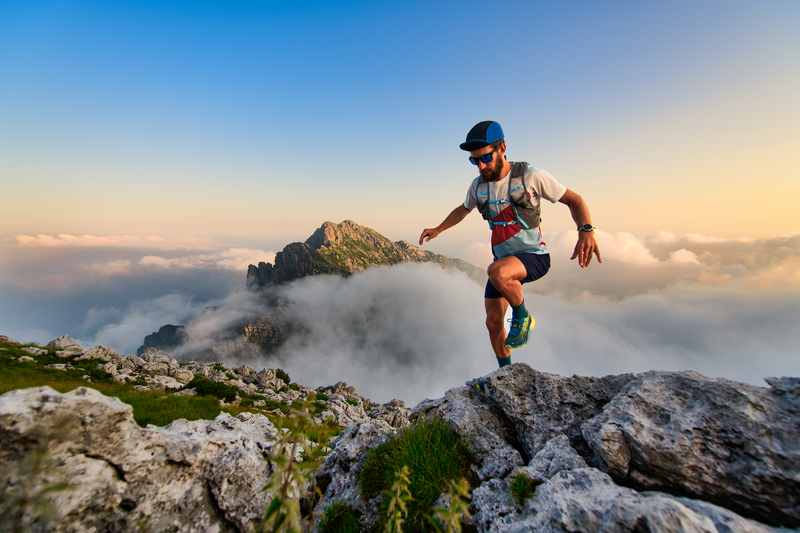
95% of researchers rate our articles as excellent or good
Learn more about the work of our research integrity team to safeguard the quality of each article we publish.
Find out more
ORIGINAL RESEARCH article
Front. Microbiol. , 01 February 2024
Sec. Phage Biology
Volume 15 - 2024 | https://doi.org/10.3389/fmicb.2024.1358909
Flagellotropic bacteriophages are interesting candidates as therapeutics against pathogenic bacteria dependent on flagellar motility for colonization and causing disease. Yet, phage resistance other than loss of motility has been scarcely studied. Here we developed a soft agar assay to study flagellotropic phage F341 resistance in motile Campylobacter jejuni. We found that phage adsorption was prevented by diverse genetic mutations in the lipooligosaccharides forming the secondary receptor of phage F341. Genome sequencing showed phage F341 belongs to the Fletchervirus genus otherwise comprising capsular-dependent C. jejuni phages. Interestingly, phage F341 encodes a hybrid receptor binding protein (RBP) predicted as a short tail fiber showing partial similarity to RBP1 encoded by capsular-dependent Fletchervirus, but with a receptor binding domain similar to tail fiber protein H of C. jejuni CJIE1 prophages. Thus, C. jejuni prophages may represent a genetic pool from where lytic Fletchervirus phages can acquire new traits like recognition of new receptors.
Flagella-driven motility of pathogenic bacteria is considered an important virulence factor as it is essential for establishing gut colonization, as well as mediating epithelial cell adhesion (Haiko and Westerlund-Wikström, 2013). In addition, flagella also function as initial attachment sites for many bacteriophages (phages), the natural predators of bacteria (Iino and Mitani, 1967; Raimondo et al., 1968; Lotz et al., 1977; Pate et al., 1979; Zhilenkov et al., 2006; Evans et al., 2010a,b; Guerrero-Ferreira et al., 2011; Choi et al., 2013; Baldvinsson et al., 2014). It is believed that by binding to flagella, phages increase their capture range and thus increase the chances of finding suitable hosts in complex environments like the gut. Such phages are generally referred to as flagellotropic phages, and they are considered interesting candidates for therapeutic applications against pathogenic bacteria relying on motility to cause disease.
Most phages attaching to flagella require flagellar rotation to establish infection promoting the translocation of the phage along the flagella filament down to the bacterial surface like a “nut-on-a-bolt” (Schade et al., 1967; Ravid and Eisenbach, 1983; Samuel et al., 1999; Evans et al., 2010a; Guerrero-Ferreira et al., 2011; Yen et al., 2012; Choi et al., 2013; Baldvinsson et al., 2014; Sørensen et al., 2015). Several studies propose that when flagellotropic phages reach the bacterial body they interact with a secondary receptor, where injection of the phage genome takes place (Schade et al., 1967; Lotz et al., 1977; Pate et al., 1979; Bender et al., 1989; Samuel et al., 1999; Guerrero-Ferreira et al., 2011; Baldvinsson et al., 2014). Yet, only very few studies describe the nature of the secondary receptor such as pili recognized by Caulobacter phage ϕCbK, lipopolysaccharides recognized by Agrobacterium phage 7-7-1, and the AcrABZ-TolC complex proposed for Salmonella phage Chi (Guerrero-Ferreira et al., 2011; Gonzalez et al., 2018; Esteves et al., 2021). Also, only in the case of phage 7-7-1 have receptor binding proteins (RBPs) been proposed for flagellotropic phages (Gonzalez and Scharf, 2021). Furthermore, little is known about how resistance may develop under conditions where there is selective pressure to maintain motility, such as during host colonization.
Often in vitro selection and characterization of phage resistant mutants is used as a method to identify both phage resistance mechanisms and surface components important for phage infection. Yet, in vitro experiments investigating flagellotropic phage resistance development often result in loss of motility or mutations in flagella associated genes (Lotz et al., 1977; Coward et al., 2006; Evans et al., 2010b; Choi et al., 2013). As a result, it is difficult to identify secondary surface structures important for flagellotropic phage infection in vitro and to study resistance development that may better reflect in vivo conditions.
Flagellotropic phages have been proposed as a promising intervention strategy against the widespread foodborne pathogen Campylobacter jejuni that relies on flagellar motility for establishing intestinal colonization and human disease (El-Shibiny et al., 2009; Carvalho et al., 2010; Hammerl et al., 2014). As human disease is often associated with the consumption of contaminated chicken meat due to high levels of C. jejuni colonization in broiler chickens (Igwaran and Okoh, 2019), some studies have investigated the use of flagellotropic Campylobacter phages to lower C. jejuni prevalence in experimental chickens (El-Shibiny et al., 2009; Hammerl et al., 2014). It was for example shown that the oral administration of flagellotropic phage CP220 resulted in a 2-log decline in C. jejuni HPC5 cecal counts (El-Shibiny et al., 2009). Another study showed that the combination of flagellotropic phage CP68 with capsular-dependent phage CP14 led to a 3-log reduction in C. jejuni counts in fecal samples 48 h after administration, yet the reduction was not maintained over time (Hammerl et al., 2014). Despite the promising results, phage resistance development was detected in both studies, yet the underlying mechanism was not determined. One study reporting resistance development towards flagellotropic Campylobacter phages in vitro describes the loss of bacterial motility (Coward et al., 2006). Thus, it is currently unknown how resistance may develop towards flagellotropic Campylobacter phages when there is selective pressure to maintain motility such as during colonization of the host.
Most flagellotropic Campylobacter phages described so far belong to the Firehammervirus genus, but no receptors have been identified for these phages. C. jejuni produces two polar flagella, one at each cell end, and motility occurs in repetitive short runs and reversals where the direction is coordinated by wrapping one flagellum around the bacterial body (Cohen et al., 2020). The flagellum is formed by two flagellins, FlaA and FlaB, that are post-translationally glycosylated with pseudaminic acid and derivatives thereof. Some strains also contain legionaminic acid and related derivatives on the flagella (Burnham and Hendrixson, 2018). Flagellin glycosylation is essential for flagella assembly and is determined by the gene content in the O-linked glycosylation locus (Goon et al., 2003). The bacterial body is also highly glycosylated as C. jejuni produces capsular polysaccharides (CPS), lipooligosaccharides (LOS), and N-linked glycosylated outer membrane proteins (Burnham and Hendrixson, 2018). The CPS normally consists of two to three backbone sugars that are often modified with O-methyl phosphoramidate (MeOPN) and O-methyl groups at different locations (Karlyshev et al., 2005a; McNally et al., 2007). The LOS are short oligosaccharides divided into an inner and outer core and some C. jejuni strains have the outer core further modified with sialic acid (Gilbert et al., 2002). The gene content in the CPS, LOS, and O-linked glycosylation loci varies between strains and several of these genes are furthermore phase variably expressed due to the presence of homopolymeric nucleotide tracts resulting in frameshift mutations during gene replication (van der Woude, 2011; Bayliss et al., 2012). Several capsular-dependent C. jejuni phages have been characterized that all belong to the Fletchervirus genus. These phages use the MeOPN modification of the CPS as a common receptor (Sørensen et al., 2015; Gencay et al., 2018; Sørensen et al., 2021a). The Fletchervirus phages described so far encoded up to four different receptor binding proteins (rbp1–rpb4) where RBP1 is responsible for recognizing MeOPN (Sørensen et al., 2021a). However, flagellotropic Campylobacter phages infect C. jejuni independently of the CPS demonstrating that CPS is not a receptor for this group of phages (Baldvinsson et al., 2014; Sørensen et al., 2015). In addition, the receptor binding proteins encoded by flagellotropic Campylobacter phages have not been identified yet.
C. jejuni phage F341 is dependent on motility for successful host infection and binds to the flagella using the distal tail fibers (Baldvinsson et al., 2014). Phage F341 can however also infect a non-motile mutant of its propagation host C. jejuni NCTC12658 with a low efficiency supporting the interaction with a secondary receptor present on the bacterial body (Baldvinsson et al., 2014). Yet, the specific surface components recognized by phage F341 as well as its genetic content and genetic relationship with other C. jejuni phages have not been determined. Here we aimed to develop an in vitro assay to study phage resistance development against flagellotropic phages when motility is maintained using C. jejuni NCTC12658 and phage F341 as model organisms. We further aimed to (i) identify the secondary receptor of phage F341, (ii) determine its genetic content and (iii) identify the receptor binding proteins responsible for host recognition.
