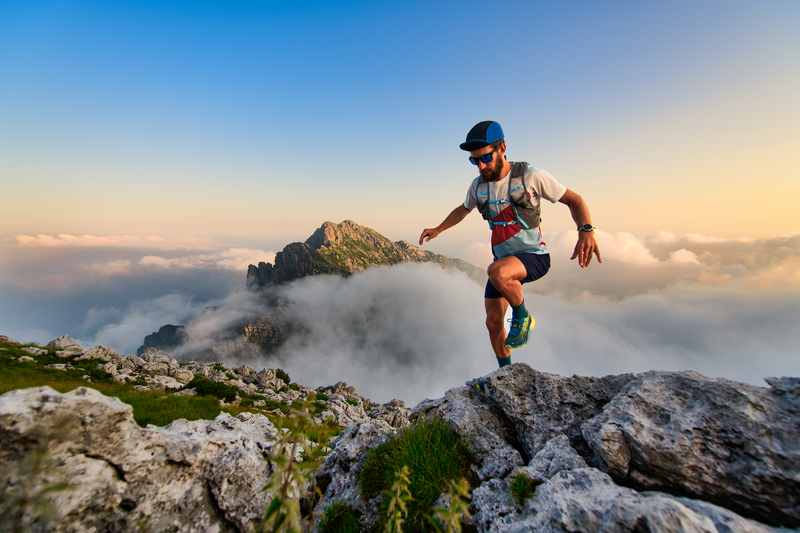
95% of researchers rate our articles as excellent or good
Learn more about the work of our research integrity team to safeguard the quality of each article we publish.
Find out more
ORIGINAL RESEARCH article
Front. Microbiol. , 01 May 2024
Sec. Food Microbiology
Volume 15 - 2024 | https://doi.org/10.3389/fmicb.2024.1367297
This article is part of the Research Topic Microbial Food Safety in Retail Stores and Restaurants View all 8 articles
This research aimed to address the issue of aflatoxin B1 (AFB1) contamination, which posed severe health and economic consequences. This study involved exploring unique species resources in the Qinghai-Tibet Plateau, screening strains capable of degrading AFB1. UPLC-Q-Orbitrap HRMS and NMR were employed to examine the degradation process and identify the structure of the degradation products. Results showed that Bacillus amyloliquefaciens YUAD7, isolated from yak dung in the Qinghai-Tibet Plateau, removed 91.7% of AFB1 from TSB-AFB1 medium with an AFB1 concentration of 10 μg/mL (72 h, 37°C, pH 6.8) and over 85% of AFB1 from real food samples at 10 μg/g (72 h, 37°C), exhibiting strong AFB1 degradation activity. Bacillus amyloliquefaciens YUAD7’s extracellular secretions played a major role in AFB1 degradation mediated and could still degrade AFB1 by 43.16% after boiling for 20 min. Moreover, B. amyloliquefaciens YUAD7 demonstrated the capability to decompose AFB1 through processes such as hydrogenation, enzyme modification, and the elimination of the -CO group, resulting in the formation of smaller non-toxic molecules. Identified products include C12H14O4, C5H12N2O2, C10H14O2, C4H12N2O, with a structure consisting of dimethoxyphenyl and enoic acid, dimethyl-amino and ethyl carbamate, polyunsaturated fatty acid, and aminomethyl. The results indicated that B. amyloliquefaciens YUAD7 could be a potentially valuable strain for industrial-scale biodegradation of AFB1 and providing technical support and new perspectives for research on biodegradation products.
Aflatoxin (AFT) is a highly carcinogenic and teratogenic mycotoxin, primarily produced as a secondary metabolite by Aspergillus flavus and Aspergillus parasiticus fungi (Maxwell et al., 2021). AFT contamination affected 60–80% of food and feed worldwide, particularly products derived from various types of silage, resulting in annual economic losses reaching trillions (Song et al., 2022). Among the 20 types of aflatoxins identified, aflatoxin B1 (AFB1) is the most toxic and has been classified as a Group I human carcinogen by the International Agency for Research on Cancer (IARC; Suman, 2021; Zhang et al., 2024). Furthermore, although there have been some physical and chemical techniques for AFB1 removal, defects, including inefficient detoxification, nutrient preservation, costliness, and toxic by-products, existed before widespread implementation (Yan et al., 2022; Loi et al., 2023). Consequently, searching for safe and effective large-scale AFB1 detoxification strategies has become a focal point of current scientific research.
Microbial degradation is an attractive technology for AFB1 cleanup due to its high specificity efficiency and ecological sustainability without compromising food safety. Several beneficial microorganisms had identified for reducing AFB1 levels in contaminated media according to the researchers, including Pseudomonas spp. (Adebo et al., 2016; Biessy and Filion, 2021), Rhodococcus spp. (Risa et al., 2018; Alvarez et al., 2019), Bacillus spp. (Xia et al., 2017; Xu et al., 2017; Shu et al., 2018; Wang Y. et al., 2018), Escherichia coli (Wang L. et al., 2018), Pleurotus spp. (Sharma et al., 2021), and Aspergillus niger (Yu et al., 2021). While numerous strain capable of degrading AFB1 have been screened, particularly Bacillus strain stood out as attractive candidates due to their high degradation efficiency (Yang et al., 2023). For example, Wang Y. et al. (2018) observed that B. licheniformis BL010 reduced AFB1 levels by 89.1% (120 h, 30°C, AFB1 concentration of 0.5 μg/mL). Shu et al. (2018) found that B. velezensis DY3108 reduced AFB1 levels by 82.0% (within 72 h, 30°C, AFB1 concentration of 5 μg/mL). Despite the outstanding degradation performance exhibited by the screened Bacillus strains at low AFB1 concentrations (not exceeding 5 μg/mL), this is insufficient to meet the requirements for industrially treating high-concentration AFB1 contamination. Industrial AFB1 pollution concentrations can reach as high as 10 μg/g, and there are still no strains capable of effectively removing AFB1 at such elevated concentrations. Previous research indicates that strains screened from extreme environments, particularly the Qinghai-Tibet Plateau, possess stronger temperature resistance, stress tolerance, and unique functionalities than strains selected under other conditions (Bai et al., 2021). The research enriched and domesticated strains from extreme environments aim to address large-scale and high-concentration AFB1 pollution in industrial settings, which provides a new resource for the industrial-scale removal of high-concentration AFB1.
Although biodegradable products are often considered safe, it is essential to assess their safety, as the degraded products may still be hazardous. In recent years, some technological approaches have been used to study the biodegradation products of AFB1. It was determined the composition and chemical formula of AFB1 degradation products by HPLC-Q-TOF-MS in tea-derived Aspergillus niger RAF106 (Fang et al., 2020), and investigated the degradation products of AFB1 by two Bacillus Strains using LC-Triple-TOF-MS (Wang et al., 2022). As mentioned above, mass spectrometry was used to detect the degradation products of AFB1, which could only determine the chemical composition and formula of the products. The structural information of the products could only be inferred based on software calculations by mass spectrometry. Additionally, the interdisciplinary research applied NMR to determine the composition and structures of compounds in complex samples (Zhu et al., 2019; Reif et al., 2021). This study utilizes NMR, which has high sensitivity and resolution, and can generate two-dimensional and multidimensional spectra, making it a powerful technique for determining complex compound structures. It is essential to ensure the safety and effectiveness of biodegradation pathways by identifying the composition and structures of biodegradation products of AFB1. Defining the degradation pathway will contribute to selectively screening for microbial strains that effectively degrade AFB1 while minimizing potential hazards.
