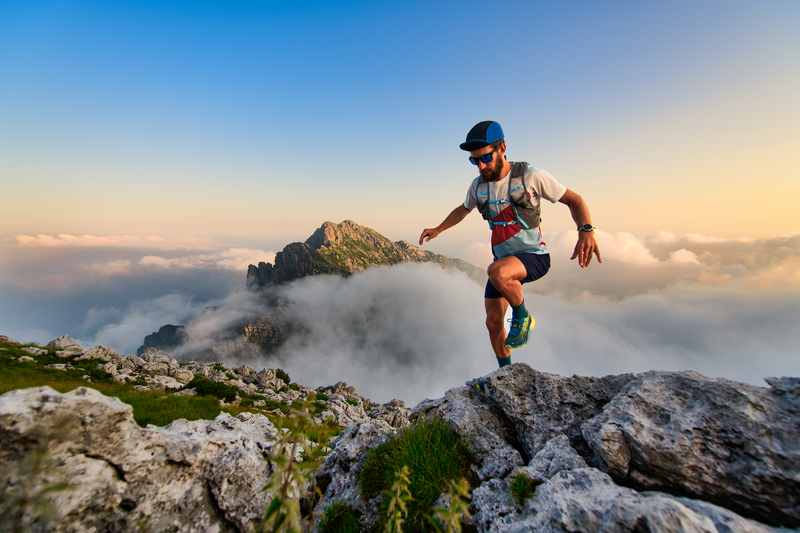
94% of researchers rate our articles as excellent or good
Learn more about the work of our research integrity team to safeguard the quality of each article we publish.
Find out more
REVIEW article
Front. Microbiol. , 24 January 2024
Sec. Infectious Agents and Disease
Volume 15 - 2024 | https://doi.org/10.3389/fmicb.2024.1345027
This article is part of the Research Topic Insights in Infectious Agents and Disease: 2023/2024 View all 33 articles
Otitis media is an inflammatory disorder of the middle ear caused by airways-associated bacterial or viral infections. It is one of the most common childhood infections as globally more than 80% of children are diagnosed with acute otitis media by 3 years of age and it is a common reason for doctor’s visits, antibiotics prescriptions, and surgery among children. Otitis media is a multifactorial disease with various genetic, immunologic, infectious, and environmental factors predisposing children to develop ear infections. Streptococcus pneumoniae, Haemophilus influenzae, and Moraxella catarrhalis are the most common culprits responsible for acute otitis media. Despite the massive global disease burden, the pathogenesis of otitis media is still unclear and requires extensive future research. Antibiotics are the preferred treatment to cure middle ear infections, however, the antimicrobial resistance rate of common middle ear pathogens has increased considerably over the years. At present, pneumococcal and influenza vaccines are administered as a preventive measure against otitis media, nevertheless, these vaccines are only beneficial in preventing carriage and/or disease caused by vaccine serotypes. Otitis media caused by non-vaccine serotype pneumococci, non-typeable H. influenza, and M. catarrhalis remain an important healthcare burden. The development of multi-species vaccines is an arduous process but is required to reduce the global burden of this disease. Many novel vaccines against S. pneumoniae, non-typeable H. influenza, and M. catarrhalis are in preclinical trials. It is anticipated that these vaccines will lower the disease burden and provide better protection against otitis media. To study disease pathology the rat, mouse, and chinchilla are commonly used to induce experimental acute otitis media to test new therapeutics, including antibiotics and vaccines. Each of these models has its advantages and disadvantages, yet there is still a need to develop an improved animal model providing a better correlated mechanistic understanding of human middle ear infections, thereby underpinning the development of more effective otitis media therapeutics. This review provides an updated summary of current vaccines against otitis media, various animal models of otitis media, their limitations, and some future insights in this field providing a springboard in the development of new animal models and novel vaccines for otitis media.
Otitis media (OM) is inflammation within the middle ear (ME), most frequently occurring in children. OM is caused by a variety of factors including bacterial or viral infections, allergy, Eustachian tube (ET) malfunction, physiological/immunological/pathological factors within the ME, as well as genetic and environmental factors (Yeo, 2018). Clinical manifestations of OM vary, depending on the type of exudate and the duration of the disease (Lieberthal et al., 2013). Acute OM (AOM) is the usual symptomatic presentation caused by an ongoing viral or bacterial infection accompanying fever, lethargy, otorrhea, irritability, vomiting, diarrhea, and in severe cases hearing loss. There is often a connection between AOM, and recent upper respiratory tract infections (URTI) caused by bacteria or viruses, in part because the ME is contiguous with URT via the ET. The less-developed ET/ME anatomy of young children is suggested as one factor that predisposes them to AOM (Toll and Nunez, 2012). ME disease recurs in many children, termed recurrent AOM (rAOM) (Granath, 2017). Some children are prone to develop AOM mainly due to immune dysfunctions. If they develop 4 AOM episodes in 6 months or 4 episodes in 12 months period, they are defined as otitis-prone children (Alho et al., 1991; Pichichero, 2020). Non-purulent fluid accumulation behind the tympanic membrane is a sign of OM with effusion (OME) which is often not associated with pain and may occur following AOM, rAOM, or other upper respiratory infections (Rosenfeld et al., 2016). OME makes children more prone to bacterial and viral infections. If it persists for extended periods, resulting in chronic OM (COM) (Granath, 2017), it can result in damage to the ME and conductive hearing loss. This is especially important for preschool children whose speech development may be delayed by this condition (Simon et al., 2018). The typical characteristics of COM are ear pain, ear discharge, and hearing impairment which may persist even after surgery, leading to many restrictions in daily life (Lucidi et al., 2022). Pro-inflammatory cytokines like TNF-α and IL-1, produced to combat bacterial infection during an AOM episode, set up an inflammatory response leading to the evolution of COM, which may include epithelial remodeling (Juhn et al., 2008). However, the exact mechanisms leading to COM are still poorly understood. Chronic suppurative OM (CSOM) is characterized by persistent or recurrent ear drainage (otorrhoea) for more than 2–6 weeks. During CSOM, bacterial pathogens may also infect the mucosa of the ME through the external canal (Jensen et al., 2017).
It is estimated that nearly 80% of young children (6–24 months old) suffer from at least one episode of OM each year (Tong et al., 2018). Its high prevalence in infants and children, despite low associated mortality, makes it a major health burden worldwide as it poses a substantial impact on the development of children, their families, and the health care system. OM is a frequent cause of visiting doctors, antibiotic prescriptions, and surgery among children (Coker et al., 2010) and the annual cost of OM to the healthcare system has been estimated to be more than $5 billion in the United States (Rovers, 2008) and $100 – $400 million in Australia (Kong and Coates, 2009). According to the World Health Organization (WHO), nearly 28 thousand people die due to OM globally, and nearly 50% of permanent hearing loss cases are caused by OM (Acuin, 2004). Indigenous populations across the world are at high risk for OM including Native Americans, the Alaskan, Canadian and Greenland Inuit, and Australian Aborigines (Bluestone, 1998; Daly et al., 2010).
The etiology of OM is often polymicrobial where the most common bacterial pathogens associated with AOM are Streptococcus pneumoniae (S. pneumoniae), non-typeable Haemophilus influenzae (NTHi), and Moraxella catarrhalis (Mcat). Contribution to disease by each pathogen varies by study and detection method [(see Tamir et al., 2023] for review of recent reports of microbiology of OM). The viruses that are commonly associated with OM include respiratory syncytial virus (RSV), coronaviruses, influenza viruses, adenoviruses, human metapneumovirus, human bocavirus, and picornaviruses (Pettigrew et al., 2011; Ngo et al., 2022). The most commonly associated pathogens with CSOM are Staphylococcus aureus and Pseudomonas aeruginosa (Afolabi et al., 2012; Khattak et al., 2017). Fungi and anaerobic bacteria have also been associated with OM/CSOM (Prakash et al., 2013). The anaerobic bacteria associated with OM/CSOM are Prevotella melaninogenica, Clostridium spp., Peptococcus spp., and Fusobacterium spp. (Brook, 2008). Bacteria that are commonly associated with OME are coagulase-negative staphylococci, Veillonella spp., and S. aureus (Daniel et al., 2012). Viral infections predispose the host to bacterial infections of the ME, particularly influenza A virus, coronavirus, and respiratory syncytial virus (RSV) [reviewed by (Marom et al., 2012)]. For the development of OM when the tympanic membrane is intact, the pathogen needs to establish nasopharyngeal colonization by gaining access to the tympanic cavity via the ET, as illustrated in Figure 1.
Figure 1. Anatomy of the human ear and pathogenesis of acute otitis media. The human ear is composed of three parts: the outer ear, the middle ear, and the inner ear. The tympanic membrane (eardrum) separates the outer ear, from the middle ear. The middle ear is composed of the middle ear cavity and the middle ear bones, which are attached to the tympanic membrane. The Eustachian tube connects the middle ear cavity to the nasopharynx. A viral or bacterial upper respiratory tract infection initiates inflammation of the nasopharynx and the Eustachian tube leading to the blockage of the latter trapping fluid inside the middle ear, leading to increased adherence and colonization of bacteria. Eustachian tube dysfunction also leads to negative middle ear pressure, allowing bacteria and/or viruses in the nasopharynx to move into the middle ear causing infection and inflammation.
The ME mucosa, like other mucosal surfaces in the body, has a local immune system that plays a critical role in protecting against infections. The ME mucosa and URT are connected anatomically, and their immune systems are interconnected as well (Massa et al., 2021; Kurono, 2022). To prevent OM, enhancement of mucosal immune responses in the nasopharynx (NP) and ME is desired, however, how the immune protection in the ME mucosa relates to URT is still poorly understood.
Antibiotics are frequently used as a treatment strategy for OM, nonetheless, high morbidity and the alarming rise in antibiotic resistance over the years have increased interest in the development of alternative strategies such as vaccination. The development of several vaccines has been achieved to combat disease caused by S. pneumoniae, such as a 14-valent pneumococcal polysaccharide vaccine (1977), the 23-valent pneumococcal polysaccharide vaccine (1983), a 7-valent pneumococcal conjugate vaccine (2000), and a 13-valent pneumococcal conjugate vaccine (2010) (Grabenstein and Klugman, 2012). Although these vaccines are effective against OM, they do however present the risk of further serotype replacement (Weinberger et al., 2011; Lo et al., 2019). Pneumococcal vaccine development has received priority because pneumococcus causes life-threatening pneumonia and invasive diseases, including sepsis and meningitis. Vaccines against NTHi and Mcat, however, are not currently available, despite both species causing significant morbidity through OM, pneumonia, exacerbations of chronic lung disease, and other diseases of respiratory mucosa in adults and children. Advances have been made in the development of vaccines targeting the major otopathogens, and many potential vaccine antigens from these bacteria have been investigated. This review aims to offer an updated summary of candidate vaccine antigens, including their putative or known functions. Furthermore, it also discusses currently used animal models of OM, which are crucial for relevant pre-clinical assessment of candidate antigens and vaccine formulations.
An ideal vaccine antigen will possess some essential features leading to broad protection against the target pathogen. First, the vaccine antigen needs to be conserved among clinically relevant strains so that it can provide the necessary coverage (Murphy, 2005). The majority of bacterial otopathogens are highly adapted to the colonization of the human URT and are frequent asymptomatic colonizers. As such, surface-exposed regions are under immune-pressure. Many surface-exposed epitopes have variable sequences between strains or are expressed at different levels between strains, and this inter-strain variability needs to be known (Karls and Perkins-Balding, 2013). If a targeted gene is not present in all strains, or it is antigenically variable between strains, then one or more additional vaccine candidates may be required for optimal protection. The development of multi-protein vaccines is becoming increasingly recognized as necessary for pathogens with highly variable antigens between and within strains (Watson et al., 2019). However, such formulations can lead to antigenic competition, or masking of key antigenic epitopes leading to compromised immune responses to one or more antigens (Michel et al., 2022). This requires analysis of antigens and responses in animal and human trials.
Secondly, it is highly desirable that the antigen is exposed on the surface of the pathogen so that this region can be recognized by the antibodies. Ideally, the antigen is essential for colonization or pathogenesis, and its function is known (Murphy, 2005). Moreover, if the candidate vaccine has some toxicity, genetic or chemical modification will be required (Rappuoli, 1999). Importantly, it is necessary to establish if there is a risk of autoimmunity, e.g., if there is significant sequence or structural similarity to host antigens (Kraus et al., 1989). Most importantly, the vaccine antigen needs to be immunogenic in the host and elicit a lasting protective immune response upon immunization, induced by humoral or cellular immunity (Murphy, 2005).
During the last few decades, both computational and experimental methods have been utilized to identify potential vaccine antigens for major pathogens involved in OM. In this review, we focus on those potential antigens that have progressed substantially as vaccine candidates and show potential for inclusion in vaccines that will reduce the burden of OM.
Streptococcus pneumoniae is naturally found in the human NP, and this nasopharyngeal carriage is important for active infection and transmission of the pathogen (McDaniel and Swiatlo, 2004). Its capsular polysaccharides (CPSs) are important virulence factors, especially for invasive disease that can be classified into 100 serotypes, based on chemical and immunological profiles (Ganaie et al., 2020). The most identified pathogen in OM following culture is S. pneumoniae (Tamir et al., 2023).
Currently, two types of pneumococcal vaccines are available. Both are based on CPS: (A) the 23-valent pneumococcal polysaccharide-based vaccine (PPV-23); and (B) the 7-, 10-, 13-, and 20-valent pneumococcal conjugate vaccines (PCV-7, −10, −13, −20).
PPV23 is based on the CPS antigens from 23 different serotypes of S. pneumoniae and is recommended for individuals aged 65 and older, as well as those aged 2 to 64 years who have multiple chronic conditions, such as diabetes and chronic cardiovascular disease (CDC, 2019). Most bacterial CPS are poorly immunogenic in young children, in part because they are T-cell independent antigens and induce little immune memory. Newborns and infants up to the age of 1.5–2 years of age are unable to produce antibodies to bacterial CPS due to less developed immunity (Rijkers et al., 1998), therefore, there is much debate surrounding the use of pneumococcal polysaccharide vaccines in young children (Borrow et al., 2012). In PCVs, the pneumococcal polysaccharide is coupled to a carrier protein to convert the antigens from T-cell independent to T-cell dependent antigens for enhanced immunogenicity (Pichichero, 2013). PCVs have been incorporated into the national immunization programs of 148 out of 194 World Health Organization (WHO) member states as of the end of 2020 (CDC, 2020). There has been a decline in OM and NP colonization by vaccine serotypes of S. pneumoniae with these vaccines, however, their use has been linked with replacement by non-vaccine serotypes of S. pneumoniae as well as NTHi and Mcat (Barenkamp et al., 2017; Kaur et al., 2017; Ben-Shimol et al., 2019; Pereira et al., 2023). Pneumococcal serotype replacement after vaccination has been noted for both childhood carriage (Lee et al., 2017) and in AOM (Gene et al., 2013), and in some cases the replacing strains are antibiotic resistant (Lo et al., 2019), so that vaccination may change the distribution of resistance and potential treatment failures not only for URTI/OM but also for pneumonia or invasive disease. A study in Israel evaluated the efficacy of PCV7 and PCV13 and reported that these vaccines reduced pneumococcal AOM by 77%, however, an increase in AOM cases due to non-vaccine serotypes was observed (Ben-Shimol et al., 2014). Similarly, a Costa Rican study of AOM in children reported that pneumococcus had a lower frequency and NTHi cultured at a higher frequency in vaccinated vs. unvaccinated children (Abdelnour et al., 2015). A 14-year observational study on the effects of PCV7, PCV10, and PCV13 in Swedish preschool children showed that Mcat and NTHi were proportionally more common than S. pneumoniae after PCV introduction in NP culture of PCV-immunized children with URTI (Littorin et al., 2021). A global surveillance study that used whole-genome sequencing for analysis reported that non-PCV serotypes15B and 15C (15B/C), 12F, and 35B/D were among the most prevalent pneumococcal serotypes in the post-PCV13 era (Lo et al., 2019). In Italy, non-vaccine serotype 24F has been reported as a prevalent serotype in pneumococcal otorrhea cases (Sings et al., 2019). Such epidemiological studies of pneumococcal serotypes from both carriage and OM inform future changes to polyvalent vaccines. Notably, PCV-20 includes several recently emergent serotypes (1, 3, 4, 5, 6A, 6B, 7F, 8, 9 V, 10A, 11A, 12F, 14, 15B, 18C, 19A, 19F, 22F, 23F, and 33F) and is now approved for use in all age groups in the United States (ACIP, 2023).
As there are at least 100 pneumococcus serotypes, increasing the number of vaccine serotypes in the PCV vaccines is more complex and expensive. In summary, both PPV and PCVs induce serotype-specific immunity and their usage has been linked with the emergence of replacement serotypes (Weinberger et al., 2011; McElligott et al., 2015; Kaur et al., 2016; Kawaguchiya et al., 2017). Nonetheless, the success of PCVs in reducing childhood mortality and morbidity due to pneumonia and invasive disease is significant, for example, see a recent review of pneumococcal disease in children in the United States (Walter and Smith, 2022).
Despite the success of PCVs, the challenge of regular re-formulation, and the theoretical and practical challenges of including more serotypes means the search for protein antigens has continued, aimed at developing pneumococcal vaccines targeting conserved and universally present pneumococcal proteins to complement or ultimately substitute PCVs. A protein-based vaccine would theoretically be simpler and cheaper to make than a conjugate vaccine. Several protein antigens have been investigated in human trials, individually, and in multivalent formulations. Table 1 summarizes antigens and vaccine candidates for pneumococcal diseases [Also recently reviewed by (Duke and Avci, 2023)].
Pneumolysin (Ply) is a cholesterol-dependent cytolysin that has haemolytic properties, and aids in bacterial pathogenesis and infection. Vaccination with a detoxified or non-toxic form of Ply (dPly) is known to confer protection against multiple serotypes in animal models (Alexander et al., 1994; Ogunniyi et al., 2001; Denoël et al., 2011). A genetically mutated pneumolysin derivative (PlyD1) was safe, robustly immunogenic, and induced neutralizing antibody responses in a phase 1 trial (NCT01444352) (Kamtchoua et al., 2013). More recently, a genetic toxoid of Ply with two amino acid mutations (G293S and L460D) termed Ply-D protected mice from the lethal challenge of various clinical pneumococcal isolates (Thanawastien et al., 2021).
Pneumococcal histidine triad protein D (PhtD) is a highly conserved adhesin protein. The PhtD-based vaccines protect immunized mice against NP and lung colonization of pneumococcus (Khan and Pichichero, 2012; Kaur et al., 2014b; Brookes et al., 2015; Ochs et al., 2016). Combining PlyD1 and PhtD, and including PHiD-10 (conjugated to protein D of H. influenzae), Odutola et al. (2016, 2017, 2019) reported on phase 2 trials in African children (NCT01262872), while the same vaccine was assessed in toddlers in Czech republic for safety, immunogenicity, and non-inferiority to PCV-10 (Urbancikova et al., 2017). None of these trials assessed protection against OM. Pneumococcal surface protein A (PspA) is a choline-binding protein that is located on the cell surface in approximately all pneumococcus strains and is involved in the complement-mediated clearance of bacteria. PspA was shown to be safe during phase I clinical trials (Briles et al., 2000b). However, PspA shows homology to the human cardiac myosin and must be modified to prevent possible autoimmune disease or cardiac inflammation (Ginsburg et al., 2012). PspA recently underwent phase 1a clinical trial as part of a multi-element vaccine alongside PlyD (NCT04087460, 2022). Pneumococcal choline-binding protein A (PcpA) is a surface-exposed protein that is conserved among different pneumococcal strains (Glover et al., 2008). A bivalent PcpA/PhtD vaccine was immunogenic and safe in humans during a phase 1 clinical study (NCT01444339) (Bologa et al., 2012), and induced functionally relevant antibodies (Ochs et al., 2016). A trivalent vaccine containing recombinant PcpA, PhtD, and PlyD1 (designed from serotype 6B) protected infant mice from the serotype 6A challenge (Verhoeven et al., 2014). This vaccine formulation was considered safe and immunogenic in a phase 1 study including adults, toddlers, and infants (NCT01764126) (Brookes et al., 2015). Furthermore, serum IgG antibody responses against PhtD, PcpA, and PlyD1 were in synchrony, indicating they are similarly immunogenic and therefore compatible with combining in a trivalent protein vaccine (Ren et al., 2015).
Another serotype-independent pneumococcal protein vaccine candidate called PnuBioVax™ is expected to offer broader coverage at a low unit price. This vaccine is formulated by fermenting a genetically modified S. pneumoniae TIGR cell substrate. Following harvesting, the protein antigens are detergent-extracted and purified using ion exchange chromatography (Cecchini et al., 2015). During phase 1 human trials (NCT02572635), this vaccine demonstrated good safety and immunogenicity profile (Entwisle et al., 2017; Hill et al., 2018).
Other antigens have been investigated in animal models and show promise for inclusion in a multivalent protein vaccine. Kong et al. developed a PspA vaccine with an intranasal vaccine delivery system based on a nanometre-sized hydrogel (nanogel) composed of a cationic cholesteryl group-bearing pullulan (cCHP). Intranasal vaccination with the cCHP-PspA vaccine protected mice from a lethal S. pneumoniae Xen10 challenge by reducing the invasion and colonization of bacteria in the upper and lower respiratory tracts (Kong et al., 2013). Wang et al. utilized a bacterium-like particle (BLP) delivery system designed to express PspA on the BLP surface. Intranasal delivery of this vaccine-induced PcpA-specific IgG and IgA antibodies in mice and protected the animals from a lethal challenge with S. pneumoniae (Wang et al., 2018). A recombinant PspA-based protein vaccine (PspAB1-5) consisting of the B region fragments from clades 1 to 5 of both families resulted in improved C3 complement component depositioning in immunized mice. The antibodies induced were cross-reactive against pneumococci from clades 1, 2, and 5. Consequently, the PspA vaccines based on the B region of all clades may be able to provide better protection against S. pneumoniae (Akbari et al., 2019).
