- 1Center for Innovative Biomedicine and Biotechnology (CIBB), University of Coimbra, Coimbra, Portugal
- 2UCIBIO—Applied Molecular Biosciences Unit, Laboratory of Microbiology, Department of Biological Sciences, Faculty of Pharmacy, University of Porto, Porto, Portugal
- 3Associate Laboratory i4HB—Institute for Health and Bioeconomy, Faculty of Pharmacy, University of Porto, Porto, Portugal
- 4Faculty of Nutrition and Food Sciences, University of Porto, Porto, Portugal
- 5School of Medicine and Biomedical Sciences, University of Porto (ICBAS-UP), Porto, Portugal
- 6ESS, Polytechnic Institute of Porto, Porto, Portugal
The rise of antibiotic resistance in the food chain is influenced by the use of antimicrobial agents, such as antibiotics, metals, and biocides, throughout the entire farm-to-fork continuum. Besides, non-clinical reservoirs potentially contribute to the transmission of critical pathogens such as multidrug-resistant (MDR) Klebsiella pneumoniae. However, limited knowledge exists about the population structure and genomic diversity of K. pneumoniae circulating in conventional poultry production. We conducted a comprehensive characterization of K. pneumoniae across the whole chicken production chain (7 farms; 14 flocks + environment + meat, 56 samples; 2019–2022), exploring factors beyond antibiotics, like copper and quaternary ammonium compounds (QACs). Clonal diversity and adaptive features of K. pneumoniae were characterized through cultural, molecular (FT-IR), and whole-genome-sequencing (WGS) approaches. All except one flock were positive for K. pneumoniae with a significant increase (p < 0.05) from early (n = 1/14) to pre-slaughter (n = 11/14) stages, most (n = 6/7) persisting in chicken meat batches. Colistin-resistant K. pneumoniae rates were low (4%-n = 1/24 positive samples), while most samples carried MDR strains (67%-n = 16/24) and copper-tolerant isolates (63%-n = 15/24, with sil and pco gene clusters; MICCuSO4 ≥ 16 mM), particularly at pre-slaughter. Benzalkonium chloride consistently exhibited activity against K. pneumoniae (MIC/MBC range = 4–64 mg/L) from representative strains independently of the presence or absence of genes linked to QACs tolerance. A polyclonal K. pneumoniae population, discriminated by FT-IR and WGS, included various lineages dispersed throughout the chicken’s lifecycle at the farm (ST29-KL124, ST11-KL106, ST15-KL19, ST1228-KL38), until the meat (ST1-KL19, ST11-KL111, ST6405-KL109, and ST6406-CG147-KL111), or over years (ST631-49 KL109, ST6651-KL107, ST6406-CG147-KL111). Notably, some lineages were identical to those from human clinical isolates. WGS also revealed F-type multireplicon plasmids carrying sil + pco (copper) co-located with qacEΔ1 ± qacF (QACs) and antibiotic resistance genes like those disseminated in humans. In conclusion, chicken farms and their derived meat are significant reservoirs for diverse K. pneumoniae clones enriched in antibiotic resistance and metal tolerance genes, some exhibiting genetic similarities with human clinical strains. Further research is imperative to unravel the factors influencing K. pneumoniae persistence and dissemination within poultry production, contributing to improved food safety risk management. This study underscores the significance of understanding the interplay between antimicrobial control strategies and non-clinical sources to effectively address the spread of antimicrobial resistance.
1 Introduction
Intensive poultry production is a crucial sector of the global food industry. It faces significant challenges in addressing the growing demand for poultry products, especially antibiotic-free chicken (Mottet and Tempio, 2017; Karcher and Mench, 2018). Ensuring biosecurity, which includes proper hygiene practices, vaccination programs, regular monitoring of flock health and effective farm management strategies, is essential to prevent disease transmission between flocks and farms. However, despite these efforts, intensive chicken production relies heavily on antibiotics and coccidiostats (Karcher and Mench, 2018; Gržinić et al., 2023). This reliance becomes even more concerning when considering that poultry presents potential risks to human health as it can be a reservoir of zoonotic pathogens causing infectious diseases and can contribute to the spread of antimicrobial resistance (AMR) within the food chain [Golden et al., 2021; European Food Safety Authority (EFSA) and European Centre for Disease Prevention and Control (ECDC), 2023]. While antibiotics have traditionally been seen as the main drivers of AMR, recent restrictions on their use in food-producing animals, as well as alternative antimicrobial approaches, suggest the contribution of other compounds as selectors of antibiotic-resistant bacteria (Rebelo et al., 2023).
Metals and biocides are used in food-animal production for various purposes, including as feed additives, growth promoters, antiseptics, and disinfectants, to decrease the dependence on antibiotics [EMA Committee for Medicinal Products for Veterinary Use (CVMP) and EFSA Panel on Biological Hazards (BIOHAZ) et al., 2017; Rebelo et al., 2023]. Copper-Cu is one of the metals commonly added to chicken feed. Its antimicrobial properties not only improve animal nutrition and productivity (e.g., by modulating gut microbiota) but also reduce disease risks, thereby boosting the overall flock health (Broom et al., 2021; El Sabry et al., 2021). Furthermore, biocides formulated with quaternary ammonium compounds (QACs) are used to disinfect surfaces, equipment, feeding systems, and water sources. Their versatility and broad-spectrum antimicrobial activity assist in controlling and preventing pathogens dissemination between flocks within poultry facilities (Chen et al., 2023). Genes associated with tolerance to metals and QACs often share the same genetic contexts with antibiotic-resistance genes (Slifierz et al., 2015; Li et al., 2022; Pereira et al., 2023). Thus, the use of copper-supplemented feed and QAC-based biocides could contribute to co-selection effects (Webber et al., 2015; Kampf, 2018; Rebelo et al., 2023).
Current intensive chicken production involves large-scale operations that span breeding and hatching to rearing, processing, and distribution. However, the impact of diverse antimicrobial strategies used throughout farm to fork on the spread of AMR remains underexplored [EMA Committee for Medicinal Products for Veterinary Use (CVMP) and EFSA Panel on Biological Hazards (BIOHAZ) et al., 2017; Rebelo et al., 2023]. To effectively address AMR, a One Health approach – which emphasizes coordinated efforts across the domains of animals, humans, and the environment – is essential. This approach is not only crucial for elucidating the origins of less common foodborne pathogens such as K. pneumoniae but also for understanding emergent reservoirs and vectors of AMR genes outside the clinical setting (Wyres and Holt, 2018). Our prior study unveiled a significant occurrence of copper tolerance and multidrug resistance among K. pneumoniae strains found in chicken flocks (Mourão et al., 2023). We also identified K. pneumoniae lineages and plasmids carrying sil + pco copper tolerance and variable antibiotic resistance genes, resembling those identified in human clinical isolates worldwide (Mourão et al., 2023). These findings highlight the urgent need to further investigate the factors, drivers, and sources that contribute to the selection and persistence of multidrug resistant (MDR) K. pneumoniae within the poultry production chain. However, there has been limited research focusing on the early stages of chicken rearing (e.g., one-day-old chicks) and the in-house poultry environment, including cleaned poultry houses post-vacancy, where broilers are raised for 30–35 days before being sent to slaughter for meat production (Daehre et al., 2018; Zhai et al., 2020).
This study aims to provide a comprehensive analysis of the occurrence, diversity, and persistence of K. pneumoniae in the whole poultry production chain (from one-day-old chicks to chicken meat) between 2019 and 2022. Furthermore, we assessed the contribution of factors other than antibiotics (use of copper and QACs) as putative selective agents of AMR genes and bacteria that are clinically relevant. Identifying the transmission sources and pathways for MDR K. pneumoniae will pave the way for devising effective strategies to mitigate its dissemination, ensuring animal welfare, environmental sustainability, public health, and overall improved food safety.
2 Materials and methods
2.1 Sampling design at the chicken farm and slaughterhouse processing plant
Our sampling included seven Portuguese intensive-based chicken farms with conventional indoor and floor-raised production systems in compliance with EU legislation, as indicated by the operator (ADAS, 2016). Six similar farms (arbitrarily designated as A, B, C, E, G, and H) previously studied (Mourão et al., 2023) and one (I) recently restructured with modern grow-out poultry house facilities were selected. In all farms, colistin was banned since January 2018, while copper was routinely used as an additive in inorganic formulation feed (far below the maximum dose of 25 mg/kg of Cu, according to EU Regulation 2018/1039). The vacancy period varied from 11 to >15 days, being each poultry house depopulated, cleaned, and disinfected using similar routine cleaning and disinfection procedures. The biocide active compounds used were benzalkonium chloride (BZC) or didecyl dimethyl ammonium chloride (DDAC) and hydrogen peroxide for water distribution systems.
In each of the seven farms, female, and male mixed one-day-old chicks (Ross 308 strain) were randomly distributed by two poultry houses at arrival (around 5,000–60,000 chickens per flock/house). A total of 14 flocks were followed and sampling (n = 56 samples) was carried out over three different periods during the production cycle (the length ranged from 26 to 43 days) between February and May 2022 (Figure 1). The initial sampling period included collecting samples from one-day-old chicken transport boxes (P0; n = 14 samples; each sample comprising 12 boxes). These were obtained using stick swabs, with each swab tube containing 10 mL of Buffered Peptone Water-BPW and used in 4 chicken boxes (a total of three swabs per sample). Additionally, the inside of cleaned poultry houses (P1; n = 14 samples) were sampled using the boot swab technique, which employs dry-foot swabs in a zigzag pattern, according to EU Regulation 200/2012. In the second period, the inside of grow-out houses containing the same flocks the day before slaughter (P2; n = 14 samples; after >25 days) was collected using the boot swab technique. In the third period, raw chicken meat samples (P3; n = 14 batches) from the same flocks were collected after slaughter and air chilling at the poultry production slaughterhouses, immediately before distribution for retail sale. Each sample (50 g) was processed as a pool of neck skin from 10 to 15 carcasses of the same batch (each batch corresponded to one flock from the same farm and poultry house slaughtered at the same time; according to EU Regulation 1086/2011).
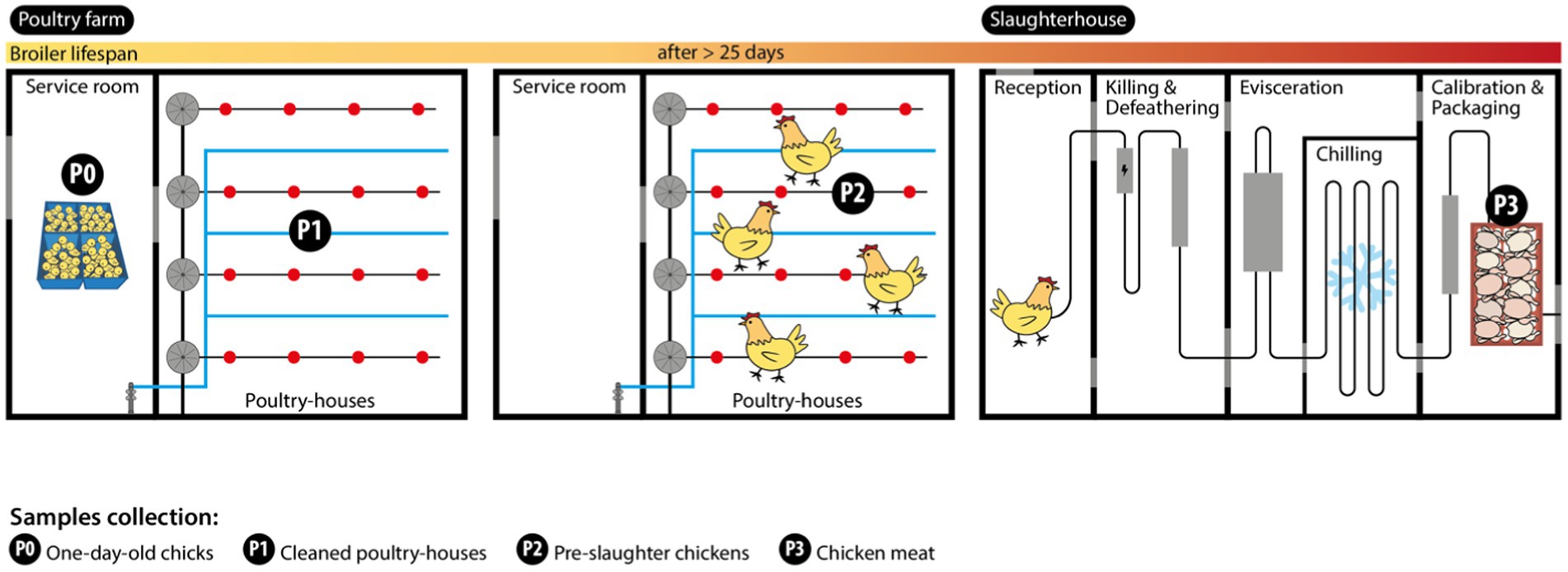
Figure 1. Sampling strategy at the chicken farm and slaughterhouse processing plant. Sample collection points are indicated by P0, P1, P2, and P3.
All previous samples were collected in sterile plastic bags/containers, transported at 4°C to the laboratory, and processed on the same day. Subsequent sample processing was performed using cultural/molecular approaches as described in the following sections.
