- 1College of Food Science and Technology, Huazhong Agriculture University, Wuhan, Hubei, China
- 2Liunan District Modern Agricultural Industry Service Center of Liuzhou City, Liuzhou, Guangxi, China
- 3Institute of Infection and Immunity, Taihe Hospital, Hubei University of Medicine, Shiyan, China
In order to produce fermented bamboo shoots with functional properties, two strains of lactic acid bacteria were selected for inoculation and fermentation. One strain, Lactiplantibacillus plantarum R1, exhibited prominent potential probiotic properties (including gastrointestinal condition tolerance, adhesion ability, antimicrobial ability, and antibiotic resistance), while the other, Levilactobacillus brevis R2, demonstrated the capability of high γ-aminobutyric acid (GABA) production (913.99 ± 14.2 mg/L). The synergistic inoculation of both strains during bamboo shoot fermentation led to a remarkable increase in GABA content (382.31 ± 12.17 mg/kg), surpassing that of naturally fermented bamboo shoots by more than 4.5 times and outperforming mono-inoculated fermentation. Simultaneously, the nitrite content was maintained at a safe level (5.96 ± 1.81 mg/kg). Besides, inoculated fermented bamboo shoots exhibited an increased crude fiber content (16.58 ± 0.04 g/100 g) and reduced fat content (0.39 ± 0.02 g/100 g). Sensory evaluation results indicated a high overall acceptability for the synergistically inoculated fermented bamboo shoots. This study may provide a strategy for the safe and rapid fermentation of bamboo shoots and lay the groundwork for the development of functional vegetable products enriched with GABA.
1 Introduction
Bamboo shoots are known as the “King of Forest Vegetables” due to their low-fat content and high levels of dietary fiber, protein and minerals. However, the challenge with fresh bamboo shoots is their rapid lignification after harvesting (Zhang et al., 2020), which significantly reduces their food quality and commercial value. To address this issue, various processing methods are employed, including fermentation, drying, steaming, and pickling. Out of these methods, fermentation leads to significant changes in the content of amino acids, organic acids, aromatic compounds, esters, and other substances, resulting in a unique flavor, which has contributed to the growing popularity of fermented bamboo shoots.
Similar to most fermented vegetables, the fermentation process of bamboo shoots is significantly influenced by lactic acid bacteria (LAB), which include various species such as Lactobacillus sp., Leuconostoc sp., Weissella sp., and Pediococcus sp. (Guan et al., 2020; Chen C. et al., 2022; Li et al., 2022; Das et al., 2023). Indeed, numerous studies have demonstrated the intricate connection between complex microbial communities and the production of distinctive flavor compounds in fermented bamboo shoots. Substances like p-cresol, lactic acid, acetic acid, 1-octen-3-one, and others contribute to the unique and characteristic flavors developed during the fermentation process (Chen C. et al., 2022; Tang et al., 2024). However, the unpredictable microbial succession and challenging-to-control fermentation environment can lead to quality issues, including the formation of undesirable components, off-flavors, and undesirable coloration. In response to these challenges, researchers have proposed the method of inoculated fermentation. Lactiplantibacillus plantarum stands out as one of the most commonly employed strains in inoculated fermentation. It demonstrates effective inhibition of nitrite and pathogenic bacteria, while also enhances the overall flavor of products (Zhang et al., 2022; Yu et al., 2023). Additionally, other strains such as Levilactobacillus brevis, Lactobacillus sake, and Leuconostoc mesenteroides have also shown positive impacts on the fermentation food (Kim et al., 2017; Xia et al., 2017; Huang et al., 2021). Furthermore, researchers discovered that employing multiple strains for synergistic fermentation not only enhances the safety of the fermentation process but also contributes to a more robust flavor profile (Luo et al., 2020; Hu et al., 2021). The inoculation of Leuconostoc citreum and L. plantarum for bamboo shoots fermentation demonstrated significant inhibition of the growth of Enterococcus and Clostridium, suggesting potential benefits. Moreover, the inoculation ratio of the two strains had a notable impact on the flavor compounds after fermentation (Lu et al., 2022). However, there is limited research on the application of synergistic inoculation fermentation in bamboo shoots, and further investigations are warranted on different LAB strains and their effects on the nutrition and flavor.
Gamma-aminobutyric acid (GABA) is a non-protein amino acid widely distributed in plants, animals and microorganisms. It is synthesized through the decarboxylation of L-glutamic acid (L-Glu) catalyzed by the enzyme glutamic acid decarboxylase (GAD, EC 4.1.1.15). Serving as an important inhibitory neurotransmitter, GABA has a wide range of physiological functions, such as improving sleep, treating diabetes, alleviating anxiety, and combating obesity (Pannerchelvan et al., 2023). Compared to GABA obtained from plant and animal sources, microbial fermentation offers several advantages, including high efficiency, safety, and freedom from seasonal and geographical limitations. Microorganisms such as fungi, yeasts, and bacteria can be employed in the fermentation of GABA. Lactic acid bacteria, which are considered as Generally Recognized as Safe (GRAS) microorganisms, have been reported to be capable of producing GABA in large quantities. Strains such as L. plantarum (Park et al., 2021), L. brevis (Kanklai et al., 2021), Pediococcus pentosus (Thuy et al., 2021), Lactobacillus fermentum (Rayavarapu et al., 2019), and Lactococcus lactis (Santos-Espinosa et al., 2020) have been found to possess the ability to produce GABA. GABA-rich fermented foods have also been studied. For example, soy yogurt was fermented with L. plantarum. The results demonstrated its efficacy in restoring insulin-producing function in hyperglycemic mice and promoting the re-establishment of a healthy gut microbiota (Weng et al., 2023). Besides, co-culture strategies are also employed as significant methods to enhance fermentation quality and promote the accumulation of GABA. An illustrative instance involved the fermentation of turmeric and roasted soybean flour using the microorganism strains Bacillus subtilis HA and L. plantarum K154. In the final stage of fermentation, co-fermentation exhibited a notably higher GABA accumulation, surpassing the single-fermented system by as much as 1.78% (Lim et al., 2016).
Currently, the majority of research on GABA-enriched fermented foods has primarily centered on milk and vegetable juice (Maria Ramos and Maria Poveda, 2022; Jin et al., 2023; Weng et al., 2023). Limited attention has been directed toward fermented vegetables and this is particularly true for bamboo shoots. Meanwhile, reports on the synergistic fermentation of bamboo shoots, providing both quality and functionality, are quite scarce. In our study, L. plantarum R1 and L. brevis R2 were selected based on their commendable potential probiotic attributes and noteworthy GABA production, respectively. We endeavored to enhance the GABA content in fermented bamboo shoots through a mixed fermentation process involving these two strains. Concurrently, we aimed to achieve enhanced nutritional quality while ensuring the safety and desirable sensory attributes. This strategic approach might hold promise for the development of functional fermented bamboo shoots.
2 Materials and methods
2.1 Isolation of LAB strains
LAB strains were isolated from fermented bamboo shoots obtained from Liuzhou, Guangxi, China. The isolation process involved serial dilutions of 1 mL of fermented bamboo shoot liquid using sterile saline (0.9% NaCl, w/v). Then it was plated onto Chalmers (MC) agar medium (Hope Bio-Technology Co., Ltd., Qingdao, China). The plates were then incubated at 37°C for 48 h. After the incubation period, single colonies with transparent circle were selected and streaked onto de Man Rogosa Sharpe (MRS) agar medium (Hope Bio-Technology Co., Ltd., Qingdao, China) to obtain pure cultures. The isolated strains were initially identified using Gram staining and peroxidase tests. Subsequently, the pure cultures were preserved by storing them at −80°C with the addition of 40% glycerol for further analysis.
2.2 Molecular identification
DNA extraction from the isolates was extracted using the Bacteria DNA Isolation Mini Kit (Vazyme Biotech Co., Ltd., Nanjing, China). PCR reactions were carried out in a total volume of 50 μL, containing 25 μL of Mix (Vazyme Biotech Co., Ltd., Nanjing, China), 21 μL of sterilized ultrapure water, 2 μL of forward primer 27F (5’-AGAGTTTGATCCTGGCTCAG-3′), 2 μL of reverse primer 1492R (5’-GGTTACCTTGTTACGACTT-3′), and 5 μL of DNA template. The PCR amplification procedure was performed using a XP Thermal Cycler (Bioer Technology Co., Ltd., Hangzhou, China). The PCR conditions were as follows: an initial denaturation step of 95°C for 5 min, followed by 30 cycles of denaturation at 95°C for 30 s, annealing at 50°C for 30 s, and extension at 72°C for 90 s. Finally, there was a single extension step at 72°C for 10 min. The PCR product was purified and sequenced by Tsingke Biotech Co., Ltd., Beijing, China. BLAST analysis was conducted using the NCBI database.1
2.3 Potential probiotic characterization
2.3.1 Acid and bile salts tolerances
The acid and bile salt tolerances of the LAB strains were performed as described by Xia et al. (2021) with slight modifications. To assess tolerance to acidic conditions, the MRS broth was adjusted to pH 2.5 and pH 3.5 using hydrochloric acid. Tolerance to bile salts was examined by supplementing the MRS broth with 0.3 and 0.5% (w/v) of bile salts (Wokai Biotechnology Co., Ltd., Beijing, China). The LAB cells cultured for 24 h were washed three times with sterile phosphate buffer saline (PBS) of pH 7.4 and resuspended in PBS. Subsequently, 100 μL of the bacterial suspension was mixed with 10 mL of modified MRS broth (MRS adjusted pH or supplemented with bile salts) and incubated for 3 h at 37°C. To determine the viable cells, a plate count method was employed using MRS agar, and the results were expressed as log colony forming unit (CFU) /mL. The survival rate was calculated using the following equation:
where Log N is the log number of viable cells at the end of the test and Log N0 is the log number of initial viable cells.
2.3.2 Antimicrobial activity
The antimicrobial activity was measured using the agar well diffusion method (Abdollahzadeh et al., 2014). The LAB cells were incubated overnight and the supernatant was collected by centrifugation at 1,902 × g for 15 min. Indicator strains including Escherichia coli ATCC35218, Salmonella enteritidis, Pseudomonas aeruginosa ATCC27853, and Staphylococcus aureus ATCC6538 were selected as common pathogens. The overnight culture of indicator bacteria was spread evenly on LB agar (Hope Bio-Technology Co., Ltd., Qingdao, China). Then wells were prepared using a 0.8 cm Oxford cup and 150 μL of the supernatant was added. After incubating at 37°C for 12 h, the diameter of the inhibition zone was measured. Uninoculated MRS broth was used as a control in the experiment.
