- 1The Francis Crick Institute, London, United Kingdom
- 2Pioneer Research Laboratories, San Francisco, CA, United States
- 3Pivot Bio, Berkeley, CA, United States
- 4Department of Ecology and Evolutionary Biology, University of Colorado, Boulder, CO, United States
- 5University of Florida, Gainesville, FL, United States
- 6London Institute of Medical Sciences, London, United Kingdom
- 7UK Centre for Astrobiology, University of Edinburgh, Edinburgh, United Kingdom
Nature exhibits an enormous diversity of organisms that thrive in extreme environments. From snow algae that reproduce at sub-zero temperatures to radiotrophic fungi that thrive in nuclear radiation at Chernobyl, extreme organisms raise many questions about the limits of life. Is there any environment where life could not “find a way”? Although many individual extremophilic organisms have been identified and studied, there remain outstanding questions about the limits of life and the extent to which extreme properties can be enhanced, combined or transferred to new organisms. In this review, we compile the current knowledge on the bioengineering of extremophile microbes. We summarize what is known about the basic mechanisms of extreme adaptations, compile synthetic biology’s efforts to engineer extremophile organisms beyond what is found in nature, and highlight which adaptations can be combined. The basic science of extremophiles can be applied to engineered organisms tailored to specific biomanufacturing needs, such as growth in high temperatures or in the presence of unusual solvents.
Introduction
Extremophilic microbes have long been studied in hopes of better understanding the origin and limits of life. Extremophile biology is also relevant to biomanufacturing (Ye et al., 2023), where large-scale growth occurs in non-natural, extreme chemical conditions ranging from the use of toxic waste streams as feedstocks to the intentional production of toxic chemicals like butane. The space science community hopes to push the capabilities of biomanufacturing even further for in situ resource utilization (ISRU) (Cockell, 2022), especially on human missions to the moon, Mars, and beyond. This will require microbes that are well adapted to chemically unusual feedstocks derived in part from highly oxidized Moon regolith or perchlorate-containing Mars regolith. A microbe that can thrive, growing and metabolizing at high rates, in extreme bioprocessing conditions can enable robust, high-yield, and low-cost synthesis of biological products. We aim to not just understand the basic science of extremophile biology, but also how that basic science supports current and future extremophilic bioengineering.
Precedent for bioengineering extremophilic traits
Extremophilic tools and traits can be engineered in two ways. Individual extremophilic enzymes such as thermostable amylases, cold-adapted β-glucosidases, DNA-dependent DNA polymerases, and high pH tolerant lipases enable extremophilic catalysis abilities for varied uses (Chien et al., 1976; Zhu et al., 2020). Whole extremophile organisms, including engineered strains, are used to produce ectoine, polyhydroxyalkanoate, polyhydroxybutyrate, and other bioproducts (Tan et al., 2011; Chavan et al., 2021; Hu et al., 2024). Growth in extreme environments also enables new kinds of fermentation, such as non-sterile continuous production in seawater, and gas fermentation in waste gasses from steel production (Yue et al., 2014; Molitor et al., 2016). Biomanufacturing in terrestrial or space processes will require advances in basic fermentation approaches. Desirable extremophilic traits such as tolerance to potentially toxic waste-derived feedstocks, growth in extreme environments hostile to contaminants, and desiccation tolerance open access to entirely new types of bioproduction processes (Chen and Jiang, 2018; Averesch et al., 2023).
The table below summarizes the current understanding of how each category of extremophile adaptation works, and whether there is precedent for deliberately endowing a new organism with this adaptation through bioengineering (Table 1). Growth of microorganisms in some extremes, such as low or high temperature and high radiation, have been comprehensively explored, and are mechanistically quite well understood. Other extremes require more complex equipment to simulate, most notably altered gravity and high and low pressure. This presents a substantial barrier for experimentation. For these extremes, initial studies have been completed, but more research is needed to replicate and interpret results.
Low gravity is an especially challenging extreme to study due to the cost and technical complexity of conducting experiments. Low gravity eliminates convection, which may substantially alter the function of microorganisms in ways that may be difficult to simulate on the ground. Low gravity simulation devices can prevent sedimentation but do not eliminate convection (Vroom et al., 2022), and it remains unknown the extent to which these devices are a good proxy for low gravity microbial growth. To reliably investigate low gravity conditions, experiments must be done on experimental platforms in space, such as in Low Earth Orbit.
Low atmospheric pressure would similarly benefit from further research. One paper adapts Bacillus subtilis to grow better in liquid culture exposed to low-pressure atmospheric conditions (Nicholson et al., 2010). However, it is unclear whether the experimental setup for this study selects for ‘growth at low pressure’ or simply growth in less well-aerated media. Analysis of the accumulated mutations suggests evolution was driven by the particular experimental conditions rather than low pressure. Mutations in rnjB, an RNAse, improves growth at 27°C regardless of air pressure (Waters et al., 2015), and can be interpreted as an adaptation of B. subtilis for low temperature. The rnjB mutation could account for most of the fitness gain, although additional mutations were also observed (Waters et al., 2021) impacting membrane fluidity and the regulation of anaerobic metabolism such as increased expression of nitrate reductases. Studies of organisms growing on solid media under low pressure may clarify some of these issues.
Tolerance to extremes can be engineered by directly transferring specific genes that improve microbes performance. For example, the transfer of the carrot gene hps17.7 to Saccharomyces cerevisiae improved both growth rate and maximum culture density under low temperature (25°C) and acidic conditions (pH 4) plus high osmolarity (0.8 M sorbitol). Percentage of survival at 47°C increased from 15% of the wild type to 38% of the engineered strain (Ko et al., 2017). This is one of the examples where genetic parts from one species can improve survival for another.
Thrive versus survive
When we envision using extremophiles for biomanufacturing, we require microbes that are capable of rapidly producing biomass under extreme conditions, not just surviving in a dormant state. The literature often conflates the ability to survive temporary exposure to extreme conditions with the ability to thrive and reproduce. Here, we explore the known limits of five well-studied extremophilic organisms; Bacillus subtilis, Deinococcus radiodurans, Thermus aquaticus, Psycromonas ingrahaii, and Thermus thermophilus; and their ability to thrive and survive in extreme environments (Figure 1, references in Supplementary Table S1). Engineered extremophiles should be measured against two separate benchmarks: their ability to thrive in an extreme environment (divide under extreme conditions) and separately their ability to survive (tolerate and reproduce after temporary exposure to even more extreme conditions). A specific example of this distinction can be seen between related alkalophilic and alkaline-tolerant Bacillus spp. (Guffanti et al., 1980). These strains differ in pH homeostatic mechanisms and growth pH range, though their cytoplasmic pH ranges share a common alkaline limit.
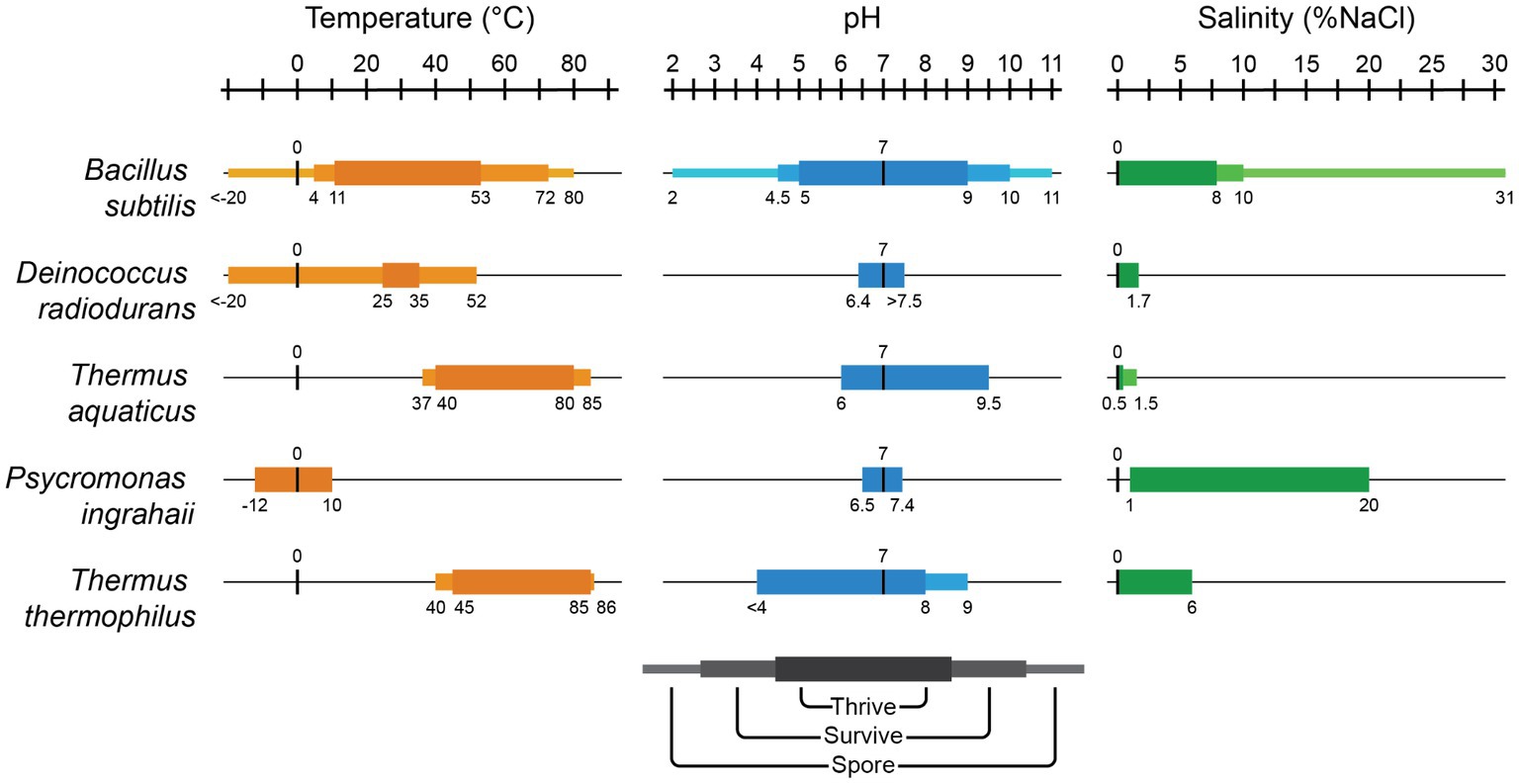
Figure 1. Microbes can survive conditions too extreme for growth. A visualization of the thriving and survival limits of organisms. For a list of citations for each organism in each condition, see Table S1. Graphs indicate the limits of thriving and survival in extreme conditions of temperature, pH, and salinity (% NaCl) for each of the five organisms.
Some organisms are capable of entering dormant states, becoming unable to replicate, but even more capable of surviving extreme conditions. For example, Bacillus experiences sporulation in which a copy of the genome to be encased in a metabolically inactive and well protected desiccated spore. When favorable conditions return, the spore can germinate, shedding its protective layers and initiating the formation of a new vegetative cell through the reactivation of essential cellular processes (McKenney et al., 2013). Organisms when in sporulated form can survive even more extreme conditions than their non-supported equivalents (Cho and Chung, 2020).
