- Laboratório de Microbiologia Molecular e Clínica, Universidade São Francisco, Bragança Paulista, Brazil
Klebsiella pneumoniae is among the most relevant pathogens worldwide, causing high morbidity and mortality, which is worsened by the increasing rates of antibiotic resistance. It is a constituent of the host microbiota of different mucosa, that can invade and cause infections in many different sites. The development of new treatments and prophylaxis against this pathogen rely on animal models to identify potential targets and evaluate the efficacy and possible side effects of therapeutic agents or vaccines. However, the validity of data generated is highly dependable on choosing models that can adequately reproduce the hallmarks of human diseases. The present review summarizes the current knowledge on animal models used to investigate K. pneumoniae infections, with a focus on mucosal sites. The advantages and limitations of each model are discussed and compared; the applications, extrapolations to human subjects and future modifications that can improve the current techniques are also presented. While mice are the most widely used species in K. pneumoniae animal studies, they present limitations such as the natural resistance to the pathogen and difficulties in reproducing the main steps of human mucosal infections. Other models, such as Drosophila melanogaster (fruit fly), Caenorhabditis elegans, Galleria mellonella and Danio rerio (zebrafish), contribute to understanding specific aspects of the infection process, such as bacterial lethality and colonization and innate immune system response, however, they but do not present the immunological complexity of mammals. In conclusion, the choice of the animal model of K. pneumoniae infection will depend mainly on the questions being addressed by the study, while a better understanding of the interplay between bacterial virulence factors and animal host responses will provide a deeper comprehension of the disease process and aid in the development of effective preventive/therapeutic strategies.
Introduction
Klebsiella pneumoniae is a widely distributed bacterium that colonizes the human skin, mouth, respiratory and gastrointestinal (GI) tracts asymptomatically. It is also one of the main causative agents of hospital infections such as urinary tract infections, pneumonia, liver abscesses, meningitis, and sepsis, being considered an opportunistic pathogen (Conlan et al., 2012; Gorrie et al., 2017; Joseph et al., 2021; Osbelt et al., 2021). In addition, K. pneumoniae is among the most relevant strains when considering the increase in antibiotic resistance worldwide, being classified by the World Health Organization (WHO) as a priority pathogen for which new drugs are needed (WHO, 2017). However, relying on the discovery of new drugs alone may not be enough to suppress the advance of these infections, especially when caused by multidrug-resistant isolates, so new strategies are fundamental to stop the steady increase of cases.
Infections caused by multi-drug resistant bacteria (including K. pneumoniae) pose a great burden to healthcare systems worldwide, from the hundreds of thousands of deaths, to the reduced life expectancy and disabilities, along with the cost of the treatment. K. pneumoniae is among the four main causes of death by antibiotic resistance bacteria, which are responsible for 929,000 annual deaths according to a study published in 2022 (Antimicrobial Resistance Collaborators, 2022).
K. pneumoniae strains can be classified in two major categories, namely classical (or common) and hypervirulent strains, based on traits such as the hypermucoviscosity (HMV) phenotype and increased expression of siderophores and fimbriae (Chang et al., 2021; Dai and Hu, 2022). There are a few differences in the profile of the infections caused by those types of K. pneumoniae. Usually, most cases occur within healthcare environments and are caused by classical strains. Such strains are commonly multi-drug resistant, especially to beta-lactams, including carbapenems. The most common infections caused by the classical strains are urinary tract infections, pneumonia and bacteremia (Paczosa and Mecsas, 2016; Dai and Hu, 2022).
Hypervirulent strains, on the other hand, are acquired in the community and are more invasive, being able to colonize additional sites and cause further damage, when compared to the classical strains. Another important difference between classical and hypervirulent strains is the historically higher susceptibility of the hypervirulent strains to antimicrobials, which is becoming less prevalent in hospital-acquired K. pneumoniae in many regions, especially in lower- or middle-income countries (Dai and Hu, 2022; Pulingam et al., 2022). Nevertheless, a very worrying development is the increase in reports of resistant hypervirulent strains leading to more severe, often fatal infections (Choby et al., 2020; Dai and Hu, 2022). A compilation of the most common infections caused by classical and hypervirulent strains of K. pneumoniae is shown in Figure 1.
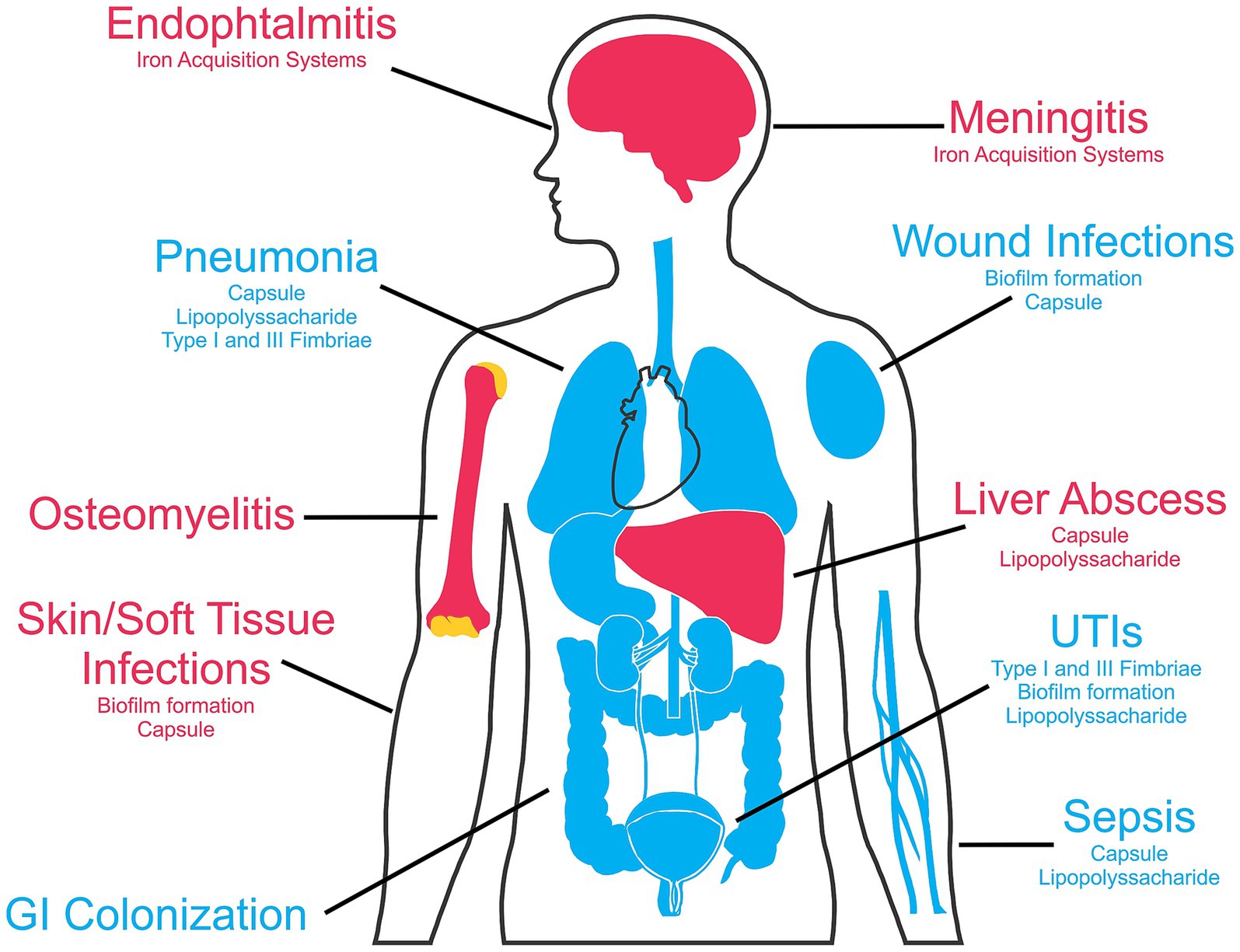
Figure 1. Klebsiella pneumoniae human infection sites and main associated virulence factors. The blue color indicates the infections caused by classical K. pneumoniae strains, while diseases commonly associated with hypervirulent strains are marked in red. This figure was partly generated using templates from Servier Medical Art (Servier), and SlidesGo (Freepik), licensed under a Creative Commons Attribution 3.0 unported license.
Overall, when considering the diversity of host niches that K. pneumoniae can infect and the overall disease burden, animal models are an important tool to elucidate infection mechanisms and develop new, safer therapeutic and prophylactic strategies against this pathogen. The present review focused on the in vivo platforms deployed to evaluate the pathogenicity of K. pneumoniae during infection of the main mucosal sites (gastrointestinal, respiratory and genitourinary). We explored the methodologies used to establish infection and the results achieved in each animal model. Complex vertebrates with anatomical features and immune response similar to ours, like rodents and primates, were compared with simpler models, such as invertebrates and zebrafish, considering specific disease outcomes, comparative virulence, and host defense mechanisms (Figure 2). The different techniques were compared regarding complexity, requirement for specific equipment, relevance of the results and applicability to humans, and the more robust techniques were highlighted in each case.
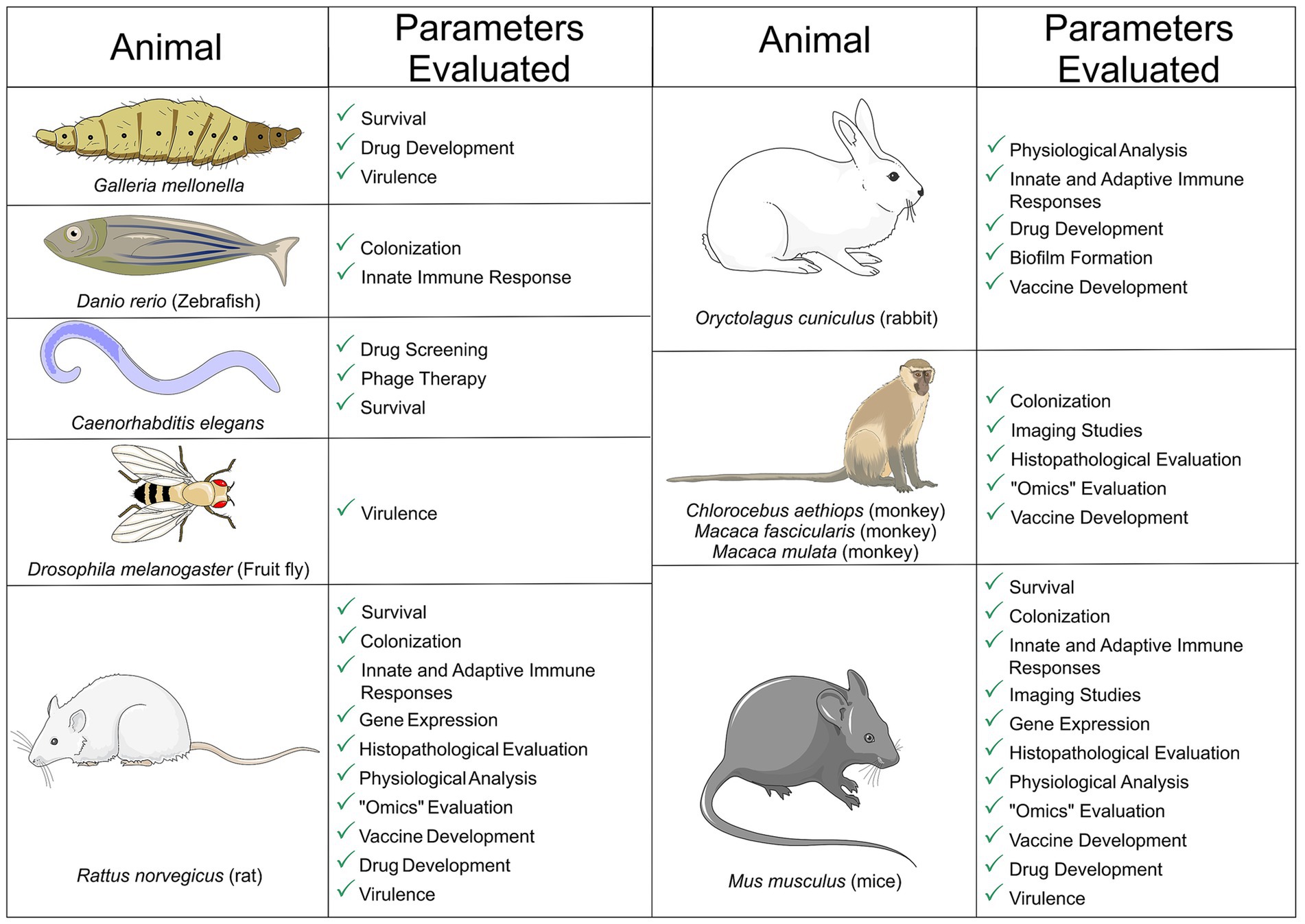
Figure 2. Animal models of Klebsiella pneumoniae mucosal infections. The main infection outcomes are shown for each model. The figure was partly generated using Servier Medical Art, provided by Servier, licensed under a Creative Commons Attribution 3.0 unported license. This figure was partly generated using templates from Servier Medical Art (Servier), licensed under a Creative Commons Attribution unported license.
Finally, we reviewed the current models used to evaluate the host-pathogen interactions during disease, and the efficacy of therapeutic agents and/or potential vaccine candidates against infections by K. pneumoniae.
This review includes papers written in English, chosen using the keywords correlated to the topics “animal models,” “Klebsiella pneumoniae” and “mucosal infection,” with an emphasis in articles published within the last 10 years in journals indexed in the PubMed database. Approval by animal research ethics committee was also used as a selection criterium.
Animal models of infection by Klebsiella pneumoniae
Animal models of Klebsiella pneumoniae respiratory infections
Hospital or community-acquired K. pneumoniae respiratory infections represent a serious public health threat, causing high mortality due to the common development into bacteremia (Antimicrobial Resistance Collaborators, 2022; Chen et al., 2022). K. pneumoniae is one of the main causes of pneumonia worldwide as a result from widespread colonization (Martin and Bachman, 2018; Chen et al., 2022). In some countries, the reports of K. pneumoniae community-acquired pneumonia cases which progressed to bacteremia, has surpassed those caused by Streptococcus pneumoniae—the leading cause of bacterial pneumonia (Lin et al., 2010).