Elongation factor Tu (EF-Tu) is a universally expressed surface protein that is highly conserved in different S. pneumoniae. EF-Tu has chaperone activity and mediates peptide biosynthesis, protein folding, and cellular stress response (Gregersen and Bross, 2010). A vaccine containing recombinant EF-Tu from S. pneumoniae strain D39 protected immunized mice against lethal challenges with serotype 2 and a multidrug-resistant serotype 15A, and increased the levels of cytokines including TNFα, IL-6, IL-17, and IFN-γ. The anti-EF-Tu serum demonstrated an enhanced phagocytic activity against S. pneumoniae, irrespective of its serotypes (Nagai et al., 2019). Hence, EF-Tu presents as a potential broad-spectrum vaccine candidate against common pneumococcal serotypes. LytB is an endo-β-N acetylglucosaminidase involved in the formation of biofilm, separation of daughter cells, and pathogenesis (Bai et al., 2014). An anti-LytB antiserum demonstrated significant protection in mice from a lethal pneumococcal challenge (Wizemann et al., 2001). In another study mice immunized with LytB showed enhanced complement-mediated immunity against various pneumococcal serotypes. Anti-LytB serum-stimulated neutrophil-mediated phagocytosis against S. pneumoniae (Corsini et al., 2016). Thus, LytB could be an effective antigen in a pneumococcus vaccine.
In an interesting approach, Mann et al. fused peptides of choline-binding protein A (CbpA) with a non-toxic Ply (L460D). In mice, this fusion was more effective than L460D at reducing nasal colonization, OM, pneumonia, OM, and meningitis (Mann et al., 2014).
Shi et al. developed three live recombinant Salmonella Typhi vectors expressing PspA pneumococcal protein. These live vectors were shown to be highly immunogenic in mice and highly susceptible to killing in human blood (Shi et al., 2010). In a phase I clinical trial, administration of these live attenuated Salmonella strains was safe and well-tolerated in healthy adult subjects. The immunogenicity profile of these live vectors was limited perhaps because of pre-existing cross-reactive antibodies, therefore further genetic manipulation is needed to develop a candidate with improved immunogenicity (Frey et al., 2013).
Whole-cell vaccines are an attractive alternative to polysaccharide-based vaccines because they take advantage of whole-cells expressing various protein antigens without involving the purification of individual antigens. Live-attenuated or killed whole-cell vaccines from unencapsulated S. pneumoniae can provide serotype-independent protection in animal models. One caveat is that use of live attenuated strains must be used cautiously in populations with a risk of impaired or sub-optimal immune status. The pneumococcal whole-cell vaccine (wSp) consists of a killed, unencapsulated pneumococcal strain delivered with an alum-based adjuvant. The purpose was to provide a cost-effective vaccine with broader coverage. In phase 1 studies in healthy adults in the United States (NCT01537185), the vaccine demonstrated a good safety, tolerability, and immunogenicity profile (Keech et al., 2020). It has also been tested in phase 1 and 2 trials in healthy adults and toddlers in Kenya to evaluate its safety and immunogenicity which was reported to be satisfactory (NCT02543892). Although it has not been assessed for its efficacy in the human paediatric OM population, a subcutaneous dose of wSp decreased the density of pneumococcus in the ME of mice, however, it could not protect from S. pneumoniae-induced OM (Manning et al., 2019). A whole-cell vaccine consisting of an ethanol-killed capsule-deficient S. pneumoniae mutant prevented the colonization of serotype 19F and 4 strains in mice (Xu et al., 2014; Jang et al., 2019). Chan et al. reported a multiple-antigen vaccine (MAV) based on bacterial lysates. In addition to having the advantages of a whole-cell vaccine, the preparation of this vaccine improved the amount of surface antigens as it had heat shock proteins (Hsps), PspA, and Ply proteins. In animal studies, MAV was able to elicit functional antibody production against multiple serotypes of S. pneumoniae, including non-vaccine serotypes (Chan et al., 2022). Based on these observations, it can be anticipated that the MAV approach may confer serotype-independent protection against S. pneumoniae.
Immunization of mice with a live attenuated whole-cell vaccine based on S. pneumoniae D39 (lacking pep27 and comD genes to eliminate the reversion of the bacterium to wild-type phenotype) showed significantly higher IgG titers against serotype D39. This immunization also reduced colonization regardless of the serotype. Furthermore, this strain depicted a good safety profile in both normal and immunocompromised mice. Thus, the Δpep27ΔcomD strain presents efficiency and safety in preventing pneumococcal infections (Kim et al., 2019). Another live attenuated vaccine strain has the pro-lipoprotein diacylglycerol transferase (lgt) gene deletion from the capsule of the pneumococcal strain TIGR4 (TIGR4Δlgt). This strain evokes a significantly reduced inflammatory response and is reduced in virulence. Intranasal immunization of mice resulted in protection against invasive pneumococcal infections (Jang et al., 2019). Thus, TIGR4Δlgt is an attractive broad-spectrum vaccine candidate.
NTHi commonly colonizes the URT and is predominantly present in the upper respiratory nasopharyngeal microbiota. It is associated with various non-invasive infections including OM, non-bacteremic pneumonia, and sinusitis (Van Eldere et al., 2014). NTHi is the main culprit of recurrent and chronic OM (Pichichero et al., 2008; Casey et al., 2010; Jalalvand and Riesbeck, 2018). As discussed earlier, PCVs have contributed to the increased proportion of OM associated with NTHi strains (Van Eldere et al., 2014). Prevention of NTHi-associated OM could reduce the global burden of OM by an estimated 350 million fewer annual cases of AOM episodes (Barenkamp, 2013). Since NTHi improves the NP colonization of Mcat (Andrade et al., 2018), therefore, to infect the ME and establish polymicrobial OM a symbiotic relationship between Mcat and NTHi is suspected (Armbruster et al., 2010). Given these observations, it can be anticipated that effective vaccine strategies specifically against NTHi-induced OM may also confer an indirect benefit for the prevention or resolution of polymicrobial OM.
The search for a safer broadly cross-reactive immuno-protective NTHi antigen has been expanding recently, however, to achieve this goal there are significant obstacles, mainly due to the heterogeneous nature of moieties present on the surface of NTHi: most surface-exposed antigens are variably present across strains, antigenically variable, phase variable, or a combination of more than one of these. At present, no NTHi-specific vaccines are licensed and the only NTHi antigen incorporated in a licensed vaccine is protein D as the protein conjugate in Synflorix™ (PHiD-CV). PhiD-CV is also in experimental vaccines with other NTHi antigens. Both PHiD-CV and PCV-10 likely reduce all-cause OM, but there is not strong evidence that Protein D in this formulation reduces carriage of NTHi or OM disease caused by NTHi (de Sévaux et al., 2020; Beissbarth et al., 2021). Given these observations and the expansive heterogeneity of NTHi strains, a multicomponent vaccine is essential to confer adequate protection against NTHi (Price et al., 2015; Jalalvand and Riesbeck, 2018).
Numerous NTHi vaccine candidates have been investigated in the past years. In this section, we review only those candidates who presented great potential to undergo further development.
Many NTHi proteins including H. influenzae adhesin protein (Hap), H. influenzae autotransporter (Hia), Protein D (PD), outer-membrane protein 6 (P6), and outer-membrane protein 26 (OMP26) have been studied by several groups for the prevention of NTHi disease (see review by (Khan et al., 2016)). Antisera raised in guinea pigs against high molecular weight (HMW) proteins HMW1/HMW2 or Hia proteins showed opsonophagocytic activity against a wide range of NTHi strains (Winter and Barenkamp, 2014). Given these observations, a vaccine formulated with HMW1/HMW2 and Hia proteins may protect against a broader range of NTHi strains. Pre-clinical studies with these proteins revealed that PD immunization with OMP26 produced lowered antibody responses against PD, however, PD immunization with P6 did not mask PD immune responses (Michel et al., 2022), emphasizing the necessity of compatibility studies when combining antigens. Mice immunized with a truncated adhesin protein F (PF) showed faster clearance of NTHi infections compared to mice immunized with a control peptide (Jalalvand et al., 2014). Type IV pili (Tfp) are crucial for NTHi biofilm development, adherence, competence, and twitching motility (Das et al., 2017). Antisera raised against recombinant soluble PilA (rsPilA) dispersed in vitro formed NTHi biofilms and blocked NTHi-induced OM in chinchilla (Novotny et al., 2015b, 2017).
A recombinant fusion protein consisting of immunologically important components of protein E (PE) and PilA was evaluated in pre-clinical trials. PE-PilA-induced anti-PilA antibodies halted NTHi biofilm development and disrupted in vitro established biofilms, as seen for rsPilA. After the intranasal NTHi challenge, NP colonization was significantly reduced in immunized mice, and in chinchillas, symptoms of experimental OM were notably hindered (Ysebaert et al., 2019). A multi-component NTHi vaccine consisting of a free recombinant PD and a recombinant fusion protein PE-PilA was tested in a phase 1 clinical trial in healthy adults (NCT01657526), with a good safety profile and acceptable reactogenicity (Leroux-Roels et al., 2016). A further development added the Mcat antigen UspA2 to this combination, to test a multi-component vaccine in two-dose and three-dose phase 1 and 2 clinical trials, showing promising safety and immunogenicity profiles in healthy adults and adults aged 50–71 with a history of smoking (De Smedt et al., 2021; Galgani et al., 2022).
Other vaccine candidates have not yet been tested in human trials but show promise in a range of animal studies. Intracellular elongation factor thermal-unstable (EF-Tu) was identified recently as a novel NTHi surface protein (Thofte et al., 2018); and it was reported that antibodies against EF-Tu can result in complement-dependent killing of NTHi (Thofte et al., 2019), indicating the potential of this protein as a NTHi vaccine antigen. To date, no further investigations in animal models have been reported.
In a parallel approach to the recombinant PE-PilA, Novotny et al. found that ChimV4, a fusion of P5 peptide and rsPilA resolved NTHi-induced OM when delivered transcutaneously (Novotny et al., 2015a). The formulation also prevented OM in a viral-bacterial model of experimental disease. Transcutaneous immunization with chimV4 + dmLT significantly increased mature B-cell phenotypes and antibody-secreting cells within nasal-associated lymphoid tissues (Novotny et al., 2017; Novotny and Bakaletz, 2020). A bioinformatics approach to identify candidate peptides could result in more rapid development of polyvalent protein antigens (see section 2.2.5 on recombinant polypeptide antigens).
One challenge for mucosal pathogens is eliciting a response to target bacteria at the surfaces. Building on previous studies that showed NTHi P6 as a promising candidate (DeMaria et al., 1996; Bakaletz et al., 1999; Kodama et al., 2000;), Kodama et al. found that prior treatment with CCL20 enhanced murine responses and bacterial clearance following intranasal P6 immunization (Kodama et al., 2011a). Similarly, P6 was tested with FMS-like tyrosine kinase receptor 3 ligand as a mucosal adjuvant and this combination also improved nasopharyngeal clearance of NTHi and dendritic cell recruitment (Kodama et al., 2011b; Kodama and Suzuki, 2011). Nasal immunization with P6 protein alone produced minimal or no antigen-specific immune responses and hence no effective protection against NTHi (Bertot et al., 2004; Abe et al., 2006; Noda et al., 2011). More recently, when combined with cCHP, a cationic cholesteryl pullulan nanogel, cCHP-P6 nanogel nasal vaccine induced P6-specific mucosal IgA and serum IgG responses without additional biologically active adjuvant. The P6-specific IgG titers were equivalent to those generated by the intramuscularly administered vaccine containing alum adjuvant (Nakahashi-Ouchida et al., 2022). This highlights that route of delivery, and adjuvant choice are crucial to assess vaccines to reduce disease at mucosal surfaces.
LOS are a major component of the Gram-negative bacterial outer membrane and thus, in theory, they are an attractive target for vaccine development. In NTHi, five different antigenically heterogeneous LOS serotypes I-V are produced (Campagnari et al., 1987), but these glycans are phase-variable within a strain and occur during infection (Fox et al., 2014). Despite this, several studies examined LOS as a candidate vaccine. In a mouse model of NP colonization, intranasal immunization with detoxified LOS-tetanus toxoid (dLOS-TT) demonstrated a substantial reduction of the same LOS type III NTHi strains as well as types IV and V, but only 50% reduction of type I and 29% reduction of type II NTHi strains (Hirano et al., 2003). Parenteral immunization with dLOS conjugates in chinchillas produced anti-LOS antibodies in serum or ME which were bactericidal and opsonophagocytic and lowered homologous NTHi-induced OM (Sun et al., 2000). Notably, dLOS was tested in a phase I study of healthy adults vaccinated intramuscularly with dLOS-TT resulting in increases in serum antibodies, with acceptable safety. However, no further human trials have proceeded. An alternate approach is to target a glycan present in bacteria but absent from host tissues. Ketodeoxyoctonic acid (KDO) is present in most lipo-polysaccharides (LPS)/LOS, as well as in some CPS. A monoclonal antibody, 6E4, raised against KDO is bactericidal against 12 out of 33 tested strains of NTHi in vitro (Apicella et al., 2018). Using a glycoconjugate composed of either multiple KD residues or a KDO-N-acetyl-lactosamine conjugated to an immunogenic protein may be an approach to develop a component of a vaccine that would target many bacterial species.
OMVs produced by Gram-negative bacteria are enriched in outer membrane components, including major and minor outer membrane proteins and LOS. The functional activity of NTHi OMV-specific antisera and the protective ability of NTHi OMVs as vaccine antigens in the chinchilla OM model were tested. Immunization of chinchillas with OMVs isolated from HMW1/HMW2- and Hia- Hia-expressing NTHi prevented experimental OM (Winter and Barenkamp, 2017). In another study, immunization of NTHi-derived OMVs along with CpG-MPLA adjuvant induced the production of protective antibodies and cytokines in mice (Behrouzi et al., 2020). Several licensed and effective vaccines targeting meningococcal disease are based on OMVs. The presence of immunodominant proteins can lead to strain-specific responses, but either the removal of strain-specific antigens or the addition of conserved antigens can address this problem (Pizza et al., 2020). This approach may be feasible for both NTHi and Mcat but requires significant development.
Adding a lipid moiety to a recombinant protein is expected to increase the immunogenicity through Toll-Like Receptor 2 (TLR2) signaling of antigen-presenting cells and T-helper 17 cells (Th17) to evoke or enhance cellular responses in the nasal-associated lymphoid tissue (NALT). Recently, the effects of lipidation vs. non-lipidation of recombinant P6 and OMP26 were compared in a mouse model. Lipidated P6 and OMP26 elicited nearly 10- to 100-fold higher IgG antibody levels, and lipidated antigens also reduced NP colonization and ME bullae NTHi density more than non-lipidated formulation (Kaur and Pichichero, 2022). Therefore, lipidation of NTHi antigens represents a promising approach in the development of novel NTHi vaccine formulations (Table 2).
Table 2. Potential vaccine antigens of non-typeable Haemophilus influenzae at various stages of development.
As noted above, combining protein antigens can be achieved by identifying immunologically relevant epitopes, and generating fusion proteins (see PE-PilA and ChimV4). Since bacterial genomes have been available, bioinformatics analysis has identified putative surface-exposed proteins, in an approach described as “reverse vaccinology,” an approach that contributed to antigens in the GlaxoSmithKline vaccine against serogroup B meningococcus (Masignani et al., 2019). Significant improvements in bioinformatic analysis tools, availability of increasing numbers of genome sequences for each bacterial species, and increased understanding of immunologically relevant sequences and structure allow more sophisticated and rapid screening for candidate proteins, domains, or short peptide sequences. Recently Whitby et al. identified 56 putative surface proteins conserved in 26 genomes (selected to represent diverse strains). Potential surface exposed regions were identified using molecular modeling. Antisera were raised in rats against 10 synthetic peptides corresponding to surface-exposed regions highly conserved in strains. Five of the antisera were protective in the infant rat model of invasive NTHi infection establishing their in vivo efficiency (Whitby et al., 2015). A different bioinformatics platform identified two similar proteins as being future NTHi vaccine candidates (D’Mello et al., 2019).
The same group extended their strategy to produce a single polypeptide comprising 9 unique peptides from 6 different surface proteins. Immunization of chinchillas with this polypeptide resulted in faster clearance of NTHi-induced OM (Whitby et al., 2020). This approach has the potential to be applied to other pathogens that have complex diversity in surface antigens, including Mcat. In the coming years, it can be anticipated that high throughput bacterial genomics, computational-based vaccine candidate identification approaches, and experimental validation will identify additional vaccine candidates for NTHi.
Mcat is a Gram-negative diplococcus that is typically present in the NP of most infants and children. Mcat is commonly associated with OM, however, the exact percentage of Mcat-induced OM differs between studies because of alterations in sample collection, methods of bacterial detection, and geographical location. It is the first otopathogen that colonizes the NP and the first otopathogen to initiate an episode of OM (Kaur et al., 2014a; Ngo et al., 2016), in some susceptible populations colonizing within a month of birth (Beissbarth et al., 2021). Mcat, like NTHi, is also a key pathogen in exacerbations of COPD (Murphy et al., 2019). Mcat can be treated with antibiotics, but almost all strains are now beta-lactamase positive, potentially promoting the survival of otherwise beta-lactam-sensitive bystander bacteria (Verduin et al., 2002).
Mcat vaccine can help prevent NTHi and pneumococcal infections by excluding a potential co-pathogen that can induce passive protection from β-lactam antibiotic therapy (Perez and Murphy, 2017). However, Mcat vaccine development is lagging behind that of S. pneumoniae and NTHi. Learning from the NTHi vaccine approach, it is likely that an effective Mcat vaccine may need multiple antigens, as it also has a range of antigenically and phase variable antigens. Nevertheless, various factors deter the progress of Mcat vaccine development, including the absence of a known correlate of protection and a suitable animal model mimicking Mcat infection in humans (Perez and Murphy, 2019) (and see section below on animal models). Regardless of these barriers, noteworthy strides have been made in the last few years in the identification of potential antigens, efficient adjuvant formulations, and immunization routes using both computational and experimental methods [see Table 3 and a review by (Perez and Murphy, 2017)]. However, several potential vaccine candidates showed initial promise, but for which there are few recent reports of progress. This may reflect the lower priority that Mcat appears to have or the lack of high-throughput animal models of OM that can be used to test their efficacy.
As discussed earlier, presently only one Mcat protein antigen has been tested in clinical trials (UspA2). UspA2 interacts with host structures and extracellular matrix proteins and induces bacterial adherence and serum resistance (Singh et al., 2010; Su et al., 2013; Singh et al., 2015). Vaccination with UspA2 induced protective antibodies in mice (Chen et al., 1996). UspA2 has been reported to have variations in sequence and structure (Su et al., 2013), which may affect antibody recognition and binding. Despite this, anti-UspA2 antibodies raised in mice, rabbits, and guinea pigs were able to induce complement-mediated killing of many, but not all Mcat strains of different origins (Ysebaert et al., 2021). The NTHi-Mcat vaccine containing UspA2 was immunogenic in the phase 1 clinical trial, with a satisfactory safety profile both in healthy individuals and adults with a smoking history (Van Damme et al., 2019). A 4-year follow-up (NCT03201211) found that immune responses to NTHi antigens persisted over an extended period, whereas UspA2 did not (De Smedt et al., 2021). However, in all human trials to date, baseline titers of serum antibodies against UspA2 are relatively high, suggesting either natural immune responses to UspA2 or similar proteins in Mcat, or cross-reactivity with an antigen from another source. This observation complicates the interpretation of data in clinical trials on the persistence of serum antibody responses. In a randomized, placebo-controlled, phase 2b trial (NCT03281876) of adults with a history of acute exacerbations of COPD, this vaccine showed good safety, however, did not demonstrate efficacy in lowering the yearly rate of severe or moderate exacerbations of COPD (Andreas et al., 2022; Arora et al., 2022).
Several antigens have shown promise in early animal trials but have few recent reports. This includes Hemagglutinin Moraxella IgD binding protein (Hag/MID), PilA2 (the major protein subunit of Tfp), and outer-membrane protein CD (OMP CD). However, they have been identified as targets of natural antibody production in COPD patients, or children (LaFontaine et al., 2009; Ren et al., 2019). OMP CD is an adhesin and a porin protein (Murphy et al., 2003) with surface-exposed epitopes and is considered conserved among different Mcat strains (Sarwar et al., 1992; Murphy et al., 1993). Murine immunization with OMP CD increased Mcat clearance following pulmonary challenge (Liu et al., 2007). Hag/MID is an OMP involved in human erythrocyte agglutination (Pearson et al., 2002; Forsgren et al., 2003; LaFontaine et al., 2009). Hag/MID shows sequence diversity among different strains, however, the adhesive domain region is conserved. A truncated Hag/MID induced a protective response in mice (Forsgren et al., 2004). PilA2 was present in 57.5% of 106 tested clinical Mcat isolates (Luke-Marshall et al., 2011). It was required for the adherence of Mcat to in vitro cultured human epithelial cells and NP colonization in a chinchilla model (Luke et al., 2004, 2007). At present, no data is available on measuring the immune responses to the PilA2-based vaccine in animal models or humans. Ren et al. studied natural antibody production against OMP CD, Hag, and PilA2 in stringently defined otitis-prone (sOP) children compared to non-otitis-prone children (NOP). All proteins elicited antibodies, but the sOP population showed reduced serum antibody responses (Ren et al., 2019). Serum and sputum sample analysis from COPD patients revealed that a Hag/MID domain encompassing amino acids 706 to 863 was a target of serum IgG and sputum IgA (LaFontaine et al., 2009).