2.2 Screening of Klebsiella pneumoniae
Klebsiella pneumoniae was recovered from Simmons citrate agar plates with 1% inositol (SCAi), the most suitable selective medium for Klebsiella recovery, directly from the suspended sample and after enrichment. A common initial step consisted of mixing swabs (three pooled swab tubes-P0 and two swab foot-P1 and P2) or weighing 25 g of pooled meat samples (P3) into 1/10 mL of BPW and BPW supplemented with 3.5 mg/L colistin. The direct culture method included spreading an aliquot of 100 μL of BPW and BPW + colistin after 1 h at room temperature (resuscitation step) on SCAi supplemented or not with colistin (3.5 mg/L). The enrichment approach involved the same procedure but after a previous incubation of BPW and BPW + colistin at 37°C for 16–18 h. All the SCAi plates were incubated at 37°C for 48 h. One to five colonies of each presumptive morphotype were selected for identification. Isolates were identified presumptively by CHROMagar™ Orientation and then by PCR for K. pneumoniae (Bialek-Davenet et al., 2014).
2.3 Klebsiella pneumoniae diversity between samples
We used Fourier Transform Infrared (FT-IR) spectroscopy with attenuated total reflectance (ATR) to infer isolates’ relatedness between isolates identified in the same or different samples. After growth under standardized culture conditions (Mueller-Hinton agar; 37°C/18 h), a colony was directly deposited and air-dried on the ATR accessory of the FT-IR instrument. Spectra were acquired in a Spectrum Two instrument (Perkin-Elmer, United States) under standard conditions (4,000–600 cm−1, 4 cm−1 resolution, and 16 scan co-additions). The region between 1,200 and 900 cm−1 was compared with each other, and with those from two different machine-learning classification models, used to predict K. pneumoniae capsular (KL) types: (i) a Random Forest classification model that enables identification of up to 33 KL-types from well-characterized international K. pneumoniae clones from the clinical setting (Novais Â. et al., 2023); and (ii) a Random Forest classification model to allow identification of up to 21 KL-types (11 in common with the previous model) based on a spectral database of poultry isolates identified in previous studies (Mourão et al., 2023). Isolate relatedness and prediction of KL-types were inferred as described previously (Novais Â. et al., 2023). Isolates predicted to have the same KL-type were considered putatively related. FT-IR-based assignments were confirmed by PCR of the wzi gene and further sequencing at Eurofins Genomics1 to infer K-type using BIGSdb2 (Brisse et al., 2013).
2.4 Antimicrobial susceptibility to antibiotics
Susceptibility to 17 antibiotics (amoxicillin + clavulanic acid-30 μg, amikacin-30 μg, aztreonam-30 μg, cefepime-30 μg, cefotaxime-5 μg, cefoxitin-30 μg, ceftazidime-10 μg, chloramphenicol-30 μg, ciprofloxacin-5 μg, gentamicin-10 μg, kanamycin-30 μg, meropenem-10 μg, nalidixic acid-30 μg, sulfamethoxazole-300 μg, tetracycline-30 μg, tobramycin-10 μg, and trimethoprim-5 μg) was determined using the disc diffusion method. The Minimum Inhibitory Concentration (MIC) of colistin was determined using the reference broth microdilution method (European Committee for Antimicrobial Susceptibility Testing, 2016). Escherichia coli ATCC 25922 was used as the control strain. The results of both assays were interpreted using the European Committee on Antimicrobial Susceptibility Testing (2022) and, when this was not possible, the guidelines of Clinical and Laboratory Standards Institute (2022). Isolates categorized as “susceptible, increased exposure” (EUCAST guidelines) or “intermediate resistant” (CLSI guidelines) were classified as susceptible. Multidrug resistance was considered when the isolates were resistant to three or more antibiotics from different families (in addition to ampicillin, to which all K. pneumoniae are intrinsically resistant). Screening of colistin resistance genes (mcr-1-5 and mcr-6-9) was performed for all isolates using two multiplex PCR (Rebelo et al., 2018; Borowiak et al., 2020).
2.5 Antimicrobial susceptibility to copper
Copper-Cu susceptibility in representative isolates (different farms, flocks, and genomic backgrounds) was evaluated using the agar dilution method under anaerobic conditions (Mourão et al., 2016). Briefly, the MIC was determined using Mueller-Hinton 2 agar freshly prepared and supplemented with different copper sulfate (CuSO4) concentrations (0.5 to 36 mM) and a final adjustment to pH = 7.2. The plates were then inoculated with 0.001 mL suspension (107 CFU/mL) of each isolate and incubated at 37°C under anaerobic conditions for 18 h-20 h. The MIC was identified as the lowest concentration where no visible growth was observed. Control strains included Escherichia coli ED8739 carrying the plasmid pRJ1004 with the sil and pco cluster (MICCuSO4 = 16-20 mM) and Enterococcus lactis BM4105RF without acquired copper tolerance genes (MICCuSO4 = 2–4 mM; Novais C. et al., 2023). All K. pneumoniae isolates were screened for the silA copper tolerance gene using a PCR, given its strong association with the presence of an intact sil operon and a Cu tolerance (CuT) phenotype (Mourão et al., 2016, 2023).
2.6 Antimicrobial susceptibility to benzalkonium chloride
The MIC and minimum bactericidal concentrations (MBC) of benzalkonium chloride (BZC; CAS 68391-01-5, VWR) were determined for representative sequenced isolates (n = 45; different clones, sample types, farms, years and the presence or absence of QAC tolerance genes) using the Mueller-Hinton broth microdilution method (pH = 7.2; 37°C/20 h; Clinical and Laboratory Standards Institute, 2018). Briefly, a 96-well microtiter plate containing serial two-fold dilutions of BZC (concentration ranging from 0.125 to 128 mg/L) was used to assess the susceptibility of bacterial suspensions in log-phase growth (adjusted to reach a final inoculum of 5 × 105 CFU/mL in each well) at 37°C for 20 h. Microdilution panels were freshly prepared before each assay. The first concentration of BZC without visible growth was considered the MIC (Clinical and Laboratory Standards Institute, 2018).
To determine the MBCBZC, 10 μL of each well without visible growth from the 96-well MIC plate were incubated onto brain heart infusion agar plates at 37°C for 24 h, as defined by the Clinical and Laboratory Standards Institute (1999). The MBC was defined as the lowest QAC concentration where the colony count was equal to or less than the CLSI-specified rejection value, based on the final inoculum count of each well (Clinical and Laboratory Standards Institute, 1999). Each experiment was repeated three times and the MIC/MBC values corresponded to the mean of these determinations. To ensure assay reproducibility, the Enterococcus faecalis ATCC 29212 (without any known QACs tolerance genes) was included as a control strain (MICBZC and MBCBZC varied between 1 and 4 mg/L; Pereira et al., 2023).
2.7 Genomic analysis by whole-genome sequencing
We then aimed to elucidate the sources and persistence of specific clonal lineages and/or MDR plasmids along the chicken production. For that, we compared 68 genomes, including 48 sequenced de novo in the present study (n = 31 from 2022 farms and n = 17 from 2019 to 2020 farms) and 20 obtained in the previous study (Mourão et al., 2023), representing different farms, stages, timespans, and KL-types. Genomic DNA was extracted from the isolates using the Wizard Genomic DNA purification kit (Promega Corporation, Madison, WI) and the final concentration was measured with a Qubit 3.0 Fluorometer (Invitrogen, Thermo Fisher Scientific, United States). Subsequently, the isolates were sequenced using the Illumina NovaSeq 6,000 S4 PE150 XP (Illumina, San Diego, CA, United States) at Eurofins Genomics (see text footnote 1). FastQC v0.11.9 (Andrews, 2010) and MultiQC v1.13.dev0 (Ewels et al., 2016) with default parameters were used for the quality control of the raw sequence data. If needed, the reads were filtered using BBDuk v39.01,3 followed by de novo assembly with SPAdes v3.15.5 (Bankevich et al., 2012) integrated within Unicycler v0.5.0 (Wick et al., 2017). QUAST v5.0.2 (Gurevich et al., 2013) was used for the quality evaluation of assemblies and CheckM v1.2.2 (Parks et al., 2015) and BUSCO v5.4.6 (Simão et al., 2015) for genome completeness assessment.
The assemblies were annotated using the RAST server (Aziz et al., 2008). Genomes’ assemblies were then submitted to Pathogenwatch v2.3.34 which provided information on the capsular polysaccharide (K) and lipopolysaccharide (O) locus types and serotypes using Kaptive (Wyres and Holt, 2016), but also multi-locus sequence type (MLST; Diancourt et al., 2005) and core genome MLST (cgMLST). To infer a neighbor-joining tree for phylogenetic analysis, Pathogenwatch calculates pairwise single nucleotide polymorphism (SNP) distances between genomes based on a concatenated alignment of 1,972 core genes (Argimón et al., 2021). We then compared our genomes to those in Pathogenwatch, using cgMLST single linkage clustering, and selected those that had <10 allele differences (threshold = 10) and <21 SNPs (David et al., 2019) as the most closely related. All genome metadata in the neighbor-joining trees were added using iToL (Letunic and Bork, 2021).
Prediction of antibiotic resistance determinants (acquired and chromosomal mutations), and virulence traits (yersiniabactin-YbST, colibactin-CbST, aerobactin-AbST, salmochelin-SmST, and regulators of mucoid phenotype-RmpA, and RmpA2) was performed with Kleborate v2.3.0 (Lam et al., 2021). ABRicate v1.0.15 with in-house databases was used to detect additional K. pneumoniae virulence genes from BIGSdb-Pasteur,6 and the chaperone-usher pili system (kpiABCDEFG; Gato et al., 2020). The BacMet2 database, which is experimentally confirmed and available from http://bacmet.biomedicine.gu.se/, was manually curated to exclusively include proteins found in Klebsiella pneumoniae (including AcrAB-TolC, CpxAR, EmmdR, EmrDE, KdeA, KexD, KmrA, KpnEFO, MdtKM, OqxAB, PhoPQ, QacEΔ1, QacF, SugE, and TehA). This database, along with an in-house database of acquired metal tolerance proteins (including ArsR1H-ArsD1A1A2-ArsCBA3D2R2, PcoGE1ABCDRSE2, SilESRCFBAGP, MerRTPCADE, and TerZABCDEF-TerWY1XY2Y3) were used as reference for identifying biocide (QACs) and metal tolerance, respectively. The BLASTX 2.14.0+ (Camacho et al., 2009), with additional parameters “-evalue 0.001 -query_gencode 11,” was employed to perform the sequence alignment of the bacterial genomes against both databases. Confirmation of antibiotic-resistant novel alleles was performed using AMRFinderPlus v3.11.4 (Feldgarden et al., 2021).
Plasmid replicon typing was performed on all WGS-selected isolates using ABRicate v1.0.1 (see text footnote 5) with PlasmidFinder database (from 18 July 2023) and pMLST v2.0 (Camacho et al., 2009; Carattoli et al., 2014) from the Centre for Genomic and Epidemiology.7 IncFIIK plasmids were further characterized, as described by Villa et al. (2010).8 We used the MOB-recon tool v3.1.0 from the MOB-suite package to confirm the location of the metal tolerance genes and reconstruct putative plasmids based on draft assemblies (Robertson and Nash, 2018; Robertson et al., 2020). Metal tolerance genes were considered part of a specific plasmid when identified by MOB-recon or when located on the same contig as the replicon/incompatibility determinant.
2.8 Statistical analysis
Differences in occurrence, antimicrobial resistance, and copper tolerance among K. pneumoniae P0, P1, P2, and P3 samples as well as isolates were analyzed by Fisher’s exact test (α = 0.05) using Prism software, version 8.1.1 (GraphPad).
3 Results
3.1 Occurrence of Klebsiella pneumoniae by poultry samples
We detected K. pneumoniae in 43% (n = 24/56) of the samples collected throughout all stages of the poultry production chain. The highest occurrence was found in pre-slaughter fecal chicken samples (79%-11/14 flocks; in all but one farm), followed by derived chicken meat (50%-7/14 batches; all farms), and less frequently in one-day-old chicks (7%-1/14 flocks; one farm). Notably, K. pneumoniae was also detected in cleaned poultry houses (36%-5/14 flocks; four farms; Figure 2). Although differences were observed between farms, a significant increase in occurrence was found between one-day-old (1/14 flocks) and pre-slaughter (11/14 flocks) chickens (p < 0.05), and most (n = 6/7 flocks) of these maintained K. pneumoniae carriage in chicken meat at the slaughter stage. Only three out of 11 pre-slaughter flocks carrying K. pneumoniae were raised in cleaned poultry houses that had previously tested positive for these bacteria (Figure 2).