Somatic adaption versus genetic mutation
The extremophile literature often focuses on genome-level changes as the primary drivers of extremophilic properties. However, simply modulating the expression of endogenous genes is a more subtle yet equally profound aspect of the survival strategy of extremophiles. The dynamic nature of gene expression allows organisms to swiftly react to their environment, mounting an immediate defense against stressors without the long-term commitments tied to genetic mutations or new gene acquisition. For example, heavy water (D2O) stress in a variety of organisms is most consistent with adaptation being driven by changes in gene expression, rather than by genomic mutations (Katz and Crespi, 1966), and heterologous expression of a Deinococcus radiodurans transcriptional regulator alone can improve varied stress tolerances in multiple species (Wang et al., 2020). Extremophile bioengineering studies should separately characterize the contribution of gene reregulation, and the contribution of genome-level changes.
The root cause of stress in extreme conditions
In order to flourish, the cell must perform many essential functions, such as maintaining appropriate redox balance, membrane fluidity, protein stability balance, and limiting damage to DNA and proteins (Figure 2A). Studies of model microbes like E. coli and B. subtilus as well as non-model microbes like Lactobacillus delbrueckii and Acidithiobacillus ferrooxidans have begun to isolate the proximal mechanism by which extreme conditions disrupt these essential functions (Table 2). There are four broad classes of proximal cases of stress in extreme conditions: generation of reactive oxygen species (ROS), damaging DNA or proteins in ways that break or form chemical bonds, destabilizing the fold of a protein in ways that do not break chemical bonds, and altering the fluidity of the cell membrane. Different stressors most directly impact one or several of these proximal causes of stress. For example, high temperature directly destabilizes folded proteins and increases the error rate during DNA replication (Velichko et al., 2012).
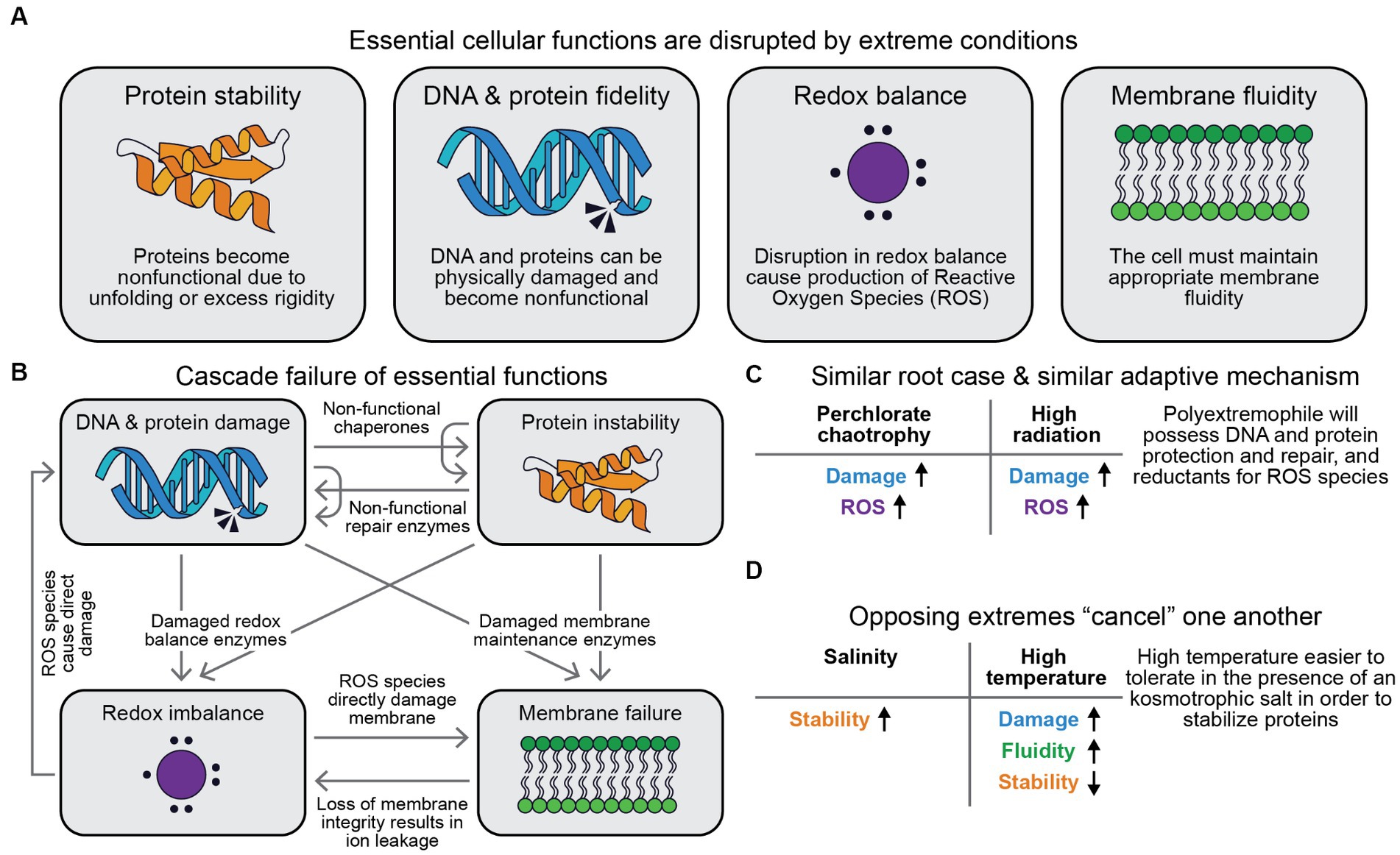
Figure 2. Root causes of stress under extreme conditions. There are several essential cellular functions that are frequently the proximal cause of disruption in extreme conditions (A). When an essential function is disrupted, it can lead to disruption of other essential functions, resulting in a cascade of failure (B). Resistance mechanisms can protect against multiple extremes with the same root cause (C), and stressors with opposite root causes can be easier to tolerate together than separately (D).
In addition to direct disruption of important cellular functions, breakdown of one function can lead to a further cascade of failures, such as unfolded DNA repair proteins further increasing the effective DNA replication error rate (Figure 2B). Understanding the root cause of stress in extreme conditions can allow us to reason about which polyextremophiles are biophysically realistic, and which adaptations they might possess. Organisms can tolerate multiple extremes at once if those extremes have the same root cause and thus a similar tolerance mechanism (Figure 2C), for example, the same genes enhance tolerance to both perchlorate and UV radiation in E. coli (Lamprecht-Grandío et al., 2020). For example: perchlorate tolerance and high radiation tolerance are both enabled by improved DNA and protein protection and repair (Slade and Radman, 2011; Lamprecht-Grandío et al., 2020). Freezing environment threats comprise crystals formation and local/temporal solute concentration. Similarly, salt tolerance and freeze–thaw tolerance can be synergistic: a metagenomic study of organisms from brines and alkaline lakes found that these salt-tolerant microbes are also 1,000-fold more resistant to freeze–thaw due to high intracellular levels of osmolytes and biofilm formation (Wilson et al., 2012). Conversely, some combinations of extremes are easier to tolerate together than separately because they exert opposing root causes (Figure 2D). High temperature is easier to tolerate in the presence of kosmotropic salts such as NaCl (Chin et al., 2010). Low temperature is easier to tolerate in the presence of chaotropic salts such as MgCl2 (Hallsworth et al., 2007; Chin et al., 2010). Haloalkaliphiles demonstrate adaptations to combined stresses (Wiegel and Kevbrin, 2004; Mesbah and Wiegel, 2012) and apparent trade-offs between adaptation to each separate stress (Mesbah and Wiegel, 2011; Banciu and Muntyan, 2015).
However, more research is needed into the mechanism of action of different stress adaptations. Not all extremophile organisms exhibit correlation between growth in multiple extremes that would be expected from Table 2. Early studies showed the D. radiodurans stress response to ionizing radiation shares mechanisms with desiccation response, suggesting adaptation to desiccation causes radiation tolerance (Shukla et al., 2007; Ujaoney et al., 2017). Later studies show desiccation stress and radiation tolerance are not correlated in anaerobes, while presence of certain manganese complexes was predictive of radiation tolerance across bacteria, fungi, archaea, and eukarya (Daly, 2009; Sharma et al., 2017; Beblo-Vranesevic et al., 2018). It is unknown how many different adaptations may be used to cope with a particular combination of extremes, if any. For example, there are no known microbes that grow robustly at extreme high and low pH (Jin and Kirk, 2018) or at extreme high and low temperature (Wiegel, 1990), though some organisms are claimed to tolerate ranges of 10 pH units and 60°C (Pandey et al., 2014). This may demonstrate a fundamental limit for microbial metabolism, or it may be a reflection of evolution in environments with limited variation in pH and temperature. Knowledge of compatibility, trade-offs, and relative efficiency between known adaptations will improve design and bioengineering of microbes with extremophilic traits.
Approaches for polyextremophile bioengineering
There are several approaches to engineer or enhance extreme properties in microbes depending on the type of stress to be addressed and the amount of prior knowledge about tolerance mechanisms. Biocontainment is an overarching consideration when engaging with an extremophile engineering campaign to prevent the release and uncontrolled spread of genetically engineered organisms. Rational design requires adding exogenous DNA with a known function, such as the tardigrade Dsup DNA repair gene which enhances survival by 2 orders of magnitude when transferred to E. coli grown in harsh conditions (Puig et al., 2021). Knowledge of specific extremophilic genes like tardigrade Dsup, as well as genome-scale models such as flux balance analysis (Noirungsee et al., 2024; Saldivar et al., 2024), can both be invaluable for engineering (Swayambhu et al., 2020). If there are no known genes with the needed function, metagenomic libraries sourced from extreme environments can search for genes that confer protection without foreknowledge of the sequence-function relationships (Biver et al., 2014; Culligan et al., 2014; Forsberg et al., 2016; Ausec et al., 2017).
With or without rational genetic engineering, directed evolution can be applied to improve desired properties. In directed evolution, where a library of variants from a starting organism are made, fitness is measured, and improved variants are used as the starting point for iterative rounds of improvement. In cases where an organism’s ability to grow in new extreme conditions is the evolutionary goal, the process is called adaptive lab evolution (Mavrommati et al., 2022). Changes across the whole proteome, not just a single protein or pathway, are required for global adaptations to multi-target stresses (Fernandes et al., 2023) such as high temperature (Deatherage et al., 2017), high salinity (Dhar et al., 2011), and high oxidative stress (Papiran and Hamedi, 2021). Some metabolic adaptations such as improved growth on alternative carbon sources (Chen et al., 2020; Espinosa et al., 2020) or efficient photosynthesis in high light (Dann et al., 2021) have been demonstrated, each requiring tens to hundreds of mutations. These adaptations can produce trade-offs, where fitness in the original growth conditions or resistance to other extremes is reduced (Caspeta and Nielsen, 2015; Cheung et al., 2021). Alternating selective conditions, such as switching between high and low temperature between growths, can produce different adaptations than selection in constant conditions (Lambros et al., 2021; Carpenter et al., 2023). Some trade-offs may be a reflection of the chosen selective conditions, not an underlying innate limit of biology.