2.3.3 Cell surface hydrophobicity
The determination of cell surface hydrophobicity was conducted following the method described by Albayrak and Duran (2021). Briefly, the LAB cells were suspended in PBS to obtain an OD600 of 0.8 (A0). Subsequently, 3 mL of the cell suspension was added to 1 mL of xylene and ethyl acetate, respectively, vortexed for 2 min, and allowed to stand at 37°C for 1 h. The absorbance (At) of the aqueous phase at 600 nm was determined. Cell surface hydrophobicity (%) was calculated according to the following formula:
2.3.4 Auto-aggregation assay
The auto-aggregation assay of the LAB cells was performed based on the method previously described (Albayrak and Duran, 2021) with slight modifications. The cell suspensions were adjusted to approximately an OD600 of 0.8 (A0). Subsequently, a total of 3 mL of the cell suspensions were transferred to a test tube, vortexed for 10 s and kept at 37°C for 6 h. The absorbance (At) of the upper liquid layer at 600 nm was determined at 2 and 6 h, respectively. Auto-aggregation (%) was calculated according to the following formula:
2.3.5 Antibiotic susceptibility
The antibiotic susceptibility testing was performed using the Kirby Bauer Disk diffusion method (García-Núñez et al., 2022). The strains were cultured in MRS broth to a concentration of 108 CFU/mL and spread evenly on the MRS agar plates. Antibiotic disks (Oxoid Ltd., Basingstoke, Hans, United Kingdom) containing various antibiotics, including Amikacin (30 μg), Chloramphenicol (30 μg), Kanamycin (30 μg), Sulfamethoxazole/Trimethoprim (25 μg), Ampicillin/Sulbactam (20 μg), Tetracycline (30 μg), Cefotaxime (30 μg), and Nalidixic Acid (30 μg) were placed on the agar surface. The plates were then incubated at 37°C for 24 h. The diameter of the inhibition zone was measured, and the results were interpreted as follows: resistant (≤ 15 mm), intermediate (15–21 mm), or susceptible (≥ 21 mm).
2.4 GABA measurement
LAB strains were cultured in MRS broth supplemented with 1% (w/v) monosodium glutamate (MSG) for 24 h. Then the culture supernatant was obtained through centrifugation at 6,010 × g for 5 min. To conduct the initial screening of GABA-producing strains, thin-layer chromatography (TLC) was employed (Park et al., 2021). A developing solvent composed of butyl alcohol, glacial acetic acid, and distilled water in a ratio of 4:1:1 (v:v:v) was used. Visualization of the TLC plates was carried out using a 0.5% (w/v) ninhydrin solution.
Quantitative analysis of GABA was conducted using an ultra-performance liquid chromatography (UPLC) method (Kim et al., 2022). An Acquity UPLC® system (Waters Corporation Inc., Milford, United States) coupled with a 2,996 photodiode array detector was employed. The supernatant obtained from the LAB strains was derivatized with o-phthalaldehyde (OPA) (Macklin, Biochemical Co., Ltd., Shanghai, China) for 2 min, followed by filtration using a 0.45 μm filter. The analysis of GABA content was performed using an InfinityLab Poroshell 120 EC-C18 column (3.0 × 150 mm, 2.7 μm) (Agilent Technologies Inc., Santa Clara, United States). The mobile phase solution system was comprised of (A) 5 g/L of sodium acetate at pH 7.2 and (B) a mixture of acetonitrile, methanol, and sodium acetate in a ratio of 40:40:20 (v:v:v) at pH 7.2. The gradient elution conditions of mobile phase A were applied as following: 0 min, 92%; 12 min, 50%; 15 min, 50%; 18 min, 92%. The system was operated at a flow rate of 0.8 mL/min at 30°C with detection performed at a wavelength of 340 nm.
2.5 Bamboo shoots fermentation
2.5.1 Sample preparation
L. plantarum R1 and L. brevis R2 were suspended with PBS for use as fermentation agents. Fresh bamboo shoots were sourced from Guangdong, China. After cleaning, old roots were removed, and the shoots were peeled and cut into uniform strips. The bamboo shoots were placed in sterilized 200 mL glass jars at a ratio of 1:2 (w:v) with cooled boiling water. L. plantarum R1, L. brevis R2, mixture of L. plantarum R1 and L. brevis R2 (1:1) were inoculated with 3% (v/v) of the total inoculum, respectively. The natural fermentation group did not receive any LAB inoculation. The glass jars were then sealed and the fermentation was conducted at room temperature (26°C) for a duration of 14 days.
2.5.2 Determination of pH value and total titratable acidity
During the fermentation process, samples were collected every 2 days for pH and TTA analysis. The bamboo shoots (5 g) were homogenized with 10 mL of water and centrifuged at 6,010 × g for 5 min. The pH of the supernatant was measured using a pH meter (INESA Co., Ltd., Shanghai, China). The determination of TTA was conducted as described by Zhao et al. (2022) and the TTA was expressed as g/100 g with lactic acid as a standard.
2.5.3 Determination of nitrite content
For nitrite analysis, the bamboo shoots (5 g) were used to precipitate proteins with a combination of potassium ferricyanide and zinc acetate. Afterward, it was set aside to allow for the removal of fat. The resulting supernatant, obtained after filtration, was employed to determine nitrite content using the N-(1-naphthyl)-ethylenediamine dihydrochloride spectrophotometric method according to GB 5009.33–2016 in China.
2.5.4 Determination of GABA content
After 14 days of fermentation, 5 g of the bamboo shoots were mixed with 10 mL of water, subjected to ultrasound to promote dissolution, and then filtered through a 0.22 μm filter membrane to collect the filtrate. The filtrate was further derivatized using OPA and the content of GABA was determined using the UPLC method.
2.5.5 Determination of nutritional composition
Following a 14-day fermentation period, the protein, total sugars, reducing sugars, fat, and crude fiber contents in bamboo shoots were assessed. Protein content was determined using Bradford reagent (Sangon Biotech Co., Ltd., Shanghai, China). The anthrone method was employed for the quantification of total sugars, while reducing sugars were assessed using the dinitrosalicylic acid method (Singhal et al., 2021). The determination of fat content adhered to the methodology outlined by Hellwig et al. (2022). The evaluation of crude fiber content was measured subsequent to acid and alkali treatment, following the procedure described by Azeez et al. (2022).
2.5.6 Sensory analysis
After a 14-day fermentation period, the bamboo shoots were selected for sensory evaluation. A total of 5 descriptors containing color, flavor, taste, texture, and overall acceptability were selected. The blind evaluation panel comprised 10 judges (5 men and 5 women) experienced in sensory evaluation. Scores were assigned on a scale ranging from 1 (dislike extremely) to 10 (like extremely).
2.6 Statistical analysis
Principal component analysis (PCA) and heat map were performed using the combination of SPSS 26.0 (IBM Inc., United States) and OriginPro 2023b (OriginLab Corporation, Northampton, United States). Data were normalized before applying the feature selection algorithms and weighting results presented between 0 and 1, in which weight closer to 1 indicated the priority of the studied strains. All experiments were repeated three times and all data were expressed as means ± standard deviation. The statistical analysis was completed using SPSS 26.0 (IBM Inc., United States) and significant differences between groups were evaluated by the Duncan test. The statistical significance level was defined as p < 0.05.
3 Result and discussion
3.1 Isolation and molecular identification of LAB strains
Insoluble calcium carbonate in MC medium can be converted to calcium lactate by lactic acid produced by LAB strains, resulting in the appearance of transparent circles. Thirty-five strains that could produce large transparent circles were isolated, all of which were Gram-positive, catalase-negative, and rod-shaped or globular. Based on the similar phenotypes, 20 isolates were selected for 16S rRNA gene identification and the sequences were analyzed using BLAST. As shown in Table 1, the isolates were identified as Lactiplantibacillus plantarum, Levilactobacillus brevis, Pediococcus acidilactici, Pediococcus pento-saceus, Limosilactobacillus fermentum, and Lacticaseibacillus paracasei. Sequences have been submitted to the NCBI database.
3.2 Potential probiotic properties
3.2.1 Acid and bile salts tolerance
The journey of lactic acid bacteria through the gastrointestinal tract confronts a series of survival challenges, including exposure to gastric acid, digestive enzymes, and bile salts. The capacity to withstand low pH levels and bile salts is especially imperative for the bacteria. All LAB strains displayed remarkable acid tolerance (Figure 1A). When cultured at pH 2.5 and pH 3.5 for 3 h, all strains demonstrated robust acid tolerance, with survival rates surpassing 70%. Additionally, the cell densities of all strains remained within the range of 106–108 CFU/mL. For example, L. plantarum R1 and L. brevis R2 achieved survival rates of 77.49 and 76.17%, respectively, at pH 2.5. On the other hand, there were significant differences in the tolerance of LAB isolates to bile salts (p < 0.05) (Figure 1B). P. pentosaceus, L. fermentum, and L. paracasei exhibited poor bile salts tolerance, with survival rates at approximately 50% when exposed to 0.3% bile salts. In contrast, other strains displayed higher bile salts tolerance, with P. acidilactici R9 and L. plantarum L1 demonstrating the highest bile salts tolerance, as their survival rates remained above 80% even in the presence of 0.5% bile salts. And other strains such as L. plantarum R1 and L. brevis R2 had greater than 70% survival in 0.5% bile salts. Indeed, extensive studies have consistently demonstrated that the tolerance of LAB to acid and bile salts varies significantly based on the species and specific strains being investigated. Experiments involving the exposure of LAB to acid and bile salts have demonstrated that some strains exhibited survival rates of up to 80% or higher. Conversely, there were strains that displayed limited survivability under these conditions (Tokatlı et al., 2015; Wang et al., 2021; Khushboo et al., 2023).