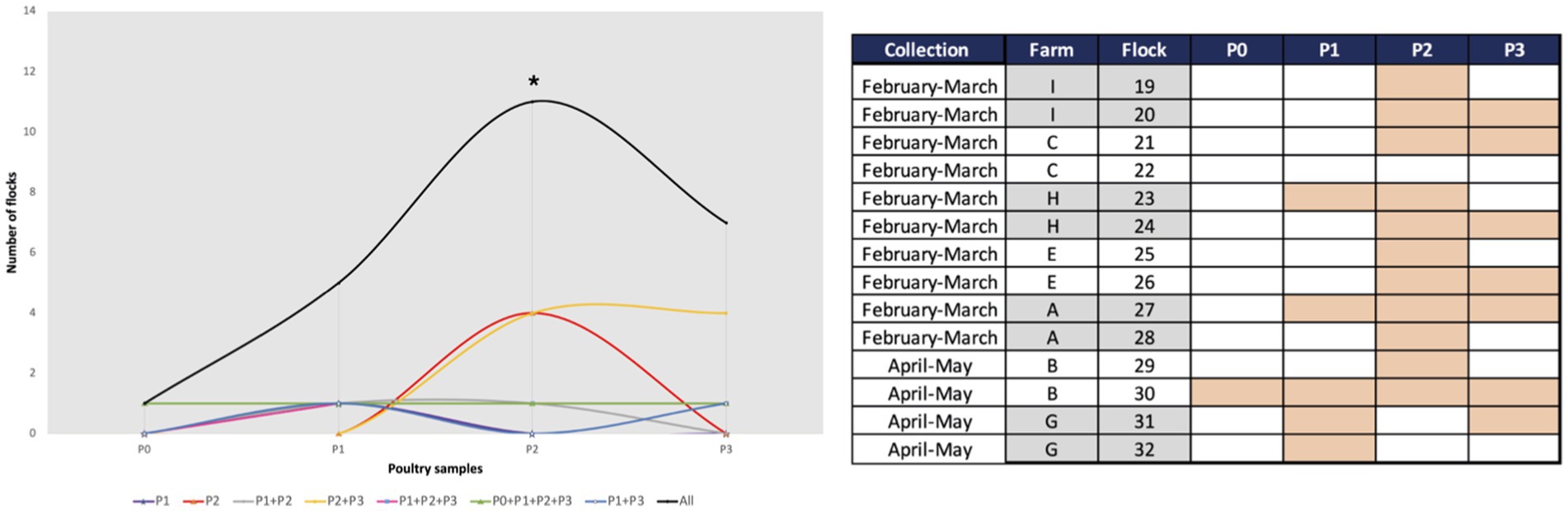
Figure 2. Occurrence of Klebsiella pneumoniae in poultry samples from the farm (P0, P1, and P2) and chicken meat (P3). *p < 0.05 (Fisher’s exact test) when comparing P0 with P2. Farms were arbitrarily designated by capital letters as A, B, C, E, G, H and I. The presence of K. pneumoniae is indicated by a filled rectangle in the table.
3.2 Diversity of Klebsiella pneumoniae along the poultry chain
From the 24 positive samples, we recovered 99 isolates of K. pneumoniae. Most isolates (>80%) were recovered from pre-slaughter chickens (65%-n = 64/99, 11 flocks, all but one farm) and chicken meat (16%-n = 16/99, 8 flocks, all farms; Figure 3). With FT-IR, we were able to discriminate 24 putative KL-types, some of them identified in >4 isolates in the same (KL23, KL109) or different samples (KL19, KL30, KL38, KL111). Furthermore, 54/99 (55%) isolates were correctly identified as carrying 14 KL-types included in the classification models used (KL3, KL10, KL14, KL19, KL21, KL23, KL28, KL30, KL38, KL64, KL102, KL106, KL109, KL111). Additionally, 15/99 (15%) isolates were grouped in six (n = 2–4 isolates each) highly related spectral profiles. We used this information to select representative isolates by sample, antibiotic susceptibility profile, and CuT based on the presence of the silA gene for further characterization by wzi sequencing and/or whole genome sequencing.
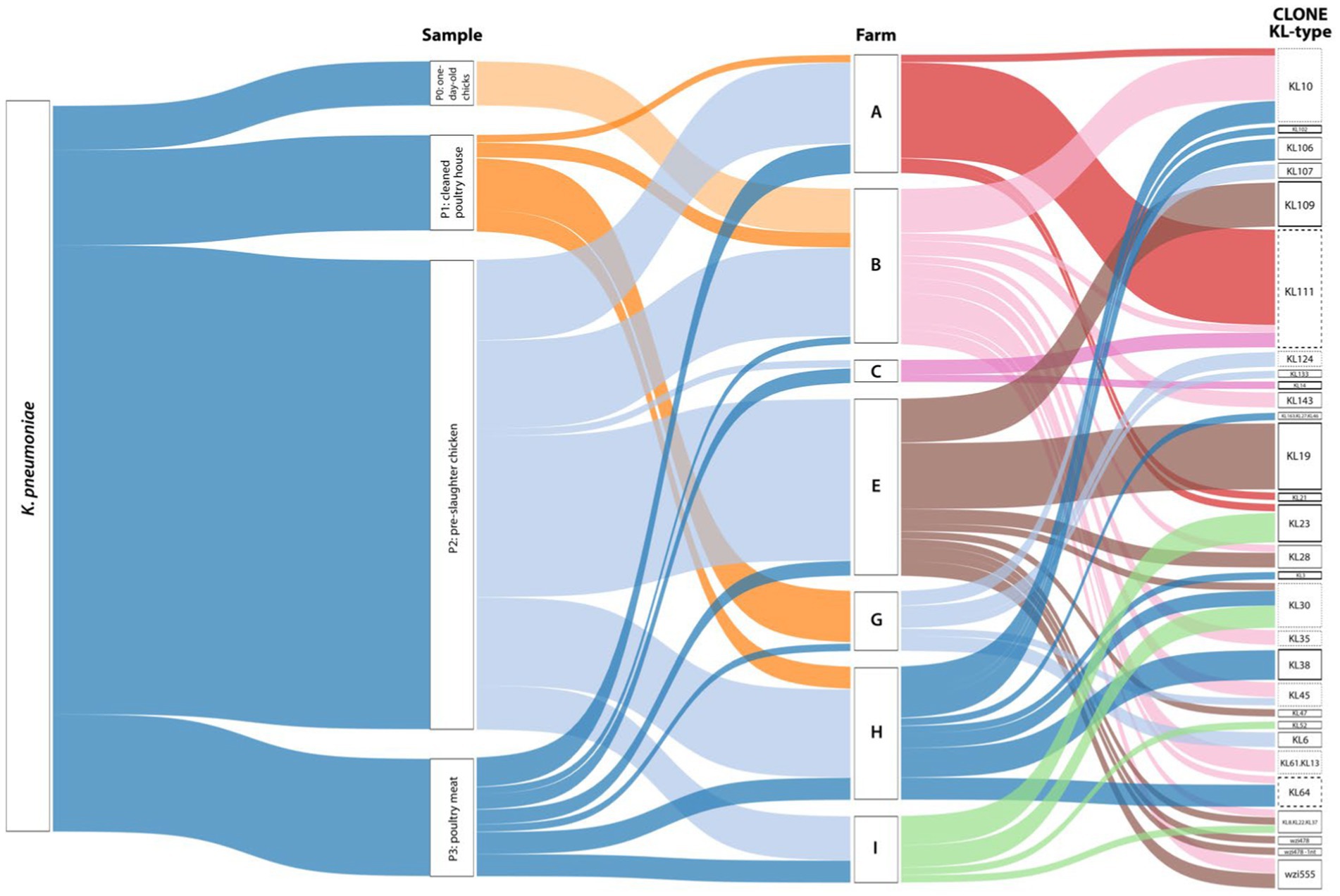
Figure 3. Sankey diagram representing, from left to right, the occurrence and diversity of Klebsiella pneumoniae by sample, farm, and KL-type. The width of each connection is proportional to the number of positive hits. The bold black line represents KL-types where 100% of the isolates were correctly identified by FTIR (KL3, KL14, KL19, KL21, KL23, KL38, KL102, KL109). The bold and dashed black line represents KL-types where at least 75% of the isolates were correctly identified by FTIR (KL64, KL111). The black dashed line represents KL-types where all the isolates exhibit highly related profiles recognized by FT-IR (KL10, KL30, KL35, KL45, KL124, KL61.KL13). The sensitivity and specificity of FTIR for KL-typing were 78% and 80%, respectively. Farms were arbitrarily designated by capital letters as A, B, C, E, G, H, and I. The Sankey diagram was generated using Tableau Desktop 2023.3 (https://www.tableau.com/).
Considering FT-IR and sequencing data, a total of 28 capsular locus (KL)-types were identified (13 detected in more than one sample), with the highest diversity observed in the pre-slaughter samples (Figure 3). The most frequent KL-types included KL111 (16%-n = 16/99 isolates; P2 + P3; 3 farms/4 flocks), KL10 (10%-n = 10/99; P0 + P2; 3 farms/3 flocks), KL19 (9%-n = 9/99; P2; 1 farm/2 flocks), KL30 (6%-n = 6/99; P2 + P3; 3 farms/3 flocks), KL109 (6%-n = 6/99; P2; 1 farm/1 flock) and KL23 (5%-n = 5/99; P1 + P2; 2 farms/2 flocks). Although less frequent, other KL types were shared by different farms, flocks, or stages (Figure 3; Supplementary Table S1).
3.3 Antibiotic susceptibility of Klebsiella pneumoniae recovered from poultry chain
More than 50% of the 24 positive samples showed at least one K. pneumoniae isolate with decreased susceptibility to ciprofloxacin (83%-n = 20/24) or resistance to nalidixic acid (58%-n = 14/24), tetracycline (71%-n = 17/24), sulphonamides (67%-n = 16/24) or trimethoprim (63%-n = 15/24; Figure 4). Overall, 56% (n = 55/99) of the 99 identified isolates were MDR (Supplementary Table S1) and were recovered in most positive samples (67%-n = 16/24) from all farms. Samples carrying MDR isolates were significantly more frequent in pre-slaughter (P2) than in chicken meat (P3) stages (p < 0.05; Figure 4). Approximately 25% (n = 6/24) of samples (including those from cleaned poultry houses, pre-slaughter chickens, and chicken meat) from diverse farms/flocks, had at least one isolate resistant to extended-spectrum-cephalosporins (including n = 6 isolates/3 samples with an ESBL phenotype). Colistin-resistant isolates (MIC > 16 mg/L; mcr negative) were identified only in one pre-slaughter flock positive sample (4%-n = 1/24), with all of them from the same clone KL109 recovered from SCAi + colistin plates. Most of the other isolates (n = 91/99) were recovered using SCAi plate with (n = 56/91) or without (n = 35/91) an enrichment step.
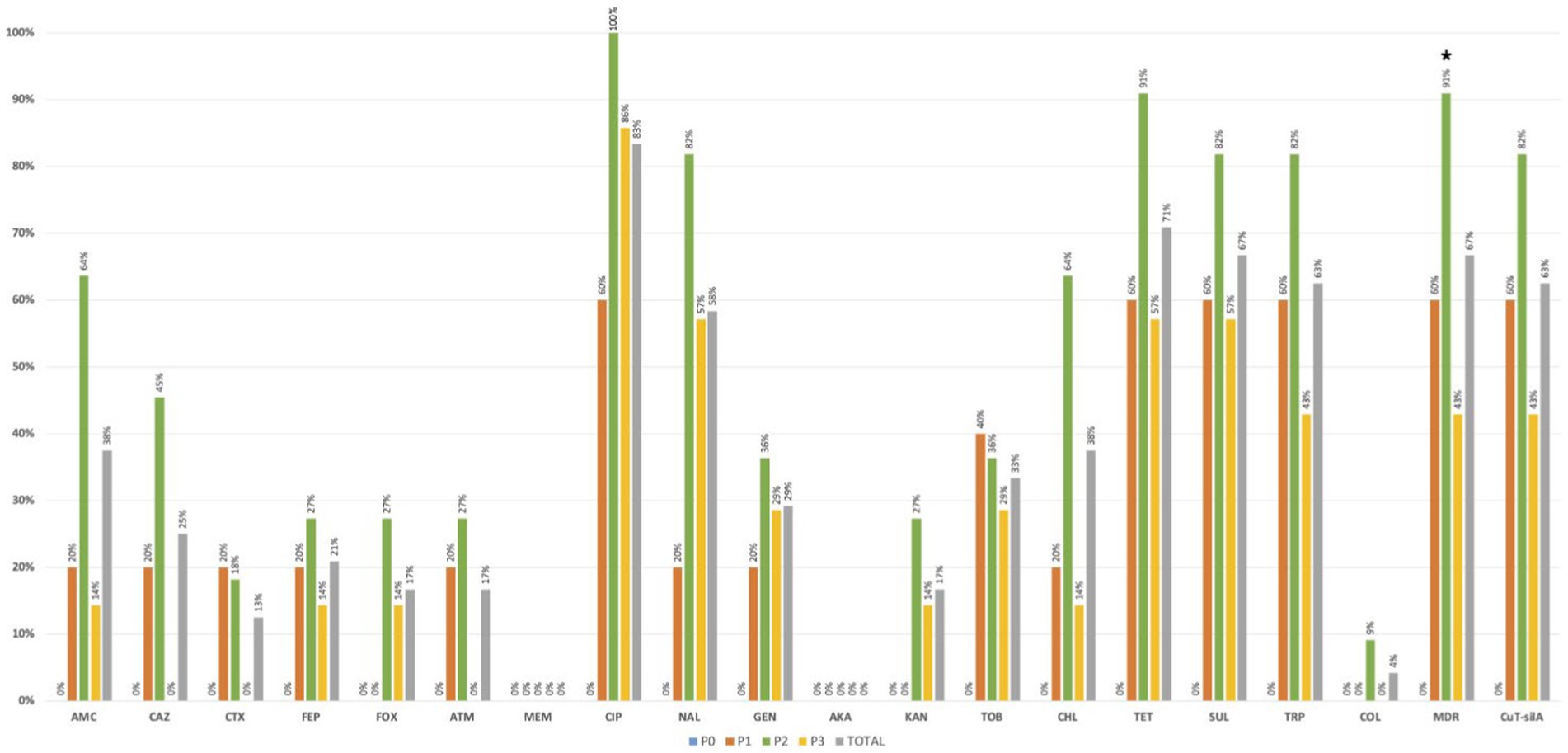
Figure 4. Occurrence of antibiotic-resistant Klebsiella pneumoniae in positive poultry samples at farm stages (P0, P1, and P2) and chicken meat (P3). *p < 0.05 (Fisher exact test). AMC, amoxicillin + clavulanic acid; CAZ, ceftazidime; CTX, cefotaxime; FEP, cefepime; FOX, cefoxitin; ATM, aztreonam; MEM, meropenem; CIP, ciprofloxacin; NAL, nalidixic acid; GEN, gentamicin; AKA, amikacin; KAN, kanamycin; TOB, tobramycin; CHL, chloramphenicol; TET, tetracycline; SUL, sulphonamides; TRP trimethoprim; COL, colistin; MDR, multidrug resistance; CuT-silA+, copper-tolerance silA gene.
