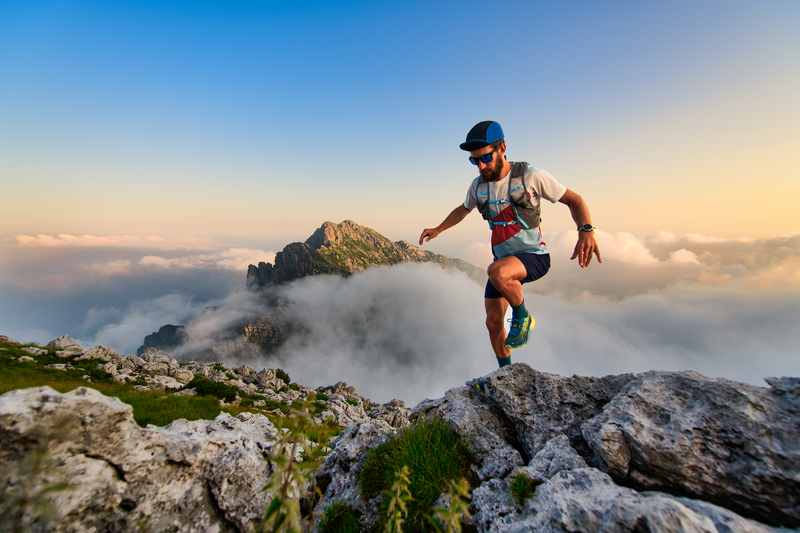
95% of researchers rate our articles as excellent or good
Learn more about the work of our research integrity team to safeguard the quality of each article we publish.
Find out more
ORIGINAL RESEARCH article
Front. Microbiol. , 24 January 2024
Sec. Antimicrobials, Resistance and Chemotherapy
Volume 15 - 2024 | https://doi.org/10.3389/fmicb.2024.1343988
Introduction: Fungal keratitis (FK) poses a severe threat to vision, potentially leading to blindness if not promptly addressed. Clitoria ternatea flower extracts have a history of use in Ayurvedic and Indian traditional medicines, particularly for treating eye ailments. This study investigates the antifungal and antibiofilm effects of Clitoria ternatea flower extracts on the FK clinical isolate Coniochaeta hoffmannii. Structural details and key compound identification were analysed through FTIR and GC-MS.
Methods: The minimum inhibitory concentration (MIC) and minimum fungicidal concentration (MFC) of Clitoria ternatea flower extracts were determined using broth dilution and well plate techniques. Biofilm inhibitory activity was assessed through microscopic evaluation, while anti-irritant and cytotoxic properties were evaluated using CAE-EI and MTT assays. Through GC-MS and FT-IR analysis the compounds dissolved in the extract and their functional group were studied, and their toxicity screening and pharmacokinetic prediction were conducted in silico. Subsequently, compounds with high corneal permeability were further identified, and molecular docking and simulation studies at 150 ns were used to investigate their interactions with fungal virulence factors and human inflammatory proteins.
Results and Discussion: At a concentration of 250 µg/mL, the Clitoria ternatea flower extract displayed effective biofilm inhibition. MIC and MFC values were determined as 500 and 1000 µg/mL, respectively. CAE-EI and MTT assays indicated no significant irritant and cytotoxic effects up to a concentration of 3 mg/mL. Compounds like 9,9-dimethoxybicyclo[3.3.1]nonane-2,4-dione showed high corneal permeability with strong and stable interactions with fungal virulence cellobiose dehydrogenase, endo β 1,4 xylanase, and glucanase, as well as corneal inflammation-associated human TNF-α and Interleukin IL-1b protein targets. The findings indicate that extracts from C. ternatea flowers could be formulated for an effective and safe alternative for developing new topical FK therapeutics.
Fungal keratitis (FK) is a severe eye disease that presents substantial risks and challenges, especially for outdoor workers, particularly those working in agricultural areas. Over the past three decades, the number of FK cases has shown a significant rise (Hoffman et al., 2021). Currently, FK accounts for 40%–50% of all cases of microbial keratitis. Alarmingly, it is projected that in less developed nations, more than 600,000 people could suffer from vision loss caused by FK, with outdoor workers being particularly vulnerable (Brown et al., 2022). While being immunocompromised, using contact lenses, experiencing ocular surface trauma (OCD), and suffering vegetative trauma are the primary risk factors for FK, it can also be caused by both filamentous fungi and yeast-like fungi (Ting et al., 2021). Our research team recently isolated a new pathogen, Coniochaeta hoffmannii, responsible for causing FK in a 71-year-old patient from a low-income agrarian background (Poomany Arul Soundara Rajan et al., 2023). Individuals who are economically disadvantaged and reside in rural areas with limited access to affordable antifungal medications face the highest risk of developing sight-threatening infections caused by FK (Koffi et al., 2021). The currently used topical therapy antifungals, including amphotericin B, fluconazole, itraconazole, natamycin, and voriconazole, exhibit limited effectiveness in treating FK due to their poor corneal penetration (Sanap et al., 2022). Additionally, the increasing emergence of antifungal antibiotic resistance among FK-causative fungi poses a significant challenge to ophthalmologists (Maharana et al., 2016). Recently several studies have revealed that antibiotics like natamycin and other azoles are becoming ineffective against different fungal pathogens. The exorbitant use of triazole antifungals in agriculture led to the development of azole resistance among several fungal strains. This is a major concern worldwide because even individuals who have never been exposed to these antifungals have been found to carry these resistant strains (Prajna et al., 2022). Considering the complex origin of fungal causatives of this disease, the increasing prevalence of antibiotic-resistant corneal infections, and their projected significant impact on impoverished agricultural communities, the exploration of new therapeutic alternatives and approaches to treating FK is imperative.
In ancient times, folk medicine was relied upon for its effectiveness and affordability in treating illnesses (Krupa et al., 2019). There has been an increased focus on researching bioactive organic therapies, leading to the discovery of over 200,000 medicinal compounds derived from plants. This research is a response to the rising antimicrobial resistance of microbes to conventional drugs (Guimarães et al., 2021). Traditional herbal remedies for eye conditions have been used by ethnic nomadic populations worldwide and extensively studied through ethnobiological research. Throughout history, people have relied on topical medicinal products derived from various plants to treat microbial keratitis. One such plant is Clitoria ternatea L., the Asian pigeonwings pea plant. This perennial climber from the Fabaceae family has been widely utilized in ancient medical systems like Ayurveda. It is valued for its numerous medical benefits, including its antidiabetic, analgesic, antipyretic, antioxidant, anticancer, and antimicrobial properties (Singh et al., 2018; Lakshan et al., 2019). C. ternatea flower and leaf extracts have effective antimicrobial and antibiofilm properties (Islam et al., 2023). The tribal and nomadic ethnic groups worldwide traditionally use the floral paste of this plant to treat several human ailments, including eye infections, headaches, boils, and skin ailments. Many examples of these traditional uses have been reported, including those by the Irulas of Kodiakkarai, India (Ragupathy and Newmaster, 2009) and several Indonesian communities (Afrianto et al., 2020). The Indian Ayurvedic medicinal system also uses powdered root water mixes for treating eye diseases, headaches, and dyspepsia, which are very common. Their ocular irritation-reducing capability is also widely recognized (Jamil et al., 2018). Their therapeutic effects are believed to be bestowed by the various secondary metabolites like alkaloids, flavonoids, glycosides, resin, saponins, steroids, tannins, and phenols they possess (Tripathi et al., 2023). Hence, the present study aims at assessing the FK therapeutic potential of C. ternatea flower extracts through in vitro and in silico experiments. The current research objective is to determine whether these extracts could be developed and incorporated into safe and effective FK-treating topical agents. Specifically, the study will evaluate the extracts’ ability to inhibit fungal growth, prevent biofilm formation, minimize irritation, penetrate the cornea, and assess their toxicity.