Moraxella catarrhalis-adherence protein (McaP) is an adhesin and an autotransporter having esterase and phospholipase B activities (Timpe et al., 2003). Moreover, this protein is highly conserved as 98–100% amino acid sequence similarity was observed among 8 studied strains (Timpe et al., 2003; Lipski et al., 2007). Mouse anti-Mcap serum hindered the binding of Mcat and recombinant E. coli expressing McaP to human respiratory epithelial cells (Timpe et al., 2003; Lipski et al., 2007). Outer-membrane protein E (OMP E) is a porin and is involved in the binding and transport of fatty acids. Gene sequence analysis showed that the ompE gene stayed stable during the colonization process (Martin et al., 1993). OMP E also expressed a highly conserved surface epitope (Bhushan et al., 1997). Further investigations are needed to gain a clear understanding of McaP and OMP E immunogenicity, its different antigenic domains, and the protective immune responses they elicit. M35 is an OMP that acts as a general porin and is essential for energy source uptake for Mcat (Easton et al., 2005). M35 is suggested to be essential for in vivo colonization and resistance mechanisms (Easton et al., 2008). M35 gene sequence showed high conservation (99.6–100%) among 18 tested isolates. In immunoblot analysis, mouse anti-M35 serum displayed binding to whole-cell protein preparations from all the tested isolates (Easton et al., 2005). Anti-sera from M35 immunized mice did not show bactericidal activity, however, it improved opsonic activity. In another study, mucosal immunization of mice with recombinant M35 through intra Peyer’s patch increased clearance of bacteria from the lungs of Mcat-challenged mice (Easton et al., 2011).
Moraxella catarrhalis filamentous hemagglutinin adhesin-like protein (Mha)B1 and MhaB2 (exoproteins), and MhaC (transporter) (Balder et al., 2007), which are also named M. catarrhalis hemagglutinin-like protein (Mch)A1 and MchA2 for the secreted proteins and MchB for the transporter are potential vaccine candidates (Plamondon et al., 2007). A mutant Mcat lacking MhaB1 and MhaB2 could not colonize the chinchilla NP, emphasizing the importance of these proteins. Immunization of chinchillas with a polypeptide shared by MhaB1 and MhaB2 induced antibodies that interfered with the colonization of Mcat and promoted bacterial clearance (Balder et al., 2011; Shaffer et al., 2013).
Murphy et al. screened the genome of Mcat strain ATCC 43617 for potential surface proteins. This identified several substrate-binding proteins (SBPs) of the ABC transporter family. The group evaluated CysP, AfeA, OppA, and Sbp2 for their immunogenic potential which are studied by other groups as well (Yang et al., 2011; Otsuka et al., 2014; Murphy et al., 2016; Otsuka et al., 2016; Murphy et al., 2017). CysP is involved in the uptake of sulfate, AfeA binds ferrous, ferric, zinc, and manganese ions, OppA is likely an oligopeptide binding protein of the oligopeptide permease ABC transport system, and Sbp2 mediates the uptake of arginine, a strict growth requirement of Mcat. All were found to express accessible surface epitopes, to be conserved within Mcat strains, and to provide enhanced clearance in a lung challenge model in mice (Yang et al., 2011; Otsuka et al., 2014; Murphy et al., 2016, 2017).
Moraxella surface proteins (Msp) including Msp22, Msp75, and Msp78 showed 97–99% homology in amino acid sequence among 10 tested strains (Ruckdeschel et al., 2008). Mucosal and systemic immunizations of mice with recombinant Msp22 and Msp75 induced IgG and IgA antibodies. Mouse and rabbit anti-sera to recombinant Msp22 and Msp75 were able to recognize corresponding proteins in numerous Mcat strains. In addition, intranasal immunization of mice with recombinant Msp22 substantially lowered the bacterial load in the lungs of Mcat challenged mice (Ruckdeschel et al., 2009; Smidt et al., 2013). Therefore, these proteins are attractive Mcat vaccine antigens, nonetheless, more studies are required to uncover their detailed functions and immunogenicity in humans.
Peptide-based vaccines offer numerous advantages, such as the exclusion of deleterious parts from full-length antigens, ease of chemical modification, absence of infectious material, and ease of production and storage. Although they generally have poor immunogenicity, this can however be compensated for by using modified formulations of the vaccine (Purcell et al., 2007). Lactoferrin-binding protein A (LbpA) is a receptor for human lactoferrin and is involved in iron transport. Whole LbpA, both native and recombinant was reported to be non-immunogenic (Du et al., 1998; Yu et al., 1999). Yassin et al. used immune-informatics analysis to identify a peptide from LbpA named peptide A which showed high immunogenicity in mice and successfully cleared Mcat from mouse lungs. Furthermore, anti-peptide LbpA antibodies were bactericidal against heterogeneous Mcat strains (Yassin et al., 2016). Peptide A is the first promising peptide-based vaccine against Mcat, and it warrants further investigation.
LOS comprises a major virulence factor of Mcat and performs a vital role in eliciting inflammatory immune responses (Hassan et al., 2012). It also mediates serum resistance (Zaleski et al., 2000) and adherence to human epithelial cells (Peng et al., 2005). LOS from Mcat is relatively conserved, with three major serotypes (Holme et al., 1999; Verduin et al., 2002; Ren et al., 2011b), and no phase variation within a strain (unlike NTHi). In common with CPS, LOS glycans are poorly immunogenic unless conjugated to a carrier protein. Removal of toxic Lipid A moieties is also necessary. Detoxified LOS (dLOS) is conjugated to a carrier protein. Immunization of rabbits and mice with the conjugates derived from all three serotypes induces significant levels of antigens-specific mucosal and serum antibodies. Bactericidal antibodies were also identified in immunized animals (Jiao et al., 2002; Yu and Gu, 2005, 2007). Ren et al. generated two mutant LOS from Mcat strain O35E to produce conserved LOS antigens and exclude a potential autoimmune response in humans. These mutant LOS had one or two terminal galactopyranose (Galp) residues deleted and conjugated to tetanus toxoid (TT). These conjugates exhibited broad-spectrum protection and induced high levels of serum IgG with bactericidal activity against all three serotypes (Ren et al., 2011b). In another study, dLOS-protein conjugates from all three serotypes were combined and immunized mice developed humoral and cell-mediated immunity which enhanced pulmonary clearance of six strains of all three serotypes of Mcat (Ren et al., 2011a). In a similar approach, truncated (common) LOS was conjugated to recombinant OMP26 of NTHi. This elicited complement-mediated bactericidal activity against tested strains of serotype A and Mcat and one NTHi strain (Singh et al., 2020).
As mentioned above, D’Mello et al. used a computational approach to identify potential candidate antigens against a range of criteria, from NTHi and Mcat (D’Mello et al., 2019). Top Mcat candidates identified included a porin protein, an iron transporter protein, and 2 unstudied conserved-hypothetical proteins (D’Mello et al., 2019). Soltan et al. applied a different reverse vaccinology approach to identify potential protein candidates, including criteria of being essential, outer membrane-localized, involved in virulence, antigenic with no human homologs, with appropriate molecular weight and less than two transmembrane helices. Only LPS assembly protein LptD and outer membrane protein assembly factor BamA met these criteria. From this, several peptides of each were combined to construct a theoretical multi-epitope candidate peptide (Soltan et al., 2021). It is likely that future publications will reveal which of these are validated and can be further developed, but as with NTHi, a multi-component vaccine is likely required.
In summary, numerous Mcat antigens have revealed outstanding immunogenicity, induced functional antibodies, and generated protective responses in animal models. Nonetheless, there has been no clinical testing on them. Future studies are needed on these antigens to make advances in Mcat vaccine development.
Animal models are an important tool to study, (1) pathogens of OM; (2) the microenvironment during disease progression; (3) how pathogen-specific immunity helps resolve acute OM; and (4) vaccination strategies against this disease before they can be applied to humans. Over the years, several animal models have been developed for studying OM (see a recent review by (Davidoss et al., 2018)). There was much interest in the use of guinea pigs as an animal model in the 1960s and 1970s, mainly because of their popularity in other branches of medical research. However, since the late 1970s, the chinchilla has been used for investigating OM. This is primarily due to the chinchilla’s large bulla that makes access to the ME easy. In the 1980s, gerbils were popular because of their propensity to develop cholesteatoma (Bakaletz, 2009). However, since 2010 interest in mouse models of OM has increased significantly because of the increased ease of genetic manipulation, and identification of mouse host mutants with susceptibility to OM (Geng et al., 2019). A summary of these animal models is provided in Table 4. Since most human bacterial pathogens do not naturally colonize or cause disease in animals, infection of ME or NP in animals can yield different immune responses to that in humans (Sabirov and Metzger, 2008). For a given model, a pathogenic organism must meet certain criteria to be considered pathogenic that is: (a) the pathologies induced by the pathogen in the animal must resemble those observed in humans; (b) otomicroscopy, tympanometry, and histopathology, pathologies can be objectively recorded; and, (c) the organism is able to reproduce in the ME space (Doyle, 1989).
To mimic human OM, an ideal model is one in which intranasal (IN) instillation of bacteria leads to NP colonization, and ascent of bacteria through the ET to the ME. As host-adapted pathogens, the three major otopathogens exhibit the differential capacity to survive, colonize, replicate, and directly ascend to the ME. Some animal models of OM use direct instillation of bacteria into the ME.
In the IN-inoculation method, the entry portal for the pathogen into the ME is analogous to the human disease process, because NP is the first place where bacteria and viruses colonize and reproduce before invading the ME cavity. This method can be highly reproducible if inoculum volume is maintained with negligible aspiration or swallowing of the inoculum which can be assisted through aesthesia before IN inoculation. Commonly used microorganisms to induce OM in experimental settings are S. pneumoniae, NTHi, methicillin-resistant S. aureus, and Pseudomonas aeruginosa. Some animal models use prior infection, or co-infection of the virus to facilitate bacterial OM (Appell et al., 1971; Meek III et al., 1999; McCullers and Rehg, 2002; Faisca and Desmecht, 2007; McCullers et al., 2007). Notably, Mcat will rarely survive in the murine mucosa. Bacteria can be inoculated directly into the bullae, or by trans-tympanic inoculation into the ME, leading to the induction of OM. These methods have high reproducibility and precision as the exact number of microorganisms in the inoculum can be determined leading to less variability between individual animals. A drawback of these methods is the bypass of NP colonization. Furthermore, surgical skills are required to use the trans-bullar approach. Inconsistency in this process can cause damage to the nearby blood vessels (Parks et al., 1996; Pinilla et al., 2001). In comparison, the trans-tympanic approach is easier to perform, using a fine needle to deliver the inoculum through the tympanic membrane. Sometimes, injected material can drain through the tympanic membrane hole leading to imprecise inoculum volume as compared to intra-bullar inoculation. The hole made in the tympanic membrane can be a channel for contamination. In addition, pressure equilibration can result in increased drainage of ME effusions through this hole. Thus, the trans-tympanic delivery method is appropriate for those species having direct trans-canal access (Ryan et al., 2006).
Non-infectious animal models have also been reported. Several mouse models have been generated by utilizing LPS or peptidoglycan-polysaccharides (Li et al., 2015; Zhang et al., 2015; Kim et al., 2016). Ovalbumin has been used to induce an eosinophilic OM via IN administration in a mouse model or a trans-tympanic injection in a guinea pig model (Matsubara et al., 2014; Kim et al., 2016). Moreover, histamine has been used in guinea pigs to induce AOM (Kozan et al., 2015).
OM induction strategies are therefore dependent upon the experimental requirements and the type of animal being used. The IN-challenge model is preferred for inducing OM while assessing protective immunity in ME against NP colonization. A direct ME challenge could, however, be preferred for determining the extent of ME inflammatory responses as well as studying the protective immunity against ME challenge.
Several animal models have been developed that examine the pathological changes associated with OM, in the absence of pathogen challenge. The principal anatomical cause of OME is ET dysfunction. To mimic this, animal models of OME are generated through ligation or cauterization of ET utilizing a trans-oral or trans-neck approach (Piltcher et al., 2002), or by injecting chemical materials through the tympanic membrane (Aynali et al., 2011). For the first approach, the ET orifice is cauterized, and the cartilage portion of the ET is ligated with nylon or an electrical cautery (Piltcher et al., 2002). Cauterization or ligation of the ET is however an irreversible process. The trans-neck method is a precise approach for blocking the tube, but it is more laborious as compared to the trans-oral approach. On the other hand, the trans-oral approach is easy, however, it is difficult to get consistent results with this method (Huang et al., 2012). Injecting chemicals via the tympanic membrane is comparatively simpler to perform than the methods mentioned above. Injection of histamine solution has been used to induce OME in rats (Aynali et al., 2011).
The choice of the experimental animal depends on anatomical, physiological, economic, spatial, and psychological factors, as well as experimental objectives. Depending on the research objective, animal model chosen, and access to equipment, there may be variations in studies. Therefore, it is crucial to understand the characteristics of the chosen model’s auditory system, along with its advantages, disadvantages, and limitations.
Rats exhibit the highest compatibility in terms of ME anatomy and histology to human infants and children as compared to other rodents, therefore, they are preferred models of AOM (Cayé-Thomasen and Tos, 2002). In addition, most human pathogens readily infect rat ME (Chaney et al., 2011) and the opening pressure of the ET is equivalent to that in humans (Hellstorm and Stenfors, 1983). The rat AOM course bears a close resemblance to that of humans (Hermansson et al., 1988), and ciliary clearance tracts and histological cell types are also analogous to humans (Daniel III et al., 1982). As a result of the relatively large tympanic bulla in the rat, bacteria can easily be inoculated into ME via the tympanic membrane or the bulla. Once infection has occurred, it usually resolves within 10–12 days without ME effusion signs and affecting tympanic membrane preservation (Hermansson et al., 1988; Prellner et al., 1999). It is also possible to induce a persistent OME in rats which can last for more than 16 weeks upon using appropriate inoculum (Piltcher et al., 2002). Moreover, rats are not prone to develop general sepsis which further enhances their usefulness (Prellner et al., 1999). Rat models of AOM with S. pneumoniae and OME model with ET obstruction by dental material are reported (Hermansson et al., 1988; Piltcher et al., 2002). An allergen-induced OME rat model was established using an intraperitoneal injection of ovalbumin and a subsequent intratympanic injection of ovalbumin into the ME (Hardy et al., 2001; Zhang et al., 2022). Rat OM model with methicillin-resistant S. aureus and P. aeruginosa injected into the ME cavity via the tympanic membrane have been developed (Yadav et al., 2017). Magnuson et al. utilized Haemophilus influenzae type b and NTHi strain 3,655 to inoculate the ME of Sprague–Dawley rats and studied the course of AOM development. Rats developed AOM with both bacterial strains 4 days post-inoculation (Magnuson et al., 1997). Rat models of OM with cholesteatoma have also been developed utilizing different chemical compounds such as dimethyl-benzanthrancene (DMBA) or propylene glycol injections into the ME (Klein-Szanto et al., 1982; Huang et al., 1988). An acute secretory OM (SOM) rat model was developed by delivering endotoxin into the ME cavity through the eardrum (Li et al., 2021). Mcat can induce OM in rats, but only with high dose, trans-tympanic inoculation, and OM is shorter lived than that caused by NTHi or S. pneumoniae (Westman et al., 1999).
Another rodent that is favored for AOM research is the chinchilla which displays general susceptibility to human bacterial and viral pathogens of OM (See review by (Bakaletz, 2009)). An advantage of using the chinchilla is that its tympanic membrane is almost the same size as humans. In addition, its large bulla facilitates the inoculation of pathogens and collection of ME effusions (Watanabe et al., 1982). The temporal progression and natural history of the disease process resemble human OM (DeMaria et al., 1996; Bakaletz, 2009). However, this species has a multi-loculated bulla, which is easily prone to fibrosis, with the ability to seal a chamber of the bulla and prevent infection from spreading. The external auditory canal of chinchillas is elongated and S-shaped, making it difficult to inspect the tympanic membrane with an ordinary otoscope and making the trans-tympanic challenge more difficult. It is only widely available in North and South America; therefore, lack of easy availability hinders its application. The rate of inner ear complications is high in chinchillas, and they tend to develop general sepsis, especially the younger chinchillas, and have a high mortality rate. Furthermore, chinchillas are not as capable of withstanding adverse conditions in laboratory settings as rats. Chinchillas tend to shed their fur at the insignificant sign of distress (Murrah et al., 2015).
AOM models of chinchilla with S. pneumoniae, H. influenzae type b, NTHi strain 86-028NP, and other ME-associated aerobic and anaerobic microbes have been developed through trans-bullar inoculation into the ME (Fulghum et al., 1982; Morton et al., 2012; Guan et al., 2014). Cholesteatomatous chronic OM chinchilla models have also been developed through the use of propylene glycol injection (Masaki et al., 1988).
Mongolian gerbils have been widely used to establish AOM models for the study of AOM and analysis of efficacies of different antimicrobials. The small size and cost-effectiveness of Mongolian gerbils made them popular in OM studies. They have a reasonably large ME making it easy to inoculate through the overlying skin. It is also easy to sample ME fluid, however, they have narrow external ear canals. Mongolian gerbils normally have healthy ears and rarely experience OM, while cholesteatoma is common in elderly gerbils (Chole et al., 1981).
Compared to chinchillas and rats, fewer studies using gerbils as a model for bacterially induced AOM are reported. Direct bacterial ME inoculation has been used with S. pneumoniae type 3, Haemophilus influenzae type b, and NTHi strain 119 (Fulghum et al., 1982, 1985; Von Unge et al., 1997; Cenjor et al., 1998; Larsson et al., 2003; Unge et al., 2009). For non-bacterial ME disease, cholesteatoma development or other ME disease was reported in gerbils through ligation of the external ear canal, ET blocking by electrocauterization or glue, and chemical injections into the bulla (Larsson et al., 2005; Yamamoto-Fukuda et al., 2010, 2011).
The MEs of guinea pigs have structural similarities to those of mice and rats, but the size of bulla in this animal is smaller compared to gerbils and chinchillas (Hellström et al., 1982; Foxwell et al., 1998). Several studies have used the AOM guinea pig model developed by using non-typeable S. pneumoniae and S. pneumoniae type 3 (Sp3) via a trans-bullar approach (Parks et al., 1996; Dai et al., 2009; Guan and Gan, 2013). LPS from Klebsiella pneumoniae was injected into the ME of the guinea pig to develop an OME guinea pig model (Ohashi et al., 1991; Dai and Gan, 2008). Yu et al. developed a guinea pig model of OME by reversible ET obstruction (Yu et al., 2013). Cholesteatoma guinea pig models with chemical inoculants in the bulla have also been developed (Yamamoto-Fukuda et al., 2011).
The NP-ET-ME complex of primates has been employed to model the normal and pathological activities of the human ET. There have been very few studies on monkey models of OM. The Rhesus monkey model has been used to evaluate the impact of allergic rhinitis in the development of ET obstruction and OME (Friedman et al., 1981; Doyle et al., 1985).
Dohar et al. (1998, 2005) established a CSOM cynomolgus monkey model by infection of P. aeruginosa to investigate the safety and efficacy profile of topical ciprofloxacin hydrochloride for treating experimental CSOM. The tympanic membrane of cynomolgus monkeys was perforated and the ME was injected with a strain of P. aeruginosa which forms biofilms (Dohar et al., 1998, 2005). Although monkeys share more similarities to humans than rodents, their large size makes their handling in experimental settings very difficult. They are costly and not readily available, and modern ethical considerations have also reduced their use.
Since 2010, mouse OM models have significantly increased as compared to other models and now the mouse is more utilized in OM research because it offers many remarkable advantages over other animals [see review by (Bhutta, 2012)]. The wide availability of reagents allows complex analysis of immunological progression and host responses. Importantly, several genetic, transgenic, and gene deletion strains are available that can be used in studying various aspects of OM pathophysiology as well as host susceptibility to OM (Mitchell et al., 1997; Hardisty et al., 2003; MacArthur et al., 2006; Zheng et al., 2006).
Although mouse models offer many benefits as model systems for OM, yet there are some limitations to consider. The small size of the tympanic membrane in mice makes it difficult to access as compared to larger rodents. Even with the use of a microscope, surgical procedures on mice can be challenging. Inoculating and removing fluid from the mouse ME is difficult, and sampling large amounts of effusion is impractical because of the small size of the ME. The mouse is less hardy in tolerating general anaesthesia and surgical bleeding. Since major pathogens of OM such as NTHi, S. pneumoniae, and Mcat are not natural murine pathogens (Murphy, 2005; Doherty et al., 2006), variations can be observed in patterns of colonization and immune responses resulting from infecting mouse ME and NP than those observed in humans. This notable limitation is also associated with other rodent models. Nonetheless, it can be overcome via direct ME inoculation or multiple IN inoculations. Genetically modified mice which are more susceptible to human pathogens can be used for this purpose. Moreover, the use of mutant bacterial strains capable of adhering and invading the murine mucosa more effectively than wild-type bacteria offers another approach (Tu et al., 2007).
To study the natural course of OM in mice, Dewan et al. have described a novel pathogen capable of transmission between mice that causes OM (Dewan et al., 2019; Ma et al., 2022). Bordetella pseudohinzii efficiently replicates in the NP, quickly escalates the ET, and colonizes the ME. The resulting acute and chronic histopathological transformations with an increasing decline in hearing acuity closely represent OM in humans. Laboratory mice experimentally inoculated with a very small inoculum of B. pseudohinzii consistently had their MEs colonized and subsequently transferred it to cage mates (Dewan et al., 2019; Ma et al., 2022).