All bacterial strains and information on phage F341 are listed in Supplementary Table S1. Campylobacter jejuni was standardly grown on base II plates with 5% calf blood (BA) at 37°C under microaerobic conditions (6% O2, 6% CO2, 88% H2N2 or 6% O2, 6% CO2, 88% N2).
The genome sequences of C. jejuni strain NCTC12658 and Campylobacter phage F341 are deposited in NCBI Genbank under the NZ_CP081480.1 and OQ864999 Accession numbers. The fastq sequencing files of the LJ variants can be found at the European Nucleotide Archive under project Accession number PRJEB61440.
C. jejuni strains of interest were grown under standard growth conditions and harvested into cation adjusted (1 mM CaCl2, 10 mM MgSO4). Brain Hearth Infusion broth (Oxoid) (CBHI) and subsequently adjusted to OD600 of 0.01 in 10 mL cultures. Phage F341 was added to a 10 mL culture containing C. jejuni strain NCTC12658 to reach a multiplicity of infection (MOI) of 0.001, whereas no phage was added to a NCTC12568 control culture and a culture containing NCTC12658∆motA. All cultures were incubated for 24 h under standard growth conditions. Following incubation, serial dilutions (10−4–10−8) of the cultures were made in phosphate buffered saline, and 100 μL of each serial dilution was plated onto BA plates with 5 mL of NZCYM overlay agar with 0.6% agar concentration that had been pre-dried for 45 min under a flow hood. The plates were then incubated under standard growth conditions for 2–3 days until single colonies were visible. Swarming colonies appearing on plates where serial dilutions of the NCTC12658 + phage F341 culture had been plated were re-streaked on regular BA plates, harvested into BHI, and stored at −80°C. Swarming colonies named LJ1–LJ14 were later tested for phage sensitivity and motility as mentioned below.
Motility assays were performed as described previously (Sørensen et al., 2011). In brief, C. jejuni strains of interest were grown at standard conditions for 18–24 h. Cells were then harvested into BHI and adjusted to an OD600 of 0.1. One microliter of the suspension was spotted in the center of five Heart infusion broth (HIB) (Difco) 0.25% agar plates that had been pre-dried for 45 min in a flow hood. Plates were incubated under standard growth conditions and growth zones representing motility were measured after 24, 42, and 48 h. Measurements and standard deviations represent the mean counts from two independent experiments.
Phage F341 was propagated on C. jejuni NCTC12658 as described previously using the double-layer agar method (Baldvinsson et al., 2014). Phages from lysed plates were harvested into 5 mL SM buffer (50 mM Tris-HCl, pH-7.5, 100 mM CaCl, 8 mM MgSO4) and stored at 4°C. Titration and standard plaque assays were performed as previously described using spot assays on bacterial lawns (Sørensen et al., 2021a). Briefly, tenfold serial dilutions of the F341 phage stock were made in SM buffer up to 10−7, and three aliquots of 10 μL of the undiluted stock (100) and each dilution were spotted on bacterial lawns with the strains of interest. Plates were incubated for 18–24 h at 37°C under standard C. jejuni growth conditions and plaques were counted and the mean plaque forming units per ml (PFU/mL) were calculated. The RBP antibody plaque assay was performed as previously described (Sørensen et al., 2021a). Briefly, anti-F341_RBP serum and pre-serum (serum extracted before immunization) were added at a 1:10 ratio to the phage F341 stock for 1 h at room temperature before serial dilutions were made and spotted on NCTC12658 lawns. All experiments were performed in duplicate and the data presented are the means from two independent experiments.
Phage adsorption assays were performed as previously described (Baldvinsson et al., 2014). Briefly, C. jejuni cultures were washed and adjusted to an OD600 of 0.4 in CBHI and inoculated with phage F341 at an MOI of 0.001. Cultures were incubated at 37°C and samples containing free phages were collected at 0, 30, 60, and 90 min and filtered through a 0.22 μm sterile syringe filter (Millipore). Filtered samples were stored at 4°C until enumeration (plaque assay). Free phages were enumerated on C. jejuni NCTC12658 and calculated as the percentage of free phages compared to time point zero for each sample (phage PFU/mL at time zero was accounted as 100%). The data are representative of two independent experiments and represent the mean percentages of free phages and standard deviations thereof.
Genomic DNA from phage F341 was isolated as described previously (Sørensen et al., 2021a). The DNA concentration was estimated visually based on 0.7%–1% agarose gel electrophoresis as Campylobacter phages contain modified DNA (Crippen et al., 2019) that cannot be measured correctly by fluorometric quantification. Chromosomal DNA from C. jejuni NCTC12658 was isolated using Maxwell 16 Tissue DNA extraction kit (Promega) (Illumina sequencing) and the MagAttract HMW DNA kit (Qiagen) (SMRT sequencing, Pacific Biosciences) according to the manufacturer’s instructions. Chromosomal DNA from the LJ variants was isolated using Maxwell 16 Tissue DNA extraction kit (Promega) according to the manufacturer’s instructions.
Genome sequencing of C. jejuni NCTC12658 was done on the Illumina platform (Illumina Hiseq and Miseq) and on a PacBio RS II device (Pacific Biosciences, Menlo Park, CA, United States) using P6/C4 chemistry using one SMRT cell. The complete de novo genome was assembled using SMRT Analysis version 2.3 and the HGAP3 algorithm. Average coverage was 634-fold. The genome was annotated using the NCBI Prokaryotic Genome Annotation Pipeline (PGAP). Genome sequencing of phage F341 resistant NCTC12658 variants LJ1, LJ6, LJ8, LJ9, LJ10, LJ11, and LJ13 was performed using the Illumina platform (Illumina Miseq). Sequencing of LJ4 was not performed due to failed library preparation. Basic variant detection analysis was performed using CLC genomics workbench version 21.0.3 using default settings. Reads mapping to the NCTC12658 reference genome were as follows for the phage F341 resistant variants; LJ1: 384.052, LJ6: 674.826, LJ8: 656.167, LJ9: 535.977, LJ10: 565.008, LJ11: 537.104 and LJ13: 460.315 with coverage of detected SNP’s ranging from 10-fold to 132-fold. De novo assembly of the phage F341 resistant variants was performed using CLC genomic workbench version 21.0.3 with a total number of contigs generated: 144 (LJ1), 157 (LJ6), 141 (LJ8), 173 (LJ9), 113 (LJ10), 151 (LJ11) and 39 (LJ13).
The phage F341 genome was sequenced on a PacBio RS II device (Pacific Biosciences, Menlo Park, CA, United States) using P6/C4 chemistry using one SMRT cell. Sequencing produced 30,591 reads with a mean read length of 25,853. The complete de novo genome was assembled using SMRT Analysis version 2.3 and the HGAP3 algorithm. The average coverage of the phage F341 genome was 6,006-fold. The phage genome was annotated using CPT Galaxy and WebApollo platforms (Afgan et al., 2018). Comparative genomics of Fletchervirus phages was performed in CLC main workbench version 20.0.4 using the whole genome alignment 20.1 plugin tool with default settings. Upper comparison gradient: ANI (average nucleotide identity), lower comparison: AP (alignment percentage). Detailed in silico analysis of phage F341 proteins was performed using InterPro (Mitchell et al., 2019), BLASTp (Johnson et al., 2008), HHpred (Zimmermann et al., 2018), Colab AlphaFold2 (Mirdita et al., 2022) and Dali server (Holm, 2022). Proteins are visualized using PyMOL (Schrödinger, 2015).
Pulsed field gel electrophoresis (PFGE) analyses of C. jejuni NCTC12658 and LJ variants were performed according to Ribot et al. (2001) using two restriction enzymes, SmaI and KpnI (New England Biolabs), separately.
The deletion of 05515-05525 in NCTC12658 was constructed by replacing most of the genes (the first 287 bp of 05525 and the last 36 bp of 05515 are maintained) with a kanamycin (kan) cassette by homologous recombination. A vector was constructed by fusing three PCR amplicons as described below using In-fusion cloning (Takara) and used for homologous recombination. Two PCR fragments (746 bp and 829 bp) flanking the region upstream and downstream of the 05515-05525 deletion were amplified from C. jejuni NCTC12658 using the primers LOS_F_up and LOS_R_up and LOS_F_down and LOS_R_down, respectively. The kan gene (1,398 bp) was amplified from pBCα3 (Bijlsma et al., 1999) using the primers Kan + LOS_F and Kan + LOS_R. The kan gene was then inserted between the upstream and downstream 05515-05525 flanking PCR fragments and simultaneously inserted into the pGEM-7Zf vector (Promega) in the BamHI and XhoI sites using the In-Fusion kit according to the manufacturer’s instructions. The resulting plasmid pMP810 was transformed into Escherichia coli StellarTM Competent Cells (Takara) according to manufacturer’s instructions and verified by sequencing. Purified pMP802 plasmid was electroporated into C. jejuni NCTC12658 promoting homologous recombination of the flanking 05515-05525 regions to create NCTC12658Δ05515-05525. The mutant was verified by PCR using the LOS_control_F and LOS_control_R primers and sequencing. Plasmids and primers are listed in Supplementary Table S1.