The study used the biofilm-forming clinical keratitis isolate C. hoffmannii (GenBankaccession number: MN453262.1), previously identified (Figure 1A) and isolated from a 70-year-old patient with an agrarian background by our team. The organism was used to evaluate the MIC, MFC, and biofilm inhibitory effects of floral extracts from C. ternatea. The growth colony morphology of this strain was examined on Congo red agar. To confirm its biofilm-forming nature, the isolate was streaked on Congo red agar supplemented with Congo red stain, sucrose, and BHI agar (HiMedia Laboratories, India). Afterwards, the inoculated plates were incubated at 27°C for 24–48 h by the method outlined (Khadija et al., 2019). The growth characteristics of the formed colony were observed and documented.
Figure 1. (A) Light microscopy gram-stained image (100×) of C. hoffmannii; (B) photo of C. ternatea blue sangupushpam flower.
The blue-colored flowers of the Asian pigeon wings (Figure 1B), locally known as Sangu Pushpam, were collected in and around Tirunelveli, Tamil Nadu, India, at GPS coordinates 8° 44′ 28.3992” N; 77° 41′ 40.6536″ E, between September and December 2022. The plant voucher specimen has been identified as C. ternatea L. using the website http://www.worldfloraonline.org. The initial identification was confirmed by the Southern Regional Office of the Botanical Survey of India in Coimbatore. The flowers were collected, dried in the shade, and ground into a coarse powder. The Soxhlet extraction method was used to extract the powdered flower using a sequential gradient of solvents that ranged from nonpolar to polar, including petroleum ether, ethyl acetate, acetone (SRL, Mumbai, India), and water in the ratio 1:10. The solvents were filtered through Whatman™ filter paper and condensed with a help of using a rotary evaporator (RV10 IKA®). The resulting solvent-free extracts were stored at 4°C for later use (Rajput et al., 2021).
Floral extracts from C. ternatea were evaluated for their antifungal activity on potato dextrose agar (PDA) plates following the well diffusion method. C. hoffmannii inoculum was cultured in a PDB medium for 48 h at 100 rpm and 27°C. The yeast cell suspension density was adjusted to 0.5 McFarland units using a spectrophotometer (Jasco V-770, Japan). The PDA plates were evenly coated with the prepared inoculum. The solvent-free plant extracts were mixed with DMSO (SD Fine Chemicals Ltd., Mumbai, India) at concentrations (µg/mL) of 1,000, 1,500, 2000, and 2,500. Agar plates were prepared with wells for adding the extracts. Fluconazole (HiMedia Laboratories, Mumbai, India) served as a positive control, whereas the negative control was DMSO. The inhibitory zone’s diameter was measured in millimetres after 48 h of incubation at 27°C (Parveen et al., 2018).
The MIC and MFC values of C. ternatea flower extracts were evaluated using broth dilution and plate methods against C. hoffmannii. Four extracts were obtained by diluting concentrations from 0.5 to 2.5 mg/mL in DMSO using two-fold serial dilutions. A culture mixture of 48-h-old C. hoffmannii culture at 1.5 × 108 CFU/mL mixed with 100 mL of PDB was prepared. The MIC was determined by adding different amounts of plant extracts (0.25–2.5 mg/mL) to various test tubes. The MIC is the minimum concentration of the extract required to inhibit the growth of the inoculum after a 48-h incubation period at 27°C. To examine MFC, 10 μL of culture from tubes showing no visible growth was added onto a PDA plate. The MFC was calculated as the lowest concentration of extract which allows no fungal growth during incubation on PDA agar plates (Sahal et al., 2020). The floral extract of C. ternatea with the highest inhibition rate at the lowest concentration was chosen for further investigation in subsequent tests.
A 96-well microtiter plate experiment was employed for the determination CFEA sample’s ability to suppress C. hoffmannii biofilm formation. C. hoffmannii culture (0.5 McFarland) was diluted with freshly sterilized PDB (1:100). The cell solution (180 μL) was mixed with plant samples (20 μL) of varying MIC concentrations (1/8, 1/4, 1/2, and 1) and dispensed into each well of 96-well plates. The controls are PDB medium with only culture and DMSO without plant extract. The plates were cleaned and stained for 15 min with 0.1% crystal violet after 48 h of incubation at 27°C. After that, they were washed again, and 95% ethanol was used to get rid of well’s leftover stains. Then, the culture was transferred to a new sterile plate, and the OD at 595 nm was measured with a UV–visible spectrometer (Ganesh Kumar et al., 2023). The biofilm inhibition percentage was calculated using the formula [(Control OD570 nm − Treated OD570 nm)/ Control OD570 nm] × 100.
The CFEA’s biofilm inhibitory activity was assessed using the coverslip method. A concentration of 1.5 × 108 CFU/mL of C. hoffmanii was prepared in 12-well microtiter plates using sterile PDP (1:100) as a diluent. Different amounts of CFEA (ranging from 1/8 MIC to 1/4 MIC, 1/2 MIC, and 1 MIC) were added to the wells alongside positive and negative controls. Sterile coverslips were placed vertically in the wells, and the plates were incubated for 48 h at 27°C. After incubation, the coverslip was removed, and any unattached planktonic cells were removed by rinsing with sterile PBS. The attached biofilm growth was then stained with 0.1% crystal violet. After removing the excess dye with sterile PBS and air drying the coverslip, the biofilm formation was observed using a light microscope under 100× magnifications, and the outcomes were recorded (Al-Ghanayem, 2022).
The DPPH method was utilized to evaluate the CFEA sample’s antioxidant capacity using ascorbic acid as the standard. Varied concentrations of the extract (200, 100, 50, 30, and 10 mg/mL) were mixed with DMSO and an equal volume of DPPH in ethanol (0.004%), and the mixture was kept in the dark for another 30 min. The extract’s free radical scavenging capabilities were assessed by monitoring the purple DPPH color change to yellow and measuring its OD at 517 nm. The IC50 value, which represents the amount of extract needed to eliminate 50% of the DPPH, was then calculated using the described method (Rajput et al., 2021).
The plant CFEA sample’s bioactive compound profiling was carried out using a Shimadzu QP2020 NX GC–MS. The instrument had a single quadrupole, a 190 L/s / 170 L/s (He) differential exhaust turbomolecular pump, a 1,035 kPa pressure range flow controller, and a flame ionization sensor. A 1 mg/mL plant sample was prepared and introduced into the instrument at a split ratio of 1:20 after a 3-min preheating phase at 50°C. The gas chromatograph separation was performed using an Rxi-5sil MS column. The column’s initial temperature was set at 280°C for 2 min, then gradually rose to 330°C at a rate of 12°C every 40 min. The carrier helium gas flow rate was set at 1 mL/min through the injection port maintained at 280°C. For mass spectrometry analysis, an ionization potential of 70 eV was used, along with electron ionization (EI) and transmission line temperatures of 260°C and 280°C, respectively. The sample was fully scanned with a cut-off time of 3 min, from 25 to 500 amu. The gas chromatograph ran for a total of 39 min, with the mass spectrometry analysis taking place between 5 and 40 min. Unidentified compounds within the extract were identified using the Shimadzu GC–MS solution™ Ver.4 software, which utilized the “NIST20R-library” (Syeda and Riazunnisa, 2020).
The major functional groups and structural features of the phytoactive chemicals in the plant sample were determined by FTIR spectral analysis. The infrared (IR) spectral details were acquired employing a Nicolet iS5 FT-IR spectrometer, scanning the mid-IR band 32 times at a resolution of 2 cm−1. The plant samples were placed in an IR chamber with iD3 ATR attachments before analysis. The resulting spectral details were collected, examined, and processed using Thermo Fischer Scientific OMNIC software, which compared the output spectrum with a reference spectrum from a library to identify the functional groups.
The mutagenic potential, carcinogenicity, and eye irritation ability of the phytocompounds were predicted using the STopTox web portal (Pokharkar et al., 2022). The Swiss-ADME program and ADMET lab 2.0 were used to predict the ADME pharmacokinetic properties. Additionally, the physicochemical properties that influence corneal permeability, such as molecular weight (MW), molecular volume (MV), hydrogen-bond donor (HBD), hydrogen-bond acceptor (HBA), total hydrogen bonds (HBtot), octanol–water partition coefficient (logP), and distribution coefficient logD7.0, were recorded using ADMET lab 2.0 software (Jha et al., 2022).