Extremophile engineering can combine strategies logically (Figure 3). If there are known genes with known functions conferring resistance to the target extreme condition, they can be used as a starting point for engineering and directed evolution. If no usable sequence-function relationships are known, metagenomic screens and functional genomics can discover new genes with the desired function (Mirete et al., 2016). Adaptive laboratory evolution can be used to further integrate, refine and evolve the transferred genes within the original genome, adjusting molecular interactions, protein stability, expression levels, burden, etc.
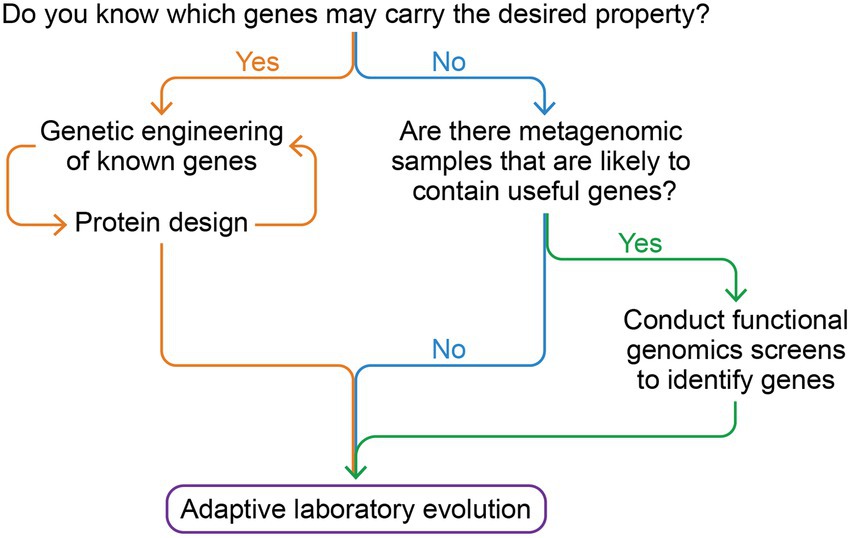
Figure 3. Polyextremophiles bioengineering approaches. This graph sketches the current approaches taken to evolve microorganisms, which can be combined in series to maximize the resilience achieved.
Conclusion
Our consistently improving understanding of extremophiles and their mechanisms of adaptation, together, provide new opportunities to actively engineer new extremophilic capabilities. Today, extremophile properties that are simple to simulate in the lab (especially high temperature tolerance, radiation tolerance, and salt tolerance) are thoroughly studied, well mechanistically understood, and the ability to deliberately engineer these properties in target microbes has been explored. To expand our capacity to engineer biology, scientists need new tools to identify and culture unusual extremophile microbes under stringent growth conditions, such as high pressure or low gravity. Climate scientists and the biomanufacturing industry can provide the essential insights to identify the best opportunities for the engineering of extreme biology. As the field shifts to focus toward bioengineering, so too will the vocabulary — the reporting for studies must focus more directly on measuring rates of biomass or protein production under extreme conditions, rather than simply reporting binary survival or death.
Unanswered questions remain about the extent to which extremophile properties can be combined, enhanced, or transferred to new microbes. Today, we know enough about the root causes of stress under extreme conditions to deliberately equip microbes with adaptations that are likely to enhance performance in a new extreme environment. However, natural organisms continue to surprise us with new mechanisms for adaptation, making it valuable to continue to sample and study new wildtype organisms from extreme environments. Further study into the fundamental limits of life, and new methods for systematic probes of these limits, will allow us to engineer custom microbes designed to thrive in the exotic, artificial niches encountered in the future.
Author contributions
JCA: Writing – original draft, Writing – review & editing. JM: Writing – review & editing. DS: Writing – review & editing. UN: Writing – review & editing. SP: Writing – review & editing. LV: Writing – review & editing. PS: Writing – review & editing. AH: Writing – review & editing. CC: Writing – review & editing. ED: Writing – original draft, Writing – review & editing.
Funding
The author(s) declare financial support was received for the research, authorship, and/or publication of this article. JCA, JM, and ED were supported by the Francis Crick Institute which receives its core funding from Cancer Research UK (CC2239), the UK Medical Research Council (CC2239), and the Wellcome Trust (CC2239), and a Steel Perlot Early Investigator Grant. DS, UN and ED are supported by funding from The Astera Institute. AH is supported by Medical Research Council core funding (MC-A658-5TY40).
Conflict of interest
The authors declare that the research was conducted in the absence of any commercial or financial relationships that could be construed as a potential conflict of interest.
Publisher's note
All claims expressed in this article are solely those of the authors and do not necessarily represent those of their affiliated organizations, or those of the publisher, the editors and the reviewers. Any product that may be evaluated in this article, or claim that may be made by its manufacturer, is not guaranteed or endorsed by the publisher.
Supplementary material
The Supplementary material for this article can be found online at: https://www.frontiersin.org/articles/10.3389/fmicb.2024.1341701/full#supplementary-material
References
Abdel-Banat, B. M. A., Hoshida, H., Ano, A., Nonklang, S., and Akada, R. (2010). High-temperature fermentation: how can processes for ethanol production at high temperatures become superior to the traditional process using mesophilic yeast? Appl. Microbiol. Biotechnol. 85, 861–867. doi: 10.1007/s00253-009-2248-5
Aguilar, R., Khan, L., Arslanovic, N., Birmingham, K., Kasliwal, K., Posnikoff, S., et al. (2023). Multivalent binding of the tardigrade Dsup protein to chromatin promotes yeast survival and longevity upon exposure to oxidative damage. Res. Sq. 2023:883. doi: 10.21203/rs.3.rs-3182883/v1
An, R., Jia, Y., Wan, B., Zhang, Y., Dong, P., Li, J., et al. (2014). Non-enzymatic depurination of nucleic acids: factors and mechanisms. PLoS One 9:e115950. doi: 10.1371/journal.pone.0115950
Atasoy, M., Álvarez Ordóñez, A., Cenian, A., Djukić-Vuković, A., Lund, P. A., Ozogul, F., et al. (2024). Exploitation of microbial activities at low pH to enhance planetary health. FEMS Microbiol. Rev. 48:62. doi: 10.1093/femsre/fuad062
Ausec, L., Berini, F., Casciello, C., Cretoiu, M. S., van Elsas, J. D., Marinelli, F., et al. (2017). The first acidobacterial laccase-like multicopper oxidase revealed by metagenomics shows high salt and thermo-tolerance. Appl. Microbiol. Biotechnol. 101, 6261–6276. doi: 10.1007/s00253-017-8345-y
Averesch, N. J. H., Berliner, A. J., Nangle, S. N., Zezulka, S., Vengerova, G. L., Ho, D., et al. (2023). Microbial biomanufacturing for space-exploration-what to take and when to make. Nat. Commun. 14:2311. doi: 10.1038/s41467-023-37910-1
Banciu, H. L., and Muntyan, M. S. (2015). Adaptive strategies in the double-extremophilic prokaryotes inhabiting soda lakes. Curr. Opin. Microbiol. 25, 73–79. doi: 10.1016/j.mib.2015.05.003
Banfield, D., Spiga, A., Newman, C., Forget, F., Lemmon, M., Lorenz, R., et al. (2020). The atmosphere of Mars as observed by InSight. Nat. Geosci. 13, 190–198. doi: 10.1038/s41561-020-0534-0
Beblo-Vranesevic, K., Bohmeier, M., Perras, A. K., Schwendner, P., Rabbow, E., Moissl-Eichinger, C., et al. (2018). Lack of correlation of desiccation and radiation tolerance in microorganisms from diverse extreme environments tested under anoxic conditions. FEMS Microbiol. Lett. 365:44. doi: 10.1093/femsle/fny044
Beney, L., Mille, Y., and Gervais, P. (2004). Death of Escherichia coli during rapid and severe dehydration is related to lipid phase transition. Appl. Microbiol. Biotechnol. 65, 457–464. doi: 10.1007/s00253-004-1574-x
Bhardwaj, A., Sam, L., Martín-Torres, F. J., Zorzano, M.-P., and Fonseca, R. M. (2017). Martian slope streaks as plausible indicators of transient water activity. Sci. Rep. 7:7074. doi: 10.1038/s41598-017-07453-9
Bicalho, I. C., Mognon, J. L., Shimoyama, J., Ataíde, C. H., and Duarte, C. R. (2012). Separation of yeast from alcoholic fermentation in small hydrocyclones. Sep. Purif. Technol. 87, 62–70. doi: 10.1016/j.seppur.2011.11.023
Billi, D., and Potts, M. (2002). Life and death of dried prokaryotes. Res. Microbiol. 153, 7–12. doi: 10.1016/S0923-2508(01)01279-7
Billi, D., Wright, D. J., Helm, R. F., Prickett, T., Potts, M., and Crowe, J. H. (2000). Engineering desiccation tolerance in Escherichia coli. Appl. Environ. Microbiol. 66, 1680–1684. doi: 10.1128/AEM.66.4.1680-1684.2000
Biver, S., Stroobants, A., Portetelle, D., and Vandenbol, M. (2014). Two promising alkaline β-glucosidases isolated by functional metagenomics from agricultural soil, including one showing high tolerance towards harsh detergents, oxidants and glucose. J. Ind. Microbiol. Biotechnol. 41, 479–488. doi: 10.1007/s10295-014-1400-0
Boiteau, R. M., Mende, D. R., Hawco, N. J., McIlvin, M. R., Fitzsimmons, J. N., Saito, M. A., et al. (2016). Siderophore-based microbial adaptations to iron scarcity across the eastern Pacific Ocean. Proc. Natl. Acad. Sci. USA 113, 14237–14242. doi: 10.1073/pnas.1608594113
Bolhuis, H., Palm, P., Wende, A., Falb, M., Rampp, M., Rodriguez-Valera, F., et al. (2006). The genome of the square archaeon Haloquadratum walsbyi: life at the limits of water activity. BMC Genomics 7:169. doi: 10.1186/1471-2164-7-169
Cabello-Yeves, P. J., and Rodriguez-Valera, F. (2019). Marine-freshwater prokaryotic transitions require extensive changes in the predicted proteome. Microbiome 7:117. doi: 10.1186/s40168-019-0731-5
Carpenter, A. C., Feist, A. M., Harrison, F. S. M., Paulsen, I. T., and Williams, T. C. (2023). Have you tried turning it off and on again? Oscillating selection to enhance fitness-landscape traversal in adaptive laboratory evolution experiments. Metab. Eng. Commun. 17:e00227. doi: 10.1016/j.mec.2023.e00227
Caspeta, L., and Nielsen, J. (2015). Thermotolerant yeast strains adapted by laboratory evolution show trade-off at ancestral temperatures and preadaptation to other stresses. MBio 6:e00431. doi: 10.1128/mBio.00431-15