The research focused on screening strains capable of efficiently degrading AFB1 from the Qinghai-Tibet Plateau’s extreme environment, which was easily found in ensiled forage and animal manure. We used UPLC-Q-Orbitrap HRMS to determine the chemical composition of the products and applied NMR to elucidate the structure of degradation products, thereby predicting the degradation pathway. This experimental screening of AFB1-degrading strains from the Qinghai-Tibet Plateau enriches the biological resources available for AFB1 degradation and introduced novel insights into the study of the composition, structure, and degradation pathways of these products.
From July to October 2022, samples (Silage corn, Silage alfalfa, and animal feces) were collected from the Qinghai-Tibet Plateau region, including Diebu County, Xiahe County, Gaotai County and Sunan County, Tianzhu Tibetan Autonomous County in Gansu Province. Xining City, Haixi Mongolian and Tibetan Autonomous Prefecture in Qinghai Province. Each 25 g sample was thoroughly mixed with 225 mL of sterile physiological saline solution. Subsequently, a gradient dilution was performed to achieve dilution levels of 10−3~10−7. All dilutions were evenly spread onto a culture medium with coumarin as the sole carbon source (CM medium; L-1distilled water): 10 g coumarin, 0.25 g KH2PO4, 1 g NH4NO3, 1 g CaCl2, 0.25 g MgSO4·7H2O, 1 mg FeSO4, and 15 g agar (Rao et al., 2017). The cultures were then incubated at 37°C for 72 h. Colonies displaying growth on the CM plates were chosen for the degradation and rescreening experiments of AFB1.
The preparation method for TSB-AFB1 liquid medium was as follows: Take 1 mL of AFB1 standard solutions (Sigma-Aldrich, St. Louis, MO, United States) with concentrations of 200, 400, 600, 800, 1,000 μg/mL, and add them to 100 mL of TSB liquid medium, respectively. The final AFB1 concentrations in TSB-AFB1 liquid medium were 2, 4, 6, 8, 10 μg/mL, with the pH adjusted to 6.8. Following a modified method based on Chen et al. (2022), a 10 mL bacterial suspension with a concentration of 108 CFU/mL was inoculated into 100 mL TSB-AFB1 liquid medium (with AFB1 concentrations of 2, 4, 6, 8, 10 μg/mL), and the cultures were shaken culture (37°C, 180 rpm, 72 h). A sterile TSB-AFB1 was used as a control. The second screening of initial strains was based on their ability to degrade AFB1 in TSB-AFB1 medium with a concentration of 10 μg/mL AFB1. Evaluated the degradation ability of the finally selected strains toward varying concentrations of AFB1 using TSB-AFB1 medium with different concentrations (2, 4, 6, 8, 10 μg/mL).
To 5 mL of the test solution, 20.0 mL of acetonitrile-water solution (V:V/84:16) was added. After shaking for 20 min and centrifugation (8,000 rpm, 8 min), 4 mL of the supernatant was taken and subjected to three consecutive extractions with an equal volume of chloroform. The extract was evaporated under nitrogen at 55°C. The precipitate was dissolved in 1 mL of DMSO, filtered through a 0.22 μm organic membrane, and the filtrate was analyzed for AFB1 content using High-Performance Liquid Chromatography (HPLC) system (Waters Acquity, Milford, MA, United States).
HPLC conditions for AFB1 detection: Equipped with a BEH C18 chromatographic column (100 mm × 2.1 mm, 1.7 μm) and a 360 nm ultraviolet detector. Column temperature: 40°C; mobile phase: acetic acid ammonium/methanol; injection volume: 10 μL; flow rate: 0.2 mL/min.
In both cases, the percentage of AFB1 degradation was calculated by following formula, where C was the AFB1 Paek area in treatment, and F was the AFB1 peak area in control:
The maximum value of AFB1 contamination in food was 10 μg/g, we artificially contaminated real food samples to reach this concentration. Experimental samples applied easily contaminated foods like maize, cheese, and peanuts. To prepare these artificially contaminated samples, 10 mL of AFB1 standard solution (1,000 μg/mL) was added separately to 1 kg of maize, cheese, and peanuts. Thorough mixing ensured a final AFB1 concentration of 10 μg/g. Following this, each 1 kg artificially contaminated food sample received a uniform spray of 100 mL bacterial suspension (108 CFU/mL), followed by shading incubation (37°C, 72 h). Treatment groups comprised M-YUAD7, C-YUAD7, and P-YUAD7. Positive controls included food samples artificially contaminated with AFB1 but without the inoculated strain (C-M, C-C, C-P). Negative controls consisted of naturally incubated food samples (N-M, N-C, N-P), and strains were inoculated into food samples without artificial AFB1 contamination (UnM-YUAD7, UnC-YUAD7, UnP-YUAD7). All control groups were also incubated without light (37°C, 72 h). The pretreatment steps for detecting AFB1 content in actual food samples were as follows: 20 g of the sample was mixed with 180 mL distilled water in a juice extractor, blended at high speed for 30 s, and subsequently filtered through four layers of gauze. The obtained pulverized extract will be retained for subsequent AFB1 content detection, following the same procedures as in step 2.2.
For physiological and biochemical identification of the target strain, a conventional microbial biochemical identification kit (Beijing Luqiao, Beijing, China) was employed, following the guidelines of “Bergey’s Manual of Systematic Bacteriology” (Garrity, 1994).
The genomic DNA of YUAD7 was extracted via a SanPrep DNA purification kit (Sangon Biotech, Shanghai, China), following the manufacturer’s guidelines. Subsequently, a combination of PacBio RS II Single Molecule Real Time (SMRT, Pacific Biosciences, MenloPark, CA, United States) and Illumina sequencing platforms (Illumina Novaseq 6000, Shanghai, China) were employed for sequencing. The accession number PRJNA964696 at the US National Center for Biotechnology Information (NCBI) was assigned to the sequence data of B. amyloliquefaciens YUAD7.
The CDSs were predicted with gene annotation performed using GO and KEGG (Fang et al., 2020) using sequence alignment tools such as BLAST,1 Diamond (Version 0.8.35) and HMMER.2 All data were quantified and visualized on the CGView data platform3 and the Chiplot online platform.4
The AFB1 degradation capabilities of cell-free supernatant, intracellular extracts, and dead-cell bacterial suspensions were evaluated using a previously described method (Rao et al., 2017; Cai et al., 2020). The cell-free supernatant, intracellular extracts, and bacterial suspension of dead cells were incubated with TSB-AFB1 medium (AFB1 concentration of 10 μg/mL) at 37°C with 180 rpm shaking for 72 h. Control cultures consisted of TSB medium or sterile phosphate buffer supplemented (PBS) with AFB1(concentration of 10 μg/mL). The residual AFB1 was then analyzed as previously described.
