie, R. D., Pizzolato, P., and Donaldson, P. T. (1974). Hematoxylin substitutes: gallein as a biological stain. Stain Technol. 49, 339–346. doi: 10.3109/10520297409117009
Liu, Y., Tan, S., Huang, L., Abramovitch, R. B., Rohde, K. H., Zimmerman, M. D., et al. (2016). Immune activation of the host cell induces drug tolerance in Mycobacterium tuberculosis both in vitro and in vivo. J. Exp. Med. 213, 809–825. doi: 10.1084/jem.20151248
Mackay, G. M., Zheng, L., van den Broek, N. J., and Gottlieb, E. (2015). Analysis of cell metabolism using LC-MS and isotope tracers. Meth. Enzymol. 561, 171–196. doi: 10.1016/bs.mie.2015.05.016
Manca, B., Buffi, G., Magri, G., Del Vecchio, M., Taddei, A. R., Pezzicoli, A., et al. (2023). Functional characterization of the gonococcal polyphosphate pseudo-capsule. PLoS Pathog. 19:e1011400. doi: 10.1371/journal.ppat.1011400
McCune, R. M. Jr, and Tompsett, R. (1956). Fate of Mycobacterium tuberculosis in mouse tissues as determined by the microbial enumeration technique: I. The persistence of drug-susceptible tubercle bacilli in the tissues despite prolonged antimicrobial therapy. J. Exp. Med. 104, 737–762. doi: 10.1084/jem.104.5.737
Mehra, A., and Philips, J. A. (2014). Analysis of mycobacterial protein secretion. Bio Protoc. 4:e1159. doi: 10.21769/BioProtoc.1159
Mehta, M., Rajmani, R. S., and Singh, A. (2016). Mycobacterium tuberculosis WhiB3 responds to vacuolar pH-induced changes in mycothiol redox potential to modulate phagosomal maturation and virulence. J. Biol. Chem. 291, 2888–2903. doi: 10.1074/jbc.M115.684597
Mookherjee, N., Brown, K. L., and Hancock, R. E. W. (2013). “Cathelicidins,” in Handbook of Biologically Active Peptides, ed A. J. Kastin (Boston, MA: Academic Press), 77–84.
Morris, R. P., Nguyen, L., Gatfield, J., Visconti, K., Nguyen, K., Schnappinger, D., et al. (2005). Ancestral antibiotic resistance in Mycobacterium tuberculosis. Proc. Natl. Acad. Sci. U. S. A. 102, 12200–12205. doi: 10.1073/pnas.0505446102
Nandakumar, M., Nathan, C., and Rhee, K. Y. (2014). Isocitrate lyase mediates broad antibiotic tolerance in Mycobacterium tuberculosis. Nat. Commun. 5:4306. doi: 10.1038/ncomms5306
Neville, N., Roberge, N., Ji, X., Stephen, P., Lu, J. L., Jia, Z. A., et al. (2021). Dual-specificity inhibitor targets polyphosphate kinase 1 and 2 enzymes to attenuate virulence of Pseudomonas aeruginosa. MBio 12:e0059221. doi: 10.1128/mBio.00592-21
Nguyen, D., Joshi-Datar, A., Lepine, F., Bauerle, E., Olakanmi, O., Beer, K., et al. (2011). Active starvation responses mediate antibiotic tolerance in biofilms and nutrient-limited bacteria. Science 334, 982–986. doi: 10.1126/science.1211037
Nguyen, L., and Thompson, C. J. (2006). Foundations of antibiotic resistance in bacterial physiology: the mycobacterial paradigm. Trends Microbiol. 14, 304–312. doi: 10.1016/j.tim.2006.05.005
Nikaido, H. (1994). Prevention of drug access to bacterial targets: permeability barriers and active efflux. Science 264, 382–388. doi: 10.1126/science.8153625
Ojha, A. K., Baughn, A. D., Sambandan, D., Hsu, T., Trivelli, X., Guerardel, Y., et al. (2008). Growth of Mycobacterium tuberculosis biofilms containing free mycolic acids and harbouring drug-tolerant bacteria. Mol. Microbiol. 69, 164–174. doi: 10.1111/j.1365-2958.2008.06274.x