The main models used to investigate the hallmarks of respiratory infections by K pneumoniae are mouse and rat, with a few studies being conducted in other mammals such as rabbits and monkeys. The different models of respiratory infections (summarized in Table 1) will be discussed individually in the next sections.
Mouse infection models
In mice, the main method to induce pneumonia is the direct instillation of bacteria in the mice nasopharynx through the nasal cavity (Wieland et al., 2011; Geller et al., 2018). This model has the advantages of the absence of any surgical procedure, in addition to the easy and quick manipulation of the animal. An important factor to consider in this type of infection is the volume of bacteria applied to the mouse. Lower volumes such as 5–10 μL can carry little to none of the inoculum into the lungs and usually promote only local nasopharyngeal colonization. To cause pneumonia, volumes between 25 and 50 μL are necessary, coupled with anesthesia to allow aspiration into the lungs. Interestingly, higher volumes do not appear to enhance lung delivery (Southam et al., 2002) and increase the risk of death by suffocation. Several different mouse strains have been tested using this inoculation model, including BALB/c (Kumar et al., 2020), C57BL/6 J (Wolff et al., 2021), CD-1 (Russo et al., 2015), Swiss (Jain et al., 2015), ICR (Yoshida et al., 2001), C3H/HeN and C3H/HeJ (parental toll-like receptor 4 deficient strain) (Branger et al., 2004), MF1 (Mabrook et al., 2022) and Kunming mice (Li-Juan et al., 2022). Similarly, there was also a great variety in the methods of anesthesia, which included, halothane (Lavender et al., 2005), isoflurane (Meijer et al., 2021), ketamine (Dong et al., 2021), ketamine and medetomidine (Otto et al., 2021) or xylazine mix (Zhang et al., 2021). A pneumonia model using direct bacterial instillation was established to evaluate the yersiniabactin receptor FyuA as a recombinant vaccine candidate against respiratory K pneumoniae infections in BALB/c mice. In the study, the authors used 103 colony forming units (CFU) of a K2 isolate, American Type Culture Collection (ATCC) 43816 diluted in 50 μL. The control group showed an increased bacterial burden in the lungs, 48 h following infection, as well as higher levels of the inflammatory cytokines interleukin 17 (IL-17), tumor necrosis factor α (TNF-α) and interleukin 1β (IL-1β), oxidative stress markers myeloperoxidase (MPO) and nitrous oxide (NO), and histological damage, while recombinant FyuA immunized mice exhibited increased levels of interleukin 6 (IL-6) and interleukin 12 (IL-12), with a reduced bacterial burden in the lungs, 48 h following infection (Kumar et al., 2020). A similar methodology was applied to C57BL6/J mice, using the same bacterial strain (ATCC 43816) to analyze changes in the lung and gut microbiome following respiratory infection by K. pneumoniae and Streptococci. An early production of TNF-α was detected in the mouse lungs, followed by lung colonization at 12 h and dissemination to the bloodstream at 18 h post infection. These changes were accompanied by a shift in bacterial distribution in the lungs, with an increase in Klebsiella and decrease in Streptococci.
Another method of inoculation of bacteria is directly in the trachea, via a small surgery, whereas an incision exposing the trachea allows the inoculum, with volume ranging from 25 to 30 μL, to be delivered with aid of a 26- or 30-gage needle (Zeng et al., 2003; Xu et al., 2014). The mouse lineage most frequently used is C57BL/6 background (Zeng et al., 2003, 2005; Barletta et al., 2012; Xu et al., 2014; Vieira et al., 2016; Morinaga et al., 2019), although NIH/Swiss (Fagundes et al., 2012) and BALB/c mice have also been tested (Deng et al., 2004; Xu et al., 2014; Liu J. et al., 2022). Anesthesia was performed either with a xylazine-ketamine mix (Zeng et al., 2003, 2005; Barletta et al., 2012; Fagundes et al., 2012; Xu et al., 2014; Vieira et al., 2016) or isoflurane (Morinaga et al., 2019). Following the procedure, the incision is closed with surgical staples or Vetbond (Rosen et al., 2015). One example of pneumonia induced by intratracheal bacteria inoculation is the work conducted by Zeng and colleagues (Zeng et al., 2003), in which infection was caused by intratracheal delivery of 103 CFU of the ATCC 43816 strain in 30 μL of final volume, through a 26-gage needle. The authors compared the immune response in control mice versus those previously injected with a recombinant, Ad5 human adenovirus-based platform expressing the macrophage inflammatory protein-1 alpha complementary DNA. The latter exhibited recruitment of neutrophils and activated NK cells to the lungs, as well as increased expression of gamma interferon (IFN-γ), which correlated with reduced bacterial counts in the lungs and bloodstream (Zeng et al., 2003). However, tracheal inoculation is possible without the need of surgical procedures. The suspension can be given straight to the lungs of the anesthetized animals by placing a metallic cannula with an inner tube, at the opening of the left bronchus, with 20 μL of volume injected with a micro-injection syringe. The bacteria are suspended in melt agar medium, which serves as an infection enhancer, providing a protective milieu for the inoculum. This method allowed the stable colonization of mice and rat lungs by multiple pathogens, including K. pneumoniae (Hoover et al., 2017). However, the authors conclude that this model is not suitable for evaluating survival as an endpoint, but rather should be used to determine CFU counts in the lungs. Intratracheal spraying was also performed to induce pneumonia in BALB/c mice anesthetized with pentobarbital. The mice were given 25 μL of K. pneumoniae 700603, at a concentration of 3 × 109 (Gou et al., 2021). Similarly, K. pneumoniae NTUH-K2044 (hypervirulent K1 strain, 2 × 104 CFU) was aerosolized and given in the tracheal bifurcation of female C57BL/6Cnc mice anesthetized with pentobarbital (Zheng et al., 2022). This infection model using the same hypervirulent strain was lethal for the mice within 48 h of challenge. The transcriptome of the infected animals showed an increased expression of genes related to acute inflammatory responses after 12 h of infection, with migration of granulocytes, monocytes and macrophages (Zheng et al., 2022).
K. pneumoniae-induced pneumonia can also be achieved with retropharyngeal inoculation. In this model, the inoculum was administered in C57BL/6 J mice, after isoflurane anesthesia (Bachman et al., 2015; Holden et al., 2016). This pneumonia model was used to evaluate the contribution of siderophore secretion for lung inflammation and bacteria dissemination during infection. A mutant ΔtonB strain that secretes, but does not import the siderophores, induced the release of IL-6 and the chemokines CXCL1 and CXCL2 in lung homogenates, colonized the lungs and spread to the spleen. Comparatively, mutant strains lacking different combinations of siderophores (enterobactin, salmochelin, and yersiniabactin), showed mixed results. Overall, the authors propose that siderophores play an essential role in the pathogenicity of K. pneumoniae respiratory infections, via interference with the immune system (Holden et al., 2016).
Another delivery mechanism is via laryngoscopy. Following pentobarbital anesthesia, the animal is positioned in a 45-degree angle and with aid of a laryngoscope blade, the trachea is exposed, and the bacteria are injected with a soft-end needle (Gu et al., 2021). Using the method developed by Spoelstra et al. (2007), a vaccine based on inactivated Acinetobacter baumanii was able to cross-protect against K. pneumoniae pneumonia, following intranasal challenge. Using the same pneumonia model, an inactivated, whole cell vaccine based on a K. pneumoniae isolate also reduced the death toll in the immunized mice (Gu et al., 2021).
A gavage or feeding needle can also be used to inoculate and establish the infection. This model was carried out in ICR:CD-1 (Swiss background) and Swiss mice, where 106 to 107 CFU of K. pneumoniae was given in 30–50 μL of volume directly in the trachea, through the blunt-end needle, in anesthetized animals (Cortés et al., 2002; Rouse et al., 2006). Following the inoculation of 107 CFU of an extended-spectrum beta lactamase (ESBL) and non-ESBL producing K. pneumoniae strains, the animals were treated with different cephalosporins and the ability of the drugs to prevent colonization was evaluated. In the group challenged with the ESBL-producing strain, neither of the antimicrobials were capable to prevent colonization, while in the non-ESBL group, antibiotic treatment blocked colonization in some animals (Rouse et al., 2006).
It is also possible to deliver bacteria directly in the trachea by positioning the pipette tip above the mouse vocal cords (Zhao et al., 2015; Olonisakin et al., 2016; Griepentrog et al., 2020). The authors used a 200 μL tip to deliver an inoculum of 103 CFU of ATCC 43816 in the colonization experiments (104 in the lethal model) in wild-type C57BL6/J and the isogenic, thrombospondin-1 negative mutants. The work demonstrated that in the mutant group, the animals survived longer, with a reduced bacterial burden in the lungs and spleen and had a lower pulmonary histopathology score. Cytokine and MPO expression levels were also reduced (Zhao et al., 2015).
Similarly, the tongue pull technique can be used to reach the trachea. With aid of a forceps, the tongue is pulled out and the bacteria are inoculated in the trachea of the anesthetized animal (Zhang G. et al., 2019). This technique was used to deliver 50 μL of the ATCC 43816 strain to C57BL6/J mice. The animals treated with imipenem in combination with andrographolide sulfonate (an anti-inflammatory agent), showed 100% survival rate after challenge, while also reducing the CFU count in the lungs, with controlled inflammation and reduced lung tissue damage parameters (Zhang G. et al., 2019).
An otoscope can also be used to deliver the bacteria into the lungs (Nikouee et al., 2021). This is an interesting technique, since other methods such as tracheostomy can be stressful and induce bleeding and inflammation in the animal (Thomas et al., 2014). With the aid of the otoscope, a catheter was positioned in the trachea to deliver the inoculum to anesthetized ICR mice under immunosuppression with cyclophosphamide. The study intended to conduct a real-time monitoring of infection using a bioluminescent K. pneumoniae strain and found that the group treated with the highest immunosuppressor dosage presented increased bioluminescence in the lungs and tissue damage (Hu et al., 2020).
This method was also applied to male C57BL6/J mice, using 3 × 107 CFU of the ATCC 43816 strain to evaluate the role of Beclin-1, an autophagy initiation factor, in pneumosepsis. Overexpression of Beclin-1 resulted in increased autophagy activation and reduced the burden, inflammation and tissue damage during infection, in comparison with the wild-type animals (Nikouee et al., 2021).
Although some of the endpoints varied among the studies, the results suggest that different methods of inoculation promote lung disease with inflammatory infiltrates and increased bacterial loads, and therefore may be used to investigate K. pneumoniae infection. An important aspect to be considered is bacterial dissemination from the lungs to other sites—a situation that is frequently observed in humans and is associated with poor prognosis. When considering the reviewed studies, invasiveness is majorly associated with the virulence and dose of the strain used to cause infection, and less a result of the inoculation method. Hypervirulent strains possessing multiple virulence factors, such as KPPR1-derived strains (including ATCC 43816) are highly lethal to mice and provide an interesting platform for investigating disseminated disease. However, since they kill the mice fast, these strains are usually not ideal for evaluating adaptive immune responses. Classical strains, on the other hand, are rapidly cleared from mice and are not suitable for survival studies (Russo et al., 2018, 2021).
A limitation common to all mouse studies is that mice do not die of bacterial pneumonia, but from disseminated infection with extremely high bacterial loads in the blood and major organs. Human patients, on the other hand, may present much lower bacterial counts and perish from the intense inflammatory responses associated with infection.
Rat infection models
In rats, pneumonia models were established either bilaterally or on the left side-only. For the induction of pneumonia in both lungs, Sprague–Dawley or RP–AEur–RijHsd rats were used. The animals were anesthetized with either isoflurane or medetomidine, intubated, immobilized vertically, and inoculated with 60 μL of ESBL and carbapenemase-producing K. pneumoniae isolates (KPC) (van der Weide et al., 2020a,b) to test the efficacy of antibiotic treatment. The use of tigecycline prevented the death of all animals against KPC-induced pneumonia/septicemia, while the group treated with meropenem was not protected. Meanwhile, in the ESBL group, all animals survived when treated with meropenem (Van der Weide et al., 2020b). Another study used tracheal instillation with 2.4 × 108 CFU of strain 46,114 of K. pneumoniae to induce pneumonia in Sprague–Dawley rats anesthetized with chloral hydrate. By employing this inoculation method, the effects of the Dusuqing granules, a compound based on an herb used in Chinese traditional medicine, was evaluated in the lung inflammatory process caused by K. pneumoniae infection. The treatment was able to reduce the tissue inflammation and damage. Cytokine and chemokine levels were reduced in the lungs of the treated animals, as were the leukocytes in the blood and the bronchoalveolar lavage fluid The authors hypothesize that the anti-inflammatory modulation is related to the downregulation of the NF-κB/MAPK signaling pathway (Mei et al., 2017).
An alternative delivery method in rats is the use of a 22-gage catheter intranasally, through the trachea, in which 50 μL of the K. pneumoniae CMCC (B) 46,117 suspension was given to Sprague–Dawley rats (Gu et al., 2022). Using the catheter to deliver the bacterial load, the pneumonia model was employed to investigate the effects of the coadministration of azithromycin with a traditional Chinese medicine formulation. The antibiotic mix improved the bacterial clearance and reduced inflammation parameters (Gu et al., 2022) Direct instillation of the bacterial suspension is also possible in rats. To establish an exudative pneumonia model of K. pneumoniae, Wistar male rats were given 50 μL of ATCC 1705 intranasally, in combination with lipopolysaccharide (LPS) administered intraperitoneally daily, for 5 days. This model was used to evaluate the effects of a traditional Chinese medicine formulation in the prophylaxis or treatment of lung infection. While the compound did not show direct antibacterial activity, it reduced the CFU counts in the bronchoalveolar lavage fluid (BALF) and histological damage in the lungs, while improving physiological parameters. Seric IL-6 and alveolar lavage IL-1β cytokine expression was also reduced in the treated group. While the treatment did induce some enhancement, the pre-treatment showed no effect in the pneumonia establishment (Wang et al., 2022). Similarly, female Sprague–Dawley rats anesthetized with isoflurane received 50 μL of an inoculum containing 107 CFU of a multi-drug resistant clinical strain with similar volumes in both nares. The authors used a zinc oxide nanoparticle in combination with sulphadiazine, delivered through aerosolization, to evaluate protection against pneumonia. After 4 days, the bacterial counts in the lungs were reduced and the histopathological signs of inflammation tissue damage were diminished (Aljohani et al., 2022).