3.4 Copper tolerance of Klebsiella pneumoniae recovered from poultry chain
The copper tolerance gene silA was observed in 52% (n = 51/99) of K. pneumoniae isolates from all farms and most poultry samples with K. pneumoniae (63%-n = 15/24), with similar rates between sampling stages (p > 0.05; Figure 4). More than 80% (n = 43/51) of silA-positive isolates were MDR (p < 0.05) and were also found to be more resistant to amoxicillin + clavulanic acid, ciprofloxacin, nalidixic acid, tetracycline, sulphonamides, gentamicin, and trimethoprim than the silA negative ones (p < 0.05; Figure 5). Copper susceptibility assays were performed in 53% (n = 52/99) of K. pneumoniae isolates carrying or not copper tolerance genes representative of different farms, flocks, KL-types, and antibiotic resistance profiles (Supplementary Table S1). Phenotypic results were congruent with the genotype since all isolates with MICCuSO4 ≥ 16 mM carried the silA gene whereas those with MICCuSO4 < 16 mM did not (Supplementary Table S1).
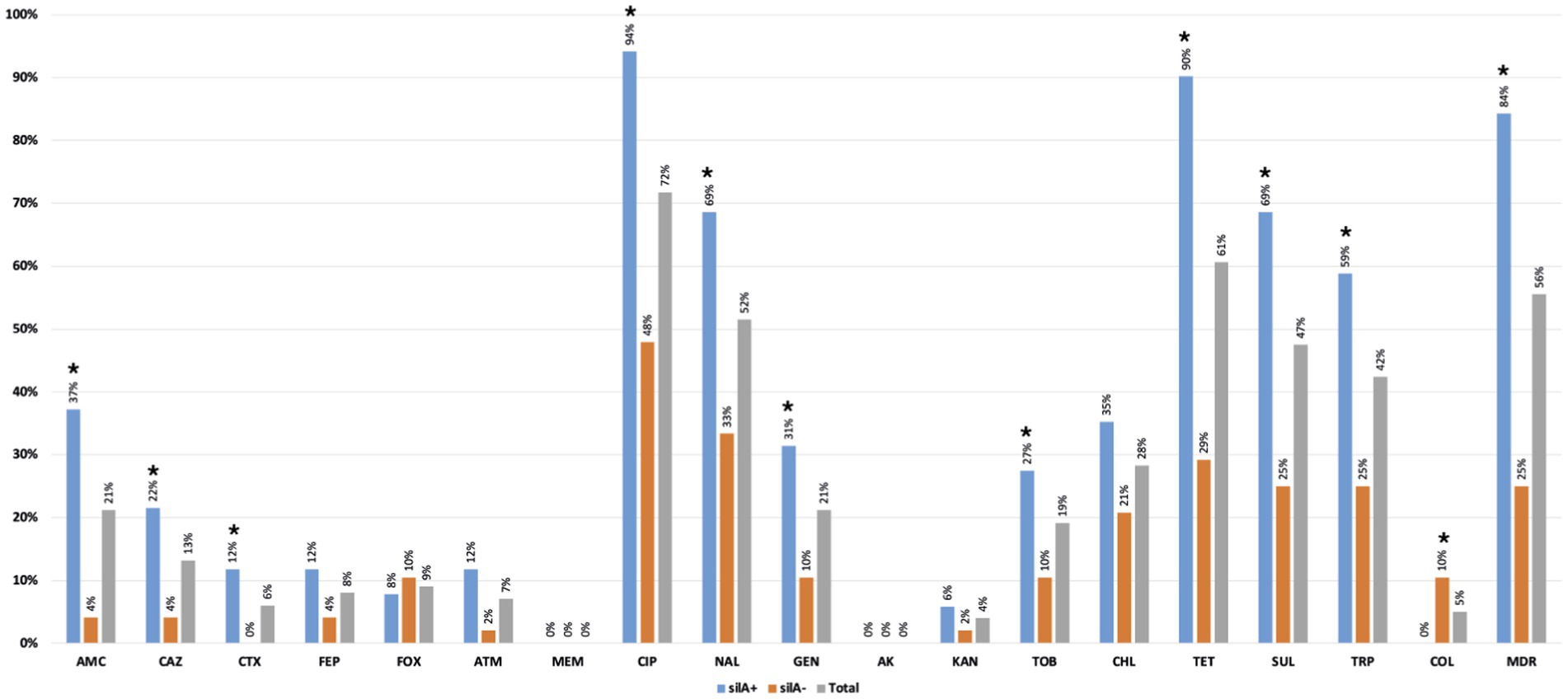
Figure 5. Percentage of antibiotic resistance detected among silA+ (n = 51) and silA− (n = 48) Klebsiella pneumoniae isolates. *p < 0.05 (Fisher exact test). AMC, amoxicillin + clavulanic acid; CAZ, ceftazidime; CTX, cefotaxime; FEP, cefepime; FOX, cefoxitin; ATM, aztreonam; MEM, meropenem; CIP, ciprofloxacin; NAL, nalidixic acid; GEN, gentamicin; AKA, amikacin; KAN, kanamycin; TOB, tobramycin; CHL, chloramphenicol; TET, tetracycline; SUL, sulphonamides; TRP trimethoprim; COL, colistin; MDR, multidrug resistance.
3.5 Benzalkonium chloride tolerance of Klebsiella pneumoniae recovered from poultry chain
The susceptibility to BZC was determined for 45 out of 99 K. pneumoniae isolates (all carrying genes previously associated with QAC tolerance), with diverse epidemiological and clonal backgrounds (Supplementary Table S2). The MICBZC ranged between 4 and 64 mg/L, with MIC50 = 16 mg/L and MIC90 = 32 mg/L. The highest MICBZC of 64 mg/L [non-wild-type using Epidemiological Cut-off-ECOFF of 32 mg/L proposed by Morrissey et al. (2014)] was observed in the ST280-KL23 lineage from a pre-slaughter chicken sample. Ten QACs’ genotypes (19 to 24 genes each) were identified (Figure 6). A variable occurrence of emrE (n = 1, 1 ST), qacF (n = 7, 5 STs), qacEΔ1 (n = 22, 14 STs); kexD (n = 37, 25 STs); oqxAB (n = 43, 30 STs) and kmrA (n = 44, 30 STs) was found, with the remaining genes detected in 100% of the genomes (Figure 6). However, no differences were observed between MIC distribution and clones, sources, MDR phenotype, or the presence or absence of genes previously associated with QAC tolerance, including qac genes (Figure 6; Supplementary Table S2). The only exception was the ST6552-KL109 isolate lacking kmrA, coding for an efflux pump of the Major Facilitator Superfamily, and presenting MICBZC of 4 mg/L. The MBCBZC for all tested isolates was the same as the MICBZC. Klebsiella pneumoniae showing the highest MBCBZC of 32–64 mg/L comprised isolates from diverse farms, sources, and clones, including one of the most prevalent in the present study (ST11-KL106, farm H).
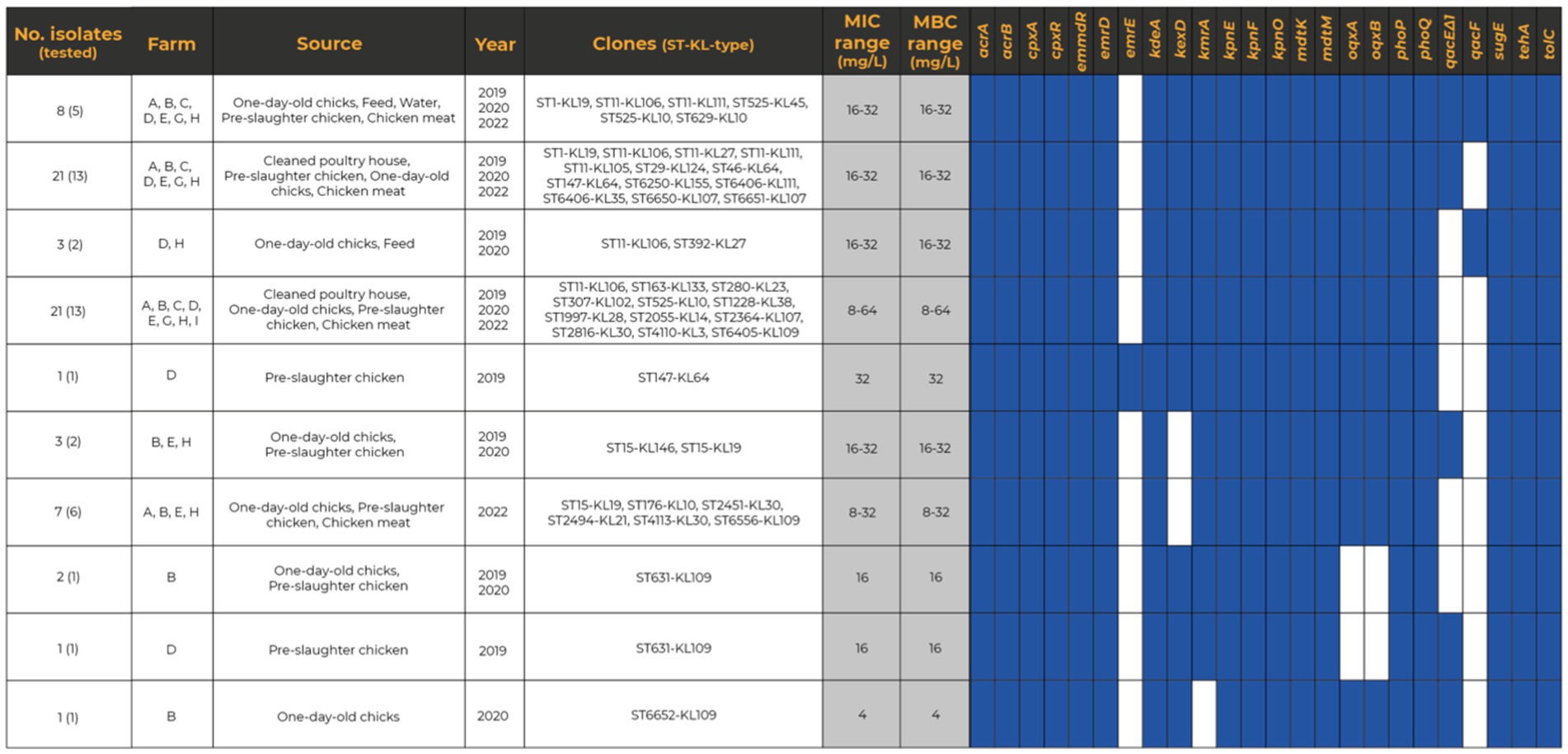
Figure 6. Heatmap representing the distribution of QAC tolerance genes across representative (n = 45) Klebsiella pneumoniae isolates. The presence of a specific QAC tolerance gene is indicated by a filled blue rectangle.
3.6 Whole-genome analysis of Klebsiella pneumoniae poultry-associated isolates
The genetic relatedness of 68 representative K. pneumoniae isolates revealed a clonal diverse population from 2019 to 2022 [this collection and (Mourão et al., 2023)]. Overall, the isolates were assigned to 31 STs (7 new STs) and 37 lineages, including the globally successful ST11-KL106, ST11-KL111, ST15-KL19, ST147-KL64, ST280-KL23, and ST307-KL102 (Figure 7; Supplementary Table S2). Based on the cgMLST analysis and the proposed thresholds (<10 allele differences), 15 clusters (differing by 0–207 SNPs) were identified among poultry K. pneumoniae (Figure 7; Supplementary Table S3). Those clusters included isolates from different sample types from the same farm: pre-slaughter chicken feces (ST15-KL19), water and chicken (ST11-KL106) pre-slaughter chicken feces and cleaned poultry houses (ST1228-KL38; ST6651-KL107), pre-slaughter chicken feces and derived meat (ST1-KL19; ST11-KL111; ST6405-KL109), or cleaned poultry-house and chicken meat (ST29-KL124). Furthermore, some lineages (e.g., ST631-KL109, ST6651-KL107, ST6406-CG147-KL111) were detected over time (Figure 7).