The following receptor proteins were selected based on their functional activity and association with human inflammation: Cellobiose dehydrogenase (Uniprot ID: A0A2I7VT52), Endo-1,4-β-xylanase (Uniprot ID: A0A2I7VT94), and Glucanase (Uniprot ID: A0A2I7VT76). Additionally, human TNF-α (PDB ID: 2az5) and Interleukin IL-1b (PDB ID: 1ITB) were also chosen. These protein structures were obtained in pdb format from the UniProt1 and RCBS2 databases. To enhance the accuracy of our analysis, unused ligands, co-factors, and water molecules were eliminated using Molegro Molecular Viewer software, and the structures were subsequently exported in PDB format. The proteins were prepared by eliminating co-factors and water molecules as well as adding of polar hydrogen atoms and Kollman charges. This extensive preparation ensures that the target proteins are properly designed and ready for further computational research.
The ligand structures of 9,9-dimethoxybicyclo[3.3.1]nonane-2,4-dione-; 2,5-O Methylene-D-mannitol; Glycerol 1,2 diacetate; D-Glucitol, 1,4-anhydro; and 1,2,3-Propanetriol were verified using the PubChem database.3 Their respective 3D structures were downloaded in Structure Data File (SDF) format. The ligands in sdf format were then converted to pdbqt format for further investigations using the OpenBabel GUI software.
The AutoDock 1.5.6, an automated protein-ligand docking tool, was used to predict the binding affinity between phytochemical ligands from plant samples and five protein receptors. Fluconazole (PubChem ID: 3365), a commonly available commercial antifungal drug used as a topical azole, was used as a positive control. The grid parameter file (GPF) with a grid box was built using the Lamarckian Genetic algorithm. The constructed grid box’s dimensions (spacing; npts (x, y, z); center (x, y, z)) for the selected receptors are as follows: A0A2I7VT52 (0.897, (18.415, −15.886, −3.835), (126,110,110)); A0A2I7VT94 (0.919, (−6.622,-18.687,-28.162), (90,92,112)); A0A2I7VT76 (0.731, (3.210, 5.88, −14.513), (110, 96, 112)); 2az5 (0.936, (13.680, 71.619, 27.007), (92, 76, 80)); 1ITB (0.936, (39.651, 4.651, 14.919), (126, 80, 48)). MD studies were conducted using the Lamarckian genetic algorithm (LMA) and an empirical free energy function as outlined by Forli et al. (2016). A population size of 300 and 50 iterations of the genetic algorithm were employed to create the docking parameter file (DPF) using AutoDock Tools (ADT). The complexes generated from the lowest-energy conformation in each run were organized into clusters (Sunny et al., 2022).
The binding free energy of the receptor, ligand and their complexes were calculated using the MM-GBSA module of the software (Schrödinger suite Schrödinger, New York, 2021). The OPLS4 force field and rotamer algorithm were employed to assess the relative energy of these complexes. The free-binding energy equation is expressed as follows:
A lower negative score indicates a stronger binding energy.
MDS were performed on the top-ranked receptor complexes, including cellobiose dehydrogenase, endo β 1,4 xylanase, glucanase, TNF-α, and Interleukin IL-1b, using the Schrödinger software’s Maestro platform Desmond tool. The binding scores from the MDS studies were examined for the top-ranked ligands, specifically 2,5-O-Methylene-D-mannitol and 9,9-dimethoxy bicyclo [3.3.1] nonane-2,4-dione. The receptors were preprocessed, hydrogen bond optimized, and OPLS4 force field minimized before MDS. A hydration model was created by solvating the receptor and ligand combination in a 3D orthorhombic box using the SPC water model. The MD simulations were run for 150 ns with 1,000 projections under the NPT ensemble, while maintaining constant temperature (T), pressure (P), and number of atoms (N). The model system was relaxed using standard Desmond settings before the simulation began. A simulation interactions diagram was used to analyze the complex’s stability, revealing RMSD (root mean square distance) data. Throughout the simulation period, protein–ligand connections were determined using hydrogen bonds, hydrophobic interactions, ionic interactions, and water bridges (Schrödinger: Desmond Molecular Dynamics System, NY, 2021) as described (Gunaseelan et al., 2022).
The CFEA phytochemical’s toxicity on SIRC (NCCS, Pune, India) cells was assessed using MTT colorimetric assay. DMEM media (Gibco, United States) with FBS (10%) and antibiotic solution (1%) supplementation was used growing the cells until reaching 1 × 105 cells/mL concentration in a 96-well culture plate. After incubation at 37°C for 24–48 h, the wells were rinsed with sterile PBS and treated with various amounts of plant samples in a serum-free DMEM medium. The plate was then incubated for 24 h at 37°C with 5% CO2. Following that, 10 μL of MTT (5 mg/mL) was added to each well and incubated until a purple precipitate appeared under an inverted microscope. The well plates were washed with 1X PBS after the spent culture and MTT were removed. The formed formazan crystals were dissolved by adding 100 μL of DMSO and shaking the plate for 5 min. Cell viability percentages were calculated by quantifying the MTT reduction to formazan crystal through measuring the OD values at 570 nm using the microplate reader (Thermo Fisher Scientific, United States) as described by Chainumnim et al. (2022).
The in vitro hen’s egg chorioallantoic membrane-embryo irritation (CAE-EI) experiment, as described by Rangel et al. (2020) was utilized to assess the CFEA sample’s potential for causing ocular irritation. Fertilized White Leghorn chicken eggs were obtained from the Veterinary College and Research Institute in Tirunelveli after being incubated for 9 days. The eggshells were sterilized with ethanol, and a rotary dentist saw blade was used to carefully remove them without harming the chorioallantoic membrane (CAM). To test for eye irritation, a solution containing 0.3 mL of the extracted sample in a 0.5% DMSO solution was applied to the CAM. The positive control used was 0.1 N NaOH, and the negative control was 0.9% NaCl. Following the ICCVAM methodology, the CAM reactions such as vascular lysis or coagulation were observed after 5 min. The resulting irritation scores, ranging from 0 to 21, were assigned to categorize irritants as none (0–0.09), weak (1–4.9), medium (5–9.9), and strong (10–21), and recorded for analysis (Luepke and Kemper, 1986).
The data from the experiments performed thrice were reported as mean with ± standard deviation. SPSS 22.0 was used to carry out a one-way ANOVA analysis and the p-values less than 0.05 were considered as statistically significant.
Ongoing research is being conducted on the potential therapeutic benefits of the blue flowers of C. ternatea, which have traditionally been used to treat eye ailments. The study found that C. ternatea flower extracts had significant antifungal activity against C. hoffmannii, a clinical isolate fungus. CFEA showed the strongest inhibitory effect at the lowest concentration. The water extract had no inhibitory effects, while petroleum ether and acetone extracts had reduced inhibitory effects. The average inhibition zone of the active extracts ranged from 9.83 ± 0.28 to 23 ± 1.0 mm. The CFEA extract showed the most potent antifungal activity, ranging from 11.50 ± 0.50 to 23 ± 1.0 mm (Figure 2A). The DMSO used as a negative control had no inhibitory effects. In contrast, the positive control, fluconazole, resulted in an inhibition zone of 23 ± 0.5 mm. The results demonstrate that C. hoffmanni is more susceptible to CFEA extract than other extracts, having MIC and MFC values of 0.5 and 1 mg mL−1, respectively.
Figure 2. The graph represents (A) antifungal activity of C. ternatea L. flower extracts against C. hoffmannii at varying concentrations. (B) Antibiofilm inhibition percentage of CFEA against C. hoffmannii. (C) CFEA antioxidant activity at various concentrations and its comparison with standard ascorbic acid.
Under an optical microscope, living cells stained with crystal violet were observed as part of the antibiofilm assay (Figure 3). The ability of CFEA to effectively inhibit biofilms was demonstrated by exposing C. hoffmannii to varying doses of the compound (Figure 2B). The intensity of biofilm formation decreased as the concentration of CFEA increased, indicating a dose-dependent relationship for inhibiting biofilm formation. The least effective MIC doses for inhibiting biofilm growth were 1/4 and 1/2.
Figure 3. Antibiofilm activity under light microscope in 100× magnification (A) control without plant extract (B) DMSO only (C) treated with 1/8 MIC of CFEA (D) treated with 1/4 MIC of CFEA (E) treated with 1/2 MIC of CFEA (F) treated with 1 MIC of CFEA.