Left side pneumonia models were established in female RP–AEur–RijHsd rats, where the animals were anesthetized with fluanisone and fentanyl, then pentobarbital. After the procedure, the left bronchus was intubated, and a bacterial load of 106 CFU of K. pneumoniae strain 43,816 were administered to the left lung, diluted in 200 μL of saline (Schiffelers et al., 2000, 2001a,b; Bakker-Woudenberg et al., 2001). The model was used to demonstrate the kinetics of the deposition and the effect of polyethylene glycol coating of liposomes in the target sites. Lung inflammation induced by K. pneumoniae infection resulted in enhanced liposomal deposition, possibly through increased capillary permeability in the inflamed tissue (Schiffelers et al., 2000).
Lobar pneumonia has also been successfully induced in rats using a catheter surgically inserted through the trachea. In female Wistar rats, 107 CFU of K. pneumoniae National Collection of Type Cultures (NCTC) 5055 (K2/O1 strain) in 1 mL of final volume was given straight to the lungs, following an incision in the rat’s trachea, while the animal was anesthetized with pentobarbital, and immobilized in a supinated position (Chhibber et al., 2003, 2004). One of the applications of this model was the use of LPS, or liposomes with a LPS coating, as a vaccine against K. pneumoniae pneumonia. Both the pure LPS and the LPS-coated liposomes were able to reduce the bacterial burden in the lungs (Chhibber et al., 2004). A similar model was applied in Sprague–Dawley rats, whereas 200 μL of the inoculum (1.3 × 108 CFU of an ESBL-producing, clinical isolate) was administered with a 26-gage needle, following the exposal of the trachea. The anesthesia was induced with ketamine. This model was used to demonstrate the effects of the exposure of the animals to different concentrations of nitric oxide and oxygen in the treatment of K. pneumonia-induced pneumonia. Varied combinations of nitric oxide and lowered oxygen concentration were shown to reduce the total bacterial count in the lungs and blood, while diminishing the expression of the proinflammatory cytokines TNF-α and intercellular adhesion molecule 1 (ICAM-1) expression levels (Sun et al., 2006). In summary, rats have been used as a model for K. pneumoniae pneumonia mainly to evaluate the efficacy of antibiotic treatment and experimental vaccines. One advantage of rats is the possibility of inducing lobar pneumonia, as observed in many human patients, especially in community-acquired respiratory infections. Also, the larger size compared to mice facilitates direct access to the lower respiratory tract through surgery or cannulation, allowing the direct delivery of the inoculum. Larger size and increased weight may also be more suitable for evaluating adaptive immune responses in studies using survival as an endpoint, since rats tend to survive longer periods than mice after challenge. Nevertheless, rats require more space and increased maintenance costs when compared to mice and have fewer advocated methodologies for their study in K. pneumoniae infections.
Other animal models
Besides mice and rat models, pulmonary K. pneumoniae infection was also induced in cynomolgus macaques. In that study, 108 and 1010 CFU of a carbapenem-resistant ST258 strain, were inoculated in 8–10 years old female primates with aid of a bronchoscope, delivering the bacterial load directly into the lungs. In both infection groups, the K. pneumoniae load was able to induce pneumonia, which was then used to test the potential protective effect of K. pneumoniae capsular polysaccharide (CPS) against respiratory infection. The CPS formulation reduced the bacterial burden in the lungs, inducing antibodies that promoted opsonophagocytic killing by polymorphonuclear leukocytes (PMN) in vitro. The advantage in the use of primates as a study subject is the closer similarities to humans when compared to rodents, sharing anatomical and immune features, while also maintaining the susceptibility to infection, which is considerably higher in mice (Malachowa et al., 2019). However, the manipulation and maintenance of the animals are more laborious and demanding when compared to small rodents. Only a few specialized animal facilities are equipped to house this type of animal.
An empyema model was established using male and female white New Zealand rabbits. Following the artificial creation of pneumothorax and pleural effusion, 109 CFU of a K. pneumoniae clinical isolate in 1 mL, was given through a 16-gage cannula. This model was used to analyze the effects of gentamicin and oxygen administration in empyema, which showed that higher O2 levels can improve the infection prognosis (Shohet et al., 1987).
Another study used male, white New Zealand rabbits to investigate biofilm formation in vivo. The model consisted in the introduction of an endotracheal tube previously colonized with different biofilm-producing strains of K. pneumoniae or P. aeruginosa in the trachea of the rabbits, with a hyperthermia device. The goal was to evaluate the biofilm formation in a fever state, and with temporary 42°C pulses, biofilm formation was greatly, though not completely inhibited (Palau et al., 2023).
Rabbit models of respiratory infections can be an interesting approach, depending on the desired outcomes. In an FDA-backed study to validate new animal models, Pseudomonas aeruginosa was used to induce pneumonia and it was found to reproduce many of the typical hallmarks found in humans, such as tissue damage and inflammation, changes in gasometry values, blood pressure, and white-blood cell counts. Eventual infection metastasis was also observed, in some cases leading to death (Nguyen et al., 2021; Gras et al., 2023). However, there is still very limited research using rabbits as a model for K. pneumoniae infection, and further studies are needed to accurately determine how robust the model is in terms of reproducibility, evaluation of adaptive immune responses during infection, duration of colonization and survival.
In summary, different animal models have been used to successfully reproduce the hallmarks of Klebsiella pneumoniae respiratory infections, with mice being the most used species. However, most of the infection protocols still rely on artificial inoculation routes, involving tracheal incisions or catheters to deliver the bacteria. While these may replicate some of the infections occurring in hospital settings, they do not mimic the natural infection routes in the community.
When considering mouse models in respiratory infections, the direct intranasal inoculation of bacteria appears to be the most advantageous technique to study community acquired infections, since it can: (i) provide data on the different stages of disease pathogenesis as well as the contribution of specific virulence factors; including acquisition, transition to the lungs, establishment and dissemination; (ii) evaluate specific components of the adaptive immune response; (iii) provide reproducible results in survival/colonization without the need for highly specialized training and equipment; (iv) adequately assess the protective efficacy of therapeutic agents or vaccine candidates.
Animal models of Klebsiella pneumoniae oral/gastrointestinal infections
Gastrointestinal infections are highly prevalent diseases worldwide, where the most frequent causative agents in these infections are Gram-negative bacteria that reside in the human intestine (Joseph et al., 2021), especially Enterobacteriaceae, including K. pneumoniae (Osbelt et al., 2021). Its prevalence in hospitalized patients ranges from 3 to 18% (Gorrie et al., 2017; Joseph et al., 2021), while colonization in healthy individuals varies from approximately 6% in Europe to 20% in Africa (WHO, 2017).
Analysis of rectal and throat swabs from patients admitted in intensive care units found that 6% of these patients were colonized with K. pneumoniae. Gut colonization on admission was significantly associated with subsequent infections, with 49% of K. pneumoniae infections being caused by a strain found in the patients’ microbiota (Gorrie et al., 2017), and possibly transmitted through the fecal-oral route (Young et al., 2020). Furthermore, antibiotic treatment reduces microbial diversity in the GI tract, causing dysbiosis which favors subsequent colonization with K. pneumoniae (Martin and Bachman, 2018; Chen et al., 2022). K. pneumoniae dysbiosis in the GI correlated with the establishment of systemic infections, such as pneumonia (Wu et al., 2020; Jiang et al., 2022) and liver abscess (Zheng et al., 2021), and it also contributes to inflammatory bowel diseases (Khorsand et al., 2022).
Colonization of GI tract by K. pneumoniae is a necessary step for infection (Buffie and Pamer, 2013; Gorrie et al., 2017). Therefore, developing models that mimic this route of colonization and infection is of great importance for understanding disease pathogenesis and for the development of effective therapeutic/preventive strategies. Most animal studies investigating K. pneumoniae GI colonization used mice, since they are small, easy to breed and maintain, and reproduce infection hallmarks observed in humans (Ferreira et al., 2018; Muggeo et al., 2018; Cassini et al., 2019; Young et al., 2020; Joseph et al., 2021), as discussed in the next section and compiled in Table 2.
Mouse infection models
The best described methods of inoculation are through gavaging (intragastric administration) or direct oral administration. When the bacterial suspension is given orally, pretreatment with a large-spectrum antibiotic (or a cocktail) is usually performed in the days or weeks preceding challenge to deplete the mouse gut microbiota (Maroncle et al., 2006; Lau et al., 2008; Hennequin and Forestier, 2009). The described volumes of the bacterial suspension vary between 100 and 200 μL. BALB/c (Chiang et al., 2021), C57BL/6 (Nakamoto et al., 2019; Sequeira et al., 2020; Liu J. Y. et al., 2022), C57BL6/J (Young et al., 2020), C57BL/6 N (WT and Rag2 −/−) and C57BL/6NTac (Osbelt et al., 2021), MRL/MpJ (Kamata et al., 2020) and CFW1 (Boll et al., 2012) mouse strains have been used in colonization experiments.
Young and colleagues have described a mouse model of GI infection where the animals received the bacteria orally, diluted in a 2% sucrose solution, delivered through a pipette tip, without previous antibiotic treatment. The authors also induced the infection via gavage, with a 20-gage feeding needle (Young et al., 2020). Administration of at least 105 CFU of K. pneumoniae was enough to colonize the GI tract and oropharynx, regardless of the infection route. This model can be applied as an alternative to the standard infection protocols where the administration of antibiotics prior the colonization leads to a disruption on the native microbiota allowing K. pneumoniae to stablish colonization (Young et al., 2020). It could also be used to study community acquired K. pneumoniae infections.
The use of a gavage needle allows direct instillation in the lower GI tract; however, to neutralize the stomachal acidity, a sodium bicarbonate treatment (0.2 M in 200 μL) is performed 5 min prior to the inoculation, resulting in a successful colonization with 100 μL of the bacterial suspension (Calderon-Gonzalez et al., 2023). This model was applied in different mice strains, including C57BL/6 (Calderon-Gonzalez et al., 2023), C57BL/6 J (Lau et al., 2008; Yuan et al., 2019; Young et al., 2020; Kienesberger et al., 2022), OF1 (Maroncle et al., 2006; Hennequin and Forestier, 2009), BALB/c (Hsieh et al., 2010), BALB/cByL (Hsu et al., 2019), CF1 (Perez et al., 2011) and 129 × 1/SvJ (Han et al., 2023).
A work published by Lagrafeuille and colleagues investigated the use of probiotic bacteria as treatment against K. pneumoniae infections. They found that the addition of the cell-free supernatant from Lactobacillus plantarum impaired biofilm formation by K. pneumoniae in vitro. However, when tested in mice, the group infected with both bacteria presented a longer persistence of K. pneumoniae when compared with the control group (infected with K. pneumoniae only) (Lagrafeuille et al., 2018). This result reinforces the importance of having in vivo models to confirm the in vitro findings.
Another technique employed to colonize the gut microbiota of mice is through “contamination” of the drinking water with the intended bacteria. In the works describing the method, the animals received preventive antibiotic treatment (also in the water) with either clindamycin or streptomycin, followed by administration of water containing the bacteria (Favre-Bonté et al., 1999; Le Guern et al., 2019). Aiming to demonstrate the dynamics between GI infection and antibiotic administration, male C57BL/6 mice received 107/mL of a New Delhi metallo-beta-lactamase-1 (NDM-1) positive strain in the water, after antibiotic treatment. The authors propose a protocol for the use of the antimicrobial where, based on the timing of the colonization process, clindamycin treatment a week before the inoculation showed the best results in the colonization model. When clindamycin was given 2 or 3 weeks before or after the inoculation, gut colonization was not as successful (Le Guern et al., 2019).
Perez and colleagues used a similar model to determine the effect of the antibiotic treatment on GI colonization, establishment, and elimination by K. pneumoniae. The animals received antibiotic treatment by subcutaneous injection every day during 8 days; on day 3, they were infected with K. pneumoniae and the occurrence of colonization was monitored through fecal analysis (Perez et al., 2011). The levels of K. pneumoniae in the feces remained high during antibiotic treatment, but fell gradually afterwords until clearance, suggesting that the reduction of the commensal microbiota by antibiotic therapy favors GI colonization by K. pneumoniae (Perez et al., 2011). In humans, although many normal microbiota are not resistant to K. pneumoniae colonization, antibiotic treatment can further contribute to Klebsiella pneumoniae infection, which often occurs at the hospital environment. The inhibition of exogenous colonization can be attributed either to competition among the microbes or the interaction of the microbiota with mucosal immune defenses, intensifying its response against invaders (Buffie and Pamer, 2013).
A study by Osbelt et al. using germ-free mice transplanted with a human microbiota evaluated stool samples from healthy individuals (adults and kids) and applied an in vitro screening to identify the microbiota composition looking for microorganisms present in the healthy donors that could eliminate K. pneumoniae colonization. They identified the commensal bacterium Klebsiella oxytoca as able to strongly reduce colonization by K. pneumoniae (Osbelt et al., 2021). K. oxytoca was shown to cooperate with other commensal bacteria to displace K. pneumoniae from the GI tract, which could potentially be applied as a probiotic treatment to prevent K. pneumoniae infections during hospitalization.
Rat infection models
In female Wistar rats, the GI tract colonization was obtained with an intragastric tubing, inserted surgically through the esophagus, following chloral hydrate anesthesia, with an inoculum of 1 mL containing a mixture of neomycin-resistant Escherichia coli and K. pneumoniae. In the co-colonization model, the infection lasted longer in animals that received the neomycin treatment when compared to the group who received the bacteria and the irrigation fluid only, without the antimicrobial agent (Ruijs and van der Waaij, 1986). A different instillation approach of the bacteria is via gavage, where 2 × 109 CFU of bacteria diluted in 1 mL was administered to male Sprague–Dawley rats, following treatment with an antibiotic cocktail. Both the bacterial load and the antimicrobials were administered through gavage. K. pneumoniae was recovered from stool samples up to 16 days after challenge. The study described how the use of different types of antimicrobials can influence in the transmission of resistance genes via plasmids in the rat GI tract (Ye et al., 2019).