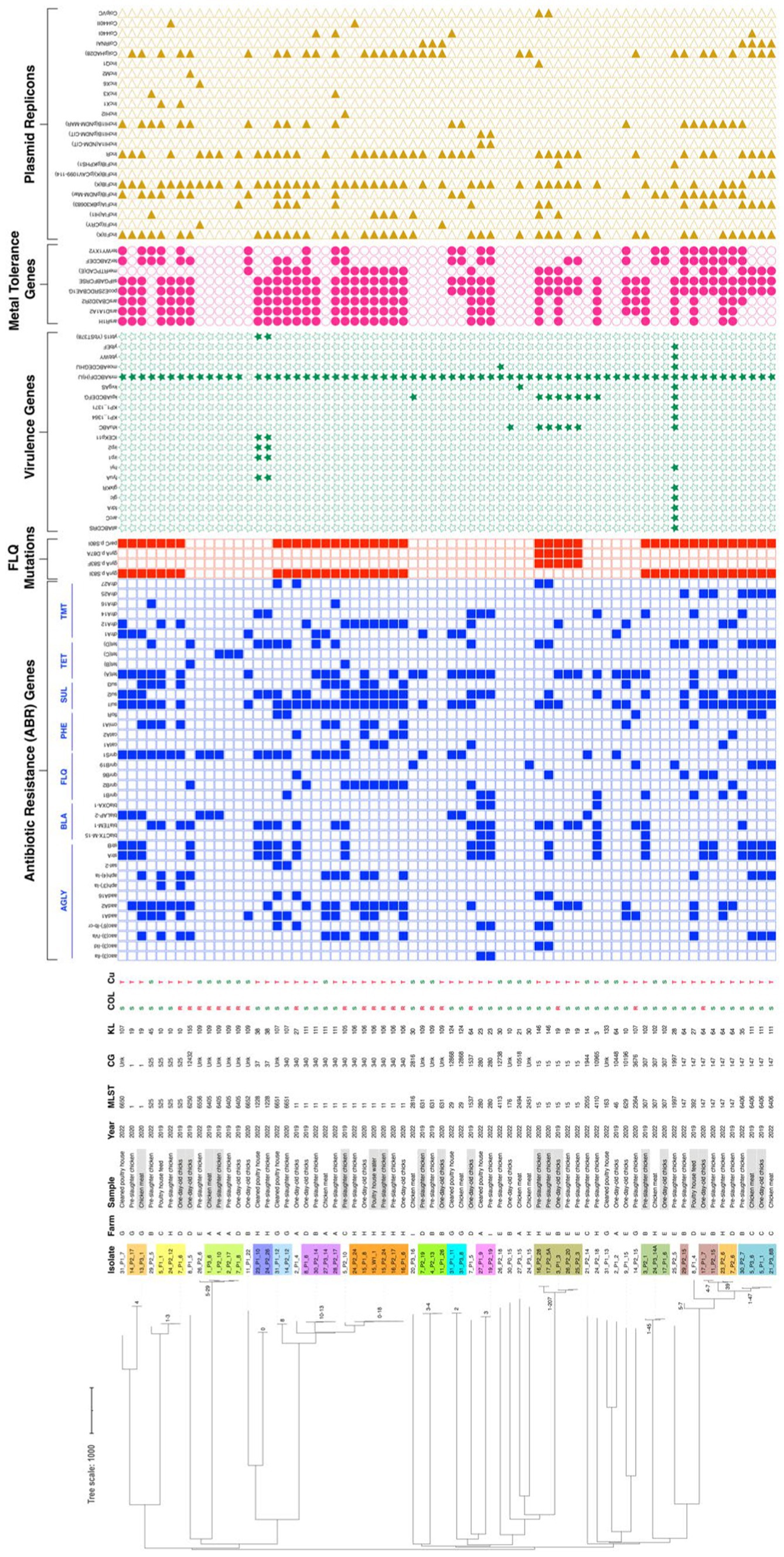
Figure 7. A neighbor-joining tree representing the phylogenetic relationships among the 68 Klebsiella pneumoniae genomes recovered between 2019 and 2022 was constructed from the Pathogenwatch pairwise-distance matrix [i.e., based on single nucleotide polymorphisms (SNPs) called in 1,972 core genes]. Scale bar units represent substitutions per variant site. The SNPs among our isolates from the 15 main clusters (<10 alleles difference) are represented in each branch. The isolates from samples exhibiting gray shading correspond to the ones already published by Mourão et al. (2023). The associated metadata for all isolates was added using iTOL (https://itol.embl.de/). Each colored-filled shape represents the presence of relevant antibiotic resistance, virulence-associated, metal-tolerance genes, and plasmid replicons associated with well-defined incompatibility groups. Only known mutations conferring fluoroquinolone resistance are presented. Klebsiella intrinsic antibiotic resistance (blaSHV-1, blaSHV-11, blaSHV-26, blaSHV-27, blaSHV-28, blaSHV-38, blaSHV-142, blaSHV-187, fosA, oxqAB) and metal tolerance (arsBCR, cusABFCRS) genes were not represented. AGLY, aminoglycosides; BLA, β-lactams; CG, Clonal Group; FLQ, fluoroquinolones; KL, K-Locus; MLST, Multilocus Sequence Typing; PHE, phenicols; SUL, sulphonamides; TET, tetracycline; TMT, trimethoprim; Unk, unknown.
To investigate the phylogenetic relationship of our genomes with others, we conducted a thorough analysis using Pathogenwatch, incorporating diverse sources, regions, and timeframes (Figure 8; Supplementary Figure S1). The analysis revealed less than 10 alleles differences in 18 lineages, with ST11-KL27, ST11-KL111, ST15-KL19, ST15-KL146, ST280-KL23, ST392-KL27, ST1537-KL64 and ST1997-KL28 with fewer than 21 SNPs (a threshold recently xx) proposed for K. pneumoniae transmission in healthcare settings; David et al., 2019) when compared to genomes from various sources (Figure 8). Most were linked to genomes from human infections in diverse EU countries, including Portugal (ST1997-KL28; Figure 8).
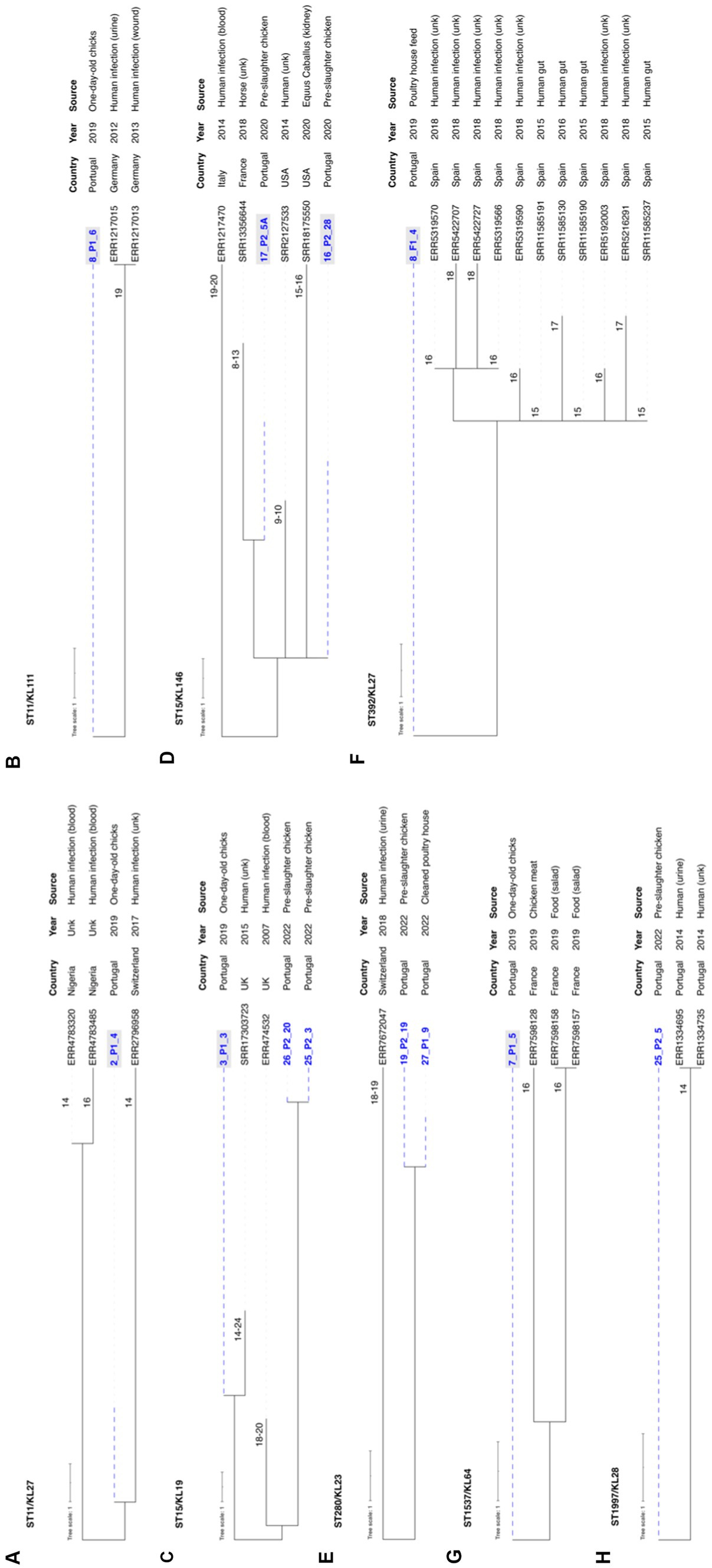
Figure 8. Neighbor-joining trees representing the phylogenetic relationships among the 68 Klebsiella pneumoniae genomes recovered between 2019 and 2022 and those available in Pathogenwatch with less than 21 SNPs. (A) ST11-KL27, (B) ST11-KL111, (C) ST15-KL19, (D) ST15-KL146, (E) ST392-KL27, (F) ST1537-KL64, (G) ST280-KL23, and (H) ST1997-KL28. The genome selection was performed using the cgMLST single linkage clustering to include the ones with less than 10 allele differences (threshold = 10)*. Then these genomes were used to infer a neighbor-joining tree from the Pathogenwatch pairwise-distance matrix (i.e., based on single nucleotide polymorphisms-SNPs called in 1,972 core genes). Scale bar units represent substitutions per variant site. The number of substitutions between our isolates and the ones available in Pathogenwatch are represented in each branch. All isolates’ associated metadata (country, collection date, and source of isolation) was added using iTOL (https://itol.embl.de/). *Klebsiella pneumoniae isolates from clonal lineages with <10 allele differences include: 2019–2020 collection—ST1-KL19, ST11-KL105, ST11-KL106, ST11-KL27, ST11-KL111, ST15-KL19, ST15-KL146, ST147-KL64, ST307-KL102, ST392-KL27, ST1537-KL64, and ST6250-KL155 (1); 2022 collection – ST11-KL106, ST11-KL111, ST15-KL19, ST147-KL64, ST280-KL23, ST307-KL102, ST525-KL45, ST1228-KL38, ST2055-KL14, ST1997-KL28, ST4110-KL3. The isolates exhibiting gray shading correspond to the ones already published by Mourão et al. (2023).
The WGS revealed a high load and diversity in antibiotic resistance genes between 2019 and 2022 (Figure 7; Supplementary Table S2). Thirty-eight acquired genes (7 classes) were detected, including clinically-relevant ones (blaCTX-M-15, qnrB, and qnrS). More than 75% (n = 51/68) of the genomes carried genes coding for ≥3 classes of antibiotics, with the most frequent including aminoglycosides (strA/strB/aadA), sulphonamides (sul1/sul2), tetracyclines (tet(A)/tet(D)), trimethoprim (dfrA) and phenicols (catA, cmlA; Figure 7; Supplementary Table S2). The most frequent determinant of resistance to ciprofloxacin was the presence of chromosomal mutations in the topoisomerase genes gyrA and parC (59%-n = 40/68) over 2019–2022. The ParC S80I mutation was present in all genomes, independently of the clonal lineage. The single GyrA S83I mutation was observed in all genomes except for ST15, which had two other simultaneous gyrA mutations (S83F and D87A). Furthermore, we noticed a decrease in isolates carrying chromosomal mutations implicated in colistin resistance between 2019 and 2020 (12 lineages) and 2022 (1 lineage ST6556/KL109; Figure 7; Supplementary Table S1). Concerning the virulence genes, all but one isolate carried the chromosomally encoded fimbriae cluster (mrkABCDFHIJ). The worldwide-disseminated high-risk clone ST15 isolates carried the ferric uptake (kfuABC) and the chaperone-usher pili systems (kpiABCDEFG) whereas ST1228 isolates the yersiniabactin siderophore (ybt15-YbST378/ICEKp11) in addition to the genes encoding for proteins required for yersiniabactin (irp1, irp2) and siderophores (fyuA gene; Figure 7).