Chavan, S., Yadav, B., Tyagi, R. D., and Drogui, P. (2021). A review on production of polyhydroxyalkanoate (PHA) biopolyesters by thermophilic microbes using waste feedstocks. Bioresour. Technol. 341:125900. doi: 10.1016/j.biortech.2021.125900
Chavez, C., Cruz-Becerra, G., Fei, J., Kassavetis, G. A., and Kadonaga, J. T. (2019). The tardigrade damage suppressor protein binds to nucleosomes and protects DNA from hydroxyl radicals. eLife 8:7682. doi: 10.7554/eLife.47682
Chen, G.-Q., and Jiang, X.-R. (2018). Next generation industrial biotechnology based on extremophilic bacteria. Curr. Opin. Biotechnol. 50, 94–100. doi: 10.1016/j.copbio.2017.11.016
Chen, F. Y.-H., Jung, H.-W., Tsuei, C.-Y., and Liao, J. C. (2020). Converting Escherichia coli to a synthetic Methylotroph growing solely on methanol. Cell 182, 933–946.e14. doi: 10.1016/j.cell.2020.07.010
Cheung, C. H. P., Alorabi, M., Hamilton, F., Takebayashi, Y., Mounsey, O., Heesom, K. J., et al. (2021). Trade-offs between antibacterial resistance and fitness cost in the production of metallo-β-lactamases by enteric bacteria manifest as sporadic emergence of carbapenem resistance in a clinical setting. Antimicrob. Agents Chemother. 65:e0241220. doi: 10.1128/AAC.02412-20
Chien, A., Edgar, D. B., and Trela, J. M. (1976). Deoxyribonucleic acid polymerase from the extreme thermophile Thermus aquaticus. J. Bacteriol. 127, 1550–1557. doi: 10.1128/jb.127.3.1550-1557.1976
Chin, J. P., Megaw, J., Magill, C. L., Nowotarski, K., Williams, J. P., Bhaganna, P., et al. (2010). Solutes determine the temperature windows for microbial survival and growth. Proc. Natl. Acad. Sci. U. S. A. 107, 7835–7840. doi: 10.1073/pnas.1000557107
Cho, W.-I., and Chung, M.-S. (2020). Bacillus spores: a review of their properties and inactivation processing technologies. Food Sci. Biotechnol. 29, 1447–1461. doi: 10.1007/s10068-020-00809-4
Christ, D., and Chin, J. W. (2008). Engineering Escherichia coli heat-resistance by synthetic gene amplification. Protein Eng. Des. Sel. 21, 121–125. doi: 10.1093/protein/gzm085
Cockell, C. S. (2022). Bridging the gap between microbial limits and extremes in space: space microbial biotechnology in the next 15 years. Microb. Biotechnol. 15, 29–41. doi: 10.1111/1751-7915.13927
Collard, J. M., Corbisier, P., Diels, L., Dong, Q., Jeanthon, C., Mergeay, M., et al. (1994). Plasmids for heavy metal resistance in Alcaligenes eutrophus CH34: mechanisms and applications. FEMS Microbiol. Rev. 14, 405–414. doi: 10.1111/j.1574-6976.1994.tb00115.x
Cordell, D., and White, S. (2014). Life’s bottleneck: sustaining the World's phosphorus for a food secure future. Annu. Rev. Environ. Resour. 39, 161–188. doi: 10.1146/annurev-environ-010213-113300
Corral, P., Amoozegar, M. A., and Ventosa, A. (2019). Halophiles and their biomolecules: recent advances and future applications in biomedicine. Mar. Drugs 18:33. doi: 10.3390/md18010033
Cray, J. A., Russell, J. T., Timson, D. J., Singhal, R. S., and Hallsworth, J. E. (2013). A universal measure of chaotropicity and kosmotropicity. Environ. Microbiol. 15, 287–296. doi: 10.1111/1462-2920.12018
Cray, J. A., Stevenson, A., Ball, P., Bankar, S. B., Eleutherio, E. C. A., Ezeji, T. C., et al. (2015). Chaotropicity: a key factor in product tolerance of biofuel-producing microorganisms. Curr. Opin. Biotechnol. 33, 228–259. doi: 10.1016/j.copbio.2015.02.010
Culligan, E. P., Marchesi, J. R., Hill, C., and Sleator, R. D. (2014). Combined metagenomic and phenomic approaches identify a novel salt tolerance gene from the human gut microbiome. Front. Microbiol. 5:189. doi: 10.3389/fmicb.2014.00189
Cycil, L. M., Hausrath, E. M., Ming, D. W., Adcock, C. T., Raymond, J., Remias, D., et al. (2021). Investigating the growth of algae under low atmospheric pressures for potential food and oxygen production on Mars. Front. Microbiol. 12:733244. doi: 10.3389/fmicb.2021.733244
D’Amico, S., Collins, T., Marx, J.-C., Feller, G., and Gerday, C. (2006). Psychrophilic microorganisms: challenges for life. EMBO Rep. 7, 385–389. doi: 10.1038/sj.embor.7400662
Daly, M. J. (2009). A new perspective on radiation resistance based on Deinococcus radiodurans. Nat. Rev. Microbiol. 7, 237–245. doi: 10.1038/nrmicro2073
Daly, C., Widrlechner, M. P., Halbleib, M. D., Smith, J. I., and Gibson, W. P. (2012). Development of a new USDA Plant hardiness zone map for the United States. J. Appl. Meteorol. Climatol. 51, 242–264. doi: 10.1175/2010JAMC2536.1
Dann, M., Ortiz, E. M., Thomas, M., Guljamow, A., Lehmann, M., Schaefer, H., et al. (2021). Enhancing photosynthesis at high light levels by adaptive laboratory evolution. Nat. Plants 7, 681–695. doi: 10.1038/s41477-021-00904-2
DasSarma, S., and DasSarma, P. (2015). Halophiles and their enzymes: negativity put to good use. Curr. Opin. Microbiol. 25, 120–126. doi: 10.1016/j.mib.2015.05.009
Deatherage, D. E., Kepner, J. L., Bennett, A. F., Lenski, R. E., and Barrick, J. E. (2017). Specificity of genome evolution in experimental populations of Escherichia coli evolved at different temperatures. Proc. Natl. Acad. Sci. U. S. A. 114, E1904–E1912. doi: 10.1073/pnas.1616132114
Deguchi, S., Shimoshige, H., Tsudome, M., Mukai, S. A., Corkery, R. W., Ito, S., et al. (2011). Microbial growth at hyperaccelerations up to 403,627 × g. Proc. Natl. Acad. Sci. 108, 7997–8002. doi: 10.1073/pnas.1018027108
DeLeon-Rodriguez, N., Lathem, T. L., Rodriguez-R, L. M., Barazesh, J. M., Anderson, B. E., Beyersdorf, A. J., et al. (2013). Microbiome of the upper troposphere: species composition and prevalence, effects of tropical storms, and atmospheric implications. Proc. Natl. Acad. Sci. U. S. A. 110, 2575–2580. doi: 10.1073/pnas.1212089110
Devi, A., Singh, A., Bajar, S., Pant, D., and Din, Z. U. (2021). Ethanol from lignocellulosic biomass: An in-depth analysis of pre-treatment methods, fermentation approaches and detoxification processes. J. Environ. Chem. Eng. 9:105798. doi: 10.1016/j.jece.2021.105798
Dhar, R., Sägesser, R., Weikert, C., Yuan, J., and Wagner, A. (2011). Adaptation of Saccharomyces cerevisiae to saline stress through laboratory evolution. J. Evol. Biol. 24, 1135–1153. doi: 10.1111/j.1420-9101.2011.02249.x
Díaz-Rullo, J., Rodríguez-Valdecantos, G., Torres-Rojas, F., Cid, L., Vargas, I. T., González, B., et al. (2021). Mining for perchlorate resistance genes in microorganisms from sediments of a hypersaline pond in Atacama Desert. Chile. Front. Microbiol. 12:723874. doi: 10.3389/fmicb.2021.723874
Dong, Z., Sun, T., Zhang, W., and Chen, L. (2023). Improved salt tolerance of Synechococcus elongatus PCC 7942 by heterologous synthesis of compatible solute ectoine. Front. Microbiol. 14:1123081. doi: 10.3389/fmicb.2023.1123081
Dunlop, M. J., Dossani, Z. Y., Szmidt, H. L., Chu, H. C., Lee, T. S., Keasling, J. D., et al. (2011). Engineering microbial biofuel tolerance and export using efflux pumps. Mol. Syst. Biol. 7:487. doi: 10.1038/msb.2011.21
Duri, L. G., Caporale, A. G., Rouphael, Y., Vingiani, S., Palladino, M., de Pascale, S., et al. (2022). The potential for lunar and Martian regolith simulants to sustain plant growth: a multidisciplinary overview. Front. Astron. Space Sci. 8:7821. doi: 10.3389/fspas.2021.747821
Eichler, A., Hadland, N., Pickett, D., Masaitis, D., Handy, D., Perez, A., et al. (2021). Challenging the agricultural viability of martian regolith simulants. Icarus 354:114022. doi: 10.1016/j.icarus.2020.114022
Espinosa, M. I., Gonzalez-Garcia, R. A., Valgepea, K., Plan, M. R., Scott, C., Pretorius, I. S., et al. (2020). Adaptive laboratory evolution of native methanol assimilation in Saccharomyces cerevisiae. Nat. Commun. 11:5564. doi: 10.1038/s41467-020-19390-9
Fernandes, T., Osório, C., Sousa, M. J., and Franco-Duarte, R. (2023). Contributions of adaptive laboratory evolution towards the enhancement of the biotechnological potential of non-conventional yeast species. J. Fungi (Basel) 9:186. doi: 10.3390/jof9020186
Ferras, E., Minier, M., and Goma, G. (1986). Acetonobutylic fermentation: improvement of performances by coupling continuous fermentation and ultrafiltration. Biotechnol. Bioeng. 28, 523–533. doi: 10.1002/bit.260280408
Ferrer, M., Chernikova, T. N., Yakimov, M. M., Golyshin, P. N., and Timmis, K. N. (2003). Chaperonins govern growth of Escherichia coli at low temperatures. Nat. Biotechnol. 21, 1266–1267. doi: 10.1038/nbt1103-1266
Fletcher, E., and Baetz, K. (2020). Multi-faceted systems biology approaches present a cellular landscape of phenolic compound inhibition in Saccharomyces cerevisiae. Front. Bioeng. Biotechnol. 8:539902. doi: 10.3389/fbioe.2020.539902
Fletcher, E., Gao, K., Mercurio, K., Ali, M., and Baetz, K. (2019). Yeast chemogenomic screen identifies distinct metabolic pathways required to tolerate exposure to phenolic fermentation inhibitors ferulic acid, 4-hydroxybenzoic acid and coniferyl aldehyde. Metab. Eng. 52, 98–109. doi: 10.1016/j.ymben.2018.11.010
Forsberg, K. J., Patel, S., Witt, E., Wang, B., Ellison, T. D., and Dantas, G. (2016). Identification of genes conferring tolerance to lignocellulose-derived inhibitors by functional selections in soil metagenomes. Appl. Environ. Microbiol. 82, 528–537. doi: 10.1128/AEM.02838-15
Fox-Powell, M. G., Hallsworth, J. E., Cousins, C. R., and Cockell, C. S. (2016). Ionic strength is a barrier to the habitability of Mars. Astrobiology 16, 427–442. doi: 10.1089/ast.2015.1432