A 1.439 bp fragment including F341_rbp and the downstream F341_034 chaperone plus an additional TAA stop codon was synthetically produced and inserted into the NdeI and XhoI cloning sites of pET28a + producing the F341_RBP protein with an N-terminal his-tag used for purification of the protein.
The F341_RBP was expressed and purified as previously described using E. coli BL21-CodonPlus (DE3)-RIL competent cells (Agilent Technologies) and 0.5 mM IPTG (isopropyl β-D-1-thiogalactopyranoside) induction (Sørensen et al., 2021a). The F341_RBP was purified using Ni2+-NTA-affinity chromatography (His GraviTrap, GE Healthcare) according to the manufacturer’s instructions and exchanged into PBS buffer using 10 kDA Amicon® Ultra-15 Centrifugal filter units. The purified protein was used to raise polyclonal rabbit antibodies generated by Davids Biotechnologie GmbH (Germany). Anti-F341_RBP serum (anti-RBP serum) was purified on a protein A column and stored at −20°C until use. The binding ability of purified anti-RBP serum to phage F341 was confirmed by standard Western blotting.
Immunogold electron microscopy was performed as described previously (Sørensen et al., 2021a). Staining was performed as previously reported with 2% (w/v) uranyl acetate on freshly prepared carbon films (Sørensen et al., 2015). Grids were analyzed in a Tecnai 10 transmission electron microscope (TEM) (FEI Thermo Fisher, Eindhoven, The Netherlands) at an acceleration voltage of 80 kV. Micrographs were taken with a MegaView G2 charge-coupled device camera (EMSIS, Muenster, Germany).
Data in the graphs and figures are representative of at least two independent experiments and show mean values with error bars that represent the standard deviations as reported in the figure legends.
To investigate phage resistance in C. jejuni against flagellotropic phages when motility is maintained, we developed a soft agar assay that would allow us to screen for motile phage resistant variants in vitro. We exposed C. jejuni NCTC12658 to phage F341 in liquid culture for 24 h followed by plating serial dilutions on double layered soft agar (SA) plates where swarming/motility of single colonies could be observed (Figure 1A). To evaluate motility levels, we also plated the C. jejuni NCTC12658 wildtype (motile) and an NCTC12658∆motA mutant (non-motile) as controls. As expected, many colonies of the NCTC12658 wildtype exhibited swarming behavior, while no swarming was observed for the NCTC12658∆motA colonies (Figure 1A). Most colonies originating from NCTC12658 exposed to phage F341 demonstrated no swarming behavior, consistent with previous findings that loss of motility rapidly occurs when C. jejuni is exposed to flagellotropic phages in vitro. Still, some motile colonies could be observed (Figure 1A), suggesting that phage F341 resistance without loss of motility can also develop in vitro. To verify that the swarming colonies exposed to phage F341 indeed were fully motile and resistant to phage F341, we isolated 14 swarming colonies from the SA plate (named LJ1 to LJ14) and investigated phage F341 sensitivity by plaque formation and assessed motility in soft agar. Six of the LJ colonies were still highly motile but had not gained phage resistance, as F341 plaque formation was comparable to the NCTC12658 wildtype (data not shown). However, eight colonies (LJ1, LJ4, LJ6, LJ8, LJ9, LJ10, LJ11, and LJ13) demonstrated complete resistance to phage F341 as no lysis nor plaque formation was observed, while still exhibiting motility at levels comparable to the NCTC12658 wildtype (Figure 1B). To determine if phage F341 resistance was associated with lack of phage binding, we investigated whether F341 could adsorb to the eight motile phage resistant LJ strains (Figure 1C). Our data showed that for all eight LJ strains, phage adsorption was prevented suggesting changes in surface structures such as alteration or masking of the receptor recognized by phage F341. Thus, our soft agar assay allowed us to isolate motile C. jejuni NCTC12658 variants in vitro exhibiting complete resistance towards flagellotropic phage F341.
Figure 1. Motile phage F341 resistant C. jejuni NCTC12658 variants (LJ strains) isolated in vitro demonstrate lack of phage binding. (A) Soft agar (SA) assay with C. jejuni NCTC12658, NCTC12568∆motA and NCTC12658 exposed to phage F341 demonstrating different levels of swarming and non-swarming colonies. Examples of swarming colonies spreading out on the agar plate by flagellar motility are indicated by arrows. (B) Phage F341 infection and motility profiles of isolated swarming NCTC12658 colonies exposed to phage F341 (LJ strains). (C) Phage F341 adsorption assay of phage resistant LJ strains. t equals the timepoint in minutes where samples were collected, and the number of free phages determined. Pfu/ml of free phages at time zero was accounted as 100%. The images represent two independent experiments; however, the LJ colonies were only isolated from plates from one of the independent experiments. Error bars and ± indicated mean standard deviations.
To identify genetic changes that could account for phage F341 resistance, we sequenced the genomes of seven phage resistant NCTC12658 variants (LJ1, LJ6, LJ8, LJ9, LJ10, LJ11, and LJ13) with Illumina sequencing. We also genome sequenced the NCTC12658 wildtype strain using both Illumina and Pacific Bioscience (PacBio) SMRT sequencing to fully assemble the genome. We then used the complete genome of NCTC12658 and the sequencing reads of the seven phage resistant variants to detect single nucleotide polymorphisms (SNPs) and larger gene insertions or deletions (Table 1; Supplementary Tables S2, S3). To identify potential genomic rearrangements we also performed de novo assembly and pulsed field gel electrophoresis (PFGE), but no rearrangements were observed (Supplementary Figure S1).
Table 1. Single nucleotide polymorphisms (SNPs) and gaps observed in motile phage F341 resistant NCTC12658 variants in the lipooligosaccharide (LOS) locus following basic variant detection analysis.
As the genome of LJ9 was not fully covered by the sequencing we could not identify genetic changes associated with phage F341 resistance in this variant. However, our analysis identified a limited number of SNPs (≤14) and two gene deletions in the remaining phage resistant NCTC12658 variants (Supplementary Results; Table 1; Supplementary Tables S2, S3). Most SNPs were associated with insertions or deletions (indels) in homopolymeric G (polyG) or A (polyA) tracts causing phase variable gene expression in C. jejuni. Such indels are expected within a given C. jejuni population due to the stochastic nature of slipped strand mispairing of such regions during DNA replication (van der Woude, 2011; Bayliss et al., 2012). Thus, some SNPs may not be due to phage F341 exposure but could be a random mutation event originating from picking single LJ colonies. Also, different homopolymeric tract lengths were in some cases observed between the two sequencing methods for the NCTC12658 wildtype. Hence, when gene expression was found to be both turned on and off in the wildtype NCTC12658 strain sensitive to phage F341, or when different homopolymeric tract lengths were observed that did not lead to differences in the gene expression state, the SNP was not considered relevant. In addition, our experimental data showed that phage resistance was associated with lack of phage adsorption, indicating genetic changes associated with surface structures. Genetic changes not considered relevant for phage F341 resistance development are presented in Supplementary Results and Supplementary Tables S2, S3.
Notably, four out of the seven LJ strains (LJ1, LJ6, LJ8, and LJ11) contain SNPs in genes encoding sugar transferases modifying the LOS structure of C. jejuni NCTC12658 (Table 1). NCTC12658 contains the same LOS locus as C. jejuni NCTC11168 producing an inner LOS core containing ketodeoxytonic acid, heptose, phosphoethanolamine and glucose, and an outer LOS core containing N-acetylgalactoseamine, N-acetylneuraminic acid (sialic acid), glucose and galactose (Figure 2A) (Karlyshev et al., 2005b). LJ6, LJ8, and LJ11 all contain polyA tract variations that either switch off gene expression or lead to the formation of truncated protein products of K5A08_05515, ctsIII, and K5A08_05525, respectively. These genes are responsible for modifying the outer LOS core with galactose and sialic acid, and the inner LOS core with glucose residues suggesting that these LJ strains contain diverse LOS structures compared to the NCTC12658 wildtype (Table 1; Figure 2A). As polymeric tracts found at the gene end in C. jejuni have been proposed to influence transcription and/or translation of the downstream gene (Kim et al., 2012), the polyA tract variation of cstIII in LJ8 may instead affect the downstream neuB1 involved in sialic acid biosynthesis (Table 1). A single nucleotide deletion was observed also in the K5A08_05515 gene in LJ1 introducing an early stop and a truncated protein product. Furthermore, large gaps were found in the LOS loci covering the K5A08_05520 and K5A08_05525 gene regions in LJ10 and LJ13 (Table 1; Supplementary Table S3). The deletion of these two genes in the LOS locus was furthermore confirmed by the de novo assembly of LJ13 (data not shown). K5A08_05520 has been proposed to link the outer LOS core to the inner LOS core. Thus, six out of the seven phage F341 resistant NCTC12658 variants show diverse genetic changes that may all result in a different LOS structure as compared to the wildtype NCTC12658 (Figure 2A).