Very few studies have focused on animal models to investigate oral mucosal infections by K. pneumoniae. A screening of K. pneumoniae strains displaying the HMV phenotype was conducted in African green monkeys, rhesus and cynomolgus macaques, tested via polymerase chain reaction (PCR) of oropharyngeal and rectal swabs. Most of the K. pneumoniae-positive cultures were negative for the HMV phenotype, while 19 of the 307 animals tested positive for HMV-positive strains. The work demonstrated that K. pneumoniae is able to colonize the oral microbiota in non-human primates, most likely through the fecal-oral route (Burke et al., 2009).
Another study investigated the protective potential of Dentavax, a formulation based on inactivated K. pneumoniae, Streptococcus pyogenes, Staphylococcus aureus, Candida albicans, and Lactobacillus acidophilus strains. Chinchillas were immunized and challenged with 200 μL (5 × 109 CFU/mL) of bacterial suspensions containing all the bacterial species in the formulation, delivered with injections made with a tuberculin syringe, in six different points of the oral cavity. The immunized animals showed a faster recovery, with fewer inflammatory histological signs. In the peripheral blood of the immunized animals, increased phagocytic activity of polymorphonuclear leukocytes were observed, while the sera showed immunoglobulin G (IgG) production against the bacteria included in the vaccine. Also, specific secretory IgA (S-IgA) production was detected in the feces (Marinova et al., 2000).
As mentioned for respiratory infection models, rodents are the preferred animals to evaluate K. pneumoniae GI infections. Several inoculation techniques have been employed to successfully establish colonization. However, an important issue regarding GI models is the presence of a natural microbiota that greatly impacts the infection outcomes. An alternative to surpass this limitation is the use of animals with a humanized microbiota, that allows the evaluation of bacterial interactions during infection, mimicking the conditions found in the human host. Another important aspect of GI infections is the effect of previous antibiotic treatment on dysbiosis, that leads to K. pneumoniae infections in humans; this can also be evaluated using mice with a humanized microbiota, providing important insights for future antibiotic treatment.
In summary, considering the differences between the mouse GI microbiome—which is naturally resistant to K. pneumoniae colonization—and the human microbiome, a more permissive environment for K. pneumoniae, an ideal model would employ animals transplanted with human bacteria. The oral route of administration is preferable because it better mimics the natural acquisition of the pathogen and allows the investigation of host defense mechanisms activated during the bacterial transition from the oral cavity to the lower GI tract. However, when considering specific infections such as liver abscess, a more artificial route, such as intraperitoneal injection may be used (Wu et al., 2022). Though, depending on the invasiveness of the strain, oral inoculation can also lead to infection in other sites as liver and spleen (Hsieh et al., 2012).
Animal models of Klebsiella pneumoniae urinary tract infections
Urinary tract infections (UTI) are among the most common infections in the community and in hospital settings. An UTI can progress to pyelonephritis, kidney damage and sepsis. Bacteria are the main causative agents of UTI, including Gram-positive and Gram-negative pathogens, with the most prevalent being Escherichia coli and in second position, Klebsiella pneumoniae. Both are commonly identified in infections of variable severity (Flores-Mireles et al., 2015). A prediction using statistical models showed that just in the year of 2019, around 65,000 deaths were credited to urinary infections caused by multi-drug resistant bacteria. With another 200,000 deaths indirectly related to such infections (Li et al., 2022). Another reason of concern is the increasing rates of antimicrobial resistance in UTIs, which limit the therapeutic choices and worsen the disease burden, reinforcing the urgent need for new therapies and prophylactic strategies (Flores-Mireles et al., 2015).
In that sense, animal models are important for studying the host-pathogen interactions during urinary tract infections, and for testing new antibiotics/vaccines. As described for other mucosal infection models, most animal studies evaluating UTI are performed in mice, which are a better option than rats, as they have a greater number of glycolipid receptors in the urethral tissue, thus promoting better adhesion. Furthermore, the bladders of mice and humans share conserved proteins named uroplakins, which play a role in fimbriae-mediated bacterial adhesion (Murray et al., 2021).
The next section includes the animal models used to study UTIs. These studies are summarized in Table 3.
Mouse infection models
The most described method of bladder inoculation is via a catheter inserted directly through the urethra. Anesthetized animals are placed horizontally and catheterized. After the insertion, with the aid of a syringe, the bacterial suspension or treatment is inoculated into the lower urinary tract (bladder) of female mice (Thai et al., 2010).
Many different mouse strains have been used to study K. pneumoniae urinary infections, including C3H/HeN (Rosen et al., 2008a,b), C3H/HeJ (Rosen et al., 2008c), BALB/c (Gomes et al., 2020) and CBA/J (Saenkham et al., 2020; Mason et al., 2023). Anesthesia is usually performed using methoxyflurane (Rosen et al., 2008a,b,c) or a ketamine and xylazine mixture (Gomes et al., 2020). A study evaluating the association between diabetes and urinary tract infections inoculated C3H/HeN, C3H/HeJ and C57BL/6 mice with different bacterial strains, including a cystitis isolate of Klebsiella pneumoniae TOP52 1721, using a transurethral catheter. After the infection period, the bladder and kidneys were aseptically removed for bacterial counts. The TOP52 1721 strain was able to cause infection in the bladder and kidneys of mice with diabetes at higher titers than in healthy mice, indicating an increased susceptibility to UTIs in that group (Rosen et al., 2008c).
A study characterized the molecular difference of FimH of a uropathogenic E. coli (UPEC) isolate UTI89 and Klebsiella pneumoniae cystitis isolate TOP52. Strains used for the urinary tract infection model were UTI89 cystitis isolate UPEC; UTI89 ΔfimH (mutant strain lacking the adhesin FimH); TOP52 1721, a K. pneumoniae cystitis isolate; and TOP52 ΔfimK (mutant strain lacking the fimbriae regulator FimK). The infection was induced by inoculating 50 μL of a 1 to 2×107 CFU suspension the strains via the urethra, into 8-week-old female C3H/HeN mice, and to perform the quantification of bacteria present in the animals’ tissues, the bladder and kidneys were collected aseptically after 6 h, 1 and 14 days, and plated. The bacterial titers in the bladder were higher at all timepoints for E. coli UTI89 strain (which had fimbriae) when compared to UTI89 ΔfimH (FimH mutant), which was also seen between the wild-type K. pneumoniae TOP52 strain, in comparison to the FimH-negative mutant. The bladder CFU counts of the UPEC group was higher than the K. pneumoniae group. However, 14 days post-infection, the recovered CFU was similar. Colonization of the kidneys was initially higher in the UPEC group but became similar to K. pneumoniae in later data points. Wild type and mutant K. pneumoniae strains showed equal titers in all measured times. The study demonstrated that for K. pneumoniae, FimH did not have a critical role in the initial steps of bladder infection, however it became required in later stages. For E. coli UTI89, FimH appears to play a more prominent role. Overall, the study shows the role that FimH in the bladder colonization and invasion, infection persistence and development of intracellular bacterial communities (IBCs) in both strains (Rosen et al., 2008b).
Using the same infection model, Rosen et al. evaluated the role of the FimK regulator in the urinary tract infection process. K. pneumoniae lacking fimK showed an increased type I fimbriae expression, which reflected in the increased CFU count in the bladder and kidneys, and IBC formation in the animal group infected with the mutant strain, when compared to the wild type. The authors propose that the downregulated type I fimbriae expression could partially explain how UTIs caused by E. coli infections are more common when compared to K. pneumoniae (Rosen et al., 2008a).
A study by Gomes et al., describing the transcriptional regulator of the kpfR gene cluster demonstrated that the regulator plays an important role in K. pneumoniae pathogenicity in urinary tract of mice. In the study, female BALB/c mice were inoculated via the transurethral route with a K2 clinical strain isolated from a patient diagnosed with UTI or its isogenic KpfR-negative mutant. The mutant, which exhibited a hyper fimbriated phenotype, displayed reduced ability to colonize the mouse bladder and was cleared faster than the wild-type strain. The authors suggest that overexpression of fimbriae in the mutant promotes a more robust immune response that leads to quick bacterial elimination by the host (Gomes et al., 2020).
A similar infection model used a silicone tube attached to a needle, which was inserted in the bladder through the urethra opening of C57BL/6NCr mice under isoflurane anesthesia. In this study, 50 μL of the 107 CFU bacterial suspension were inoculated. Using this model, the role of type I and III K. pneumoniae fimbriae in the colonization of the urinary tract was evaluated in the presence (or absence) of the silicone implant in the bladder, which was used to simulate a urinary catheter. In the presence of the implant, colonization of the bladder was augmented, especially when the insertion had been performed 24 h prior to the inoculation. K. pneumoniae strains lacking the type I and type III fimbriae demonstrated a reduced ability to colonize the bladder in both the catheterized and un-catheterized groups at the 6- and 48-h endpoints. Finally, the number of bacteria recovered from the implants was lower in the group inoculated with the mutant lacking type III fimbriae, suggesting a bigger impact of this fimbriae in the colonization of the abiotic surface (Murphy et al., 2013).
The murine model of urinary tract infection was also used for assessing the impact of hyperglycosuria on bacterial colonization by Klebsiella pneumoniae. The excess glucose excretion was induced using dapagliflozin, a drug for controlling diabetes. The K. pneumoniae strain used was KPPR1 and the experiments were conducted on adult (4–6 weeks) female CBA/J mice. The infection was induced by inoculation of 108 CFU of the KPPR1 strain into the bladder via transurethral route. A syringe pump connected to a polyethylene tube, with a constant, low flow, was used to prevent leakage of the inoculum due to urinary reflux. Urine was collected at 6, 24, 48 h, or 7 days, and plated for bacterial count; the bladder, spleen and liver were removed aseptically and plated as well. A higher bacterial load was evident in both urine and bladder of the mice with hyperglycosuria. This group also exhibited a greater systemic spread as other organs, such as spleen and liver showed a higher bacterial load, indicating that high glucose levels promote UTI by K. pneumoniae (Saenkham et al., 2020).
In conclusion, mice have been extensively used as models of K. pneumoniae UTI, including local bladder infection and ascending pyelonephritis. In that regard, the increased vesical-ureteric reflux presented by the C3H/HeJ strain—a retrograde urine flow resulting from a congenital anomaly of the urinary tract (Murawski et al., 2010)—makes it an ideal model to investigate more complicated, ascending infections, like those observed in human patients with the same condition.
An important aspect that remains to be explored is the gender-related differences in UTI by K. pneumoniae. There are protocols available to induce infection in male mice using transurethral instillation (Zychlinsky Scharff et al., 2017) or surgically through an abdominal incision followed by direct bladder inoculation using a needle (Olson et al., 2016). Studies with E. coli have demonstrated that male mice develop more severe, chronic infections that are influenced by androgen exposure (Olson et al., 2016). Therefore, UTI studies using male mice are necessary to evaluate gender-related differences in K. pneumoniae infections.
Rat infection model
A few studies have used rats to investigate UTI by Klebsiella pneumoniae. In female Sprague–Dawley rats anesthetized with pentobarbital, bladder colonization was achieved with a catheter, in which, 50 μL of a 5 × 109 bacterial suspension incorporated in agar beads were inoculated to the animals. Using this urinary infection model, the effectiveness of a treatment with Lactobacillus casei prior to the infection was evaluated. L. casei was also incorporated into beads and while it is not able to colonize the kidneys of the animals, the colonization of the urinary tract with L. casei prevented the installment of UTI and pyelonephritis in the animals who received the lactobacilli-coated beads (Reid et al., 1985). A similar method was used in Wistar (CFHB) rats, in which following anesthesia the abdominal area was massaged to expel the urine. Then, a urinary cannula was used to introduce the inoculum, in a final volume of 1 mL. When the inoculation step was finished, a clamp was used in the urethral meatus to prevent bacterial leakage and taken out after 10 min (Camprubí et al., 1993; Regué et al., 2004). This protocol was used to evaluate the role of the O-antigen and CPS in the UTI development. Strains lacking the O-antigen showed a substantial decrease in the ability to colonize the rat’s kidneys and bladder, whereas, in the infection model tested, the K-antigen did not appear to bear the same level of importance (Camprubí et al., 1993).
Other UTI animal models
An ex vivo porcine model was employed to induce a catheter-associated UTI. The model is based on a modified Foley catheter, which was introduced in the urethral tract of a euthanized female pig. With a silicone tube, the catheter was placed, then inflated to stay in place throughout the experiment. The catheter contained pre-formed 24 h bacterial biofilms, including a carbapenem-resistant K. pneumoniae strain. Following the introduction, the apparatus was irrigated with a combination of antibiotic solutions, then segmented for bacterial quantification. The animal’s urethra and bladder were also analyzed (Vargas-Cruz et al., 2019). The model reproduced the parameters of catheter associated infections in human patients, while showing that the antibiotic irrigation was able to reduce bacterial colonization of the urinary tract.
As discussed for respiratory and GI infections, mice have been used in most of the studies investigating UTI by K. pneumoniae, with transurethral injection using catheters being the main technique for bacterial inoculation. This inoculation route results in direct delivery of bacteria in the bladder, but it has a few limitations: the size and position of the mouse urethra make it hard to access; urine flow may contribute to bacterial leaking during the procedure, resulting in variations in the number of CFU inoculated. Despite those limitations, local infections in the urinary tract are an important tool to investigate the contribution of K. pneumoniae virulence factors to disease and develop new therapeutics/vaccines to control UTIs.
Transurethral inoculation of K. pneumoniae may also be employed to evaluate ascending UTIs—an important feature in urinary tract infections in humans. It is also a simpler technique that requires some level of expertise, but the animal manipulation does not demand specific equipment or local surgery, which includes the recovery process as a potential issue. Therefore, it should be considered as the gold standard technique for UTI studies. Conversely, given the prevalence of catheter-induced UTIs in hospital dwellings, models that mimic this type of device should be considered when evaluating nosocomial infections.
Non-mammal disease models in Klebsiella pneumoniae
Very few models were described for K. pneumoniae infections using animals other than rodents. A recent work by Zhang and colleagues have used zebrafish (Danio rerio) to investigate the variations in infection by different K. pneumoniae strains, and the innate immune responses mounted against these bacteria. The fish were infected by immersion in a bacterial suspension for 8 or 24 h, followed by organ collection and bacterial counting. The intestines were the organs with the highest bacterial loads (Zhang X. et al., 2019).