At concentrations (mg/mL) of 10, 50, 100, 200, and 300, the antioxidant levels of CFEA were observed to increase by 72%, 78%, 83%, 91%, and 92%, respectively. These results closely matched those of the control group, with ascorbic acid indicating the strong antioxidant capacity of CFEA (Figure 2C). The IC50 of CFEA was found to be 1 mg, demonstrating its ability to reduce DPPH radical scavenging activity by 50% at this concentration. This study highlights the impressive antioxidant effects of CFEA and its effectiveness in eliminating DPPH radicals.
Upon comparing the CFEA extract to the “NIST20R library,” the GCMS spectral data analysis revealed the presence of 23 phytochemicals in different quantities. The GC–MS chromatogram (Figure 4) indicates 23 peaks in the CFEA. These include compounds such as Hexanoic acid, 2-ethyl-, anhydride (49.18%); n-Hexadecanoic acid (11.86%); 1,2,3-Propanetriol, 1-acetate (11.71%); Glycerol 1,2-diacetate (5.58%); Hexadecanoic acid, octyl ester (4.03%); Phthalic acid, diethyl ester (3.89%); 1-Hexacosene (3.70%); 9-Tricosene, (Z)- (1.26%); D-Glucitol, 1,4-anhydro- (1.04%); Phenol, 2,4-bis (1,1-dimethyl ethyl)- (0.99%); Sorbitol (0.87%); Benzofuran, 2,3-dihydro- (0.77%); 2,5-O-Methylene-D-mannitol (0.73%); Isopropyl hexacosyl ether (0.66%); Phthalic acid, bis(2-ethylhexyl) ester (0.65%); Hexadecanoic acid, 3-hydroxy-, methyl ester (0.57%); 5-Hydroxymethyl-2-furaldehyde (0.47%); Propanoic acid, 2-methyl-, nonyl ester (0.47%); 2-Methylhexacosane (0.45%); 1-Docosanol, methyl ether (0.44); Dotriacontyl isopropyl ether (0.43%); 9,9-dimethoxy bicyclo [3.3.1] nonane-2,4-dione- (0.27%) and Sulfurous acid, dodecyl 2-propyl ester (0.23%).
The CFEA FTIR spectrum revealed several functional groups of CFEA phytocompounds. Major absorption peaks were found at specific wave numbers (cm−1): 851, 1,047, 1,238, 1,371, 1,438, 1739, 2,987, and 3,328, which confirms the presence of significant chemical bonding groups. The peak at 1,047 cm−1 corresponds to the CO-O-CO bond stretch in the anhydride group, while the peak at 851 cm−1 represents the C=C alkene group bend. Peaks at 1,371 cm−1, 1,438 cm−1, and 3,328 cm−1 indicate the O-H groups of phenols, carboxylic acids, and alcohols, respectively. Additionally, peak values at 1,238 cm−1, 1,739 cm−1, and 2,987 cm−1 represent the C-O, C=O, and C-H stretches of alkyl aryl ethers, carbonyl esters, and alkane groups (Figure 5).
The corneal permeability and other pharmacological parameters of compounds are crucial for formulating topical ophthalmic medications. This data includes MW, MV, HBD, HBA, total hydrogen bonds (HBtot), Log P, and Log D. Some CFEA phytocompounds adhere to the Lipinski rule of drug-likeness, showing potential for conversion into orally bioavailable medications and topical treatments for FK. However, some CFEA compounds violate Lipinski’s rule, and some are predicted to have ocular irritation, carcinogenic, mutagenic, or mutagenic potential. However, some compounds of CFEA violate Lipinski’s rule. They include Sorbitol, 9-tricosene, (Z)-, 1-hexacosene, 2-methylhexacosane, Hexadecanoic acid, octyl ester, bis(2-ethylhexyl) phthalate, and Isopropyl hexacosyl ether, each with one violation and Dotriacontyl isopropyl ether with two violations. The compounds Glycerol 1,2-diacetate, D-Glucitol, 1,4-anhydro-, 1,2,3-Propanetriol, 1-acetate, 9,9-dimethoxybicyclo[3.3.1]nonane-2,4-dione-, and 2,5-O Methylene-D-mannitol are designated as corneal-permeable, druggable phytocompounds based on their properties. Table 1 provides each compound’s physiochemical and pharmacokinetic properties.
Table 1. GC–MS analysis of phytocompounds present in CFEA, their physiochemical, and pharmacokinetic properties.
The CFEA druggable phytocompounds, such as 9,9-dimethoxy bicyclo [3.3.1] nonane-2,4-dione; 2,5-O Methylene-D-mannitol; Glycerol 1,2-diacetate; D-Glucitol, 1,4-anhydro- and 1,2,3-Propanetriol, 1-acetate, showed significant affinity when docked with fungal enzymes Cellobiose dehydrogenase, Endo-1,4-β-xylanase, and Glucanase, with binding score ranging from −2.05 kcal/mol to −5.19 kcal/mol. Among these compounds, 9,9-dimethoxy bicyclo [3.3.1] nonane-2,4-dione- exhibited the highest binding values of −5.19 kcal/mol, −5.06 kcal/mol, and −4.7 kcal/mol with Cellobiose dehydrogenase, Endo β 1,4 xylanase, and glucanase, respectively (Figure 6). The amino acids involved in the hydrogen bond interaction of 9,9-dimethoxy bicyclo [3.3.1] nonane-2,4-dione- with the three amino acids were Gln735, Gly802, Arg158, Thr59, and Lys200. The inhibition constants for these interactions were 156.22 μM, 195.21 μM, and 356 μM, respectively. These druggable compounds also showed good in silico interaction with corneal inflammation-inducing proteins human TNF-α and Interleukin IL-1b, with binding energies from −5.41 kcal/mol to −8.56 kcal/mol. Among these compounds, 2,5-O-Methylene-D-mannitol and Bicyclo [3.3.1] nonane-2,4-dione, 9,9-dimethoxy- exhibited the lowest binding values with the targeted proteins. Table 2 shows the binding score of five ligands with fungal virulence and inflammatory receptorsprotein, as well as the distances between Van der Waal’s interaction bonds and the number of hydrogen bonds formed. These lowest binding scores show that the CFEA compounds are more efficient in interacting and suppressing the fungal virulence and inflammatory proteins, thereby reducing the fungal pathogenicity and corneal inflammation. The calculated binding free energy of the docked complexes as calculated by MM-GBSA suggests a strong ligand-receptor affinity. Specifically, the relative binding free energies of glucanase and cellobiose dehydrogenase with 9,9-dimethoxy bicyclo[3.3.1]nonane-2,4-dione are −8.41765 kcal/mol and − 38.2048 kcal/mol, respectively. Additionally, the binding free energy between Endo β 1,4 xylanase and 2,5-O-Methylene-D-mannitol is determined to be −39.7775 kcal/mol.
Figure 6. (A) 2D interactions between 2,5-O-Methylene-D-mannitol with human TNF-α, (B) 2D interactions between 9,9-dimethoxybicyclo[3.3.1]nonane-2,4-dione-, with human Interleukin IL-1b,2D interactions between 9,9-dimethoxybicyclo[3.3.1]nonane-2,4-dione-, (C) with Cellobiose dehydrogenase, (D) Endo β 1,4 xylanase, and (E) Glucanase.
Table 2. Results of molecular docking analysis showing docking scores, inhibition constant, and interacting amino acid residues.
A 150 ns MDS was performed on the top docked complexes to evaluate the stability of the simulated systems and analyze the hydrogen bonds, hydrophobic, ionic, and water bridge interactions. The results indicate strong structural stability and interactions within the docking complexes, as evidenced by the calculated RMSD, RMSF, and protein-ligand contact. Figures 7A,B illustrate the RMSD of Interleukin IL-1b with the 2,5-O-Methylene-D-mannitol complex and glucanase with the 9,9-Dimethoxybicyclo (3.3.1) nona-2,4-dione-complex. The RMSD values for the ligand and receptor fluctuated around 3 Å throughout the simulation, indicating the degree of stability of the complex. Strong and sustained contact between the ligand and protein is evident. According to Figure 7C, the residues Lys27, Leu29, Asn129, Arg9, Glu11, Ile13, Leu15, Arg25, Pro26, Ile90, Lys91, and Ser93 exhibit hydrogen bond, hydrophobic, and water bridge interactions. Additionally, Asn55, Trp56, Arg57, Trp58, Thr59, Asn67, Tyr100, His117, Tyr119, Thr121, Asn122, Lys200, and Asn219 are shown in Figure 7D to interact through hydrogen bond, hydrophobic, and water bridges, demonstrating the stability of the complex.