Figure 2. Phage F341 uses lipooligosaccharides as a secondary receptor for successful infection of its host C. jejuni NCTC12658. (A) Schematic representation of the lipooligosaccharides (LOS) putatively produced by C. jejuni NCTC12658, the LJ phage F341 resistant NCTC12658 variants and the C. jejuni NCTC12658ΔLOS (C. jejuni NCTC12658Δ05515-05525) mutant. (B) Standard plaque assays of phage F341 on its propagation host C. jejuni NCTC12658, the non-motile C. jejuni NCTC12658ΔmotA mutant and C. jejuni NCTC12658ΔLOS. (C) Mean F341 log10 PFU/mL values from standard plaque assays on C. jejuni NCTC12658, C. jejuni NCTC12658ΔmotA and C. jejuni NCTC12658ΔLOS. 3 × 10 μL of undiluted phage F341 stock and tenfold serial dilutions up to 10−7 are spotted clockwise from the top of all plaque assay plates. The images represent 2–4 independent experiments and error bars indicated standard deviations. Gal, galactose; GalNAc, N-acetylgalactoseamine; Neu5Ac, N-acetylneuraminic acid (sialic acid); Hep, heptose; Glu, glucose; Kdo, ketodeoxytonic acid. See also Supplementary Figure S2.
As the genome analysis of the phage F341 resistant motile NCTC12658 variants showed several genetic changes in the LOS locus, we speculated if phage F341 could be dependent on LOS as a secondary receptor for successful host infection. Many of the SNPs and gene deletions were found in the K5A08_05515–K5A08_05525 gene region responsible for both modifying and linking the inner and the outer LOS core. We therefore created a C. jejuni NCTC12658Δ05515-05525 (NCTC12658ΔLOS) deletion mutant only producing the inner LOS core lacking modifying glucose residues (Figure 2A). Before conducting further experiments, we confirmed that the NCTC12658ΔLOS mutant was still motile at levels similar to the NCTC12658 wildtype (Supplementary Figure S2). We then investigated phage F341 sensitivity by plaque formation and compared the results to those obtained on the NCTC12658 wildtype and the non-motile NCTC12658ΔmotA mutant. While phage F341 infected the non-motile NCTC12658ΔmotA mutant with a lower efficiency of plating as demonstrated previously (Baldvinsson et al., 2014), no lysis nor plaque formation was observed on the NCTC12658ΔLOS mutant (Figures 2B,C). These results demonstrate that phage F341 is completely dependent on the LOS for successful infection thereby functioning as a secondary receptor in C. jejuni NCTC12658.
To determine the genetic content of Campylobacter phage F341, we performed whole genome sequencing. Sequencing revealed a genome size of 131.68 kb with an average GC content of 26%, and BLASTn searches showed that phage F341 belongs to the Fletchervirus genus. This is consistent with a genome size of approximately 130 kb being characteristic for phages belonging to this genus (Javed et al., 2014). In addition, phage F341 encodes five tRNA’s and a large number (12) of putative homing endonucleases which has also previously been observed in other Fletchervirus phages (Javed et al., 2014). To further investigate the genetic relationship between phage F341 and other Fletchervirus phages, we performed comparative genomics using the genome sequences of 18 Fletchervirus phages in our collection (Sørensen et al., 2021a). Comparative genomics showed 89%–96% average nucleotide identity (alignment percentage: 85%–94%, average: 88.4%) between the F341 genome and the 18 Fletchervirus genome sequences (Figure 3A) with the closest relative being phage F336 (alignment percentage: 92%, average 96%). Thus, phage F341 shows high sequence similarity to other Fletchervirus phages and we were only able to detect five genes (F341_034, F341_035, F341_109, F341_128 and F341_135) that were either only observed in phage F341, or which showed no or very low sequence similarity to genes/translated proteins encoded by other Fletchervirus phages (Table 2; Supplementary Table S4). Further detailed in silico analyses demonstrated that F341_109 and F341_128 show structural similarity to baseplate wedge proteins. F341_109 also demonstrates structural similarity to adaptor proteins forming base plate attachment sites for tail fibers in other phages (Supplementary Table S4). F341_135 is likely encoding fibritin which in E. coli phage T4 forms neck/collar fibers and exhibits chaperone function by promoting the assembly of the long tail fibers and their attachment to the tail base plate (Tao et al., 1997). Interestingly, this gene was not observed at the corresponding position in other Fletchervirus genomes currently available. The remaining two unique F341 genes (F341_034 and F341_035) were located within the receptor binding protein (RBP) region for capsular-dependent Fletchervirus phages and will be discussed in more detail in the following paragraph. Thus, only a few genes diverge in phage F341 compared to other Fletchervirus phages, yet proposed functions suggest differences in tail associated genes involved in host recognition.
Figure 3. Flagellotropic phage F341 belongs to the Fletchervirus genus but shows limited sequence similarity to related phages in the RBP region. (A) Comparative genomics of phage F341 and 18 Fletchervirus phages. Whole genome alignment visualized as a heat map for average nucleotide identity. Closest relatives are further indicated by the trees. (B) Alignment of the receptor binding protein region in Fletchervirus phages dependent on the capsular polysaccharide for infection and phage F341. Phage F358 is used as a representative of capsular-dependent Fletchervirus phages. (C) Protein alignment of the RBP of phage F341 and the tail fiber protein H of the CJIE1 prophage in C. jejuni strain HF5-4A-4. Comparative genomics was performed using CLC main workbench version 20.0.4 using the whole genome alignment 20.1 tool with default settings. See also Supplementary Figures S3, S4.
Fletchervirus phages are usually dependent on capsular polysaccharides for infection of C. jejuni and encode up to four receptor binding proteins (rbp1–rpb4) (Sørensen et al., 2021a). In these phages, the RBPs are located between a conserved putative recombination endonuclease and putative antiholin (Sørensen et al., 2021a). However, phage F341 encodes a unique 882 bp gene (F341_035, rbp) downstream of the recombination endonuclease, demonstrating no hits to other genes when using BLASTn. When aligning the putative rbp gene in F341 to the rbp region of capsular-dependent Fletchervirus phages, a 26.5% sequence identity to rbp1 (1.338 bp) could however be observed (Figure 3B). Further protein alignment showed that F341_RBP shares some sequence similarity with the N-terminal of RBP1 up to the point of the pectin lyase domain found in these proteins (Supplementary Figure S3). Interestingly, BLASTp demonstrated sequence similarity to the C-terminal of the tail fiber protein H encoded by the cryptic CJIE1 prophage in C. jejuni strain HF5-4A-4 (Figure 3C; Table 2). Complete alignment of the protein sequences of F341_RBP and the tail fiber protein H showed an almost identical sequence in the far C-terminal of these two RBPs (Figure 3C). These results suggest that F341 encodes a novel hybrid RBP with similarity to RBP1 encoded by the lytic capsular-dependent Fletchervirus phages and the RBP (tail fiber protein H) of a CJIE1 prophage.
In both the Fletchervirus phages and the CJIE1 prophages, a putative chaperone for correct RBP folding is found downstream of the RBPs containing a DUF4376 domain in the C-terminal (Zampara et al., 2021; Sørensen et al., 2021a). Also downstream of the putative rbp gene in phage F341, a hypothetical protein encoded by gene F341_034 with a DUF4376 domain in the C-terminal was observed (Table 2). The F341_034 gene shows sequence similarity in the N-terminal region to a putative chaperone located next to of the tail fiber protein H in C. jejuni HF5-4A-4, whereas the C-terminal region shows similarity to the putative chaperone located downstream of the rbp genes in capsular-dependent Fletchervirus phages (Table 2; Supplementary Figure S4). Thus, these results suggest that F341_034 encodes a putative novel hybrid chaperone associated with the correct folding of the F341 RBP.
To experimentally prove the function of the proposed RBP encoded by phage F341, we raised polyclonal antibodies against the purified protein and used these in an antibody plaque assay to test the inhibition of phage infection. Also, labeled antibodies were applied during transmission electron microscopy to determine the location of the putative RBP on the phage particle. From these experiments, we could confirm that F341_035 encodes the RBP of phage F341, as phage F341 infection of its host was completely blocked in the presence of the RBP antibodies, i.e., no lysis nor plaque formation was observed (Figures 4A,B). Antibodies previously raised against RBP1 from capsular-dependent Fletchervirus phage F358 showed no effect on phage F341 infection as expected (Figure 4B). The lack of lysis and plaque formation is similar to what we observed on the NCTC12658 LOS deletion mutant suggesting that the F341 RBP is recognizing and binding to the LOS (Figure 2B). Also, transmission electron microscopy of phage F341 using immunogold labeled RBP antibodies demonstrated binding to the distal tail fibers/spikes (Figure 4C).
Figure 4. Phage F341 encodes and expresses a unique hybrid RBP with a short tail fiber morphology. (A) Standard and anti-RBP serum plaque assays of phage F341 on its host C. jejuni NCTC12658. (B) Mean F341 log10 pfu/ml values from standard and anti-RBP serum plaque assays on C. jejuni NCTC12658. Anti-RBP1 is serum produced against RBP1 from capsular-dependent Fletchervirus phage F358 (C) Transmission electron microscopy of negatively stained phage F341 and when mixed with immunogold labeled anti-RBP serum. Labeled antibodies bound to phage F341 tail fibers are indicated by arrows. (D) AlphaFold2 predictions of homotrimers of RBP1 from capsular-dependent Fletchervirus phage F358, the RBP from flagellotropic Fletchervirus phage F341 and the tail fiber protein H of the CJIE1 prophage in C. jejuni strain HF5-4A-4. The pectin lyase domain in RBP1 is indicated in cyan blue, whereas the C-terminal region demonstrating sequence similarity between F341_RBP and the tail fiber protein H is indicated in light pink. 3 × 10 μL of undiluted phage F341 stock and tenfold serial dilutions up to 10−7 are spotted clockwise from the top of all plaque assay plates. Anti-RBP and anti-RBP1 serum was added at a 1:10 ratio to the phage F341 stock for 1 h at room temperature before the serial dilutions were prepared and spotted on the NCTC12658 lawn. The images represent 2–4 independent experiments and error bars indicated standard deviations. See also Supplementary Figure S5.