França, M. B., Panek, A. D., and Eleutherio, E. C. A. (2007). Oxidative stress and its effects during dehydration. Comp. Biochem. Physiol. A Mol. Integr. Physiol. 146, 621–631. doi: 10.1016/j.cbpa.2006.02.030
Gall, J. E., Boyd, R. S., and Rajakaruna, N. (2015). Transfer of heavy metals through terrestrial food webs: a review. Environ. Monit. Assess. 187:201. doi: 10.1007/s10661-015-4436-3
Gayen, P., Sankarasubramanian, S., and Ramani, V. K. (2020). Fuel and oxygen harvesting from Martian regolithic brine. Proc. Natl. Acad. Sci. USA 117, 31685–31689. doi: 10.1073/pnas.2008613117
Gekas, V., Gonzalez, C., Sereno, A., Chiralt, A., and Fito, P. (1998). Mass transfer properties of osmotic solutions. I. Water activity and osmotic pressure. Int. J. Food Prop. 1, 95–112. doi: 10.1080/10942919809524570
Greffe, V. R. G., and Michiels, J. (2020). Desiccation-induced cell damage in bacteria and the relevance for inoculant production. Appl. Microbiol. Biotechnol. 104, 3757–3770. doi: 10.1007/s00253-020-10501-6
Guan, N., and Liu, L. (2020). Microbial response to acid stress: mechanisms and applications. Appl. Microbiol. Biotechnol. 104, 51–65. doi: 10.1007/s00253-019-10226-1
Guazzaroni, M.-E., Morgante, V., Mirete, S., and González-Pastor, J. E. (2013). Novel acid resistance genes from the metagenome of the Tinto River, an extremely acidic environment. Environ. Microbiol. 15, 1088–1102. doi: 10.1111/1462-2920.12021
Guffanti, A. A., Blanco, R., Benenson, R. A., and Krulwich, T. A. (1980). Bioenergetic properties of alkaline-tolerant and Alkalophilic strains of Bacillus firmus. Microbiology 119, 79–86. doi: 10.1099/00221287-119-1-79
Gumulya, Y., Zea, L., and Kaksonen, A. H. (2022). In situ resource utilisation: the potential for space biomining. Miner. Eng. 176:107288. doi: 10.1016/j.mineng.2021.107288
Haifer, C., Paramsothy, S., Borody, T. J., Clancy, A., Leong, R. W., and Kaakoush, N. O. (2021). Long-term bacterial and fungal dynamics following Oral lyophilized fecal microbiota transplantation in Clostridioides difficile infection. mSystems 6:20. doi: 10.1128/mSystems.00905-20
Hallsworth, J. E., Yakimov, M. M., Golyshin, P. N., Gillion, J. L. M., D'Auria, G., de Lima Alves, F., et al. (2007). Limits of life in MgCl2-containing environments: chaotropicity defines the window. Environ. Microbiol. 9, 801–813. doi: 10.1111/j.1462-2920.2006.01212.x
Han, S., Hu, Z., and Lei, A. (2008). Expression and function analysis of the metallothionein-like (MT-like) gene from Festuca rubra in Chlamydomonas reinhardtii chloroplast. Sci China C Life Sci 51, 1076–1081. doi: 10.1007/s11427-008-0136-3
Hand, K. P., and Chyba, C. F. (2007). Empirical constraints on the salinity of the Europan ocean and implications for a thin ice shell. Icarus 189, 424–438. doi: 10.1016/j.icarus.2007.02.002
Haque, M. A., and Russell, N. J. (2004). Strains of Bacillus cereus vary in the phenotypic adaptation of their membrane lipid composition in response to low water activity, reduced temperature and growth in rice starch. Microbiology 150, 1397–1404. doi: 10.1099/mic.0.26767-0
Hecht, M. H., Kounaves, S. P., Quinn, R. C., West, S. J., Young, S. M. M., Ming, D. W., et al. (2009). Detection of perchlorate and the soluble chemistry of martian soil at the Phoenix lander site. Science 325, 64–67. doi: 10.1126/science.1172466
Heinz, J., Waajen, A. C., Airo, A., Alibrandi, A., Schirmack, J., and Schulze-Makuch, D. (2019). Bacterial growth in chloride and perchlorate brines: Halotolerances and salt stress responses of Planococcus halocryophilus. Astrobiology 19, 1377–1387. doi: 10.1089/ast.2019.2069
Hema, R., Senthil-Kumar, M., Shivakumar, S., Chandrasekhara Reddy, P., and Udayakumar, M. (2007). Chlamydomonas reinhardtii, a model system for functional validation of abiotic stress responsive genes. Planta 226, 655–670. doi: 10.1007/s00425-007-0514-2
Hendrix, A. R., Hurford, T. A., Barge, L. M., Bland, M. T., Bowman, J. S., Brinckerhoff, W., et al. (2019). The NASA roadmap to ocean worlds. Astrobiology 19, 1–27. doi: 10.1089/ast.2018.1955
Hoffman, J. H., Hodges, R. R., McElroy, M. B., Donahue, T. M., and Kolpin, M. (1979). Composition and structure of the venus atmosphere: results from Pioneer Venus. Science 205, 49–52. doi: 10.1126/science.205.4401.49
Hossein Helalat, S., Bidaj, S., Samani, S., and Moradi, M. (2019). Producing alcohol and salt stress tolerant strain of Saccharomyces cerevisiae by heterologous expression of pprI gene. Enzym. Microb. Technol. 124, 17–22. doi: 10.1016/j.enzmictec.2019.01.008
Hu, Q., Sun, S., Zhang, Z., Liu, W., Yi, X., He, H., et al. (2024). Ectoine hyperproduction by engineered Halomonas bluephagenesis. Metab. Eng. 82, 238–249. doi: 10.1016/j.ymben.2024.02.010
Huang, B., Li, D.-G., Huang, Y., and Liu, C.-T. (2018). Effects of spaceflight and simulated microgravity on microbial growth and secondary metabolism. Mil. Med. Res. 5:18. doi: 10.1186/s40779-018-0162-9
Huang, C.-J., Lu, M.-Y., Chang, Y.-W., and Li, W.-H. (2018). Experimental evolution of yeast for high-temperature tolerance. Mol. Biol. Evol. 35, 1823–1839. doi: 10.1093/molbev/msy077
Irihimovitch, V., and Yehudai-Resheff, S. (2008). Phosphate and sulfur limitation responses in the chloroplast of Chlamydomonas reinhardtii. FEMS Microbiol. Lett. 283, 1–8. doi: 10.1111/j.1574-6968.2008.01154.x
Janto, B., Ahmed, A., Ito, M., Liu, J., Hicks, D. B., Pagni, S., et al. (2011). Genome of alkaliphilic Bacillus pseudofirmus OF4 reveals adaptations that support the ability to grow in an external pH range from 7.5 to 11.4. Environ. Microbiol. 13, 3289–3309. doi: 10.1111/j.1462-2920.2011.02591.x
Jayakody, L. N., and Jin, Y.-S. (2021). In-depth understanding of molecular mechanisms of aldehyde toxicity to engineer robust Saccharomyces cerevisiae. Appl. Microbiol. Biotechnol. 105, 2675–2692. doi: 10.1007/s00253-021-11213-1
Jin, Q., and Kirk, M. F. (2018). PH as a primary control in environmental microbiology: 1. Thermodynamic perspective. Front. Environ. Sci. Eng. China 6:21. doi: 10.3389/fenvs.2018.00021
Jones, D. L., and Oburger, E. (2011). “Solubilization of phosphorus by soil microorganisms” in Phosphorus in action: Biological processes in soil phosphorus cycling. eds. E. Bünemann, A. Oberson, and E. Frossard (Berlin, Heidelberg: Springer), 169–198.
Kasahara, R., Sato, T., Tamegai, H., and Kato, C. (2009). Piezo-adapted 3-isopropylmalate dehydrogenase of the obligate piezophile Shewanella benthica DB21MT-2 isolated from the 11,000-m depth of the Mariana trench. Biosci. Biotechnol. Biochem. 73, 2541–2543. doi: 10.1271/bbb.90448
Kasiviswanathan, P., Swanner, E. D., Halverson, L. J., and Vijayapalani, P. (2022). Farming on Mars: treatment of basaltic regolith soil and briny water simulants sustains plant growth. PLoS One 17:e0272209. doi: 10.1371/journal.pone.0272209
Katz, J. J., and Crespi, H. L. (1966). Deuterated organisms: cultivation and uses. Science 151, 1187–1194. doi: 10.1126/science.151.3715.1187
Kearney, P. C., Woolson, E. A., and Ellington, C. P. Jr. (1972). Persistence and metabolism of chlorodioxins in soils. Environ. Sci. Technol. 6, 1017–1019. doi: 10.1021/es60071a010
Kellermann, M. Y., Yoshinaga, M. Y., Valentine, R. C., Wörmer, L., and Valentine, D. L. (2016). Important roles for membrane lipids in haloarchaeal bioenergetics. Biochim. Biophys. Acta 1858, 2940–2956. doi: 10.1016/j.bbamem.2016.08.010
Klingler, J. M., Mancinelli, R. L., and White, M. R. (1989). Biological nitrogen fixation under primordial Martian partial pressures of dinitrogen. Adv. Space Res. 9, 173–176. doi: 10.1016/0273-1177(89)90225-1
Klinke, H. B., Thomsen, A. B., and Ahring, B. K. (2004). Inhibition of ethanol-producing yeast and bacteria by degradation products produced during pre-treatment of biomass. Appl. Microbiol. Biotechnol. 66, 10–26. doi: 10.1007/s00253-004-1642-2
Ko, E., Kim, M., Park, Y., and Ahn, Y.-J. (2017). Heterologous expression of the carrot Hsp17.7 gene increased growth, cell viability, and protein solubility in transformed yeast (Saccharomyces cerevisiae) under heat, cold, acid, and osmotic stress conditions. Curr. Microbiol. 74, 952–960. doi: 10.1007/s00284-017-1269-z
Kobayashi, T., Sakamoto, A., Kashiwagi, K., Igarashi, K., Moriya, T., Oshima, T., et al. (2022). Alkaline stress causes changes in polyamine biosynthesis in Thermus thermophilus. Int. J. Mol. Sci. 23:523. doi: 10.3390/ijms232113523
Kruyer, N. S., Realff, M. J., Sun, W., Genzale, C. L., and Peralta-Yahya, P. (2021). Designing the bioproduction of Martian rocket propellant via a biotechnology-enabled in situ resource utilization strategy. Nat. Commun. 12:6166. doi: 10.1038/s41467-021-26393-7
Kumar, S., Paul, D., Bhushan, B., Wakchaure, G. C., Meena, K. K., and Shouche, Y. (2020). Traversing the ‘Omic’ landscape of microbial halotolerance for key molecular processes and new insights. Crit. Rev. Microbiol. 46, 631–653. doi: 10.1080/1040841X.2020.1819770
Kuroda, K., Shibasaki, S., Ueda, M., and Tanaka, A. (2001). Cell surface-engineered yeast displaying a histidine oligopeptide (hexa-his) has enhanced adsorption of and tolerance to heavy metal ions. Appl. Microbiol. Biotechnol. 57, 697–701. doi: 10.1007/s002530100813
Kwon, Y. W., Bae, J.-H., Kim, S.-A., and Han, N. S. (2018). Development of freeze-thaw tolerant Lactobacillus rhamnosus GG by adaptive laboratory evolution. Front. Microbiol. 9:2781. doi: 10.3389/fmicb.2018.02781