Several mouse mutations from the ethylnitrosourea (ENU) mutagenesis program have been described that develop spontaneous OM. The deaf mouse mutant Jeff (Jf) is a single locus OM model that was discovered from the ENU program. A notable conductive hearing loss along with pus and fluid in the ME cavity is observed in heterozygous Jeff mouse postnatal day 35 and it develops a CSOM with a high rate of inflammation. Jeff mouse has a mutation in an F-box gene (FBXO11). The expression of this gene occurs in the epithelial cells of the ME from the latter stages of embryonic development until day 13 after birth (Hardisty et al., 2003). Homozygous Jeff mouse, on the other hand, demonstrates facial clefting, cleft palate, and perinatal death (Hardisty-Hughes et al., 2006). A study genotyped 13 single nucleotide polymorphisms (SNPs) in FBXO11 establishing the link between FBXO11 polymorphisms and chronic OM with effusion/recurrent OM (COME/ROM) (Segade et al., 2006). The Junbo (Jbo) mouse, again from the ENU mutagenesis program, has a mutation in the inflammatory-signaling regulator Evi1 (Parkinson et al., 2006). The heterozygote develops spontaneous CSOM. Another important mouse model from the ENU program is a genetic OM-one (gom1) mutant mouse. This mouse is prone to develop OME and develops many characteristics of OM such as craniofacial abnormalities, ME effusion, epithelial hyperplasia, and hearing loss. Thus, the gom1 mouse provides a remarkable tool for elucidating OM pathogenesis, presenting similar pathological changes and auditory dysfunction as those observed in human OM patients (Zheng et al., 2022). Gene targeting and other transgenic modifications have been used to develop genetic mouse models to investigate OM susceptibility in human patients with genetic defects. Patients having velo-cardio-facial syndrome/DiGeorge syndrome (VCFS/DGS) with 22q11 deletions commonly develop chronic OM (Funke et al., 2001). In bacterial artificial chromosome (BAC) transgenic mice, overexpression of the TBX1 transcription factor (an equivalent of human VCFS/DGS in mice) and three other transgenes resulted in similar defects as seen in VCFS/DGS patients (Liao et al., 2004). Mice with a deletion in the p73 locus (p73−/− mice) exhibited a 100% occurrence of OM (Yang et al., 2000). Deficiency of lymphocyte function-associated antigen-1 (LFA-1−/− (CD11a/CD18)), in mice resulted in an elevated OM rate along with a notably high mortality rate (Prince et al., 2001). A dysmorphology of ET and abnormal ME cavity is observed in eya4 knockout mice leading to the development of OME (Depreux et al., 2008). A point mutation in the nischarin protein causes chronic OM resulting in conductive hearing loss development (Crompton et al., 2017). The mutant mouse strain known as ages-with-stiffened-joints (asj), carrying a point mutation in the Enpp1 gene, exhibits early-onset conductive hearing loss and defects in the ME with approximately 90% of the mutant mice have hearing loss (Tian et al., 2016). Furthermore, mutations in the EDA, EDAR, and EDARADD genes have been linked with the onset of nasopharyngitis, rhinitis, and OM (Azar et al., 2016).
In addition to investigating spontaneous OM in genetic mouse trains, several studies have investigated the known human otopathogens in these strains of mice. A high susceptibility to ME infections with S. pneumoniae was seen in lysozyme knockout mice (M−/−) mice leading to more pronounced inflammation in ME compared to wild-type mice (Shimada et al., 2008). Junbo heterozygote mice had NTHi intranasally inoculated, resulting in NTHi ascending and establishing in the ME (Hood et al., 2016). This mouse strain was also used to study vaccine responses (Hood et al., 2016) and cellular immune responses to NTHi OME (Vikhe et al., 2019). Kurabi et al. used Asc−/− knockout mice to develop an OM model using NTHi strain 3,655. A comparison of responses to NTHi in the ME between wild-type and Asc−/− mice showed persistent inflammation and delayed clearing of NTHi from the ME cavity of the latter (Kurabi et al., 2015). To define the function of CCL3 (a potent effector of inflammation) in OM, ccl3−/− mice were infected with NTHi to induce OM. The ccl3−/− mouse had prolonged mucosal hyperplasia and impaired bacterial elimination (Deniffel et al., 2017). Moreover, a genetic mouse model having a mutation in a G protein-coupled receptor was demonstrated to develop spontaneous OME (Kerschner et al., 2013). To date, there are no reports of Mcat establishing OM in these mice. Although great leaps forward have been made in the development of genetic mouse models of OM, nonetheless, more genetic OM models are needed to improve our grasp on genome-related interactions between hosts and pathogens to aid in the discovery of innovative therapies for better and alternative treatment approaches for human OM conditions.
The “humanized” mouse has become an invaluable tool for studying the human immune system in recent years. Son et al. developed the first humanized mouse model to study OM which had engraftment of CD34+ hematopoietic stem cells from human fetal liver and recapitulated the acute OM process utilizing NTHi. This model mimics the inflammatory responses of the ME to bacterial infection, immune cells’ recruitment, and typical recovery process thus allowing researchers to investigate human immunity in OM in preclinical settings (Son et al., 2022).
A major challenge to Mcat vaccine development is the unavailability of a suitable animal model and a dependable correlate of protection. Under experimental settings, Mcat does not readily survive and replicate in the rodent NP. Although, chinchilla has been used in evaluating the NTHi and S. pneumoniae vaccine antigens, however, it readily clears Mcat from the ME (Bakaletz, 2009). Therefore, either NTHi and/or viruses are used in chinchillas to study Mcat infections and vaccine antigens (Armbruster et al., 2010; Brockson et al., 2012; Shaffer et al., 2013; Perez et al., 2014), or direct intra-bullae inoculation (Blakeway et al., 2019). Nevertheless, the chinchilla NP colonization model, the mouse lung clearance model, or the in vitro functional assays are unable to provide a fixed correlate of protection for assessment in animal models. Therefore, the development of improved animal models for evaluating potential Mcat vaccines is highly desired.
Vaccines are required to lower the burden of childhood OM disease worldwide and would also reduce the burden of bacterial diseases caused by these pathogens in other susceptible populations. Given the fact that OM is a polymicrobial disease, it presents numerous challenges for vaccine development. It would be most advantageous to develop an OM vaccine that targets all three causative bacteria to confer better protection against the disease. Progress has been made in identifying and investigating vaccine targets against OM, and several candidates from three major pathogens of OM are in different stages of development as vaccine antigens. Moreover, many of these antigens are ready for preclinical and early clinical testing. Investment in these studies will enable valuable advances in the development of new vaccines with better efficacy and coverage. Nonetheless, further research is required to discover and analyze new vaccine antigens. A crucial aspect of developing antigen-based strategies is to use highly conserved antigens that are shared between similar bacterial species. The combination of reverse vaccinology and immuno-informatics is increasingly being adopted in the search for new vaccine candidates. The combination of these two approaches has the potential to reduce both time and costs before taking a vaccine into clinical trials thus providing a better estimate of how human populations would respond to the vaccine candidate worldwide.
Several animal models of OM are available. Researchers studying OM should be familiar with the strengths and limitations of these models to select the model that best fits their experimental needs. While chinchillas and rats have been favored animals to date for OM research, recently, many mouse models have been developed through NP colonization and ME infection. Many groups have used these models to demonstrate induction of protection against experimental OM and NP carriage following IN vaccine administration. Knockout and transgenic mice have proved to be vital for revealing the roles of different components of a host immune response relevant to susceptibility. Furthermore, the humanized mouse model offers the potential to execute a range of studies on human immunity in OM. However, there is still a need to develop an appropriate OM model that will allow rapid and high throughput assessment of Mcat vaccine candidates in preventing disease. Moreover, a mouse model of OM developed using polymicrobial infection (NTHi, S. pneumoniae, and Mcat) would allow the assessment of polymicrobial OM vaccines. Until we have a vaccine effective against the three major otopathogens, the global burden of acute and chronic OM morbidity will continue.
AZ: Conceptualization, Writing – original draft, Writing – review & editing. JW: Supervision, Writing – review & editing. IG: Supervision, Writing – review & editing. IP: Supervision, Writing – review & editing.
The author(s) declare financial support was received for the research, authorship, and/or publication of this article. Harvey Coates PhD Scholarship from the Earbus Foundation of Western Australia, along with GUPRS and GUIPRS Scholarships for AZ are acknowledged.
We are grateful to the Earbus Foundation of Western Australia for funding the OM vaccine project and granting the Harvey Coates Fellowship to AZ.
The authors declare that the research was conducted in the absence of any commercial or financial relationships that could be construed as a potential conflict of interest.
All claims expressed in this article are solely those of the authors and do not necessarily represent those of their affiliated organizations, or those of the publisher, the editors and the reviewers. Any product that may be evaluated in this article, or claim that may be made by its manufacturer, is not guaranteed or endorsed by the publisher.
Abdelnour, A., Arguedas, A., Dagan, R., Soley, C., Porat, N., Castrejon, M. M., et al. (2015). Etiology and antimicrobial susceptibility of middle ear fluid pathogens in Costa Rican children with otitis media before and after the introduction of the 7-valent pneumococcal conjugate vaccine in the National Immunization Program: acute otitis media microbiology in Costa Rican children. Medicine 94:e320. doi: 10.1097/MD.0000000000000320
Abe, N., Kodama, S., Hirano, T., Eto, M., and Suzuki, M. (2006). Nasal vaccination with CpG oligodeoxynucleotide induces protective immunity against nontypeable Haemophilus influenzae in the nasopharynx. Laryngoscope 116, 407–412. doi: 10.1097/01.mlg.0000199740.04730.d4
ACIP (2023). "ACIP updates: recommendations for use of 20-valent pneumococcal conjugate vaccine in children-United States, 2023", in: MMWR Morb mortal Wkly rep. 72, 1072.
Acuin, J. (2004). “Chronic suppurative otitis media: burden of illness and management options” in Chronic suppurative otitis media: Burden of illness and management options, 83.
Adlowitz, D. G., Hiltke, T., Lesse, A. J., and Murphy, T. F. (2004). Identification and characterization of outer membrane proteins G1a and G1b of Moraxella catarrhalis. Vaccine 22, 2533–2540. doi: 10.1016/j.vaccine.2003.12.016
Adlowitz, D. G., Kirkham, C., Sethi, S., and Murphy, T. F. (2006). Human serum and mucosal antibody responses to outer membrane protein G1b of Moraxella catarrhalis in chronic obstructive pulmonary disease. FEMS Immunol. Med. Microbiol. 46, 139–146. doi: 10.1111/j.1574-695X.2005.00020.x
Afolabi, O., Salaudeen, A., Ologe, F., Nwabuisi, C., and Nwawolo, C. (2012). Pattern of bacterial isolates in the middle ear discharge of patients with chronic suppurative otitis media in a tertiary hospital in north Central Nigeria. Afr. Health Sci. 12, 362–367.
Akbari, E., Negahdari, B., Faraji, F., Behdani, M., Kazemi-Lomedasht, F., and Habibi-Anbouhi, M. (2019). Protective responses of an engineered PspA recombinant antigen against Streptococcus pneumoniae. Biotechnol. Rep. 24:e00385. doi: 10.1016/j.btre.2019.e00385
Alexander, J. E., Lock, R. A., Peeters, C., Poolman, J. T., Andrew, P. W., Mitchell, T. J., et al. (1994). Immunization of mice with pneumolysin toxoid confers a significant degree of protection against at least nine serotypes of Streptococcus pneumoniae. Infect. Immun. 62, 5683–5688. doi: 10.1128/iai.62.12.5683-5688.1994
Alho, O. P., Koivu, M., and Sorri, M. (1991). What is an 'otitis-prone' child? Int. J. Pediatr. Otorhinolaryngol. 21, 201–209. doi: 10.1016/0165-5876(91)90001-r
Andrade, D. C., Borges, I. C., Bouzas, M. L., Oliveira, J. R., Käyhty, H., Ruuskanen, O., et al. (2018). Antibody responses against Streptococcus pneumoniae, Haemophilus influenzae and Moraxella catarrhalis in children with acute respiratory infection with or without nasopharyngeal bacterial carriage. Infect. Dis. 50, 705–713. doi: 10.1080/23744235.2018.1463451
Andreas, S., Testa, M., Boyer, L., Brusselle, G., Janssens, W., Kerwin, E., et al. (2022). Non-typeable Haemophilus influenzae–Moraxella catarrhalis vaccine for the prevention of exacerbations in chronic obstructive pulmonary disease: a multicentre, randomised, placebo-controlled, observer-blinded, proof-of-concept, phase 2b trial. Lancet Respir. Med. 10, 435–446. doi: 10.1016/S2213-2600(21)00502-6
Apicella, M. A., Coffin, J., Ketterer, M., Post, D. M. B., Day, C. J., Jen, F. E., et al. (2018). Nontypeable Haemophilus influenzae Lipooligosaccharide expresses a terminal Ketodeoxyoctanoate in vivo, which can be used as a target for bactericidal antibody. MBio 9, 10–1128. doi: 10.1128/mBio.01401-18
Appell, L., Kovatch, R., Reddecliff, J., and Gerone, P. J. (1971). Pathogenesis of Sendai virus infection in mice. Am. J. Vet. Res. 32, 1835–1841.
Armbruster, C. E., Hong, W., Pang, B., Weimer, K. E., Juneau, R. A., Turner, J., et al. (2010). Indirect pathogenicity of Haemophilus influenzae and Moraxella catarrhalis in polymicrobial otitis media occurs via interspecies quorum signaling. MBio 1, 10–1128. doi: 10.1128/mBio.00102-10
Arora, A. K., Chinsky, K., Keller, C., Mayers, I., Pascual-Guardia, S., Vera, M. P., et al. (2022). A detailed analysis of possible efficacy signals of NTHi-Mcat vaccine against severe COPD exacerbations in a previously reported randomised phase 2b trial. Vaccine 40, 5924–5932. doi: 10.1016/j.vaccine.2022.08.053
Aynali, G., Yariktaş, M., Yasan, H., Karahan, N., Başpinar, S., Tüz, M., et al. (2011). The effects of methylprednisolone, montelukast and indomethacine in experimental otitis media with effusion. Int. J. Pediatr. Otorhinolaryngol. 75, 15–19. doi: 10.1016/j.ijporl.2010.09.024
Azar, A., Piccinelli, C., Brown, H., Headon, D., and Cheeseman, M. (2016). Ectodysplasin signalling deficiency in mouse models of hypohidrotic ectodermal dysplasia leads to middle ear and nasal pathology. Hum. Mol. Genet. 25, 3564–3577. doi: 10.1093/hmg/ddw202
Bafroee, A. S. T., Siadat, S. D., Mousavi, S. F., Aghasadeghi, M. R., Khorsand, H., Nejati, M., et al. (2016). Recombinant C-terminal 311 amino acids of HapS adhesin as a vaccine candidate for nontypeable Haemophilus influenzae: a study on immunoreactivity in Balb/C mouse. Microb. Pathog. 98, 106–111. doi: 10.1016/j.micpath.2016.06.033
Bai, X.-H., Chen, H.-J., Jiang, Y.-L., Wen, Z., Huang, Y., Cheng, W., et al. (2014). Structure of pneumococcal peptidoglycan hydrolase LytB reveals insights into the bacterial cell wall remodeling and pathogenesis. J. Biol. Chem. 289, 23403–23416. doi: 10.1074/jbc.M114.579714
Bakaletz, L. O. (2009). Chinchilla as a robust, reproducible and polymicrobial model of otitis media and its prevention. Expert Rev. Vaccines 8, 1063–1082. doi: 10.1586/erv.09.63
Bakaletz, L. O., Kennedy, B.-J., Novotny, L. A., Duquesne, G., Cohen, J., and Lobet, Y. (1999). Protection against development of otitis media induced by nontypeable Haemophilus influenzae by both active and passive immunization in a chinchilla model of virus-bacterium superinfection. Infect. Immun. 67, 2746–2762. doi: 10.1128/IAI.67.6.2746-2762.1999
Balder, R., Hassel, J., Lipski, S., and Lafontaine, E. R. (2007). Moraxella catarrhalis strain O35E expresses two filamentous hemagglutinin-like proteins that mediate adherence to human epithelial cells. Infect. Immun. 75, 2765–2775. doi: 10.1128/IAI.00079-07
Balder, R., Shaffer, T., Wiese, L., and LaFontaine, E. (2011). “Vaccination with Moraxella catarrhalis MhaB1/B2 proteins enhance clearance in a chinchilla nasopharyngeal colonization model” in American Society for Microbiology general meeting (New Orleans LA2011).
Barenkamp, S. J. (2013). A new human colonization model for nontypeable Haemophilus influenzae. J. Infect. Dis. 208, 717–719. doi: 10.1093/infdis/jit242
Barenkamp, S. J., Chonmaitree, T., Hakansson, A. P., Heikkinen, T., King, S., Nokso-Koivisto, J., et al. (2017). Panel 4: report of the microbiology panel. Otolaryngol. Head Neck Surg. 156, S51–S62.
Behrouzi, A., Bouzari, S., Vaziri, F., Fateh, A., Afrough, P., Vijeh Motlagh, A. D., et al. (2017). Recombinant truncated E protein as a new vaccine candidate against nontypeable H. influenzae: its expression and immunogenic evaluation. Microb. Pathog. 110, 431–438. doi: 10.1016/j.micpath.2017.07.025
Behrouzi, A., Mianroodi, R. A., Afrough, P., Ayadi, A., and Serajian, A. (2020). Evaluation of immunological responses against outer membrane vesicles (OMV) of nontypeable Haemophilus influenzae using MPLA-CpG adjuvant as a vaccine candidate. Iran. J. Microbiol. 12, 417–423. doi: 10.18502/ijm.v12i5.4602
Beissbarth, J., Wilson, N., Arrowsmith, B., Binks, M. J., Oguoma, V., Lawrence, K., et al. (2021). Nasopharyngeal carriage of otitis media pathogens in infants receiving 10-valent non-typeable Haemophilus influenzae protein D conjugate vaccine (PHiD-CV10), 13-valent pneumococcal conjugate vaccine (PCV13) or a mixed primary schedule of both vaccines: a randomised controlled trial. Vaccine 39, 2264–2273. doi: 10.1016/j.vaccine.2021.03.032
Ben-Shimol, S., Givon-Lavi, N., Kotler, L., Greenberg, D., and Dagan, R. (2019). “2903. Post PCV13 dynamics of NonVaccine serotype (NVT): disproportionate increase of the additional PCV20 candidate serotypes in respiratory and invasive disease in young children” in Open forum infectious diseases
Ben-Shimol, S., Givon-Lavi, N., Leibovitz, E., Raiz, S., Greenberg, D., and Dagan, R. (2014). Near-elimination of otitis media caused by 13-valent pneumococcal conjugate vaccine (PCV) serotypes in southern Israel shortly after sequential introduction of 7-valent/13-valent PCV. Clin. Infect. Dis. 59, 1724–1732. doi: 10.1093/cid/ciu683
Bertot, G. M., Becker, P. D., Guzmán, C. A., and Grinstein, S. (2004). Intranasal vaccination with recombinant P6 protein and adamantylamide dipeptide as mucosal adjuvant confers efficient protection against otitis media and lung infection by nontypeable Haemophilus influenzae. J. Infect. Dis. 189, 1304–1312. doi: 10.1086/382508
Bhushan, R., Kirkham, C., Sethi, S., and Murphy, T. F. (1997). Antigenic characterization and analysis of the human immune response to outer membrane protein E of Branhamella catarrhalis. Infect. Immun. 65, 2668–2675. doi: 10.1128/iai.65.7.2668-2675.1997
Bhutta, M. F. (2012). Mouse models of otitis media: strengths and limitations. Otolaryngol. Head Neck Surg. 147, 611–614.
Blakeway, L. V., Tan, A., Jurcisek, J. A., Bakaletz, L. O., Atack, J. M., Peak, I. R., et al. (2019). The Moraxella catarrhalis phase-variable DNA methyltransferase ModM3 is an epigenetic regulator that affects bacterial survival in an in vivo model of otitis media. BMC Microbiol. 19, 1–14. doi: 10.1186/s12866-019-1660-y
Bluestone, C. D. (1998). Epidemiology and pathogenesis of chronic suppurative otitis media: implications for prevention and treatment. Int. J. Pediatr. Otorhinolaryngol. 42, 207–223. doi: 10.1016/S0165-5876(97)00147-X
Bologa, M., Kamtchoua, T., Hopfer, R., Sheng, X., Hicks, B., Bixler, G., et al. (2012). Safety and immunogenicity of pneumococcal protein vaccine candidates: monovalent choline-binding protein a (PcpA) vaccine and bivalent PcpA–pneumococcal histidine triad protein D vaccine. Vaccine 30, 7461–7468. doi: 10.1016/j.vaccine.2012.10.076
Borrow, R., Heath, P. T., and Siegrist, C. A. (2012). Use of pneumococcal polysaccharide vaccine in children: what is the evidence? Curr. Opin. Infect. Dis. 25, 292–303. doi: 10.1097/QCO.0b013e3283531b0f
Briles, D. E., Ades, E., Paton, J. C., Sampson, J. S., Carlone, G. M., Huebner, R. C., et al. (2000a). Intranasal immunization of mice with a mixture of the pneumococcal proteins PsaA and PspA is highly protective against nasopharyngeal carriage of Streptococcus pneumoniae. Infect. Immun. 68, 796–800. doi: 10.1128/IAI.68.2.796-800.2000
Briles, D. E., Hollingshead, S. K., King, J., Swift, A., Braun, P. A., Park, M. K., et al. (2000b). Immunization of humans with recombinant pneumococcal surface protein a (rPspA) elicits antibodies that passively protect mice from fatal infection with Streptococcus pneumoniae bearing heterologous PspA. J. Infect. Dis. 182, 1694–1701. doi: 10.1086/317602
Brockson, M. E., Novotny, L. A., Jurcisek, J. A., McGillivary, G., Bowers, M. R., and Bakaletz, L. O. (2012). Respiratory syncytial virus promotes Moraxella catarrhalis-induced ascending experimental otitis media. PLoS One 7:e40088. doi: 10.1371/journal.pone.0040088
Brook, I. (2008). The role of anaerobic bacteria in chronic suppurative otitis media in children: implications for medical therapy. Anaerobe 14, 297–300. doi: 10.1016/j.anaerobe.2008.12.002
Brookes, R. H., Ming, M., Williams, K., Hopfer, R., Gurunathan, S., Gallichan, S., et al. (2015). Passive protection of mice against Streptococcus pneumoniae challenge by naturally occurring and vaccine-induced human anti-PhtD antibodies. Hum. Vaccin. Immunother. 11, 1836–1839. doi: 10.1080/21645515.2015.1039210
Brooks, W. A., Chang, L.-J., Sheng, X., Hopfer, R., and Team, P. S. (2015). Safety and immunogenicity of a trivalent recombinant PcpA, PhtD, and PlyD1 pneumococcal protein vaccine in adults, toddlers, and infants: a phase I randomized controlled study. Vaccine 33, 4610–4617. doi: 10.1016/j.vaccine.2015.06.078
Bullard, B., Lipski, S. L., and Lafontaine, E. R. (2005). Hag directly mediates the adherence of Moraxella catarrhalis to human middle ear cells. Infect. Immun. 73, 5127–5136. doi: 10.1128/IAI.73.8.5127-5136.2005
Campagnari, A. A., Gupta, M., Dudas, K., Murphy, T., and Apicella, M. (1987). Antigenic diversity of lipooligosaccharides of nontypable Haemophilus influenzae. Infect. Immun. 55, 882–887. doi: 10.1128/iai.55.4.882-887.1987
Casey, J. R., Adlowitz, D. G., and Pichichero, M. E. (2010). New patterns in the otopathogens causing acute otitis media six to eight years after introduction of pneumococcal conjugate vaccine. Pediatr. Infect. Dis. J. 29, 304–309. doi: 10.1097/INF.0b013e3181c1bc48
Cayé-Thomasen, P., and Tos, M. (2002). Histopathologic differences due to bacterial species in acute otitis media. Int. J. Pediatr. Otorhinolaryngol. 63, 99–110. doi: 10.1016/S0165-5876(01)00641-3
CDC (2019). Pneumococcal Polysaccharide Vaccine (PPSV23): What You Need to Know. Available at: https://www.cdc.gov/vaccines/hcp/vis/vis-statements/ppv.html (Accessed December 20, 2023).
