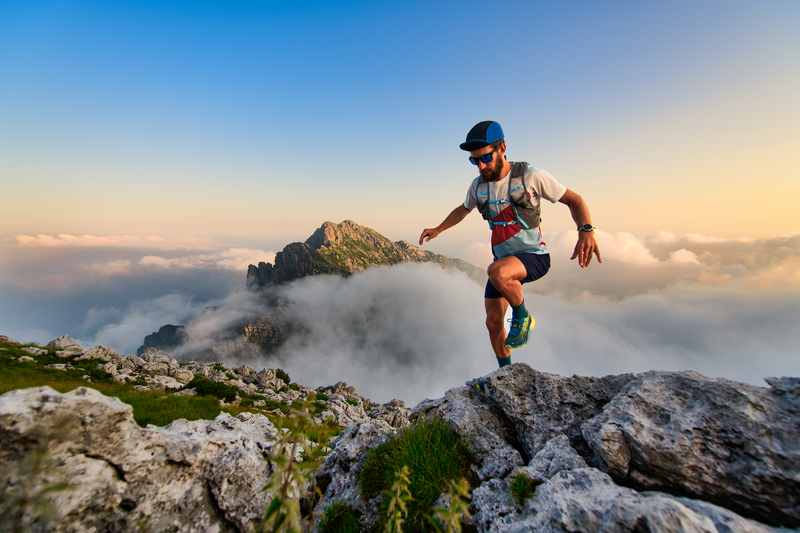
95% of researchers rate our articles as excellent or good
Learn more about the work of our research integrity team to safeguard the quality of each article we publish.
Find out more
REVIEW article
Front. Microbiol. , 14 February 2024
Sec. Microbiotechnology
Volume 15 - 2024 | https://doi.org/10.3389/fmicb.2024.1339469
This article is part of the Research Topic Pharmaceutically Active Micropollutants – How Serious is the Problem and is there a Microbial Way Out? View all 6 articles
Pharmaceuticals, recognized for their life-saving potential, have emerged as a concerning class of micropollutants in the environment. Even at minute concentrations, chronic exposure poses a significant threat to ecosystems. Various pharmaceutically active micropollutants (PhAMP), including antibiotics, analgesics, and hormones, have been detected in underground waters, surface waters, seawater, sewage treatment plants, soils, and activated sludges due to the absence of standardized regulations on pharmaceutical discharge. Prolonged exposureof hospital waste and sewage treatment facilities is linked to the presence of antibiotic-resistant bacteria. Conventional water treatment methods prove ineffective, prompting the use of alternative techniques like photolysis, reverse osmosis, UV-degradation, bio-degradation, and nano-filtration. However, commercial implementation faces challenges such as incomplete removal, toxic sludge generation, high costs, and the need for skilled personnel. Research gaps include the need to comprehensively identify and understand various types of pharmaceutically active micropollutants, investigate their long-term ecological impact, develop more sensitive monitoring techniques, and explore integrated treatment approaches. Additionally, there is a gap in understanding the socio-economic implications of pharmaceutical pollution and the efficacy of public awareness campaigns. Future research should delve into alternative strategies like phagotherapy, vaccines, and natural substance substitutes to address the escalating threat of pharmaceutical pollution.
Pharmaceuticals, considered one of the greatest milestones in development of science for mankind, have expanded the life spans, cured many from lethal ailments and greatly refined the standards of our lives. This milestone has become a serious environmental threat, and pharmaceuticals are now considered rapidly growing environmental pollutants (Patel et al., 2019). Since past three decades, it has been found that pharmaceutical residues are present in almost all ecological bodies, such as surface water (lake, river, stream and water from sea), under groundwater, water from treatment plant, effluents and influents (Hanif et al., 2020).
Pharmaceutically active micropollutants (PhAMPs) are used in agricultural, medicinal and biotechnological field. These substances include hormones, antibiotics and drugs. Major sources of PhAMPs in the environment come from human and veterinary applications and other sources are the excretion and release into the surroundings by sewage treatment plants (STPs; El-Fattah et al., 2023).
The global usage of pharmaceutically active compound (PhACs) has been estimated to approximately 100,000 tons and beyond per year (Jayasiri et al., 2013). Some of these PhACs are employed immensely as non-prescriptive medications and after consumption, they get excreted with urinal discharge and feces as metabolites. Global consumption of antibiotics has surged with the rate of 30 percent in the last 20 years, more than half of which is due to inappropriate use, resulting in large amounts getting released into our ecosystem (Kraemer et al., 2019). Prescence of PhACs in ecosystems in higher concentration contributed to health risks for land as well as water ecosystem including humans.
Present setup of Waste-Water Treatment Plants (WWTPs) are not fabricated in a way to eliminate such pollutants. This is because pharmaceuticals have poor biodegradability and good hydrophilicity, it becomes difficult to get-rid of them from water using old techniques for wastewater treatment. Also, they interact with micro- plastics often present in water at the surface which act as a carrier of pharmaceuticals in water system via π–π interaction, due to their hydrophobic/hydrophilic nature (Koelmans et al., 2022). The usual processes employed in treating waste- water (from municipal sources) cannot remove these micro level pollutants. This results into mixing of the unused pharmaceuticals into surface water, which leads to negative impact on the aquatic ecosystem and may also hinder with the production of drinking water (Gao et al., 2021; Petrović et al., 2021; Daughton and Ternes, 2022; Pedersen and Pedersen, 2022; Jin et al., 2023; Li et al., 2023).
As the traditional treatment methods are not capable enough for the treatment and elimination of the micropollutants, other substitutive methods can be employed such as coagulation – flocculation, adsorption (on activated carbon), oxidation, filtration (membrane bioreactor), use of biocatalysts (isolated or as whole organisms like bacteria, fungi and algae) (Silva et al., 2019) and nanomaterials can help curbing the problem effectively (Bhuyan and Ahmaruzzaman, 2023; Sanjeev et al., 2023; Sigonya et al., 2023).
One of the important reasons, why there has been great concern regarding pharmaceutical products and compounds, is that they bring out biological effects. They are fabricated in a very stable ways, so that they can be stocked for long durations and readily consumed. They are lipophilic in nature so as to cross the host membrane, and to reach the site of action, especially the ones consumed via oral route, they must have resistance toward enzymes and must not be hydrolysable at lower pH and must be highly mobile in liquid system (Mert et al., 2018; Mauro et al., 2021).
By virtue of such characteristics, pharmaceutical compounds get bio-accumulated, making them persistent and recalcitrant in nature and may adversely affect our ecosystem (aquatic as well as terrestrial). It has also been reported that PhAMPs can also enter food chains.
PhAMPs are usually present in water bodies in very trace amounts, in ranges of ngL−1 to μgL−1. Presence of PhAMPs in trace amounts and their heterogeneity convolute the detection and examination processes and pose hurdles for water treatment plants. Major sources of PhAMPs are shown in Table 1 and Figure 1.
Table 1. Categorization of pharmaceutically active micropollutants and their sources in the water bodies.
The excessive and improper prescription of antibiotics in human health and farming are associated with the abundance of antibiotics in the surroundings, especially in aquatic systems (Majumder et al., 2020). These actions result in the release of antimicrobials and/or in the form of metabolites in water bodies. This ultimately contributes toward surging antimicrobial resistance in microbes, by obtaining genes from the reservoir of Antibiotic Resistance Genes (ARGs) leading to their fast growth. It has been found that aquatic environments (primary habitats) and treatment plants act as a point for discharging a large chunk of pharmaceutical substances. Consequently, bacteria get optimal conditions where they develop and spread antibiotic resistance leading to the spread of ARGs. In this way, antibiotics and ARGs disseminate and get circulated between humans, fauna, and the environment. The secondary habitats include environments such as hospitals, nursing homes (where susceptible individuals with exposure to bacterial genetic exchange are present) and animal farms. The used pharmaceuticals or their metabolites from secondary habitats enter into wastewater treatment plants, where they get indulged with microbes (Table 2).