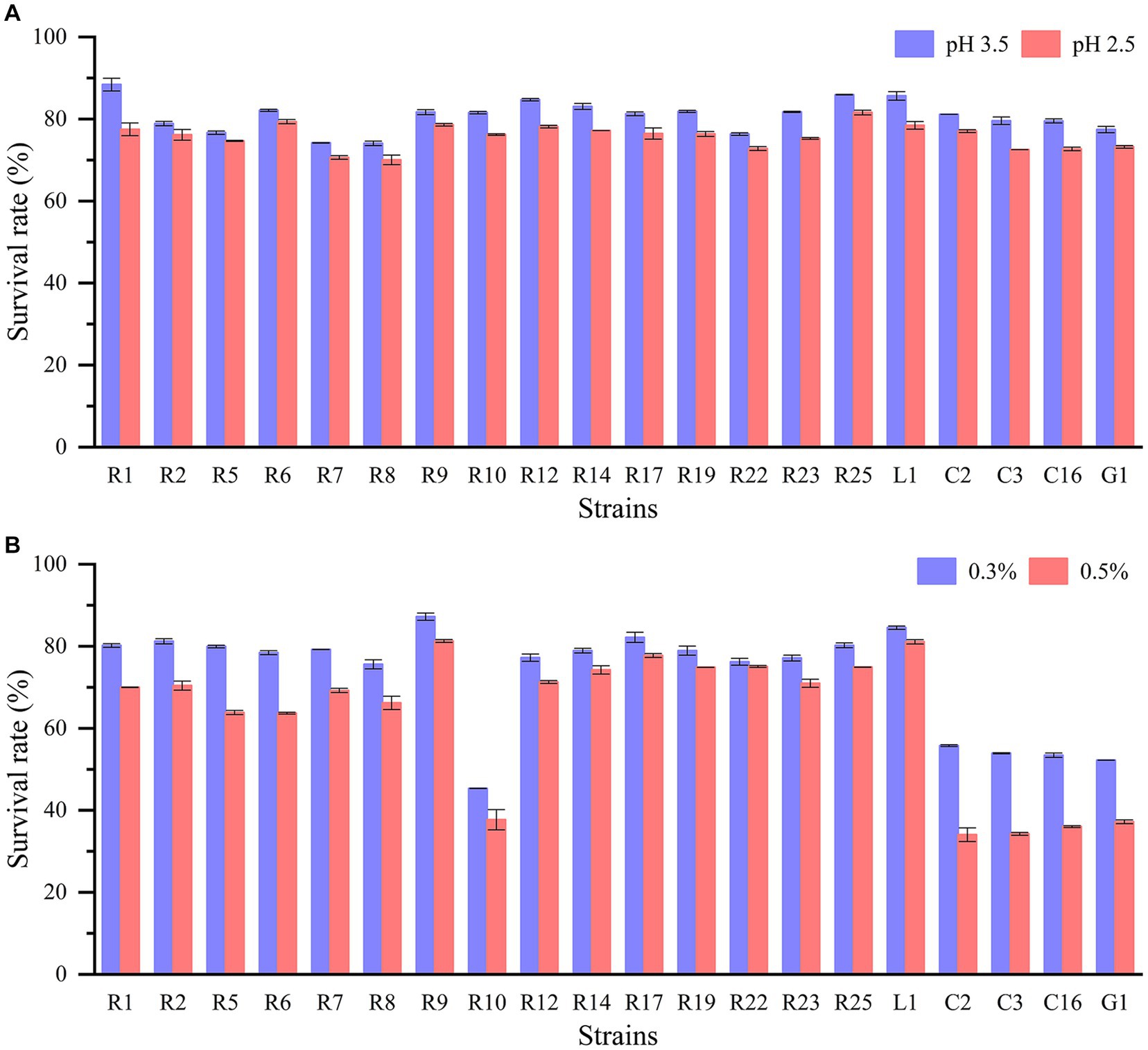
Figure 1. Acid and bile salt tolerance of LAB strains: (A) survival rate in pH 3.5 and pH 2.5; (B) survival rate in 0.3 and 0.5% bile salt.
3.2.2 Antimicrobial activity
One of the notable advantages of LAB is their capacity to inhibit the growth of foodborne pathogens. This inhibitory effect arises primarily from the production of various bioactive compounds, including organic acids, bacteriocins, and hydrogen peroxide (Chen J. et al., 2022). As shown in Table 2, the antimicrobial ability of the strains showed significant differences (p < 0.05). L. plantarum exhibited the most pronounced inhibitory effect against all four pathogens. P. acidilactici R9 and P. pentosaceus R10 also exhibited strong inhibitory abilities, with their inhibitory effects being more prominent against Gram-negative bacteria compared to Gram-positive bacteria. However, L. fermentum and L. paracasei displayed the weakest inhibitory abilities, particularly against Gram-positive bacteria, which is similar to the findings of Trindade et al. (2022) and Younas et al. (2022). This is seemingly attributed to the ability of LAB in inhibiting or eradicating biofilms (Shokri et al., 2018; Ibrahim et al., 2021), a mechanism that proves more challenging when confronted with the thicker peptidoglycan cell wall structure characteristic of Gram-positive bacteria.
3.2.3 Cell surface hydrophobicity and auto-aggregation
The cell surface hydrophobicity and auto-aggregation ability of LAB strains are closely linked to their capacity to adhere to epithelial cells and carry out their functional roles. Significant differences (p < 0.05) in cell surface hydrophobicity were observed among different strains (Figure 2A). L. paracasei G1 displayed relatively high hydrophobicity in both ethyl acetate and xylene, exceeding 50%. Following closely were L. plantarum R1 and R6, both exhibiting hydrophobicity levels surpassing 40%. Notably, L. brevis R5, R7, and R8 showed substantial disparities with hydrophobicity exceeding 80% in ethyl acetate but falling below 30% in xylene. In contrast, P. acidilactici R9 and P. pentosaceus R10 displayed minimal hydrophobicity. Figure 2B illustrates the auto-aggregation ability of the isolates. The rate of auto-aggregation increased over time for all strains. Among the strains tested, L. plantarum R6 exhibited the most notable auto-aggregation ability, achieving a rate of 49.18% after a 6-h standing period. In stark contrast, L. brevis R2 demonstrated the lowest auto-aggregation ability, registering only 5.39% after 6 h. Wang et al. (2021) reported that the hydrophobicity in xylene and auto-aggregation of the commercial strain L. rhamnosus GG were 14.35 and 20.35%, respectively. In another study, the hydrophobicity in xylene of LAB strains isolated from artisanal white cheese ranged from 1 to 41.6%. And hydrophobicity in ethyl acetate showed a broader range from 8.2 to 50.2%. Interestingly, there was no significant correlation between these two solvents (Albayrak and Duran, 2021). It has been demonstrated that the adhesion properties of LAB are typically strain-specific rather than species-specific, and are subject to influence by factors such as growth conditions, incubation time, and bacterial surface structure (García-Cayuela et al., 2014).
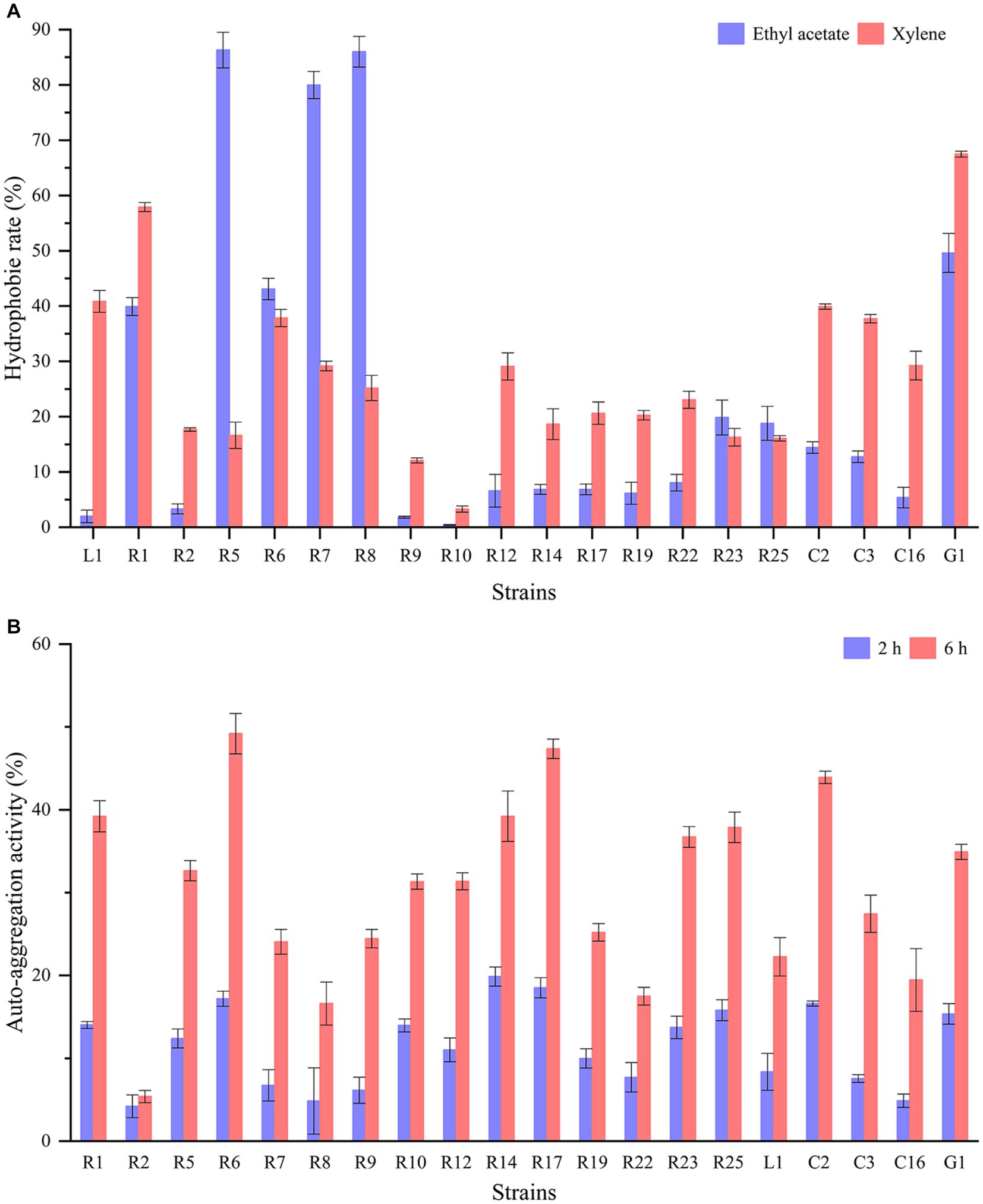
Figure 2. Hydrophobicity and auto-aggregation of LAB strains: (A) hydrophobicity in ethyl acetate and xylene; (B) auto-aggregation after 2 h and 6 h.