Laadan, B., Wallace-Salinas, V., Carlsson, Å. J., Almeida, J. R. M., Rådström, P., and Gorwa-Grauslund, M. F. (2014). Furaldehyde substrate specificity and kinetics of Saccharomyces cerevisiae alcohol dehydrogenase 1 variants. Microb. Cell Factories 13:112. doi: 10.1186/s12934-014-0112-5
Lambros, M., Pechuan-Jorge, X., Biro, D., Ye, K., and Bergman, A. (2021). Emerging adaptive strategies under temperature fluctuations in a laboratory evolution experiment of Escherichia Coli. Front. Microbiol. 12:724982. doi: 10.3389/fmicb.2021.724982
Lamprecht-Grandío, M., Cortesão, M., Mirete, S., de la Cámara, M. B., de Figueras, C. G., Pérez-Pantoja, D., et al. (2020). Novel genes involved in resistance to both ultraviolet radiation and perchlorate from the metagenomes of hypersaline environments. Front. Microbiol. 11:453. doi: 10.3389/fmicb.2020.00453
LaPanse, A. J., Burch, T. A., Tamburro, J. M., Traller, J. C., Pinowska, A., and Posewitz, M. C. (2023). Adaptive laboratory evolution for increased temperature tolerance of the diatom Nitzschia inconspicua. Microbiology 12:e1343. doi: 10.1002/mbo3.1343
Larsson, E. M., Murray, R. M., and Newman, D. K. (2024). Engineering the soil bacterium Pseudomonas synxantha 2-79 into a Ratiometric bioreporter for phosphorus limitation. ACS Synth. Biol. 13, 384–393. doi: 10.1021/acssynbio.3c00642
Laskowska, E., and Kuczyńska-Wiśnik, D. (2020). New insight into the mechanisms protecting bacteria during desiccation. Curr. Genet. 66, 313–318. doi: 10.1007/s00294-019-01036-z
Lebre, P. H., De Maayer, P., and Cowan, D. A. (2017). Xerotolerant bacteria: surviving through a dry spell. Nat. Rev. Microbiol. 15, 285–296. doi: 10.1038/nrmicro.2017.16
Lennen, R. M., Lim, H. G., Jensen, K., Mohammed, E. T., Phaneuf, P. V., Noh, M. H., et al. (2023). Laboratory evolution reveals general and specific tolerance mechanisms for commodity chemicals. Metab. Eng. 76, 179–192. doi: 10.1016/j.ymben.2023.01.012
Levakov, I., Ronen, Z., and Dahan, O. (2019). Combined in-situ bioremediation treatment for perchlorate pollution in the vadose zone and groundwater. J. Hazard. Mater. 369, 439–447. doi: 10.1016/j.jhazmat.2019.02.014
Li, H., Cong, Y., Lin, J., and Chang, Y. (2015). Enhanced tolerance and accumulation of heavy metal ions by engineered Escherichia coli expressing Pyrus calleryana phytochelatin synthase. J. Basic Microbiol. 55, 398–405. doi: 10.1002/jobm.201300670
Liszkowska, W., and Berlowska, J. (2021). Yeast fermentation at low temperatures: adaptation to changing environmental conditions and formation of volatile compounds. Molecules 26:1035. doi: 10.3390/molecules26041035
Liu, Z. L. (2021). Reasons for 2-furaldehyde and 5-hydroxymethyl-2-furaldehyde resistance in Saccharomyces cerevisiae: current state of knowledge and perspectives for further improvements. Appl. Microbiol. Biotechnol. 105, 2991–3007. doi: 10.1007/s00253-021-11256-4
Liu, Z., Fels, M., Dragone, G., and Mussatto, S. I. (2021). Effects of inhibitory compounds derived from lignocellulosic biomass on the growth of the wild-type and evolved oleaginous yeast Rhodosporidium toruloides. Ind. Crop. Prod. 170:113799. doi: 10.1016/j.indcrop.2021.113799
Mapstone, L. J., Leite, M. N., Purton, S., Crawford, I. A., and Dartnell, L. (2022). Cyanobacteria and microalgae in supporting human habitation on Mars. Biotechnol. Adv. 59:107946. doi: 10.1016/j.biotechadv.2022.107946
Mavrommati, M., Daskalaki, A., Papanikolaou, S., and Aggelis, G. (2022). Adaptive laboratory evolution principles and applications in industrial biotechnology. Biotechnol. Adv. 54:107795. doi: 10.1016/j.biotechadv.2021.107795
McKenney, P. T., Driks, A., and Eichenberger, P. (2013). The Bacillus subtilis endospore: assembly and functions of the multilayered coat. Nat. Rev. Microbiol. 11, 33–44. doi: 10.1038/nrmicro2921
Menzel, U., and Gottschalk, G. (1985). The internal pH of Acetobacterium wieringae and Acetobacter aceti during growth and production of acetic acid. Arch. Microbiol. 143, 47–51. doi: 10.1007/BF00414767
Mesbah, N. M., and Wiegel, J. (2011). The Na+−translocating F1FO-ATPase from the halophilic, alkalithermophile Natranaerobius thermophilus. Biochim. Biophys. Acta 1807, 1133–1142. doi: 10.1016/j.bbabio.2011.05.001
Mesbah, N. M., and Wiegel, J. (2012). Life under multiple extreme conditions: diversity and physiology of the halophilic alkalithermophiles. Appl. Environ. Microbiol. 78, 4074–4082. doi: 10.1128/AEM.00050-12
Min, J., Pham, C. H., and Gu, M. B. (2003). Specific responses of bacterial cells to dioxins. Environ. Toxicol. Chem. 22, 233–238. doi: 10.1002/etc.5620220201
Mirete, S., Morgante, V., and González-Pastor, J. E. (2016). Functional metagenomics of extreme environments. Curr. Opin. Biotechnol. 38, 143–149. doi: 10.1016/j.copbio.2016.01.017
Molitor, B., Richter, H., Martin, M. E., Jensen, R. O., Juminaga, A., Mihalcea, C., et al. (2016). Carbon recovery by fermentation of CO-rich off gases - turning steel mills into biorefineries. Bioresour. Technol. 215, 386–396. doi: 10.1016/j.biortech.2016.03.094
Monje, O., Richards, J. T., Carver, J. A., Dimapilis, D. I., Levine, H. G., Dufour, N. F., et al. (2020). Hardware validation of the advanced plant habitat on ISS: canopy photosynthesis in reduced gravity. Front. Plant Sci. 11:673. doi: 10.3389/fpls.2020.00673
Mullins, E. A., Starks, C. M., Francois, J. A., Sael, L., Kihara, D., and Kappock, T. J. (2012). Formyl-coenzyme a (CoA):oxalate CoA-transferase from the acidophile Acetobacter aceti has a distinctive electrostatic surface and inherent acid stability. Protein Sci. 21, 686–696. doi: 10.1002/pro.2054
Musgrave, M. E., Kuang, A., and Matthews, S. W. (1997). Plant reproduction during spaceflight: importance of the gaseous environment. Planta 203, S177–S184. doi: 10.1007/PL00008107
Mykytczuk, N. C. S., Trevors, J. T., Ferroni, G. D., and Leduc, L. G. (2010). Cytoplasmic membrane fluidity and fatty acid composition of Acidithiobacillus ferrooxidans in response to pH stress. Extremophiles 14, 427–441. doi: 10.1007/s00792-010-0319-2
Mykytczuk, N. C. S., Trevors, J. T., Leduc, L. G., and Ferroni, G. D. (2007). Fluorescence polarization in studies of bacterial cytoplasmic membrane fluidity under environmental stress. Prog. Biophys. Mol. Biol. 95, 60–82. doi: 10.1016/j.pbiomolbio.2007.05.001
Naganuma, T., and Uematsu, H. (1998). Dive Europa: a search-for-life initiative. Biol. Sci. Space 12, 126–130. doi: 10.2187/bss.12.126
Nakashima, M., Yamagami, R., Tomikawa, C., Ochi, Y., Moriya, T., Asahara, H., et al. (2017). Long and branched polyamines are required for maintenance of the ribosome, tRNAHis and tRNATyr in Thermus thermophilus cells at high temperatures. Genes Cells 22, 628–645. doi: 10.1111/gtc.12502
National Research Council, Division on Engineering and Physical Sciences. (2002). Space studies board, aeronautics and Space Engineering Board and Committee on precursor measurements necessary to support human operations on the surface of Mars. Safe on Mars: Precursor measurements necessary to support human operations on the Martian surface. Washington, DC: National Academies Press.
Newsome, L., Morris, K., and Lloyd, J. R. (2014). The biogeochemistry and bioremediation of uranium and other priority radionuclides. Chem. Geol. 363, 164–184. doi: 10.1016/j.chemgeo.2013.10.034
Nhung, N. T. H., Nguyen, X.-T. T., Long, V. D., Wei, Y., and Fujita, T. (2022). A review of soil contaminated with dioxins and biodegradation technologies: current status and future prospects. Toxics 10:278. doi: 10.3390/toxics10060278
Nicholson, W. L., Fajardo-Cavazos, P., Fedenko, J., Ortíz-Lugo, J.́. L., Rivas-Castillo, A., Waters, S. M., et al. (2010). Exploring the low-pressure growth limit: evolution of Bacillus subtilis in the laboratory to enhanced growth at 5 kilopascals. Appl. Environ. Microbiol. 76, 7559–7565. doi: 10.1128/AEM.01126-10
Noirungsee, N., Changkhong, S., Phinyo, K., Suwannajak, C., Tanakul, N., and Inwongwan, S. (2024). Genome-scale metabolic modelling of extremophiles and its applications in astrobiological environments. Environ. Microbiol. Rep. 16:e13231. doi: 10.1111/1758-2229.13231
Pan, J., Wang, J., Zhou, Z., Yan, Y., Zhang, W., Lu, W., et al. (2009). IrrE, a global regulator of extreme radiation resistance in Deinococcus radiodurans, enhances salt tolerance in Escherichia coli and Brassica napus. PLoS One 4:e4422. doi: 10.1371/journal.pone.0004422
Pandey, A., Dhakar, K., Sharma, A., Priti, P., Sati, P., and Kumar, B. (2014). Thermophilic bacteria that tolerate a wide temperature and pH range colonize the Soldhar (95 °C) and Ringigad (80 °C) hot springs of Uttarakhand, India. Ann. Microbiol. 65, 809–816. doi: 10.1007/s13213-014-0921-0
Papiran, R., and Hamedi, J. (2021). Adaptive evolution of Lactococcus lactis to thermal and oxidative stress increases biomass and Nisin production. Appl. Biochem. Biotechnol. 193, 3425–3441. doi: 10.1007/s12010-021-03609-6
Patel, M. R., Bérces, A., Kerékgyárto, T., Rontó, G., Lammer, H., and Zarnecki, J. C. (2004). Annual solar UV exposure and biological effective dose rates on the Martian surface. Adv. Space Res. 33, 1247–1252. doi: 10.1016/j.asr.2003.08.036
PHL. UPR Arecibo - habitable worlds catalog. Available at: https://phl.upr.edu/hwc.