The sewage treatment centers being the tertiary niches, offer ideal environment for possible blending and genetic interchange between pharmaceutical compounds and the bacteria (Mutuku et al., 2022). Soil, sediments, surface and underground water provide the ultimate habitat wherein microbes from other habitats mingle and get associated with the larger bacterial groups in the existing habitat. This results in the interlinking of these niches, generating a point for breeding bacteria (antibiotic resistant) and ARGs, flowing in the environment and with time can re-enter into the terrestrial bodies. The regulation of the introduction of pharmaceutically active micropollutants into these habitats can be achieved by pre-treating hospital waste and creating awareness on consequences of inappropriate usage of anti-microbial agents.
Studies have suggested that environments which are exposed to very high levels of antibiotic contamination show increased antibiotic resistance indicators (Tiwari et al., 2017). This can be well understood by an example of a study, wherein it was found that the ciprofloxacin concentration was present 1,000 times more than the inhibitory concentration for certain bacteria in the wastewater coming from a pharmaceutical manufacturing unit. It was also reported that there were more ARGs in the water going inside the Waste Water Treatment Plants (WWTPs) than the water coming outside. Another study reported multidrug-resistant bacteria isolated at the downstream of a river receiving oxytetracycline waste (Mutuku et al., 2022).
These findings have now clearly established the association between the plethora of ARGs and anti-microbial contamination in the environment and necessitates need for advancement in understanding the complex process and interactions involved in antibiotic resistance emerging from antibiotic contamination in the surroundings (Table 3).
The pharmaceutical industry continually introduces a significant number of pharmaceutical compounds to the market, and despite the fact that less than 2 percent have been detected in the environment, the production and release of pharmaceutically active micropollutants (PhAMPs) have increased alarmingly in recent years (Rovira-Alsina and Pijuan, 2018). This surge in PhAMPs is attributed to several factors, including the expansion of pharmaceutical usage, the persistence of these compounds in the environment, and the lack of effective regulatory measures to monitor and control their release (Bilal et al., 2022; Sharma et al., 2023).
Efforts to anticipate the concentration of PhAMPs in the environment involve intricate studies that consider various factors such as consumption rates, pharmaceutical usage patterns, the efficiency of wastewater treatment plants (WWTPs), and regulatory frameworks (Rovira-Alsina and Pijuan, 2018; Bilal et al., 2022; Sharma et al., 2023). However, predicting the precise environmental fate of PhAMPs remains challenging due to the complexity of their pathways and the influence of diverse environmental variables.
Adding complexity to the issue is the phenomenon of pharmaceutical metabolism, where a significant portion of the parent compounds undergoes transformation into metabolites known as transformation products (TPs). These TPs, often hydroxylated or conjugated forms, possess the potential to induce varied physiological responses and negative effects. Importantly, these metabolites persist and are released into untreated water at treatment plants, contributing to the overall environmental load of pharmaceuticals (Santos et al., 2015; Weber et al., 2021).
While traditional processing methods in WWTPs contribute to the natural inactivation of PhAMPs, they fall short of achieving complete removal for the majority of these compounds. This inadequacy has become a primary factor contributing to the discharge of micropollutants into surface water, leading to concerns about their impact on aquatic ecosystems (Li et al., 2022; Zhang et al., 2023).
To comprehensively address the environmental impact of PhAMPs, ongoing research is crucial. Researchers aim to unravel the intricate interplay between the production, release, and environmental fate of PhAMPs. This multidisciplinary approach encompasses studies on pharmacokinetics, environmental monitoring, and the development of advanced wastewater treatment strategies. Moreover, it facilitates the creation of more effective regulatory measures to monitor pharmaceutical production, usage, and disposal, ultimately mitigating the impact of PhAMPs on aquatic ecosystems. The evolving nature of this field underscores the necessity for continuous research to adapt strategies and regulations in response to the dynamic challenges posed by pharmaceutical pollution in aquatic environments.
In distinction with long-established wastewater treatment methods, recently developed methods for wastewater treatment, including membrane filtration, advanced oxidation processes (AOPs) activated carbon adsorption etc. have shown better removal potential for PhAMPs. Bio catalytic approaches are eco- friendly and sustainable substitutes offering lower energy inputs, newest reactions and producing few or nil harmful by-products than that generated by the traditional processes. Also, high enzyme specificity for their substrates reduces the possibility of undesirable reactions. Hence, using biocatalysts are said to be as an imminent choice to efficiently degrade micropollutants from water as well as wastewater (Table 4).
Traditional wastewater treatment methods often struggle to remove PhACs, necessitating the exploration of advanced treatment technologies. Physical methods have emerged as promising tools for selectively removing PhACs from water, offering various advantages and challenges.
Several common physical methods are employed for the removal of pharmaceuticals and personal care products (PhACs) from water sources. Ion Exchange (IX) utilizes specially designed resins with charged functional groups to attract and bind PhACs with opposite charges. This method is known for its high selectivity and efficiency for specific PhACs and can be regenerated for reuse, contributing to its sustainability (Gómez-Serrano et al., 2017; García-Galán et al., 2018; Zhang et al., 2019; Mansouri et al., 2021). Another widely used method is Adsorption, where materials like activated carbon are employed to attract and retain PhACs on their surface. This method is effective for removing a diverse range of PhACs, however, it may require frequent regeneration or replacement of the adsorbent material to maintain its efficacy (Benner et al., 2020; Sharma et al., 2022). Membrane Filtration involves the use of semipermeable membranes with specific pore sizes to physically separate PhACs from water based on their size, with varying degrees of selectivity and effectiveness offered by different membrane types such as microfiltration and nanofiltration (Dolar et al., 2019; Nguyen and Chen, 2023). Coagulation and Flocculation, on the other hand, induce the aggregation of PhACs into larger particles through the addition of chemicals, facilitating their removal by sedimentation or filtration. While effective for larger-molecule PhACs, this method may not be as suitable for smaller or more soluble ones (Yu et al., 2020; Zhang X. et al., 2023).
Physical methods exhibit high removal efficiency, ease of operation, and simpler implementation, making them suitable for various water treatment applications. For instance, ion exchange not only demonstrates high removal efficiency but also offers the potential for regeneration and reuse, minimizing waste generation and enhancing its environmental friendliness. These characteristics collectively underscore the suitability of physical methods for the efficient removal of PhACs in diverse water treatment scenarios.
Several challenges are associated with the removal of pharmaceuticals and personal care products (PhACs) from wastewater using physical methods. The broad spectrum of PhACs present in wastewater, each with different properties, complicates the design of a single physical method capable of effectively removing all types of contaminants. The diversity in chemical structures and properties of PhACs necessitates a nuanced approach to address their varied characteristics. Moreover, the cost implications are substantial, especially for large-scale applications, considering the expenses associated with the procurement of materials and regeneration chemicals. Another challenge arises from competing ions present in the water, which can hinder the effectiveness of physical methods by vying with PhACs for binding sites on adsorbents or membrane pores. This competition diminishes the overall efficiency of the removal process. Additionally, the management of spent materials generated from adsorption or filtration processes poses a logistical challenge. Due to their concentrated PhAC content, these spent materials may require special handling or disposal methods to ensure environmental safety and regulatory compliance. Addressing these challenges is crucial for advancing the practical implementation and efficiency of physical methods in treating wastewater contaminated with PhACs.
Recent research has been dedicated to enhancing the performance and sustainability of physical methods for the removal of PhACs. Key strategies in this pursuit involve the exploration of new materials, with a focus on novel adsorbents exhibiting higher affinity and selectivity for specific PhACs, thus improving overall removal efficiency (Chen et al., 2022; Xu et al., 2022; Huang et al., 2023). Additionally, there is a growing emphasis on combining different physical methods within a multi-stage treatment process to provide a more comprehensive approach that can effectively address the diverse range of PhACs present in water sources (Zhao et al., 2021; Wang et al., 2022; Zhang et al., 2023). Efforts are also directed toward improving the regeneration processes for adsorbents and resins, aiming for enhanced efficiency and environmental friendliness to reduce operational costs and waste generation. Another avenue of research focuses on advanced membrane technologies, investigating new membranes with improved selectivity and antifouling properties. These advancements aim to enhance the overall efficiency and applicability of membrane filtration for the removal of PhACs, contributing to the ongoing refinement of physical methods (Ahmad and Bhatti, 2021; Jiang et al., 2022; Du et al., 2023). Collectively, these research directions signify a commitment to advancing the capabilities of physical methods, aiming for higher performance, sustainability, and broader applicability in addressing the challenges posed by pharmaceutical and personal care pollutants in water treatment processes.