The degradation of AFB1 active components in target strains was fractionated into four components, and the effects of proteinase K (PK, 1 mg/mL), sodium dodecyl sulfate (SDS, 1%), PK + SDS, and heat treatment (boiled for 20 min) on the degradation of AFB1 were investigated. Subsequently, each component was incubated with sterile PBS containing AFB1 at a 10 μg/mL concentration at 37°C. A sterile PBS with AFB1 concentration at 10 μg/mL was used as a control. After 72 h, residual AFB1 was detected, as described earlier.
The survival monitoring assay utilized L-02 standard cells obtained from the Cell Preservation Bank at the Chinese Academy of Sciences in Shanghai, representing a human normal liver cell line. The NC group was cultured in RPMI 1640 medium, while the CC group was cultivated in AFB1-RPMI 1640 solution (AFB1 concentration at 10 μg/mL). The EG group was exposed to RPMI 1640 medium with 10% degradation solution. Then, L-02 cells were seeded at a density of 100,000 cells per well in a 24-well plate and incubated in RPMI 1640 medium at 37°C for 24 h under a 5% CO2 atmosphere to synchronize the population. Subsequently, the medium was replaced with fresh medium containing the test mentioned above samples. The survival monitoring assay was performed using the Vi-cell system (Thermo Scientific, Waltham, MA), and the cell morphology of each sample was compared with that of its corresponding control.
To assess the mutagenic potential of the degradation products, the Ames test using the Genotoxic Ames kit (Iphase Bio Technology, Suzhou, China) following the manufacturer’s instructions and the protocol outlined by Chen et al. (2022). The degradation products obtained from a 72-h co-culture with the AFB1-degrading bacterium and AFB1 were incubated with Salmonella Typhimurium TA100 or TA102 at 37°C for 48 h (EG). The count of S. typhimurium colonies was documented, and the results were expressed as the number of revertant colony-forming units (CFUs). Positive controls consisted of samples extracted from TSB medium with AFB1(CC), while negative controls included extracts from TSB medium without AFB1(NC).
The degradation liquid was concentrated to afford a crude residue which was suspended in H2O and then extracted with petroleum ether, EtOAc, and n-BuOH to afford fractions.
The petroleum ether fraction (50 g) subjected to silica gel column chromatography (200–300 mesh, Qingdao Haiyang Chemical Factory, Qingdao, China) with DCM and MeOH (1:0~0:1, v/v) gradient elution was distributed as two fractions (Fr1, 15 g, Fr2 6.3 g). Fr1(15 g) was chromatographed on an ODS with MeOH/H2O (0:1~0:1, v/v), and then separated by preparative HPLC (MeOH/H2O, 40:60, V/V; flow rate, 4 mL/min) to yield compounds 1 (tR = 8.7 min, 20.4 mg), 2 (tR = 6.8 min, 14.6 mg).
Fr2(6.3 g) was separated by silica gel column chromatography (cc, 70 × 245 mm) with DCM/MeOH (1:0 ~ 0:1, V/V) to provide two subfractions (Fr2-1, 1.3 g; Fr2-2, 0.8 g). Fr2-1(1.3 g) was further separated by preparative HPLC (MeOH/H2O, 35:65, V/V; flow rate, 4 mL/min) to yield 3 (tR = 7.6 min, 26.3 mg), yield 4 (tR = 5.4 min, 10.5 mg).
The UPLC-Q-Orbitrap included a UPLC system equipped with an autoinjector and quaternary UPLC pump (Waters Acquity, Milford, MA, United States), and quadrupole/electrostatic field orbitrap high-resolution mass spectrometry (Q-Orbitrap HRMS; Thermo Scientific, Waltham, MA). The chromatograph was equipped with a BEH C18 column (100 mm × 2.1 mm, 1.7 μm) and operated at a column temperature of 40°C. Samples were injected and eluted using a mobile solvent containing mobile phase A, which was an aqueous solution containing 0.1% formic acid and 5 mmol/L ammonium formate, and mobile phase B, a methanol solution containing 0.1% formic acid and 5 mmol/L ammonium formate. The mass spectrometry parameters were set similar to that in previous study (Lai et al., 2023).
The 1H and 13C NMR experiments data were obtained by Bruker DPX-400 spectrometer in dimethyl sulfoxide-d6 (DMSO-d6; DPX-400, Bruker, Switzerland). The sample was equipped with a 5 mm NORELL NMR tube and operated at 25°C. The data was analyzed using MestreNova software (ver. 14.2, Mestrelab Research, Escondido, CA). Then, BioTransformer 3.0 software5 was used to predict the AFB1 degradation pathway of YUAD7 based on the structure of degradation products.
The results were presented as mean ± standard deviation (SD). Statistical analysis was performed using SPSS software and involved ANOVA followed by Duncan’s test. Different lowercase letters in the bars of each group indicated significant differences between treatments (p < 0.05).
As shown in Table 1, 23 strains capable of utilizing coumarin were obtained using coumarin as the sole carbon source for enrichment and acclimation. YUAD7 exhibited the highest degradation rate, achieving a 91.7% degradation within 72 h in TSB-AFB1 solution with an AFB1 concentration of 10 μg/mL. Therefore, YUAD7 was chosen as the target strain for further research.
YUAD7’s ability to degrade AFB1 at different concentrations was assessed under consistent incubation time and temperature condition. As depicted in Figure 1A, When the AFB1 concentration was below 6 μg/mL, apart from the initial 24-h, the degradation percentages of AFB1 by YUAD7 were consistently similar across treatments during the 96-h incubation. The degradation rates nearly reached their maximum at 72-h, exceeding 99%. Furthermore, when the AFB1 concentrations were 8 and 10 μg/mL, YUAD7 exhibited time-dependent degradation, achieving a reduction of over 94% by 96-h (AFB1 10 μg/mL). Considering the maximum level in raw cereal grains (Campos-Avelar et al., 2021), 10 μg/mL AFB1 was chosen for the subsequent research.
Figure 1. The strain YUAD7 degrades AFB1 in different substrates. (A) The degradation of different concentrations of AFB1 by strain YUAD7 in TSB-AFB1 medium. (B) YUAD7 degrades AFB1 in real food samples.
YUAD7 was employed to degrade AFB1 in real food samples, as shown in Figure 1B. AFB1 levels in all negative control treatments were below the limit of detection [LOD: 0.003 μg/g (mL)]. In the positive controls of maize, cheese, and peanuts, AFB1 levels were 10.84 ± 0.17, 10.73 ± 0.35, and 9.83 ± 0.26 μg/g, respectively. After inoculating YUAD7 for AFB1 degradation, the levels in maize, cheese, and peanuts were reduced to 1.62 ± 0.08, 1.43 ± 0.06, and 1.24 ± 0.05 μg/g, respectively. When AFB1 contamination in food reached 10 μg/g, YUAD7 effectively removed over 85% of AFB1 from real food samples within 72 h.
Strain YUAD7 morphological, characteristics physiological and biochemical characteristics were shown in Supplementary Figure 1 and Supplementary Table 1. Referencing Bergey’s Manual of Systematic Bacteriology (Garrity, 1994), the strain YUAD7 was preliminarily identified as belonging to the Bacillus.