The zebrafish model is also suitable to analyze neutrophil and macrophage migration during K. pneumoniae infection, since they harbor a mammalian-like innate immune system; however, for evaluation of adaptive responses, a mammalian model is required (Zhang X. et al., 2019). Another limitation of this model is the lack of control in the bacterial loads infecting each animal since they are immersed in the suspension. In the same work, the authors used a Galleria mellonella infection model. This is a convenient model to determine the virulence of a bacterial strain or even to compare two or more strains’ behavior during infection, which was the case in the cited study. The authors were able to screen among different strains and select the one with the highest potential of causing infection after colonization, which was achieved via injections with 1×106 CFU, in 10 μL, of a K. pneumoniae suspension, using a Hamilton syringe into the haemocoel of the larvae proleg (Zhang X. et al., 2019). The signs of a successful infection included changes in pigmentation, from the typical clear color to a darker tone, combined with the lack of motility are signs of lethality in the larvae (Harding et al., 2013).
Another in vivo, non-vertebrate model platform, used to evaluate therapies against K. pneumoniae infections is the nematode Caenorhabditis elegans. In a phage therapy assay, K. pneumoniae, E. coli and Enterobacter cloacae strains were used in a liquid-phase infection model, in which, either the prophylactic or therapeutic use of the bacteriophages were able to increase the survival of C. elegans against the pathogens alone or in combination (Manohar et al., 2022). Antimicrobial screening studies were also conducted in C. elegans; using different concentrations of multiple antimicrobials in a lethality assay against carbapenem-resistant K. pneumoniae isolates. The lethality model showed comparable results to the usual Kirby-Bauer disk diffusion resistance test, for most of the drugs. Employing the same survival model, dose-dependent drug toxicity was also analyzed and provided data consistent with preconized protocols. Based on the screening results, a therapy protocol was selected and used in two hospitalized patients (Yao et al., 2022).
Comparative virulence of K. pneumoniae isolates was assessed in a Drosophila melanogaster (fruit fly) model. Multirresistant isolates with matte and mucoid phenotypes were tested in colonization/ lethality assay. The animals (3–5 days old) were injected with increasing bacterial loads using a 10-μm needle. The isolates only killed a small number of flies, however, the CFU counts in the mucoid phenotype group was higher when compared to the non-mucoid strains. It is unclear if the lethality was not achieved due to the type of bacterial strain, or the fly model is not adequate for this type of analysis (Lee et al., 2018).
Overall, animal models of K. pneumoniae employing non-mammalian species are still scarce, and future studies are required to assess the potential applicability of the data to human infections. Nevertheless, the current results demonstrate that these models could contribute to our understanding of K. pneumoniae pathogenesis, more specifically in drug screening or comparative virulence assays.
Limitations and shortcomings of animal models for the study of Klebsiella pneumoniae infections
As described throughout this review, mice studies represent the most used model to investigate K. pneumoniae infections in different mucosal sites. However, this model has important limitations such as a high intrinsic resistance to K. pneumoniae strains, including clinical strains, and differences in K. pneumoniae lethality in different mice lineages (Mizgerd and Skerrett, 2008). Meanwhile, primates appear to be more susceptible to K. pneumoniae infections, which makes sense when considering the high anatomical and immunological similarities between humans and primates. However, they are extremely expensive and require specialized animal facilities, which greatly limits their use. There is also an ethical concern regarding the use of highly sentient beings in experimentation when other options are available. Therefore, improving the rodent models and combining in vitro techniques will provide more applicable results.
A particularity of K. pneumoniae is the differences between the classical and hypervirulent strains. Often, hypervirulent strains are lethal in mouse studies, however, that may not be the intention of the study (colonization studies, for example). There is a lack of well described bacterial strains leading to non-lethal infections. Unlike infection models for better stablished pathogens, such as Streptococcus pneumoniae (Chiavolini et al., 2008), the choice of the bacterial strains ends up being difficult, since there are few correlates of virulence or lethality in mice. Recent studies investigating the genetic diversity of K. pneumoniae clinical isolates (Martin et al., 2023) and correlation of certain virulence factors with disease potential in mice (Russo et al., 2021) provide valuable insights in the pathogenesis of K. pneumoniae. However, the data are from systemic infections resulting from subcutaneous or intraperitoneal challenges; it remains to be investigated whether the same is true for mucosal infections. Even when looking at survival as an endpoint, the current animal models may not represent the natural stages of disease development seen in patients infected with K. pneumoniae, which limit the extrapolation of the results to clinical scenarios.
As an option to replace the use of animals in the basic research of UTI treatments, an in vitro model was developed, using real urine, simulating the physiological conditions of the urinary tract with a Foley catheter passing through the cap of a conical tube, surrounded by tryptone soy agar. The bacterial growth was evaluated by analyzing the bacterial counts in the catheter (Gaonkar et al., 2003).
Techniques for animal replacement involve the use of artificial environments that mimic human niches, as in 3D cell culture or microfluidic systems, exemplified by lab- or organ-on-a-chip technologies. The tridimensional cell culture models resemble natural tissue structures, resulting in functional organoids maintained in culture conditions, allowing an increase in the development speed in the pre-clinical stages of research. Though the technique is in constant evolution, there are still limitations and questions about its reproducibility and biological significance (Shah et al., 2023). Similarly, the microfluid chip models are also a promising alternative, being able to reproduce physiological events such as the physical forces and cell organization (Feaugas and Sauvonnet, 2021). However, there are also drawbacks in its application: the systems are still in validation process, without well-established standard procedures, and the complexity of the human systems make it almost impossible to replicate identically (Leung et al., 2022). Therefore, although animal replacement techniques have important limitations, especially considering systemic studies (like for instance, in vaccine development), there is definitely a huge potential for development of novel therapies.
Another option is the substitution of the typical vertebrate models by invertebrates, such as C. elegans, D. melanogaster, or Galleria mellonella. Just like any in vivo model, there are advantages and disadvantages. Invertebrates are cheaper, often easy to manipulate and are less complex when compared to vertebrate mammals. Nonetheless, there are also limitations in the application of those models. In the G. mellonella case in particular, the larvae has an innate immune system somewhat similar to ours, but it lacks an adaptative response (Ménard et al., 2021). In K. pneumoniae infection models, G. mellonella has been used with vastly different goals, such as to evaluate phage-mediated survival (Feng et al., 2023), synergistic activity of antimicrobials (Ribeiro et al., 2023) and pathogenicity (Liu et al., 2023). However, when used to compare hypervirulent and common K. pneumoniae strains, the model did not reproduce the same differences observed in outbred mice (Russo and MacDonald, 2020).
Similarly, C. elegans has been used as a platform to study K. pneumoniae infection, antimicrobial drugs activity (Yao et al., 2022) and bacteriophage therapy efficacy (Manohar et al., 2022). Survival studies and analysis of K. pneumoniae virulence can also be achieved using D. melanogaster (Lee et al., 2018). There is a description of colonization and bacterial metastasis in zebrafish, using different clinical K. pneumoniae strains isolated from patients’ gut microbiota (Zhang X. et al., 2019). All those studies represent interesting approaches to investigate some traits of K. pneumoniae infections, but they do not allow a detailed analysis of the host immune responses during infection, nor the mechanisms responsible for increased survival after vaccination/treatment. A recent review in vaccine development against K. pneumoniae can be found in Assoni et al. (2021).
Conclusion
Mammals, and specifically, rodents like mice and rats, are the most widely models for studying K. pneumoniae infection. The anatomical similarities and comparable immune responses, combined with the easy handling and availability of a variety of strains make these animals the gold standard in K. pneumoniae research in vivo. However, they present important limitations, instigating the development of more physiological approaches, which mimic the hallmarks of human infections and allow a better understanding of the host-pathogen interactions during disease. Transgenic animals with humanized immune systems, and/or those with humanized microbiota may represent a more physiological platform to study K. pneumoniae infections. Additionally, inoculation procedures that closely emulate the natural pathogenic process may provide a better understanding of the different infection stages.
The choice of the most appropriate animal model must also consider the anatomical and immunological specificities of a particular strain, which greatly impact the outcome of the study. The use of neutropenic mice, for instance, usually provides increased bacterial burden, while anatomical differences in the urinary tract may favor more severe diseases. These traits, as well as the use of animals with specific mutations, could provide reproducible and stable infections, allowing the evaluation of intended outcomes, which could be further correlated with human patients.
Given the ability of K. pneumoniae to colonize different host niches, causing diseases of variable severity, it is important to develop models that replicate the conditions (from the pathogen and the host) associated with bacterial persistence in each host tissue. In that sense, whole transcriptome and proteome profiling may help identify the virulence factors involved in different stages of disease and how these factors interact to promote bacterial persistence in different sites within the host. Furthermore, the use microbial communities (co-infection) may better represent the natural environment in which K. pneumoniae colonizes the host, since different microbes are known to cooperate or compete for the same host niche. Finally, since K. pneumoniae is known to form biofilms in vitro and in vivo, the use of biofilm bacteria instead of planktonic may help unveil disease mechanisms which are unique to this bacterial phenotype. This has already been demonstrated during colonization of the bladder and may also be important in other tissues such as the lungs.
Author contributions
LA: Writing – review & editing, Writing – original draft. AC: Writing – original draft. BV: Writing – original draft. BM: Writing – original draft. AL: Writing – original draft. TC: Writing – review & editing, Supervision, Conceptualization. MD: Writing – review & editing, Writing – original draft, Supervision, Funding acquisition, Conceptualization.
Funding
The author(s) declare that financial support was received for the research, authorship, and/or publication of this article. This work was supported by Fundação de Amparo à Pesquisa do Estado de São Paulo (FAPESP) (grant 2023/10579-8) and Coordenação de Aperfeiçoamento de Pessoal de Nível Superior (CAPES) (scholarship grant: 88887.601281/2021-00).
Acknowledgments
We thank Casa Nossa Senhora da Paz for the financial support with the publication fee.
Conflict of interest
The authors declare that the research was conducted in the absence of any commercial or financial relationships that could be construed as a potential conflict of interest.
Publisher’s note
All claims expressed in this article are solely those of the authors and do not necessarily represent those of their affiliated organizations, or those of the publisher, the editors and the reviewers. Any product that may be evaluated in this article, or claim that may be made by its manufacturer, is not guaranteed or endorsed by the publisher.
References
Aljohani, F. S., Rezki, N., Aouad, M. R., Hagar, M., Bakr, B. A., Shaaban, M. M., et al. (2022). Novel 1,2,3-Triazole-sulphadiazine-ZnO hybrids as potent antimicrobial agents against Carbapenem resistant Bacteria. Antibiotics (Basel) 11:916. doi: 10.3390/antibiotics11070916
Antimicrobial Resistance Collaborators (2022). Global burden of bacterial antimicrobial resistance in 2019: a systematic analysis. Lancet 399, 629–655. doi: 10.1016/S0140-6736(21)02724-0
Assoni, L., Girardello, R., Converso, T. R., and Darrieux, M. (2021). Current stage in the development of Klebsiella pneumoniae vaccines. Infect. Dis. Ther. 10, 2157–2175. doi: 10.1007/s40121-021-00533-4
Bachman, M. A., Breen, P., Deornellas, V., Mu, Q., Zhao, L., Wu, W., et al. (2015). Genome-wide identification of Klebsiella pneumoniae fitness genes during lung infection. MBio 6:e00775. doi: 10.1128/mBio.00775-15
Bakker-Woudenberg, I. A., ten Kate, M. T., Guo, L., Working, P., and Mouton, J. W. (2001). Improved efficacy of ciprofloxacin administered in polyethylene glycol-coated liposomes for treatment of Klebsiella pneumoniae pneumonia in rats. Antimicrob. Agents Chemother. 45, 1487–1492. doi: 10.1128/AAC.45.5.1487-1492.2001
Barletta, K. E., Cagnina, R. E., Burdick, M. D., Linden, J., and Mehrad, B. (2012). Adenosine a(2B) receptor deficiency promotes host defenses against gram-negative bacterial pneumonia. Am. J. Respir. Crit. Care Med. 186, 1044–1050. doi: 10.1164/rccm.201204-0622OC
Boll, E. J., Nielsen, L. N., Krogfelt, K. A., and Struve, C. (2012). Novel screening assay for in vivo selection of Klebsiella pneumoniae genes promoting gastrointestinal colonisation. BMC Microbiol. 12:201. doi: 10.1186/1471-2180-12-201
Branger, J., Knapp, S., Weijer, S., Leemans, J. C., Pater, J. M., Speelman, P., et al. (2004). Role of toll-like receptor 4 in gram-positive and gram-negative pneumonia in mice. Infect. Immun. 72, 788–794. doi: 10.1128/IAI.72.2.788-794.2004
Buffie, C. G., and Pamer, E. G. (2013). Microbiota-mediated colonization resistance against intestinal pathogens. Nat. Rev. Immunol. 13, 790–801. doi: 10.1038/nri3535
Burke, R. L., Whitehouse, C. A., Taylor, J. K., and Selby, E. B. (2009). Epidemiology of invasive Klebsiella pneumoniae with hypermucoviscosity phenotype in a research colony of nonhuman primates. Comp. Med. 59, 589–597.
Calderon-Gonzalez, R., Lee, A., Lopez-Campos, G., Hancock, S. J., Sa-Pessoa, J., Dumigan, A., et al. (2023). Modelling the gastrointestinal carriage of Klebsiella pneumoniae infections. MBio 14:e0312122. doi: 10.1128/mbio.03121-22
Camprubí, S., Merino, S., Benedí, V. J., and Tomás, J. M. The role of the O-antigen lipopolysaccharide and capsule on an experimental Klebsiella pneumoniae infection of the rat urinary tract. FEMS Microbiol. Lett. (1993);111(1):9–13, doi: 10.1016/0378-1097(93)90175-2
Cassini, A., Högberg, L. D., Plachouras, D., Quattrocchi, A., Hoxha, A., Simonsen, G. S., et al. (2019). Attributable deaths and disability-adjusted life-years caused by infections with antibiotic-resistant bacteria in the EU and the European economic area in 2015: a population-level modelling analysis. Lancet Infect. Dis. 19, 56–66. doi: 10.1016/S1473-3099(18)30605-4
Chang, D., Sharma, L., Dela Cruz, C. S., and Zhang, D. (2021). Clinical epidemiology, risk factors, and control strategies of Klebsiella pneumoniae infection. Front. Microbiol. 12:750662. doi: 10.3389/fmicb.2021.750662
Chen, I. R., Lin, S. N., Wu, X. N., Chou, S. H., Wang, F. D., and Lin, Y. T. (2022). Clinical and microbiological characteristics of Bacteremic pneumonia caused by Klebsiella pneumoniae. Front. Cell. Infect. Microbiol. 12:903682. doi: 10.3389/fcimb.2022.903682
Chhibber, S., Aggarwal, S., and Yadav, V. (2003). Contribution of capsular and lipopolysaccharide antigens to the pathogenesis of Klebsiella pneumoniae respiratory tract infection. Folia Microbiol. (Praha) 48, 699–702. doi: 10.1007/BF02993481
Chhibber, S., Wadhwa, S., and Yadav, V. (2004). Protective role of liposome incorporated lipopolysaccharide antigen of Klebsiella pneumoniae in a rat model of lobar pneumonia. Jpn. J. Infect. Dis. 57, 150–155.