Physical methods offer valuable tools for removing PhACs from water, providing high removal efficiency, ease of operation, and potential for environmental sustainability. Ongoing research and development efforts aim to address the existing challenges and improve the performance of these methods to create a more comprehensive and sustainable approach to managing PhAC contamination in water resources. While physical methods can eliminate large-molecule organic pollutants and Produce high-quality water, they struggle with pharmaceuticals and personal care products (PhACs) due to their inherent water solubility. Only a few PhACs, like diclofenac with its higher polarity, are partially removed by physical means. To effectively address PhAC contamination, physical methods should be combined with other approaches. This requires integrating complementary techniques like chemical or organic removal or implementing waste disposal systems. Such synergistic strategies ensure a comprehensive and tailored approach to water purification, considering the unique challenges posed by PhACs and other micropollutants.
Common chemical methods include Advanced Oxidation Processes (AOPs), employing highly reactive species like hydroxyl radicals to oxidize and decompose PhACs. AOPs comprise ozonation, Fenton and photo-Fenton processes, and photocatalysis (Gao et al., 2020; Sharma et al., 2021; Zhang et al., 2023). Chlorination, utilizing chlorine as a disinfectant, offers another method, though its effectiveness varies for different PhACs and may lead to the formation of potentially harmful disinfection byproducts (He et al., 2019; Yang et al., 2022). Chemical precipitation involves adding chemicals to form insoluble precipitates encapsulating and removing PhACs, with effectiveness dependent on specific PhACs and chosen chemicals (Li et al., 2019; Zheng et al., 2020). Sonochemistry, utilizing high-frequency sound waves to generate cavitation bubbles, facilitates the violent collapse of these bubbles, creating intense localized heat and pressure that can degrade PhACs (Ghauch et al., 2020; Wang et al., 2022).
Chemical methods boast high efficiency, often exceeding 90% removal, and broad applicability to diverse water sources. They can be adapted for wastewater effluents, surface water, and groundwater treatment. Additionally, some chemical methods offer targeted degradation, selectively degrading specific PhACs while minimizing the impact on other water constituents.
However, challenges accompany these chemical methods, including the potential formation of harmful byproducts, high energy consumption for processes like AOPs and sonochemistry, and the need for careful chemical handling and disposal to ensure environmental safety. Residual toxicity of some degradation products necessitates additional treatment steps.
Recent research endeavors focus on enhancing the efficiency, selectivity, and sustainability of chemical methods for PhAC removal. These include the exploration of new catalysts for AOPs, investigation into selective degradation methods that target specific PhACs while minimizing impacts on other constituents, integration of chemical methods with other treatment technologies for a comprehensive approach, and the utilization of solar energy to drive AOPs, thereby reducing their energy footprint and enhancing sustainability (References). These ongoing efforts underscore a commitment to refining chemical methods for the removal of PhACs, ensuring both efficacy and environmental responsibility in water treatment practices. Chemical methods offer a promising approach for removing PhACs from water due to their high efficiency, broad applicability, and potential for targeted degradation. Ongoing research and development efforts aim to address the existing challenges and enhance the performance of these methods to create a more sustainable and efficient solution for PhAC contamination in water resources.
Biological methods for the removal of Pharmaceutically Active Micropollutants (PhAMPs) represent a sustainable and environmentally friendly approach to mitigate the presence of these emerging contaminants in water and wastewater. These methods harness the power of various microorganisms, such as bacteria, algae, and fungi, to biodegrade or transform PhAMPs into less harmful compounds. One of the primary biological techniques is biodegradation, where microorganisms metabolize the PhAMPs, breaking them down into simpler, non-toxic substances. In some cases, these microorganisms can be naturally occurring in wastewater treatment systems, or specific strains can be engineered for enhanced performance. Another promising approach involves the use of phytoremediation, where aquatic plants are utilized to absorb and accumulate PhAMPs, which can then be harvested or subjected to further treatment. Biological treatment methods, however, may have limitations, such as the selectivity of microorganisms and the need for specific conditions to optimize their performance. Nevertheless, they offer a sustainable solution for the removal of PhAMPs and are increasingly being integrated into water treatment strategies to safeguard water quality and the environment.
The utilization of fungal systems for biodegradation is emerging as a promising non-traditional approach in sewage treatment to eliminate micropollutants. These micropollutants encompass a variety of undesirable substances, such as pharmaceuticals and personal care products, as well as endocrine-disrupting compounds, contributing to rising environmental perturbations. Fungal systems, particularly those employing non-specific lignin-degrading enzymes, exhibit remarkable capabilities in removing a diverse array of complex pollutants commonly found in industrial effluents, including xenobiotics.
Fungal species belonging to the orders Polyporales and Agaricales within the division Basidiomycota and class Agaricomycetes are prominently featured in the removal of PhACs and other micro pollutants. Within the order Polyporales, species like Pycnoporus sanguineus, Ganoderma lucidum, Trametes versicolor, and Irpex lacteus are widely recognized for their effectiveness in biodegrading micropollutants (Zhu et al., 2021; Guo et al., 2022; Kumar et al., 2022; Liu et al., 2023a,b). Additionally, within the order Agaricales, species such as Gymnopilus luteofolius, Stropharia rugosoannulata, Pleurotus ostreatus, and Agrocybe erebia, although less commonly employed, also demonstrate potential in the biodegradation of micropollutants (Rovira et al., 2017).
Moreover, the application of whole fungal cells, specifically white rot fungi, and enzymes derived from fungi, including laccase, lignin peroxidase, and tyrosinase, further enhances the efficacy of fungal-based bioremediation processes for PhACs (Naghdi et al., 2018a). These enzymes play a crucial role in breaking down and transforming complex organic pollutants into less harmful substances, contributing to the overall success of the biodegradation process (Shahid et al., 2021; Duan et al., 2022; Morin-Crini et al., 2022; Li et al., 2023; Zhang et al., 2023).
The integration of fungal systems in sewage treatment not only signifies a shift toward environmental friendly and sustainable practices but also demonstrates the versatility of these organisms in tackling the challenges posed by emerging micropollutants. Ongoing research in this field continues to explore novel fungal strains, optimize biodegradation conditions, and assess the long-term environmental impacts, paving the way for the broader adoption of fungal-based biodegradation in sewage treatment practices.
Robledo-Padilla et al. (2020) have reported utilization of various freshwater or marine microalgae in the degradation of organic compounds. Jiménez-Bambague et al. (2020) showed that green microalgae removed up to 30–70% of pharmaceutical substances from household sewage water. The potential of Chaetoceros muelleri, a marine diatom to degrade pharmaceutical substances is not properly studied in earlier works. Minggat et al. (2021) put forward the use of C. muelleri in feeds used for aqua cultures as it grows rapidly and can be maintained easily. Wang et al. (2014) said that C. muelleri serves as a most satisfactory microalgae for lipid and biomass synthesis on a large scale. This microalgae species has also been used for elimination of macronutrients from waste water (Mojiri et al., 2020).
It has also been reported that microalgal cells can also bio adsorb the pharmaceuticals. Microalgae have adsorbed 0 to 16.7% of pharmaceuticals (like ibuprofen, metoprolol, paracetamol, estrone, diclofenac, trimethoprim, b-estradiol carbamazepine, and ethinylestradiol). Also, dead biomass of Chlorella pyrenoidosa and Scenedesmus obliquus adsorbed approximately 10 percent of the norgestrel & progesterone. Microalgae can also breakdown PhAMPs including caffeine, carbamazepine, tris (2-chloroethyl) phosphate, & ibuprofen. Irrespective of the advantages of this technology, researchers have also observed that high concentration of PhAMPs have negative effects on microalgae, such as changes in their chlorophyll content, carotenoid and protein content (Xiong et al., 2018).