Figure 7. RMSD values during the simulation of complex of (A) 9,9-dimethoxybicyclo[3.3.1]nonane-2,4-dione-, with human Interleukin IL-1b, (B) 9,9-dimethoxybicyclo[3.3.1]nonane-2,4-dione-, with glucanase; protein ligand interaction histogram of (C) 9,9-dimethoxybicyclo[3.3.1]nonane-2,4-dione-, with human Interleukin IL-1b, (D) 9,9-dimethoxybicyclo[3.3.1]nonane-2,4-dione-,with glucanase.
The CAE-EI experiment did not show any significant changes when using a 3 mg concentration of plant extract dissolved in 0.5% DMSO as shown in Figure 8. For comparison, positive and negative controls of 0.1 N NaOH and 0.9% NaCl were used. The 0.1 N NaOH caused hemorrhaging at 0.5 min, vascular coagulation and lysis at 2 min, and worsening symptoms by 5 min. In contrast, neither 0.9% NaCl nor the CFEA showed noticeable changes. The irritation score (14.05) and severity score (3) contrasted with the value 0 of 0.9% NaCl revealed their strong irritating nature. The MTT test showed that solvent-free CFEA did not harm the metabolic activity of SIRC cells, indicating that the extracts are not toxic. A cytotoxicity test on SIRC cell lines showed that cell viability ranged from 90 to 55% at 0.5 to 5.0 mg/mL (Figure 9). According to current research, CFEA is a promising candidate for the development of a new topical antifungal agent because of its ability to inhibit biofilm-forming fungi, corneal permeability, pharmacodynamic properties, non-toxicity, and safety for corneal cells like SIRC cells.
The antioxidant, antifungal, and antibiofilm properties of CFEA, along with the abundance of identified bioactive phytocompounds and their high-affinity interaction with fungal virulence target enzymes and human inflammatory proteins, have led us to propose the following mechanism of action for CFEA:
Figure 10 depicts the CFEA phytocomponents’ proposed general mechanism, qualifying them as constituents in a topical treatment to be produced to combat FK. When rich CFEA phytocompounds come into contact with the surface of the fungal cell wall, their interaction may cause a variety of damaging events at the cell wall, resulting in cell integrity breakdown and the release of internal components. They also inhibit biosynthesis as well as the development of new cell walls and biofilm. Furthermore, their in-silico druggable corneal permeability and other pharmacological features, as well as their high-affinity association with pathogenic and anti-inflammatory-linked proteins, may help to minimize post-infection inflammation in the eye.
Figure 10. Proposed anti-keratitis mechanism of CFEA; credits to BioRender.com.
This study could significantly impact developing nations with agroeconomic sectors affected by FK. According to Niu et al. (2020), over a million new cases are documented annually. The study suggests that C. ternatea flower extract could be beneficial in inhibiting the growth and formation of biofilms by the FK-causing C. hoffmannii fungus, which are crucial components for corneal infection. The current work highlights how CFEA extract could be applied for the hard-to-manage FK biofilm formation and its control, which is important since biofilms once formed become extremely resistant to antibiotics (Roscetto et al., 2020). Overall, the study strongly recommends the natural remedies application for FK treatment and prevention. The outcomes of the current study corroborate with earlier studies which revealed that traditional eye disease remedies were shown to possess strong antifungal, antibiofilm, and antioxidant properties. According to Jeyaraj et al. (2022b), C. ternatea’s flower extract exhibits potent antibacterial and antifungal properties, even against microorganisms resistant to drugs. Jeyaraj et al. (2021) found that the methanolic extract of C. ternatea flowers exhibited MIC and MFC values of 0.8 and 1.6 mg/mL, respectively, against Penicillium and Rhizopus species. More potent antifungal activities against Candida, Fusarium, and Aspergillus species were found in methanolic and ethanolic extracts of C. ternatea seeds than in non-polar solvent extracts, according to research by Mushtaq et al. (2021). With MIC and MFC values of 0.5 and 1.0 mg, the CFEA extract exhibited the highest inhibition against C. hoffmannii.
The GCMS analysis revealed that the CFEA contains numerous phytochemicals with beneficial biological effects. Some of these substances have been identified as having antimicrobial, antioxidant, and anti-inflammatory properties, including hexanoic acid, 2-ethyl anhydride, n-Hexadecanoic acid (Thakur et al., 2018), glycerol 1,2-diacetate, Hexadecanoic acid (El-Sayed et al., 2023), 1,2,3-Propanetriol, 1-acetate, benzofuran, 2,3-dihydro- (Rani et al., 2023), 3-hydroxy-methyl ester (Kumar et al., 2021), isopropyl hexacosyl ether, and 5-Hydroxymethyl-2-furaldehyde (Fitriaturosidah et al., 2022). Additionally, phenol, 2,4-bis (1,1-dimethyl ethyl)-, has been found to have antifungal and biofilm-rupturing capabilities (Sharaf, 2020), while D-Glucitol, 1,4-anhydro-, has antiophidic effects (Singh et al., 2018). Among the 23 compounds discovered through GC–MS analysis, potentially druggable molecules having corneally permeable properties, which could be used to develop topical FK treatments, were screened, and their interaction with fungal target interaction is studied with in silico analysis.
According to Karami et al. (2022), a drug designed for corneal application should be a small, lipophilic molecule with minimal hydrogen bonds, in line with the composition of the corneal layers.
In our study, the compounds such as 9,9-dimethoxybicyclo[3.3.1]nonane-2,4-dione-; 2,5-O Methylene-D-mannitol; Glycerol 1,2 diacetate; D-Glucitol, 1,4-anhydro; and 1,2,3-Propanetriol exhibited favorable characteristics like good corneal permeability, low molecular weight, and lipophilic qualities. According to Khanzada et al. (2021), there is a significant association between the antifungal phytochemicals of a plant and the target elements of fungal virulence. These authors emphasized the importance of identifying molecular targets and understanding their mode of antifungal activity, whether it involves competitive or allosteric inhibition, to develop innovative antifungal therapy strategies.
Niu et al. (2020) found that the fungus in FK initially attaches itself to the corneal cell wall, leading to inflammation and redness in the cornea. Arana et al. (2009) also noted that the extracellular elements of the fungal cell wall play a crucial role in infiltrating host cells. Specifically, the hydrolytic enzymes in the fungal cell wall contribute to the deterioration of the corneal layers and the development of pathogenicity (Challacombe et al., 2019). Molecular docking receptors such as cellobiose dehydrogenase, Endo β 1,4 xylanase, and glucanase found in C. hoffmanii’s cell wall are involved in this process. Additionally, Endo β 1,4 xylanase has been recognized for its aggressive properties towards other fungi such as Botrytis cinerea and Sclerotinia sclerotiorum (Yu et al., 2016). Most antifungal therapeutics function by damaging the fungal cell wall, causing the contents to leak out and leading to fungal death (Hasim and Coleman, 2019). Several phytochemicals from C. ternatea, such as 9,9-dimethoxybicyclo[3.3.1]nonane-2,4-dione and 2,5-O Methylene-D-mannitol, have been found to have high binding affinity, similar to fluconazole. These compounds also exhibit strong receptor and ligand binding with the lowest negative MMGBSA score. Rolta et al. (2022) reported the highest binding score interaction between fungal pathogens and 9,9-Dimethoxybicyclo[3.3.1]nona-2,4-dione. According to Ololade Zacchaeus et al. (2021), these chemicals isolated from black velvet Tamarind seed extract have therapeutic benefits, including treating eye conditions. Additionally, the 9,9-Dimethoxybicyclo[3.3.1]nona-2,4-dione molecule is non-irritating to the eyes and has anti-inflammatory qualities (Reza et al., 2023). The stability of the complex is demonstrated by the MD simulation at 150 ns. In Figure 7A, the receptor and ligand complex reached equilibrium between 30 and 125 nanoseconds, showcasing its robust and stable nature. Similarly, in Figure 7B, the complex reached equilibrium between 40 and 95 ns. The slight variation in the RMSD was attributed to the flexibility of the ligand. Despite this volatility, the strong binding between the receptor and ligand indicates that the complex is stable.