Pilling, D., Kitas, G. D., Salmon, M., and Bacon, P. A. (1989). The kinetics of interaction between lymphocytes and magnetic polymer particles. J. Immunol. Methods 122, 235–241. doi: 10.1016/0022-1759(89)90269-X
Pilling, D., Vakil, V., and Gomer, R. H. (2009). Improved serum-free culture conditions for the differentiation of human and murine fibrocytes. J. Immunol. Methods 351, 62–70. doi: 10.1016/j.jim.2009.09.011
Pizarro-Guajardo, M., Calderon-Romero, P., Castro-Cordova, P., Mora-Uribe, P., and Paredes-Sabja, D. (2016a). Ultrastructural variability of the exosporium layer of Clostridium difficile spores. Appl. Environ. Microbiol. 82, 2202–2209. doi: 10.1128/AEM.03410-15
Pizarro-Guajardo, M., Calderon-Romero, P., and Paredes-Sabja, D. (2016b). Ultrastructure variability of the exosporium layer of Clostridium difficile spores from sporulating cultures and biofilms. Appl. Environ. Microbiol. 82, 5892–5898. doi: 10.1128/AEM.01463-16
Qi, L. S., Larson, M. H., Gilbert, L. A., Doudna, J. A., Weissman, J. S., Arkin, A. P., et al. (2013). Repurposing CRISPR as an RNA-guided platform for sequence-specific control of gene expression. Cell 152, 1173–1183. doi: 10.1016/j.cell.2013.02.022
Quemard, A., Sacchettini, J. C., Dessen, A., Vilcheze, C., Bittman, R., Jacobs, W. R. Jr., et al. (1995). Enzymatic characterization of the target for isoniazid in Mycobacterium tuberculosis. Biochemistry 34, 8235–8241. doi: 10.1021/bi00026a004
Rampersad, S. N. (2012). Multiple applications of alamar blue as an indicator of metabolic function and cellular health in cell viability bioassays. Sensors 12, 12347–12360. doi: 10.3390/s120912347
Rao, N. N., Gomez-Garcia, M. R., and Kornberg, A. (2009). Inorganic polyphosphate: essential for growth and survival. Annu. Rev. Biochem. 78, 605–647. doi: 10.1146/annurev.biochem.77.083007.093039
Rao, N. N., Liu, S., and Kornberg, A. (1998). Inorganic polyphosphate in Escherichia coli: the phosphate regulon and the stringent response. J. Bacteriol. 180, 2186–2193. doi: 10.1128/JB.180.8.2186-2193.1998
Rashid, M. H., and Kornberg, A. (2000). Inorganic polyphosphate is needed for swimming, swarming, and twitching motilities of Pseudomonas aeruginosa. Proc. Natl. Acad. Sci. U. S. A. 97, 4885–4890. doi: 10.1073/pnas.060030097
Rashid, M. H., Rumbaugh, K., Passador, L., Davies, D. G., Hamood, A. N., Iglewski, B. H., et al. (2000). Polyphosphate kinase is essential for biofilm development, quorum sensing, and virulence of Pseudomonas aeruginosa. Proc. Natl. Acad. Sci. U. S. A. 97, 9636–9641. doi: 10.1073/pnas.170283397
Rawat, R., Whitty, A., and Tonge, P. J. (2003). The isoniazid-NAD adduct is a slow, tight-binding inhibitor of InhA, the Mycobacterium tuberculosis enoyl reductase: adduct affinity and drug resistance. Proc. Natl. Acad. Sci. U. S. A. 100, 13881–13886. doi: 10.1073/pnas.2235848100
Rijal, R., Cadena, L. A., Smith, M. R., Carr, J. F., and Gomer, R. H. (2020). Polyphosphate is an extracellular signal that can facilitate bacterial survival in eukaryotic cells. Proc. Natl. Acad. Sci. U. S. A. 117, 31923–31934. doi: 10.1073/pnas.2012009117
Roberge, N. A. (2021). Broad-Spectrum Inhibitor of Bacterial Polyphosphate Homeostasis Attenuates Virulence Factors and Helps Reveal Novel Physiology of K. Pneumoniaeand A. Baumannii. Ontario, CA: Queen's University, 93.