CDC (2020). Global Pneumococcal Disease and Vaccination. Available at: https://www.cdc.gov/pneumococcal/global.html (Accessed November 2023).
Cecchini, P., Entwisle, C., Joachim, M., Pang, Y., Dalton, K. A., Hill, S., et al. (2015). Next generation vaccines: development of a novel Streptococcus pneumoniae multivalent protein vaccine. Bioprocess. J. 14, 18–33.
Cenjor, C., Ponte, C., Parra, A., Nieto, E., García-Calvo, G., Giménez, M. J., et al. (1998). In vivo efficacies of amoxicillin and cefuroxime against penicillin-resistant Streptococcus pneumoniae in a gerbil model of acute otitis media. Antimicrob. Agents Chemother. 42, 1361–1364. doi: 10.1128/aac.42.6.1361
Chan, W. Y., Entwisle, C., Ercoli, G., Ramos-Sevillano, E., McIlgorm, A., Cecchini, P., et al. (2022). Corrected and republished from: "a novel, multiple-antigen pneumococcal vaccine protects against lethal Streptococcus pneumoniae challenge". Infect. Immun. 90:e0084618a. doi: 10.1128/IAI.00846-18a
Chaney, E. J., Nguyen, C. T., and Boppart, S. A. (2011). Novel method for non-invasive induction of a middle-ear biofilm in the rat. Vaccine 29, 1628–1633. doi: 10.1016/j.vaccine.2010.12.076
Chen, D., McMichael, J. C., VanDerMeid, K. R., Hahn, D., Mininni, T., Cowell, J., et al. (1996). Evaluation of purified UspA from Moraxella catarrhalis as a vaccine in a murine model after active immunization. Infect. Immun. 64, 1900–1905. doi: 10.1128/iai.64.6.1900-1905.1996
Chole, R. A., Henry, K. R., and McGinn, M. D. (1981). Cholesteatoma: spontaneous occurrence in the Mongolian gerbil Meriones unguiculatis. Otol. Neurotol. 2, 204–210.
Coker, T. R., Chan, L. S., Newberry, S. J., Limbos, M. A., Suttorp, M. J., Shekelle, P. G., et al. (2010). Diagnosis, microbial epidemiology, and antibiotic treatment of acute otitis media in children: a systematic review. JAMA 304, 2161–2169. doi: 10.1001/jama.2010.1651
Corsini, B., Aguinagalde, L., Ruiz, S., Domenech, M., Antequera, M. L., Fenoll, A., et al. (2016). Immunization with LytB protein of Streptococcus pneumoniae activates complement-mediated phagocytosis and induces protection against pneumonia and sepsis. Vaccine 34, 6148–6157. doi: 10.1016/j.vaccine.2016.11.001
Crompton, M., Purnell, T., Tyrer, H. E., Parker, A., Ball, G., Hardisty-Hughes, R. E., et al. (2017). A mutation in Nischarin causes otitis media via LIMK1 and NF-κB pathways. PLoS Genet. 13:e1006969. doi: 10.1371/journal.pgen.1006969
Cutter, D., Mason, K. W., Howell, A. P., Fink, D. L., Green, B. A., and St. Geme, III J.W. (2002). Immunization with Haemophilus influenzae hap adhesin protects against nasopharyngeal colonization in experimental mice. J. Infect. Dis. 186, 1115–1121. doi: 10.1086/344233
D’Mello, A., Ahearn, C. P., Murphy, T. F., and Tettelin, H. (2019). ReVac: a reverse vaccinology computational pipeline for prioritization of prokaryotic protein vaccine candidates. BMC Genomics 20, 1–21. doi: 10.1186/s12864-019-6195-y
Dai, C., and Gan, R. Z. (2008). Change of middle ear transfer function in otitis media with effusion model of guinea pigs. Hear. Res. 243, 78–86. doi: 10.1016/j.heares.2008.05.010
Dai, C., Li, W., and Gan, R. (2009). “Change of cochlear mechanics in acute otitis media and otitis media with effusion” in Association for Research in otolaryngology (ARO)-midwinter meeting, vol. 984
Daly, K. A., Hoffman, H. J., Kvaerner, K. J., Kvestad, E., Casselbrant, M. L., Homoe, P., et al. (2010). Epidemiology, natural history, and risk factors: panel report from the ninth international research conference on otitis media. Int. J. Pediatr. Otorhinolaryngol. 74, 231–240. doi: 10.1016/j.ijporl.2009.09.006
Daniel, H. J. III, Brinn, J. E., Fulghum, R. S., and Barrett, K. A. (1982). Comparative anatomy of eustachian tube and middle ear cavity in animal models for otitis media. Ann. Otol. Rhinol. Laryngol. 91, 82–89. doi: 10.1177/000348948209100118
Daniel, M., Imtiaz-Umer, S., Fergie, N., Birchall, J., and Bayston, R. (2012). Bacterial involvement in otitis media with effusion. Int. J. Pediatr. Otorhinolaryngol. 76, 1416–1422. doi: 10.1016/j.ijporl.2012.06.013
Das, J., Mokrzan, E., Lakhani, V., Rosas, L., Jurcisek, J. A., Ray, W. C., et al. (2017). Extracellular DNA and type IV pilus expression regulate the structure and kinetics of biofilm formation by nontypeable Haemophilus influenzae. MBio 8, e01466–e01417. doi: 10.1128/mBio.01466-17
Davidoss, N., Varsak, Y., and Santa Maria, P. (2018). Animal models of acute otitis media–a review with practical implications for laboratory research. Eur. Ann. Otorhinolaryngol. Head Neck Dis. 135, 183–190. doi: 10.1016/j.anorl.2017.06.013
Davoudi Vijeh Motlagh, A., Siadat, S. D., Abedian Kenari, S., Mahdavi, M., Behrouzi, A., and Asgarian-Omran, H. (2016). Immunization with protein D from non-Typeable Haemophilus influenzae (NTHi) induced cytokine responses and bioactive antibody production. Jundishapur J. Microbiol. 9:e36617. doi: 10.5812/jjm.36617
de Sévaux, J. L., Venekamp, R. P., Lutje, V., Hak, E., Schilder, A. G., Sanders, E. A., et al. (2020). Pneumococcal conjugate vaccines for preventing acute otitis media in children. Cochrane Database Syst. Rev. 11.
De Smedt, P., Leroux-Roels, G., Vandermeulen, C., Tasciotti, A., Di Maro, G., Dozot, M., et al. (2021). Long-term immunogenicity and safety of a non-typeable Haemophilus influenzae-Moraxella catarrhalis vaccine: 4-year follow-up of a phase 1 multicentre trial. Vaccine X 9:100124. doi: 10.1016/j.jvacx.2021.100124
DeMaria, T., Murwin, D. M., and Leake, E. R. (1996). Immunization with outer membrane protein P6 from nontypeable Haemophilus influenzae induces bactericidal antibody and affords protection in the chinchilla model of otitis media. Infect. Immun. 64, 5187–5192. doi: 10.1128/iai.64.12.5187-5192.1996
Deniffel, D., Nuyen, B., Pak, K., Suzukawa, K., Hung, J., Kurabi, A., et al. (2017). Otitis media and nasopharyngeal colonization in ccl3−/− mice. Infect. Immun. 85:1128. doi: 10.1128/iai
Denoël, P., Philipp, M. T., Doyle, L., Martin, D., Carletti, G., and Poolman, J. T. (2011). A protein-based pneumococcal vaccine protects rhesus macaques from pneumonia after experimental infection with Streptococcus pneumoniae. Vaccine 29, 5495–5501. doi: 10.1016/j.vaccine.2011.05.051
Depreux, F. F., Darrow, K., Conner, D. A., Eavey, R. D., Liberman, M. C., Seidman, C. E., et al. (2008). Eya4-deficient mice are a model for heritable otitis media. J. Clin. Invest. 118, 651–658. doi: 10.1172/jci32899
Dewan, K. K., Taylor-Mulneix, D. L., and Campos, L. L. (2019). A model of chronic, transmissible otitis media in mice. PLoS Pathog. 15:e1007696. doi: 10.1371/journal.ppat.1007696
Dohar, J. E., Alper, C. M., Rose, E. A., Doyle, W. J., Casselbrant, M. L., Kenna, M. A., et al. (1998). Treatment of chronic suppurative otitis media with topical ciprofloxacin. Ann. Otol. Rhinol. Laryngol. 107, 865–871. doi: 10.1177/000348949810701010
Dohar, J. E., Hebda, P. A., Veeh, R., Awad, M., Costerton, J. W., Hayes, J., et al. (2005). Mucosal biofilm formation on middle-ear mucosa in a nonhuman primate model of chronic suppurative otitis media. Laryngoscope 115, 1469–1472. doi: 10.1097/01.mlg.0000172036.82897.d4
Doherty, P. C., Turner, S. J., Webby, R. G., and Thomas, P. G. (2006). Influenza and the challenge for immunology. Nat. Immunol. 7, 449–455. doi: 10.1038/ni1343
Doyle, W. J. (1989). Animal models of otitis media: other pathogens. Pediatr. Infect. Dis. J. 8:S48. doi: 10.1097/00006454-198901001-00018
Doyle, W. J., Casselbrant, M. L., and Fireman, P. (1985). Monkey models of otitis media. Ann. Otol. Rhinol. Laryngol. 94, 36–37. doi: 10.1177/00034894850945S226
Du, R.-P., Wang, Q., Yang, Y.-P., Schryvers, A. B., Chong, P., Klein, M. H., et al. (1998). Cloning and expression of the Moraxella catarrhalis lactoferrin receptor genes. Infect. Immun. 66, 3656–3665. doi: 10.1128/IAI.66.8.3656-3665.1998
Duke, J. A., and Avci, F. Y. (2023). Emerging vaccine strategies against the incessant pneumococcal disease. npj Vacc. 8:122. doi: 10.1038/s41541-023-00715-w
Easton, D. M., Cripps, A. W., Foxwell, A. R., and Kyd, J. M. (2011). Mucosal immunization with the Moraxella catarrhalis porin M35 induces enhanced bacterial clearance from the lung: a possible role for opsonophagocytosis. Front. Immunol. 2:13. doi: 10.3389/fimmu.2011.00013
Easton, D. M., Maier, E., Benz, R., Foxwell, A. R., Cripps, A. W., and Kyd, J. M. (2008). Moraxella catarrhalis M35 is a general porin that is important for growth under nutrient-limiting conditions and in the nasopharynges of mice. J. Bacteriol. 190, 7994–8002. doi: 10.1128/JB.01039-08
Easton, D. M., Smith, A., Gallego, S. G., Foxwell, A. R., Cripps, A. W., and Kyd, J. M. (2005). Characterization of a novel porin protein from Moraxella catarrhalis and identification of an immunodominant surface loop. J. Bacteriol. 187, 6528–6535. doi: 10.1128/JB.187.18.6528-6535.2005
Engle, J. R., Tinling, S., and Recanzone, G. H. (2013). Age-related hearing loss in rhesus monkeys is correlated with cochlear histopathologies. PLoS One 8:e55092. doi: 10.1371/journal.pone.0055092
Entwisle, C., Hill, S., Pang, Y., Joachim, M., McIlgorm, A., Colaco, C., et al. (2017). Safety and immunogenicity of a novel multiple antigen pneumococcal vaccine in adults: a phase 1 randomised clinical trial. Vaccine 35, 7181–7186. doi: 10.1016/j.vaccine.2017.10.076
Faisca, P., and Desmecht, D. (2007). Sendai virus, the mouse parainfluenza type 1: a longstanding pathogen that remains up-to-date. Res. Vet. Sci. 82, 115–125. doi: 10.1016/j.rvsc.2006.03.009
Forsgren, A., Brant, M., Karamehmedovic, M., and Riesbeck, K. (2003). The immunoglobulin D-binding protein MID from Moraxella catarrhalis is also an adhesin. Infect. Immun. 71, 3302–3309. doi: 10.1128/IAI.71.6.3302-3309.2003
Forsgren, A., Brant, M., and Riesbeck, K. (2004). Immunization with the truncated Adhesin Moraxella catarrhalis immunoglobulin D—binding protein (MID764–913) is protective against M. catarrhalis in a mouse model of pulmonary clearance. J. Infect. Dis. 190, 352–355. doi: 10.1086/422155
Forsgren, A., Riesbeck, K., and Janson, H. (2008). Protein D of Haemophilus influenzae: a protective nontypeable H. influenzae antigen and a carrier for pneumococcal conjugate vaccines. Clin. Infect. Dis. 46, 726–731. doi: 10.1086/527396
Fox, K. L., Atack, J. M., Srikhanta, Y. N., Eckert, A., Novotny, L. A., Bakaletz, L. O., et al. (2014). Selection for phase variation of LOS biosynthetic genes frequently occurs in progression of non-typeable Haemophilus influenzae infection from the nasopharynx to the middle ear of human patients. PLoS One 9:e90505. doi: 10.1371/journal.pone.0090505
Foxwell, A. R., Kyd, J. M., and Cripps, A. W. (1998). Nontypeable Haemophilus influenzae: pathogenesis and prevention. Microbiol. Mol. Biol. Rev. 62, 294–308. doi: 10.1128/MMBR.62.2.294-308.1998
Frey, S. E., Lottenbach, K. R., Hill, H., Blevins, T. P., Yu, Y., Zhang, Y., et al. (2013). A phase I, dose-escalation trial in adults of three recombinant attenuated Salmonella Typhi vaccine vectors producing Streptococcus pneumoniae surface protein antigen PspA. Vaccine 31, 4874–4880. doi: 10.1016/j.vaccine.2013.07.049
Friedman, R., Doyle, W., Fagin, J., Bluestone, C., and Fireman, P. (1981). 912 a MONKEY MODEL FOR IgE MEDIATED OTITIS MEDIA WITH EFFUSION. Pediatr. Res. 15:594. doi: 10.1203/00006450-198104001-00937
Fulghum, R. S., Brinn, J. E., Smith, A. M., Daniel, H. J. 3rd, and Loesche, P. J. (1982). Experimental otitis media in gerbils and chinchillas with Streptococcus pneumoniae, Haemophilus influenzae, and other aerobic and anaerobic bacteria. Infect. Immun. 36, 802–810. doi: 10.1128/iai.36.2.802-810.1982
Fulghum, R. S., Hoogmoed, R. P., and Brinn, J. E. (1985). Longitudinal studies of experimental otitis media with Haemophilus influenzae in the gerbil. Int. J. Pediatr. Otorhinolaryngol. 9, 101–114. doi: 10.1016/S0165-5876(85)80010-0
Funke, B., Epstein, J. A., Kochilas, L. K., Lu, M. M., Pandita, R. K., Liao, J., et al. (2001). Mice overexpressing genes from the 22q11 region deleted in velo-cardio-facial syndrome/DiGeorge syndrome have middle and inner ear defects. Hum. Mol. Genet. 10, 2549–2556. doi: 10.1093/hmg/10.22.2549
Galgani, I., Annaratone, M., Casula, D., Di Maro, G., Janssens, M., Tasciotti, A., et al. (2022). Safety and immunogenicity of three doses of non-typeable Haemophilus influenzae-Moraxella catarrhalis (NTHi-Mcat) vaccine when administered according to two different schedules: a phase 2, randomised, observer-blind study. Respir. Res. 23:114. doi: 10.1186/s12931-022-02019-4
Ganaie, F., Saad, J. S., McGee, L., van Tonder, A. J., Bentley, S. D., Lo, S. W., et al. (2020). A new pneumococcal capsule type, 10D, is the 100th serotype and has a large cps fragment from an oral streptococcus. MBio 11, e00937–e00920. doi: 10.1128/mBio.00937-20
Gene, A., del Amo, E., Iñigo, M., Monsonis, M., Pallares, R., and Muñoz-Almagro, C. (2013). Pneumococcal serotypes causing acute otitis media among children in Barcelona (1992-2011): emergence of the multiresistant clone ST320 of serotype 19A. Pediatr. Infect. Dis. J. 32, e128–e133. doi: 10.1097/INF.0b013e31827c54dc
Geng, R., Wang, Q., Chen, E., and Zheng, Q. Y. (2019). Current understanding of host genetics of otitis media. Front. Genet. 10:1395. doi: 10.3389/fgene.2019.01395
Ginsburg, A. S., Nahm, M. H., Khambaty, F. M., and Alderson, M. R. (2012). Issues and challenges in the development of pneumococcal protein vaccines. Expert Rev. Vaccines 11, 279–285. doi: 10.1586/erv.12.5
Glover, D. T., Hollingshead, S. K., and Briles, D. E. (2008). Streptococcus pneumoniae surface protein PcpA elicits protection against lung infection and fatal sepsis. Infect. Immun. 76, 2767–2776. doi: 10.1128/IAI.01126-07
González-Miro, M., Rodríguez-Noda, L., Fariñas-Medina, M., García-Rivera, D., Vérez-Bencomo, V., and Rehm, B. H. (2017). Self-assembled particulate PsaA as vaccine against Streptococcus pneumoniae infection. Heliyon 3:e00291. doi: 10.1016/j.heliyon.2017.e00291
Grabenstein, J., and Klugman, K. (2012). A century of pneumococcal vaccination research in humans. Clin. Microbiol. Infect. 18, 15–24. doi: 10.1111/j.1469-0691.2012.03943.x
Granath, A. (2017). Recurrent acute otitis media: what are the options for treatment and prevention? Curr. Otorhinolaryngol. Rep. 5, 93–100. doi: 10.1007/s40136-017-0151-7
Gregersen, N., and Bross, P. (2010). Protein misfolding and cellular stress: an overview. Protein Misfolding Cellular Stress Disease Aging, 3–23. doi: 10.1007/978-1-60761-756-3_1
Guan, X., Chen, Y., and Gan, R. Z. (2014). Factors affecting loss of tympanic membrane mobility in acute otitis media model of chinchilla. Hear. Res. 309, 136–146. doi: 10.1016/j.heares.2013.12.005
Guan, X., and Gan, R. Z. (2013). Mechanisms of tympanic membrane and incus mobility loss in acute otitis media model of guinea pig. J. Assoc. Res. Otolaryngol. 14, 295–307. doi: 10.1007/s10162-013-0379-y
Hardisty, R. E., Erven, A., Logan, K., Morse, S., Guionaud, S., Sancho–Oliver, S., et al. (2003). The deaf mouse mutant Jeff (Jf) is a single gene model of otitis media. J. Assoc. Res. Otolaryngol. 4, 130–138. doi: 10.1007/s10162-002-3015-9
Hardisty-Hughes, R. E., Tateossian, H., Morse, S. A., Romero, M. R., Middleton, A., Tymowska-Lalanne, Z., et al. (2006). A mutation in the F-box gene, Fbxo11, causes otitis media in the Jeff mouse. Hum. Mol. Genet. 15, 3273–3279. doi: 10.1093/hmg/ddl403
Hardy, S. M., Heavner, S. B., White, D. R., Mcqueen, C. T., Prazma, J., and Pillsbury, H. C. (2001). Late-phase allergy and eustachian tube dysfunction. Otolaryngol. Head Neck Surg. 125, 339–345. doi: 10.1067/mhn.2001.119140
Hassan, F., Ren, D., Zhang, W., Merkel, T. J., and Gu, X.-X. (2012). Moraxella catarrhalis activates murine macrophages through multiple toll like receptors and has reduced clearance in lungs from TLR4 mutant mice. PLoS One 7:e37610. doi: 10.1371/journal.pone.0037610
Hellstorm, S., and Stenfors, L.-E. (1983). The pressure equilibrating function of pars flaccida in middle ear mechanics. Acta Physiol. Scand. 118, 337–341. doi: 10.1111/j.1748-1716.1983.tb07280.x
Hellström, S., Salén, B., and Stenfors, L.-E. (1982). Anatomy of the rat middle ear: a study under the dissection microscope. Cells Tissues Organs 112, 346–352. doi: 10.1159/000145527
Hermansson, A., Emgrd, P., Prellner, K., and Hellström, S. (1988). A rat model for pneumococcal otitis media. Am. J. Otolaryngol. 9, 97–101. doi: 10.1016/S0196-0709(88)80013-9
Hill, S., Entwisle, C., Pang, Y., Joachim, M., McIlgorm, A., Dalton, K., et al. (2018). Immunogenicity and mechanisms of action of PnuBioVax, a multi-antigen serotype-independent prophylactic vaccine against infection with Streptococcus pneumoniae. Vaccine 36, 4255–4264. doi: 10.1016/j.vaccine.2018.05.122
Hirano, T., Hou, Y., Jiao, X., and Gu, X.-X. (2003). Intranasal immunization with a lipooligosaccharide-based conjugate vaccine from nontypeable Haemophilus influenzae enhances bacterial clearance in mouse nasopharynx. FEMS Immunol. Med. Microbiol. 35, 1–10. doi: 10.1111/j.1574-695X.2003.tb00642.x
Holme, T., Rahman, M., Jansson, P. E., and Widmalm, G. (1999). The lipopolysaccharide of Moraxella catarrhalis: structural relationships and antigenic properties. Eur. J. Biochem. 265, 524–529. doi: 10.1046/j.1432-1327.1999.00731.x
Hood, D., Moxon, R., Purnell, T., Richter, C., Williams, D., Azar, A., et al. (2016). A new model for non-typeable Haemophilus influenzae middle ear infection in the Junbo mutant mouse. Dis. Model. Mech. 9, 69–79. doi: 10.1242/dmm.021659
Huang, C.-C., Shi, G.-S., and Yi, Z.-X. (1988). Experimental induction of middle ear cholesteatoma in rats. Am. J. Otolaryngol. 9, 165–172. doi: 10.1016/S0196-0709(88)80024-3
Huang, Q., Zhang, Z., Zheng, Y., Zheng, Q., Chen, S., Xu, Y., et al. (2012). Hypoxia-inducible factor and vascular endothelial growth factor pathway for the study of hypoxia in a new model of otitis media with effusion. Audiol. Neurootol. 17, 349–356. doi: 10.1159/000341163
Jalalvand, F., Littorin, N., Su, Y.-C., and Riesbeck, K. (2014). Impact of immunization with protein F on pulmonary clearance of nontypeable Haemophilus influenzae. Vaccine 32, 2261–2264. doi: 10.1016/j.vaccine.2014.02.082
Jalalvand, F., and Riesbeck, K. (2018). Update on non-typeable Haemophilus influenzae-mediated disease and vaccine development. Expert Rev. Vaccines 17, 503–512. doi: 10.1080/14760584.2018.1484286
Jang, A.-Y., Ahn, K. B., Zhi, Y., Ji, H.-J., Zhang, J., Han, S. H., et al. (2019). Serotype-independent protection against invasive pneumococcal infections conferred by live vaccine with lgt deletion. Front. Immunol. 10:1212. doi: 10.3389/fimmu.2019.01212
Jensen, R. G., Johansen, H. K., Bjarnsholt, T., Eickhardt-Sørensen, S. R., and Homøe, P. (2017). Recurrent otorrhea in chronic suppurative otitis media: is biofilm the missing link? Eur. Arch. Otorhinolaryngol. 274, 2741–2747. doi: 10.1007/s00405-017-4586-8
Jiao, X., Hirano, T., Hou, Y., and Gu, X.-X. (2002). Specific immune responses and enhancement of murine pulmonary clearance of Moraxella catarrhalis by intranasal immunization with a detoxified lipooligosaccharide conjugate vaccine. Infect. Immun. 70, 5982–5989. doi: 10.1128/IAI.70.11.5982-5989.2002
Juhn, S. K., Jung, M.-K., Hoffman, M. D., Drew, B. R., Preciado, D. A., Sausen, N. J., et al. (2008). The role of inflammatory mediators in the pathogenesis of otitis media and sequelae. Clin. Exp. Otorhinolaryngol. 1, 117–138. doi: 10.3342/ceo.2008.1.3.117
Kaas, J. H., and Hackett, T. A. (2000). Subdivisions of auditory cortex and processing streams in primates. Proc. Natl. Acad. Sci. 97, 11793–11799. doi: 10.1073/pnas.97.22.11793
Kamtchoua, T., Bologa, M., Hopfer, R., Neveu, D., Hu, B., Sheng, X., et al. (2013). Safety and immunogenicity of the pneumococcal pneumolysin derivative PlyD1 in a single-antigen protein vaccine candidate in adults. Vaccine 31, 327–333. doi: 10.1016/j.vaccine.2012.11.005
Karls, A. C., and Perkins-Balding, D. (2013). “Antigenic Variation” in Brenner's encyclopedia of genetics. eds. S. Maloy and K. Hughes. 2nd ed (San Diego: Academic Press), 145–147.