This study also emphasized the importance of the anti-inflammatory properties of the CFEA, thereby reducing corneal inflammation. The high-affinity interactions between C. ternatea bioactive phytochemicals and human corneal inflammation-associated protein targets indicate their post-infection corneal inflammatory ailment-reducing potential. Recently number of studies (Iamsaard et al., 2014; GOH et al., 2022; Jeyaraj et al., 2022a) revealed the C. ternatea floral extract’s potentanti-inflammation, anti-proliferation, and oxidative stress reduction like protective functions. Their different biologically active compounds may be credited with these beneficial activities. In line with the above (Srichaikul, 2018) also demonstrated the absence of adverse reactions in rats receiving 2000 mg/kg C. ternatea flower ethanol extract. Rollando et al. (2023) also confirmed the CFEA safety as a pharmaceutical component with a high selectivity index value. The current study also shows that even at 3 mg/mL exposure, the extract did not harm SIRC cells since more than 70% of cells are viable. Hence, the CFEA is classified as a non-irritating one following the definition of Stanciauskaite et al. (2021). The CAE-EI assay used in this investigation also provided additional evidence of the extract’s anti-irritant properties.
The study highlights the potential of CFEA in combating biofilm-mediated fungal infections and inflammatory diseases. It suggests that the phytocomponents of CFEA offer safe and effective synthetic alternatives for FK-like eye disorders. CFEA contains numerous phytocompounds with important multipotent qualities such as antioxidant, antifungal, and antibiofilm properties. The study also identifies low-toxic, non-irritating, corneal permeable phytochemicals in CFEA that interact well with human inflammatory proteins and fungus-induced enzymes. As a result, the development of topical FK medicines has significantly progressed, and the traditional use of C. ternatea flower extract as a natural antifungal agent has been confirmed. Further research into the phytocompounds of CFEA may lead to safe and efficient FK treatments that can prevent biofilm formation.
The original contributions presented in the study are included in the article/supplementary material, further inquiries can be directed to the corresponding author.
PY: Writing – original draft, Writing – review & editing. PJ: Methodology, Writing – review & editing. AJ: Visualization, Writing – review & editing. AS: Formal analysis, Writing – review & editing. PK: Review & editing. GP: Analysis and interpretation of results. AS: Review & writing. KM: Administration, Supervision, Writing & reviewing.
The author(s) declare financial support was received for the research, authorship, and/or publication of this article. The authors extended their appreciation to the Researchers Supporting Project number (RSPD2024R675), King Saud University, Riyadh, Saudi Arabia.
The authors also thank Arunkumar Malaisamy, International Centre for Genetic Engineering and Biotechnology (ICGEB), New Delhi, for helping us out in the computational studies.
The authors declare that the research was conducted in the absence of any commercial or financial relationships that could be construed as a potential conflict of interest.
The author(s) declared that they were an editorial board member of Frontiers, at the time of submission. This had no impact on the peer review process and the final decision.
All claims expressed in this article are solely those of the authors and do not necessarily represent those of their affiliated organizations, or those of the publisher, the editors and the reviewers. Any product that may be evaluated in this article, or claim that may be made by its manufacturer, is not guaranteed or endorsed by the publisher.
The Supplementary material for this article can be found online at: https://www.frontiersin.org/articles/10.3389/fmicb.2024.1343988/full#supplementary-material
CFEA, Clitoria ternatea flower ethyl acetate extract; DMSO, Dimethyl sulfoxide; DPPH, 1,1-diphenyl-2-picrylhydrazyl; FK, Fungal keratitis; FTIR, Fourier transform infrared; CAE-EI, Chorioallantoic egg eye irritation Test; MFC, Minimum Fungicidal Concentration; MIC, Minimum Inhibitory Concentration; MTT, 3-[4,5-dimethylthiazol-2-yl]-2,5 diphenyl tetrazolium bromide; OD, Optical density; PDA, Potato dextrose Agar; PDB, Potato Dextrose Broth; SIRC, Staten’s Serum Institute Rabbit Cornea
Afrianto, W. F., Tamnge, F., and Hasanah, L. N. (2020). Review: a relation between ethnobotany and bioprospecting of edible flower butterfly pea (Clitoria ternatea) in Indonesia. Asian J. Ethnobiol. 3, 51–61. doi: 10.13057/asianjethnobiol/y030202
Al-Ghanayem, A. A. (2022). Phytochemical analysis of Cymbopogon flexuosus (lemongrass) oil, its antifungal activity, and role in inhibiting biofilm formation in Candida albicans MTCC854. Journal of King Saud University - Science 34:102072. doi: 10.1016/j.jksus.2022.102072
Arana, D. M., Prieto, D., Román, E., Nombela, C., and Alonso‐Monge, R., and Pla, J . (2009). The role of the cell wall in fungal pathogenesis. Microbial Biotechnology 2, 308–320. doi: 10.1111/j.1751-7915.2008.00070.x
Brown, L., Kamwiziku, G., Oladele, R. O., Burton, M. J., Prajna, N. V., Leitman, T. M., et al. (2022). The case for fungal keratitis to be accepted as a neglected tropical disease. J. Fungi 8:1047. doi: 10.3390/jof8101047
Chainumnim, S., Suksamrarn, S., Jarintanan, F., Jongrungruangchok, S., Wannaiampikul, S., and Tanechpongtamb, W. (2022). Sonicated extract from the aril of Momordica Cochinchinensis inhibits cell proliferation and migration in aggressive prostate cancer cells. J. Toxicol. 2022, 1–12. doi: 10.1155/2022/1149856
Challacombe, J. F., Hesse, C. N., Bramer, L. M., McCue, L. A., Lipton, M., Purvine, S., et al. (2019). Genomes and secretomes of Ascomycota fungi reveal diverse functions in plant biomass decomposition and pathogenesis. BMC Genomics 20:976. doi: 10.1186/s12864-019-6358-x
El-Sayed, H., Hamada, M. A., Elhenawy, A. A., Sonbol, H., and Abdelsalam, A. (2023). Acetylcholine Esterase Inhibitory Effect, Antimicrobial, Antioxidant, Metabolomic Profiling, and an In Silico Study of Non-Polar Extract of The Halotolerant Marine Fungus Penicillium chrysogenum MZ945518. Microorganisms 11:769. doi: 10.3390/microorganisms11030769
Fitriaturosidah, I., Kusnadi, J., Nurnasari, E., and Hariyono, B. (2022). Phytochemical screening and chemical compound of green roselle (Hibiscus sabdariffa L.) and potential antibacterial activities. IOP Conf. Ser. Earth Environ. Sci. 974:012118. doi: 10.1088/1755-1315/974/1/012118