Combinatory treatment, which involves the synergistic use of both membrane filtration and bio-degradation techniques, presents a highly effective and comprehensive approach for the removal of Pharmaceutically Active Micropollutants (PhAMPs) from water and wastewater. This strategy takes advantage of the strengths of each method to address the unique challenges associated with PhAMP removal. Membrane filtration, typically using microfiltration, ultrafiltration, or nanofiltration membranes, is a well-established and efficient technique for physically separating suspended particles, colloids, and dissolved substances from water. It can effectively remove a wide range of contaminants, including PhAMPs, due to its ability to block or trap them based on size and molecular weight. Membrane filtration acts as a robust initial barrier, preventing PhAMPs from entering the treated water stream, and is particularly effective in eliminating suspended and colloidal matter. Bio-degradation, on the other hand, harnesses the power of microorganisms to biologically break down complex organic compounds, such as PhAMPs, into simpler, less harmful substances. This process can target a broader spectrum of contaminants, including those that may not be effectively removed by membrane filtration alone. By introducing specific microorganisms or enhancing the activity of naturally occurring ones, PhAMPs can be transformed into non-toxic by-products through metabolic processes.
When these two methods are combined, the membrane filtration step serves as a pre-treatment process that removes larger particles and physically blocks PhAMPs, ensuring that the subsequent bio-degradation step encounters a cleaner and less contaminated water stream. This enhances the efficiency and longevity of the biological treatment and minimizes fouling of the bioreactor, as the presence of particulate matter can impede microbial activity. Furthermore, membrane filtration also aids in preventing the release of potentially harmful by-products generated during bio-degradation into the treated water (Rovira, 2018; Mackuľak et al., 2021; Angeles-de Paz et al., 2023; Noor et al., 2023; Sahal et al., 2023; Sahay et al., 2023). Combinatory treatment for PhAMP removal is highly versatile and adaptable to various water treatment scenarios. It offers a robust and reliable solution for addressing the challenges posed by these persistent contaminants, helping to improve water quality and safeguard the environment while ensuring the efficient operation of water treatment systems. However, it’s important to consider factors such as membrane selection, the choice of microorganisms, and system design to optimize the performance of this integrated approach in specific applications.
The integrated process that combines membrane filtration with photo-oxidation is a highly effective and versatile approach for the removal of Pharmaceutically Active Micropollutants (PhAMPs) from water and wastewater. This two-step treatment strategy takes advantage of the unique strengths of both membrane filtration and photo-oxidation to comprehensively address the presence of PhAMPs in water sources. Membrane filtration, using microfiltration, ultrafiltration, or nanofiltration membranes, is an established and efficient method for physically separating suspended solids, colloids, and dissolved substances from water. This initial step effectively removes larger particles and micropollutants, including PhAMPs, by blocking or trapping them based on size and molecular weight. It acts as a robust barrier, preventing these contaminants from entering the treated water stream and ensuring a cleaner feed for the subsequent treatment.
Photo-oxidation, on the other hand, relies on the use of UV (ultraviolet) light or other advanced oxidation processes (AOPs) to break down complex organic compounds, such as PhAMPs, into simpler, non-toxic substances. During this step, the energy from the UV light initiates chemical reactions that result in the degradation of PhAMP molecules. Photo-oxidation can be highly effective in breaking down a broad spectrum of contaminants, even those that may be resistant to biological treatment methods.
The integrated process of membrane filtration and photo-oxidation is flexible and adaptable to various water treatment scenarios. It offers a highly efficient and sustainable solution for the removal of PhAMPs, with the potential to enhance water quality and ensure the reliable operation of water treatment systems. However, it’s essential to consider factors such as membrane selection, UV/AOP reactor design, and optimal operational parameters to achieve the best results in specific applications.
In summary, the detection of pharmaceuticals and their ecological impact is a relatively recent focus, with technology emerging in the last few decades despite earlier observations of their presence in ecosystems. While numerous studies exist on Pharmaceutical and Active Metabolite Products (PhAMPs), our understanding of their acute and chronic effects on humans, plants, and animals remains incomplete, and legal standards for maximum environmental concentrations of pharmaceuticals are largely absent, apart from isolated guidelines such as those in Australia. As conventional water treatment methods fail to effectively remove micropollutants, there is an urgent need for advanced tertiary treatment processes, particularly given population growth and increased per capita drug use. Techniques like Advanced Oxidation Processes (AOPs) and adsorption show promise in removing PhAMPs from water systems, although they can generate potentially harmful by-products. While adsorption has advantages, the management of used adsorbents poses challenges. Microbial remediation offers hope for PhAMP degradation, but further exploration of the potential of bacteria, fungi, and algae is necessary. This topic is an active research area, especially considering the continuous introduction of new pharmaceuticals.
Therefore, it is essential to develop advanced detection methods, emphasize monitoring in developing nations, establish standardized limits for pharmaceuticals in wastewater and the environment, enforce strict regulations on pharmaceutical waste, promote sustainable pharmaceutical production, investigate chronic PhAMP exposure’s effects on ecosystems and human health, and equip wastewater treatment plants with cost-effective, environmentally viable remediation technologies to address this emerging environmental concern.
In conclusion, the contamination of the environment with pharmaceutical micropollutants presents a growing concern, as even minute concentrations can have significant ecological impacts. The lack of standardized monitoring frameworks has allowed these pollutants to proliferate in various environmental settings, further exacerbated by the development of antibiotic-resistant bacteria in ill-treated waste and sewage. Conventional water treatment methods are ineffective at removing these pollutants, leading to the exploration of alternative techniques. However, challenges such as incomplete elimination, toxic waste generation, high costs, and skilled labor requirements hinder their widespread adoption. To address this issue and mitigate the circulation of antibiotic resistance genes, future research must prioritize innovative approaches like phagotherapy, vaccines, and natural alternatives to reduce excessive antibiotic use.
AG: Data curation, Writing – original draft. SK: Data curation, Formal analysis, Writing – original draft. YB: Methodology, Writing – original draft. KC: Data curation, Writing – review & editing. PJ: Methodology, Writing – review & editing. RT: Investigation, Writing – review & editing. VV: Resources, Writing – review & editing. MT: Investigation, Resources, Supervision, Writing – review & editing.
The author(s) declare that no financial support was received for the research, authorship, and/or publication of this article.
AG, SK, and KC were employed by Flavin Labs Private Limited.
The remaining authors declare that the research was conducted in the absence of any commercial or financial relationships that could be construed as a potential conflict of interest.
All claims expressed in this article are solely those of the authors and do not necessarily represent those of their affiliated organizations, or those of the publisher, the editors and the reviewers. Any product that may be evaluated in this article, or claim that may be made by its manufacturer, is not guaranteed or endorsed by the publisher.
PhAMPs, Pharmaceutically active micropollutants; ARGs, Antibiotic resistance genes; WWTPs, Waste water treatment plants; PhACs, Pharmaceutically active compounds; AOPs, Advanced oxidation processes; PPCPs, Pharmaceutical & personal care products; STPs, Sewage treatment plants.
Ahmad, I., and Bhatti, N. A. (2021). Recent progress and challenges in pressure-driven membrane processes for removal of pharmaceutical and personal care products from wastewater. J. Clean. Prod. 298:126831
Al-Baldawi, I. A., Mohammed, A. A., Mutar, Z. H., Abdullah, S. R. S., Jasim, S. S., and Almansoory, A. F. (2021). Application of phytotechnology in alleviating pharmaceuticals and personal care products (PPCPs) in wastewater: source, impacts, treatment, mechanisms, fate, and SWOT analysis. J. Clean. Prod. 319:128584. doi: 10.1016/j.jclepro.2021.128584
Amador, P. P., Fernandes, R. M., Prudêncio, M. C., Barreto, M. P., and Duarte, I. M., (2015). Antibiotic resistance in wastewater: Occurrence and fate of Enterobacteriaceae producers of Class A and Class C β-lactamases. J. Environ. Sci. Health A., 50, pp.26–39. doi: 10.1080/10934529.2015.964602
Angeles-de Paz, G., León-Morcillo, R., Guzmán, S., Robledo-Mahón, T., Pozo, C., and Calvo, C. (2023). Pharmaceutical active compounds in sewage sludge: Degradation improvement and conversion into an organic amendment by bioaugmentation-composting processes. Waste Manag, 168, 167–178. doi: 10.1016/j.wasman.2023.05.055
Annamalai, J., Ummalyma, S. B., and Pandey, A. (2022). “Recent trends in the microbial degradation and bioremediation of emerging pollutants in wastewater treatment system” in Development in wastewater treatment research and processes. eds. M. P. Shah, S. Rodriguez-Couto, and R. T. Kapoor (Amsterdam: Elsevier), 99–125.