3.2.4 Antibiotic susceptibility
The emergence of microbial resistance, attributed to the widespread use of antibiotics, poses a significant threat in various fields including medicine and food production. The antibiotic susceptibility of the isolates is presented in Table 3. It is noteworthy that all strains demonstrated either no susceptibility or poor susceptibility to antibiotics including Kanamycin, Nalidixic Acid, and Amikacin. Furthermore, all strains excluding L. plantarum also exhibited no susceptibility to Sulfamethoxazole/Trimethoprim. In contrast, Tetracycline, Chloramphenicol, Ampicillin/Sulbactam, and Cefotaxime were found to be effective in inhibiting the growth of all strains. Same as our findings, studies have found that LAB strains possess resistance to antibiotics such as Nalidixic Acid, Kanamycin, Amikacin, Gentamicin, and Streptomycin (García-Núñez et al., 2022; Duche et al., 2023). Fortunately, this resistance is considered as an inherent characteristic of LAB. Researchers have confirmed that some resistance is intrinsic to LAB, as resistance genes are encoded within their chromosomes and do not undergo horizontal transmission (Argyri et al., 2013). There are also instances where LAB exhibit apparent resistance despite lacking the associated resistance genes (Ou et al., 2022). Additionally, it has been assumed that membrane impermeability may also contribute to the resistance(Elkins and Mullis, 2004). Conversely, numerous studies have noted that LAB strains display sensitivity to Tetracycline, Chloramphenicol, and Cefotaxime (Albayrak and Duran, 2021; García-Núñez et al., 2022; Ou et al., 2022; Duche et al., 2023), with variations in susceptibility observed among different strains and species toward the same antibiotic.
3.2.5 Selection of LAB strains with favorable potential probiotic properties
PCA and heat map have emerged as pivotal tools for the screening of functional strains (Wang et al., 2021). To comprehensively assess and compare the properties of the isolates for the selection of probiotic candidates for bamboo shoot fermentation, we employed PCA, considering six attributes: acid resistance, bile salt resistance, antimicrobial activity, cell surface hydrophobicity, auto-aggregation, and antibiotic susceptibility. The same attribute with multiple results was compared using the comprehensive rating method with equal weight assigned to each result. As shown in Figure 3A, principal component 1 (PC1) and principal component 2 (PC2) occupied 46.0 and 26.2% of the variance, respectively. The distribution of the strains was observed across four quadrants. L. plantarum were densely concentrated in quadrants one and four, whereas L. fermentum, L. brevis, and L. paracasei were primarily distributed in quadrants two and three. Additionally, a heat map was utilized to visualize the properties of LAB strains (Figure 3B). The strains were categorized into four groups: A, B, C and D. Group A exhibited notable cell hydrophobicity but limited acid tolerance and antimicrobial activity, while group B displayed low bile salt tolerance. Group D excelled in all other characteristics, except for hydrophobicity. Intriguingly, all members of Group D were identified as L. plantarum. Based on the combined insights from PCA and the heatmap, we concluded that L. plantarum R1 showcased the most potential probiotic properties among the tested strains. Consequently, L. plantarum R1 was selected as one of the strains for subsequent bamboo shoot fermentation.
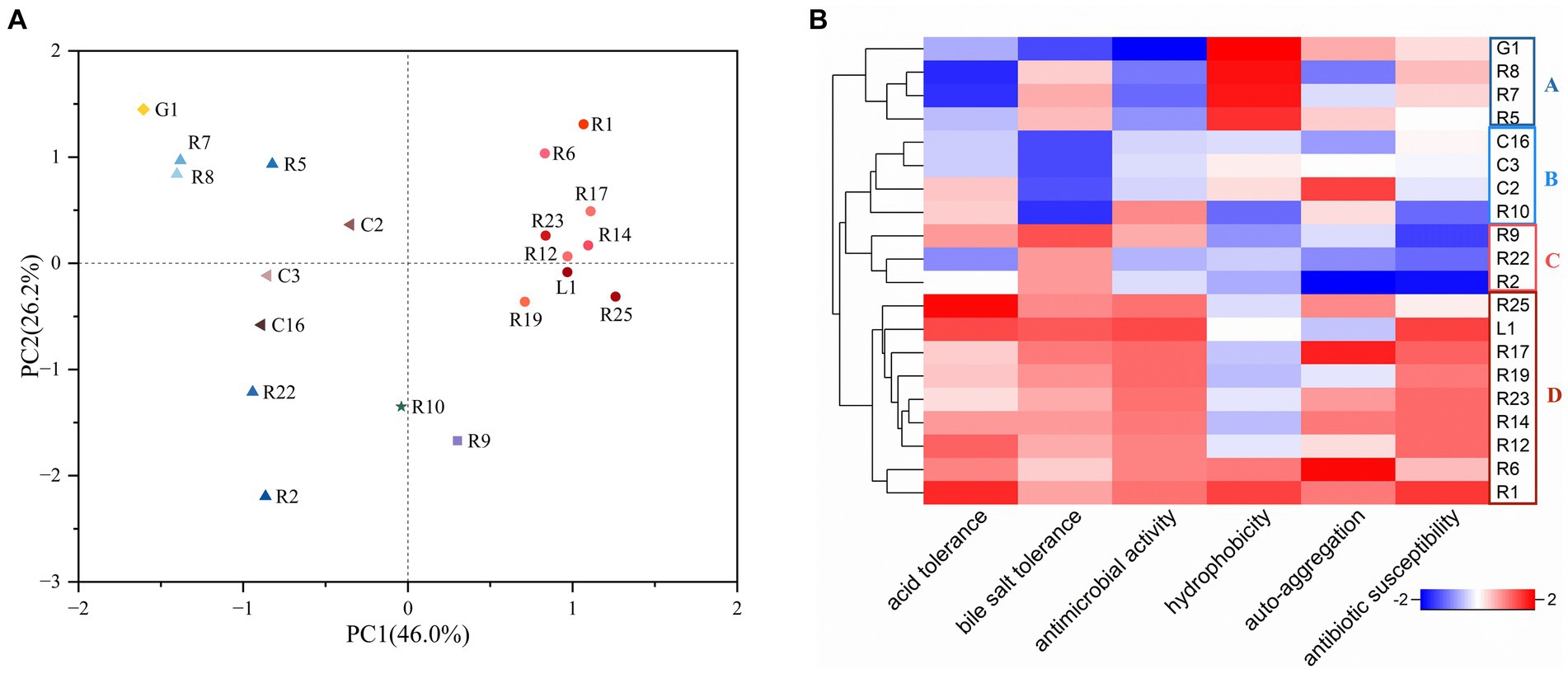
Figure 3. Selection of LAB strains with favorable probiotic properties (acid and bile salt tolerance, antimicrobial activity, hydrophobicity, auto-aggregation, and antibiotic susceptibility). (A) Principal component analysis (PCA), the percentages: the values contributed by the principal components to the sample differences; (B) Heat map, the redder the color is, the better the probiotic property is.
3.3 Screening of LAB strains for high GABA production
The preliminary screening of GABA-producing strains was conducted through TLC (Figure 4A). All strains displayed spots that closely matched the position of the GABA standard. Notably, the spot corresponding to L. brevis R2 exhibited the most pronounced coloration, followed by L. brevis R22. Following the initial screening, a quantitative assessment of GABA production was carried out using UPLC method, as depicted in Figures 4B,C. L. brevis R2 emerged as the most prolific GABA producer, showcasing a significantly high GABA production level (p < 0.01) of 913.99 ± 14.2 mg/L. Furthermore, L. brevis R22 also demonstrated a noteworthy distinction in GABA production compared to the other strains, with a recorded production level of 406.79 ± 0.81 mg/L. Consequently, L. brevis R2 was selected as another strain for subsequent bamboo shoot fermentation.
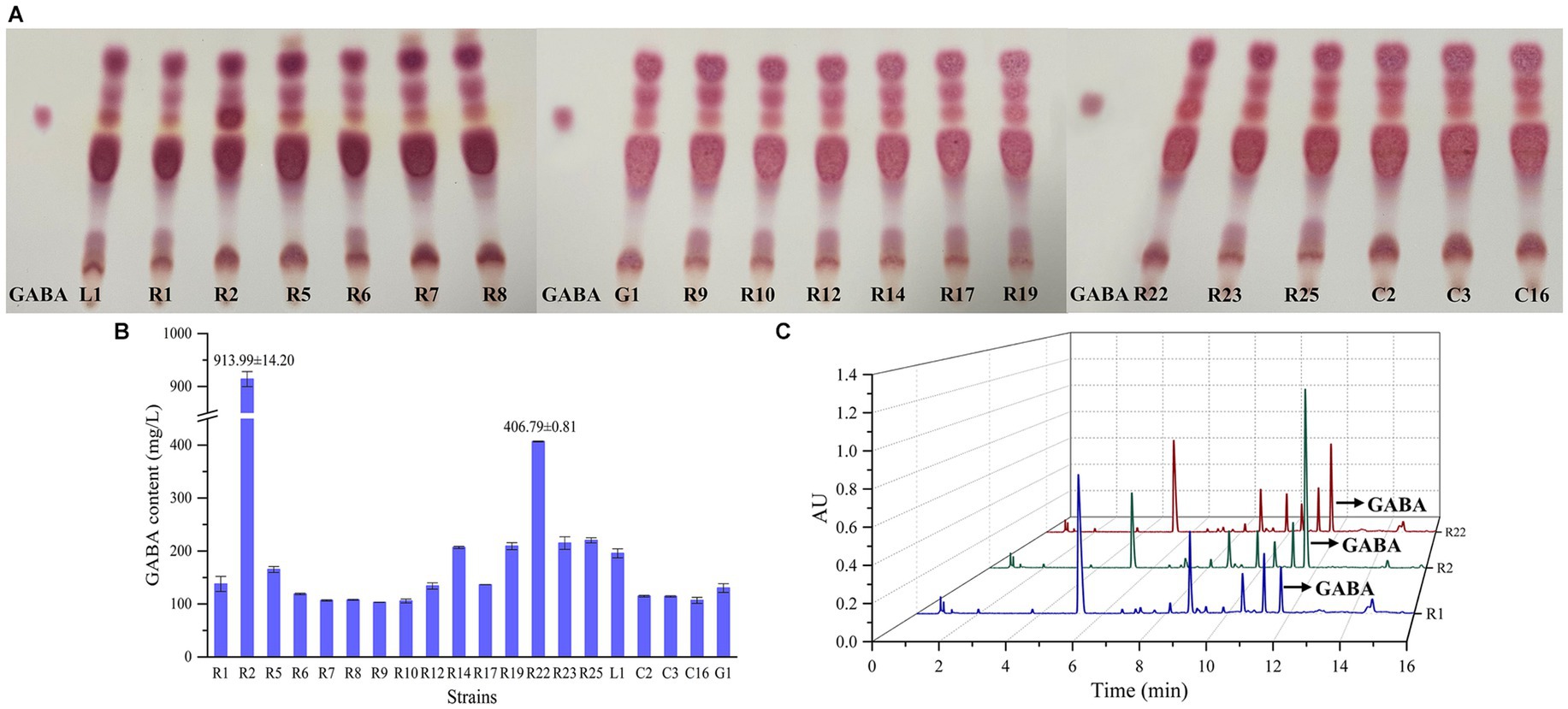
Figure 4. Screening of GABA-producing LAB strains: (A) thin layer chromatography (TLC) screening of GABA–producing LAB; (B) GABA content (μg/mL) of LAB using ultra-performance liquid chromatography (UPLC) method; (C) chromatographic profile of GABA content in supernatants of L. plantarum R1, L. brevis R2 and L. brevis R22 analyzed, the retention time of GABA is about 11.7 min.