To further characterize the hybrid RBP of phage F341, we modeled the protein structure using AlphaFold2 (Mirdita et al., 2022). For comparisons, we also modeled the structures of RBP1 from capsular-dependent Fletchervirus phage F358 and the tail fiber protein H found in the CJIE1 prophage in the C. jejuni HF5-4A-4 genome (Figure 4D). As RBPs often fold into homotrimers, we predicted all three proteins both as monomers and homotrimers (Figure 4D; Supplementary Figure S5). The F358 RBP1 homotrimer demonstrates a typical tailspike protein (TSP) morphology, i.e., short, rigid and stocky, consisting of two distinct regions, an N-terminal head-like domain and a C-terminal comprised of the pectin lysase domain forming parallel ß-helices. A Dali search (Holm, 2022) comparing protein structures in 3D, further demonstrates high structural similarity between the RBP1 pectin lyase domain and the enzymatic domain of the capsule-degrading tail spike protein (TSP) Gp38 from Klebsiella phage KP32 (Squeglia et al., 2020). The CJIE1 tail fiber protein H from C. jejuni HF5-4A-4 demonstrates typical tail fiber (TF) morphology, i.e., long and slim, with an N-terminal globular head domain, a shaft/stem formed primarily of α-helices and a distal C-terminal knob-like structure consisting of a ß-sandwich. The phage F341 RBP also demonstrates TF morphology similar to the CJIE1 tail fiber protein H although the fiber is much shorter. Overall, the F341 RBP structure consists of an N-terminal head domain followed by a short shaft/stem region and a distal C-terminal ß-sandwich knob-like structure. The distal C-terminal demonstrating sequence similarity between F341_RBP and the CJIE1 tail fiber protein H is responsible for forming the ß-sandwich knob-like structure and a short α-helical hinge region connected to the shaft/stem. Thus, these results suggest that the distal C-terminal knob-like region is responsible for the interaction with the surface receptor. To further investigate, if the predicted F341 RBP structure resembles other known structural characterized RBPs, we ran a Dali search (Holm, 2022), but no significant hits were observed (data not shown). However, we would propose that the F341 RBP shows some structural similarity to the short tail fibers encoded by gp12 in E. coli phage T4 and to the tail fibers encoded by E. coli phage T7 (van Raaij et al., 2001; Garcia-Doval and van Raaij, 2012). In conclusion, our results show that even though phage F341 belongs to the Fletchervirus genus it encodes an unique hybrid RBP with a short tail fiber morphology essential for infection of its C. jejuni host.
Flagellotropic phages have been proposed as a promising intervention strategy against pathogenic bacteria that rely on flagellar motility for establishing gut colonization and human disease (Esteves and Scharf, 2022). Yet, little is known about how resistance may develop towards such phages when there is a selective pressure to maintain motility, such as during host colonization, as such conditions are difficult to simulate and study in vitro. Also, only few studies describe other surface components in addition to flagella that are important for flagellotropic phage infection (Guerrero-Ferreira et al., 2011; Gonzalez et al., 2018; Esteves et al., 2021). Thus, new in vitro approaches to study flagellotropic phage-host interactions when motility is maintained are required to evaluate the potential of these microorganisms as therapeutics. Here we developed a soft agar assay that allowed us to isolate motile C. jejuni NCTC12658 variants in vitro exhibiting complete resistance towards flagellotropic phage F341. By characterizing these variants, we found that phage resistance was associated with mutations in the lipooligosaccharides (LOS) forming the secondary receptor of phage F341. Further genomic characterization of phage F341 showed that it belonged to the Fletchervirus genus, normally comprising phages dependent on capsular polysaccharides (CPS) for infection (Sørensen et al., 2015, 2021a). However, phage F341 contains a hybrid receptor binding protein (RBP) of both lytic Fletchervirus phage and CJIE1 prophage origin promoting the recognition of novel surface receptors for phages of this genus.
We previously found that resistance development in C. jejuni against CPS-dependent Fletchervirus phages was strictly associated with phase variation of surface structures. This was caused by polyG tract variation in the transferases producing the common MeOPN receptor and other CPS modifications both in vitro and in vivo (Sørensen et al., 2011, 2012; Aidley et al., 2017; Gencay et al., 2018). Here we found that resistance development towards Fletchervirus phage F341 was also associated with changes in surface structures, although by very diverse genetic changes, i.e., gene deletions, point mutations and polyA tract variations although all present in the LOS locus. Several surface structures in C. jejuni are prone to phase variation due to the presence of polyG tracts within the reading frame of associated genes often encoding sugar transferases (Parkhill et al., 2000). However, only two genes (wlaN and K5A08_05565) in the LOS locus of C. jejuni NCTC12658 contain such tracts and are phase variably regulated (Linton et al., 2000; Gilbert et al., 2002). wlaN encodes a ß-1,3-galactosyltransferase responsible for adding a terminal ß-linked galactose to the outer LOS core, while K5A08_05565 is a homolog of cj1144-45 in C. jejuni NCTC11168 encoding a putative α-1,4-galactosyltransferase associated with terminal α-linked galactose units (Semchenko et al., 2012). As our results demonstrate that phage F341 most likely is binding to several LOS moieties present both in the outer and inner LOS core, turning off the expression of genes that modify the very distal part of the outer LOS core such as wlaN and K5A08_05565, may not be sufficient to generate phage F341 resistance. On the contrary, numerous polyA tracts of ≥7 A’s are found in many LOS genes and may hence also be prone to phase variation, although a high mutational frequency has not been reported for these. Our results show that phase variation as a result of polyA tract variation is also associated with rapid phage resistance development in C. jejuni in vitro. Despite the different genetic mutations, we still only observed phage resistance development in C. jejuni against Fletchervirus phages linked to surface structure diversity that prevents phage adsorption. One might speculate that this could be a bias from the experimental setup and the in vitro laboratory settings. Yet previous work performed in our group using phage F341 and C. jejuni NCTC11168 in a chicken model, also demonstrated changes in the LOS structure of C. jejuni recovered from the chickens following phage exposure, although the data were not statistically significant (Baldvinsson, unpublished). This illustrates that our simple in vitro soft agar assay may still be a valuable tool for screening potential phage resistance development against flagellotropic phages simulating in vivo conditions where motility is maintained.
Notably, the LOS structures of C. jejuni strains like NCTC12658, which contain sialic acid, show molecular mimicry of the gangliosides on human neurons (Szymanski et al., 2003). Antibodies produced against C. jejuni LOS surface structures following human infection can therefore lead to autoimmune disorders such as Guillan-Barre and Miller-Fisher syndrome (Ang et al., 2002; Yuki et al., 2004). It has previously been shown that a single nucleotide mutation in gene cj1135 (C. jejuni NCTC11168) was associated with different ganglioside mimicry (Gilbert et al., 2002). Gene K5A08_05515 in C. jejuni NCTC12658 is a homolog of cj1135 and was one of the genes where mutations were observed following phage F341 exposure. Thus, it will be important to evaluate the potential impact of the altered LOS structures concerning human disease development if phage F341 were to be applied therapeutically against C. jejuni.
We previously demonstrated that phage F341 interacts with both the flagella and the bacterial body using the distal tail fibers (Baldvinsson et al., 2014). Here we found that the distal tail fibers are formed by a hybrid RBP that is structurally predicted as a short tail fiber and essential for the interaction with the secondary LOS receptor. Flagellotropic phage 7-7-1 also use the distal tail fibers for the interaction with both the flagella and the body of its Agrobacterium hosts (Lotz et al., 1977). Phage 7-7-1 likewise contains short tail fibers and uses the lipopolysaccharides (LPS) as a secondary receptor (Gonzalez et al., 2018). As the flagella in both C. jejuni and Agrobacterium are highly glycosylated (Deakin et al., 1999; Zebian et al., 2016) it is tempting to speculate if the short tail fibers of phage F341 and 7-7-1 are binding loosely to sugar moieties present on the flagella that may appear similar in structure to those of the secondary LOS and LPS receptors, respectively. Indeed, phage adsorption to flagella filaments is generally reversible supporting a loose interaction (Raimondo et al., 1968; Lotz et al., 1977; Baldvinsson et al., 2014). Although the glycans present on the flagella of C. jejuni NCTC12658 have not been determined, the strain encodes an almost identical O-linked flagella glycosylation locus as strain NCTC11168. Previous work has shown that flagellins in C. jejuni NCTC11168 are modified with legionaminic acid that indeed is an analog of sialic acid present in the LOS outer core (Zebian et al., 2016; Schoenhofen et al., 2017). Our results suggest that phage F341 interacts with sialic acid in addition to several other types of carbohydrate moieties present in both the inner and outer LOS core, as mutations in associated LOS genes lead to phage resistance. We furthermore propose that the short tail fibers formed by the hybrid receptor binding protein of phage F341 show some structural similarity to short tail fibers encoded by gp12 in E. coli phage T4. The short Gp12 tail fibers of phage T4 bind very firmly and irreversible to the LPS core region of E. coli to ensure a fixed structure during DNA injection (Thomassen et al., 2003). Thus, we speculate if multiple LOS binding sites may serve a similar purpose for the hybrid RBP of phage F341. We further envision that this may in addition allow phage F341 to distinguish between LOS and flagella interaction, thereby preventing premature DNA injection upon interaction with the flagella. Yet, as flagella and flagellar motility is needed for efficient phage F341 infection, the flagella interaction may also be a prerequisite for efficient binding to the secondary receptor, e.g., by inducing conformational changes in the tail baseplate. Further studies are needed to show if the hybrid RBP of phage F341 indeed is able to interact with legionaminic acid on the flagella. Yet, our study sheds new light on how host recognition and interaction may be accomplished by the distal fibers of flagellotropic phages.