Whole genome sequencing was performed on strain YUAD7, and the results were shown in Supplementary Table 2 and Figure 2A. The complete genome of YUAD7 was a circular chromosome with a size of 4,028,188 bp and a G + C content of 46.4%. The DNA coding sequences encompassed 3,628,166 bp, constituting approximately 90.07% of the total genome. Following genome assembly and prediction annotation, a total of 4,172 predicted genes were identified, of which 4,054 were CDSs and 118 were RNA genes. Upon manual prediction and annotation, 3,218 and 2,106 predicted genes were annotated in the GO and KEGG databases. Based on the analysis of 16S rRNA and 30 housekeeping genes, including acnA, dacB, licR, mfd, lplJ, lpdA, lepA, secA, budA, atpA, gltB, bamA, rfbF, rpoC, rpoB, leuS, dnaE, thrS, pheT, hsdR, recJ, pbpA, SerA, dinG, mobl, rarD, gltB, xynB, pyc, and lpdA, the closest relative of YUAD7 was Bacillus. amyloliquefaciens strain (Supplementary Figure 2). Consequently, the strain mentioned above was designated as Bacillus. amyloliquefaciens YUAD7.
Figure 2. Genome characterization of the YUAD7 strain. (A) Circular representation of the Bacillus amyloliquefaciens YUAD7 complete genome. From the outmost: 1, Genome structure positive and negative strands; 2, GC Skew; 3, GC content. (B) GO analysis of annotated coding genes in B. amyloliquefaciens YUAD7 genome. From the outmost: 1, GO ID. 2, Background genes. 3, Upregulated and downregulated genes. 4, Enrichment coefficients. (C) KEGG pathway classification of annotated coding genes in B. amyloliquefaciens YUAD7 genome.
YUAD7’s CDSs annotation information in the GO and KEGG databases was shown in Figures 2B,C, respectively. Figure 2B indicated that the annotation information of YUAD7’s encoded genes in the GO database mainly fell into three categories: biological process, cellular component, and molecular function, such as oxidation-dependent protein catabolic process (GO: 0070407), polysaccharide biosynthetic process (GO: 0000271), carbohydrate catabolic process (GO: 0044193), hydroxyisourate hydrolase complex (GO: 0106232), O6-methyl-dGTP hydrolase activity (GO: 0106433), and stearyl deacetylase activity (GO: 0034084). Furthermore, in Figure 2C, KEGG annotation information demonstrated that the functions annotated by gene sequences in the primary metabolic pathways could be divided into four categories: cellular processes, environmental information processing, genetic information processing, and metabolism. These findings provide an understanding of the functional genes and metabolic pathways involved in AFB1 degradation by B. amyloliquefaciens YUAD7 at the genomic level. These predicted metabolic pathways and functional genes were closely associated with the process of AFB1 degradation by the strain.
All the data indicated that B. amyloliquefaciens YUAD7 could serve as a beneficial and safe bacterium to be applied in food and feed processing.
In order to investigate the mechanism of AFB1 removal by B. amyloliquefaciens YUAD7, the study tested the efficiency of cell-free supernatant, intracellular extracts, and dead cells in degrading AFB1. As shown in Figure 3A, after 72-h of cultivation, cell-free supernatant could remove 75.42% ± 3.09% of AFB1 (10 μg/mL), while the removal rates for dead cells and intracellular extracts were 4.36% ± 0.61% and 12.63% ± 2.05%, respectively. The cell-free supernatant of B. amyloliquefaciens YUAD7 was more effective in reducing AFB1 than dead cells and intracellular extracts (p < 0.05). These results suggested that the removal of AFB1 by B. amyloliquefaciens YUAD7 was mainly dependent on degradation, and the cell-free supernatant was the main active component in the AFB1 degradation process. SDS, proteinase K (PK), and PK + SDS effected on the activity of YUAD7’s cell-free supernatant in degrading AFB1 was shown in Figure 3B, AFB1 degradation capacity of the cell-free supernatant rated down to 52.85% ± 2.25%, 13.26% ± 2.88%, and 7.36% ± 0.95%. The result indicated that the AFB1 degradation agents in B. amyloliquefaciens YUAD7 cell-free supernatant included not only enzymes or proteins but also other non-protein components. Additionally, the cell-free supernatant still exhibited AFB1 degradation activity of 43.16 ± 3.54% even after boiling for 20 min (Figure 3B).
Figure 3. Identification of active substances and factors influencing the activity of strain YUAD7 in the degradation of AFB1. (A) AFB1 degradation by diverse cell components of Bacillus amyloliquefaciens YUAD7 during 72-h incubation with 10 μg/mL AFB1 at 37°C. (B) Effects of heat, PK, SDS, and PK + SDS on AFB1 degradation mediated by the cell-free supernatant after co-incubation for 72-h with 10 μg/mL AFB1 at 37°C.
The study examined the impact of AFB1 and its degradation products by B. amyloliquefaciens YUAD7 on the lifespan of L-02 cells. As shown in Figure 4A, there was no statistical difference (p > 0.05) in cell viability between the EG group and the NC group, with L-02 cell survival rates exceeding 92%. However, the CC group exhibited a 62.5% decrease in the average lifespan of L-02 cells. The morphological changes of L-02 cells under different treatment conditions within 72-h of cultivation were shown in Figure 4B. Compared to the NC group, the EG group showed no significant differences in cell morphology (p > 0.05). In contrast, the CC group exhibited cell elongation, lysis phenomena, and a significant reduction in cell viability. These results showed that B. amyloliquefaciens YUAD7 degraded AFB1 into metabolites without toxicity to the L-02 cells. Meanwhile, the Ames test was employed to evaluate the mutagenic potential of AFB1 degradation products facilitated by B. amyloliquefaciens YUAD7. Compared to the control group, a roughly twofold increase in the count of revertant CFUs from S. typhimurium TA100 and TA102 was noted in the AFB1 group (CC). However, there was no statistically significant difference in revertant CFUs between the degradation products (EG) and the control group (NC; Figure 4C), indicating that B. amyloliquefaciens YUAD7 transformed AFB1 into metabolites with diminished mutagenicity.
Figure 4. Toxicological results of YUAD7 degradation products. (A) Changes in the lifespan of L-02 cells caused by different treatment groups. (B) Changes in cell morphology in different treatment groups within 72 h. (C) The Ames mutagenicity assay. NC group: medium without AFB1. EG group: medium with AFB1 degradation liquid of YUAD7. CC group: medium with AFB1.
Chemical components of AFB1 degradation products by strain YUAD7 were analyzed using the UPLC-Q-Orbitrap HRMS method. The characteristic ions of AFB1 standard compounds, which were m/z 213, 128, 115, 77, 69, and 43, respectively (Figure 5A). Comparing the fragment ions of AFB1 and the compounds in degradation solution, compound 1–4 had high homology with the fragment ions of AFB1, and compound 1–4 were the products of the degradation of AFB1 by the B. amyloliquefaciens YUAD7 (Supplementary Figure 3).
Figure 5. The mass spectra and chemical formula of AFB1 standards and the compounds in degradation solution. (A) C17H12O6. (B) C12H14O4. (C) C5H12N2O2. (D) C10H14O2. (E) C4H12N2O.