Chiang, M. K., Hsiao, P. Y., Liu, Y. Y., Tang, H. L., Chiou, C. S., Lu, M. C., et al. (2021). Two ST11 Klebsiella pneumoniae strains exacerbate colorectal tumorigenesis in a colitis-associated mouse model. Gut Microbes 13:1980348. doi: 10.1080/19490976.2021.1980348
Chiavolini, D., Pozzi, G., and Ricci, S. (2008). Animal models of Streptococcus pneumoniae disease. Clin. Microbiol. Rev. 21, 666–685. doi: 10.1128/CMR.00012-08
Choby, J. E., Howard-Anderson, J., and Weiss, D. S. (2020). Hypervirulent Klebsiella pneumoniae - clinical and molecular perspectives. J. Intern. Med. 287, 283–300. doi: 10.1111/joim.13007
Conlan, S., Kong, H. H., and Segre, J. A. (2012). Species-level analysis of DNA sequence data from the NIH human microbiome project. PLoS One 7:e47075. doi: 10.1371/journal.pone.0047075
Cortés, G., Alvarez, D., Saus, C., and Albertí, S. (2002). Role of lung epithelial cells in defense against Klebsiella pneumoniae pneumonia. Infect. Immun. 70, 1075–1080. doi: 10.1128/IAI.70.3.1075-1080.2002
Dai, P., and Hu, D. (2022). The making of hypervirulent Klebsiella pneumoniae. J. Clin. Lab. Anal. 36:e24743. doi: 10.1002/jcla.24743
Deng, J. C., Zeng, X., Newstead, M., Moore, T. A., Tsai, W. C., Thannickal, V. J., et al. (2004). STAT4 is a critical mediator of early innate immune responses against pulmonary Klebsiella infection. J. Immunol. 173, 4075–4083. doi: 10.4049/jimmunol.173.6.4075
Dong, G., Xu, N., Wang, M., Zhao, Y., Jiang, F., Bu, H., et al. (2021). Anthocyanin extract from purple sweet potato exacerbate Mitophagy to ameliorate Pyroptosis in Klebsiella pneumoniae infection. Int. J. Mol. Sci. 22:11422. doi: 10.3390/ijms222111422
Fagundes, C. T., Amaral, F. A., Vieira, A. T., Soares, A. C., Pinho, V., Nicoli, J. R., et al. (2012). Transient TLR activation restores inflammatory response and ability to control pulmonary bacterial infection in germfree mice. J. Immunol. 188, 1411–1420. doi: 10.4049/jimmunol.1101682
Favre-Bonté, S., Licht, T. R., Forestier, C., and Krogfelt, K. A. (1999). Klebsiella pneumoniae capsule expression is necessary for colonization of large intestines of streptomycin-treated mice. Infect. Immun. 67, 6152–6156. doi: 10.1128/IAI.67.11.6152-6156.1999
Feaugas, T., and Sauvonnet, N. (2021). Organ-on-chip to investigate host-pathogens interactions. Cell. Microbiol. 23:e13336. doi: 10.1111/cmi.13336
Feng, J., Li, F., Sun, L., Dong, L., Gao, L., Wang, H., et al. (2023). Characterization and genome analysis of phage vB_KpnS_SXFY507 against Klebsiella pneumoniae and efficacy assessment in galleria mellonella larvae. Front. Microbiol. 14:1081715. doi: 10.3389/fmicb.2023.1081715
Ferreira, R. L., da Silva, B. C. M., Rezende, G. S., Nakamura-Silva, R., Pitondo-Silva, A., Campanini, E. B., et al. (2018). High prevalence of multidrug-resistant Klebsiella pneumoniae harboring several virulence and beta-lactamase encoding genes in a Brazilian intensive care unit. Front. Microbiol. 9:3198. doi: 10.3389/fmicb.2018.03198
Flores-Mireles, A. L., Walker, J. N., Caparon, M., and Hultgren, S. J. (2015). Urinary tract infections: epidemiology, mechanisms of infection and treatment options. Nat. Rev. Microbiol. 13, 269–284. doi: 10.1038/nrmicro3432
Gaonkar, T. A., Sampath, L. A., and Modak, S. M. (2003). Evaluation of the antimicrobial efficacy of urinary catheters impregnated with antiseptics in an in vitro urinary tract model. Infect. Control Hosp. Epidemiol. 24, 506–513. doi: 10.1086/502241
Geller, B. L., Li, L., Martinez, F., Sully, E., Sturge, C. R., Daly, S. M., et al. (2018). Morpholino oligomers tested in vitro, in biofilm and in vivo against multidrug-resistant Klebsiella pneumoniae. J. Antimicrob. Chemother. 73, 1611–1619. doi: 10.1093/jac/dky058
Gomes, A. É. I., Pacheco, T., Dos Santos, C. D. S., Pereira, J. A., Ribeiro, M. L., Darrieux, M., et al. (2020). Functional insights from KpfR, a new transcriptional regulator of Fimbrial expression that is crucial for Klebsiella pneumoniae pathogenicity. Front. Microbiol. 11:601921. doi: 10.3389/fmicb.2020.601921
Gorrie, C. L., Mirceta, M., Wick, R. R., Edwards, D. J., Thomson, N. R., Strugnell, R. A., et al. (2017). Gastrointestinal carriage is a major reservoir of Klebsiella pneumoniae infection in intensive care patients. Clin. Infect. Dis. 65, 208–215. doi: 10.1093/cid/cix270
Gou, S., Li, B., Ouyang, X., Ba, Z., Zhong, C., Zhang, T., et al. (2021). Novel broad-Spectrum antimicrobial peptide derived from Anoplin and its activity on bacterial pneumonia in mice. J. Med. Chem. 64, 11247–11266. doi: 10.1021/acs.jmedchem.1c00614
Gras, E., Vu, T. T. T., Nguyen, N. T. Q., Tran, V. G., Mao, Y., Tran, N. D., et al. (2023). Development and validation of a rabbit model of Pseudomonas aeruginosa non-ventilated pneumonia for preclinical drug development. Front. Cell. Infect. Microbiol. 13:1297281. doi: 10.3389/fcimb.2023.1297281
Griepentrog, J. E., Zhang, X., Lewis, A. J., Gianfrate, G., Labiner, H. E., Zou, B., et al. (2020). Frontline science: rev-Erbα links blue light with enhanced bacterial clearance and improved survival in murine Klebsiella pneumoniae pneumonia. J. Leukoc. Biol. 107, 11–25. doi: 10.1002/JLB.4HI0519-155R
Gu, X., Gao, R., Li, Y., Liu, J., Wu, Y., and Xu, H. (2022). Combination effect of azithromycin with TCM preparation Xiyanping injection against Klebsiella pneumoniae infection in rats. Phytomedicine 104:154332. doi: 10.1016/j.phymed.2022.154332
Gu, H., Zeng, X., Peng, L., Xiang, C., Zhou, Y., Zhang, X., et al. (2021). Vaccination induces rapid protection against bacterial pneumonia via training alveolar macrophage in mice. eLife :e69951:10. doi: 10.7554/eLife.69951
Han, B., Zhang, X., Wang, L., and Yuan, W. (2023). Dysbiosis of gut microbiota contributes to uremic cardiomyopathy via induction of IFNγ-producing CD4(+) T cells expansion. Microbiol. Spectr. 11:e0310122. doi: 10.1128/spectrum.03101-22
Harding, C. R., Schroeder, G. N., Collins, J. W., and Frankel, G. (2013). Use of Galleria mellonella as a model organism to study Legionella pneumophila infection. J. Vis. Exp. 81:e50964. doi: 10.3791/50964
Hennequin, C., and Forestier, C. (2009). oxyR, a LysR-type regulator involved in Klebsiella pneumoniae mucosal and abiotic colonization. Infect. Immun. 77, 5449–5457. doi: 10.1128/IAI.00837-09
Holden, V. I., Breen, P., Houle, S., Dozois, C. M., and Bachman, M. A. (2016). Klebsiella pneumoniae Siderophores induce inflammation, bacterial dissemination, and HIF-1α stabilization during pneumonia. MBio 7:e01397-16. doi: 10.1128/mBio.01397-16
Hoover, J. L., Lewandowski, T. F., Mininger, C. L., Singley, C. M., Sucoloski, S., and Rittenhouse, S. (2017). A robust pneumonia model in immunocompetent rodents to evaluate antibacterial efficacy against S. pneumoniae, H. influenzae, K. pneumoniae, P. aeruginosa or A. baumannii. J. Vis. Exp. 119:55068. doi: 10.3791/55068
Hsieh, P. F., Lin, H. H., Lin, T. L., and Wang, J. T. (2010). CadC regulates cad and tdc operons in response to gastrointestinal stresses and enhances intestinal colonization of Klebsiella pneumoniae. J. Infect. Dis. 202, 52–64. doi: 10.1086/653079
Hsieh, P. F., Lin, T. L., Yang, F. L., Wu, M. C., Pan, Y. J., Wu, S. H., et al. (2012). Lipopolysaccharide O1 antigen contributes to the virulence in Klebsiella pneumoniae causing pyogenic liver abscess. PLoS One 7:e33155. doi: 10.1371/journal.pone.0033155
Hsu, C. R., Chang, I. W., Hsieh, P. F., Lin, T. L., Liu, P. Y., Huang, C. H., et al. (2019). A novel role for the Klebsiella pneumoniae sap (sensitivity to antimicrobial peptides) transporter in intestinal cell interactions, innate immune responses, liver abscess, and virulence. J. Infect. Dis. 219, 1294–1306. doi: 10.1093/infdis/jiy615
Hu, X., Cai, Y., Wang, Y., Wang, R., Wang, J., and Zhang, B. (2020). Imaging of bioluminescent Klebsiella pneumoniae induced pulmonary infection in an immunosuppressed mouse model. J. Int. Med. Res. 48:300060520956473. doi: 10.1177/0300060520956473
Jain, R. R., Mehta, M. R., Bannalikar, A. R., and Menon, M. D. (2015). Alginate microparticles loaded with lipopolysaccharide subunit antigen for mucosal vaccination against Klebsiella pneumoniae. Biologicals 43, 195–201. doi: 10.1016/j.biologicals.2015.02.001
Jiang, Q., Xu, Q., Kenéz, Á., Chen, S., and Yang, G. (2022). Klebsiella pneumoniae infection is associated with alterations in the gut microbiome and lung metabolome. Microbiol. Res. 263:127139. doi: 10.1016/j.micres.2022.127139
Joseph, L., Merciecca, T., Forestier, C., Balestrino, D., and Miquel, S. (2021). From Klebsiella pneumoniae colonization to dissemination: An overview of studies implementing murine models. Microorganisms 9:1282. doi: 10.3390/microorganisms9061282
Kamata, K., Watanabe, T., Minaga, K., Hara, A., Sekai, I., Otsuka, Y., et al. (2020). Gut microbiome alterations in type 1 autoimmune pancreatitis after induction of remission by prednisolone. Clin. Exp. Immunol. 202, 308–320. doi: 10.1111/cei.13509
Khorsand, B., Asadzadeh Aghdaei, H., Nazemalhosseini-Mojarad, E., Nadalian, B., Nadalian, B., and Houri, H. (2022). Overrepresentation of Enterobacteriaceae and Escherichia coli is the major gut microbiome signature in Crohn's disease and ulcerative colitis; a comprehensive metagenomic analysis of IBDMDB datasets. Front. Cell. Infect. Microbiol. 12:1015890. doi: 10.3389/fcimb.2022.1015890
Kienesberger, S., Cosic, A., Kitsera, M., Raffl, S., Hiesinger, M., Leitner, E., et al. (2022). Enterotoxin tilimycin from gut-resident Klebsiella promotes mutational evolution and antibiotic resistance in mice. Nat. Microbiol. 7, 1834–1848. doi: 10.1038/s41564-022-01260-3
Kumar, A., Harjai, K., and Chhibber, S. (2020). Early cytokine response to lethal challenge of Klebsiella pneumoniae averted the prognosis of pneumonia in FyuA immunized mice. Microb. Pathog. 144:104161. doi: 10.1016/j.micpath.2020.104161
Lagrafeuille, R., Miquel, S., Balestrino, D., Vareille-Delarbre, M., Chain, F., Langella, P., et al. (2018). Opposing effect of Lactobacillus on in vitro Klebsiella pneumoniae in biofilm and in an in vivo intestinal colonisation model. Benef. Microbes 9, 87–100. doi: 10.3920/BM2017.0002
Lau, H. Y., Huffnagle, G. B., and Moore, T. A. (2008). Host and microbiota factors that control Klebsiella pneumoniae mucosal colonization in mice. Microbes Infect. 10, 1283–1290. doi: 10.1016/j.micinf.2008.07.040
Lavender, H., Jagnow, J. J., and Clegg, S. (2005). Klebsiella pneumoniae type 3 fimbria-mediated immunity to infection in the murine model of respiratory disease. Int. J. Med. Microbiol. 295, 153–159. doi: 10.1016/j.ijmm.2005.04.001
Le Guern, R., Grandjean, T., Bauduin, M., Figeac, M., Millot, G., Loquet, A., et al. (2019). Impact of the timing of antibiotic administration on digestive colonization with Carbapenemase-producing Enterobacteriaceae in a murine model. Antimicrob. Agents Chemother. 63:e00360-19. doi: 10.1128/AAC.00360-19
Lee, H., Baek, J. Y., Kim, S. Y., Jo, H., Kang, K., Ko, J. H., et al. (2018). Comparison of virulence between matt and mucoid colonies of Klebsiella pneumoniae coproducing NDM-1 and OXA-232 isolated from a single patient. J. Microbiol. 56, 665–672. doi: 10.1007/s12275-018-8130-3
Leung, C. M., de Haan, P., Ronaldson-Bouchard, K., Kim, G.-A., Ko, J., Rho, H. S., et al. (2022). A guide to the organ-on-a-chip. Nat. Rev. Methods Prim. 2:33. doi: 10.1038/s43586-022-00118-6
Li, X., Fan, H., Zi, H., Hu, H., Li, B., Huang, J., et al. (2022). Global and regional burden of bacterial antimicrobial resistance in urinary tract infections in 2019. J. Clin. Med. 11:2817. doi: 10.3390/jcm11102817