GABA-producing LAB have been extensively screened from a variety of fermented foods due to their remarkable ability to convert L-glutamic acid into GABA. For instance, 262.06 ± 15.42 mg/L GABA was successfully produced by L. brevis DSM 32386 isolated from traditional “wild” Alpine cheese (Mancini et al., 2019). In another study, L. plantarum FNCC 260 isolated from fermented cassava was found to produce 809.2 mg/L of GABA after 60 h of culture (Yogeswara et al., 2021). Furthermore, several strains including L. fermentum, P. pentosaceus, and L. brevis, identified from various fermented foods, have shown the capability to produce GABA at high yields (Rayavarapu et al., 2019; Pakdeeto et al., 2022). It has been noted that all LAB strains possessing the GAD gene exhibit the capacity to synthesize GABA (Pannerchelvan et al., 2023). Additionally, the yield of GABA is influenced by various factors such as concentration of L-glutamic acid, pH, temperature, and the size of inoculum.
3.4 Bamboo shoots fermentation
3.4.1 Changes in pH and TTA during bamboo shoots fermentation
In fermented foods, pH and TTA plays a crucial role in determining taste and serves as a key indicator of maturity. As shown in Figure 5A, the pH levels in all groups experienced a rapid decline in the initial 2 days. Notably, the pH in the inoculated fermentation was lower than that in the natural fermentation, with L. plantarum R1 exhibiting the most pronounced effect on pH reduction. By the 4th day, pH levels began to increase and gradually stabilized within the range of 3.7–4.3. At the end of fermentation, the pH values were 4.26 for naturally fermented bamboo shoots (NFB), 3.79 for inoculated L. plantarum R1 (IFB-R1), 4.00 for inoculated L. brevis R2 (IFB-R2), and 3.79 for synergistic fermentation by both strains (IFB-R1 + R2). The TTA changes shown in Figure 5B revealed a trend of rapid increase followed by a gradual leveling off in all groups. Inoculation with L. plantarum R1 resulted in the most significant increase in TTA, reaching a peak value of 1.23 g/100 g on the 10th day of fermentation, which was 1.8 times higher than that of natural fermentation. This was followed by the synergistic use of L. plantarum R1 and L. brevis R2 with a peak value of 1.11 g/100 g. Our findings demonstrate that inoculated fermentation led to a more substantial reduction in pH and increase in TTA, effectively shortening the fermentation cycle compared to natural fermentation. This aligns with prior studies (Zhang et al., 2022; Chen et al., 2023). The introduction of LAB strains swiftly established dominance in the fermentation process. Their growth and metabolic activity resulted in the production of substantial acidic compounds, consequently lowering the pH values and inhibiting the growth of pathogenic microorganisms throughout the fermentation process. In addition, L. plantarum R1 exhibited higher acid production capability compared to L. brevis R2. This is attributed to the fact that L. plantarum R1, a homofermentative strain, solely converted glucose to lactic acid during fermentation. On the other hand, the heterofermentative strain, L. brevis R2, produced additional products such as carbon dioxide and ethanol alongside lactic acid.
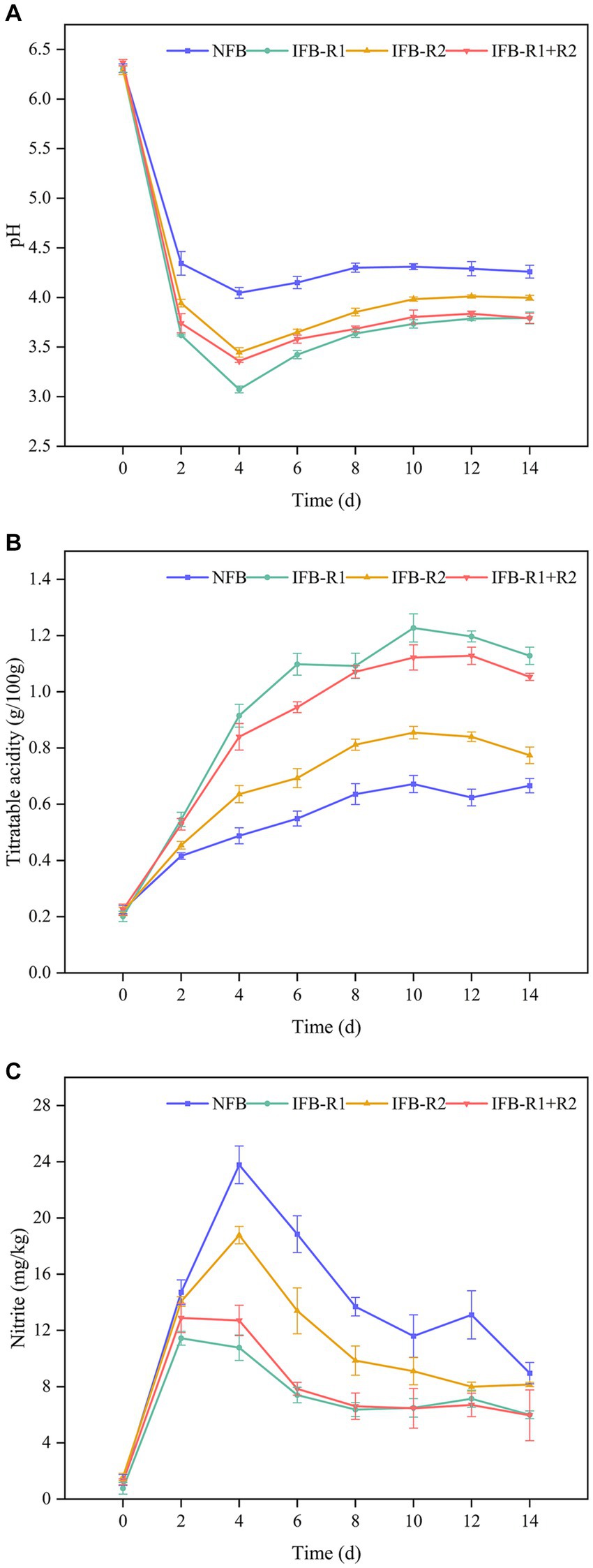
Figure 5. pH, total titratable acidity (TTA) and nitrite content of bamboo shoots during fermentation process: (A) pH values; (B) TTA; (C) nitrite content. NFB: naturally fermented bamboo shoots; IFB-R1: fermented bamboo shoots inoculated with L. plantarum R1; IFB-R2: fermented bamboo shoots inoculated with L. brevis R2; IFB-R1 + R2: fermented bamboo shoots inoculated with L. plantarum R1 and L. brevis R2.
3.4.2 Changes in nitrite during bamboo shoots fermentation
Excessive intake of nitrite can lead to methemoglobinemia and elevate the risk of gastrointestinal cancers, underscoring the critical need to control nitrite levels in fermented bamboo shoots. As illustrated in Figure 5C, in the initial stages of fermentation, nitrite levels exhibited a gradual increase, with the addition of LAB strains demonstrating a mitigating effect, notably more pronounced with the use of L. plantarum R1. Subsequently, nitrite levels decreased, reaching 8.95 ± 0.76 mg/kg (NFB), 6.00 ± 0.27 mg/kg (IFB-R1), 8.15 ± 0.15 mg/kg (IFB-R2), and 5.96 ± 1.81 mg/kg (IFB-R1 + R2) at the end of fermentation. It is evident that the addition of L. plantarum R1 proved more effective in controlling nitrite levels, whether in mono-fermentation or co-fermentation. Fortunately, all four sets of data complied with the Chinese regulatory limits for nitrite content in fermented vegetables (< 20 mg/kg, GB 2762–2022). Consistent with our findings, studies by Wu R. et al. (2015) and Yu et al. (2023) also observed a pattern of initial nitrite increase followed by a decline during vegetable fermentation. This phenomenon might be attributed to the activity of nitrate-reducing microorganisms capable of converting nitrate to nitrite under anaerobic conditions in the early stages of fermentation. However, as fermentation progresses, LAB assumed dominance as the primary flora, which created an acidic environment that not only inhibited the growth of nitrate-reducing bacteria but also contributed to nitrite metabolism.
3.4.3 Changes in GABA during bamboo shoots fermentation
As shown in Figure 6, it was observed that the mono-inoculation of L. plantarum R1 did not lead to a significant increase in GABA content in bamboo shoots after 14 days of fermentation (p > 0.05). However, the introduction of L. brevis R2 alone resulted in an elevated GABA content of 349.60 ± 10.20 mg/kg, and the combined inoculation of L. plantarum R1 and L. brevis R2 further increased the GABA content to 382.31 ± 12.17 mg/kg. This represented an increase of over four times compared to natural fermentation (70.27 ± 6.04 mg/kg). Similarly, Moore et al. (2021)reported an enhanced concentration of GABA in cucumbers through the fermentation process, while Hirose et al. (2021) observed a substantial rise in GABA concentration in pickled luffa when inoculated with L. brevis (from 5.3 mg/100 g to 23.8 mg/100 g).