Compound 1 was isolated as white powder. Its molecular formula was established as C12H14O4 by UPLC-Q-Qrbitrap HRMS (m/z 222.0892), sharing several homologous fragment ions with AFB1, such as 213, 128, 115, 77, 69, 43, etc. (Figure 5B). Its 1H NMR spectrum (Table 2) showed characteristic signals for the hydrogen of ABX coupling system on the benzene ring [δH7.34 (1H, d, J = 8.2 Hz, H-6), 6.75(1H, dd, J = 8.1, 2.3 Hz, H-1), and 6.52(1H, d, J = 2.2 Hz, H-3)]; δH3.80(3H, s, H-7), 2.42(3H, s, H-9),3.86(3H, s, H-12)] were three methoxy hydrogen signals. δH6.14 (1H, q, J = 1.3 Hz, H-10) was the hypomethyl hydrogen signal. Analysis of the 13C NMR data (Table 2) revealed 12 carbon signals, including 6 carbon signals on the benzene ring, three methoxy carbon signals (δC55.67, 20.44, 55.92), and three sp3 hybridized carbon data[δC153.39(C-8), 115.33(C-10), 170.01(C-11)] which indicated that compound 1 had the basic skeleton of dimethoxy benzene. The determination of the -CH linkage position and its sequential arrangement within compound 1 was determined by HSQC spectra (Supplementary Figure 6). The benzene ring structure suggested by HSQC correlations from δH7.34 (1H, d, J = 8.2 Hz, H-6) to δC129.36(C-6), δH6.75(1H, dd, J = 8.1, 2.3 Hz, H-1) to δC107.87(C-1), δH6.52(1H, d, J = 2.2 Hz, H-3) to δC97.85(C-3). The three methyl groups were at C7, C9 and C12 positions, respectively, [δH3.80 (3H, s) to δC55.67(C-7), δH2.42 (3H, s) to δC20.44(C-9), δH3.86 (3H, s) to δC55.92(C-12). Finally, the hydrocarbon formation pertaining to compound 1 was precisely ascribed through the chemical formula, 1H NMR, 13C NMR, HSQC, and the structure of compound 1 was (2-4-dimethoxyphenyl) but-2-enoic acid (Figure 6A).
Figure 6. Structure of the main products of AFB1 degradation by strain YUAD7. (A) C12H14O4. (B) C5H12N2O2. (C) C10H14O2. (D) C4H12N2O.
Compound 2 was isolated as a yellow powder with the molecular formula C5H12N2O2, which was established by UPLC-Q-Orbitrap HRMS (m/z 132.0898), sharing several homologous fragment ions with AFB1 such as 128, 115, 77, 69, 43, etc. (Figure 5C). The 1H NMR data (Table 2) of 2 showed the hydrogen signal of two methoxies [δH 2.38 (3H, s, H-1″, H-1a´´)]. δH 2.89 (2H, t, J = 6.7 Hz, H-2″) and 4.26 (2H, t, J = 6.7 Hz, H-3″) were two hydrogen signals on methylene, and δH 4.88 (2H, s, H-4″) was the nitrile hydrogen signal. 13C NMR spectra (Table 2) showed the presence of 5 carbon signals. Among them, 2 signals belonged to 2 methoxies [δC 45.89 (C-1″) and (C-1a″)]. δC 58.52 (C-2″) and 62.73(C-3″) were the methylene carbon signal. δC157.14 (C-4″) were the aminoester group carbon signal. Based on the above date, as well as HSQC data (Supplementary Figure 9), 2 was speculated 2-(dimethylamino) ethyl carbamate (Figure 6B).
Compound 3 was obtained as a yellow powder and the UPLC-Q-Orbitrap HRMS data of 3 (m/z 166.0993, calcd for C10H14O2) indicated its molecular formula of C10H14O2, sharing several homologous fragment ions with AFB1 such as 128, 115, 77, 69, 43, etc. (Figure 5D). In the 1H NMR data (Table 2) δH 1.67–1.61(3H, m, H-1′, H-3′) were the characteristic hydrogen signals of the methoxy. δH 5.38 (1H, th, J = 6.6, 1.6 Hz, H-4′) and 6.51(1H, tq, J = 5.9,1.4 Hz, H-6′) were two methenyl hydrogen signals. δH 2.74 (2H, dddq, J = 7.1, 6.2, 2.0, 1.0 Hz, H-5′) and 3.00 (2H, dp, J = 5.8,1.0 Hz, H-8′) were two hydrogen signals on methylene. δH 9.83–9.75 (1H, m, H-9′, H-9a′) were two formyl hydrogen signals. The 13C NMR data (Table 2) of 3 showed 10 carbon signals, including 2 methoxy hydrogen signals, 2 methene signals, 2 methenyl signals, 2 formyl signals, and 2 other sp3 hybrid carbon signals. The above HRMS and NMR data (Table 2; Supplementary Figure 12) indicated that 3 was polyunsaturated fatty acid structure (Figure 6C).
Compound 4 was isolated as a yellow powder and its molecular formula was deduced as C4H12N2O on the basis of its UPLC-Q-Orbitrap HRMS data (m/z 104.0949), sharing several homologous fragment ions with AFB1 such as 77, 69, 43 etc. (Figure 5E). The 1H NMR data (Table 2; Supplementary Figure 15) of 4 showed the hydrogen signals of the aminomethyl group [δH 2.88–2.68 (2H, m, H-1‴), 1.43 (2H, t, J = 6.5 Hz, H-2‴)]. δH 1.82–1.51(2H, m, H-3‴, H-4‴) and δH 3.53 (2H, d, J = 11.7, H-5‴) were the hydrogen signals of methylen. δH 5.45 (2H, s, H-5a‴) was the aminogruppe hydrogen signal. The 13C NMR data (Table 2) of 4 showed 4 carbon signals, including 3 carbon signals on methylene signals, 1 aminomethyl group signal. The compound 4 was the 1-aminooxy-4-aminobutane (Figure 6D).
The structure of degradation products by the strain were determined using NMR as shown in Figure 6. The chemical structures of the products were primarily consisted of dimethoxyphenyl and enoic acid (compound 1), dimethylamino and ethyl carbamate (compound 2), polyunsaturated fatty acid (compound 3), and aminomethyl (compound 4). Among these four product structures, there were no structures that were associated with the high toxicity of AFB1, including the furan ring double bond, coumarin lactone ring, and cyclopentenone ring.
Based on the structure of the degradation products, it could be inferred that B. amyloliquefaciens YUAD7 mainly degraded AFB1 through secondary degradation (Figure 7). Primary degradation was achieved through hydrogenation and enzyme modification, directly cleaving the coumarin moiety (at positions 10, 11, and 15) and the cyclopentenone ring (at position 14) structures from the AFB1 parent structure. Simultaneously, the modification disrupted the furan ring structure at positions 8 and 9, resulting in the formation of compound C12H14O4. Moreover, an enzymatic modification added reactions that collided with the [N+H+] ion precursor bound, possibly forming the compound C5H12N2O2. Secondary degradation involved further decomposition of the primary degradation products C12H14O4 and C5H12N2O2, primarily through the removal of the -CO moiety. In this process, the products underwent additional structural adjustments and cleavage, forming simpler compounds such as C10H14O2 and C4H12N2O.