Kaur, R., Casey, J. R., and Pichichero, M. E. (2016). Emerging Streptococcus pneumoniae strains colonizing the nasopharynx in children after 13-valent (PCV13) pneumococcal conjugate vaccination in comparison to the 7-valent (PCV7) era, 2006-2015. Pediatr. Infect. Dis. J. 35, 901–906. doi: 10.1097/INF.0000000000001206
Kaur, R., Czup, K., Casey, J. R., and Pichichero, M. E. (2014a). Correlation of nasopharyngeal cultures prior to and at onset of acute otitis media with middle ear fluid cultures. BMC Infect. Dis. 14:640. doi: 10.1186/s12879-014-0640-y
Kaur, R., Morris, M., and Pichichero, M. E. (2017). Epidemiology of acute otitis media in the postpneumococcal conjugate vaccine era. Pediatrics 140. doi: 10.1542/peds.2017-0181
Kaur, R., and Pichichero, M. (2022). Lipidation of Haemophilus influenzae antigens P6 and OMP26 improves immunogenicity and protection against nasopharyngeal colonization and ear infection. Infect. Immun. 90, e00678–e00621. doi: 10.1128/iai.00678-21
Kaur, R., Surendran, N., Ochs, M., and Pichichero, M. E. (2014b). Human antibodies to PhtD, PcpA, and ply reduce adherence to human lung epithelial cells and murine nasopharyngeal colonization by Streptococcus pneumoniae. Infect. Immun. 82, 5069–5075. doi: 10.1128/IAI.02124-14
Kawaguchiya, M., Urushibara, N., and Kobayashi, N. (2017). Multidrug resistance in non-PCV13 serotypes of Streptococcus pneumoniae in northern Japan, 2014. Microb. Drug Resist. 23, 206–214. doi: 10.1089/mdr.2016.0054
Keech, C. A., Morrison, R., Anderson, P., Tate, A., Flores, J., Goldblatt, D., et al. (2020). A phase 1 randomized, placebo-controlled, observer-blinded trial to evaluate the safety and immunogenicity of inactivated Streptococcus pneumoniae whole-cell vaccine in adults. Pediatr. Infect. Dis. J. 39, 345–351. doi: 10.1097/inf.0000000000002567
Kennedy, B. J., Novotny, L. A., Jurcisek, J. A., Lobet, Y., and Bakaletz, L. O. (2000). Passive transfer of antiserum specific for immunogens derived from a nontypeable Haemophilus influenzae adhesin and lipoprotein D prevents otitis media after heterologous challenge. Infect. Immun. 68, 2756–2765. doi: 10.1128/iai.68.5.2756-2765.2000
Kerschner, J. E., Hong, W., Taylor, S. R., Kerschner, J. A., Khampang, P., Wrege, K. C, et al. (2013). A novel model of spontaneous otitis media with effusion (OME) in the Oxgr1 knock-out mouse. International Journal of Pediatric Otorhinolaryngology 77, 79–84.
Khan, M. N., and Pichichero, M. E. (2012). Vaccine candidates PhtD and PhtE of Streptococcus pneumoniae are adhesins that elicit functional antibodies in humans. Vaccine 30, 2900–2907. doi: 10.1016/j.vaccine.2012.02.023
Khan, M. N., Ren, D., Kaur, R., Basha, S., Zagursky, R., and Pichichero, M. E. (2016). Developing a vaccine to prevent otitis media caused by nontypeable Haemophilus influenzae. Expert Rev. Vaccines 15, 863–878. doi: 10.1586/14760584.2016.1156539
Khattak, S. F., Sheikh, N. A., Aleem, A., Farooq, M., and Nadeem, K. (2017). Microbiological profile from middle ear and nasopharynx in patients suffering from chronic active mucosal otitis media. J. Ayub Med. Coll. Abbottabad 29, 610–613.
Kim, D. K., Park, H. E., Back, S. A., Park, H. R., Kim, S. W., Park, Y., et al. (2016). Otitis media with effusion in an allergic animal model: a functional and morphological study. Int. J. Pediatr. Otorhinolaryngol. 84, 6–11. doi: 10.1016/j.ijporl.2016.02.018
Kim, S.-J., Seon, S. H., Luong, T. T., Ghosh, P., Pyo, S., and Rhee, D.-K. (2019). Immunization with attenuated non-transformable pneumococcal pep27 and comD mutant provides serotype-independent protection against pneumococcal infection. Vaccine 37, 90–98. doi: 10.1016/j.vaccine.2018.11.027
Klein-Szanto, A., Terzaghi, M., Mirkin, L., Martin, D., and Shiba, M. (1982). Propagation of normal human epithelial cell populations using an in vivo culture system. Description and applications. Am. J. Pathol. 108, 231–239.
Kodama, S., Abe, N., Hirano, T., and Suzuki, M. (2011a). A single nasal dose of CCL20 chemokine induces dendritic cell recruitment and enhances nontypable Haemophilus influenzae-specific immune responses in the nasal mucosa. Acta Otolaryngol. 131, 989–996. doi: 10.3109/00016489.2011.576429
Kodama, S., Hirano, T., Noda, K., Umemoto, S., and Suzuki, M. (2011b). Nasal immunization with plasmid DNA encoding P6 protein and immunostimulatory complexes elicits nontypeable Haemophilus influenzae-specific long-term mucosal immune responses in the nasopharynx. Vaccine 29, 1881–1890. doi: 10.1016/j.vaccine.2010.12.129
Kodama, S., Suenaga, S., Hirano, T., Suzuki, M., and Mogi, G. (2000). Induction of specific immunoglobulin a and Th2 immune responses to P6 outer membrane protein of nontypeable Haemophilus influenzae in middle ear mucosa by intranasal immunization. Infect. Immun. 68, 2294–2300. doi: 10.1128/IAI.68.4.2294-2300.2000
Kodama, S., and Suzuki, M. (2011). “Nasal-associated lymphoid tissue immunity and vaccine development” in Recent advances in tonsils and mucosal barriers of the upper airways (Basel, Switzerland: Karger Publishers), 110–112.
Kong, K., and Coates, H. L. (2009). Natural history, definitions, risk factors and burden of otitis media. Med. J. Aust. 191, S39–S43. doi: 10.5694/j.1326-5377.2009.tb02925.x
Kong, I. G., Sato, A., Yuki, Y., Nochi, T., Takahashi, H., Sawada, S., et al. (2013). Nanogel-based PspA intranasal vaccine prevents invasive disease and nasal colonization by Streptococcus pneumoniae. Infect. Immun. 81, 1625–1634. doi: 10.1128/IAI.00240-13
Kozan, G., Aktan, B., Sakat, M. S., Kurt, S., Öner, F., and Kara, A. (2015). Effect of systemic clarithromycin and prednisolone on histamine-induced otitis media in guinea pigs. Acta Otolaryngol. 135, 978–984. doi: 10.3109/00016489.2015.1058526
Kraus, W., Ohyama, K., Snyder, D., and Beachey, E. (1989). Autoimmune sequence of streptococcal M protein shared with the intermediate filament protein, vimentin. J. Exp. Med. 169, 481–492. doi: 10.1084/jem.169.2.481
Kurabi, A., Lee, J., Wong, C., Pak, K., Hoffman, H. M., Ryan, A. F., et al. (2015). The inflammasome adaptor ASC contributes to multiple innate immune processes in the resolution of otitis media. Innate Immun. 21, 203–214. doi: 10.1177/1753425914526074
Kurono, Y. (2022). The mucosal immune system of the upper respiratory tract and recent progress in mucosal vaccines. Auris Nasus Larynx 49, 1–10. doi: 10.1016/j.anl.2021.07.003
Kyd, J. M., and Cripps, A. W. (1998). Potential of a novel protein, OMP26, from nontypeable Haemophilus influenzae to enhance pulmonary clearance in a rat model. Infect. Immun. 66, 2272–2278. doi: 10.1128/IAI.66.5.2272-2278.1998
Kyd, J. M., Cripps, A. W., Novotny, L. A., and Bakaletz, L. O. (2003). Efficacy of the 26-kilodalton outer membrane protein and two P5 fimbrin-derived immunogens to induce clearance of nontypeable Haemophilus influenzae from the rat middle ear and lungs as well as from the chinchilla middle ear and nasopharynx. Infect. Immun. 71, 4691–4699. doi: 10.1128/IAI.71.8.4691-4699.2003
LaFontaine, E. R., Snipes, L. E., Bullard, B., Brauer, A. L., Sethi, S., and Murphy, T. F. (2009). Identification of domains of the hag/MID surface protein recognized by systemic and mucosal antibodies in adults with chronic obstructive pulmonary disease following clearance of Moraxella catarrhalis. Clin. Vaccine Immunol. 16, 653–659. doi: 10.1128/CVI.00460-08
Larsson, C., Dirckx, J. J., Bagger-Sjöbäck, D., and von Unge, M. (2005). Pars flaccida displacement pattern in otitis media with effusion in the gerbil. Otol. Neurotol. 26, 337–343. doi: 10.1097/01.mao.0000169770.31292.75
Larsson, C., Dirckx, J. J., Decraemer, W. F., Bagger-Sjöbäck, D., and von Unge, M. (2003). Pars flaccida displacement pattern in purulent otitis media in the gerbil. Otol. Neurotol. 24, 358–364. doi: 10.1097/00129492-200305000-00002
Lavinsky, L., and Goycoolea, M. (1997). “In search of a teaching, training and experimental model for otological surgery” in Otitis media today: Proceedings of the third extraordinary symposium on recent advances in otitis media, June 1–5 (Copenhagen, Denmark: Kugler Publications), 341.
Lee, G. M., Kleinman, K., Pelton, S., Lipsitch, M., Huang, S. S., Lakoma, M., et al. (2017). Immunization, antibiotic use, and pneumococcal colonization over a 15-year period. Pediatrics 140. doi: 10.1542/peds.2017-0001
Leroux-Roels, G., Maes, C., De Boever, F., Traskine, M., Rüggeberg, J. U., and Borys, D. (2014). Safety, reactogenicity and immunogenicity of a novel pneumococcal protein-based vaccine in adults: a phase I/II randomized clinical study. Vaccine 32, 6838–6846. doi: 10.1016/j.vaccine.2014.02.052
Leroux-Roels, G., Van Damme, P., Haazen, W., Shakib, S., Caubet, M., Aris, E., et al. (2016). Phase I, randomized, observer-blind, placebo-controlled studies to evaluate the safety, reactogenicity and immunogenicity of an investigational non-typeable Haemophilus influenzae (NTHi) protein vaccine in adults. Vaccine 34, 3156–3163. doi: 10.1016/j.vaccine.2016.04.051
Li, L., Guo, X., Olszewski, E., Fan, Z., Ai, Y., Han, Y., et al. (2015). Expression of surfactant protein-a during LPS-induced otitis media with effusion in mice. Otolaryngol. Head Neck Surg. 153, 433–439. doi: 10.1177/0194599815587699
Li, T., Zeng, W., and Liu, R. (2021). Effect of Erdosteine on middle ear effusion in rats by mediating TLR4 signaling pathway. BioMed. Res. Int. 2021:9968907. doi: 10.1155/2021/9968907
Liao, J., Kochilas, L., Nowotschin, S., Arnold, J. S., Aggarwal, V. S., Epstein, J. A., et al. (2004). Full spectrum of malformations in velo-cardio-facial syndrome/DiGeorge syndrome mouse models by altering Tbx1 dosage. Hum. Mol. Genet. 13, 1577–1585. doi: 10.1093/hmg/ddh176
Lieberthal, A. S., Carroll, A. E., Chonmaitree, T., Ganiats, T. G., Hoberman, A., Jackson, M. A., et al. (2013). The diagnosis and management of acute otitis media. Pediatrics 131, e964–e999. doi: 10.1542/peds.2012-3488
Lipski, S. L., Akimana, C., Timpe, J. M., Wooten, R. M., and Lafontaine, E. R. (2007). The Moraxella catarrhalis autotransporter McaP is a conserved surface protein that mediates adherence to human epithelial cells through its N-terminal passenger domain. Infect. Immun. 75, 314–324. doi: 10.1128/IAI.01330-06
Littorin, N., Rünow, E., Ahl, J., Resman, F., and Riesbeck, K. (2021). Decreased prevalence of Moraxella catarrhalis in addition to Streptococcus pneumoniae in children with upper respiratory tract infection after introduction of conjugated pneumococcal vaccine: a retrospective cohort study. Clin. Microbiol. Infect. 27:630.e1. e636. doi: 10.1016/j.cmi.2020.04.033
Liu, D.-F., McMichael, J. C., and Baker, S. M. (2007). Moraxella catarrhalis outer membrane protein CD elicits antibodies that inhibit CD binding to human mucin and enhance pulmonary clearance of M. catarrhalis in a mouse model. Infect. Immun. 75, 2818–2825. doi: 10.1128/IAI.00074-07
Lo, S. W., Gladstone, R. A., Van Tonder, A. J., Lees, J. A., Du Plessis, M., Benisty, R., et al. (2019). Pneumococcal lineages associated with serotype replacement and antibiotic resistance in childhood invasive pneumococcal disease in the post-PCV13 era: an international whole-genome sequencing study. Lancet Infect. Dis. 19, 759–769. doi: 10.1016/S1473-3099(19)30297-X
Lucidi, D., Cantaffa, C., Nocini, R., Martone, A., Alicandri-Ciufelli, M., Marchioni, D., et al. (2022). Quality of life after surgical treatment for chronic otitis media: a systematic review of the literature. J. Personal. Med. 12:1959. doi: 10.3390/jpm12121959
Luke, N. R., Howlett, A. J., Shao, J., and Campagnari, A. A. (2004). Expression of type IV pili by Moraxella catarrhalis is essential for natural competence and is affected by iron limitation. Infect. Immun. 72, 6262–6270. doi: 10.1128/IAI.72.11.6262-6270.2004
Luke, N. R., Jurcisek, J. A., Bakaletz, L. O., and Campagnari, A. A. (2007). Contribution of Moraxella catarrhalis type IV pili to nasopharyngeal colonization and biofilm formation. Infect. Immun. 75, 5559–5564. doi: 10.1128/IAI.00946-07
Luke-Marshall, N. R., Sauberan, S. L., and Campagnari, A. A. (2011). Comparative analyses of the Moraxella catarrhalis type-IV pilus structural subunit PilA. Gene 477, 19–23. doi: 10.1016/j.gene.2011.01.010
Ma, L., Sedney, C., Su, Y., Dewan, K. K., Linz, B., and Harvill, E. T. (2022). Contribution of a novel pertussis toxin-like factor in mediating persistent otitis media. Frontiers in cellular and infection. Microbiology 12:795230. doi: 10.3389/fcimb.2022.795230
MacArthur, C. J., Hefeneider, S. H., Kempton, J. B., Parrish, S. K., McCoy, S. L., and Trune, D. R. (2006). Evaluation of the mouse model for acute otitis media. Hear. Res. 219, 12–23. doi: 10.1016/j.heares.2006.05.012
Magnuson, K., Hermansson, A., Melhus, Å., and Hellström, S. (1997). The tympanic membrane and middle ear mucosa during non-typeable Haemophilus influenzae and Haemophilus influenzae type b acute otitis media: a study in the rat. Acta Otolaryngol. 117, 396–405. doi: 10.3109/00016489709113412
Mann, B., Thornton, J., Heath, R., Wade, K. R., Tweten, R. K., Gao, G., et al. (2014). Broadly protective protein-based pneumococcal vaccine composed of pneumolysin toxoid–CbpA peptide recombinant fusion protein. J. Infect. Dis. 209, 1116–1125. doi: 10.1093/infdis/jit502
Manning, J., Dunne, E. M., Wang, N., Pedersen, J. S., Ogier, J. M., Burt, R. A., et al. (2019). Effect of a pneumococcal whole cell vaccine on influenza A-induced pneumococcal otitis media in infant mice. Vaccine 37, 3495–3504. doi: 10.1016/j.vaccine.2019.03.013
Marom, T., Nokso-Koivisto, J., and Chonmaitree, T. (2012). Viral–bacterial interactions in acute otitis media. Curr Allergy Asthma Rep 12, 551–558. doi: 10.1007/s11882-012-0303-2
Martin, N. L., Rawling, E. G., Wong, R. S., Rosok, M., and Hancock, R. E. (1993). Conservation of surface epitopes in Pseudomonas aeruginosa outer membrane porin protein OprF. FEMS Microbiol. Lett. 113, 261–266. doi: 10.1111/j.1574-6968.1993.tb06524.x
Masaki, M., Wright, C. G., Lee, D. H., and Meyerhoff, W. L. (1988). Effects of otic drops on chinchilla tympanic membrane. Arch. Otolaryngol. 114, 1007–1011.
