- 1Department of Critical Care Medicine, The Central Hospital of Shaoyang City and Affiliated Shaoyang Hospital, Hengyang Medical College, University of South China, Shaoyang, China
- 2Institute of Pathogenic Biology, Basic Medical School, Hengyang Medical School, University of South China, Hunan Provincial Key Laboratory for Special Pathogens Prevention and Control, Hengyang, China
Mycoplasma pneumoniae causes respiratory tract infections, affecting both children and adults, with varying degrees of severity ranging from mild to life-threatening. In recent years, a new class of regulatory RNAs called long non-coding RNAs (lncRNAs) has been discovered to play crucial roles in regulating gene expression in the host. Research on lncRNAs has greatly expanded our understanding of cellular functions involving RNAs, and it has significantly increased the range of functions of lncRNAs. In lung cancer, transcripts associated with lncRNAs have been identified as regulators of airway and lung inflammation in a process involving protein complexes. An excessive immune response and antibacterial immunity are closely linked to the pathogenesis of M. pneumoniae. The relationship between lncRNAs and M. pneumoniae infection largely involves lncRNAs that participate in antibacterial immunity. This comprehensive review aimed to examine the dysregulation of lncRNAs during M. pneumoniae infection, highlighting the latest advancements in our understanding of the biological functions and molecular mechanisms of lncRNAs in the context of M. pneumoniae infection and indicating avenues for investigating lncRNAs-related therapeutic targets.
1 Introduction
Mycoplasma pneumoniae, an atypical bacterium, is one of the smallest prokaryotic microorganisms without a cell wall (Shimizu, 2015). There are 200 known mycoplasma species, including six main species, which can cause human respiratory and reproductive tract diseases, among other diseases (Combaz-Söhnchen and Kuhn, 2017; Gómez Rufo et al., 2021). M. pneumoniae is one of the main pathogenic mycoplasmas, and it is a significant cause of respiratory tract infections. It causes endemic and epidemic primary atypical pneumonia, tracheobronchitis, pharyngitis, and asthma worldwide. M. pneumoniae pneumonia is the most significant disease associated with M. pneumoniae infection (Shimizu, 2016; Waites et al., 2017; Tsai et al., 2021). In addition, M. pneumoniae can cause infections outside the lungs (de Groot et al., 2017) by penetrating host cell membranes and invading respiratory tract mucous membranes, resulting in a pronounced inflammatory response outside the respiratory system (Poddighe, 2018). The severity of the diseases caused by M. pneumoniae ranges from mild to life-threatening (Waites et al., 2017). The dominant pathogenic mechanisms of M. pneumoniae are direct cytotoxicity and adhesion to host cells, immune evasion, and inflammation-induced damage (Jiang et al., 2021). The pathogenic mechanisms of extrapulmonary manifestations also involve direct injury mediated by inflammatory factors, indirect injury caused by the host immune response, and vascular occlusion (Hu et al., 2022).
Genes, which direct an organism’s development and function, include sequences with and without protein-coding functions (García-Andrade et al., 2022). Long non-coding RNAs (lncRNAs) comprise >200 nucleotides that do not code for proteins (Mattick et al., 2023). LncRNAs are widely expressed and play key roles in gene expression regulation. LncRNAs mainly interact with microRNAs (miRNAs), mRNAs, DNAs, and proteins, and they can thereby modulate gene expression in a variety of ways, e.g., by modulating chromatin function or regulating membraneless nuclear body assembly and function (Zhang et al., 2019; Statello et al., 2021a). LncRNAs are newly discovered regulators in many diseases, and there is a growing body of literature suggesting a relationship between lncRNAs and M. pneumoniae infection (Gu et al., 2020; Sun et al., 2022).
LncRNAs can be used by the host to modulate immune-related gene expression in order to resist M. pneumoniae invasion or decrease the damage caused by M. pneumoniae invasion, and M. pneumoniae can evade immune clearance by modulating the host lncRNAs (Wen et al., 2020).