The B. amyloliquefaciens YUAD7 strains, isolated from Tibetan Plateau yak manure in this study, exhibited remarkable degradation capabilities of AFB1. Bacillus amyloliquefaciens YUAD7 could degrade AFB1 at concentrations ranging from 1 to 6 μg/mL by more than 99% and 8 to 10 μg/mL by more than 91% after a 72-h incubation. These results surpassed the 84% reduction of AFB1 concentration at 1–5 μg/mL reported for B. amyloliquefaciens WF2020 (Chen et al., 2022) and were notably higher than the 73 and 40% reductions reported for B. amyloliquefaciens B10 (Xiong et al., 2022) and S8C (Ali et al., 2021), respectively. Moreover, after 96 h of incubation, YUAD7 exhibited an impressive degradation of 94.60% for AFB1 (10 μg/mL). Among the Bacillus Spp. with the highest reported degradation efficiency, including B. amyloliquefaciens B10 (Xiong et al., 2022), B. amyloliquefaciens WF2020 (Chen et al., 2022), B. velezensis AD8 (Zhao et al., 2022), B. albus YUN5 (Kumar et al., 2023), B. subtilis JSW-1 (Xia et al., 2017), and B. licheniformis CFR1 (Rao et al., 2017), none achieved efficiency exceeding 90% in previous studies. The utilization of B. amyloliquefaciens YUAD7 held the promise of significant time and cost reductions, making this process more effective. In terms of ensuring the safety of probiotic microorganisms in food and feed, the FAO/WHO guidelines emphasized that probiotics were the best microorganisms to include in food and feed (Song et al., 2022). Bacillus amyloliquefaciens was recognized as an intestinal probiotic for humans and mammals (Marchese et al., 2018).
Bacillus amyloliquefaciens YUAD7 primarily removed AFB1 through degradation, with extracellular proteins or enzymes as the main active substances. This finding consisted with previous research on AFB1 degradation by Bacillus species, including B. amyloliquefaciens WF2020 (Chen et al., 2022), B. licheniformis CFR1 (Rao et al., 2017), B. velezensis DY3108 (Shu et al., 2018), and B. mojavensis RC3B (Gonzalez et al., 2019). Moreover, the cell-free supernatant of B. amyloliquefaciens YUAD7 could still degrade AFB1 by 43.2% after boiling for 20 min, which was higher than that of B. amyloliquefaciens WF2020 (Chen et al., 2022). Since mesophilic bacteria or enzymes often failed to endure the harsh reaction conditions required in industrial processes, it was highly beneficial that thermostable extracellular proteins or enzymes might provide robust and efficient catalyst substitutes that could withstand the harsh reaction conditions required in industrial processes.
Previous studies have reported the complete genome sequences of other Bacillus strains (Fang et al., 2020; Chen et al., 2022), however, these analyses needed more comprehensive annotation of functional genes and metabolic pathways. The functional bacteria research had indicated that through whole-genome annotation, the Enterobacter roggenkampii ED5 strain predicted metabolic processes and functional genes related to biological control (Guo D. J. et al., 2020). In this study, based on whole-genome prediction, metabolic processes related to AFB1 degradation included cellular processes, environmental information processing, genetic information processing, and metabolism. Functional genes involved were the oxidation-dependent protein catabolic process (GO: 0070407), polysaccharide biosynthetic process (GO: 0000271), and carbohydrate catabolic process (GO: 0044193). The full-genome analysis of B. amyloliquefaciens YUAD7 represented a valuable tool for identifying and categorizing genes involved in the biodegradation of AFB1, enabling a comprehensive insight into the functional genes and metabolic pathways underlying this process.
Meanwhile, the safety of microorganisms in food and feed was ensured through toxicological analysis of YUAD7 degradation products. As the AFB1 toxin caused the most damage to liver cells (Alvarez et al., 2019), normal human hepatocytes L-02 were chosen as the experimental subjects for the study to respond more sensitively to the toxicity of the degradation products on cells. The results were similar to those of studies on B. licheniformis ANSB821 (Guo Y. et al., 2020), which degraded AFB1 products assaying to L-02 cells. In summary, using B. amyloliquefaciens YUAD7 for AFB1 degradation was safe, as both the strain and the degradation products were non-toxic. Non-toxic products contributed to reducing the processing steps for by-products during the removal of AFB1 contamination in food and feed processing and ensured the safety of the degradation process.
The hydrogenation degradation pathway of YUAD7 was similar to the degradation pathway found by Aspergillus niger FS10. The Aspergillus niger FS10 degraded AFB1 by successive hydrolysis-decarboxylation, breaking down the large AFB1 molecule into non-toxic small molecules and removing the methoxy group from the benzene ring (Qiu et al., 2021). The enzymatic modification degradation pathway was similar to the degradation of AFB1 by B. licheniformis ANSB821, in which product presence of K and Na elements in the degradation products could result from enzyme binding to AFB1 through modification, addition to AFB1 molecules, and formation of [N+K+] and [N+Na+] ion precursors through collisions (Guo Y. et al., 2020). Candida versatilis CGMCC 3790 degraded AFB1 through the addition and hydrolysis pathway, resulting in four products with a chemical formula similar to that of YUAD7 degradation products (Li et al., 2018). Through structural analysis of metabolites and speculation of metabolic pathways, it confirmed that YUAD7 degradation sites were the double bonds of the furan ring, vanillin endolipid ring, and pentenone ring structure of AFB1, and the carcinogenic, teratogenic, and mutagenic toxicity sites in AFB1 was degraded through hydrolysis, enzyme modification, and loss of the -CO group for biological detoxification.
In the future, the incorporation of 14C labeling technology will be anticipated, enabling the labeling of C atoms in AFB1 and subsequently facilitating the meticulous tracking of its intricate degradation trajectory.
Bacillus amyloliquefaciens YUAD7, isolated from the extreme environment of the Qinghai-Tibet Plateau, can efficiently degrade AFB1 at 10 μg/mL, with a remarkable 91.7% efficiency within 72 h. It also removes over 85.0% of AFB1 from real food samples (AFB1 concentration 10 μg/g) within the same timeframe, establishing it as one of the most effective strains for degrading high AFB1 concentrations. The YUAD7 strain primarily degraded AFB1 through extracellular secretions and exhibited excellent thermal stability. Furthermore, B. amyloliquefaciens YUAD7 transformed AFB1 into non-toxic small molecular compounds, including C12H14O4, C5H12N2O2, C10H14O2, and C4H12N2O, through processes such as hydrogenation, enzyme modification, and the loss of the -CO group. This capability was valuable for reducing AFB1 contamination in food and feed processing. However, the degradation pathway of AFB1 by the YUAD7 strain was inferred based on the structure of the degradation products in this study. In the future, 14C tracing technology will be employed to meticulously trace the degradation pathway of YUAD7 in AFB1 within real food samples. This will provide a more precise understanding of the degradation metabolic pathway, offering technical support for the application of YUAD7.
The datasets presented in this study can be found in online repositories. The names of the repository/repositories and accession number(s) can be found in the article/Supplementary material.