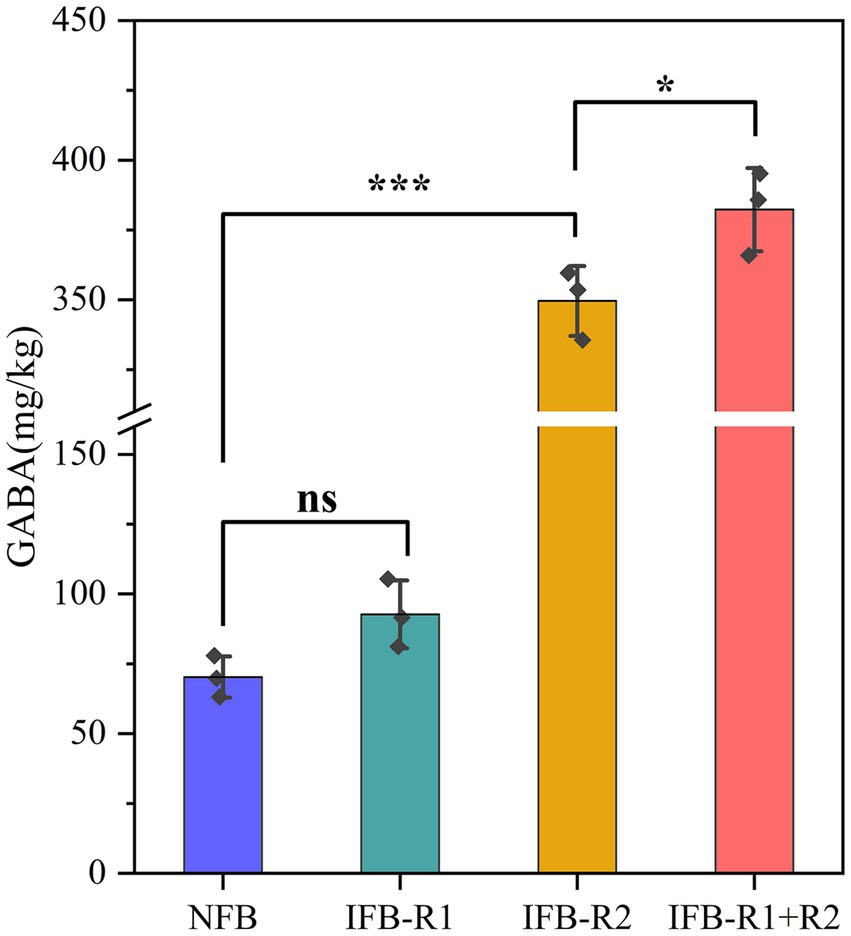
Figure 6. GABA content of bamboo shoots after 14-day fermentation. Significant differences are marked with asterisks between groups (“ns” means p > 0.05; “*” means p < 0.05; “***” means p < 0.001).
It is evident that the co-fermentation of the two strains greatly promotes GABA production. In a study focused on fermented milks, the co-culture of Streptococcus thermophilus and L. brevis demonstrated increased GABA production (Wu Q. et al., 2015). Similarly, another study showed that co-fermentation with Leuconostoc mesenteroides SM and L. plantarum K154 led to a notable increase in GABA content (Kwon et al., 2016). The production of GABA and the activity of GAD are intricately linked. Liu et al. (2023) concluded that the optimum pH for GAD is 3.6, while the ideal pH values may vary somewhat depending on the source of GAD, it generally functions more effectively under acidic conditions. We deduce that L. plantarum R1 creates a conducive acidic environment, thereby enhancing the activity and expression of GAD. At the same time, the substantial production of GABA contributes to the slight rise in pH during fermentation. The transport of glutamate for intracellular synthesis of GABA, facilitated by specialized transporter proteins, as well as the release of GABA into the extracellular environment, leads to the consumption of H+, resulting in an increase in ambient alkalinity (Cataldo et al., 2020). Furthermore, it has been proposed that traditional fermenters have a heightened efficiency in breaking down proteins into polypeptides. This provides L. brevis with ample nutrients to promote its growth. However, it should be noted that the precise mechanism by which GABA production is increased through the co-cultivation of L. plantarum R1 and L. brevis R2 still needs to be further investigated. In conclusion, the co-inoculation of L. plantarum R1 and L. brevis R2 for bamboo shoot fermentation emerges as an optimal approach to increase the GABA content in bamboo shoots.
3.4.4 Nutritional composition of fermented bamboo shoots
The above experiments revealed that the co-inoculation of the two strains proved more advantageous for the fermentation of bamboo shoots compared to the mono-inoculation. To further investigate the nutritional qualities of the fermented bamboo shoots, protein, total sugars, reducing sugars, fat and crude fiber were analyzed. As shown in Table 4, the protein content of bamboo shoots increased during fermentation. NFB and IFB-R1 + R2 showed increases of 0.47 g/100 g and 0.66 g/100 g, respectively, compared to the fresh bamboo shoots (FBS). It was observed that inoculation with LAB strains in synergistic fermentation significantly enhanced the protein content (p < 0.05), consistent with findings by Singhal et al. (2021), Azeez et al. (2022), and Wang et al. (2023). Both total and reducing sugars decreased compared to the FBS, attributed to the microbial metabolic activities during fermentation, converting sugars into alcohols and organic acids. The fat content in bamboo shoots remained low both before and after fermentation, with a slight decrease after fermentation due to active microbial activities. Regarding crude fiber, the content increased from 13.92 g/100 g to 15.80 g/100 g and 16.58 g/100 g after natural fermentation and inoculated fermentation, respectively. Similar observations were made in other studies, such as oat bran, where crude fiber content increased from 14.36 to 15.83% through fermentation (Mustafa et al., 2022). Abdul Rahman et al. (2017) also found an increase in acid detergent fiber and neutral detergent fiber through fermentation. This suggests that fermented bamboo shoots are a promising option for low-fat and high-fiber foods.

Table 4. Changes in nutritional composition (g/100 g, fresh weight) of bamboo shoots before and after fermentation.
3.4.5 Sensory quality of fermented bamboo shoots
To further investigate the sensory qualities of the co-fermented bamboo shoots, ten judges were invited to perform sensory evaluations. As depicted in Figure 7, a significant difference in color evaluation between NFB and IFB-R1 + R2 was observed (p < 0.05). However, there were no significant differences in flavor, taste, texture, and overall acceptability (p > 0.05). The uniform and creamy white coloration of IFB-R1 + R2 stood in stark contrast to the yellowish or partially browned appearance of NFB, which was the primary factor contributing to the higher scores. The rapid decrease in pH values during inoculated fermentation contributed to the degradation of lutein and chlorophyll, resulting in a lighter color. Conversely, the activities of enzymes like polyphenol oxidase and peroxidase appeared to increase the likelihood of browning in NFB. Regarding flavor and taste, the majority of judges noted that the acidity and freshness of IFB-R1 + R2 more closely resembled commercially available products. The short fermentation time for NFB had not yet allowed it to attain the desired level of acidity. Overall, after only 14-day fermentation, the inoculated fermented bamboo shoots exhibited superior sensory characteristics compared to naturally fermented ones.
4 Conclusion
In this study, L. plantarum R1 and L. brevis R2 were screened and identified as optimal strains for inoculating fermented bamboo shoots. The synergistic fermentation approach resulted in a substantial enhancement of GABA content in bamboo shoots compared to individual strain fermentations. Notably, this co-fermentation led to an impressive 4.5-fold surge in GABA content when compared to natural fermentation. Meanwhile, the nitrite content in bamboo shoots was reduced to a safe level of 5.96 mg/kg. Indeed, the fermentation process has led to an increase in protein and crude fiber contents and a reduction in fat content, which suggests that fermented bamboo shoots could offer a more nutritious and potentially healthier option. Sensory evaluations confirmed the superior flavor profile of co-inoculated fermented bamboo shoots. However, further optimization of the inoculation and fermentation conditions is crucial to achieve even higher quality bamboo shoots. Additionally, more experiments are warranted to validate whether GABA-enriched fermented bamboo shoots can offer enhanced health benefits. In summary, our research provides a novel strategy for bamboo shoot fermentation processing and offers fresh perspectives for the production of functional foods with health-promoting properties.
Data availability statement
The original contributions presented in the study are included in the article/supplementary material, further inquiries can be directed to the corresponding authors.
Author contributions
MC: Conceptualization, Data curation, Formal analysis, Investigation, Methodology, Software, Validation, Visualization, Writing – original draft. HX: Conceptualization, Funding acquisition, Project administration, Resources, Supervision, Writing – review & editing. XZ: Investigation, Methodology, Validation, Writing – review & editing. DT: Conceptualization, Resources, Validation, Writing – review & editing. HZ: Investigation, Methodology, Writing – review & editing. ZH: Investigation, Methodology, Writing – review & editing. AG: Conceptualization, Methodology, Project administration, Resources, Supervision, Writing – review & editing. JL: Conceptualization, Funding acquisition, Project administration, Resources, Supervision, Writing – review & editing.
Funding
The author(s) declare financial support was received for the research, authorship, and/or publication of this article. This research was funded by the Major Science and Technology Fund of Guangxi Province (AB23026035) and the Hubei Province Health and Family Planning Scientific Research Project (no. WJ2023M168).
Conflict of interest
The authors declare that the research was conducted in the absence of any commercial or financial relationships that could be construed as a potential conflict of interest.
Publisher’s note
All claims expressed in this article are solely those of the authors and do not necessarily represent those of their affiliated organizations, or those of the publisher, the editors and the reviewers. Any product that may be evaluated in this article, or claim that may be made by its manufacturer, is not guaranteed or endorsed by the publisher.