Although phage F341 is dependent on flagella and LOS for host infection compared to the majority of Fletchervirus phages that rely on capsular polysaccharides, we could only identify five genes/proteins unique to this phage including the hybrid RBP and putative RBP chaperone. The remaining three genes all appear to take part in the correct folding and attachment of the hybrid RBP to the F341 tail base plate. Interestingly, we found that the hybrid receptor binding protein identified in phage F341 demonstrated similarity to the tail fiber H protein encoded by a CJIE1 prophage. Only few prophages have been observed in C. jejuni genomes originally identified as integrated elements CJIE1, CJIE2, and CJIE4 in strain RM1221, where CJIE1 shows a Mu-like structure (Parker et al., 2006). It has not been possible to induce or propagate CJIE1 phages except in rare cases suggesting that the majority of these prophages are no longer functional and rather represent phage remnants thus being cryptic prophages (Clark and Ng, 2008; Tanoeiro et al., 2022). We previously confirmed that the tail fiber protein H functions as a receptor binding protein by using this gene from C. jejuni strain CAMSA2147 to engineer pyocins targeting Campylobacter (Zampara et al., 2021). We did however not identify the receptor recognized by this protein, although our data showed that it was not linked to capsular polysaccharides nor flagella as seen for the lytic phages infecting C. jejuni (Zampara et al., 2021). Comparison of CJIE1 regions in different C. jejuni strains show various genetic configurations with an overall high sequence similarity except in the tail fiber protein H encoded gene suggesting that these proteins may interact with diverse receptors (Clark and Ng, 2008). This is contrary to the four RBPs identified in the lytic CPS-dependent Fletchervirus phages that so far all show high sequence similarity across different phages (Sørensen et al., 2021a). Many C. jejuni strains including NCTC12568 do not contain any prophage elements and previous work suggests that the rate of loss and gain of CJIE1 is quite high showing no apparent correlation with colonization levels or human disease (Barton et al., 2007). Yet, the presence of these prophages in very diverse C. jejuni strains suggests an early evolutionary origin as well as a benefit of maintaining prophage associated genes although their role remains indecipherable. It is therefore unclear how phage F341 may have acquired the hybrid receptor binding protein and what environmental pressures may have selected for this unique phage. We previously also observed other genes that are shared among C. jejuni prophages and lytic phages although only annotated as hypothetical proteins (Sørensen et al., 2021b). This suggests that acquisition or exchange of genetic content between these groups of phages may be a more common phenomenon. This would indeed allow the otherwise highly conserved Fletchervirus phages to acquire novel traits such as those observed for phage F341. However, as only a limited number of lytic Campylobacter phages have been sequenced so far, more genomes are needed in order to understand the evolutionary relationship between C. jejuni prophages and lytic phages.
In conclusion, we here present a simple in vitro assay for investigating flagellotropic phage-host interactions when bacterial motility is maintained simulating in vivo conditions. In addition, our work further expands on the current knowledge of flagellotropic phages, but also on our understanding of how Fletchervirus phages infecting C. jejuni have evolved to accomplish new types of host recognition.
The datasets presented in this study can be found in online repositories. The names of the repository/repositories and accession number(s) can be found in the article/Supplementary material.
LO: Formal analysis, Investigation, Methodology, Writing – review & editing. AS: Investigation, Writing – review & editing. HN: Investigation, Methodology, Resources, Writing – review & editing. AV: Investigation, Methodology, Writing – review & editing. JK: Investigation, Resources, Writing – review & editing. MS: Conceptualization, Formal analysis, Funding acquisition, Investigation, Methodology, Resources, Supervision, Writing – original draft, Writing – review & editing.
The author(s) declare financial support was received for the research, authorship, and/or publication of this article. The work presented here was supported by the Danish Council of Independent Research (Grant 4184-00109B), the Danish AgriFish Agency of Ministry of Environment and Food (Grant 34009-14-0873), and Intralytix, Inc. for financial support. We also acknowledge funding of JK by Martin J. Loessner, ETH Zurich.
The authors are thankful to laboratory technician Vi Phuong Thi Nguyen for general assistance on the experimental work and to Stephen J. Ahern for the initial annotation of the phage F341 genome. The authors thank Anna Bratus-Neuenschwander and the staff of the Functional Genomics Center Zurich for their excellent technical support on the genome sequencing of phage F341. Angela Back (Max Rubner-Institut) is acknowledged for her technical assistance with the TEM analysis. Finally, the authors thank Professor Lone Brøndsted for valued discussion and input on the manuscript.
The authors declare that the research was conducted in the absence of any commercial or financial relationships that could be construed as a potential conflict of interest.
All claims expressed in this article are solely those of the authors and do not necessarily represent those of their affiliated organizations, or those of the publisher, the editors and the reviewers. Any product that may be evaluated in this article, or claim that may be made by its manufacturer, is not guaranteed or endorsed by the publisher.
The Supplementary material for this article can be found online at: https://www.frontiersin.org/articles/10.3389/fmicb.2024.1358909/full#supplementary-material
Afgan, E., Baker, D., Batut, B., van den Beek, M., Bouvier, D., Cech, M., et al. (2018). The galaxy platform for accessible, reproducible and collaborative biomedical analyses: 2018 update. Nucleic Acids Res. 46, W537–W544. doi: 10.1093/nar/gky379
Aidley, J., Sørensen, M. C. H., Bayliss, C. D., and Brøndsted, L. (2017). Phage exposure causes dynamic shifts in the expression states of specific phase-variable genes of Campylobacter jejuni. Microbiology 163, 911–919. doi: 10.1099/mic.0.000470
Ang, C. W., Laman, J. D., Willison, H. J., Wagner, E. R., Endtz, H. P., De Klerk, M. A., et al. (2002). Structure of Campylobacter jejuni lipopolysaccharides determines antiganglioside specificity and clinical features of Guillain–Barré and Miller fisher patients. Infect. Immun. 70, 1202–1208. doi: 10.1128/IAI.70.3.1202-1208.2002
Baldvinsson, S. B., Sørensen, M. C. H., Vegge, C. S., Clokie, M. R. J., and Brøndsted, L. (2014). Campylobacter jejuni motility is required for infection of the flagellotropic bacteriophage F341. Appl. Environ. Microbiol. 80, 7096–7106. doi: 10.1128/AEM.02057-14
Barton, C., Ng, L. K., Tyler, S. D., and Clark, C. G. (2007). Temperate bacteriophages affect pulsed-field gel electrophoresis patterns of Campylobacter jejuni. J. Clin. Microbiol. 45, 386–391. doi: 10.1128/JCM.01513-06
Bayliss, C. D., Bidmos, F. A., Anjum, A., Manchev, V. T., Richards, R. L., Grossier, J. P., et al. (2012). Phase variable genes of Campylobacter jejuni exhibit high mutation rates and specific mutational patterns but mutability is not the major determinant of population structure during host colonization. Nucleic Acids Res. 40, 5876–5889. doi: 10.1093/nar/gks246
Bender, R. A., Refson, C. M., and O'Neill, E. A. (1989). Role of the flagellum in cell-cycle-dependent expression of bacteriophage receptor activity in Caulobacter crescentus. J. Bacteriol. 171, 1035–1040. doi: 10.1128/jb.171.2.1035-1040.1989
Bijlsma, J. J., Vandenbroucke-Grauls, C. M., Phadnis, S. H., and Kusters, J. G. (1999). Identification of virulence genes of Helicobacter pylori by random insertion mutagenesis. Infect. Immun. 67, 2433–2440. doi: 10.1128/IAI.67.5.2433-2440.1999
Burnham, P. M., and Hendrixson, D. R. (2018). Campylobacter jejuni: collective components promoting a successful enteric lifestyle. Nat. Rev. Microbiol. 16, 551–565. doi: 10.1038/s41579-018-0037-9