Rock, J. M., Hopkins, F. F., Chavez, A., Diallo, M., Chase, M. R., Gerrick, E. R., et al. (2017). Programmable transcriptional repression in mycobacteria using an orthogonal CRISPR interference platform. Nat. Microbiol. 2:16274. doi: 10.1038/nmicrobiol.2016.274
Rohde, K. H., and Sorci, L. (2020). The prospective synergy of antitubercular drugs with NAD biosynthesis inhibitors. Front. Microbiol. 11:634640. doi: 10.3389/fmicb.2020.634640
Sampson, S. L., Dascher, C. C., Sambandamurthy, V. K., Russell, R. G., Jacobs, W. R. Jr., et al. (2004). Protection elicited by a double leucine and pantothenate auxotroph of Mycobacterium tuberculosis in guinea pigs. Infect. Immun. 72, 3031–3037. doi: 10.1128/IAI.72.5.3031-3037.2004
Sanz, G., Leray, I., Muscat, A., Acquistapace, A., Cui, T., Riviere, J., et al. (2017). Gallein, a Gbetagamma subunit signalling inhibitor, inhibits metastatic spread of tumour cells expressing OR51E2 and exposed to its odorant ligand. BMC Res. Notes 10:541. doi: 10.1186/s13104-017-2879-z
Sarabhai, S., Harjai, K., Sharma, P., and Capalash, N. (2015). Ellagic acid derivatives from Terminalia chebula Retz. increase the susceptibility of Pseudomonas aeruginosa to stress by inhibiting polyphosphate kinase. J. Appl. Microbiol. 118, 817–825. doi: 10.1111/jam.12733
Schami, A., Islam, M. N., Belisle, J. T., and Torrelles, J. B. (2023). Drug-resistant strains of Mycobacterium tuberculosis: cell envelope profiles and interactions with the host. Front. Cell. Infect. Microbiol. 13:1274175. doi: 10.3389/fcimb.2023.1274175
Schindelin, J., Arganda-Carreras, I., Frise, E., Kaynig, V., Longair, M., Pietzsch, T., et al. (2012). Fiji: an open-source platform for biological-image analysis. Nat. Methods 9, 676–682. doi: 10.1038/nmeth.2019
Sebastian, J., Nair, R. R., Swaminath, S., and Ajitkumar, P. (2020). Mycobacterium tuberculosis cells surviving in the continued presence of bactericidal concentrations of rifampicin in vitro develop negatively charged thickened capsular outer layer that restricts permeability to the antibiotic. Front. Microbiol. 11:554795. doi: 10.3389/fmicb.2020.554795
Silver, L. L. (2011). Challenges of antibacterial discovery. Clin. Microbiol. Rev. 24, 71–109. doi: 10.1128/CMR.00030-10
Singh, R., Singh, M., Arora, G., Kumar, S., Tiwari, P., Kidwai, S., et al. (2013). Polyphosphate deficiency in Mycobacterium tuberculosis is associated with enhanced drug susceptibility and impaired growth in guinea pigs. J. Bacteriol. 195, 2839–2851. doi: 10.1128/JB.00038-13
Smith, P. A., and Romesberg, F. E. (2007). Combating bacteria and drug resistance by inhibiting mechanisms of persistence and adaptation. Nat. Chem. Biol. 3, 549–556. doi: 10.1038/nchembio.2007.27
Smrcka, A. V. (2013). Molecular targeting of Galpha and Gbetagamma subunits: a potential approach for cancer therapeutics. Trends Pharmacol. Sci. 34, 290–298. doi: 10.1016/j.tips.2013.02.006
Sureka, K., Dey, S., Datta, P., Singh, A. K., Dasgupta, A., Rodrigue, S., et al. (2007). Polyphosphate kinase is involved in stress-induced mprAB-sigE-rel signalling in mycobacteria. Mol. Microbiol. 65, 261–276. doi: 10.1111/j.1365-2958.2007.05814.x