Masignani, V., Pizza, M., and Moxon, E. R. (2019). The development of a vaccine against meningococcus B using reverse vaccinology. Front. Immunol. 10:751. doi: 10.3389/fimmu.2019.00751
Massa, H. M., Spann, K. M., and Cripps, A. W. (2021). Innate immunity in the middle ear mucosa. Front. Cell. Infect. Microbiol. 11:764772. doi: 10.3389/fcimb.2021.764772
Matsubara, A., Nishizawa, H., Kurose, A., Nakagawa, T., Takahata, J., and Sasaki, A. (2014). An experimental study of inner ear injury in an animal model of eosinophilic otitis media. Acta Otolaryngol. 134, 227–232. doi: 10.3109/00016489.2013.859395
McCullers, J. A., Karlström, Å., Iverson, A. R., Loeffler, J. M., and Fischetti, V. A. (2007). Novel strategy to prevent otitis media caused by colonizing Streptococcus pneumoniae. PLoS Pathog. 3:e28. doi: 10.1371/journal.ppat.0030028
McCullers, J. A., and Rehg, J. E. (2002). Lethal synergism between influenza virus and Streptococcus pneumoniae: characterization of a mouse model and the role of platelet-activating factor receptor. J. Infect. Dis. 186, 341–350. doi: 10.1086/341462
McDaniel, L. S., and Swiatlo, E. (2004). Pneumococcal disease: pathogenesis, treatment, and prevention. Infect. Dis. Clin. Pract. 12, 93–98. doi: 10.1097/01.idc.0000121024.62151.c9
McElligott, M., Vickers, I., Meehan, M., Cafferkey, M., Cunney, R., and Humphreys, H. (2015). Noninvasive pneumococcal clones associated with antimicrobial nonsusceptibility isolated from children in the era of conjugate vaccines. Antimicrob. Agents Chemother. 59, 5761–5767. doi: 10.1128/AAC.00990-15
Meek, R. B. III, Berrebi, A. S., McGrew, B. M., Spirou, G. A., Cuff, C. F., and Wetmore, S. J. (1999). Immunologic and histologic observations in Reo virus-induced otitis Media in the Mouse. Ann. Otol. Rhinol. Laryngol. 108, 31–38. doi: 10.1177/000348949910800105
Michel, L. V., Kaur, R., Gleghorn, M. L., Holmquist, M., Pryharski, K., Perdue, J., et al. (2022). Haemophilus influenzae protein D antibody suppression in a multi-component vaccine formulation. FEBS Open bio 12, 2191–2202. doi: 10.1002/2211-5463.13498
Mitchell, R., Pereira, K. D., and Lazar, R. H. (1997). Fat graft myringoplasty in children–a safe and successful day-stay procedure. J. Laryngol. Otol. 111, 106–108. doi: 10.1017/S002221510013659X
Mokrzan, E. M., Novotny, L. A., Brockman, K. L., and Bakaletz, L. O. (2018). Antibodies against the majority subunit (PilA) of the type IV pilus of Nontypeable Haemophilus influenzae disperse Moraxella catarrhalis from a dual-species biofilm. MBio 9:e02423–18. doi: 10.1128/mBio.02423-18
Mokrzan, E. M., Ward, M. O., and Bakaletz, L. O. (2016). Type IV pilus expression is upregulated in nontypeable Haemophilus influenzae biofilms formed at the temperature of the human nasopharynx. J. Bacteriol. 198, 2619–2630. doi: 10.1128/JB.01022-15
Morton, D. J., Hempel, R. J., Seale, T. W., Whitby, P. W., and Stull, T. L. (2012). A functional tonB gene is required for both virulence and competitive fitness in a chinchilla model of Haemophilus influenzae otitis media. BMC. Res. Notes 5, 1–7. doi: 10.1186/1756-0500-5-327
Murphy, T. F. (2005). Vaccine development for non-typeable Haemophilus influenzae and Moraxella catarrhalis: progress and challenges. Expert Rev. Vaccines 4, 843–853. doi: 10.1586/14760584.4.6.843
Murphy, T. F., Brauer, A. L., Aebi, C., and Sethi, S. (2005). Identification of surface antigens of Moraxella catarrhalis as targets of human serum antibody responses in chronic obstructive pulmonary disease. Infect. Immun. 73, 3471–3478. doi: 10.1128/IAI.73.6.3471-3478.2005
Murphy, T. F., Brauer, A. L., Johnson, A., Wilding, G. E., Koszelak-Rosenblum, M., and Malkowski, M. G. (2017). A cation-binding surface protein as a vaccine antigen to prevent Moraxella catarrhalis otitis media and infections in chronic obstructive pulmonary disease. Clin. Vaccine Immunol. 24. doi: 10.1128/cvi.00130-17
Murphy, T. F., Brauer, A. L., Pettigrew, M. M., LaFontaine, E. R., and Tettelin, H. (2019). Persistence of Moraxella catarrhalis in chronic obstructive pulmonary disease and regulation of the hag/MID adhesin. J. Infect. Dis. 219, 1448–1455. doi: 10.1093/infdis/jiy680
Murphy, T. F., Kirkham, C., DeNardin, E., and Sethi, S. (1999). Analysis of antigenic structure and human immune response to outer membrane protein CD of Moraxella catarrhalis. Infect. Immun. 67, 4578–4585. doi: 10.1128/IAI.67.9.4578-4585.1999
Murphy, T. F., Kirkham, C., Johnson, A., Brauer, A. L., Koszelak-Rosenblum, M., and Malkowski, M. G. (2016). Sulfate-binding protein, CysP, is a candidate vaccine antigen of Moraxella catarrhalis. Vaccine 34, 3855–3861. doi: 10.1016/j.vaccine.2016.05.045
Murphy, T. F., Kirkham, C., and Lesse, A. J. (1993). The major heat-modifiable outer membrane protein CD is highly conserved among strains of Branhamella catarrhalis. Mol. Microbiol. 10, 87–97. doi: 10.1111/j.1365-2958.1993.tb00906.x
Murphy, T. F., Kirkham, C., Liu, D.-F., and Sethi, S. (2003). Human immune response to outer membrane protein CD of Moraxella catarrhalis in adults with chronic obstructive pulmonary disease. Infect. Immun. 71, 1288–1294. doi: 10.1128/IAI.71.3.1288-1294.2003
Murrah, K. A., Pang, B., Richardson, S., Perez, A., Reimche, J., King, L., et al. (2015). Nonencapsulated Streptococcus pneumoniae causes otitis media during single-species infection and during polymicrobial infection with nontypeable Haemophilus influenzae. Pathogens Dis. 73:ftu011. doi: 10.1093/femspd/ftu011
Nagai, K., Domon, H., Maekawa, T., Hiyoshi, T., Tamura, H., Yonezawa, D., et al. (2019). Immunization with pneumococcal elongation factor Tu enhances serotype-independent protection against Streptococcus pneumoniae infection. Vaccine 37, 160–168. doi: 10.1016/j.vaccine.2018.11.015
Nakahashi-Ouchida, R., Mori, H., Yuki, Y., Umemoto, S., Hirano, T., Uchida, Y., et al. (2022). Induction of mucosal IgA-mediated protective immunity against Nontypeable Haemophilus influenzae infection by a cationic Nanogel-based P6 nasal vaccine. Front. Immunol. 13:819859. doi: 10.3389/fimmu.2022.819859
NCT02543892 (2018). A Study of a Vaccine for Pneumonia in Adults and Toddlers in Kenya. Available at: https://clinicaltrials.gov/ct2/show/NCT02543892 (Accessed November 2023).
NCT04087460 (2022). Phase 1a Clinical trial of a Pneumococcal Vaccine (PICTPV). Available at: https://clinicaltrials.gov/study/ NCT04087460?term= NCT04087460&rank=1 (Accessed December 2023).
Ngo, C. C., Massa, H. M., McMonagle, B. A., Perry, C. F., Nissen, M. D., Sloots, T. P., et al. (2022). Predominant bacterial and viral otopathogens identified within the respiratory tract and middle ear of urban Australian children experiencing otitis media are diversely distributed. Front. Cell. Infect. Microbiol. 12:14. doi: 10.3389/fcimb.2022.775535
Ngo, C. C., Massa, H. M., Thornton, R. B., and Cripps, A. W. (2016). Predominant bacteria detected from the middle ear fluid of children experiencing otitis media: a systematic review. PLoS One 11:e0150949. doi: 10.1371/journal.pone.0150949
Noda, K., Kodama, S., Umemoto, S., Nomi, N., Hirano, T., and Suzuki, M. (2011). Th17 cells contribute to nontypeable Haemophilus influenzae-specific protective immunity induced by nasal vaccination with P6 outer membrane protein and α-galactosylceramide. Microbiol. Immunol. 55, 574–581. doi: 10.1111/j.1348-0421.2011.00352.x
Novotny, L. A., and Bakaletz, L. O. (2020). Transcutaneous immunization with a nontypeable Haemophilus influenzae dual adhesin-directed immunogen induces durable and boostable immunity. Vaccine 38, 2378–2386. doi: 10.1016/j.vaccine.2020.01.052
Novotny, L. A., Clements, J. D., and Bakaletz, L. O. (2015a). Therapeutic transcutaneous immunization with a band-aid vaccine resolves experimental otitis media. Clin. Vaccine Immunol. 22, 867–874. doi: 10.1128/CVI.00090-15
Novotny, L. A., Clements, J. D., Goodman, S. D., and Bakaletz, L. O. (2017). Transcutaneous immunization with a band-aid prevents experimental otitis media in a polymicrobial model. Clin. Vaccine Immunol. 24, e00563–e00516. doi: 10.1128/CVI.00563-16
Novotny, L. A., Jurcisek, J. A., Ward, M. O. Jr., Jordan, Z. B., Goodman, S. D., and Bakaletz, L. O. (2015b). Antibodies against the majority subunit of type IV pili disperse nontypeable H aemophilus influenzae biofilms in a LuxS-dependent manner and confer therapeutic resolution of experimental otitis media. Mol. Microbiol. 96, 276–292. doi: 10.1111/mmi.12934
Novotny, L. A., Pichichero, M. E., Denoël, P. A., Neyt, C., Vanderschrick, S., Dequesne, G., et al. (2002). Detection and characterization of pediatric serum antibody to the OMP P5-homologous adhesin of nontypeable Haemophilus influenzae during acute otitis media. Vaccine 20, 3590–3597. doi: 10.1016/S0264-410X(02)00306-7
Ochs, M. M., Williams, K., Sheung, A., Lheritier, P., Visan, L., Rouleau, N., et al. (2016). A bivalent pneumococcal histidine triad protein D-choline-binding protein a vaccine elicits functional antibodies that passively protect mice from Streptococcus pneumoniae challenge. Hum. Vaccin. Immunother. 12, 2946–2952. doi: 10.1080/21645515.2016.1202389
Odutola, A., Ota, M. O. C., Antonio, M., Ogundare, E. O., Saidu, Y., Foster-Nyarko, E., et al. (2017). Efficacy of a novel, protein-based pneumococcal vaccine against nasopharyngeal carriage of Streptococcus pneumoniae in infants: a phase 2, randomized, controlled, observer-blind study. Vaccine 35, 2531–2542. doi: 10.1016/j.vaccine.2017.03.071
Odutola, A., Ota, M. O. C., Antonio, M., Ogundare, E. O., Saidu, Y., Owiafe, P. K., et al. (2019). Immunogenicity of pneumococcal conjugate vaccine formulations containing pneumococcal proteins, and immunogenicity and reactogenicity of co-administered routine vaccines - a phase II, randomised, observer-blind study in Gambian infants. Vaccine 37, 2586–2599. doi: 10.1016/j.vaccine.2019.03.033
Odutola, A., Ota, M. O., Ogundare, E. O., Antonio, M., Owiafe, P., Worwui, A., et al. (2016). Reactogenicity, safety and immunogenicity of a protein-based pneumococcal vaccine in Gambian children aged 2-4 years: a phase II randomized study. Hum. Vaccin. Immunother. 12, 393–402. doi: 10.1080/21645515.2015.1111496
Ogunniyi, A. D., Woodrow, M. C., Poolman, J. T., and Paton, J. C. (2001). Protection against Streptococcus pneumoniae elicited by immunization with pneumolysin and CbpA. Infect. Immun. 69, 5997–6003. doi: 10.1128/IAI.69.10.5997-6003.2001
Ohashi, Y., Nakai, Y., Esaki, Y., Ohno, Y., Sugiura, Y., and Okamoto, H. (1991). Experimental otitis media with effusion induced by lipopolysaccharide from Klebsiella pneumoniae mucociliary pathology of the Eustachian tube. Acta Otolaryngol. 111, 105–115. doi: 10.3109/00016489109134989
Otsuka, T., Kirkham, C., Brauer, A., Koszelak-Rosenblum, M., Malkowski, M. G., and Murphy, T. F. (2016). The vaccine candidate substrate binding protein SBP2 plays a key role in arginine uptake, which is required for growth of Moraxella catarrhalis. Infect. Immun. 84, 432–438. doi: 10.1128/iai.00799-15
Otsuka, T., Kirkham, C., Johnson, A., Jones, M. M., and Murphy, T. F. (2014). Substrate binding protein SBP2 of a putative ABC transporter as a novel vaccine antigen of Moraxella catarrhalis. Infect. Immun. 82, 3503–3512. doi: 10.1128/iai.01832-14
Parkinson, N., Hardisty-Hughes, R. E., Tateossian, H., Tsai, H.-T., Brooker, D., Morse, S., et al. (2006). Mutation at the Evi1 locus in Junbo mice causes susceptibility to otitis media. PLoS Genet. 2:e149. doi: 10.1371/journal.pgen.0020149
Parks, R., Huang, C., and Haddad, J. (1996). Middle ear catalase distribution in an animal model of otitis media. Eur. Arch. Otorhinolaryngol. 253, 445–449. doi: 10.1007/BF00179947
Pearson, M. M., Lafontaine, E. R., Wagner, N. J., St. Geme, J. W. III, and Hansen, E. J. (2002). A hag mutant of Moraxella catarrhalis strain O35E is deficient in hemagglutination, autoagglutination, and immunoglobulin D-binding activities. Infect. Immun. 70, 4523–4533. doi: 10.1128/IAI.70.8.4523-4533.2002
Peng, D., Hong, W., Choudhury, B. P., Carlson, R. W., and Gu, X.-X. (2005). Moraxella catarrhalis bacterium without endotoxin, a potential vaccine candidate. Infect. Immun. 73, 7569–7577. doi: 10.1128/IAI.73.11.7569-7577.2005
Pereira, D. R. R., Pereira, M. R., Pereira, M. B. R., Costa, S. S., Mott, M. P., and Cantarelli, V. (2023). Otopathogens in the middle ear and nasopharynx of children with recurrent acute otitis media. Int. J. Pediatr. Otorhinolaryngol. 169:111552. doi: 10.1016/j.ijporl.2023.111552
Perez, A. C., and Murphy, T. F. (2017). A Moraxella catarrhalis vaccine to protect against otitis media and exacerbations of COPD: an update on current progress and challenges. Hum. Vaccin. Immunother. 13, 2322–2331. doi: 10.1080/21645515.2017.1356951
Perez, A. C., and Murphy, T. F. (2019). Potential impact of a Moraxella catarrhalis vaccine in COPD. Vaccine 37, 5551–5558. doi: 10.1016/j.vaccine.2016.12.066
Perez, A. C., Pang, B., King, L. B., Tan, L., Murrah, K. A., Reimche, J. L., et al. (2014). Residence of Streptococcus pneumoniae and Moraxella catarrhalis within polymicrobial biofilm promotes antibiotic resistance and bacterial persistence in vivo. Pathog Dis 70, 280–288. doi: 10.1111/2049-632x.12129
Pettigrew, M. M., Gent, J. F., Pyles, R. B., Miller, A. L., Nokso-Koivisto, J., and Chonmaitree, T. (2011). Viral-bacterial interactions and risk of acute otitis media complicating upper respiratory tract infection. J. Clin. Microbiol. 49, 3750–3755. doi: 10.1128/JCM.01186-11
Pichichero, M. E. (2013). Protein carriers of conjugate vaccines: characteristics, development, and clinical trials. Hum. Vaccin. Immunother. 9, 2505–2523. doi: 10.4161/hv.26109
Pichichero, M. E. (2020). Immunologic dysfunction contributes to the otitis prone condition. J. Infect. 80, 614–622. doi: 10.1016/j.jinf.2020.03.017
Pichichero, M. E., Casey, J. R., Hoberman, A., and Schwartz, R. (2008). Pathogens causing recurrent and difficult-to-treat acute otitis media, 2003-2006. Clin. Pediatr. 47, 901–906. doi: 10.1177/0009922808319966
Pichichero, M. E., Kaur, R., Casey, J. R., Sabirov, A., Khan, M. N., and Almudevar, A. (2010). Antibody response to Haemophilus influenzae outer membrane protein D, P6, and OMP26 after nasopharyngeal colonization and acute otitis media in children. Vaccine 28, 7184–7192. doi: 10.1016/j.vaccine.2010.08.063
Piltcher, O. B., Swarts, J. D., Magnuson, K., Alper, C. M., Doyle, W. J., and Hebda, P. A. (2002). A rat model of otitis media with effusion caused by eustachian tube obstruction with and without Streptococcus pneumoniae infection: methods and disease course. Otolaryngol. Head Neck Surg. 126, 490–498. doi: 10.1067/mhn.2002.124935
Pinilla, M., Ramírez-Camacho, R., Jorge, E., Trinidad, A., and Vergara, J. (2001). Ventral approach to the rat middle ear for otologic research. Otolaryngol. Head Neck Surg. 124, 515–517. doi: 10.1067/mhn.2001.115370
Pizza, M., Bekkat-Berkani, R., and Rappuoli, R. (2020). Vaccines against meningococcal diseases. Microorganisms 8:1521. doi: 10.3390/microorganisms8101521
Plamondon, P., Luke, N. R., and Campagnari, A. A. (2007). Identification of a novel two-partner secretion locus in Moraxella catarrhalis. Infect. Immun. 75, 2929–2936. doi: 10.1128/IAI.00396-07
Prakash, R., Juyal, D., Negi, V., Pal, S., Adekhandi, S., Sharma, M., et al. (2013). Microbiology of chronic suppurative otitis media in a tertiary care setup of Uttarakhand state. Ind. N Am J Med Sci 5, 282–287. doi: 10.4103/1947-2714.110436
Prellner, K., Hermansson, A., White, P., Melhus, Å., and Briles, D. (1999). Immunization and protection in pneumococcal otitis media studied in a rat model. Microb. Drug Resist. 5, 73–82. doi: 10.1089/mdr.1999.5.73
Price, E. P., Sarovich, D. S., Nosworthy, E., Beissbarth, J., Marsh, R. L., Pickering, J., et al. (2015). Haemophilus influenzae: using comparative genomics to accurately identify a highly recombinogenic human pathogen. BMC Genomics 16:641. doi: 10.1186/s12864-015-1857-x
Prince, J. E., Brayton, C. F., Fossett, M. C., Durand, J. A., Kaplan, S. L., Smith, C. W., et al. (2001). The differential roles of LFA-1 and mac-1 in host defense against systemic infection with Streptococcus pneumoniae. J. Immunol. 166, 7362–7369. doi: 10.4049/jimmunol.166.12.7362
Prymula, R., Pazdiora, P., Traskine, M., Rüggeberg, J. U., and Borys, D. (2014). Safety and immunogenicity of an investigational vaccine containing two common pneumococcal proteins in toddlers: a phase II randomized clinical trial. Vaccine 32, 3025–3034. doi: 10.1016/j.vaccine.2014.03.066
Prymula, R., Szenborn, L., Silfverdal, S.-A., Wysocki, J., Albrecht, P., Traskine, M., et al. (2016). “Safety and reactogenicity of the booster dose of 2 investigational protein-based pneumococcal vaccine formulations in toddlers: a phase ii randomized trial” in Open forum infectious diseases, vol. 745 (Oxford, United Kingdom: Oxford University Press).
Purcell, A. W., McCluskey, J., and Rossjohn, J. (2007). More than one reason to rethink the use of peptides in vaccine design. Nat. Rev. Drug Discov. 6, 404–414. doi: 10.1038/nrd2224
Rappuoli, R. (1999). The vaccine containing recombinant pertussis toxin induces early and long-lasting protection. Biologicals 27, 99–102. doi: 10.1006/biol.1999.0189
Ren, D., Almudevar, A. L., Murphy, T. F., Lafontaine, E. R., Campagnari, A. A., Luke-Marshall, N., et al. (2019). Serum antibody response to Moraxella catarrhalis proteins in stringently defined otitis prone children. Vaccine 37, 4637–4645. doi: 10.1016/j.vaccine.2017.07.027
Ren, D., Almudevar, A. L., and Pichichero, M. E. (2015). Synchrony in serum antibody response to conserved proteins of Streptococcus pneumoniae in young children. Hum. Vaccin. Immunother. 11, 489–497. doi: 10.4161/21645515.2014.990861
Ren, D., Xie, H., Zhang, W., Hassan, F., Petralia, R. S., Yu, S., et al. (2011a). Intranasal immunization of the combined lipooligosaccharide conjugates protects mice from the challenges with three serotypes of Moraxella catarrhalis. PLoS One 6:e29553. doi: 10.1371/journal.pone.0029553
Ren, D., Yu, S., Gao, S., Peng, D., Petralia, R. S., Muszynski, A., et al. (2011b). Mutant lipooligosaccharide-based conjugate vaccine demonstrates a broad-spectrum effectiveness against Moraxella catarrhalis. Vaccine 29, 4210–4217. doi: 10.1016/j.vaccine.2011.03.102
Rijkers, G. T., Sanders, E. A. M., Breukels, M. A., and Zegers, B. J. M. (1998). Infant B cell responses to polysaccharide determinants. Vaccine 16, 1396–1400. doi: 10.1016/S0264-410X(98)00098-X
Ronander, E., Brant, M., Eriksson, E., Mörgelin, M., Hallgren, O., Westergren-Thorsson, G., et al. (2009). Nontypeable Haemophilus influenzae adhesin protein E: characterization and biological activity. J. Infect. Dis. 199, 522–531. doi: 10.1086/596211
Rosenfeld, R. M., Shin, J. J., Schwartz, S. R., Coggins, R., Gagnon, L., Hackell, J. M., et al. (2016). Clinical practice guideline: otitis media with effusion (update). Otolaryngol. Head Neck Surg. 154, S1–S41.