Footnotes
References
Abdollahzadeh, E., Rezaei, M., and Hosseini, H. (2014). Antibacterial activity of plant essential oils and extracts: the role of thyme essential oil, nisin, and their combination to control Listeria monocytogenes inoculated in minced fish meat. Food Control 35, 177–183. doi: 10.1016/j.foodcont.2013.07.004
Abdul Rahman, N., Abd Halim, M. R., Mahawi, N., Hasnudin, H., Al-Obaidi, J. R., and Abdullah, N. (2017). Determination of the use of Lactobacillus plantarum and Propionibacterium freudenreichii application on fermentation profile and chemical composition of corn silage. Biomed. Res. Int. 2017:e2038062. doi: 10.1155/2017/2038062
Albayrak, Ç. B., and Duran, M. (2021). Isolation and characterization of aroma producing lactic acid bacteria from artisanal white cheese for multifunctional properties. LWT 150:112053. doi: 10.1016/j.lwt.2021.112053
Argyri, A. A., Zoumpopoulou, G., Karatzas, K.-A. G., Tsakalidou, E., Nychas, G.-J. E., Panagou, E. Z., et al. (2013). Selection of potential probiotic lactic acid bacteria from fermented olives by in vitro tests. Food Microbiol. 33, 282–291. doi: 10.1016/j.fm.2012.10.005
Azeez, S. O., Chinma, C. E., Bassey, S. O., Eze, U. R., Makinde, A. F., Sakariyah, A. A., et al. (2022). Impact of germination alone or in combination with solid-state fermentation on the physicochemical, antioxidant, in vitro digestibility, functional and thermal properties of brown finger millet flours. LWT 154:112734. doi: 10.1016/j.lwt.2021.112734
Cataldo, P. G., Villegas, J. M., Savoy de Giori, G., Saavedra, L., and Hebert, E. M. (2020). Enhancement of γ-aminobutyric acid (GABA) production by Lactobacillus brevis CRL 2013 based on carbohydrate fermentation. Int. J. Food Microbiol. 333:108792. doi: 10.1016/j.ijfoodmicro.2020.108792
Chen, Y., Chen, L., Liu, L., Bi, X., and Liu, X. (2023). Characteristics of microbial communities in fermentation of pickled ginger and their correlation with its volatile flavors. Food Biosci. 53:102736. doi: 10.1016/j.fbio.2023.102736
Chen, C., Cheng, G., Liu, Y., Yi, Y., Chen, D., Zhang, L., et al. (2022). Correlation between microorganisms and flavor of Chinese fermented sour bamboo shoot: roles of Lactococcus and Lactobacillus in flavor formation. Food Biosci. 50:101994. doi: 10.1016/j.fbio.2022.101994
Chen, J., Pang, H., Wang, L., Ma, C., Wu, G., Liu, Y., et al. (2022). Bacteriocin-producing lactic acid Bacteria strains with antimicrobial activity screened from Bamei pig Feces. Food Secur. 11:709. doi: 10.3390/foods11050709
Das, R., Tamang, B., Najar, I. N., Thakur, N., and Mondal, K. (2023). First report on metagenomics and their predictive functional analysis of fermented bamboo shoot food of Tripura, north East India. Front. Microbiol. 14:1158411. doi: 10.3389/fmicb.2023.1158411
Duche, R. T., Singh, A., Wandhare, A. G., Sangwan, V., Sihag, M. K., Nwagu, T. N. T., et al. (2023). Antibiotic resistance in potential probiotic lactic acid bacteria of fermented foods and human origin from Nigeria. BMC Microbiol. 23:142. doi: 10.1186/s12866-023-02883-0
Elkins, C. A., and Mullis, L. B. (2004). Bile-mediated aminoglycoside sensitivity in Lactobacillus species likely results from increased membrane permeability attributable to cholic acid. Appl. Environ. Microbiol. 70, 7200–7209. doi: 10.1128/AEM.70.12.7200-7209.2004
García-Cayuela, T., Korany, A. M., Bustos, I., Gómez de Cadiñanos, L. P., Requena, T., Peláez, C., et al. (2014). Adhesion abilities of dairy Lactobacillus plantarum strains showing an aggregation phenotype. Food Res. Int. 57, 44–50. doi: 10.1016/j.foodres.2014.01.010
García-Núñez, I. M., Santacruz, A., Serna-Saldívar, S. O., Hernandez, S. L. C., and Amaya Guerra, C. A. (2022). Assessment of potential probiotic and Synbiotic properties of lactic acid Bacteria grown in vitro with starch-based soluble corn Fiber or inulin. Food Secur. 11:4020. doi: 10.3390/foods11244020
Guan, Q., Zheng, W., Mo, J., Huang, T., Xiao, Y., Liu, Z., et al. (2020). Evaluation and comparison of the microbial communities and volatile profiles in homemade suansun from Guangdong and Yunnan provinces in China. J. Sci. Food Agric. 100, 5197–5206. doi: 10.1002/jsfa.10569
Hellwig, C., Rousta, N., Wikandari, R., Taherzadeh, M. J., Häggblom-Kronlöf, G., Bolton, K., et al. (2022). Household fermentation of leftover bread to nutritious food. Waste Manag. 150, 39–47. doi: 10.1016/j.wasman.2022.06.038
Hirose, N., Maeda, G., Tanahara, N., Takara, K., and Wada, K. (2021). Suitability of lactic acid bacteria for the production of pickled luffa (Luffa cylindrica Roem). Food Sci. Technol. Res. 27, 57–61. doi: 10.3136/fstr.27.57
Hu, W., Yang, X., Ji, Y., and Guan, Y. (2021). Effect of starter cultures mixed with different autochthonous lactic acid bacteria on microbial, metabolome and sensory properties of Chinese northeast sauerkraut. Food Res. Int. 148:110605. doi: 10.1016/j.foodres.2021.110605
Huang, Y., Jia, X., Yu, J., Chen, Y., Liu, D., and Liang, M. (2021). Effect of different lactic acid bacteria on nitrite degradation, volatile profiles, and sensory quality in Chinese traditional paocai. LWT 147:111597. doi: 10.1016/j.lwt.2021.111597
Ibrahim, S. A., Ayivi, R. D., Zimmerman, T., Siddiqui, S. A., Altemimi, A. B., Fidan, H., et al. (2021). Lactic acid Bacteria as antimicrobial agents: food safety and microbial food spoilage prevention. Food Secur. 10:3131. doi: 10.3390/foods10123131
Jin, Y., Wu, J., Hu, D., Li, J., Zhu, W., Yuan, L., et al. (2023). Gamma-aminobutyric acid-producing Levilactobacillus brevis strains as probiotics in Litchi juice fermentation. Food Secur. 12:302. doi: 10.3390/foods12020302
Kanklai, J., Somwong, T. C., Rungsirivanich, P., and Thongwai, N. (2021). Screening of GABA-producing lactic acid Bacteria from Thai fermented foods and probiotic potential of Levilactobacillus brevis F064A for GABA-fermented mulberry juice production. Microorganisms 9:33. doi: 10.3390/microorganisms9010033
Khushboo, K., Karnwal, A., and Malik, T. (2023). Characterization and selection of probiotic lactic acid bacteria from different dietary sources for development of functional foods. Front. Microbiol. 14:1170725. doi: 10.3389/fmicb.2023.1170725
Kim, S.-H., Kang, K. H., Kim, S. H., Lee, S., Lee, S.-H., Ha, E.-S., et al. (2017). Lactic acid bacteria directly degrade N-nitrosodimethylamine and increase the nitrite-scavenging ability in kimchi. Food Control 71, 101–109. doi: 10.1016/j.foodcont.2016.06.039
Kim, J., Yoon, Y.-W., Kim, M.-S., Lee, M.-H., Kim, G.-A., Bae, K., et al. (2022). Gamma-aminobutyric acid fermentation in MRS-based medium by the fructophilic Lactiplantibacillus plantarum Y7. Food Sci. Biotechnol. 31, 333–341. doi: 10.1007/s10068-022-01035-w
Kwon, S.-Y., Garcia, C. V., Song, Y.-C., and Lee, S.-P. (2016). GABA-enriched water dropwort produced by co-fermentation with Leuconostoc mesenteroides SM and Lactobacillus plantarum K154. LWT 73, 233–238. doi: 10.1016/j.lwt.2016.06.002
Li, J., Liu, Y., Xiao, H., Huang, H., Deng, G., Chen, M., et al. (2022). Bacterial communities and volatile organic compounds in traditional fermented salt-free bamboo shoots. Food Biosci. 50:102006. doi: 10.1016/j.fbio.2022.102006
Lim, J.-S., Garcia, C. V., and Lee, S.-P. (2016). Optimized production of GABA and γ-PGA in a turmeric and roasted soybean mixture co-fermented by Bacillus subtilis and Lactobacillus plantarum. Food Sci. Technol. Res. 22, 209–217. doi: 10.3136/fstr.22.209
Liu, S., Wen, B., Du, G., Wang, Y., Ma, X., Yu, H., et al. (2023). Coordinated regulation of Bacteroides thetaiotaomicron glutamate decarboxylase activity by multiple elements under different pH. Food Chem. 403:134436. doi: 10.1016/j.foodchem.2022.134436
Lu, H., Huang, C., Yu, K., and Liu, Z. (2022). Effects of mixed inoculation of Leuconostoc citreum and Lactobacillus plantarum on suansun (sour bamboo shoot) fermentation. Food Biosci. 47:101688. doi: 10.1016/j.fbio.2022.101688
Luo, Y., Liu, Y., Ren, T., Wang, B., Peng, Y., Zeng, S., et al. (2020). Sichuan paocai fermented by mixed-starter culture of lactic acid bacteria. Food Sci. Nutr. 8, 5402–5409. doi: 10.1002/fsn3.1833
Mancini, A., Carafa, I., Franciosi, E., Nardin, T., Bottari, B., Larcher, R., et al. (2019). In vitro probiotic characterization of high GABA producing strain Lactobacilluas brevis DSM 32386 isolated from traditional “wild” alpine cheese. Ann. Microbiol. 69, 1435–1443. doi: 10.1007/s13213-019-01527-x
Maria Ramos, I., and Maria Poveda, J. (2022). Fermented sheep’s milk enriched in gamma-amino butyric acid (GABA) by the addition of lactobacilli strains isolated from different food environments. LWT Food Sci. Technol. 163:113581. doi: 10.1016/j.lwt.2022.113581