Pokorny, N. J., Boulter-Bitzer, J. I., Hart, M. M., Storey, L., Lee, H., and Trevors, J. T. (2005). Hypobaric bacteriology: growth, cytoplasmic membrane polarization and total cellular fatty acids in Escherichia coli and Bacillus subtilis. Int. J. Astrobiol. 4, 187–193. doi: 10.1017/S1473550405002727
Prakash, O., Nimonkar, Y., and Shouche, Y. S. (2013). Practice and prospects of microbial preservation. FEMS Microbiol. Lett. 339, 1–9. doi: 10.1111/1574-6968.12034
Preiss, L., Hicks, D. B., Suzuki, S., Meier, T., and Krulwich, T. A. (2015). Alkaliphilic Bacteria with impact on industrial applications, concepts of early life forms, and bioenergetics of ATP synthesis. Front. Bioeng. Biotechnol. 3:75. doi: 10.3389/fbioe.2015.00075
Puig, J., Knödlseder, N., Quera, J., Algara, M., and Güell, M. (2021). DNA damage protection for enhanced bacterial survival under simulated low earth orbit environmental conditions in Escherichia coli. Front. Microbiol. 12:789668. doi: 10.3389/fmicb.2021.789668
Puja, H., Mislin, G. L. A., and Rigouin, C. (2023). Engineering Siderophore biosynthesis and regulation pathways to increase diversity and availability. Biomol. Ther. 13:959. doi: 10.3390/biom13060959
Reichard, J. F., Dalton, T. P., Shertzer, H. G., and Puga, A. (2006). Induction of oxidative stress responses by dioxin and other ligands of the aryl hydrocarbon receptor. Dose Response 3, 306–331. doi: 10.2203/dose-response.003.03.003
Roncarati, D., and Scarlato, V. (2017). Regulation of heat-shock genes in bacteria: from signal sensing to gene expression output. FEMS Microbiol. Rev. 41, 549–574. doi: 10.1093/femsre/fux015
Roumagnac, M., Pradel, N., Bartoli, M., Garel, M., Jones, A. A., Armougom, F., et al. (2020). Responses to the hydrostatic pressure of surface and subsurface strains of Pseudothermotoga elfii revealing the Piezophilic nature of the strain originating from an oil-producing well. Front. Microbiol. 11:588771. doi: 10.3389/fmicb.2020.588771
Rzymski, P., Poniedziałek, B., Hippmann, N., and Kaczmarek, Ł. (2022). Screening the survival of Cyanobacteria under perchlorate stress. Potential implications for Mars in situ resource utilization. Astrobiology 22, 672–684. doi: 10.1089/ast.2021.0100
Saldivar, A., Ruiz-Ruiz, P., Revah, S., and Zuñiga, C. (2024). Genome-scale flux balance analysis reveals redox trade-offs in the metabolism of the thermoacidophile Methylacidiphilum fumariolicum under auto-, hetero-and methanotrophic conditions. Front. Syst. Biol. 4:1612. doi: 10.3389/fsysb.2024.1291612
Sardessai, Y. N., and Bhosle, S. (2004). Industrial potential of organic solvent tolerant bacteria. Biotechnol. Prog. 20, 655–660. doi: 10.1021/bp0200595
Scales, N. C., Huynh, K. T., Weihe, C., and Martiny, J. B. H. (2023). Desiccation induces varied responses within a soil bacterial genus. Environ. Microbiol. 25, 3075–3086. doi: 10.1111/1462-2920.16494
Schalck, T., Van den Bergh, B., and Michiels, J. (2021). Increasing solvent tolerance to improve microbial production of alcohols, Terpenoids and aromatics. Microorganisms 9:249. doi: 10.3390/microorganisms9020249
Scherer, P., Neumann, L., Demirel, B., Schmidt, O., and Unbehauen, M. (2009). Long term fermentation studies about the nutritional requirements for biogasification of fodder beet silage as mono-substrate. Biomass Bioenergy 33, 873–881. doi: 10.1016/j.biombioe.2009.01.011
Schwendner, P., and Schuerger, A. C. (2020). Exploring microbial activity in low-pressure environments. Curr. Issues Mol. Biol. 38, 163–196. doi: 10.21775/cimb.038.163
Seager, S., Petkowski, J. J., Gao, P., Bains, W., Bryan, N. C., Ranjan, S., et al. (2021). The Venusian lower atmosphere haze as a depot for desiccated microbial life: a proposed life cycle for persistence of the Venusian aerial biosphere. Astrobiology 21, 1206–1223. doi: 10.1089/ast.2020.2244
Sévin, D. C., Stählin, J. N., Pollak, G. R., Kuehne, A., and Sauer, U. (2016). Global metabolic responses to salt stress in fifteen species. PLoS One 11:e0148888. doi: 10.1371/journal.pone.0148888
Shang, Y., Duan, Z., Huang, W., Gao, Q., and Wang, C. (2012). Improving UV resistance and virulence of Beauveria bassiana by genetic engineering with an exogenous tyrosinase gene. J. Invertebr. Pathol. 109, 105–109. doi: 10.1016/j.jip.2011.10.004
Sharma, G., and Curtis, P. D. (2022). The impacts of microgravity on bacterial metabolism. Life 12:774. doi: 10.3390/life12060774
Sharma, A., Gaidamakova, E. K., Grichenko, O., Matrosova, V. Y., Hoeke, V., Klimenkova, P., et al. (2017). Across the tree of life, radiation resistance is governed by antioxidant Mn2+, gauged by paramagnetic resonance. Proc. Natl. Acad. Sci. USA 114, E9253–E9260. doi: 10.1073/pnas.1713608114
Shukla, M., Chaturvedi, R., Tamhane, D., Vyas, P., Archana, G., Apte, S., et al. (2007). Multiple-stress tolerance of ionizing radiation-resistant bacterial isolates obtained from various habitats: correlation between stresses. Curr. Microbiol. 54, 142–148. doi: 10.1007/s00284-006-0311-3
Slade, D., and Radman, M. (2011). Oxidative stress resistance in Deinococcus radiodurans. Microbiol. Mol. Biol. Rev. 75, 133–191. doi: 10.1128/MMBR.00015-10
Soliman, G. S. H., and Trüper, H. G. (1982). Halobacterium pharaonis sp. nov., a new, extremely Haloalkaliphilic Archaebacterium with low magnesium requirement. Zentralbl. Bakteriol. Mikrobiol. Hyg. 3, 318–329. doi: 10.1016/S0721-9571(82)80045-8
Solomon, J. B., Lee, C. C., Jasniewski, A. J., Rasekh, M. F., Ribbe, M. W., and Hu, Y. (2020). Heterologous expression and engineering of the Nitrogenase cofactor biosynthesis scaffold NifEN. Angew. Chem. Int. Ed. Engl. 59, 6887–6893. doi: 10.1002/anie.201916598
Soto, A., Allona, I., Collada, C., Guevara, M. A., Casado, R., Rodriguez-Cerezo, E., et al. (1999). Heterologous expression of a plant small heat-shock protein enhances Escherichia coli viability under heat and cold stress. Plant Physiol. 120, 521–528. doi: 10.1104/pp.120.2.521
Sturr, M. G., Guffanti, A. A., and Krulwich, T. A. (1994). Growth and bioenergetics of alkaliphilic Bacillus firmus OF4 in continuous culture at high pH. J. Bacteriol. 176, 3111–3116. doi: 10.1128/jb.176.11.3111-3116.1994
Sun, J., He, X., Le, Y., Al-Tohamy, R., and Ali, S. S. (2024). Potential applications of extremophilic bacteria in the bioremediation of extreme environments contaminated with heavy metals. J. Environ. Manag. 352:120081. doi: 10.1016/j.jenvman.2024.120081
Swayambhu, G., Moscatello, N., Atilla-Gokcumen, G. E., and Pfeifer, B. A. (2020). Flux balance analysis for media optimization and genetic targets to improve heterologous Siderophore production. iScience 23:101016. doi: 10.1016/j.isci.2020.101016
Syu, M. J. (2001). Biological production of 2,3-butanediol. Appl. Microbiol. Biotechnol. 55, 10–18. doi: 10.1007/s002530000486
Tachikawa, S., Nagano, H., Ohnishi, A., and Nagasaka, Y. (2022). Advanced passive thermal control materials and devices for spacecraft: a review. Int. J. Thermophys. 43:91. doi: 10.1007/s10765-022-03010-3
Takimoto, R., Tatemichi, Y., Aoki, W., Kosaka, Y., Minakuchi, H., Ueda, M., et al. (2022). A critical role of an oxygen-responsive gene for aerobic nitrogenase activity in Azotobacter vinelandii and its application to Escherichia coli. Sci. Rep. 12:4182. doi: 10.1038/s41598-022-08007-4
Tan, D., Xue, Y.-S., Aibaidula, G., and Chen, G.-Q. (2011). Unsterile and continuous production of polyhydroxybutyrate by Halomonas TD01. Bioresour. Technol. 102, 8130–8136. doi: 10.1016/j.biortech.2011.05.068
Tanghe, A., Van Dijck, P., Dumortier, F., Teunissen, A., Hohmann, S., and Thevelein, J. M. (2002). Aquaporin expression correlates with freeze tolerance in baker’s yeast, and overexpression improves freeze tolerance in industrial strains. Appl. Environ. Microbiol. 68, 5981–5989. doi: 10.1128/AEM.68.12.5981-5989.2002
Tatemichi, Y., Nakahara, T., Ueda, M., and Kuroda, K. (2021). Construction of recombinant Escherichia coli producing nitrogenase-related proteins from Azotobacter vinelandii. Biosci. Biotechnol. Biochem. 85, 2209–2216. doi: 10.1093/bbb/zbab144
Terui, Y., Ohnuma, M., Hiraga, K., Kawashima, E., and Oshima, T. (2005). Stabilization of nucleic acids by unusual polyamines produced by an extreme thermophile, Thermus thermophilus. Biochem. J. 388, 427–433. doi: 10.1042/BJ20041778
Teunissen, A., Dumortier, F.ṃ., Gorwa, M. F., Bauer, J., Tanghe, A., Loïez, A., et al. (2002). Isolation and characterization of a freeze-tolerant diploid derivative of an industrial baker’s yeast strain and its use in frozen doughs. Appl. Environ. Microbiol. 68, 4780–4787. doi: 10.1128/AEM.68.10.4780-4787.2002
Tindall, B. J., Mills, A. A., and Grant, W. D. (1980). An alkalophilic red halophilic bacterium with a low magnesium requirement from a Kenyan soda lake. Microbiology 116, 257–260. doi: 10.1099/00221287-116-1-257
Tosca, N. J., Knoll, A. H., and McLennan, S. M. (2008). Water activity and the challenge for life on early Mars. Science 320, 1204–1207. doi: 10.1126/science.1155432
Trumbo, S. K., Brown, M. E., and Hand, K. P. (2019). Sodium chloride on the surface of Europa. Sci. Adv. 5:eaaw7123. doi: 10.1126/sciadv.aaw7123
Tymczyszyn, E. E., Gómez-Zavaglia, A., and Disalvo, E. A. (2005). Influence of the growth at high osmolality on the lipid composition, water permeability and osmotic response of Lactobacillus bulgaricus. Arch. Biochem. Biophys. 443, 66–73. doi: 10.1016/j.abb.2005.09.004
Ujaoney, A. K., Padwal, M. K., and Basu, B. (2017). Proteome dynamics during post-desiccation recovery reveal convergence of desiccation and gamma radiation stress response pathways in Deinococcus radiodurans. Biochim. Biophys. Acta, Proteins Proteomics 1865, 1215–1226. doi: 10.1016/j.bbapap.2017.06.014
Upchurch, R. G., Meade, M. J., Hightower, R. C., Thomas, R. S., and Callahan, T. M. (1994). Transformation of the fungal soybean pathogen Cercospora kikuchii with the selectable marker bar. Appl. Environ. Microbiol. 60, 4592–4595. doi: 10.1128/aem.60.12.4592-4595.1994
Vahdani, F., Ghafouri, H., Sarikhan, S., and Khodarahmi, R. (2019). Molecular cloning, expression, and functional characterization of 70-kDa heat shock protein, DnaK, from Bacillus halodurans. Int. J. Biol. Macromol. 137, 151–159. doi: 10.1016/j.ijbiomac.2019.06.217
van Dijk, M., Mierke, F., Nygård, Y., and Olsson, L. (2020). Nutrient-supplemented propagation of Saccharomyces cerevisiae improves its lignocellulose fermentation ability. AMB Express 10:157. doi: 10.1186/s13568-020-01070-y
Van Hecke, W., Bockrath, R., and De Wever, H. (2019). Effects of moderately elevated pressure on gas fermentation processes. Bioresour. Technol. 293:122129. doi: 10.1016/j.biortech.2019.122129
Van Vuuren, D. P., Bouwman, A. F., and Beusen, A. H. W. (2010). Phosphorus demand for the 1970–2100 period: a scenario analysis of resource depletion. Glob. Environ. Change 20, 428–439. doi: 10.1016/j.gloenvcha.2010.04.004
Varize, C. S., Bücker, A., Lopes, L. D., Christofoleti-Furlan, R. M., Raposo, M. S., Basso, L. C., et al. (2022). Increasing ethanol tolerance and ethanol production in an industrial fuel ethanol Saccharomyces cerevisiae strain. Fermentation 8:470. doi: 10.3390/fermentation8100470
Velichko, A. K., Petrova, N. V., Kantidze, O. L., and Razin, S. V. (2012). Dual effect of heat shock on DNA replication and genome integrity. Mol. Biol. Cell 23, 3450–3460. doi: 10.1091/mbc.e11-12-1009
Vera-Bernal, M., and Martínez-Espinosa, R. M. (2021). Insights on cadmium removal by bioremediation: the case of Haloarchaea. Microbiol. Res. 12, 354–375. doi: 10.3390/microbiolres12020024
Verseux, C., Heinicke, C., Ramalho, T. P., Determann, J., Duckhorn, M., Smagin, M., et al. (2021). A low-pressure, N2/CO2 atmosphere is suitable for cyanobacterium-based life-support systems on Mars. Front. Microbiol. 12:611798. doi: 10.3389/fmicb.2021.611798
Vilchez, S., and Manzanera, M. (2011). Biotechnological uses of desiccation-tolerant microorganisms for the rhizoremediation of soils subjected to seasonal drought. Appl. Microbiol. Biotechnol. 91, 1297–1304. doi: 10.1007/s00253-011-3461-6
Vimercati, L., Hamsher, S., Schubert, Z., and Schmidt, S. K. (2016). Growth of high-elevation Cryptococcus sp. during extreme freeze–thaw cycles. Extremophiles 20, 579–588. doi: 10.1007/s00792-016-0844-8
Von Arx, A. V., and Delgado, A. (1991). Convective heat transfer on Mars. Maryland: American Institute of Physics.