Li-Juan, L., Kang, S., Zhi-Juan, L., Dan, L., Feng, X., Peng, Y., et al. (2022). Klebsiella pneumoniae infection following H9N2 influenza a virus infection contributes to the development of pneumonia in mice. Vet. Microbiol. 264:109303. doi: 10.1016/j.vetmic.2021.109303
Lin, Y. T., Jeng, Y. Y., Chen, T. L., and Fung, C. P. (2010). Bacteremic community-acquired pneumonia due to Klebsiella pneumoniae: clinical and microbiological characteristics in Taiwan, 2001-2008. BMC Infect. Dis. 10:307. doi: 10.1186/1471-2334-10-307
Liu, J., Ding, H., Zhao, M., Tu, F., He, T., Zhang, L., et al. (2022). Functionalized erythrocyte membrane-coated nanoparticles for the treatment of Klebsiella pneumoniae-induced Sepsis. Front. Microbiol. 13:901979. doi: 10.3389/fmicb.2022.901979
Liu, J. Y., Lin, T. L., Chiu, C. Y., Hsieh, P. F., Lin, Y. T., Lai, L. Y., et al. (2022). Decolonization of carbapenem-resistant Klebsiella pneumoniae from the intestinal microbiota of model mice by phages targeting two surface structures. Front. Microbiol. 13:877074. doi: 10.3389/fmicb.2022.877074
Liu, P., Yang, A., Tang, B., Wang, Z., Jian, Z., Liu, Y., et al. (2023). Molecular epidemiology and clinical characteristics of the type VI secretion system in Klebsiella pneumoniae causing abscesses. Front. Microbiol. 14:1181701. doi: 10.3389/fmicb.2023.1181701
Mabrook, M., Abd El-Aziz, A. M., Ali, Y. M., and Hassan, R. (2022). Inhibition of CL-11 reduces pulmonary inflammation in a mouse model of Klebsiella pneumoniae lung infection. Microb. Pathog. 164:105408. doi: 10.1016/j.micpath.2022.105408
Malachowa, N., Kobayashi, S. D., Porter, A. R., Freedman, B., Hanley, P. W., Lovaglio, J., et al. (2019). Vaccine protection against multidrug-resistant Klebsiella pneumoniae in a nonhuman primate model of severe lower respiratory tract infection. MBio 10:e02994-19. doi: 10.1128/mBio.02994-19
Manohar, P., Loh, B., Elangovan, N., Loganathan, A., Nachimuthu, R., and Leptihn, S. (2022). A multiwell-plate Caenorhabditis elegans assay for assessing the therapeutic potential of bacteriophages against clinical pathogens. Microbiol. Spectr. 10:e0139321. doi: 10.1128/spectrum.01393-21
Marinova, S., Tchorbadjiiska, L., Petrunov, B., Cvetanov, J., Nenkov, P., Konstantinova, D., et al. (2000). Immunostimulating and protective effects of an oral polybacterial immunomodulator 'Dentavax' in a rabbit experimental model. Int. J. Immunopharmacol. 22, 843–854. doi: 10.1016/S0192-0561(00)00044-8
Maroncle, N., Rich, C., and Forestier, C. (2006). The role of Klebsiella pneumoniae urease in intestinal colonization and resistance to gastrointestinal stress. Res. Microbiol. 157, 184–193. doi: 10.1016/j.resmic.2005.06.006
Martin, R. M., and Bachman, M. A. (2018). Colonization, infection, and the accessory genome of Klebsiella pneumoniae. Front. Cell. Infect. Microbiol. 8:4. doi: 10.3389/fcimb.2018.00004
Martin, M. J., Stribling, W., Ong, A. C., Maybank, R., Kwak, Y. I., Rosado-Mendez, J. A., et al. (2023). A panel of diverse Klebsiella pneumoniae clinical isolates for research and development. Microb. Genom. 9:mgen000967. doi: 10.1099/mgen.0.000967
Mason, S., Vornhagen, J., Smith, S. N., Mike, L. A., Mobley, H. L. T., and Bachman, M. A. (2023). The Klebsiella pneumoniae ter operon enhances stress tolerance. Infect. Immun. 91:e0055922. doi: 10.1128/iai.00559-22
Mei, X., Wang, H. X., Li, J. S., Liu, X. H., Lu, X. F., Li, Y., et al. (2017). Dusuqing granules (DSQ) suppress inflammation in Klebsiella pneumonia rat via NF-κB/MAPK signaling. BMC Complement. Altern. Med. 17:216. doi: 10.1186/s12906-017-1736-x
Meijer, M. T., de Vos, A. F., Scicluna, B. P., Roelofs, J. J., Abou Fayçal, C., Orend, G., et al. (2021). Tenascin-C deficiency is associated with reduced bacterial outgrowth during Klebsiella pneumoniae-evoked Pneumosepsis in mice. Front. Immunol. 12:600979. doi: 10.3389/fimmu.2021.600979
Ménard, G., Rouillon, A., Cattoir, V., and Donnio, P. Y. (2021). Galleria mellonella as a suitable model of bacterial infection: past, present and future. Front. Cell. Infect. Microbiol. 11:782733. doi: 10.3389/fcimb.2021.782733
Mizgerd, J. P., and Skerrett, S. J. (2008). Animal models of human pneumonia. Am. J. Physiol. Lung Cell. Mol. Physiol. 294, L387–L398. doi: 10.1152/ajplung.00330.2007
Morinaga, Y., Take, Y., Sasaki, D., Ota, K., Kaku, N., Uno, N., et al. (2019). Exploring the microbiota of upper respiratory tract during the development of pneumonia in a mouse model. PLoS One 14:e0222589. doi: 10.1371/journal.pone.0222589
Muggeo, A., Guillard, T., Klein, F., Reffuveille, F., Francois, C., Babosan, A., et al. (2018). Spread of Klebsiella pneumoniae ST395 non-susceptible to carbapenems and resistant to fluoroquinolones in north-eastern France. J. Glob. Antimicrob. Resist. 13, 98–103. doi: 10.1016/j.jgar.2017.10.023
Murawski, I. J., Maina, R. W., Malo, D., Guay-Woodford, L. M., Gros, P., Fujiwara, M., et al. (2010). The C3H/HeJ inbred mouse is a model of vesico-ureteric reflux with a susceptibility locus on chromosome 12. Kidney Int. 78, 269–278. doi: 10.1038/ki.2010.110
Murphy, C. N., Mortensen, M. S., Krogfelt, K. A., and Clegg, S. (2013). Role of Klebsiella pneumoniae type 1 and type 3 fimbriae in colonizing silicone tubes implanted into the bladders of mice as a model of catheter-associated urinary tract infections. Infect. Immun. 81, 3009–3017. doi: 10.1128/IAI.00348-13
Murray, B. O., Flores, C., Williams, C., Flusberg, D. A., Marr, E. E., Kwiatkowska, K. M., et al. (2021). Recurrent urinary tract infection: a mystery in search of better model systems. Front. Cell. Infect. Microbiol. 11:691210. doi: 10.3389/fcimb.2021.691210
Nakamoto, N., Sasaki, N., Aoki, R., Miyamoto, K., Suda, W., Teratani, T., et al. (2019). Gut pathobionts underlie intestinal barrier dysfunction and liver T helper 17 cell immune response in primary sclerosing cholangitis. Nat. Microbiol. 4, 492–503. doi: 10.1038/s41564-018-0333-1
Nguyen, N. T. Q., Gras, E., Tran, N. D., Nguyen, N. N. Y., Lam, H. T. H., Weiss, W. J., et al. (2021). Pseudomonas aeruginosa Ventilator-Associated Pneumonia Rabbit Model for Preclinical Drug Development. Antimicrob. Agents Chemother. 65:e0272420. doi: 10.1128/AAC.02724-20
Nikouee, A., Kim, M., Ding, X., Sun, Y., and Zang, Q. S. (2021). Beclin-1-dependent autophagy improves outcomes of pneumonia-induced Sepsis. Front. Cell. Infect. Microbiol. 11:706637. doi: 10.3389/fcimb.2021.706637
Olonisakin, T. F., Li, H., Xiong, Z., Kochman, E. J., Yu, M., Qu, Y., et al. (2016). CD36 provides host protection against Klebsiella pneumoniae intrapulmonary infection by enhancing lipopolysaccharide responsiveness and macrophage phagocytosis. J. Infect. Dis. 214, 1865–1875. doi: 10.1093/infdis/jiw451
Olson, P. D., Hruska, K. A., and Hunstad, D. A. (2016). Androgens enhance male urinary tract infection severity in a new model. J. Am. Soc. Nephrol. 27, 1625–1634. doi: 10.1681/ASN.2015030327
Osbelt, L., Wende, M., Almasi, E., Derksen, E., Muthukumarasamy, U., Lesker, T. R., et al. (2021). Klebsiella oxytoca causes colonization resistance against multidrug-resistant K. pneumoniae in the gut via cooperative carbohydrate competition. Cell Host Microbe 29, 1663–1679.e7. doi: 10.1016/j.chom.2021.09.003
Otto, N. A., Pereverzeva, L., Leopold, V., Ramirez-Moral, I., Roelofs, J., van Heijst, J. W. J., et al. (2021). Hypoxia-inducible factor-1α in macrophages, but not in neutrophils, is important for host defense during Klebsiella pneumoniae-induced Pneumosepsis. Mediat. Inflamm. 2021, 1–12. doi: 10.1155/2021/9958281
Paczosa, M. K., and Mecsas, J. (2016). Klebsiella pneumoniae: going on the offense with a strong defense. Microbiol. Mol. Biol. Rev. 80, 629–661. doi: 10.1128/MMBR.00078-15
Palau, M., Muñoz, E., Larrosa, N., Gomis, X., Márquez, E., Len, O., et al. (2023). Hyperthermia prevents in vitro and in vivo biofilm formation on endotracheal tubes. Microbiol. Spectr. 11:e0280722. doi: 10.1128/spectrum.02807-22
Perez, F., Pultz, M. J., Endimiani, A., Bonomo, R. A., and Donskey, C. J. (2011). Effect of antibiotic treatment on establishment and elimination of intestinal colonization by KPC-producing Klebsiella pneumoniae in mice. Antimicrob. Agents Chemother. 55, 2585–2589. doi: 10.1128/AAC.00891-10
Pulingam, T., Parumasivam, T., Gazzali, A. M., Sulaiman, A. M., Chee, J. Y., Lakshmanan, M., et al. (2022). Antimicrobial resistance: prevalence, economic burden, mechanisms of resistance and strategies to overcome. Eur. J. Pharm. Sci. 170:106103. doi: 10.1016/j.ejps.2021.106103
Regué, M., Hita, B., Piqué, N., Izquierdo, L., Merino, S., Fresno, S., et al. (2004). A gene, uge, is essential for Klebsiella pneumoniae virulence. Infect. Immun. 72, 54–61. doi: 10.1128/IAI.72.1.54-61.2004
Reid, G., Chan, R. C., Bruce, A. W., and Costerton, J. W. (1985). Prevention of urinary tract infection in rats with an indigenous Lactobacillus casei strain. Infect. Immun. 49, 320–324. doi: 10.1128/iai.49.2.320-324.1985
Ribeiro, A., Chikhani, Y., Valiatti, T. B., Valêncio, A., Kurihara, M. N. L., Santos, F. F., et al. (2023). In vitro and in vivo synergism of Fosfomycin in combination with Meropenem or Polymyxin B against KPC-2-producing Klebsiella pneumoniae clinical isolates. Antibiotics (Basel) 12:237. doi: 10.3390/antibiotics12020237
Rosen, D. A., Hilliard, J. K., Tiemann, K. M., Todd, E. M., Morley, S. C., and Hunstad, D. A. (2015). Klebsiella pneumoniae FimK Promotes Virulence in Murine Pneumonia. J. Infect. Dis. 213, 649–658. doi: 10.1093/infdis/jiv440
Rosen, D. A., Hung, C. S., Kline, K. A., and Hultgren, S. J. (2008c). Streptozocin-induced diabetic mouse model of urinary tract infection. Infect. Immun. 76, 4290–4298. doi: 10.1128/IAI.00255-08
Rosen, D. A., Pinkner, J. S., Jones, J. M., Walker, J. N., Clegg, S., and Hultgren, S. J. (2008a). Utilization of an intracellular bacterial community pathway in Klebsiella pneumoniae urinary tract infection and the effects of FimK on type 1 pilus expression. Infect. Immun. 76, 3337–3345. doi: 10.1128/IAI.00090-08
Rosen, D. A., Pinkner, J. S., Walker, J. N., Elam, J. S., Jones, J. M., and Hultgren, S. J. (2008b). Molecular variations in Klebsiella pneumoniae and Escherichia coli FimH affect function and pathogenesis in the urinary tract. Infect. Immun. 76, 3346–3356. doi: 10.1128/IAI.00340-08
Rouse, M. S., Hein, M. M., Anguita-Alonso, P., Steckelberg, J. M., and Patel, R. (2006). Ceftobiprole medocaril (BAL5788) treatment of experimental Haemophilus influenzae, Enterobacter cloacae, and Klebsiella pneumoniae murine pneumonia. Diagn. Microbiol. Infect. Dis. 55, 333–336. doi: 10.1016/j.diagmicrobio.2006.01.029
Ruijs, G. J., and van der Waaij, D. (1986). Experimental whole gut irrigation in the rat. Scand. J. Infect. Dis. 18, 469–475. doi: 10.3109/00365548609032367
Russo, T. A., and MacDonald, U. (2020). The galleria mellonella infection model does not accurately differentiate between Hypervirulent and classical Klebsiella pneumoniae. mSphere 5, 1–7. doi: 10.1128/msphere.00850-19
Russo, T. A., MacDonald, U., Hassan, S., Camanzo, E., LeBreton, F., Corey, B., et al. (2021). An assessment of Siderophore production, Mucoviscosity, and mouse infection models for defining the virulence Spectrum of Hypervirulent Klebsiella pneumoniae. mSphere 6:e00045-21. doi: 10.1128/mSphere.00045-21