This review summarizes the broad categories and common regulatory mechanisms of lncRNAs, the roles of lncRNAs in various diseases, and the defense mechanisms involving host cells’ lncRNAs against M. pneumoniae infection. It also provides an overview that indicates avenues for investigating lncRNAs-related therapeutic targets in M. pneumoniae infection and other diseases.
2 Category of lncRNAs
LncRNAs encompass a wide range of transcripts (Djebali et al., 2012) that exhibit significant diversity in terms of the presence of initiation codons, genomic location, and functional roles, making it difficult to easily characterize them. They can be broadly categorized into three types based on their mechanisms of action: (1) transcriptional regulation, (2) post-transcriptional regulation, and (3) other (Table 1) (Ma et al., 2013). The mechanisms of action of lncRNAs involved in transcriptional regulation can be further classified as (i) transcriptional interference, (ii) chromatin remodeling, and (iii) regulation effect. (The latter involves eRNAs, ncRNA-a1, Evf-2RNA, and Alpha-250/Alpha-280) (Table 1) (Ma et al., 2013). The mechanisms of action of lncRNAs involved in post-transcriptional regulation can be divided into (i) splicing regulation, (ii) translational control, lncRNAs that participate in translational control may function through binding to translation factors citation or ribosome (Ma et al., 2013), and (iii) other (the latter involves siRNA, 1/2-sbsRNA1, 21A, linc-MD1, IPS1, HULC, and BACE1-AS) (Table 1) (Rintala-Maki and Sutherland, 2009). The remaining lncRNAs can be classified into five categories based on other regulatory mechanisms: (i) protein localization (Watanabe and Yamamoto, 1994), (ii) telomere replication (Feng et al., 1995), (iii) RNA interference (Hellwig and Bass, 2008; Smekalova et al., 2016), (iv) regulation beyond transcription; unlike many other lncRNAs, promoter antisense RNAs (PAS RNAs) were initially considered to be merely passive transcription by-products of active promoters (Yang, 2022), and (v) translation regulation (Table 1).
3 Conventional lncRNAs regulatory mechanism
LncRNAs were initially thought to be merely interfering factors in gene transcription, (i.e., acting as accessory products that impede gene transcription involving RNA polymerase II), but they were later found to play essential roles in various biological activities. Notably, lncRNAs participate in transcription but prevent transcription by other chromosomes (Cabili et al., 2015).
The lncRNA LINC02159 (which is highly expressed in non-small cell lung cancer) forms a complex with Aly/REF export factor (ALYREF) through its 5-methylcytosine m5C modified sites and then binds to YAP1 mRNA, thereby increasing its stability (Chen et al., 2023). The lncRNA ADPGK-AS1, which mainly exists in mitochondria, is upregulated in artificially induced human M2 macrophages, and it binds to mitochondrial ribosomal protein MRPL35 and thereby promotes the tricarboxylic acid cycle and mitochondrial division, resulting in tumor growth (Karger et al., 2023).
The lncRNA MALAT1, also known as non-coding nuclear-enriched abundant transcript 2 (NEAT2), epigenetically regulates gene expression. Highly efficient knockdown of MALAT1 (using zinc finger nuclease-based technology) in extensive organization tumor cells confirmed that MALAT1 promotes in vitro and in vivo metastasis without affecting tumor cell proliferation (Gutschner et al., 2013). During extensive tumor cell proliferation, MALAT1 is regulated by multiple signaling pathways and has important roles in invasion and metastasis (Chen et al., 2022). MALAT1 regulates the activity of serine/arginine (SR) splicing factors, thereby influencing gene expression via alternative splicing (Tripathi et al., 2010). MALAT1 is also involved in cell cycle regulation, interacting with and promoting the cytoplasmic transport of heterogeneous nuclear ribonucleoprotein C (hnRNP C) in the G2/M phase, thereby controlling gene expression (Yang et al., 2011). Seven novel lncRNAs have been identified as competitive endogenous RNAs. Their abnormal expression leads to the widespread expression of tumorigenic genes (Figure 1A).