3.7 Whole-genome analysis of metal tolerance gene’s occurrence and location
Metal tolerance genes were observed in the majority (75%-n = 51/68) of the sequenced K. pneumoniae isolates, specifically copper/silver (sil/pco), arsenic (ars), mercury (mer), and/or tellurite (ter; Figure 7). Notably, the most frequent sil and pco cluster (69%-n = 47/68) was frequently found alongside the ars (77%-n = 36/47), ter (51%-n = 24/47), and/or mer (49%-n = 23/47) operons. We detected, on average, four plasmids per genome (ranging from 1 to 10) and 25 distinct plasmid incompatibility groups (with 1–8 replicon types per genome; Supplementary Table S2). The most prevalent groups were IncFIBK (65%-n = 44/68), followed by IncFIIK (59%-n = 40/68), and IncR (56%-n = 38/68), often in different combinations with FIA, other FIB types (such as FIBpNDM-MAR, FIBpCAV1099, FIBpKPHS1), and/or HI1B (Figure 7; Supplementary Table S2). Col plasmids were also frequently observed (54%-n = 37/68 genomes), whereas other classical incompatibility groups (IncHI2, IncX, IncQ, IncM) were infrequent.
Copper tolerance genes sil and pco were plasmid located in all but one isolate (98%-n = 46/47) primarily within IncFIBK + IncFIIK plasmids (51%-n = 24/47, approximately 80–350 Kb) of different IncFIIK groups based on pMLST (2, 4, 5, 7, 8, 21-like). Additionally, copper tolerance genes were co-localized with variable genes coding for resistance to aminoglycosides (aac(3)-IIa, aac(3)-Iva, aac(6′)-Ib-cr, aph(4)-Ia, aadA1, aadA2, aadA16, strA-strB), fluoroquinolones (qnrB1, qnrB2, qnrB6, qnrS1), chloramphenicol (catA1, catA2, cmlA1), sulphonamides (sul1, sul2, sul3), tetracycline (tetA, tetD), trimethoprim (dfrA12, dfrA14, dfrA25, dfrA27) and β-lactams (blaTEM, blaCTX-M-15, blaOXA-1; Figure 7; Supplementary Table S4). Co-location with qac tolerance genes (qacEΔ1 and/or qacE, qacF) was also observed in six isolates. These plasmids carrying sil and pco were like others described in humans across multiple countries (Supplementary Table S4), occasionally harboring blaCTX-M-15, blaDHA-1, blaNDM-1, blaOXA-1, blaSHV-30, and/or qnr genes.
4 Discussion
This is a distinctive study that explores K. pneumoniae occurrence and diversity throughout the entire chicken farm-to-fork chain from a long-term perspective. It is also one of the few studies that offer evidence that poultry serves as a reservoir and source of K. pneumoniae strains with clinically relevant features, including genes coding for antibiotic resistance, metals, and/or biocide tolerance, while the identification of clones identical to those in clinical settings further supports K. pneumoniae as a foodborne pathogen.
Our data strongly emphasize that intensively farmed chicken production and their meat are relevant sources of K. pneumoniae and antibiotic-resistant isolates. This remains true even after EU veterinary antimicrobial sales decreased by 46.5% between 2011 and 2021 (European Medicines Agency, 2022). During the studied period (this study and Mourão et al., 2023), resistance remained high to antibiotics commonly used in poultry, such as tetracycline, sulphonamides, and/or quinolones (Alliance to Save our Antibiotics, 2016; Gržinić et al., 2023). However, resistance to colistin, a critically important antibiotic for treating human infections caused by Gram-negative MDR strains, has significantly decreased from 61% in 2019–2020 samples to 4% in 2022. This reduction suggests that the efforts of the long-term ban on colistin use in food-animal production (>4 years) have yielded promising results not only by limiting mcr (Ribeiro et al., 2021) but also other colistin-resistance genotypes (Mourão et al., 2023).
In conventional chicken production farms, diverse antimicrobial treatments are commonly administered throughout the fattening period, with differences by country and region (Joosten et al., 2019; Kasabova et al., 2021). In this study, all but one flock received antibiotics during the 26–43 days production cycle, which may explain the higher occurrence of MDR K. pneumoniae in pre-slaughter chickens compared to samples from previous stages. Furthermore, the contribution of poultry house management practices like inadequate disinfection or short vacancy periods between flocks, cannot be excluded (Daehre et al., 2018; Zhai et al., 2020; Franklin-Alming et al., 2021; Kaspersen et al., 2023). Even with standard cleaning and disinfection protocols across all the studied farms, a wide variety of K. pneumoniae strains, including MDR and clinically relevant lineages, can persist within the farm environment and/or until the meat becomes available to consumers. We found closely related MDR K. pneumoniae isolates in both cleaned poultry houses and pre-slaughter chickens (ST1228-KL38, ST29-KL24, ST280-KL23) from the same or different flocks or farms. The idea that strains re-introduction might come from parent flocks seems less likely since we observed minimal contamination (1 flock) during the reception of one-day-old chickens. This study also highlights water and feed as sources of MDR K. pneumoniae lineages (ST525-KL10, ST11-KL106), but at lower rates than chicken feces, as observed in Mourão et al. (2023). Noteworthy, some K. pneumoniae lineages (such as ST1-KL19, ST11-KL111, ST6405-KL109, and ST6406-CG147-KL111) persist throughout the chickens’ lifecycle until their meat becomes available to consumers. Additionally, cross-contamination at slaughterhouses is also plausible given the identification of K. pneumoniae lineages in chicken meat samples that were not traced back to their originating farm. The higher contamination compared with the previous study (43%–50% vs. 17%) and the diversity of K. pneumoniae strains (Mourão et al., 2023), might be attributed to the cultural methodology employed (antibiotic-free selection using SCAi medium with and without BPW enrichment). This approach was specifically designed to enhance the investigation of the inherent K. pneumoniae populations, as previously reported (Rodrigues et al., 2022). FT-IR proved to be a reliable approach to assessing the clonal relationship between K. pneumoniae isolates from poultry origin, being able to correctly identify closely related isolates (representing 70% of the sample) and KL-types (78% predicted by the machine-learning models) and discard unrelated isolates or KL-types not represented by the models used, as happened for isolates from human origin (Novais Â. et al., 2023). The use of this technique represented a useful tool to quickly identify common isolates from the same or different samples and choose representative isolates to sequence by WGS, reducing the cost and time associated with typing.
In our comparative genomic analysis using WGS, we identified closely related MDR K. pneumoniae lineages such as ST15-KL19, ST11-KL111, ST280-KL23, and ST1997-KL28 shared between poultry and human clinical isolates, even when applying strict criteria of less than 21 SNPs (David et al., 2019). Additionally, certain poultry strains shared a substantial repertoire of accessory genes, including fluoroquinolone resistance mutations and/or virulence genes with clinically relevant human clones (e.g., ST15, ST147, ST307) identified in previous studies (Peirano et al., 2020; Rodrigues et al., 2022, 2023). This data strengthens the argument for the possible transmission of these strains from food animals to humans (Büdel et al., 2020; Rodrigues et al., 2022; Thorpe et al., 2022; Crippa et al., 2023; Kaspersen et al., 2023; Zou et al., 2023), solidifying poultry’s position as both a reservoir and source of globally dispersed, clinically relevant K. pneumoniae lineages (Mourão et al., 2023).
The persistence of MDR K. pneumoniae lineages within the poultry chain (this study, Kaspersen et al., 2023; Mourão et al., 2023), suggests the presence of adaptive environmental factors beyond antibiotic resistance. We observed elevated rates of K. pneumoniae carrying sil and pco operons, along with a copper tolerance phenotype (>16 mM) in poultry samples. This suggests that the incorporation of copper-supplemented chicken feed might contribute to the selection of copper-tolerant and MDR strains within such production environments. However, earlier research, whether grounded in wet lab experiments or mathematical models, has indicated that the concentrations of copper necessary to foster the emergence of copper-tolerant bacteria might be significantly below their corresponding MIC values (Gullberg et al., 2014; Arya et al., 2021). Furthermore, the coexistence of various pollutants appears to further lower the minimum selective concentration estimates for individual antimicrobials (Gullberg et al., 2014; Arya et al., 2021). The sil and pco operons are often located on plasmids that carry an array of other metal, qac, metabolic, and/or specific antibiotic resistance genes, fostering their persistence within the environment through co-selection events driven by antimicrobial usage, coupled with the frequent presence of mechanisms that prevent plasmid loss.
This study also reveals a high abundance of QACs tolerance genes in K. pneumoniae lineages, although they do not appear to correlate with phenotype, as observed in other studies regardless of testing methods (Abuzaid et al., 2012; Morrissey et al., 2014; Wu et al., 2015; Vijayakumar et al., 2018; Gual-de-Torrella et al., 2022). Nevertheless, our WGS approach identified a wide range of QAC tolerance genes, underscoring the pressing need for establishing reliable genotypic-phenotypic correlations to elucidate QAC tolerance mechanisms (Hipólito et al., 2023). While several studies have reported that bacterial strains with an elevated MIC or MBC remained susceptible to the in-use BZC concentration (Vijayakumar et al., 2018; Maillard, 2022), it is essential to comprehend the environmental factors (e.g., temperature, pH) associated with the expression of these genes (Gual-de-Torrella et al., 2022; Pereira et al., 2023) to clarify the environmental, clinical, or veterinary/industrial implications of bacteria with a reduced biocide susceptibility. Further studies are needed to investigate the impact of inappropriate biocide usage or low concentrations, which can act as stressors without killing bacterial pathogens, potentially promoting antimicrobial resistance, and facilitating the transfer of antimicrobial resistance genes (Maillard, 2022; Maillard and Pascoe, 2023).
5 Conclusion
Our study reveals chicken production as a significant reservoir hosting a diverse range of clinically relevant K. pneumoniae clones, including MDR, copper-tolerant and enriched in QAC tolerance genes. The identification of clones identical to those in clinical settings supports K. pneumoniae as a foodborne pathogen. Various sources of contamination (such as feed, water, poultry houses, and cross-contamination) contribute to the persistence of K. pneumoniae throughout the production chain, emphasizing that, despite a decrease in its occurrence, certain clones still reach chicken meat even with implemented safety measures in place.
The co-occurrence of copper and/or QAC tolerance genes on highly prevalent MDR plasmids suggests that these have been circulating in various K. pneumoniae populations and phenotypic validation (at least in the case of copper) supports the possibility that these genes may play a role in the co-selection of these plasmids or strains under certain conditions within the food production chain or other environmental settings.
Further studies are needed to assess the implications of these K. pneumoniae lineages on food safety and the risk of transmitting antibiotic resistance to humans. Additional studies are imperative to elucidate the external factors (such as environmental conditions) that drive K. pneumoniae’s adaptation toward antimicrobial resistance. Addressing these complexities can contribute to the development of effective strategies to safeguard animal welfare, enhance food safety, and mitigate public health risks associated with clinically-relevant K. pneumoniae lineages and antibiotic resistance.
Author’s note
Joana Mourão, Andreia Rebelo, Carla Novais, Luísa Peixe, and Patrícia Antunes are active members of the European Society of Clinical Microbiology and Infectious Diseases (ESCMID) Food and Water-borne Infection Study Group (EFWISG) and Ângela Novais of ESCMID Study Group for Epidemiological Markers (ESGEM).
Data availability statement
The datasets presented in this study can be found in online repositories. The names of the repository/repositories and accession number(s) can be found at: https://www.ebi.ac.uk/ena, PRJEB62836.
Ethics statement
Ethical review and approval were not required because the samples were taken from farm environmental points (e.g., boxes, floor) as well as after routine slaughter, under the auspices of the poultry farm company, which includes oversight by the veterinary team.
Author contributions
JM: Data curation, Formal analysis, Investigation, Methodology, Software, Visualization, Writing – original draft, Writing – review & editing. MM: Investigation, Methodology, Writing – review & editing. MR-A: Formal analysis, Investigation, Methodology, Writing – review & editing. AR: Investigation, Visualization, Writing – review & editing. CN: Conceptualization, Formal analysis, Funding acquisition, Investigation, Writing – review & editing. LP: Funding acquisition, Writing – review & editing. ÂN: Conceptualization, Formal analysis, Methodology, Writing – review & editing. PA: Conceptualization, Formal analysis, Funding acquisition, Investigation, Methodology, Project administration, Software, Supervision, Writing – original draft, Writing – review & editing.