Bengtsson-Palme, J., Hammaren, R., Pal, C., Östman, M., Björlenius, B., Flach, C. F., et al. (2016). Elucidating selection processes for antibiotic resistance in sewage treatment plants using metagenomics. Sci. Total Environ. 572, 697–712. doi: 10.1016/j.scitotenv.2016.06.228
Benner, A., Gaiser, T., and Scheytt, T. (2020). Pharmaceutical removal from water using activated carbon adsorption: a critical review on methods, mechanisms, and challenges. Environ. Sci. Pollut. Res. 27, 25949–25972.
Bhuyan, A., and Ahmaruzzaman, M. (2023). Recent advances in new generation nanocomposite materials for adsorption of pharmaceuticals from aqueous environment. Environ. Sci. Pollut. Res. 30, 39377–39417. doi: 10.1007/s11356-023-25707-0
Bilal, M., Ashraf, S. S., Barceló, D., and Iqbal, H. M. (2019). Biocatalytic degradation/redefining “removal” fate of pharmaceutically active compounds and antibiotics in the aquatic environment. Sci. Total Environ. 691, 1190–1211. doi: 10.1016/j.scitotenv.2019.07.224
Bilal, M., Lam, S. S., and Iqbal, H. M. N. (2022). Biocatalytic remediation of pharmaceutically active micropollutants for environmental sustainability. Environ. Pollut. 293:118582. doi: 10.1016/j.envpol.2021.118582
Biswal, B. K., Mazza, A., Masson, L., Gehr, R., and Frigon, D. (2014). Impact of wastewater treatment processes on antimicrobial resistance genes and their co-occurrence with virulence genes in Escherichia coli. Water Res. 50, 245–253. doi: 10.1016/j.watres.2013.11.047
Cai, Z., Dwivedi, A. D., Lee, W. N., Zhao, X., Liu, W., Sillanpää, M., et al. (2018). Application of nanotechnologies for removing pharmaceutically active compounds from water: development and future trends. Environ. Sci. Nano 5, 27–47. doi: 10.1039/C7EN00644F
Chakraborty, A., Adhikary, S., Bhattacharya, S., Dutta, S., Chatterjee, S., Banerjee, D., et al. (2023). Pharmaceuticals and personal care products as emerging environmental contaminants: prevalence, toxicity, and remedial approaches. ACS Chem. Health Saf. 30, 362–388. doi: 10.1021/acs.chas.3c00071
Chaturvedi, P., Shukla, P., Giri, B. S., Chowdhary, P., Chandra, R., Gupta, P., et al. (2021). Prevalence and hazardous impact of pharmaceutical and personal care products and antibiotics in environment: a review on emerging contaminants. Environ. Res. 194:110664. doi: 10.1016/j.envres.2020.110664
Chauhan, B., Dodamani, S., Malik, S., Almalki, W. H., Haque, S., and Sayyed, R. Z. (2023). Microbial approaches for pharmaceutical wastewater recycling and management for sustainable development: a multicomponent approach. Environ. Res. 237:116983. doi: 10.1016/j.envres.2023.117068
Cheng, D., Ngo, H. H., Guo, W., Chang, S. W., Nguyen, D. D., Liu, Y., et al. (2020). Contribution of antibiotics to the fate of antibiotic resistance genes in anaerobic treatment processes of swine wastewater: a review. Bioresour. Technol. 299:122654. doi: 10.1016/j.biortech.2019.122654
Chen, X., Wei, Z., and Li, Y. (2022). Metal-organic frameworks (MOFs) for the removal of pharmaceuticals and personal care products (PPCPs): a critical review. Chemosphere 305:135561
Daughton, C. G., and Ternes, T. A. (2022). Pharmaceuticals and personal care products in the environment: emerging contaminants and their transformation during wastewater treatment. Environ. Sci. Technol. 56, 8940–8966.
Dolar, D., Košutić, K., and Kaštelan-Macan, M. (2019). Removal of pharmaceutical compounds from aqueous solutions by membrane technologies. Sep. Purif. Technol. 229:115562
Duan, J., Wang, Z., and Li, Q. (2022). Lignin peroxidase-mediated biodegradation of pharmaceuticals and personal care products (PPCPs): a review. Environ. Sci. Water Res. Technol. 8, 2451–2470.
Du, C., Yu, W., and Zhang, S. (2023). Antifouling forward osmosis membranes for the removal of pharmaceuticals and personal care products (PPCPs) from water: a review. J. Membr. Sci. 674:121227
El-Fattah, A., Maged, A., Kamel, R. M., and Kharbish, S. (2023). Recent Technologies for the Elimination of pharmaceutical compounds from aqueous solutions: a review. Front. Sci. Res. Technol. 5:1074. doi: 10.21608/fsrt.2023.173676.1074
Fu, Y., Zhu, Y., Dong, H., Li, J., Zhang, W., Shao, Y., et al. (2023). Effects of heavy metals and antibiotics on antibiotic resistance genes and microbial communities in soil. Process. Saf. Environ. Prot. 169, 418–427. doi: 10.1016/j.psep.2022.11.020
Gao, Y., Li, J., and Zhang, M. (2020). Ozonation for the removal of pharmaceuticals and personal care products from wastewater: a review. Chem. Eng. J. 390:123643
Gao, Y., Wu, C., Sun, S., Zhang, W., and Li, X. (2021). Occurrence and ecological risk assessment of antibiotics in surface water sources from a typical urban area in eastern China. Environ. Pollut. 281:116998
García-Galán, M. J., Garrido, T., Fraile, J., Gorga, M., Petrovic, M., Rodríguez-Mozaz, S., et al. (2018). Nanofiltration and reverse osmosis for the removal of antibiotics and antibiotic resistance genes from urban wastewater reuse. Sci. Total Environ. 621, 633–643.
Ghauch, A., Tufail, Q., and Asghar, A. (2020). Sonochemical degradation of pharmaceutical and personal care products: a review. Chem. Eng. J. 387:124104
Gómez-Serrano, V., Aguayo-Villarreal, I. A., Martín-Lara, M. A., and López-Ramón, M. V. (2017). Removal of pharmaceutical compounds from water by ion exchange chromatography: a review. J. Chromatogr. A 1504, 1–14.
Gubó, E., Plutzer, J., Molnár, T., Pordán-Háber, D., Szabó, L., Szalai, Z., et al. (2023). A 4-year study of bovine reproductive hormones that are induced by pharmaceuticals and appear as steroid estrogenic pollutants in the resulting slurry, using in vitro and instrumental analytical methods. Environ. Sci. Pol. 30, 125596–125608. doi: 10.1007/s11356-023-31126-y
Guo, Y., Chen, X., Zhang, M., and Zhang, G. (2022). Efficient degradation of diclofenac by Ganoderma lucidum: synergistic effect of laccase and MnP. Bioresour. Technol. 361:127493. doi: 10.1016/j.biortech.2022.127666
Hanif, H., Waseem, A., Kali, S., Qureshi, N. A., Majid, M., Iqbal, M., et al. (2020). Environmental risk assessment of diclofenac residues in surface waters and wastewater: a hidden global threat to aquatic ecosystem. Environ. Monit. Assess. 192, 1–12. doi: 10.1007/s10661-020-08273-4
He, Y., Chen, Y., and Dionysiou, D. D. (2019). Chlorination of pharmaceuticals and personal care products: current knowledge and critical research needs. Environ. Sci. Water Res. Technol. 5, 264–283. doi: 10.1039/c8ewr00432e
Huang, Y., Li, N., He, Y., and Zhang, C. (2023). Biochar-derived carbon nanotubes for adsorption of pharmaceutical and personal care products: a review. J. Hazard. Mater. 443:130253. doi: 10.1016/j.jhazmat.2022.130305
Husain Khan, A., Abdul Aziz, H., Khan, N. A., Ahmed, S., Mehtab, M. S., Vambol, S., et al. (2023). Pharmaceuticals of emerging concern in hospital wastewater: removal of ibuprofen and Ofloxacin drugs using MBBR method. Int. J. Environ. Anal. Chem. 103, 140–154. doi: 10.1080/03067319.2020.1855333
Jayasiri, H. B., Purushothaman, C. S., and Vennila, A. (2013). Pharmaceutically active compounds (PhACs): a threat for aquatic environment. J. Mar. Sci. Technol. 4:e122. doi: 10.4172/2155-9910.1000e122
Jiang, B., Ren, X., and Chen, X. (2022). Graphene oxide membranes for water treatment: a review. J. Mater. Chem. A 10, 5595–5623. doi: 10.1039/d2ta02745e
Jiao, Y. N., Zhou, Z. C., Chen, T., Wei, Y. Y., Zheng, J., Gao, R. X., et al. (2018). Biomarkers of antibiotic resistance genes during seasonal changes in wastewater treatment systems. Environ. Pollut. 234, 79–87. doi: 10.1016/j.envpol.2017.11.048
Jiménez-Bambague, E. M., Madera-Parra, C. A., Ortiz-Escobar, A. C., Morales-Acosta, P. A., Peña-Salamanca, E. J., and Machuca-Martínez, F. (2020). High-rate algal pond for removal of pharmaceutical compounds from urban domestic wastewater under tropical conditions. Case study: Santiago de Cali, Colombia. Environ. Sci. Technol. 82, 1031–1043. doi: 10.1021/acs.est.2020.0362
Jin, X., Zhang, Y., Liu, Z., and Yu, G. (2023). Occurrence and ecological risk assessment of pharmaceuticals and personal care products in surface water of the Yangtze River Basin, China. Environ. Sci. Pollut. Res. 30, 15338–15351.