Ethical approval was not required for the studies on animals in accordance with the local legislation and institutional requirements because only commercially available established cell lines were used.
YT: Conceptualization, Data curation, Investigation, Methodology, Software, Validation, Writing – original draft, Writing – review & editing. XL: Conceptualization, Funding acquisition, Writing – review & editing. LD: Data curation, Methodology, Writing – original draft. SH: Data curation, Methodology, Writing – original draft.
The author(s) declare that financial support was received for the research, authorship, and/or publication of this article. This research was funded by National Natural Science Foundation of China (grant number 32171674), Lanzhou Talent Innovation and Entrepreneurship Program (grant number 2023-RC-40), Gansu Provincial Department of Education 2023 Graduate Student “Innovation Star” Program (grant number 2023CXZX-622), and Gansu Provincial Science and Technology Department Key R&D Project (grant number 20YF8NA130).
The authors declare that the research was conducted in the absence of any commercial or financial relationships that could be construed as a potential conflict of interest.
All claims expressed in this article are solely those of the authors and do not necessarily represent those of their affiliated organizations, or those of the publisher, the editors and the reviewers. Any product that may be evaluated in this article, or claim that may be made by its manufacturer, is not guaranteed or endorsed by the publisher.
The Supplementary material for this article can be found online at: https://www.frontiersin.org/articles/10.3389/fmicb.2024.1367297/full#supplementary-material
Adebo, O. A., Njobeh, P. B., and Mavumengwana, V. (2016). Degradation and detoxification of AFB1 by Staphylocococcus warneri, Sporosarcina sp. and Lysinibacillus fusiformis. Food Control 68, 92–96. doi: 10.1016/j.foodcont.2016.03.021
Ali, S., Hassan, M., Essam, T., Ibrahim, M. A., and Al-Amry, K. (2021). Biodegradation of aflatoxin by bacterial species isolated from poultry farms. Toxicon 195, 7–16. doi: 10.1016/j.toxicon.2021.02.005
Alvarez, H. M., Herrero, O. M., Silva, R. A., Hernández, M. A., Lanfranconi, M. P., Villalba, M. S., et al. (2019). Insights into the metabolism of oleaginous Rhodococcus spp. Appl. Environ. Microbiol. 85:19. doi: 10.1128/aem.00498-19
Bai, J., Ding, Z., Ke, W., Xu, D., Wang, M., Huang, W., et al. (2021). Different lactic acid bacteria and their combinations regulated the fermentation process of ensiled alfalfa: ensiling characteristics, dynamics of bacterial community and their functional shifts. J. Microbial. Biotechnol. 14, 1171–1182. doi: 10.1111/1751-7915.13785
Biessy, A., and Filion, M. (2021). Phloroglucinol derivatives in plant-beneficial Pseudomonas spp.: biosynthesis, regulation, and functions. Metabolites 11:182. doi: 10.3390/metabo11030182
Cai, M., Qian, Y., Chen, N., Ling, T., Wang, J., Jiang, H., et al. (2020). Detoxification of aflatoxin B1 by Stenotrophomonas sp. CW117 and characterization the thermophilic degradation process. Environ. Pollut. 261:114178. doi: 10.1016/j.envpol.2020.114178
Campos-Avelar, I., Colas de la Noue, A., Durand, N., Cazals, G., Martinez, V., Strub, C., et al. (2021). Aspergillus flavus growth inhibition and aflatoxin B1 decontamination by Streptomyces isolates and their metabolites. Toxins 13:340. doi: 10.3390/toxins13050340
Chen, G., Fang, Q., Liao, Z., Xu, C., Liang, Z., Liu, T., et al. (2022). Detoxification of aflatoxin B1 by a potential probiotic Bacillus amyloliquefaciens WF2020. Front. Microbiol. 13:891091. doi: 10.3389/fmicb.2022.891091
Fang, Q., Du, M., Chen, J., Liu, T., Zheng, Y., Liao, Z., et al. (2020). Degradation and detoxification of aflatoxin B1 by tea-derived aspergillus Niger RAF106. Toxins 12:777. doi: 10.3390/toxins12120777
Garrity, D. R. B. R. W. C. G. M. (1994). "Bergey's manual of systematic bacteriology," 9th Edn.; Baltimore Williams and Wilkins: Philadelphia, USA.
Gonzalez, M. L. P., Martinez, M. P., and Cavaglieri, L. R. (2019). Presence of aiiA homologue genes encoding for N-acyl homoserine lactone-degrading enzyme in aflatoxin B1-decontaminating Bacillus strains with potential use as feed additives. Food Chem. Toxicol. 124, 316–323. doi: 10.1016/j.fct.2018.12.016
Guo, Y., Qin, X., Tang, Y., Ma, Q., Zhang, J., and Zhao, L. (2020). CotA laccase, a novel aflatoxin oxidase from Bacillus licheniformis, transforms aflatoxin B1 to aflatoxin Q1 and epi-aflatoxin Q1. Food Chem. 325:126877. doi: 10.1016/j.foodchem.2020.126877
Guo, D. J., Singh, R. K., Singh, P., Li, D. P., Sharma, A., Xing, Y. X., et al. (2020). Complete genome sequence of Enterobacter Roggenkampii ED5, a nitrogen fixing plant growth promoting endophytic bacterium with biocontrol and stress tolerance properties, isolated from sugarcane root. Front. Microbiol. 11:81. doi: 10.3389/fmicb.2020.580081
Kumar, V., Bahuguna, A., Lee, J. S., Sood, A., Han, S. S., Chun, H. S., et al. (2023). Degradation mechanism of aflatoxin B1 and aflatoxin G1 by salt tolerant Bacillus albus YUN5 isolated from ‘doenjang’, a traditional Korean food. Food Res. Int. 165:112479. doi: 10.1016/j.foodres.2023.112479
Lai, G., Wen, M., Jiang, Z., Zhou, F., Huo, H.-X., Zhu, M., et al. (2023). Novel oxidation oligomer of chlorogenic acid and (−)-epigallocatechin and its quantitative analysis during the processing of Keemun black tea. J. Agric. Food Chem. 71, 15745–15753. doi: 10.1021/acs.jafc.3c04571
Li, J., Huang, J., Jin, Y., Wu, C., Shen, D., Zhang, S., et al. (2018). Mechanism and kinetics of degrading aflatoxin B1 by salt tolerant Candida versatilis CGMCC 3790. J. Hazard. Mater. 359, 382–387. doi: 10.1016/j.jhazmat.2018.05.053
Loi, M., Logrieco, A. F., Pusztahelyi, T., Leiter, É., Hornok, L., and Pócsi, I. (2023). Advanced mycotoxin control and decontamination techniques in view of an increased aflatoxin risk in Europe due to climate change. Front. Microbiol. 13:1085891. doi: 10.3389/fmicb.2022.1085891
Marchese, S., Polo, A., Ariano, A., Velotto, S., Costantini, S., and Severino, L. (2018). Aflatoxin B1 and M1: biological properties and their involvement in cancer development. Toxins 10:214. doi: 10.3390/toxins10060214