Russo, T. A., Olson, R., Fang, C. T., Stoesser, N., Miller, M., MacDonald, U., et al. (2018). Identification of biomarkers for differentiation of Hypervirulent Klebsiella pneumoniae from Classical K. pneumoniae. J. Clin. Microbiol. 56:e00776-18. doi: 10.1128/JCM.00776-18
Russo, T. A., Olson, R., MacDonald, U., Beanan, J., and Davidson, B. A. (2015). Aerobactin, but not yersiniabactin, salmochelin, or enterobactin, enables the growth/survival of hypervirulent (hypermucoviscous) Klebsiella pneumoniae ex vivo and in vivo. Infect. Immun. 83, 3325–3333. doi: 10.1128/IAI.00430-15
Saenkham, P., Jennings-Gee, J., Hanson, B., Kock, N. D., Adams, L. G., and Subashchandrabose, S. (2020). Hyperglucosuria induced by dapagliflozin augments bacterial colonization in the murine urinary tract. Diabetes Obes. Metab. 22, 1548–1555. doi: 10.1111/dom.14064
Schiffelers, R. M., Bakker-Woudenberg, I. A., and Storm, G. (2000). Localization of sterically stabilized liposomes in experimental rat Klebsiella pneumoniae pneumonia: dependence on circulation kinetics and presence of poly(ethylene)glycol coating. Biochim. Biophys. Acta 1468, 253–261. doi: 10.1016/S0005-2736(00)00265-0
Schiffelers, R. M., Storm, G., and Bakker-Woudenberg, I. A. (2001a). Host factors influencing the preferential localization of sterically stabilized liposomes in Klebsiella pneumoniae-infected rat lung tissue. Pharm. Res. 18, 780–787. doi: 10.1023/A:1011080211226
Schiffelers, R. M., Storm, G., ten Kate, M. T., and Bakker-Woudenberg, I. A. (2001b). Therapeutic efficacy of liposome-encapsulated gentamicin in rat Klebsiella pneumoniae pneumonia in relation to impaired host defense and low bacterial susceptibility to gentamicin. Antimicrob. Agents Chemother. 45, 464–470. doi: 10.1128/AAC.45.2.464-470.2001
Sequeira, R. P., McDonald, J. A. K., Marchesi, J. R., and Clarke, T. B. (2020). Commensal Bacteroidetes protect against Klebsiella pneumoniae colonization and transmission through IL-36 signalling. Nat. Microbiol. 5, 304–313. doi: 10.1038/s41564-019-0640-1
Shah, D. D., Raghani, N. R., Chorawala, M. R., Singh, S., and Prajapati, B. G. (2023). Harnessing three-dimensional (3D) cell culture models for pulmonary infections: state of the art and future directions. Naunyn Schmiedeberg's Arch. Pharmacol. 396, 2861–2880. doi: 10.1007/s00210-023-02541-2
Shohet, I., Yellin, A., Meyerovitch, J., and Rubinstein, E. (1987). Pharmacokinetics and therapeutic efficacy of gentamicin in an experimental pleural empyema rabbit model. Antimicrob. Agents Chemother. 31, 982–985. doi: 10.1128/AAC.31.7.982
Southam, D. S., Dolovich, M., O'Byrne, P. M., and Inman, M. D. (2002). Distribution of intranasal instillations in mice: effects of volume, time, body position, and anesthesia. Am. J. Physiol. Lung Cell. Mol. Physiol. 282, L833–L839. doi: 10.1152/ajplung.00173.2001
Spoelstra, E. N., Ince, C., Koeman, A., Emons, V. M., Brouwer, L. A., van Luyn, M. J., et al. (2007). A novel and simple method for endotracheal intubation of mice. Lab. Anim. 41, 128–135. doi: 10.1258/002367707779399400
Sun, Z., Sun, B., Wang, X., Wang, W., and Zhu, L. (2006). Anti-inflammatory effects of inhaled nitric oxide are optimized at lower oxygen concentration in experimental Klebsiella pneumoniae pneumonia. Inflamm. Res. 55, 430–440. doi: 10.1007/s00011-006-6029-7
Thai, K. H., Thathireddy, A., and Hsieh, M. H. (2010). Transurethral induction of mouse urinary tract infection. J. Vis. Exp. 42:2070. doi: 10.3791/2070
Thomas, J. L., Dumouchel, J., Li, J., Magat, J., Balitzer, D., and Bigby, T. D. (2014). Endotracheal intubation in mice via direct laryngoscopy using an otoscope. J. Vis. Exp. 86:50269. doi: 10.3791/50269
van der Weide, H., Cossío, U., Gracia, R., Te Welscher, Y. M., Ten Kate, M. T., van der Meijden, A., et al. (2020a). Therapeutic efficacy of novel antimicrobial peptide AA139-nanomedicines in a multidrug-resistant Klebsiella pneumoniae pneumonia-septicemia model in rats. Antimicrob. Agents Chemother. 64:e00517-20. doi: 10.1128/AAC.00517-20
Van der Weide, H., Ten Kate, M. T., Vermeulen-de Jongh, D. M. C., Van der Meijden, A., Wijma, R. A., Boers, S. A., et al. (2020b). Successful high-dosage monotherapy of Tigecycline in a multidrug-resistant Klebsiella pneumoniae pneumonia-septicemia model in rats. Antibiotics (Basel). 9:109. doi: 10.3390/antibiotics9030109
Vargas-Cruz, N., Rosenblatt, J., Reitzel, R. A., Chaftari, A. M., Hachem, R., and Raad, I. (2019). Pilot ex vivo and in vitro evaluation of a novel Foley catheter with antimicrobial Periurethral irrigation for prevention of Extraluminal biofilm colonization leading to catheter-associated urinary tract infections (CAUTIs). Biomed. Res. Int. 2019, 1–10. doi: 10.1155/2019/2869039
Vieira, A. T., Rocha, V. M., Tavares, L., Garcia, C. C., Teixeira, M. M., Oliveira, S. C., et al. (2016). Control of Klebsiella pneumoniae pulmonary infection and immunomodulation by oral treatment with the commensal probiotic Bifidobacterium longum 5(1A). Microbes Infect. 18, 180–189. doi: 10.1016/j.micinf.2015.10.008
Wang, Y., Yan, J., Wang, P., and Xu, X. (2022). Gandan Oral liquid improves exudative pneumonia by upregulating Bacteria clearance via regulating AQP5 and MUC5AC in rats. Evid. Based Complement. Alternat. Med. 2022, 1–12. doi: 10.1155/2022/3890347
WHO . Global priority list of antibiotic-resistant bacteria to guide research, discovery, and development of new antibiotics [press release]. (2017).
Wieland, C. W., van Lieshout, M. H., Hoogendijk, A. J., and van der Poll, T. (2011). Host defence during Klebsiella pneumonia relies on haematopoietic-expressed toll-like receptors 4 and 2. Eur. Respir. J. 37, 848–857. doi: 10.1183/09031936.00076510
Wolff, N. S., Jacobs, M. C., Wiersinga, W. J., and Hugenholtz, F. (2021). Pulmonary and intestinal microbiota dynamics during gram-negative pneumonia-derived sepsis. Intensive Care Med. Exp. 9:35. doi: 10.1186/s40635-021-00398-4
Wu, J., Chen, J., Wang, Y., Meng, Q., and Zhao, J. (2022). Siderophore iucA of hypermucoviscous Klebsiella pneumoniae promotes liver damage in mice by inducing oxidative stress. Biochem. Biophys. Rep. 32:101376. doi: 10.1016/j.bbrep.2022.101376
Wu, T., Xu, F., Su, C., Li, H., Lv, N., Liu, Y., et al. (2020). Alterations in the gut microbiome and Cecal metabolome during Klebsiella pneumoniae-induced Pneumosepsis. Front. Immunol. 11:1331. doi: 10.3389/fimmu.2020.01331
Xu, X., Weiss, I. D., Zhang, H. H., Singh, S. P., Wynn, T. A., Wilson, M. S., et al. (2014). Conventional NK cells can produce IL-22 and promote host defense in Klebsiella pneumoniae pneumonia. J. Immunol. 192, 1778–1786. doi: 10.4049/jimmunol.1300039
Yao, H., Xu, A., Liu, J., Wang, F., Yao, H., and Chen, J. (2022). Evaluation of in vivo antibacterial drug efficacy using Caenorhabditis elegans infected with carbapenem-resistant Klebsiella pneumoniae as a model host. Front. Pharmacol. 13:973551. doi: 10.3389/fphar.2022.973551
Ye, L., Chan, E. W. C., and Chen, S. (2019). Selective and suppressive effects of antibiotics on donor and recipient bacterial strains in gut microbiota determine transmission efficiency of blaNDM-1-bearing plasmids. J. Antimicrob. Chemother. 74, 1867–1875. doi: 10.1093/jac/dkz137
Yoshida, K., Matsumoto, T., Tateda, K., Uchida, K., Tsujimoto, S., and Yamaguchi, K. (2001). Induction of interleukin-10 and down-regulation of cytokine production by Klebsiella pneumoniae capsule in mice with pulmonary infection. J. Med. Microbiol. 50, 456–461. doi: 10.1099/0022-1317-50-5-456
Young, T. M., Bray, A. S., Nagpal, R. K., Caudell, D. L., Yadav, H., and Zafar, M. A. (2020). Animal model to study Klebsiella pneumoniae gastrointestinal colonization and host-to-host transmission. Infect. Immun. 88:e00071-20. doi: 10.1128/IAI.00071-20
Yuan, J., Chen, C., Cui, J., Lu, J., Yan, C., Wei, X., et al. (2019). Fatty liver disease caused by high-alcohol-producing Klebsiella pneumoniae. Cell Metab. 30, 675–88.e7. doi: 10.1016/j.cmet.2019.08.018
Zeng, X., Moore, T. A., Newstead, M. W., Deng, J. C., Kunkel, S. L., Luster, A. D., et al. (2005). Interferon-inducible protein 10, but not monokine induced by gamma interferon, promotes protective type 1 immunity in murine Klebsiella pneumoniae pneumonia. Infect. Immun. 73, 8226–8236. doi: 10.1128/IAI.73.12.8226-8236.2005
Zeng, X., Moore, T. A., Newstead, M. W., Hernandez-Alcoceba, R., Tsai, W. C., and Standiford, T. J. (2003). Intrapulmonary expression of macrophage inflammatory protein 1alpha (CCL3) induces neutrophil and NK cell accumulation and stimulates innate immunity in murine bacterial pneumonia. Infect. Immun. 71, 1306–1315. doi: 10.1128/IAI.71.3.1306-1315.2003
Zhang, B. Z., Hu, D., Dou, Y., Xiong, L., Wang, X., Hu, J., et al. (2021). Identification and evaluation of recombinant outer membrane proteins as vaccine candidates against Klebsiella pneumoniae. Front. Immunol. 12:730116. doi: 10.3389/fimmu.2021.730116
Zhang, G., Jiang, C., Xie, N., Xu, Y., Liu, L., and Liu, N. (2019). Treatment with andrographolide sulfonate provides additional benefits to imipenem in a mouse model of Klebsiella pneumoniae pneumonia. Biomed. Pharmacother. 117:109065. doi: 10.1016/j.biopha.2019.109065
Zhang, X., Zhao, Y., Wu, Q., Lin, J., Fang, R., Bi, W., et al. (2019). Zebrafish and galleria mellonella: models to identify the subsequent infection and evaluate the immunological differences in different Klebsiella pneumoniae intestinal colonization strains. Front. Microbiol. 10:2750. doi: 10.3389/fmicb.2019.02750
Zhao, Y., Olonisakin, T. F., Xiong, Z., Hulver, M., Sayeed, S., Yu, M. T., et al. (2015). Thrombospondin-1 restrains neutrophil granule serine protease function and regulates the innate immune response during Klebsiella pneumoniae infection. Mucosal Immunol. 8, 896–905. doi: 10.1038/mi.2014.120
Zheng, Y., Ding, Y., Xu, M., Chen, H., Zhang, H., Liu, Y., et al. (2021). Gut microbiota contributes to host defense against Klebsiella pneumoniae-induced liver abscess. J. Inflamm. Res. 14, 5215–5225. doi: 10.2147/JIR.S334581
Zheng, X., Guo, J., Cao, C., Qin, T., Zhao, Y., Song, X., et al. (2022). Time-course transcriptome analysis of lungs from mice infected with Hypervirulent Klebsiella pneumoniae via aerosolized Intratracheal inoculation. Front. Cell. Infect. Microbiol. 12:833080. doi: 10.3389/fcimb.2022.833080
Keywords: Klebsiella pneumoniae, animal models, disease pathogenesis, mucosal infection, pre-clinical
Citation: Assoni L, Couto AJM, Vieira B, Milani B, Lima AS, Converso TR and Darrieux M (2024) Animal models of Klebsiella pneumoniae mucosal infections. Front. Microbiol. 15:1367422. doi: 10.3389/fmicb.2024.1367422
Edited by:
Daniel Pletzer, University of Otago, New ZealandReviewed by:
Ricardo Calderón González, Queen's University Belfast, United KingdomJohid Malik, University of Nebraska Medical Center, United States
Carmelo Biondo, University of Messina, Italy
Antonio DiGiandomenico, AstraZeneca, United Kingdom
Copyright © 2024 Assoni, Couto, Vieira, Milani, Lima, Converso and Darrieux. This is an open-access article distributed under the terms of the Creative Commons Attribution License (CC BY). The use, distribution or reproduction in other forums is permitted, provided the original author(s) and the copyright owner(s) are credited and that the original publication in this journal is cited, in accordance with accepted academic practice. No use, distribution or reproduction is permitted which does not comply with these terms.
*Correspondence: Michelle Darrieux, c2FtcGFpb21pY2hlbGxlQHVvbC5jb20uYnI=