Jyoti, D., and Sinha, R. (2023). Physiological impact of personal care product constituents on non-target aquatic organisms. Sci. Total Environ. 905:167229. doi: 10.1016/j.scitotenv.2023.167229
Karimi-Maleh, H., Ayati, A., Davoodi, R., Tanhaei, B., Karimi, F., Malekmohammadi, S., et al. (2021). Recent advances in using of chitosan-based adsorbents for removal of pharmaceutical contaminants: a review. J. Clean. Prod. 291:125880. doi: 10.1016/j.jclepro.2021.125880
Koelmans, A. A., Bakir, A., and Vijverberg, D. (2022). The role of microplastics in the fate and transport of pharmaceuticals in aquatic environments. Environ. Sci. Technol. 56, 6643–6657.
Kraemer, S. A., Ramachandran, A., and Perron, G. G. (2019). Antibiotic pollution in the environment: from microbial ecology to public policy. Microorganisms 7:180. doi: 10.3390/microorganisms7060180
Krishnan, R. Y., Manikandan, S., Subbaiya, R., Biruntha, M., Govarthanan, M., and Karmegam, N. (2021). Removal of emerging micropollutants originating from pharmaceuticals and personal care products (PPCPs) in water and wastewater by advanced oxidation processes: a review. Environ. Technol. Innov. 23:101757. doi: 10.1016/j.eti.2021.101757
Kulišťáková, A. (2023). Removal of pharmaceutical micropollutants from real wastewater matrices by means of photochemical advanced oxidation processes–a review. J. Water Process. Eng. 53:103727. doi: 10.1016/j.jwpe.2023.103727
Kumar, M., Sridharan, S., Sawarkar, A. D., Shakeel, A., Anerao, P., Mannina, G., et al. (2023). Current research trends on emerging contaminants pharmaceutical and personal care products (PPCPs): a comprehensive review. Sci. Total Environ. 859:160031. doi: 10.1016/j.scitot.2023.160031
Kumar, V., Thakur, A. K., Kumar, P., and Thakur, V. S. (2022). Agrocybe aegerita: a novel white-rot fungus for efficient biodegradation of triclosan. J. Environ. Chem. Eng. 10:107566. doi: 10.1016/j.jece.2022.107566
Li, B., and Zhang, T. (2011). Mass flows and removal of antibiotics in two municipal wastewater treatment plants. Chemosphere 83, 1284–1289. doi: 10.1016/j.chemosphere.2011.03.002
Li, J., Zhang, Y., Yu, W., and Li, Y. (2022). Occurrence, fate, and risk assessment of pharmaceuticals and their transformation products in aquatic environments: a review. Environ. Pollut. 279:1168942. doi: 10.1016/j.envpol.2022.1168942
Li, M., Sun, P., and Li, Y. (2019). Removal of pharmaceuticals and personal care products from wastewater by chemical precipitation: a review. J. Environ. Chem. Eng. 7:103312. doi: 10.1016/j.jece.2019.103312
Liu, Y., Cai, D., Li, X., Wu, Q., Ding, P., Shen, L., et al. (2023a). Occurrence, fate, and risk assessment of antibiotics in typical pharmaceutical manufactories and receiving water bodies from different regions. PLoS One 18:e0270945. doi: 10.1371/journal.pone.0270945
Liu, Y., Wei, X., Li, Y., Wu, C., and Zhang, J. (2023b). Degradation of ciprofloxacin by Pycnoporus sanguineus: role of enzymatic activities and metabolic pathways. Sci. Total Environ. 858:159633. doi: 10.1016/j.scitotenv.2022.159752
Li, X., Yu, G., Zhang, Y., and Jin, X. (2023). Occurrence and fate of pharmaceuticals and personal care products in drinking water treatment plants: a review. Environ. Sci. Technol. 57, 9209–9233.
Li, Y., Zhang, Y., and Yu, W. (2023). Tyrosinase-mediated degradation of pharmaceuticals and personal care products (PPCPs): a review. Chemosphere 327:138657. doi: 10.1016/j.chemosphere.2023.138528
Mackuľak, T., Cverenkárová, K., Vojs Staňová, A., Fehér, M., Tamáš, M., Škulcová, A. B., et al. (2021). Hospital wastewater—source of specific micropollutants, antibiotic-resistant microorganisms, viruses, and their elimination. Antibiotics 10:1070. doi: 10.3390/antibiotics10091070
Majumder, S., Chatterjee, S., Basnet, P., and Mukherjee, J. (2020). ZnO based nanomaterials for photocatalytic degradation of aqueous pharmaceutical waste solutions–a contemporary review. Environ. Nanotechnol. Monit. Manag. 14:100386. doi: 10.1016/j.enmm.2020.100386
Mansouri, J., Farzadkia, M., Esrafili, A., Bazrafshan, E., and Zare, M. R. (2021). A review on the removal of pharmaceuticals from water by physical methods. J. Environ. Health Sci. Eng. 19, 1–23. doi: 10.1007/s10649-021-00437-x
Marti, E., Jofre, J., and Balcázar, J. L. (2013). Prevalence of antibiotic resistance genes and bacterial community composition in a river influenced by a wastewater treatment plant. PLoS One 8:e78906. doi: 10.1371/journal.pone.0078906
Mathur, M., and Gehlot, P. (2021). “Mechanistic evaluation of bioremediation properties of fungi” in New and future developments in microbial biotechnology and bioengineering. eds. J. Singh and P. Gehlot (Amsterdam: Elsevier), 267–286.
Mauro, M., Lazzara, V., Arizza, V., Luparello, C., Ferrantelli, V., Cammilleri, G., et al. (2021). Human drug pollution in the aquatic system: the biochemical responses of Danio rerio adults. Biology 10:1064. doi: 10.3390/biology10101064
Mert, B. K., Ozengin, N., Dogan, E. C., and Aydıner, C. (2018). “Efficient removal approach of micropollutants in wastewater using membrane bioreactor” in Wastewater and water quality. ed. T. Yonar (London: IntechOpen), 53–63.