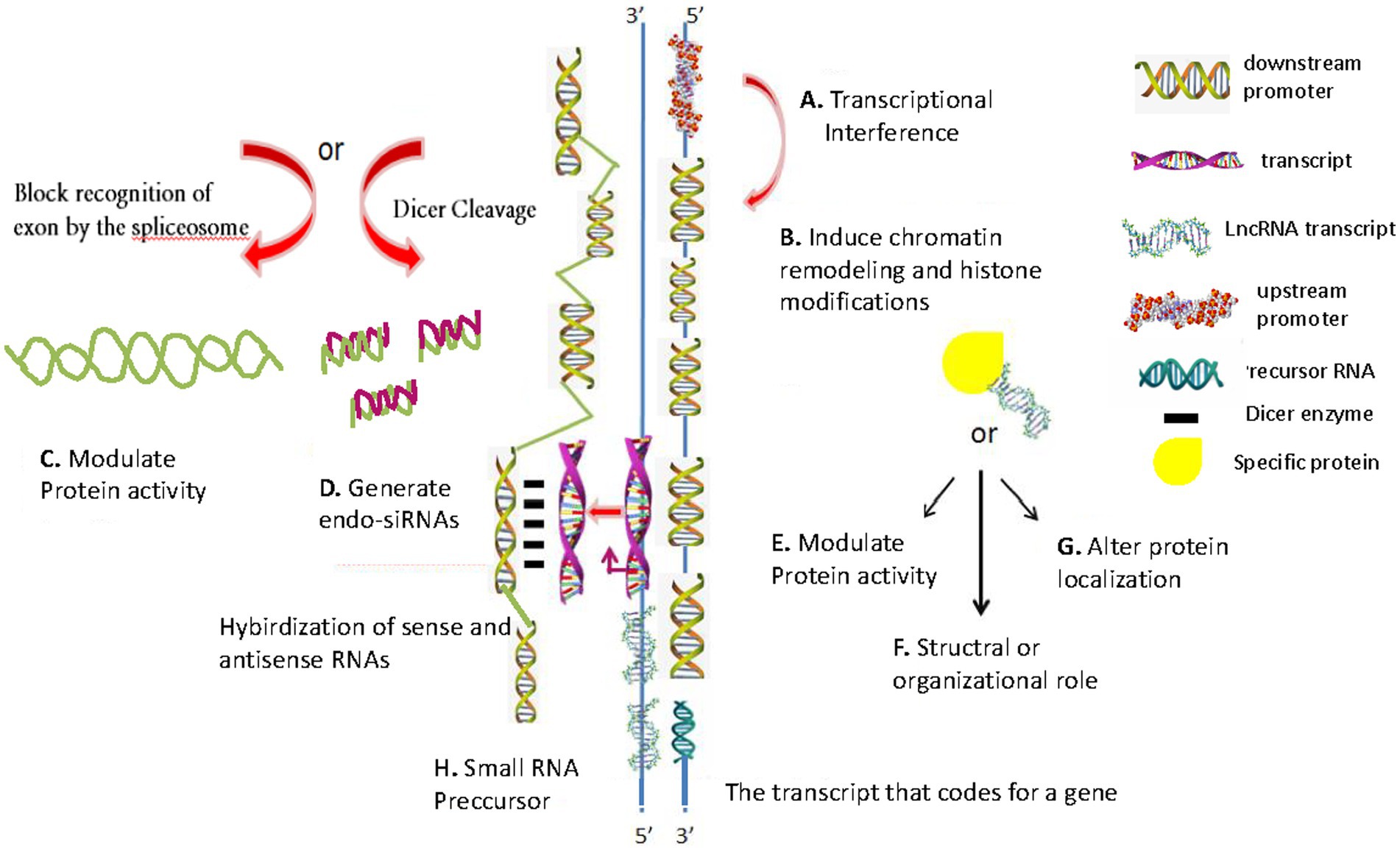
Figure 1. Schematic of conventional lncRNA regulatory mechanisms. (A) Transcription interference involves transcription from the upstream promoter region of a target protein-coding gene. (B) Inhibiting RNA polymerase II or inducing chromatin remodeling or histone modification, which interferes with target gene transcription. (C) Modulate protein activity. (D) Generating endogenous short interfering RNAs (endo-siRNAs), which target specific mRNAs (RNA interference). (E) Binding to a specific protein to modulate its activity. (F) Structural or organizational role, which catalyzes specific reactions. (G) Binding to a specific protein to alter its cellular localization. (H) Producing small RNA precursors. The diagrams are original and free of copyright restrictions.