Forli, S., Huey, R., Pique, M. E., Sanner, M. F., Goodsell, D. S., and Olson, A. J. (2016). Computational protein–ligand docking and virtual drug screening with the AutoDock suite. Nature Protocols 11, 905–919. doi: 10.1038/nprot.2016.051
Ganesh Kumar, A., Pugazhenthi, E., Sankarganesh, P., Muthusamy, C., Rajasekaran, M., Lokesh, E., et al. (2023). Cleome rutidosperma leaf extract mediated biosynthesis of silver nanoparticles and anti-candidal, anti-biofilm, anti-cancer, and molecular docking analysis. Biomass Convers. Biorefin. 1–13. doi: 10.1007/s13399-023-03806-9
Goh, S. E., Kwong, P. J., Ng, C. L., Ng, W. J., and Ee, K. Y. (2022). Antioxidant-rich Clitoria ternatea L. flower and its benefits in improving murine reproductive performance. Food Sci. Technol. 42:e25921. doi: 10.1590/fst.25921
Guimarães, R., Milho, C., Liberal, Â., Silva, J., Fonseca, C., Barbosa, A., et al. (2021). Antibiofilm potential of medicinal plants against Candida spp. Oral biofilms: a review. Antibiotics 10:1142. doi: 10.3390/antibiotics10091142
Gunaseelan, S., Arunkumar, M., Aravind, M. K., Gayathri, S., Rajkeerthana, S., Mohankumar, V., et al. (2022). Probing marine brown macroalgal phlorotannins as antiviral candidate against SARS-CoV-2: molecular docking and dynamics simulation approach. Molecular Diversity 26, 3205–3224. doi: 10.1007/s11030-022-10383-y
Hasim, S., and Coleman, J. J. (2019). Targeting the fungal cell wall: current therapies and implications for development of alternative antifungal agents. Future Medicinal Chemistry 11, 869–883. doi: 10.4155/fmc-2018-0465
Hoffman, J. J., Burton, M. J., and Leck, A. (2021). Mycotic keratitis—A global threat from the filamentous fungi. J. Fungi 7:273. doi: 10.3390/jof7040273
Iamsaard, S., Burawat, J., Kanla, P., Arun, S., Sukhorum, W., Sripanidkulchai, B., et al. (2014). Antioxidant activity and protective effect of Clitoria ternatea flower extract on testicular damage induced by ketoconazole in rats*. J. Zhejiang Univ. Sci. B 15, 548–555. doi: 10.1631/jzus.B1300299
Islam, M. A., Mondal, S. K., Islam, S., Shorna, A., Most, N., Biswas, S., et al. (2023). Antioxidant, cytotoxicity, antimicrobial activity, and in silico analysis of the methanolic leaf and flower extracts of Clitoria ternatea. Biochem. Res. Int. 2023, 1–12. doi: 10.1155/2023/8847876
Jamil, N., Mohd Zairi, M. N., Mohd Nasim, N. A., and Pa’ee, F. (2018). Influences of environmental conditions to phytoconstituents in Clitoria ternatea (butterfly pea flower) – a review. J. Sci. Technol. 10, 208–228. doi: 10.30880/jst.2018.10.02.029
Jeyaraj, E. J., Lim, Y. Y., and Choo, W. S. (2021). Extraction methods of butterfly pea (Clitoria ternatea) flower and biological activities of its phytochemicals. J. Food Sci. Technol. 58, 2054–2067. doi: 10.1007/s13197-020-04745-3
Jeyaraj, E. J., Lim, Y. Y., and Choo, W. S. (2022a). Antioxidant, cytotoxic, and antibacterial activities of Clitoria ternatea flower extracts and anthocyanin-rich fraction. Sci. Rep. 12:14890. doi: 10.1038/s41598-022-19146-z
Jeyaraj, E. J., Nathan, S., Lim, Y. Y., and Choo, W. S. (2022b). Antibiofilm properties of Clitoria ternatea flower anthocyanin-rich fraction towards Pseudomonas aeruginosa. Access Microbiol. 4. doi: 10.1099/acmi.0.000343
Jha, V., Devkar, S., Gharat, K., Kasbe, S., Matharoo, D. K., Pendse, S., et al. (2022). Screening of phytochemicals as potential inhibitors of breast cancer using structure based multitargeted molecular docking analysis. Phytomed. Plus 2:100227. doi: 10.1016/j.phyplu.2022.100227
Karami, T. K., Hailu, S., Feng, S., Graham, R., and Gukasyan, H. J. (2022). Eyes on Lipinski’s Rule of Five: A New “Rule of Thumb” for Physicochemical Design Space of Ophthalmic Drugs. Journal of Ocular Pharmacology and Therapeutics 38, 43–55. doi: 10.1089/jop.2021.0069
Khadija, B., Abbasi, A., Khan, S., Nadeem, M., Badshah, L., and Faryal, R. (2019). Isolation of pathogenic Candida species from oral cavity of postpartum females, and its association with obstetric and dental problems. Microb. Pathog. 131, 40–46. doi: 10.1016/j.micpath.2019.03.022
Khanzada, B., Akhtar, N., Okla, M. K., Alamri, S. A., Al-Hashimi, A., Baig, M. W., et al. (2021). Profiling of antifungal activities and in silico studies of natural polyphenols from some plants. Molecules 26:7164. doi: 10.3390/molecules26237164
Koffi, D., Bonouman, I. V., Toure, A. O., Kouadjo, F., N’Gou, M. R. E., Sylla, K., et al. (2021). Estimates of serious fungal infection burden in Côte d’Ivoire and country health profile. J. Med. Mycol. 31:101086. doi: 10.1016/j.mycmed.2020.101086
Krupa, J., Sureshkumar, J., Silambarasan, R., Priyadarshini, K., and Ayyanar, M. (2019). Integration of traditional herbal medicines among the indigenous communities in Thiruvarur District of Tamil Nadu, India. J. Ayurveda Integr. Med. 10, 32–37. doi: 10.1016/j.jaim.2017.07.013
Kumar, S. R., Chozhan, K., Murugesh, K. A., Rajeswari, R., and Kumaran, K. (2021). Gas chromatography-mass spectrometry analysis of bioactive compounds in chloroform extract of Psoralea corylifolia L. J. Appl. Nat. Sci. 13, 1225–1230. doi: 10.31018/jans.v13i4.2884
Lakshan, S. A. T., Jayanath, N. Y., Mendis Abeysekera, W. P. K., and Abeysekera, W. K. S. M. (2019). A commercial potential blue pea (Clitoria ternatea L.) flower extract incorporated beverage having functional properties. Evid. Based Complement. Alternat. Med. 2019, 1–13. doi: 10.1155/2019/2916914
Luepke, N. P., and Kemper, F. H. (1986). The HET-CAM test: an alternative to the draize eye test. Food Chem. Toxicol. 24, 495–496. doi: 10.1016/0278-6915(86)90099-2
Maharana, P., Sharma, N., Nagpal, R., Jhanji, V., Das, S., and Vajpayee, R. (2016). Recent advances in diagnosis and management of mycotic keratitis. Indian J. Ophthalmol. 64, 346–357. doi: 10.4103/0301-4738.185592
Mushtaq, Z., Khan, U., Seher, N., Shahid, M., Shahzad, M. T., Bhatti, A. A., et al. (2021). Evaluation of antimicrobial, antioxidant and enzyme inhibition roles of polar and non-polar extracts of Clitoria ternatea seeds. J. Anim. Plant Sci. 31, 1405–1418. doi: 10.36899/JAPS.2021.5
Niu, L., Liu, X., Ma, Z., Yin, Y., Sun, L., Yang, L., et al. (2020). Fungal keratitis: pathogenesis, diagnosis and prevention. Microb. Pathog. 138:103802. doi: 10.1016/j.micpath.2019.103802
Ololade Zacchaeus, S., Anuoluwa Iyadunni, A., Adejuyitan Johnson, A., and Uyaboerigha Daubotei, I. (2021). Black Velvet Tamarind: Phytochemical Analysis, Antiradical and Antimicrobial Properties of the Seed Extract for Human Therapeutic and Health Benefits. J. Phytopharm 10, 249–255.