Funding
The author(s) declare financial support was received for the research, authorship, and/or publication of this article. This work was supported by the Applied Molecular Biosciences Unit—UCIBIO which is financed by national funds from FCT—Fundação para a Ciência e a Tecnologia (UIDP/04378/2020 and UIDB/04378/2020), by the Associate Laboratory Institute for Health and Bioeconomy-i4HB (LA/P/0140/2020), by the AgriFood XXI I&D&I project (NORTE-01-0145-FEDER-000041) co-financed by the European Regional Development Fund (ERDF) through NORTE 2020 (Programa Operacional Regional do Norte 2014/2020) and by the University of Porto and Soja de Portugal (grant no IJUP-Empresas-2021-14). JM and ÂN were supported by national funds through FCT/MCTES in the context of the Scientific Employment Stimulus (2021.03416.CEECIND and 2021.02252.CEECIND, respectively). MR-A and AR were supported by PhD fellowships from FCT (SFRH/BD/146405/2019 and SFRH/BD/137100/2018, respectively). The authors are greatly indebted to all the financing sources.
Acknowledgments
The authors thank the Institut Pasteur teams for the curation and maintenance of BIGSdb-Pasteur databases at http://bigsdb.pasteur.fr/. We thank Sofia Ribeiro for the Figure 1 design, the students António Magalhães (FCNAUP), Francisca Pereira (FCNAUP), and Cátia Matos (FFUP) for technical support, and the staff of the participating farms and slaughterhouses for their kind cooperation.
Conflict of interest
The authors declare that the research was conducted in the absence of any commercial or financial relationships that could be construed as a potential conflict of interest.
The author(s) declared that they were an editorial board member of Frontiers, at the time of submission. This had no impact on the peer review process and the final decision.
Publisher’s note
All claims expressed in this article are solely those of the authors and do not necessarily represent those of their affiliated organizations, or those of the publisher, the editors and the reviewers. Any product that may be evaluated in this article, or claim that may be made by its manufacturer, is not guaranteed or endorsed by the publisher.
Supplementary material
The Supplementary material for this article can be found online at: https://www.frontiersin.org/articles/10.3389/fmicb.2024.1365011/full#supplementary-material
Footnotes
1. ^ https://www.eurofinsgenomics.eu/
2. ^ http://bigsdb.pasteur.fr/klebsiella/klebsiella.html
3. ^ http://sourceforge.net/projects/bbmap/
5. ^ https://github.com/tseemann/abricate
6. ^ https://bigsdb.pasteur.fr/klebsiella/
References
Abuzaid, A., Hamouda, A., and Amyes, S. G. B. (2012). Klebsiella pneumoniae susceptibility to biocides and its association with cepA, qacΔE and qacE efflux pump genes and antibiotic resistance. J. Hosp. Infect. 81, 87–91. doi: 10.1016/j.jhin.2012.03.003
ADAS (2016). Comparison of the regulatory framework and key practices in the poultry meat supply chain in the EU and USA. Association of Poultry Processors and Poultry Trade in the EU Countries Available at: https://britishpoultry.org.uk/identity-cms/wp-content/uploads/2018/05/2016-ADAS-EU-US-comparison.pdf.
Alliance to Save our Antibiotics (2016). Antibiotic use in the UK poultry sector. Available at: https://www.saveourantibiotics.org/media/1763/antibiotic-use-in-the-uk-poultry-sector.pdf.
Andrews, S. (2010). FastQC: A Quality Control Tool for High Throughput Sequence Data [Online]. Available online at: http://www.bioinformatics.babraham.ac.uk/projects/fastqc/.
Argimón, S., David, S., Underwood, A., Abrudan, M., Wheeler, N. E., Kekre, M., et al. (2021). Rapid genomic characterization and global surveillance of Klebsiella using Pathogenwatch. Clin. Infect. Dis. 73, S325–S335. doi: 10.1093/cid/ciab784
Arya, S., Williams, A., Reina, S. V., Knapp, C. W., Kreft, J.-U., Hobman, J. L., et al. (2021). Towards a general model for predicting minimal metal concentrations co-selecting for antibiotic resistance plasmids. Environ. Pollut. 275:116602. doi: 10.1016/j.envpol.2021.116602
Aziz, R. K., Bartels, D., Best, A. A., DeJongh, M., Disz, T., Edwards, R. A., et al. (2008). The RAST server: rapid annotations using subsystems technology. BMC Genomics 9:75. doi: 10.1186/1471-2164-9-75
Bankevich, A., Nurk, S., Antipov, D., Gurevich, A. A., Dvorkin, M., Kulikov, A. S., et al. (2012). SPAdes: a new genome assembly algorithm and its applications to single-cell sequencing. J. Comput. Biol. 19, 455–477. doi: 10.1089/cmb.2012.0021
Bialek-Davenet, S., Criscuolo, A., Ailloud, F., Passet, V., Nicolas-Chanoine, M.-H., Decré, D., et al. (2014). Development of a multiplex PCR assay for identification of Klebsiella pneumoniae hypervirulent clones of capsular serotype K2. J. Med. Microbiol. 63, 1608–1614. doi: 10.1099/jmm.0.081448-0
Borowiak, M., Baumann, B., Fischer, J., Thomas, K., Deneke, C., Hammerl, J. A., et al. (2020). Development of a novel mcr-6 to mcr-9 multiplex PCR and assessment of mcr-1 to mcr-9 occurrence in Colistin-resistant Salmonella enterica isolates from environment, feed, animals and food (2011–2018) in Germany. Front. Microbiol. 11:80. doi: 10.3389/fmicb.2020.00080
Brisse, S., Passet, V., Haugaard, A. B., Babosan, A., Kassis-Chikhani, N., Struve, C., et al. (2013). Wzi gene sequencing, a rapid method for determination of capsular type for Klebsiella strains. J. Clin. Microbiol. 51, 4073–4078. doi: 10.1128/JCM.01924-13
Broom, L. J., Monteiro, A., and Piñon, A. (2021). Recent advances in understanding the influence of zinc, copper, and manganese on the gastrointestinal environment of pigs and poultry. Animals 11:1276. doi: 10.3390/ani11051276
Büdel, T., Kuenzli, E., Campos-Madueno, E. I., Mohammed, A. H., Hassan, N. K., Zinsstag, J., et al. (2020). On the island of Zanzibar people in the community are frequently colonized with the same MDR Enterobacterales found in poultry and retailed chicken meat. J. Antimicrob. Chemother. 75, 2432–2441. doi: 10.1093/jac/dkaa198
Camacho, C., Coulouris, G., Avagyan, V., Ma, N., Papadopoulos, J., Bealer, K., et al. (2009). BLAST+: architecture and applications. BMC Bioinformatics 10:421. doi: 10.1186/1471-2105-10-421
Carattoli, A., Zankari, E., García-Fernández, A., Voldby Larsen, M., Lund, O., Villa, L., et al. (2014). In silico detection and typing of plasmids using PlasmidFinder and plasmid multilocus sequence typing. Antimicrob. Agents Chemother. 58, 3895–3903. doi: 10.1128/AAC.02412-14
Chen, S., Fu, J., Zhao, K., Yang, S., Li, C., Penttinen, P., et al. (2023). Class 1 integron carrying qacEΔ1 gene confers resistance to disinfectant and antibiotics in Salmonella. Int. J. Food Microbiol. 404:110319. doi: 10.1016/j.ijfoodmicro.2023.110319
Clinical and Laboratory Standards Institute (1999). Methods for determining bactericidal activity of antimicrobial agents, CLSI document M26-a. Wayne, PA: CLSI.
Clinical and Laboratory Standards Institute (2018). Methods for dilution antimicrobial susceptibility tests for Bacteria that grow aerobically, 11th ed. CLSI document M07. Wayne, PA: CLSI.
Clinical and Laboratory Standards Institute (2022). “Performance standards for antimicrobial susceptibility testing” in CLSI Document M100. 32nd ed (Wayne, PA: CLSI)
Crippa, C., Pasquali, F., Rodrigues, C., De Cesare, A., Lucchi, A., Gambi, L., et al. (2023). Genomic features of Klebsiella isolates from artisanal ready-to-eat food production facilities. Sci. Rep. 13:10957. doi: 10.1038/s41598-023-37821-7
Daehre, K., Projahn, M., Friese, A., Semmler, T., Guenther, S., and Roesler, U. H. (2018). ESBL-producing Klebsiella pneumoniae in the broiler production chain and the first description of ST3128. Front. Microbiol. 9:2302. doi: 10.3389/fmicb.2018.02302
David, S., Reuter, S., Harris, S. R., Glasner, C., Feltwell, T., Argimon, S., et al. (2019). Epidemic of carbapenem-resistant Klebsiella pneumoniae in Europe is driven by nosocomial spread. Nat. Microbiol. 4, 1919–1929. doi: 10.1038/s41564-019-0492-8
Diancourt, L., Passet, V., Verhoef, J., Grimont, P. A. D., and Brisse, S. (2005). Multilocus sequence typing of Klebsiella pneumoniae nosocomial isolates. J. Clin. Microbiol. 43, 4178–4182. doi: 10.1128/JCM.43.8.4178-4182.2005
El Sabry, M. I., Stino, F. K. R., and El-Ghany, W. A. A. (2021). Copper: benefits and risks for poultry, livestock, and fish production. Tropl. Anim. Health Prod. 53:487. doi: 10.1007/s11250-021-02915-9
EMA Committee for Medicinal Products for Veterinary Use (CVMP) and EFSA Panel on Biological Hazards (BIOHAZ)Murphy, D., Ricci, A., Auce, Z., Beechinor, J. G., Bergendahl, H., et al. (2017). EMA and EFSA joint scientific opinion on measures to reduce the need to use antimicrobial agents in animal husbandry in the European Union, and the resulting impacts on food safety (RONAFA). EFSA J. 15:e04666. doi: 10.2903/j.efsa.2017.4666
European Committee for Antimicrobial Susceptibility Testing (2016). Recommendations for MIC determination of colistin (polymyxin E)—as recommended by the joint CLSI-EUCAST Polymyxin Breakpoints Working Group. Available at: https://www.eucast.org/fileadmin/src/media/PDFs/EUCAST_files/General_documents/Recommendations_for_MIC_determination_of_colistin_March_2016.pdf.
European Committee on Antimicrobial Susceptibility Testing (2022). Breakpoint tables for interpretation of MICs and zone diameters, version 12.0. Available at: https://www.eucast.org/fileadmin/src/media/PDFs/EUCAST_files/Breakpoint_tables/v_12.0_Breakpoint_Tables.pdf.
European Food Safety Authority (EFSA) and European Centre for Disease Prevention and Control (ECDC) (2023). The European Union summary report on antimicrobial resistance in zoonotic and indicator bacteria from humans, animals and food in 2020/2021. EFSA J. 21:e07867. doi: 10.2903/j.efsa.2023.7867
European Medicines Agency (2022). Sales of veterinary antimicrobial agents in 31 European countries in 2021: Trends from 2010 to 2021: Twelfth ESVAC report. LU: Publications Office Available at: https://data.europa.eu/doi/10.2809/39517 (Accessed 11 October 2023).
Ewels, P., Magnusson, M., Lundin, S., and Käller, M. (2016). MultiQC: summarize analysis results for multiple tools and samples in a single report. Bioinformatics 32, 3047–3048. doi: 10.1093/bioinformatics/btw354
Feldgarden, M., Brover, V., Gonzalez-Escalona, N., Frye, J. G., Haendiges, J., Haft, D. H., et al. (2021). AMRFinderPlus and the reference gene catalog facilitate examination of the genomic links among antimicrobial resistance, stress response, and virulence. Sci. Rep. 11:12728. doi: 10.1038/s41598-021-91456-0
Franklin-Alming, F. V., Kaspersen, H., Hetland, M. A. K., Bakksjø, R.-J., Nesse, L. L., Leangapichart, T., et al. (2021). Exploring Klebsiella pneumoniae in healthy poultry reveals high genetic diversity, good biofilm-forming abilities and higher prevalence in turkeys than broilers. Front. Microbiol. 12:725414. doi: 10.3389/fmicb.2021.725414
Gato, E., Vázquez-Ucha, J. C., Rumbo-Feal, S., Álvarez-Fraga, L., Vallejo, J. A., Martínez-Guitián, M., et al. (2020). Kpi, a chaperone-usher pili system associated with the worldwide-disseminated high-risk clone Klebsiella pneumoniae ST-15. Proc. Natl. Acad. Sci. 117, 17249–17259. doi: 10.1073/pnas.1921393117
Golden, C. E., Rothrock, M. J., and Mishra, A. (2021). Mapping foodborne pathogen contamination throughout the conventional and alternative poultry supply chains. Poult. Sci. 100:101157. doi: 10.1016/j.psj.2021.101157
Gržinić, G., Piotrowicz-Cieślak, A., Klimkowicz-Pawlas, A., Górny, R. L., Ławniczek-Wałczyk, A., Piechowicz, L., et al. (2023). Intensive poultry farming: a review of the impact on the environment and human health. Sci. Total Environ. 858:160014. doi: 10.1016/j.scitotenv.2022.160014
Gual-de-Torrella, A., Delgado-Valverde, M., Pérez-Palacios, P., Oteo-Iglesias, J., Pascual, Á., and Fernández-Cuenca, F. (2022). In vitro activity of six biocides against carbapenemase-producing Klebsiella pneumoniae and presence of genes encoding efflux pumps. Enfermedades Infec. Microbiol. Clínica 40, 371–376. doi: 10.1016/j.eimc.2021.05.004
Gullberg, E., Albrecht, L. M., Karlsson, C., Sandegren, L., and Andersson, D. I. (2014). Selection of a multidrug resistance plasmid by sublethal levels of antibiotics and heavy metals. MBio 5, e01918–e01914. doi: 10.1128/mBio.01918-14
Gurevich, A., Saveliev, V., Vyahhi, N., and Tesler, G. (2013). QUAST: quality assessment tool for genome assemblies. Bioinformatics 29, 1072–1075. doi: 10.1093/bioinformatics/btt086
Hipólito, A., García-Pastor, L., Vergara, E., Jové, T., and Escudero, J. A. (2023). Profile and resistance levels of 136 integron resistance genes. NPJ Antimicrob. Resist. 1:13. doi: 10.1038/s44259-023-00014-3
Joosten, P., Sarrazin, S., Van Gompel, L., Luiken, R. E. C., Mevius, D. J., Wagenaar, J. A., et al. (2019). Quantitative and qualitative analysis of antimicrobial usage at farm and flock level on 181 broiler farms in nine European countries. J. Antimicrob. Chemother. 74, 798–806. doi: 10.1093/jac/dky498
Kampf, G. (2018). Biocidal agents used for disinfection can enhance antibiotic resistance in gram-negative species. Antibiotics 7:110. doi: 10.3390/antibiotics7040110
Karcher, D. M., and Mench, J. A. (2018). “Overview of commercial poultry production systems and their main welfare challenges” in Advances in poultry welfare. ed. J. A. Mench (Elsevier: Woodhead Publishing), 3–25.