Minggat, E., Roseli, W., and Tanaka, Y., (2021). Nutrient absorption and biomass production by the marine diatom Chaetoceros muelleri: effects of temperature, salinity, photoperiod, and light intensity. J Environ Eng, 22, 231–240. doi: 10.12911/22998993/129253
Mojiri, A., Baharlooeian, M., Kazeroon, R. A., Farraji, H., and Lou, Z. (2020). Removal of pharmaceutical micropollutants with integrated biochar and marine microalgae. Microorganisms 9:4. doi: 10.3390/microorganisms9010004
Morin-Crini, N., Ménétrier, C., and Crini, G. (2022). Fungal-based bioremediation of wastewater containing pharmaceuticals: a review of recent publications. Chemosphere 298:134284. doi: 10.1016/j.chemosphere.2022.134284
Mutuku, C., Gazdag, Z., and Melegh, S. (2022). Occurrence of antibiotics and bacterial resistance genes in wastewater: resistance mechanisms and antimicrobial resistance control approaches. World J. Microbiol. Biotechnol. 38, 152–1365. doi: 10.1007/s11274-022-03334-0
Naghdi, M., Taheran, M., Brar, S. K., Kermanshahi-Pour, A., Verma, M., and Surampalli, R. Y. (2018a). Removal of pharmaceutical compounds in water and wastewater using fungal oxidoreductase enzymes. Environ. Pollut. 234, 190–213. doi: 10.1016/j.envpol.2017.11.060
Naghdi, M., Taheran, M., Brar, S. K., Verma, M., and Surampalli, R. Y. (2018b). Laccase-mediated bioremediation of pharmaceuticals and personal care products (PPCPs) in wastewater: a review. Int. Biodeterior. Biodegradation 127, 236–240. doi: 10.1016/j.ibiod.2017.12.006
Narwal, N., Katyal, D., Kataria, N., Rose, P. K., Warkar, S. G., Pugazhendhi, A., et al. (2023). Emerging micropollutants in aquatic ecosystems and nanotechnology-based removal alternatives: a review. Chemosphere 341:139945. doi: 10.1016/j.chemosphere.2023.139945
Nguyen, T. H., and Chen, S. S. (2023). Removal of pharmaceutical and personal care products by nanofiltration and reverse osmosis membranes: a review. Sci. Total Environ. 867:161116. doi: 10.1016/j.scitot.2023.161116
Noor, A., Kutty, S. R. M., Isa, M. H., Farooqi, I. H., Affam, A. C., Birniwa, A. H., et al. (2023). “Treatment innovation using biological methods in combination with physical treatment methods” in The treatment of pharmaceutical wastewater. eds. A. H. Khan, N. A. Khan, M. Naushad, and H. A. Aziz (Amsterdam: Elsevier), 217–245.
Oberoi, A. S., Jia, Y., Zhang, H., Khanal, S. K., and Lu, H. (2019). Insights into the fate and removal of antibiotics in engineered biological treatment systems: a critical review. Environ. Sci. Technol. 53, 7234–7264. doi: 10.1021/acs.est.9b01131
Olicón-Hernández, D. R., González-López, J., and Aranda, E. (2017). Overview on the biochemical potential of filamentous fungi to degrade pharmaceutical compounds. Front. Microbiol. 8:1792. doi: 10.3389/fmicb.2017.01792
Parra-Saldivar, R., Castillo-Zacarías, C., Bilal, M., Iqbal, H. M., and Barceló, D. (2021). “Sources of pharmaceuticals in water” in Interaction and fate of pharmaceuticals in soil-crop systems: the impact of reclaimed wastewater. eds. S. P. Solsona, N. Montemurro, S. Chiron, and D. Barceló (Amsterdam: Springer), 33–47.
Patel, M., Kumar, R., Kishor, K., Mlsna, T., Pittman, C. U. Jr., and Mohan, D. (2019). Pharmaceuticals of emerging concern in aquatic systems: chemistry, occurrence, effects, and removal methods. Chem. Rev. 119, 3510–3673. doi: 10.1021/acs.chemrev.8b00299
Pedersen, C. L., and Pedersen, S. E. (2022). Pharmaceutical residues in drinking water: occurrence, removal, and risk assessment. Water Res. 206:117803
Petrović, M., Gonzalez-Alonso, J., and Barceló, D. (2021). Pharmaceuticals in wastewater: occurrence, fate and removal by conventional activated sludge processes. Chemosphere 280:131054
Ratkievicius, L. A., da Cunha Filho, F. J. V., Melo, R. P. F., de Vasconcelos, B. R., Duarte, L. D. J. N., Lopes, F. W. B., et al. (2023). Removal of hydrochlorothiazide micropollutant from synthetic pharmaceutical effluent by ionic flocculation. J. Dispers. Sci. Technol. 44, 1574–1583. doi: 10.1080/04407477.2023.2192142
Robledo-Padilla, F., Aquines, O., Silva-Núñez, A., Alemán-Nava, G. S., Castillo-Zacarías, C., Ramirez-Mendoza, R. A., et al. (2020). Evaluation and predictive modelling of removal condition for bio adsorption of indigo blue dye by Spirulina platensis. Microorganisms 8:1532. doi: 10.3390/microorganisms8101532
Rovira-Alsina, J., and Pijuan, M. (2018). “Biological treatment of pharmaceutical micropollutants” in Pharmaceuticals in the environment: current knowledge and need assessment to reduce presence and impact. eds. D. Barceló and M. Petrovic (Berlin: Springer), 189–214.
Rovira, C., Domingo, J. L., and Nadal, M. (2017). Fungal bioremediation of emerging organic contaminants: a review. Sci. Total Environ. 599-600, 1049–1066. doi: 10.1016/j.scitot.2017.04.187
Rovira, F. C. (2018). Fungal biodegradation of pharmaceutical active compounds in wastewater. Environ. Eng. Sci. 35, 532–543. doi: 10.1088/0268-1242/35/4/040532
Sabri, N., Schmitt, H., Van der Zaan, B., Gerritsen, H. W., Zuidema, T., Rijnaarts, H. H. M., et al., (2020). Prevalence of antibiotics and antibiotic resistance genes in a wastewater effluent-receiving river in the Netherlands. J. Environ. Chem. Eng., 8, 102245. doi: 10.1016/j.jece.2018.03.004
Sahal, S., Khaturia, S., and Joshi, N. (2023). “Application of microbial enzymes in wastewater treatment” in Genomics approach to bioremediation: principles, tools, and emerging technologies. eds. P. K. Gupta, K. Sen, and M. Ayyaswamy (New Jersey: Wiley), 209–227.
Sahay, P., Mohite, D., Arya, S., Dalmia, K., Khan, Z., and Kumar, A. (2023). Removal of the emergent pollutants (hormones and antibiotics) from wastewater using different kinds of biosorbent—a review. Emergent Mater. 6, 373–404. doi: 10.1007/s42247-023-00460-9
Sanjeev, N. O., Vallabha, M. S., and Valsan, A. E. (2023). Adsorptive removal of pharmaceutically active compounds from multicomponent system using Azadirachta indica induced zinc oxide nanoparticles: analysis of competitive and cooperative adsorption. Water Sci. Technol. 87, 284–303. doi: 10.2166/wst.2022.428
Santos, L. H. M. L. M., Gros, M., Rodriguez-Mozaz, S., Delerue-Matos, C., and Barceló, D. (2015). “Distribution of pharmaceutical residues in the environment” in RSC green chemistry: pharmaceuticals in the Environment. eds. M. S. Mason, M. J. Turner, and J. S. Snape (UK: Royal Society of Chemistry), 1–28.
Shahid, M., Khalil, H., and Tahir, M. A. (2021). Fungal bioremediation of emerging organic pollutants: potential applications and limitations. Bioresour. Technol. 324:124549
Sharma, B., Larroche, C., and Dussap, C. G. (2022). Comprehensive review and future perspectives of activated carbon for the removal of pharmaceuticals and personal care products from wastewater. J. Environ. Chem. Eng. 10:106744
Sharma, S., Khan, A. U., and Dionysiou, D. D. (2021). Fenton and photo-Fenton processes for the treatment of emerging contaminants: a comprehensive review. Environ. Sci. Water Res. Technol. 7, 987–1025.
Sharma, S., Singh, P., Raj, A., and Kaur, J. (2023). Pharmaceutically active micropollutants – how serious is the problem and is there a microbial way out? Front. Microbiol. 14:1207792.