Carvalho, C. M., Gannon, B. W., Halfhide, D. E., Santos, S. B., Hayes, C. M., Roe, J. M., et al. (2010). The in vivo efficacy of two administration routes of a phage cocktail to reduce numbers of Campylobacter coli and Campylobacter jejuni in chickens. BMC Microbiol. 10:232. doi: 10.1186/1471-2180-10-232
Choi, Y., Shin, H., Lee, J. H., and Ryu, S. (2013). Identification and characterization of a novel flagellum-dependent Salmonella-infecting bacteriophage, iEPS5. Appl. Environ. Microbiol. 79, 4829–4837. doi: 10.1128/AEM.00706-13
Clark, C. G., and Ng, L. K. (2008). Sequence variability of Campylobacter temperate bacteriophages. BMC Microbiol. 8:49. doi: 10.1186/1471-2180-8-49
Cohen, E. J., Nakane, D., Kabata, Y., Hendrixson, D. R., Nishizaka, T., and Beeby, M. (2020). Campylobacter jejuni motility integrates specialized cell shape, flagellar filament, and motor, to coordinate action of its opposed flagella. PLoS Pathog. 16:e1008620. doi: 10.1371/journal.ppat.1008620
Coward, C., Grant, A. J., Swift, C., Philp, J., Towler, R., Heydarian, M., et al. (2006). Phase-variable surface structures are required for infection of Campylobacter jejuni by bacteriophages. Appl. Environ. Microbiol. 72, 4638–4647. doi: 10.1128/AEM.00184-06
Crippen, C. S., Lee, Y. J., Hutinet, G., Shajahan, A., Sacher, J. C., Azadi, P., et al. (2019). Deoxyinosine and 7-Deaza-2-Deoxyguanosine as carriers of genetic information in the DNA of Campylobacter viruses. J. Virol. 93, e01111–e01119. doi: 10.1128/JVI.01111-19
Deakin, W. J., Parker, V. E., Wright, E. L., Ashcroft, K. J., Loake, G. J., and Shaw, C. H. (1999). Agrobacterium tumefaciens possesses a fourth flagelin gene located in a large gene cluster concerned with flagellar structure, assembly and motility. Microbiology 145, 1397–1407. doi: 10.1099/13500872-145-6-1397
El-Shibiny, A., Scott, A., Timms, A., Metawea, Y., Connerton, P., and Connerton, I. (2009). Application of a group II Campylobacter bacteriophage to reduce strains of Campylobacter jejuni and Campylobacter coli colonizing broiler chickens. J. Food Prot. 72, 733–740. doi: 10.4315/0362-028x-72.4.733
Esteves, N. C., Porwollik, S., McClelland, M., and Scharf, B. E. (2021). The multi-drug efflux system AcrABZ-TolC is essential for infection of Salmonella typhimurium by the flagellum-dependent bacteriophage chi. J. Virol. 95, e00394–e00321. doi: 10.1128/JVI.00394-21
Esteves, N. C., and Scharf, B. E. (2022). Flagellotropic bacteriophages: opportunities and challenges for antimicrobial applications. Int. J. Mol. Sci. 23:7084. doi: 10.3390/ijms23137084
Evans, T. J., Crow, M. A., Williamson, N. R., Orme, W., Thomson, N. R., Komitopoulou, E., et al. (2010a). Characterization of a broad-host-range flagellum-dependent phage that mediates high-efficiency generalized transduction in, and between, Serratia and Pantoea. Microbiology 156, 240–247. doi: 10.1099/mic.0.032797-0
Evans, T. J., Trauner, A., Komitopoulou, E., and Salmond, G. P. (2010b). Exploitation of a new flagellatropic phage of Erwinia for positive selection of bacterial mutants attenuated in plant virulence: towards phage therapy. J. Appl. Microbiol. 108, 676–685. doi: 10.1111/j.1365-2672.2009.04462.x
Garcia-Doval, C., and van Raaij, M. J. (2012). Structure of the receptor-binding carboxy-terminal domain of bacteriophage T7 tail fibers. Proc. Natl. Acad. Sci. U.S.A. 109, 9390–9395. doi: 10.1073/pnas.1119719109
Gencay, Y. E., Sørensen, M. C. H., Wenzel, C. Q., Szymanski, C. M., and Brøndsted, L. (2018). Phase variable expression of a single phage receptor in Campylobacter jejuni NCTC12662 influences sensitivity toward several diverse CPS-dependent phages. Front. Microbiol. 9:82. doi: 10.3389/fmicb.2018.00082
Gilbert, M., Karwaski, M. F., Bernatchez, S., Young, N. M., Taboada, E., Michniewicz, J., et al. (2002). The genetic bases for the variation in the lipo-oligosaccharide of the mucosal pathogen, Campylobacter jejuni. Biosynthesis of sialylated ganglioside mimics in the core oligosaccharide. J. Biol. Chem. 277, 327–337. doi: 10.1074/jbc.M108452200
Gonzalez, F., Helm, R. F., Broadway, K. M., and Scharf, B. E. (2018). More than rotating flagella: lipopolysaccharide as a secondary receptor for flagellotropic phage 7-7-1. J. Bacteriol. 200, e00363–e00318. doi: 10.1128/JB.00363-18
Gonzalez, F., and Scharf, B. E. (2021). Identification of receptor binding proteins in flagellotropic Agrobacterium phage 7-7-1. Viruses 13:1267. doi: 10.3390/v13071267
Goon, S., Kelly, J. F., Logan, S. M., Ewing, C. P., and Guerry, P. (2003). Pseudaminic acid, the major modification on Campylobacter flagellin, is synthesized via the Cj1293 gene. Mol. Microbiol. 50, 659–671. doi: 10.1046/j.1365-2958.2003.03725.x
Guerrero-Ferreira, R. C., Viollier, P. H., Ely, B., Poindexter, J. S., Georgieva, M., Jensen, G. J., et al. (2011). Alternative mechanism for bacteriophage adsorption to the motile bacterium Caulobacter crescentus. Proc. Natl. Acad. Sci. USA 108, 9963–9968. doi: 10.1073/pnas.1012388108
Haiko, J., and Westerlund-Wikström, B. (2013). The role of the bacterial flagellum in adhesion and virulence. Biology 2, 1242–1267. doi: 10.3390/biology2041242
Hammerl, J. A., Jäckel, C., Alter, T., Janzcyk, P., Stingl, K., Knüver, M. T., et al. (2014). Reduction of Campylobacter jejuni in broiler chicken by successive application of group II and group III phages. PLoS One 9:e114785. doi: 10.1371/journal.pone.0114785
Holm, L. (2022). Dali server: structural unification of protein families. Nucleic Acids Res. 50, W210–W215. doi: 10.1093/nar/gkac387
Igwaran, A., and Okoh, A. I. (2019). Human campylobacteriosis: a public health concern of global importance. Heliyon 5:e02814. doi: 10.1016/j.heliyon.2019.e02814
Iino, T., and Mitani, M. (1967). Infection of Serratia marcescens by bacteriophage chi. J. Virol. 1, 445–447. doi: 10.1128/JVI.1.2.445-447.1967
Javed, M. A., Ackermann, H. W., Azeredo, J., Carvalho, C. M., Connerton, I., Evoy, S., et al. (2014). A suggested classification for two groups of Campylobacter myoviruses. Arch. Virol. 159, 181–190. doi: 10.1007/s00705-013-1788-2
Johnson, M., Zaretskaya, I., Raytselis, Y., Merezhuk, Y., McGinnis, S., and Madden, T. L. (2008). NCBI BLAST: a better web interface. Nucleic Acids Res. 36, W5–W9. doi: 10.1093/nar/gkn201
Karlyshev, A. V., Champion, O. L., Churcher, C., Brisson, J. R., Jarrell, H. C., Gilbert, M., et al. (2005a). Analysis of Campylobacter jejuni capsular loci reveals multiple mechanisms for the generation of structural diversity and the ability to form complex heptoses. Mol. Microbiol. 55, 90–103. doi: 10.1111/j.1365-2958.2004.04374.x
Karlyshev, A. V., Ketley, J. M., and Wren, B. W. (2005b). The Campylobacter jejuni glycome. FEMS Microbiol. Rev. 29, 377–390. doi: 10.1016/j.femsre.2005.01.003
Kim, J. S., Artymovich, K. A., Hall, D. F., Smith, E. J., Fulton, R., Bell, J., et al. (2012). Passage of Campylobacter jejuni through the chicken reservoir or mice promotes phase variation in contingency genes Cj0045 and Cj0170 that strongly associates with colonization and disease in a mouse model. Microbiology 158, 1304–1316. doi: 10.1099/mic.0.057158-0
Linton, D., Gilbert, M., Hitchen, P. G., Dell, A., Morris, H. R., Wakarchuk, W. W., et al. (2000). Phase variation of a beta-1,3 galactosyltransferase involved in generation of the ganglioside GM1-like lipo-oligosaccharide of Campylobacter jejuni. Mol. Microbiol. 37, 501–514. doi: 10.1046/j.1365-2958.2000.02020.x
Lotz, W., Acker, G., and Schmitt, R. (1977). Bacteriophage 7-7-1 adsorbs to the complex flagella of Rhizobium lupini H13-3. J. Gen. Virol. 34, 9–17. doi: 10.1099/0022-1317-34-1-9
McNally, D. J., Lamoureux, M. P., Karlyshev, A. V., Fiori, L. M., Li, J., Thacker, G., et al. (2007). Commonality and biosynthesis of the O-methyl phosphoramidate capsule modification in Campylobacter jejuni. J. Biol. Chem. 282, 28566–28576. doi: 10.1074/jbc.M704413200
Mirdita, M., Schütze, K., Moriwaki, Y., Heo, L., Ovchinnikov, S., and Steinegger, M. (2022). ColabFold: making protein folding accessible to all. Nat. Methods 19, 679–682. doi: 10.1038/s41592-022-01488-1
Mitchell, A. L., Attwood, T. K., Babbitt, P. C., Blum, M., Bork, P., Bridge, A., et al. (2019). InterPro in 2019: improving coverage, classification and access to protein sequence annotations. Nucleic Acids Res. 47, D351–D360. doi: 10.1093/nar/gky1100
Parker, C. T., Quiñones, B., Miller, W. G., Horn, S. T., and Mandrell, R. E. (2006). Comparative genomic analysis of Campylobacter jejuni strains reveals diversity due to genomic elements similar to those present in C. jejuni strain RM1221. J. Clin. Microbiol. 44, 4125–4135. doi: 10.1128/JCM.01231-06