Kasabova, S., Hartmann, M., Freise, F., Hommerich, K., Fischer, S., Wilms-Schulze-Kump, A., et al. (2021). Antibiotic usage pattern in broiler chicken flocks in Germany. Front. Vet. Sci. 8:673809. doi: 10.3389/fvets.2021.673809
Kaspersen, H., Urdahl, A. M., Franklin-Alming, F. V., Ilag, H. K., Hetland, M. A. K., Bernhoff, E., et al. (2023). Population dynamics and characteristics of Klebsiella pneumoniae from healthy poultry in Norway. Front. Microbiol. 14:1193274. doi: 10.3389/fmicb.2023.1193274
Lam, M. M. C., Wick, R. R., Watts, S. C., Cerdeira, L. T., Wyres, K. L., and Holt, K. E. (2021). A genomic surveillance framework and genotyping tool for Klebsiella pneumoniae and its related species complex. Nat. Commun. 12:4188. doi: 10.1038/s41467-021-24448-3
Letunic, I., and Bork, P. (2021). Interactive tree of life (iTOL) v5: an online tool for phylogenetic tree display and annotation. Nucleic Acids Res. 49, W293–W296. doi: 10.1093/nar/gkab301
Li, X., Rensing, C., Vestergaard, G., Arumugam, M., Nesme, J., Gupta, S., et al. (2022). Metagenomic evidence for co-occurrence of antibiotic, biocide and metal resistance genes in pigs. Environ. Int. 158:106899. doi: 10.1016/j.envint.2021.106899
Maillard, J.-Y. (2022). Impact of benzalkonium chloride, benzethonium chloride and chloroxylenol on bacterial antimicrobial resistance. J. Appl. Microbiol. 133, 3322–3346. doi: 10.1111/jam.15739
Maillard, J.-Y., and Pascoe, M. (2023). Disinfectants and antiseptics: mechanisms of action and resistance. Nat. Rev. Microbiol. 22, 4–17. doi: 10.1038/s41579-023-00958-3
Morrissey, I., Oggioni, M. R., Knight, D., Curiao, T., Coque, T., Kalkanci, A., et al. (2014). Evaluation of epidemiological cut-off values indicates that biocide resistant subpopulations are uncommon in natural isolates of clinically-relevant microorganisms. PLoS One 9:e86669. doi: 10.1371/journal.pone.0086669
Mottet, A., and Tempio, G. (2017). Global poultry production: current state and future outlook and challenges. Worlds Poult. Sci. J. 73, 245–256. doi: 10.1017/S0043933917000071
Mourão, J., Marçal, S., Ramos, P., Campos, J., Machado, J., Peixe, L., et al. (2016). Tolerance to multiple metal stressors in emerging non-typhoidal MDR Salmonella serotypes: a relevant role for copper in anaerobic conditions. J. Antimicrob. Chemother. 71, 2147–2157. doi: 10.1093/jac/dkw120
Mourão, J., Ribeiro-Almeida, M., Novais, C., Magalhães, M., Rebelo, A., Ribeiro, S., et al. (2023). From farm to fork: persistence of clinically relevant multidrug-resistant and copper-tolerant Klebsiella pneumoniae Long after Colistin withdrawal in poultry production. Microbiol. Spectr. 11:e0138623. doi: 10.1128/spectrum.01386-23
Novais, C., Almeida-Santos, A. C., Paula Pereira, A., Rebelo, A., Freitas, A. R., and Peixe, L. (2023). Alert for molecular data interpretation when using Enterococcus faecium reference strains reclassified as Enterococcus lactis. Gene 851:146951. doi: 10.1016/j.gene.2022.146951
Novais, Â., Gonçalves, A. B., Ribeiro, T. G., Freitas, A. R., Méndez, G., Mancera, L., et al. (2023). Development and validation of a quick, automated and reproducible ATR FT-IR spectroscopy machine-learning model for Klebsiella pneumoniae typing. J. Clin. Microbiol. 62:e0121123. doi: 10.1128/jcm.01211-23
Parks, D. H., Imelfort, M., Skennerton, C. T., Hugenholtz, P., and Tyson, G. W. (2015). CheckM: assessing the quality of microbial genomes recovered from isolates, single cells, and metagenomes. Genome Res. 25, 1043–1055. doi: 10.1101/gr.186072.114
Peirano, G., Chen, L., Kreiswirth, B. N., and Pitout, J. D. D. (2020). Emerging antimicrobial-resistant high-risk Klebsiella pneumoniae clones ST307 and ST147. Antimicrob. Agents Chemother. 64, e01148–e01120. doi: 10.1128/AAC.01148-20
Pereira, A. P., Antunes, P., Bierge, P., Willems, R. J. L., Corander, J., Coque, T. M., et al. (2023). Unraveling Enterococcus susceptibility to quaternary ammonium compounds: genes, phenotypes, and the impact of environmental conditions. Microbiol. Spectr. 11, e02324–e02323. doi: 10.1128/spectrum.02324-23
Rebelo, A., Almeida, A., Peixe, L., Antunes, P., and Novais, C. (2023). Unraveling the role of metals and organic acids in bacterial antimicrobial resistance in the food chain. Antibiotics 12:1474. doi: 10.3390/antibiotics12091474
Rebelo, A. R., Bortolaia, V., Kjeldgaard, J. S., Pedersen, S. K., Leekitcharoenphon, P., Hansen, I. M., et al. (2018). Multiplex PCR for detection of plasmid-mediated colistin resistance determinants, mcr-1, mcr-2, mcr-3, mcr-4 and mcr-5 for surveillance purposes. Eur. Secur. 23:672. doi: 10.2807/1560-7917.ES.2018.23.6.17-00672
Ribeiro, S., Mourão, J., Novais, Â., Campos, J., Peixe, L., and Antunes, P. (2021). From farm to fork: Colistin voluntary withdrawal in Portuguese farms reflected in decreasing occurrence of mcr-1-carrying Enterobacteriaceae from chicken meat. Environ. Microbiol. 23, 7563–7577. doi: 10.1111/1462-2920.15689
Robertson, J., Bessonov, K., Schonfeld, J., and Nash, J. H. E. (2020). Universal whole-sequence-based plasmid typing and its utility to prediction of host range and epidemiological surveillance. Microb. Genomics 6:435. doi: 10.1099/mgen.0.000435
Robertson, J., and Nash, J. H. E. (2018). MOB-suite: software tools for clustering, reconstruction and typing of plasmids from draft assemblies. Microb. Genomics 4:206. doi: 10.1099/mgen.0.000206
Rodrigues, C., Hauser, K., Cahill, N., Ligowska-Marzęta, M., Centorotola, G., Cornacchia, A., et al. (2022). High prevalence of Klebsiella pneumoniae in European food products: a multicentric study comparing culture and molecular detection methods. Microbiol. Spectr. 10:e0237621. doi: 10.1128/spectrum.02376-21
Rodrigues, C., Lanza, V. F., Peixe, L., Coque, T. M., and Novais, Â. (2023). Phylogenomics of globally spread clonal groups 14 and 15 of Klebsiella pneumoniae. Microbiol. Spectr. 11:e0339522. doi: 10.1128/spectrum.03395-22
Simão, F. A., Waterhouse, R. M., Ioannidis, P., Kriventseva, E. V., and Zdobnov, E. M. (2015). BUSCO: assessing genome assembly and annotation completeness with single-copy orthologs. Bioinformatics 31, 3210–3212. doi: 10.1093/bioinformatics/btv351
Slifierz, M. J., Friendship, R. M., and Weese, J. S. (2015). Methicillin-resistant Staphylococcus aureus in commercial swine herds is associated with disinfectant and zinc usage. Appl. Environ. Microbiol. 81, 2690–2695. doi: 10.1128/AEM.00036-15
Thorpe, H. A., Booton, R., Kallonen, T., Gibbon, M. J., Couto, N., Passet, V., et al. (2022). A large-scale genomic snapshot of Klebsiella spp. isolates in northern Italy reveals limited transmission between clinical and non-clinical settings. Nat. Microbiol. 7, 2054–2067. doi: 10.1038/s41564-022-01263-0
Vijayakumar, R., Sandle, T., Al-Aboody, M. S., AlFonaisan, M. K., Alturaiki, W., Mickymaray, S., et al. (2018). Distribution of biocide resistant genes and biocides susceptibility in multidrug-resistant Klebsiella pneumoniae, Pseudomonas aeruginosa and Acinetobacter baumannii — a first report from the Kingdom of Saudi Arabia. J. Infect. Public Health 11, 812–816. doi: 10.1016/j.jiph.2018.05.011
Villa, L., García-Fernández, A., Fortini, D., and Carattoli, A. (2010). Replicon sequence typing of IncF plasmids carrying virulence and resistance determinants. J. Antimicrob. Chemother. 65, 2518–2529. doi: 10.1093/jac/dkq347
Webber, M. A., Whitehead, R. N., Mount, M., Loman, N. J., Pallen, M. J., and Piddock, L. J. V. (2015). Parallel evolutionary pathways to antibiotic resistance selected by biocide exposure. J. Antimicrob. Chemother. 70, 2241–2248. doi: 10.1093/jac/dkv109
Wick, R. R., Judd, L. M., Gorrie, C. L., and Holt, K. E. (2017). Unicycler: resolving bacterial genome assemblies from short and long sequencing reads. PLoS Comput. Biol. 13:e1005595. doi: 10.1371/journal.pcbi.1005595
Wu, G., Yang, Q., Long, M., Guo, L., Li, B., Meng, Y., et al. (2015). Evaluation of agar dilution and broth microdilution methods to determine the disinfectant susceptibility. J. Antibiot. 68, 661–665. doi: 10.1038/ja.2015.51
Wyres, K. L., and Holt, K. E. (2016). Klebsiella pneumoniae population genomics and antimicrobial-resistant clones. Trends Microbiol. 24, 944–956. doi: 10.1016/j.tim.2016.09.007
Wyres, K. L., and Holt, K. E. (2018). Klebsiella pneumoniae as a key trafficker of drug resistance genes from environmental to clinically important bacteria. Curr. Opin. Microbiol. 45, 131–139. doi: 10.1016/j.mib.2018.04.004
Zhai, R., Fu, B., Shi, X., Sun, C., Liu, Z., Wang, S., et al. (2020). Contaminated in-house environment contributes to the persistence and transmission of NDM-producing bacteria in a Chinese poultry farm. Environ. Int. 139:105715. doi: 10.1016/j.envint.2020.105715
Keywords: Klebsiella pneumoniae, antibiotic resistance, metals, chicken production, environment, meat, WGS, plasmids
Citation: Mourão J, Magalhães M, Ribeiro-Almeida M, Rebelo A, Novais C, Peixe L, Novais & and Antunes P (2024) Decoding Klebsiella pneumoniae in poultry chain: unveiling genetic landscape, antibiotic resistance, and biocide tolerance in non-clinical reservoirs. Front. Microbiol. 15:1365011. doi: 10.3389/fmicb.2024.1365011
Edited by:
Chunlei Shi, Shanghai Jiao Tong University, ChinaReviewed by:
Shifu Aggarwal, Massachusetts General Hospital and Harvard Medical School, United StatesBimal Jana, Massachusetts General Hospital and Harvard Medical School, United States
Copyright © 2024 Mourão, Magalhães, Ribeiro-Almeida, Rebelo, Novais, Peixe, Novais and Antunes. This is an open-access article distributed under the terms of the Creative Commons Attribution License (CC BY). The use, distribution or reproduction in other forums is permitted, provided the original author(s) and the copyright owner(s) are credited and that the original publication in this journal is cited, in accordance with accepted academic practice. No use, distribution or reproduction is permitted which does not comply with these terms.
*Correspondence: Patrícia Antunes, patriciaantunes@fcna.up.pt