Maxwell, L. A., Callicott, K. A., Bandyopadhyay, R., Mehl, H. L., Orbach, M. J., and Cotty, P. J. (2021). Degradation of aflatoxins B1 by atoxigenic aspergillus flavus biocontrol agents. Plant Dis. 105, 2343–2350. doi: 10.1094/PDIS-01-21-0066-RE
Qiu, T., Wang, H., Yang, Y., Yu, J., Ji, J., Sun, J., et al. (2021). Exploration of biodegradation mechanism by AFB1-degrading strain aspergillus Niger FS10 and its metabolic feedback. Food Control 121:107609. doi: 10.1016/j.foodcont.2020.107609
Rao, R. K., Vipin, A. V., Hariprasad, P., Appaiah, K. A. A., and Venkateswaran, G. (2017). Biological detoxification of aflatoxin B1 by Bacillus licheniformis CFR1. Food Control 71, 234–241. doi: 10.1016/j.foodcont.2016.06.040
Reif, B., Ashbrook, S. E., Emsley, L., and Hong, M. (2021). Solid-state NMR spectroscopy. Nat Rev Methods Primers 1:2. doi: 10.1038/s43586-020-00002-1
Risa, A., Krifaton, C., Kukolya, J., Kriszt, B., Cserhati, M., and Tancsics, A. (2018). Aflatoxin B1 and zearalenone-detoxifying profile of Rhodococcus type strains. Curr. Microbiol. 75, 907–917. doi: 10.1007/s00284-018-1465-5
Sharma, A., Sharma, A., and Tripathi, A. (2021). Biological activities of Pleurotus spp. polysaccharides: a review. J. Food Biochem. 45:13748. doi: 10.1111/jfbc.13748
Shu, X., Wang, Y., Zhou, Q., Li, M., Hu, H., Ma, Y., et al. (2018). Biological degradation of aflatoxin B1 by cell-free extracts of Bacillus velezensis DY3108 with broad pH stability and excellent thermostability. Toxins 10:330. doi: 10.3390/toxins10080330
Song, C., Yang, J., Wang, Y., Ding, G., Guo, L., and Qin, J. (2022). Mechanisms and transformed products of aflatoxin B1 degradation under multiple treatments: a review. Crit. Rev. Food Sci. Nutr. 64, 2263–2275. doi: 10.1080/10408398.2022.2121910
Suman, M. (2021). Last decade studies on mycotoxins’ fate during food processing: an overview. Curr. Opin. Food Sci. 41, 70–80. doi: 10.1016/j.cofs.2021.02.015
Wang, L., Huang, W., Shen, Y., Zhao, Y., Wu, D., Yin, H., et al. (2022). Enhancing the degradation of aflatoxin B1 by co-cultivation of two fungi strains with the improved production of detoxifying enzymes. Food Chem. 371:131092. doi: 10.1016/j.foodchem.2021.131092
Wang, L., Wu, J., Liu, Z., Shi, Y., Liu, J., Xu, X., et al. (2018). Aflatoxin B1 degradation and detoxification by Escherichia coli CG1061 isolated from chicken cecum. Front. Pharmacol. 9:1548. doi: 10.3389/fphar.2018.01548
Wang, Y., Zhang, H., Yan, H., Yin, C., Liu, Y., Xu, Q., et al. (2018). Effective biodegradation of aflatoxin B1 using the Bacillus licheniformis (BL010) strain. Toxins 10:497. doi: 10.3390/toxins10120497
Xia, X., Zhang, Y., Li, M., Garba, B., Zhang, Q., Wang, Y., et al. (2017). Isolation and characterization of a Bacillus subtilis strain with aflatoxin B1 biodegradation capability. Food Control 75, 92–98. doi: 10.1016/j.foodcont.2016.12.036
Xiong, D., Wen, J., Lu, G., Li, T., and Long, M. (2022). Isolation, purification, and characterization of a laccase-degrading aflatoxin B1 from Bacillus amyloliquefaciens B10. Toxins 14:250. doi: 10.3390/toxins14040250
Xu, L., Ahmed, M. F. E., Sangare, L., Zhao, Y., Selvaraj, J. N. S., Xing, Y., et al. (2017). Novel aflatoxin-degrading enzyme from Bacillus shackletonii L7. Toxins 9:36. doi: 10.3390/toxins9010036
Yan, Y., Zhang, X., Chen, H., Huang, W., Jiang, H., Wang, C., et al. (2022). Isolation and aflatoxin B1-degradation characteristics of a microbacterium proteolyticum B204 strain from bovine faeces. Toxins 14:525. doi: 10.3390/toxins14080525
Yang, P., Wu, W., Zhang, D., Cao, L., and Cheng, J. (2023). AFB1 microbial degradation by Bacillus subtilis WJ6 and its degradation mechanism exploration based on the comparative transcriptomics approach. Metabolites 13:785. doi: 10.3390/metabo13070785
Yu, R., Liu, J., Wang, Y., Wang, H., and Zhang, H. (2021). Aspergillus Niger as a secondary metabolite factory. Front. Chem. 9:1022. doi: 10.3389/fchem.2021.701022
Zhang, J., Sun, X., Chai, X., Jiao, Y., Sun, J., Wang, S., et al. (2024). Curcumin mitigates oxidative damage in broiler liver and ileum caused by AFB1-contaminated feed through Nrf2 signaling pathway. Animals 14:409. doi: 10.3390/ani14030409
Zhao, C., Dong, L., Zhang, F., Luo, Y., Yang, Z., Zhang, X., et al. (2022). Screening and characterization of a salt-tolerant aflatoxin B1-degrading strain isolated from Doubanjiang, a Chinese typical red pepper paste. Food Sci Technol 42:621. doi: 10.1590/fst.122621
Keywords: aflatoxin B1, Bacillus amyloliquefaciens YUAD7, biological detoxification, detoxification mechanism, degradation products
Citation: Tang Y, Liu X, Dong L and He S (2024) Screening and identification of an aflatoxin B1-degrading strain from the Qinghai-Tibet Plateau and biodegradation products analysis. Front. Microbiol. 15:1367297. doi: 10.3389/fmicb.2024.1367297
Received: 08 January 2024; Accepted: 03 April 2024;
Published: 01 May 2024.
Edited by:
Lucilla Iacumin, University of Udine, ItalyReviewed by:
Vishal Kumar, Yeungnam University, Republic of KoreaCopyright © 2024 Tang, Liu, Dong and He. This is an open-access article distributed under the terms of the Creative Commons Attribution License (CC BY). The use, distribution or reproduction in other forums is permitted, provided the original author(s) and the copyright owner(s) are credited and that the original publication in this journal is cited, in accordance with accepted academic practice. No use, distribution or reproduction is permitted which does not comply with these terms.
*Correspondence: Xiaojing Liu, bGl1eGpAZ3NhdS5lZHUuY24=
Disclaimer: All claims expressed in this article are solely those of the authors and do not necessarily represent those of their affiliated organizations, or those of the publisher, the editors and the reviewers. Any product that may be evaluated in this article or claim that may be made by its manufacturer is not guaranteed or endorsed by the publisher.
Research integrity at Frontiers
Learn more about the work of our research integrity team to safeguard the quality of each article we publish.