Parkhill, J., Wren, B. W., Mungall, K., Ketley, J. M., Churcher, C., Basham, D., et al. (2000). The genome sequence of the food-borne pathogen Campylobacter jejuni reveals hypervariable sequences. Nature 403, 665–668. doi: 10.1038/35001088
Pate, J. L., Petzold, S. J., and Umbreit, T. H. (1979). Two flagellotropic phages and one pilus-specific phage active against Asticcacaulis biprosthecum. Virology 94, 24–37. doi: 10.1016/0042-6822(79)90435-5
Raimondo, L. M., Lundh, N. P., and Martinez, R. J. (1968). Primary adsorption site of phage PBS1: the flagellum of Bacillus subtilis. J. Virol. 2, 256–264. doi: 10.1128/JVI.2.3.256-264.1968
Ravid, S., and Eisenbach, M. (1983). Correlation between bacteriophage chi adsorption and mode of flagellar rotation of Escherichia coli chemotaxis mutants. J. Bacteriol. 154, 604–611. doi: 10.1128/jb.154.2.604-611.1983
Ribot, E. M., Fitzgerald, C., Kubota, K., Swaminathan, B., and Barrett, T. J. (2001). Rapid pulsed-field gel electrophoresis protocol for subtyping of Campylobacter jejuni. J. Clin. Microbiol. 39, 1889–1894. doi: 10.1128/JCM.39.5.1889-1894.2001
Samuel, A. D., Pitta, T. P., Ryu, W. S., Danese, P. N., Leung, E. C., and Berg, H. C. (1999). Flagellar determinants of bacterial sensitivity to chi-phage. Proc. Natl. Acad. Sci. USA 96, 9863–9866. doi: 10.1073/pnas.96.17.9863
Schade, S. Z., Adler, J., and Ris, H. (1967). How bacteriophage chi attacks motile bacteria. J. Virol. 1, 599–609. doi: 10.1128/JVI.1.3.599-609.1967
Schoenhofen, I. C., Young, N. M., and Gilbert, M. (2017). Biosynthesis of legionaminic acid and its incorporation into glycoconjugates. Methods Enzymol. 597, 187–207. doi: 10.1016/bs.mie.2017.06.042
Semchenko, E. A., Day, C. J., Moutin, M., Wilson, J. C., Tiralongo, J., and Korolik, V. (2012). Structural heterogeneity of terminal glycans in Campylobacter jejuni lipooligosaccharides. PLoS One 7:e40920. doi: 10.1371/journal.pone.0040920
Sørensen, M. C. H., Gencay, Y. E., Birk, T., Baldvinsson, S. B., Jäckel, C., Hammerl, J. A., et al. (2015). Primary isolation strain determines both phage type and receptors recognised by Campylobacter jejuni bacteriophages. PLoS One 10:e0116287. doi: 10.1371/journal.pone.0116287
Sørensen, M. C. H., Gencay, Y. E., Fanger, F., Chichkova, M. A. T., Mazúrová, M., Klumpp, J., et al. (2021b). Identification of novel phage resistance mechanisms in Campylobacter jejuni by comparative genomics. Front. Microbiol. 12:780559. doi: 10.3389/fmicb.2021.780559
Sørensen, M. C. H., van Alphen, L. B., Fodor, C., Crowley, S. M., Christensen, B. B., Szymanski, C. M., et al. (2012). Phase variable expression of capsular polysaccharide modifications allows Campylobacter jejuni to avoid bacteriophage infection in chickens. Front. Cell. Infect. Microbiol. 2:11. doi: 10.3389/fcimb.2012.00011
Sørensen, M. C. H., van Alphen, L. B., Harboe, A., Li, J., Christensen, B. B., Szymanski, C. M., et al. (2011). Bacteriophage F336 recognizes the capsular phosphoramidate modification of Campylobacter jejuni NCTC11168. J. Bacteriol. 193, 6742–6749. doi: 10.1128/JB.05276-11
Sørensen, M. C. H., Vitt, A., Neve, H., Soverini, M., Ahern, S. J., Klumpp, J., et al. (2021a). Campylobacter phages use hypermutable polyG tracts to create phenotypic diversity and evade bacterial resistance. Cell Rep. 35:109214. doi: 10.1016/j.celrep.2021.109214
Squeglia, F., Maciejewska, B., Łątka, A., Ruggiero, A., Briers, Y., Drulis-Kawa, Z., et al. (2020). Structural and functional studies of a Klebsiella phage capsule depolymerase tailspike: mechanistic insights into capsular degradation. Structure 28, 613–624.e4. doi: 10.1016/j.str.2020.04.015
Szymanski, C. M., Michael, F. S., Jarrell, H. C., Li, J., Gilbert, M., Larocque, S., et al. (2003). Detection of conserved N-linked glycans and phase-variable lipooligosaccharides and capsules from Campylobacter cells by mass spectrometry and high resolution magic angle spinning NMR spectroscopy. J. Biol. Chem. 278, 24509–24520. doi: 10.1074/jbc.M301273200
Tanoeiro, L., Oleastro, M., Nunes, A., Marques, A. T., Duarte, S. V., Gomes, J. P., et al. (2022). Cryptic prophages contribution for Campylobacter jejuni and Campylobacter coli introgression. Microorganisms 10:516. doi: 10.3390/microorganisms10030516
Tao, Y., Strelkov, S. V., Mesyanzhinov, V. V., and Rossmann, M. G. (1997). Structure of bacteriophage T4 fibritin: a segmented coiled coil and the role of the C-terminal domain. Structure 5, 789–798. doi: 10.1016/s0969-2126(97)00233-5
Thomassen, E., Gielen, G., Schütz, M., Schoehn, G., Abrahams, J. P., Miller, S., et al. (2003). The structure of the receptor-binding domain of the bacteriophage T4 short tail fibre reveals a knitted trimeric metal-binding fold. J. Mol. Biol. 331, 361–373. doi: 10.1016/s0022-2836(03)00755-1
van der Woude, M. W. (2011). Phase variation: how to create and coordinate population diversity. Curr. Opin. Microbiol. 14, 205–211. doi: 10.1016/j.mib.2011.01.002
van Raaij, M. J., Schoehn, G., Burda, M. R., and Miller, S. (2001). Crystal structure of a heat and protease-stable part of the bacteriophage T4 short tail fibre. J. Mol. Biol. 314, 1137–1146. doi: 10.1006/jmbi.2000.5204
Yen, J. Y., Broadway, K. M., and Scharf, B. E. (2012). Minimum requirements of flagellation and motility for infection of Agrobacterium sp. strain H13-3 by flagellotropic bacteriophage 7-7-1. Appl. Environ. Microbiol. 78, 7216–7222. doi: 10.1128/AEM.01082-12
Yuki, N., Susuki, K., Koga, M., Nishimoto, Y., Odaka, M., Hirata, K., et al. (2004). Carbohydrate mimicry between human ganglioside GM1 and Campylobacter jejuni lipooligosaccharide causes Guillain–Barre syndrome. Proc. Natl. Acad. Sci. USA 101, 11404–11409. doi: 10.1073/pnas.0402391101
Zampara, A., Sørensen, M. C. H., Gencay, Y. E., Grimon, D., Kristiansen, S. H., Jørgensen, L. S., et al. (2021). Developing Innolysins against Campylobacter jejuni using a novel prophage receptor-binding protein. Front. Microbiol. 12:619028. doi: 10.3389/fmicb.2021.619028
Zebian, N., Merkx-Jacques, A., Pittock, P. P., Houle, S., Dozois, C. M., Lajoie, G. A., et al. (2016). Comprehensive analysis of flagellin glycosylation in Campylobacter jejuni NCTC 11168 reveals incorporation of legionaminic acid and its importance for host colonization. Glycobiology 26, 386–397. doi: 10.1093/glycob/cwv104
Zhilenkov, E. L., Popova, V. M., Popov, D. V., Zavalsky, L. Y., Svetoch, E. A., Stern, N. J., et al. (2006). The ability of flagellum-specific Proteus vulgaris bacteriophage PV22 to interact with Campylobacter jejuni flagella in culture. Virol. J. 3:50. doi: 10.1186/1743-422X-3-50
Keywords: Campylobacter, phage, phage receptor, receptor binding protein, Fletchervirus, flagella, flagellotropic phage, phage resistance
Citation: Ostenfeld LJ, Sørensen AN, Neve H, Vitt A, Klumpp J and Sørensen MCH (2024) A hybrid receptor binding protein enables phage F341 infection of Campylobacter by binding to flagella and lipooligosaccharides. Front. Microbiol. 15:1358909. doi: 10.3389/fmicb.2024.1358909
Received: 20 December 2023; Accepted: 18 January 2024;
Published: 01 February 2024.
Edited by:
Hyunjin Yoon, Ajou University, Republic of KoreaReviewed by:
Swapnil Ganesh Sanmukh, Université Clermont Auvergne, FranceCopyright © 2024 Ostenfeld, Sørensen, Neve, Vitt, Klumpp and Sørensen. This is an open-access article distributed under the terms of the Creative Commons Attribution License (CC BY). The use, distribution or reproduction in other forums is permitted, provided the original author(s) and the copyright owner(s) are credited and that the original publication in this journal is cited, in accordance with accepted academic practice. No use, distribution or reproduction is permitted which does not comply with these terms.
*Correspondence: Martine Camilla Holst Sørensen, bWNwQHN1bmQua3UuZGs=
†Retired from Max-Rubner Institut
Disclaimer: All claims expressed in this article are solely those of the authors and do not necessarily represent those of their affiliated organizations, or those of the publisher, the editors and the reviewers. Any product that may be evaluated in this article or claim that may be made by its manufacturer is not guaranteed or endorsed by the publisher.
Research integrity at Frontiers
Learn more about the work of our research integrity team to safeguard the quality of each article we publish.