Sureka, K., Sanyal, S., Basu, J., and Kundu, M. (2009). Polyphosphate kinase 2: a modulator of nucleoside diphosphate kinase activity in mycobacteria. Mol. Microbiol. 74, 1187–1197. doi: 10.1111/j.1365-2958.2009.06925.x
Thayil, S. M., Morrison, N., Schechter, N., Rubin, H., and Karakousis, P. C. (2011). The role of the novel exopolyphosphatase MT0516 in Mycobacterium tuberculosis drug tolerance and persistence. PLoS ONE 6:e28076. doi: 10.1371/journal.pone.0028076
Tunpiboonsak, S., Mongkolrob, R., Kitudomsub, K., Thanwatanaying, P., Kiettipirodom, W., Tungboontina, Y., et al. (2010). Role of a Burkholderia pseudomallei polyphosphate kinase in an oxidative stress response, motilities, biofilm formation. J. Microbiol. 48, 63–70. doi: 10.1007/s12275-010-9138-5
Umbarger, H. E. (1978). Amino acid biosynthesis and its regulation. Annu. Rev. Biochem. 47, 532–606. doi: 10.1146/annurev.bi.47.070178.002533
Van den Bergh, B., Fauvart, M., and Michiels, J. (2017). Formation, physiology, ecology, evolution and clinical importance of bacterial persisters. FEMS Microbiol. Rev. 41, 219–251. doi: 10.1093/femsre/fux001
Vilchèze, C., and Jacobs, W. R. Jr. (2019). The isoniazid paradigm of killing, resistance, and persistence in Mycobacterium tuberculosis. J. Mol. Biol. 431, 3450–3461. doi: 10.1016/j.jmb.2019.02.016
Xie, L., Rajpurkar, A., Quarles, E., Taube, N., Rai, A. S., Erba, J., et al. (2019). Accumulation of nucleolar inorganic polyphosphate is a cellular response to cisplatin-induced apoptosis. Front. Oncol. 9:1410. doi: 10.3389/fonc.2019.01410
Zhang, Q., Li, Y., and Tang, C. M. (2010). The role of the exopolyphosphatase PPX in avoidance by Neisseria meningitidis of complement-mediated killing. J. Biol. Chem. 285, 34259–34268. doi: 10.1074/jbc.M110.154393
Keywords: Mycobacterium tuberculosis, gallein, isoniazid, antibiotic tolerance, polyphosphate, metabolomics
Citation: Rijal R and Gomer RH (2024) Gallein potentiates isoniazid's ability to suppress Mycobacterium tuberculosis growth. Front. Microbiol. 15:1369763. doi: 10.3389/fmicb.2024.1369763
Received: 12 January 2024; Accepted: 01 April 2024;
Published: 15 April 2024.
Edited by:
Octavio Luiz Franco, Catholic University of Brasilia (UCB), BrazilReviewed by:
Satya Deo Pandey, University of Louisville, United StatesPetros C. Karakousis, Johns Hopkins University, United States
Copyright © 2024 Rijal and Gomer. This is an open-access article distributed under the terms of the Creative Commons Attribution License (CC BY). The use, distribution or reproduction in other forums is permitted, provided the original author(s) and the copyright owner(s) are credited and that the original publication in this journal is cited, in accordance with accepted academic practice. No use, distribution or reproduction is permitted which does not comply with these terms.
*Correspondence: Ramesh Rijal, cmlqYWxyYW1lc2hAdGFtdS5lZHU=; Richard H. Gomer, cmdvbWVyQHRhbXUuZWR1