As shown in the schematic in Figure 1, there are eight conventional lncRNAs regulatory mechanisms: (1) transcription interference involving transcription from the upstream promoter region of a target protein-coding gene (Figure 1A); (2) inhibiting RNA polymerase II or inducing chromatin remodeling or histone modification, which interferes with target gene transcription (Figure 1B); (3) generating complementary double strands involving mRNAs, which interferes with mRNA cleavage (Figure 1C); (4) generating endogenous short interfering RNAs (endo-siRNAs), which target specific mRNAs (RNA interference) (Figure 1D); (5) binding to a specific protein to modulate its activity (Figure 1E); (6) forming a ribozyme-protein complex, which catalyzes specific reactions (Figure 1F); (7) binding to a specific protein to alter its cellular localization (Figure 1G); and (8) producing small RNA precursors (Figure 1H).
Some upregulated lncRNAs play a tumor-promoting role, while downregulated lncRNAs in gastric cancer play a tumor-inhibitory role (Figure 1B) (Ahmed Shehata et al., 2021). Some lncRNAs can regulate protein activity (Figure 1C). SiRNAs or overexpression plasmids were transfected (with adequate transfection efficiency) into cells and verified using fluorescent markers (Figure 1D) (Cao et al., 2019). Some lncRNAs can form a complementary double strand with mRNA (which interferes with mRNA cleavage), and some lncRNAs can produce endo-siRNAs under the action of the Dicer enzyme (Figure 1E). Many lncRNAs are characteristically expressed in polarized tissues and specific cancer types (Xing et al., 2021). They form nucleic acid protein complexes with the proteins acting as structural components (Figure 1F) (Zhou et al., 2016), thereby altering protein localization (Figure 1G). LncRNAs (which are >200 nucleotides in length) have no protein-coding potential (Figure 1H).
4 LncRNAs in M. pneumoniae infection
4.1 LncRNAs in intrapulmonary M. pneumoniae manifestations
LncRNAs have the function of modifying cell biology (Statello et al., 2021b). LncRNAs can act with mRNAs, DNAs, proteins, and miRNAs to adjust gene expression at the epigenetic, transcriptional, post-transcriptional, translational, and post-translational levels in a variety of ways (Zhang et al., 2019). LncRNAs have many functions, including in M. pneumoniae infection, involving both: (1) transcriptional regulation, (2) post-transcriptional regulation, and (3) others (Table 1) (Wright et al., 2013). The interaction of these three regulatory mechanisms plays an important role in the M. pneumoniae infection of host cells (Dykes and Emanueli, 2017).
4.1.1 Acute respiratory distress syndrome
LncRNAs are key regulators in respiratory diseases, and they can modulate cell growth arrest. The lncRNA GAS5 plays a significant role in many inflammatory diseases, including acute lung injury, idiopathic pulmonary fibrosis, and M. pneumoniae infection (Yang et al., 2021). GAS5 overexpression enhances cellular energy production and downregulates the pro-inflammatory cytokines IL-1β and IL-6 in human acute monocytic leukemia THP-1 cells (Figure 2A). The overexpression of miR-222-3p, which targets and reverses M. pneumoniae-induced THP-1 cell energy production, reduces M. pneumoniae-induced THP-1 cell viability, and accelerates the inflammatory response. GAS5 silencing reduces M. pneumoniae-induced chondrocyte activity and exacerbates M. pneumoniae-induced host cell inflammatory injuries. These findings offer new targets for treating M. pneumoniae infection (Yang et al., 2021). When exposed to host cells, M. pneumoniae upregulates the community-acquired respiratory distress syndrome acute respiratory distress syndrome (ARDS) toxin protein (encoded by the MPN372 gene), which is involved in host-cell interactions (Medina et al., 2012).