Moore, J. F., DuVivier, R., and Johanningsmeier, S. D. (2021). Formation of γ-aminobutyric acid (GABA) during the natural lactic acid fermentation of cucumber. J. Food Compos. Anal. 96:103711. doi: 10.1016/j.jfca.2020.103711
Mustafa, G., Arshad, M. U., Saeed, F., Afzaal, M., Niaz, B., Hussain, M., et al. (2022). Comparative study of raw and fermented oat bran: nutritional composition with special reference to their structural and antioxidant profile. Fermentation 8:509. doi: 10.3390/fermentation8100509
Ou, D., Ling, N., Wang, X., Zou, Y., Dong, J., Zhang, D., et al. (2022). Safety assessment of one Lactiplantibacillus plantarum isolated from the traditional Chinese fermented vegetables—Jiangshui. Food Secur. 11:2177. doi: 10.3390/foods11152177
Pakdeeto, A., Phuengjayaem, S., Arayakarn, T., Phitchayaphon, C., Tungkajiwangkoon, S., and Tanasupawat, S. (2022). Identification of gamma-aminobutyric acid (GABA)-producing lactic acid bacteria from plant-based Thai fermented foods and genome analysis of Lactobacillus brevis GPB7-4. ScienceAsia 48, 254–262. doi: 10.2306/scienceasia1513-1874.2022.037
Pannerchelvan, S., Rios-Solis, L., Wong, F. W. F., Zaidan, U. H., Wasoh, H., Mohamed, M. S., et al. (2023). Strategies for improvement of gamma-aminobutyric acid (GABA) biosynthesis via lactic acid bacteria (LAB) fermentation. Food Funct. 14, 3929–3948. doi: 10.1039/d2fo03936b
Park, S. J., Kim, D. H., Kang, H. J., Shin, M., Yang, S.-Y., Yang, J., et al. (2021). Enhanced production of γ-aminobutyric acid (GABA) using Lactobacillus plantarum EJ2014 with simple medium composition. LWT 137:110443. doi: 10.1016/j.lwt.2020.110443
Rayavarapu, B., Tallapragada, P., and Usha, M. S. (2019). Statistical optimization of gamma-aminobutyric acid production by response surface methodology and artificial neural network models using Lactobacillus fermentum isolated from palm wine. Biocatal. Agric. Biotechnol. 22:101362. doi: 10.1016/j.bcab.2019.101362
Santos-Espinosa, A., Beltrán-Barrientos, L. M., Reyes-Díaz, R., Mazorra-Manzano, M. Á., Hernández-Mendoza, A., González-Aguilar, G. A., et al. (2020). Gamma-aminobutyric acid (GABA) production in milk fermented by specific wild lactic acid bacteria strains isolated from artisanal Mexican cheeses. Ann. Microbiol. 70:12. doi: 10.1186/s13213-020-01542-3
Shokri, D., Khorasgani, M. R., Mohkam, M., Fatemi, S. M., Ghasemi, Y., and Taheri-Kafrani, A. (2018). The inhibition effect of lactobacilli against growth and biofilm formation of Pseudomonas aeruginosa. Prob. Antim. Prot. 10, 34–42. doi: 10.1007/s12602-017-9267-9
Singhal, P., Satya, S., and Naik, S. N. (2021). Fermented bamboo shoots: a complete nutritional, anti-nutritional and antioxidant profile of the sustainable and functional food to food security. Food Chem. Mol. Sci. 3:100041. doi: 10.1016/j.fochms.2021.100041
Tang, H., Ma, J.-K., Chen, L., Jiang, L.-W., Kang, L.-Z., Guo, Y.-Y., et al. (2024). Characterization of key flavor substances and their microbial sources in traditional sour bamboo shoots. Food Chem. 437:137858. doi: 10.1016/j.foodchem.2023.137858
Thuy, D. T. B., Nguyen, A., Khoo, K. S., Chew, K. W., Cnockaert, M., Vandamme, P., et al. (2021). Optimization of culture conditions for gamma-aminobutyric acid production by newly identified Pediococcus pentosaceus MN12 isolated from “mam nem”, a fermented fish sauce. Bioengineered 12, 54–62. doi: 10.1080/21655979.2020.1857626
Tokatlı, M., Gülgör, G., Bağder Elmacı, S., Arslankoz İşleyen, N., and Özçelik, F. (2015). In vitro properties of potential probiotic indigenous lactic acid Bacteria originating from traditional pickles. Biomed. Res. Int. 2015:e315819. doi: 10.1155/2015/315819
Trindade, D. P. A., Barbosa, J. P., Martins, E. M. F., and Tette, P. A. S. (2022). Isolation and identification of lactic acid bacteria in fruit processing residues from the Brazilian Cerrado and its probiotic potential. Food Biosci. 48:101739. doi: 10.1016/j.fbio.2022.101739
Wang, X., Han, M., Zhang, M., Wang, Y., Ren, Y., Yue, T., et al. (2021). In vitro evaluation of the hypoglycemic properties of lactic acid bacteria and its fermentation adaptability in apple juice. LWT Food Sci. Technol. 136:110363. doi: 10.1016/j.lwt.2020.110363
Wang, L., Luo, Y.-Y., Zhang, Y., Zou, C.-X., Wang, P.-J., Qin, L.-K., et al. (2023). Quality analysis of ultra-fine whole pulp of bamboo shoots (Chimonobambusa quadrangularis) fermented by Lactobacillus plantarum and Limosilactobacillus reuteri. Food Biosci. 52:102458. doi: 10.1016/j.fbio.2023.102458
Weng, B. B.-C., Yuan, H.-D., Chen, L.-G., Chu, C., and Hsieh, C.-W. (2023). Soy yoghurts produced with efficient GABA (γ-aminobutyric acid)-producing Lactiplantibacillus plantarum ameliorate hyperglycaemia and re-establish gut microbiota in streptozotocin (STZ)-induced diabetic mice. Food Funct. 14, 1699–1709. doi: 10.1039/D2FO02708A
Wu, Q., Law, Y.-S., and Shah, N. P. (2015). Dairy Streptococcus thermophilus improves cell viability of Lactobacillus brevis NPS-QW-145 and its γ-aminobutyric acid biosynthesis ability in milk. Sci. Rep. 5:12885. doi: 10.1038/srep12885
Wu, R., Yu, M., Liu, X., Meng, L., Wang, Q., Xue, Y., et al. (2015). Changes in flavour and microbial diversity during natural fermentation of suan-cai, a traditional food made in Northeast China. Int. J. Food Microbiol. 211, 23–31. doi: 10.1016/j.ijfoodmicro.2015.06.028
Xia, Y., Liu, X., Wang, G., Zhang, H., Xiong, Z., Sun, Y., et al. (2017). Characterization and selection of Lactobacillus brevis starter for nitrite degradation of Chinese pickle. Food Control 78, 126–131. doi: 10.1016/j.foodcont.2017.02.046
Xia, A.-N., Meng, X.-S., Tang, X.-J., Zhang, Y.-Z., Lei, S.-M., and Liu, Y.-G. (2021). Probiotic and related properties of a novel lactic acid bacteria strain isolated from fermented rose jam. LWT 136:110327. doi: 10.1016/j.lwt.2020.110327
Yogeswara, I. B. A., Kittibunchakul, S., Rahayu, E. S., Domig, K. J., Haltrich, D., and Nguyen, T. H. (2021). Microbial production and enzymatic biosynthesis of gamma-aminobutyric acid (GABA) using Lactobacillus plantarum FNCC 260 isolated from Indonesian fermented foods. PRO 9:22. doi: 10.3390/pr9010022
Younas, S., Mazhar, B., Liaqat, I., Ali, S., Tahir, H. M., and Ali, N. M. (2022). Bacteriocin production by lactobacilli and their role as antibacterial tool against common pathogens. J. Oleo Sci. 71, 541–550. doi: 10.5650/jos.ess21424
Yu, Y., Xu, Y., Li, L., Chen, S., An, K., Yu, Y., et al. (2023). Isolation of lactic acid bacteria from Chinese pickle and evaluation of fermentation characteristics. LWT 180:114627. doi: 10.1016/j.lwt.2023.114627
Zhang, X., Han, J., Zheng, X., Yan, J., Chen, X., Zhou, Q., et al. (2022). Use of Lactiplantibacillus plantarum ZJ316 as a starter culture for nitrite degradation, foodborne pathogens inhibition and microbial community modulation in pickled mustard fermentation. Food Chem. 14:100344. doi: 10.1016/j.fochx.2022.100344
Zhang, Z., Li, C., Zhang, H., Ying, Y., Hu, Y., and Song, L. (2020). Comparative analysis of the lignification process of two bamboo shoots stored at room temperature. Plants Basel 9:1399. doi: 10.3390/plants9101399
Zhao, N., Ge, L., Lai, H., Wang, Y., Mei, Y., Huang, Y., et al. (2022). Unraveling the contribution of pre-salting duration to microbial succession and changes of volatile and non-volatile organic compounds in Suancai (a Chinese traditional fermented vegetable) during fermentation. Food Res. Int. 159:111673. doi: 10.1016/j.foodres.2022.111673
Keywords: bamboo shoots, lactic acid bacteria, GABA, fermentation, inoculation
Citation: Chen M, Xia H, Zuo X, Tang D, Zhou H, Huang Z, Guo A and Lv J (2024) Screening and characterization of lactic acid bacteria and fermentation of gamma-aminobutyric acid-enriched bamboo shoots. Front. Microbiol. 15:1333538. doi: 10.3389/fmicb.2024.1333538
Edited by:
Yiannis Kourkoutas, Democritus University of Thrace, GreeceReviewed by:
Stavros Plessas, Democritus University of Thrace, GreeceMaría Chávarri, Tecnalia Research & Innovation, Spain
Copyright © 2024 Chen, Xia, Zuo, Tang, Zhou, Huang, Guo and Lv. This is an open-access article distributed under the terms of the Creative Commons Attribution License (CC BY). The use, distribution or reproduction in other forums is permitted, provided the original author(s) and the copyright owner(s) are credited and that the original publication in this journal is cited, in accordance with accepted academic practice. No use, distribution or reproduction is permitted which does not comply with these terms.
*Correspondence: Jun Lv, dGFpaGVsdmp1bjEyM0AxNjMuY29t; Hongqiu Xia, MTM5NzgyODc4OThAMTYzLmNvbQ==; Ailing Guo, YWlsaW5nZ3VvMjM0QDE2My5jb20=