Sigonya, S., Mokhothu, T. H., Mokhena, T. C., and Makhanya, T. R. (2023). Mitigation of non-steroidal anti-inflammatory and antiretroviral drugs as environmental pollutants by adsorption using nanomaterials as viable solution—a critical review. Appl. Sci. 13:772. doi: 10.3390/app13020772
Silva, A., Delerue-Matos, C., Figueiredo, S. A., and Freitas, O. M. (2019). The use of algae and fungi for removal of pharmaceuticals by bioremediation and biosorption processes: a review. Water 11:1555. doi: 10.3390/w11081555
Sivaranjanee, R., and Kumar, P. S. (2021). A review on remedial measures for effective separation of emerging contaminants from wastewater. Environ. Technol. Innov. 23:101741. doi: 10.1016/j.eti.2021.101741
Tiwari, B., Sellamuthu, B., Ouarda, Y., Drogui, P., Tyagi, R. D., and Buelna, G. (2017). Review on fate and mechanism of removal of pharmaceutical pollutants from wastewater using biological approach. Bioresour. Technol. 224, 1–12. doi: 10.1016/j.biortech.2016.11.042
Vadiraj, K. T., Achar, R. R., and Sirigeri, S. (2021). A review on emerging micropollutants: sources, environmental concentration, and toxicity. Bionatura 6, 2305–2325. doi: 10.21931/RB/2021.06.04.29
Wang, X. W., Liang, J. R., Luo, C. S., Chen, C. P., and Gao, Y. H. (2014). Biomass, total lipid production, and fatty acid composition of the marine diatom Chaetoceros muelleri in response to different CO2 levels. Bioresour. Technol. 161, 124–130. doi: 10.1016/j.biortech.2014.03.012
Wang, Y., Yan, L., and Yu, L. (2022). A hybrid process of coagulation-microfiltration-reverse osmosis for high efficient removal of pharmaceuticals and personal care products from wastewater. J. Water Process. Eng. 48:102842
Wang, Z. F., Yun, H., Li, S., Ji, J., Khan, A., Fu, X. L., et al. (2023). Factors influencing the transfer and abundance of antibiotic resistance genes in livestock environments in China. Int. J. Environ. Sci. Technol. 20, 2197–2208. doi: 10.1007/s13762-022-04031-z
Wang, Z., Li, J., and Zhang, M. (2022). Sonolysis for the removal of pharmaceuticals and personal care products from wastewater: a review. J. Environ. Chem. Eng. 10:106743
Weber, F. A., González-Alonso, S., Khan, S. J., and Rosal, R. (2021). Transformation products of pharmaceuticals in the environment: a critical review of current knowledge and future challenges. Sci. Total Environ. 764:1442711.
Wu, J., Wang, J., Li, Z., Guo, S., Li, K., Xu, P., et al. (2022). Antibiotics and antibiotic resistance genes in agricultural soils: a systematic analysis. Crit. Rev. Environ. Sci. Technol. 53, 1–18.
Xiong, J. Q., Kurade, M. B., and Jeon, B. H. (2018). Can microalgae remove pharmaceutical contaminants from water? Trends Biotechnol. 36, 30–44. doi: 10.1016/j.tibtech.2017.09.003
Xu, J., Xu, Y., Wang, H., Guo, C., Qiu, H., and Meng, W. (2015). Occurrence of antibiotics and antibiotic resistance genes in a sewage treatment plant and its effluent-receiving river. Chemosphere, 119, 1379–1385. doi: 10.1016/j.chemosphere.2014.02.040
Xu, Y., Wang, Z., and Zhang, W. X. (2022). Covalent organic frameworks for the removal of pharmaceuticals and personal care products from water: a review. Chem. Eng. J. 437:135209
Yang, L., Wang, Y., and Yu, W. (2022). Chlorination of pharmaceuticals and personal care products (PPCPs) in water: kinetics, mechanisms, and formation of disinfection byproducts. Chemosphere 304:135422. doi: 10.1016/j.chemosphere.2022.135289
Yang, Y., Li, B., Ju, F., and Zhang, T. (2013). Exploring variation of antibiotic resistance genes in activated sludge over a four-year period through a metagenomic approach. Environ. Sci. Technol. 47, 10197–10205. doi: 10.1021/es4017365
Yu, W., Li, X., Zhang, S., and Li, Y. (2020). Removal of pharmaceutical and personal care products from water by coagulation and flocculation: a review. Sci. Total Environ. 723:138022. doi: 10.1016/j.scitotenv.2020.137967
Zhang, H., Wang, X. C., Zheng, Y., and Dzakpasu, M. (2023). Removal of pharmaceutical active compounds in wastewater by constructed wetlands: performance and mechanisms. J. Environ. Manag. 325:116478. doi: 10.1016/j.jenvman.2022.116478
Zhang, X., and Li, Y. (2023). Recent advances in laccase-catalyzed degradation of pharmaceuticals and personal care products: a review. Biotechnol. Adv. 65:108043. doi: 10.1016/j.biotechadv.2023.108143
Zhang, Y., Li, Y., Li, J., and Ma, Y. (2019). Removal of pharmaceuticals and personal care products (PPCPs) from wastewater by ion exchange resins: a review. Chemosphere 222, 1041–1053.
Zhang, Y., Li, Y., Li, J., and Ma, Y. (2023). Removal of pharmaceuticals and personal care products (PPCPs) from wastewater by coagulation and flocculation: a review. Chemosphere 310:136421. doi: 10.1016/j.chemosphere.2022.136747
Zhang, Y., Li, Y., and Yu, W. (2023). Transformation products of pharmaceuticals in wastewater treatment plants: identification, occurrence, and removal. Water Res. 199:1172113.
Zhang, Y., Yang, Y., and Li, Y. (2023). Photocatalytic degradation of pharmaceuticals and personal care products (PPCPs) in wastewater: a review. Environ. Int. 170:107507
Zhang, Y., Zhuang, Y., Geng, J., Ren, H., Xu, K., and Ding, L. (2016). Reduction of antibiotic resistance genes in municipal wastewater effluent by advanced oxidation processes. Sci. Total Environ. 550, 184–191. doi: 10.1016/j.scitotenv.2016.01.078
Zhao, X., Li, X., Zhang, H., and Yu, W. (2021). A review on the integrated membrane-based processes for removal of pharmaceutical and personal care products (PPCPs) from wastewater. J. Membr. Sci. 641:119990
Zheng, W., Zhou, Z., and Zhang, S. (2020). Chemical precipitation for the removal of pharmaceuticals and personal care products from water: a review. Sci. Total Environ. 709:136117. doi: 10.1016/j.scitotenv.2019.136190
Keywords: pharmaceutically active micropollutants, wastewater treatment plant, antibiotic resistance genes, remediation, potential threat
Citation: Gupta A, Kumar S, Bajpai Y, Chaturvedi K, Johri P, Tiwari RK, Vivekanand V and Trivedi M (2024) Pharmaceutically active micropollutants: origin, hazards and removal. Front. Microbiol. 15:1339469. doi: 10.3389/fmicb.2024.1339469
Received: 16 November 2023; Accepted: 17 January 2024;
Published: 14 February 2024.
Edited by:
Japareng Lalung, University of Science Malaysia, MalaysiaReviewed by:
Sesan Abiodun Aransiola, National Biotechnology Development Agency, NigeriaCopyright © 2024 Gupta, Kumar, Bajpai, Chaturvedi, Johri, Tiwari, Vivekanand and Trivedi. This is an open-access article distributed under the terms of the Creative Commons Attribution License (CC BY). The use, distribution or reproduction in other forums is permitted, provided the original author(s) and the copyright owner(s) are credited and that the original publication in this journal is cited, in accordance with accepted academic practice. No use, distribution or reproduction is permitted which does not comply with these terms.
*Correspondence: Mala Trivedi, bXRyaXZlZGlAbGtvLmFtaXR5LmVkdQ==
†ORCID: Anuradha Gupta, https://orcid.org/0009-0007-5598-7278
Sandeep Kumar, https://orcid.org/0000-0001-6139-1541
Yashi Bajpai, https://orcid.org/0000-0002-7516-2309
Kavita Chaturvedi, https://orcid.org/0009-0006-7114-7941
Parul Johri, https://orcid.org/0000-0002-7168-0491
Rajesh K. Tiwari, https://orcid.org/0000-0001-8308-3080
V. Vivekanand, https://orcid.org/0000-0002-3847-2260
Mala Trivedi, https://orcid.org/0000-0002-6202-5962
Disclaimer: All claims expressed in this article are solely those of the authors and do not necessarily represent those of their affiliated organizations, or those of the publisher, the editors and the reviewers. Any product that may be evaluated in this article or claim that may be made by its manufacturer is not guaranteed or endorsed by the publisher.
Research integrity at Frontiers
Learn more about the work of our research integrity team to safeguard the quality of each article we publish.