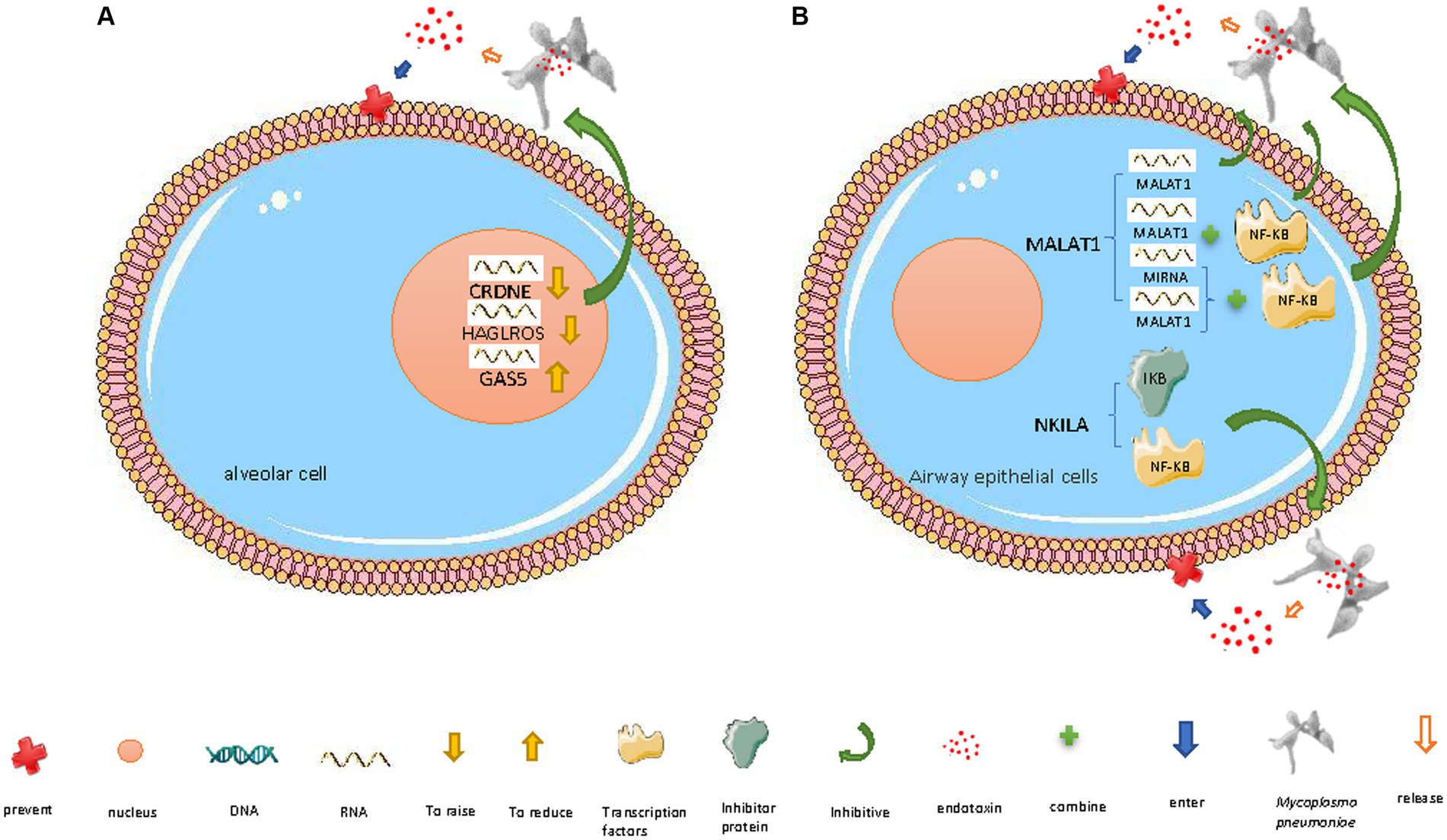
Figure 2. Mechanisms of lncRNAs defending against M. pneumoniae invasion. (A) In the nucleus: (i) lncRNA CRNDE downregulation, (ii) lncRNA HAGLROS downregulation, and (iii) lncRNA GAS5 upregulation inhibit M. pneumoniae-induced inflammatory factor release, thereby preventing damage to host cells. (B) In the cytoplasm: (i) downregulated lncRNA MALAT1 inhibits the inflammatory response triggered by M. pneumoniae endotoxin release by altering NF-κB activation; MALAT1 regulates NF-κB via three pathways: (a) competing with miRNAs that target NF-κB mRNA, (b) binding to NF-κB, and (c) directly resistance to endotoxins (ii) lncRNA NKILA inhibits the inflammatory response triggered by M. pneumoniae endotoxin release by preventing the dissociation of NF-κB from its inhibitor I-κB and thereby preventing NF-κB translocation to the nucleus. The diagrams are original and free of copyright restrictions.