Vroom, M. M., Troncoso-Garcia, A., Duscher, A. A., and Foster, J. S. (2022). Modeled microgravity alters apoptotic gene expression and caspase activity in the squid-vibrio symbiosis. BMC Microbiol. 22:202. doi: 10.1186/s12866-022-02614-x
Wadhwa, M., Carrier, B., Goreva, Y., and Thiessen, F. (2022). Sample science integrity considerations for the Mars sample return program. 44th COSPAR Scientific Assembly, No. 44.
Wagh, J., Shah, S., Bhandari, P., Archana, G., and Kumar, G. N. (2014). Heterologous expression of pyrroloquinoline quinone (pqq) gene cluster confers mineral phosphate solubilization ability to Herbaspirillum seropedicae Z67. Appl. Microbiol. Biotechnol. 98, 5117–5129. doi: 10.1007/s00253-014-5610-1
Wang, Y., Hu, B., du, S., Gao, S., Chen, X., and Chen, D. (2016). Proteomic analyses reveal the mechanism of Dunaliella salina ds-26-16 gene enhancing salt tolerance in Escherichia coli. PLoS One 11:e0153640. doi: 10.1371/journal.pone.0153640
Wang, X., Liang, Z., Hou, J., Shen, Y., and Bao, X. (2017). The absence of the transcription factor Yrr1p, identified from comparative genome profiling, increased vanillin tolerance due to enhancements of ABC transporters expressing, rRNA processing and ribosome biogenesis in Saccharomyces cerevisiae. Front. Microbiol. 8:367. doi: 10.3389/fmicb.2017.00367
Wang, L., Wang, X., He, Z. Q., Zhou, S. J., Xu, L., Tan, X. Y., et al. (2020). Engineering prokaryotic regulator IrrE to enhance stress tolerance in budding yeast. Biotechnol. Biofuels 13:193. doi: 10.1186/s13068-020-01833-6
Waters, S. M., Robles-Martínez, J. A., and Nicholson, W. L. (2021). Growth at 5 kPa causes differential expression of a number of signals in a Bacillus subtilis strain adapted to enhanced growth at low pressure. Astrobiology 21, 1076–1088. doi: 10.1089/ast.2020.2389
Waters, S. M., Zeigler, D. R., and Nicholson, W. L. (2015). Experimental evolution of enhanced growth by Bacillus subtilis at low atmospheric pressure: genomic changes revealed by whole-genome sequencing. Appl. Environ. Microbiol. 81, 7525–7532. doi: 10.1128/AEM.01690-15
West, S. J., Frant, M. S., Wen, X., Geis, R., Herdan, J., Gillette, T., et al. (1999). Electrochemistry on Mars. Am. Lab. 31, 48–54
Widrlechner, M. P., Daly, C., Keller, M., and Kaplan, K. (2012). Horticultural applications of a newly revised USDA Plant hardiness zone map. HortTechnology 22, 6–19. doi: 10.21273/HORTTECH.22.1.6
Wiegel, J. (1990). Temperature spans for growth: hypothesis and discussion. FEMS Microbiol. Lett. 75, 155–169. doi: 10.1111/j.1574-6968.1990.tb04092.x
Wiegel, J., and Kevbrin, V. V. (2004). Alkalithermophiles. Biochem. Soc. Trans. 32, 193–198. doi: 10.1042/bst0320193
Williamson, M. P., and Kitahara, R. (2019). Characterization of low-lying excited states of proteins by high-pressure NMR. Biochim. Biophys. Acta, Proteins Proteomics 1867, 350–358. doi: 10.1016/j.bbapap.2018.10.014
Wilson, S. L., Frazer, C., Cumming, B. F., Nuin, P. A. S., and Walker, V. K. (2012). Cross-tolerance between osmotic and freeze-thaw stress in microbial assemblages from temperate lakes. FEMS Microbiol. Ecol. 82, 405–415. doi: 10.1111/j.1574-6941.2012.01404.x
Wordsworth, R., Kerber, L., and Cockell, C. (2019). Enabling Martian habitability with silica aerogel via the solid-state greenhouse effect. Nat. Astron. 3, 898–903. doi: 10.1038/s41550-019-0813-0
Xiao, Y., Xiao, C., and Zhao, F. (2019). Long-term adaptive evolution of Shewanella oneidensis MR-1 for establishment of high concentration Cr(VI) tolerance. Front. Environ. Sci. Eng. China 14:3. doi: 10.1007/s11783-019-1182-8
Xue, Y., Braslavsky, I., and Quake, S. R. (2021). Temperature effect on polymerase fidelity. J. Biol. Chem. 297:101270. doi: 10.1016/j.jbc.2021.101270
Ye, J.-W., Lin, Y. N., Yi, X. Q., Yu, Z. X., Liu, X., and Chen, G. Q. (2023). Synthetic biology of extremophiles: a new wave of biomanufacturing. Trends Biotechnol. 41, 342–357. doi: 10.1016/j.tibtech.2022.11.010
Yue, H., Ling, C., Yang, T., Chen, X., Chen, Y., Deng, H., et al. (2014). A seawater-based open and continuous process for polyhydroxyalkanoates production by recombinant Halomonas campaniensis LS21 grown in mixed substrates. Biotechnol. Biofuels 7, 1–12. doi: 10.1186/1754-6834-7-108
Zea, L., Larsen, M., Estante, F., Qvortrup, K., Moeller, R., Dias de Oliveira, S., et al. (2017). Phenotypic changes exhibited by E. coli cultured in space. Front. Microbiol. 8:1598. doi: 10.3389/fmicb.2017.01598
Zea, L., Prasad, N., Levy, S. E., Stodieck, L., Jones, A., Shrestha, S., et al. (2016). A molecular genetic basis explaining altered bacterial behavior in space. PLoS One 11:e0164359. doi: 10.1371/journal.pone.0164359
Zhang, Y., and Cremer, P. S. (2006). Interactions between macromolecules and ions: the Hofmeister series. Curr. Opin. Chem. Biol. 10, 658–663. doi: 10.1016/j.cbpa.2006.09.020
Zhang, X., Cvetkovska, M., Morgan-Kiss, R., Hüner, N. P. A., and Smith, D. R. (2020). Is gene duplication driving cold adaptation in the Antarctic green alga Chlamydomonas Sp. UWO241? iScience 24:102084. doi: 10.2139/ssrn.3732378
Zhang, Y., and Gross, C. A. (2021). Cold shock response in Bacteria. Annu. Rev. Genet. 55, 377–400. doi: 10.1146/annurev-genet-071819-031654
Zhou, Z., Tang, H., Wang, W., Zhang, L., Su, F., Wu, Y., et al. (2021). A cold shock protein promotes high-temperature microbial growth through binding to diverse RNA species. Cell Discov. 7:15. doi: 10.1038/s41421-021-00246-5
Keywords: extremophile, directed evolution, functional genomics, ISRU, biomanufacturing
Citation: Caro-Astorga J, Meyerowitz JT, Stork DA, Nattermann U, Piszkiewicz S, Vimercati L, Schwendner P, Hocher A, Cockell C and DeBenedictis E (2024) Polyextremophile engineering: a review of organisms that push the limits of life. Front. Microbiol. 15:1341701. doi: 10.3389/fmicb.2024.1341701
Edited by:
Mohamed Jebbar, Université de Bretagne Occidentale, FranceReviewed by:
Francesco Venice, National Research Council (CNR), ItalyNancy Merino, Lawrence Livermore National Laboratory (DOE), United States
Copyright © 2024 Caro-Astorga, Meyerowitz, Stork, Nattermann, Piszkiewicz, Vimercati, Schwendner, Hocher, Cockell and DeBenedictis. This is an open-access article distributed under the terms of the Creative Commons Attribution License (CC BY). The use, distribution or reproduction in other forums is permitted, provided the original author(s) and the copyright owner(s) are credited and that the original publication in this journal is cited, in accordance with accepted academic practice. No use, distribution or reproduction is permitted which does not comply with these terms.
*Correspondence: Erika DeBenedictis, ZXJpa2FAcGlvbmVlci1sYWJzLm9yZw==