Parveen, S., Wani, A. H., Shah, M. A., Devi, H. S., Bhat, M. Y., and Koka, J. A. (2018). Preparation, characterization and antifungal activity of iron oxide nanoparticles. Microb. Pathog. 115, 287–292. doi: 10.1016/j.micpath.2017.12.068
Pokharkar, O., Lakshmanan, H., Zyryanov, G., and Tsurkan, M. (2022). In silico evaluation of antifungal compounds from marine sponges against COVID-19-associated mucormycosis. Marine Drugs 20:215. doi: 10.3390/md20030215
Poomany Arul Soundara Rajan, Y. A., Anitha, V., Meenakshi, R., and Kasi, M. (2023). Therapeutic contact lens-related infection by a novel pathogenic fungus Coniochaeta hoffmannii-a case report. Indian J. Med. Microbiol. 44:100361. doi: 10.1016/j.ijmmb.2023.02.004
Prajna, N. V., Lalitha, P., Krishnan, T., Rajaraman, R., Radnakrishnan, N., Srinivasan, M., et al. (2022). Patterns of antifungal resistance in adult patients with fungal keratitis in South India. JAMA Ophthalmol. 140:179. doi: 10.1001/jamaophthalmol.2021.5765
Ragupathy, S., and Newmaster, S. G. (2009). Valorizing the “Irulas” traditional knowledge of medicinal plants in the Kodiakkarai reserve Forest, India. J. Ethnobiol. Ethnomed. 5:10. doi: 10.1186/1746-4269-5-10
Rajput, M., Bithel, N., and Vijayakumar, S. (2021). Antimicrobial, antibiofilm, antioxidant, anticancer, and phytochemical composition of the seed extract of Pongamia pinnata. Arch. Microbiol. 203, 4005–4024. doi: 10.1007/s00203-021-02365-9
Rangel, K. C., Villela, L. Z., de Castro Pereira, K., Colepicolo, P., Debonsi, H. M., and Gaspar, L. R. (2020). Assessment of the photoprotective potential and toxicity of Antarctic red macroalgae extracts from Curdiea racovitzae and Iridaea cordata for cosmetic use. Algal Research 50:101984. doi: 10.1016/j.algal.2020.101984
Rani, J., Kapoor, M., Dhull, S. B., Goksen, G., and Jurić, S. (2023). Identification and Assessment of Therapeutic Phytoconstituents of Catharanthus roseus through GC-MS Analysis. Separations 10:340. doi: 10.3390/separations10060340
Reza, A. S. M. A., Sakib, M. A., Nasrin, Mst. S, Khan, J., Khan, M. F., Hossen, Md. A., et al. (2023). Lasia spinosa (L.) thw. attenuates chemically induced behavioral disorders in experimental and computational models. Heliyon 9:e16754. doi: 10.1016/j.heliyon.2023.e16754
Rollando, R., Anggita Amelia, M., Hilmi Afthoni, M., and Rega Prilianti, K. (2023). Potential cytotoxic activity of methanol extract, ethyl acetate, and n-hexane fraction from Clitoria ternatea L. on MCF-7 breast cancer cell line and molecular docking study to P53. J. Pure Appl. Chem. Res. 12, 7–14. doi: 10.21776/ub.jpacr.2023.012.01.705
Rolta, R., Shukla, S., Kashyap, A., Kumar, V., Sourirajan, A., and Dev, K. (2022). Phytocompounds of Bistorta macrophylla (D. Don) Sojak. as bioavailability enhancers of fluconazole and amphotericin B to better manage Candida species infections. doi: 10.21203/rs.3.rs-1216369/v1
Roscetto, E., Masi, M., Esposito, M., Di Lecce, R., Delicato, A., Maddau, L., et al. (2020). Anti-biofilm activity of the fungal Phytotoxin Sphaeropsidin A against clinical isolates of antibiotic-resistant bacteria. Toxins 12:444. doi: 10.3390/toxins12070444
Sahal, G., Woerdenbag, H. J., Hinrichs, W. L. J., Visser, A., Tepper, P. G., Quax, W. J., et al. (2020). Antifungal and biofilm inhibitory effect of Cymbopogon citratus (lemongrass) essential oil on biofilm forming by Candida tropicalis isolates; an in vitro study. J. Ethnopharmacol. 246:112188. doi: 10.1016/j.jep.2019.112188
Sanap, S. N., Kedar, A., Bisen, A. C., Agrawal, S., and Bhatta, R. S. (2022). A recent update on therapeutic potential of vesicular system against fungal keratitis. J. Drug Deliv. Sci. Technol. 75:103721. doi: 10.1016/j.jddst.2022.103721
Sharaf, M. (2020). Evaluation of the antivirulence activity of ethyl acetate extract of Deverra tortuosa (Desf) against Candida albicans. Egypt. Pharm. J. 19:188. doi: 10.4103/epj.epj_10_20
Singh, N. K., Garabadu, D., Sharma, P., Shrivastava, S. K., and Mishra, P. (2018). Anti-allergy and anti-tussive activity of Clitoria ternatea L. in experimental animals. J. Ethnopharmacol. 224, 15–26. doi: 10.1016/j.jep.2018.05.026
Srichaikul, B. (2018). Ultrasonication extraction, bioactivity, antioxidant activity, total flavonoid, total phenolic and antioxidant of Clitoria Ternatea Linn flower extract for anti-aging drinks. Pharmacogn. Mag. 14:322. doi: 10.4103/pm.pm_206_17
Stanciauskaite, M., Marksa, M., Ivanauskas, L., Perminaite, K., and Ramanauskiene, K. (2021). Ophthalmic in situ gels with balsam poplar buds extract: formulation, rheological characterization, and quality evaluation. Pharmaceutics 13:953. doi: 10.3390/pharmaceutics13070953
Sunny, N. E., Kaviya, A., Saravanan, P., Rajeshkannan, R., Rajasimman, M., and Kumar, S. V. (2022). In vitro and in silico molecular docking analysis of green synthesized tin oxide nanoparticles using brown algae species of Padina gymnospora and Turbinaria ornata. Biomass Convers. Biorefin. 1–12. doi: 10.1007/s13399-022-03253-y
Syeda, A. M., and Riazunnisa, K. (2020). Data on GC-MS analysis, in vitro anti-oxidant and anti-microbial activity of the Catharanthus roseus and Moringa oleifera leaf extracts. Data Brief 29:105258. doi: 10.1016/j.dib.2020.105258
Thakur, A. V., Ambwani, S., Ambwani, T. K., Ahmad, A. H., and Rawat, D. S. (2018). Evaluation of phytochemicals in the leaf extract of Clitoria ternatea Willd. through GC-MS analysis. Tropical Plant Research, 5, 200–206. doi: 10.22271/tpr.2018.v5.i2.025
Ting, D. S. J., Ho, C. S., Deshmukh, R., Said, D. G., and Dua, H. S. (2021). Infectious keratitis: an update on epidemiology, causative microorganisms, risk factors, and antimicrobial resistance. Eye 35, 1084–1101. doi: 10.1038/s41433-020-01339-3
Tripathi, S., Annapureddy, S. R., and Sahoo, S. (2023). Intra-specific pharmacognostic biochemical screening of various populations of Clitoria ternatea L. using liquid chromatography/tandem mass spectrometry product ion scanning (LC-MS2 PIS). Ind. Crop. Prod. 203:117063. doi: 10.1016/j.indcrop.2023.117063
Keywords: Fungal keratitis, C. ternatea, phytocompounds, pharmacokinetics, topical drug
Citation: Yolin Angel PASR, Jeyakumar P, Jasmin Suriya AR, Sheena A, Karuppiah P, Periyasami G, Stalin A and Murugan K (2024) Topical antifungal keratitis therapeutic potential of Clitoria ternatea Linn. flower extract: phytochemical profiling, in silico modelling, and in vitro biological activity assessment. Front. Microbiol. 15:1343988. doi: 10.3389/fmicb.2024.1343988
Received: 24 November 2023; Accepted: 04 January 2024;
Published: 24 January 2024.
Edited by:
Hazem Salaheldin Elshafie, University of Basilicata, ItalyReviewed by:
Mohamed Sharaf, Al-Azhar University, EgyptCopyright © 2024 Yolin Angel, Jeyakumar, Jasmin Suriya, Sheena, Karuppiah, Periyasami, Stalin and Murugan. This is an open-access article distributed under the terms of the Creative Commons Attribution License (CC BY). The use, distribution or reproduction in other forums is permitted, provided the original author(s) and the copyright owner(s) are credited and that the original publication in this journal is cited, in accordance with accepted academic practice. No use, distribution or reproduction is permitted which does not comply with these terms.
*Correspondence: Kasi Murugan, bXVydXRhbkBnbWFpbC5jb20=;bXVydWdhbkBtc3VuaXYuYWMuaW4=
Disclaimer: All claims expressed in this article are solely those of the authors and do not necessarily represent those of their affiliated organizations, or those of the publisher, the editors and the reviewers. Any product that may be evaluated in this article or claim that may be made by its manufacturer is not guaranteed or endorsed by the publisher.
Research integrity at Frontiers
Learn more about the work of our research integrity team to safeguard the quality of each article we publish.