Rovers, M. M. (2008). The burden of otitis media. Vaccine 26, G2–G4. doi: 10.1016/j.vaccine.2008.11.005
Ruckdeschel, E. A., Brauer, A. L., Johnson, A., and Murphy, T. F. (2009). Characterization of proteins Msp22 and Msp75 as vaccine antigens of Moraxella catarrhalis. Vaccine 27, 7065–7072. doi: 10.1016/j.vaccine.2009.09.062
Ruckdeschel, E. A., Kirkham, C., Lesse, A. J., Hu, Z., and Murphy, T. F. (2008). Mining the Moraxella catarrhalis genome: identification of potential vaccine antigens expressed during human infection. Infect. Immun. 76, 1599–1607. doi: 10.1128/IAI.01253-07
Ryan, A. F., Ebmeyer, J., Furukawa, M., Pak, K., Melhus, A., Wasserman, S. I., et al. (2006). Mouse models of induced otitis media. Brain Res. 1091, 3–8. doi: 10.1016/j.brainres.2006.02.004
Sabirov, A., and Metzger, D. W. (2008). Mouse models for the study of mucosal vaccination against otitis media. Vaccine 26, 1501–1524. doi: 10.1016/j.vaccine.2008.01.029
Sarwar, J., Campagnari, A. A., Kirkham, C., and Murphy, T. F. (1992). Characterization of an antigenically conserved heat-modifiable major outer membrane protein of Branhamella catarrhalis. Infect. Immun. 60, 804–809. doi: 10.1128/iai.60.3.804-809.1992
Segade, F., Daly, K. A., Allred, D., Hicks, P. J., Cox, M., Brown, M., et al. (2006). Association of the FBXO11 gene with chronic otitis media with effusion and recurrent otitis media: the Minnesota COME/ROM family study. Arch. Otolaryngol. Head Neck Surg. 132, 729–733. doi: 10.1001/archotol.132.7.729
Shaffer, T. L., Balder, R., Buskirk, S. W., Hogan, R. J., and Lafontaine, E. R. (2013). Use of the Chinchilla model to evaluate the vaccinogenic potential of the Moraxella catarrhalis filamentous hemagglutinin-like proteins MhaB1 and MhaB2. PLoS One 8:e67881. doi: 10.1371/journal.pone.0067881
Shi, H., Santander, J., Brenneman, K. E., Wanda, S. Y., Wang, S., Senechal, P., et al. (2010). Live recombinant Salmonella Typhi vaccines constructed to investigate the role of rpoS in eliciting immunity to a heterologous antigen. PLoS One 5:e11142. doi: 10.1371/journal.pone.0011142
Shimada, J., Moon, S. K., Lee, H. Y., Takeshita, T., Pan, H., Woo, J. I., et al. (2008). Lysozyme M deficiency leads to an increased susceptibility to Streptococcus pneumoniae-induced otitis media. BMC Infect. Dis. 8:134. doi: 10.1186/1471-2334-8-134
Simon, F., Haggard, M., Rosenfeld, R., Jia, H., Peer, S., Calmels, M.-N., et al. (2018). International consensus (ICON) on management of otitis media with effusion in children. Eur. Ann. Otorhinolaryngol. Head Neck Dis. 135, S33–S39. doi: 10.1016/j.anorl.2017.11.009
Singh, B., Al-Jubair, T., Voraganti, C., Andersson, T., Mukherjee, O., Su, Y.-C., et al. (2015). Moraxella catarrhalis binds plasminogen to evade host innate immunity. Infect. Immun. 83, 3458–3469. doi: 10.1128/IAI.00310-15
Singh, B., Blom, A. M., Unal, C., Nilson, B., Mörgelin, M., and Riesbeck, K. (2010). Vitronectin binds to the head region of Moraxella catarrhalis ubiquitous surface protein A2 and confers complement-inhibitory activity. Mol. Microbiol. 75, 1426–1444. doi: 10.1111/j.1365-2958.2010.07066.x
Singh, S., Wilson, J. C., Cripps, A. W., Massa, H., Ozberk, V., Grice, I. D., et al. (2020). Immunological characterisation of truncated lipooligosaccharide-outer membrane protein based conjugate vaccine against Moraxella catarrhalis and nontypeable Haemophilus influenzae. Vaccine 38, 309–317. doi: 10.1016/j.vaccine.2019.10.014
Sings, H. L., De Wals, P., Gessner, B. D., Isturiz, R., Laferriere, C., McLaughlin, J. M., et al. (2019). Effectiveness of 13-valent pneumococcal conjugate vaccine against invasive disease caused by serotype 3 in children: a systematic review and Meta-analysis of observational studies. Clin. Infect. Dis. 68, 2135–2143. doi: 10.1093/cid/ciy920
Smidt, M., Bättig, P., Verhaegh, S. J., Niebisch, A., Hanner, M., Selak, S., et al. (2013). Comprehensive antigen screening identifies Moraxella catarrhalis proteins that induce protection in a mouse pulmonary clearance model. PLoS One 8:e64422. doi: 10.1371/journal.pone.0064422
Soltan, M. A., Elbassiouny, N., Gamal, H., Elkaeed, E. B., Eid, R. A., Eldeen, M. A., et al. (2021). In silico prediction of a multitope vaccine against Moraxella catarrhalis: reverse vaccinology and immunoinformatics. Vaccine 9:669. doi: 10.3390/vaccines9060669
Son, Y. L., Pak, K., Muradagha, N., Heo, K. W., Leichtle, A., and Kurabi, A. (2022). Resolution of otitis media in a humanized mouse model. Front. Genet. 13:958540. doi: 10.3389/fgene.2022.958540
Su, Y.-C., Hallström, B. M., Bernhard, S., Singh, B., and Riesbeck, K. (2013). Impact of sequence diversity in the Moraxella catarrhalis UspA2/UspA2H head domain on vitronectin binding and antigenic variation. Microbes Infect. 15, 375–387. doi: 10.1016/j.micinf.2013.02.004
Sun, J., Chen, J., Cheng, Z., Robbins, J. B., Battey, J. F., and Gu, X.-X. (2000). Biological activities of antibodies elicited by lipooligosaccharide based-conjugate vaccines of nontypeable Haemophilus influenzae in an otitis media model. Vaccine 18, 1264–1272. doi: 10.1016/S0264-410X(99)00381-3
Tamir, S. O., Bialasiewicz, S., Brennan-Jones, C. G., Der, C., Kariv, L., Macharia, I., et al. (2023). ISOM 2023 research panel 4-diagnostics and microbiology of otitis media. Int. J. Pediatr. Otorhinolaryngol. 174:111741. doi: 10.1016/j.ijporl.2023.111741
Tan, T. T., Christensen, J. J., Dziegiel, M. H., Forsgren, A., and Riesbeck, K. (2006). Comparison of the serological responses to Moraxella catarrhalis immunoglobulin D-binding outer membrane protein and the ubiquitous surface proteins A1 and A2. Infect. Immun. 74, 6377–6386. doi: 10.1128/IAI.00702-06
Thanawastien, A., Joyce, K. E., Cartee, R. T., Haines, L. A., Pelton, S. I., Tweten, R. K., et al. (2021). Preclinical in vitro and in vivo profile of a highly-attenuated, broadly efficacious pneumolysin genetic toxoid. Vaccine 39, 1652–1660. doi: 10.1016/j.vaccine.2020.04.064
Thofte, O., Kaur, R., Su, Y. C., Brant, M., Rudin, A., Hood, D., et al. (2019). Anti-EF-Tu IgG titers increase with age and may contribute to protection against the respiratory pathogen Haemophilus influenzae. Eur. J. Immunol. 49, 490–499. doi: 10.1002/eji.201847871
Thofte, O., Su, Y. C., Brant, M., Littorin, N., Duell, B. L., Alvarado, V., et al. (2018). EF-Tu from non-typeable Haemophilus influenzae is an immunogenic surface-exposed protein targeted by bactericidal antibodies. Front. Immunol. 9:2910. doi: 10.3389/fimmu.2018.02910
Tian, C., Harris, B. S., and Johnson, K. R. (2016). Ectopic mineralization and conductive hearing loss in Enpp1asj mutant mice, a new model for otitis media and tympanosclerosis. PLoS One 11:e0168159. doi: 10.1371/journal.pone.0168159
Timpe, J. M., Holm, M. M., Vanlerberg, S. L., Basrur, V., and Lafontaine, E. R. (2003). Identification of a Moraxella catarrhalis outer membrane protein exhibiting both adhesin and lipolytic activities. Infect. Immun. 71, 4341–4350. doi: 10.1128/IAI.71.8.4341-4350.2003
Toll, E. C., and Nunez, D. A. (2012). Diagnosis and treatment of acute otitis media. J. Laryngol. Otol. 126, 976–983. doi: 10.1017/S0022215112001326
Tong, S., Amand, C., Kieffer, A., and Kyaw, M. H. (2018). Trends in healthcare utilization and costs associated with acute otitis media in the United States during 2008–2014. BMC Health Serv. Res. 18, 1–10. doi: 10.1186/s12913-018-3139-1
Tu, L. N., Jeong, H.-Y., Kwon, H.-Y., Ogunniyi, A. D., Paton, J. C., Pyo, S.-N., et al. (2007). Modulation of adherence, invasion, and tumor necrosis factor alpha secretion during the early stages of infection by Streptococcus pneumoniae ClpL. Infect. Immun. 75, 2996–3005. doi: 10.1128/IAI.01716-06
Tyrer, H. E., Crompton, M., and Bhutta, M. F. (2013). What have we learned from murine models of otitis media? Curr Allergy Asthma Rep 13, 501–511. doi: 10.1007/s11882-013-0360-1
Unge, M. V., Decraemer, W. F., Buytaert, J. A., and Dirckx, J. J. (2009). Evaluation of a model for studies on sequelae after acute otitis media in the Mongolian gerbil. Acta Otolaryngol. 129, 261–267. doi: 10.1080/00016480802239091
Urbancikova, I., Prymula, R., Goldblatt, D., Roalfe, L., Prymulova, K., and Kosina, P. (2017). Immunogenicity and safety of a booster dose of the 13-valent pneumococcal conjugate vaccine in children primed with the 10-valent or 13-valent pneumococcal conjugate vaccine in the Czech Republic and Slovakia. Vaccine 35, 5186–5193. doi: 10.1016/j.vaccine.2017.07.103
Van Damme, P., Leroux-Roels, G., Vandermeulen, C., De Ryck, I., Tasciotti, A., Dozot, M., et al. (2019). Safety and immunogenicity of non-typeable Haemophilus influenzae-Moraxella catarrhalis vaccine. Vaccine 37, 3113–3122. doi: 10.1016/j.vaccine.2019.04.041
Van Eldere, J., Slack, M. P., Ladhani, S., and Cripps, A. W. (2014). Non-typeable Haemophilus influenzae, an under-recognised pathogen. Lancet Infect. Dis. 14, 1281–1292. doi: 10.1016/S1473-3099(14)70734-0
Verduin, C. M., Hol, C., Fleer, A., van Dijk, H., and van Belkum, A. (2002). Moraxella catarrhalis: from emerging to established pathogen. Clin. Microbiol. Rev. 15, 125–144. doi: 10.1128/CMR.15.1.125-144.2002
Verhoeven, D., Perry, S., and Pichichero, M. E. (2014). Contributions to protection from Streptococcus pneumoniae infection using the monovalent recombinant protein vaccine candidates PcpA, PhtD, and PlyD1 in an infant murine model during challenge. Clin. Vaccine Immunol. 21, 1037–1045. doi: 10.1128/CVI.00052-14
Vikhe, P. P., Purnell, T., Brown, S. D., and Hood, D. W. (2019). Cellular content plays a crucial role in non-typeable Haemophilus influenzae infection of preinflamed Junbo mouse middle ear. Cell. Microbiol. 21:e12960. doi: 10.1111/cmi.12960
Von Unge, M., Decraemer, W., Bagger-Sjöbäck, D., and Van den Berghe, D. (1997). Tympanic membrane changes in experimental purulent otitis media. Hear. Res. 106, 123–136. doi: 10.1016/S0378-5955(97)00008-7
Walter, E. B., and Smith, M. J. (2022). Prevention of pneumococcal infections in childhood: two decades of progress. Curr. Opin. Pediatr. 34, 140–146. doi: 10.1097/MOP.0000000000001112
Wang, D., Lu, J., Yu, J., Hou, H., Leenhouts, K., Van Roosmalen, M. L., et al. (2018). A novel PspA protein vaccine intranasal delivered by bacterium-like particles provides broad protection against pneumococcal pneumonia in mice. Immunol. Investig. 47, 403–415. doi: 10.1080/08820139.2018.1439505
Watanabe, N., DeMaria, T., Lewis, D., Mogi, G., and Lim, D. (1982). Experimental otitis media in chinchillas. II. Comparison of the middle ear immune responses to S pneumoniae types 3 and 23. Ann. Otol. Rhinol. Laryngol. 93, 9–16.
Watson, P. S., Novy, P. L., and Friedland, L. R. (2019). Potential benefits of using a multicomponent vaccine for prevention of serogroup B meningococcal disease. Int. J. Infect. Dis. 85, 22–27. doi: 10.1016/j.ijid.2019.05.019
Weinberger, D. M., Malley, R., and Lipsitch, M. (2011). Serotype replacement in disease after pneumococcal vaccination. Lancet 378, 1962–1973. doi: 10.1016/S0140-6736(10)62225-8
Westman, E., Melhus, Å., HellströM, S., and Hermansson, A. (1999). Moraxella catarrhalis-induced purulent otitis media in the rat middle earL. APMIS 107, 737–746. doi: 10.1111/j.1699-0463.1999.tb01468.x
Whitby, P. W., Morton, D. J., Mussa, H. J., Mirea, L., and Stull, T. L. (2020). A bacterial vaccine polypeptide protective against nontypable Haemophilus influenzae. Vaccine 38, 2960–2970. doi: 10.1016/j.vaccine.2020.02.054
Whitby, P. W., Seale, T. W., Morton, D. J., and Stull, T. L. (2015). Antisera against certain conserved surface-exposed peptides of nontypeable Haemophilus influenzae are protective. PLoS One 10:e0136867. doi: 10.1371/journal.pone.0136867
Wilkinson, T. M. A., Schembri, S., Brightling, C., Bakerly, N. D., Lewis, K., MacNee, W., et al. (2019). Non-typeable Haemophilus influenzae protein vaccine in adults with COPD: a phase 2 clinical trial. Vaccine 37, 6102–6111. doi: 10.1016/j.vaccine.2019.07.100
Winter, L. E., and Barenkamp, S. J. (2003). HumanAntibodies specific for the high-molecular-weight adhesion proteins ofNontypeable Haemophilus influenzae mediate OpsonophagocyticActivity. Infect. Immun. 71, 6884–6891. doi: 10.1128/IAI.71.12.6884-6891.2003
Winter, L. E., and Barenkamp, S. J. (2009). Antibodies specific for the Hia adhesion proteins of nontypeable Haemophilus influenzae mediate opsonophagocytic activity. Clin. Vaccine Immunol. 16, 1040–1046. doi: 10.1128/cvi.00090-09
Winter, L. E., and Barenkamp, S. J. (2014). Antibodies to the HMW1/HMW2 and Hia adhesins of nontypeable Haemophilus influenzae mediate broad-based opsonophagocytic killing of homologous and heterologous strains. Clin. Vaccine Immunol. 21, 613–621. doi: 10.1128/CVI.00772-13
Winter, L. E., and Barenkamp, S. J. (2017). Immunogenicity of nontypeable Haemophilus influenzae outer membrane vesicles and protective ability in the chinchilla model of otitis media. Clin. Vaccine Immunol. 24, e00138–e00117. doi: 10.1128/CVI.00138-17
Wizemann, T. M., Heinrichs, J. H., Adamou, J. E., Erwin, A. L., Kunsch, C., Choi, G. H., et al. (2001). Use of a whole genome approach to identify vaccine molecules affording protection against Streptococcus pneumoniae infection. Infect. Immun. 69, 1593–1598. doi: 10.1128/IAI.69.3.1593-1598.2001
Wolter, N. E., Harrison, R. V., and James, A. L. (2014). Separating the contributions of olivocochlear and middle ear muscle reflexes in modulation of distortion product otoacoustic emission levels. Audiol. Neurotol. 19, 41–48. doi: 10.1159/000356174
Xu, X., Meng, J., Wang, Y., Zheng, J., Wu, K., Zhang, X., et al. (2014). Serotype-independent protection against pneumococcal infections elicited by intranasal immunization with ethanol-killed pneumococcal strain, SPY1. Journal of Microbiology 52, 315–323.
Yadav, M. K., Chae, S. W., Go, Y. Y., Im, G. J., and Song, J. J. (2017). In vitro multi-species biofilms of methicillin-resistant Staphylococcus aureus and Pseudomonas aeruginosa and their host interaction during in vivo colonization of an otitis media rat model. Front. Cell. Infect. Microbiol. 7:125. doi: 10.3389/fcimb.2017.00125
Yamamoto-Fukuda, T., Hishikawa, Y., Shibata, Y., Kobayashi, T., Takahashi, H., and Koji, T. (2010). Pathogenesis of middle ear cholesteatoma: a new model of experimentally induced cholesteatoma in Mongolian gerbils. Am. J. Pathol. 176, 2602–2606. doi: 10.2353/ajpath.2010.091182
Yamamoto-Fukuda, T., Takahashi, H., and Koji, T. (2011). Animal models of middle ear cholesteatoma. J. Biomed. Biotechnol. 2011:394241. doi: 10.1155/2011/394241
Yang, M., Johnson, A., and Murphy, T. F. (2011). Characterization and evaluation of the Moraxella catarrhalis oligopeptide permease a as a mucosal vaccine antigen. Infect. Immun. 79, 846–857. doi: 10.1128/IAI.00314-10
Yang, A., Walker, N., Bronson, R., Kaghad, M., Oosterwegel, M., Bonnin, J., et al. (2000). p73-deficient mice have neurological, pheromonal and inflammatory defects but lack spontaneous tumours. Nature 404, 99–103. doi: 10.1038/35003607
Yassin, G. M., Amin, M. A., and Attia, A. S. (2016). Immunoinformatics identifies a Lactoferrin binding protein a peptide as a promising vaccine with a global protective prospective against Moraxella catarrhalis. J. Infect. Dis. 213, 1938–1945. doi: 10.1093/infdis/jiw062
Yeo, S.G. (2018). "acute otitis media," in Korean Society of Otorhinolaryngology-Head and Neck Surgery. 3rd Edn (Soul, Korea: Koonja), 363–383.
Ysebaert, C., Castado, C., Mortier, M.-C., Rioux, S., Feron, C., Di Paolo, E., et al. (2021). UspA2 is a cross-protective Moraxella catarrhalis vaccine antigen. Vaccine 39, 5641–5649. doi: 10.1016/j.vaccine.2021.08.002
Ysebaert, C., Denoël, P., Weynants, V., Bakaletz, L. O., Novotny, L. A., Godfroid, F., et al. (2019). A protein E-PilA fusion protein shows vaccine potential against Nontypeable Haemophilus influenzae in mice and chinchillas. Infect. Immun. 87, 10–1128. doi: 10.1128/iai.00345-19
Yu, R. H., Bonnah, R. A., Ainsworth, S., and Schryvers, A. B. (1999). Analysis of the immunological responses to transferrin and lactoferrin receptor proteins from Moraxella catarrhalis. Infect. Immun. 67, 3793–3799. doi: 10.1128/IAI.67.8.3793-3799.1999
Yu, C., Cui, X., Chen, F., Yang, J., Qian, X., and Gao, X. (2013). Effect of glucocorticoids on aquaporin-1 in guinea pigs with otitis media with effusion. Exp. Ther. Med. 5, 1589–1592. doi: 10.3892/etm.2013.1036
Yu, S., and Gu, X.-X. (2005). Synthesis and characterization of lipooligosaccharide-based conjugate vaccines for serotype B Moraxella catarrhalis. Infect. Immun. 73, 2790–2796. doi: 10.1128/IAI.73.5.2790-2796.2005
Yu, S., and Gu, X.-X. (2007). Biological and immunological characteristics of lipooligosaccharide-based conjugate vaccines for serotype C Moraxella catarrhalis. Infect. Immun. 75, 2974–2980. doi: 10.1128/IAI.01915-06
Zaleski, A., Scheffler, N. K., Densen, P., Lee, F. K., Campagnari, A. A., Gibson, B. W., et al. (2000). Lipooligosaccharide Pk (Galα1-4Galβ1-4Glc) epitope of Moraxella catarrhalis is a factor in resistance to bactericidal activity mediated by normal human serum. Infect. Immun. 68, 5261–5268. doi: 10.1128/IAI.68.9.5261-5268.2000
Zhang, J., Chen, S., Hou, Z., Cai, J., Dong, M., and Shi, X. (2015). Lipopolysaccharide-induced middle ear inflammation disrupts the cochlear intra-strial fluid-blood barrier through down-regulation of tight junction proteins. PLoS One 10:e0122572. doi: 10.1371/journal.pone.0122572
Zhang, N., Qian, T., Sun, S., Cao, W., Wang, Z., Liu, D., et al. (2022). IL-17 is a potential therapeutic target in a rodent model of otitis media with effusion. J. Inflamm. Res. 15, 635–648. doi: 10.2147/JIR.S338598
Zheng, Q. Y., Hardisty-Hughes, R., and Brown, S. D. (2006). Mouse models as a tool to unravel the genetic basis for human otitis media. Brain Res. 1091, 9–15. doi: 10.1016/j.brainres.2006.01.046
Keywords: otitis media, childhood disease, Streptococcus pneumoniae, Haemophilus influenzae, Moraxella catarrhalis, hearing loss, vaccine, animal models
Citation: Zahid A, Wilson JC, Grice ID and Peak IR (2024) Otitis media: recent advances in otitis media vaccine development and model systems. Front. Microbiol. 15:1345027. doi: 10.3389/fmicb.2024.1345027
Received: 27 November 2023; Accepted: 08 January 2024;
Published: 24 January 2024.
Edited by:
Axel Cloeckaert, Institute National de recherche pour l’agriculture, l’alimentation et l’environnement (INRAE), FranceReviewed by:
Kalyan K. Dewan, University of Georgia, United StatesCopyright © 2024 Zahid, Wilson, Grice and Peak. This is an open-access article distributed under the terms of the Creative Commons Attribution License (CC BY). The use, distribution or reproduction in other forums is permitted, provided the original author(s) and the copyright owner(s) are credited and that the original publication in this journal is cited, in accordance with accepted academic practice. No use, distribution or reproduction is permitted which does not comply with these terms.
*Correspondence: I. Darren Grice, ZC5ncmljZUBncmlmZml0aC5lZHUuYXU=; Ian R. Peak, aS5wZWFrQGdyaWZmaXRoLmVkdS5hdQ==
Disclaimer: All claims expressed in this article are solely those of the authors and do not necessarily represent those of their affiliated organizations, or those of the publisher, the editors and the reviewers. Any product that may be evaluated in this article or claim that may be made by its manufacturer is not guaranteed or endorsed by the publisher.
Research integrity at Frontiers
Learn more about the work of our research integrity team to safeguard the quality of each article we publish.