GAS5 was downregulated in lung epithelial cells treated with lipopolysaccharide (which can cause ARDS), suggesting that GAS5 is involved in the development of ARDS. The GAS5/miR-200c-3p/ACE2 signaling axis is involved in the apoptosis of ARDS lung epithelial cells. These findings offer new therapeutic targets for ARDS and enrich our understanding of the GAS5-mediated regulation of lung injury, which is of great significance for understanding the pathogenesis of ARDS (Li et al., 2018).
4.1.2 Acute pneumonia
M. pneumoniae lipopolysaccharide can enter human embryonic lung WI-38 fibroblasts, induce inflammatory damage, and destroy the cells by triggering lncRNA HAGLROS upregulation. M. pneumoniae can induce inflammatory damage in WI-38 cells by modulating the miR-100/NF-κB axis. HAGLROS upregulation inhibits miRNA-100 (which therefore no longer targets and suppresses NF-κB3), thereby increasing NF-κB activity. HAGLROS knockout prevents NF-κB activation and thereby enhances WI-38 cell viability, inhibits apoptosis, and mitigates M. pneumoniae-induced cell damage (Figure 2A). Reducing the expression of miR-100 activates NF-κB3 and thereby causes WI-38 cell damage and apoptosis; this effect of reducing the expression of miR-100 can be prevented by NF-κB3 knockout (Figure 2A) (Liu et al., 2018). During M. pneumoniae-induced pneumonia, type I alveolar epithelial cells defend against M. pneumoniae infection by activating the innate immune response (Yamamoto et al., 2012), including the PI3K/AKT/NFκB pathway (Yang et al., 2021). The HAGLROS/miR-100/NF-κB axis may be a new target for the treatment of M. pneumoniae infection (Liu et al., 2018).
Another lncRNA that can regulate NF-κB activity is NKILA, which can exert an anti-inflammatory effect in airway epithelial cells. NKILA can mask the phosphorylation motif of I-κB (an inhibitor of NF-κB) and thereby prevent I-κB degradation and NF-κB translocation (Figure 2B) (Liu et al., 2015; Ke et al., 2018; Wang et al., 2018; Yu et al., 2018), inhibiting M. pneumoniae-induced inflammatory response genes (Peschke et al., 2014). NKILA is decreased and cytokines (IL-8 and TNF-α) are increased in bronchoalveolar lavage fluid from children infected with M. pneumoniae and NKILA knockdown in airway epithelial cells promotes M. pneumoniae-induced cytokine secretion NKILA exerts its anti-inflammatory effect by weakening the negative feedback loop of NF-κB signaling that regulates cytokine secretion (Figure 2B) (Zhang et al., 2021).
Moreover, downregulation of the lncRNA CRNDE and upregulation of miR-141 (which inhibits NF-κB and is targeted by CRNDE) inhibit the M. pneumoniae endotoxin-induced apoptosis and inflammatory response of human embryonic lung MRC-5 fibroblasts, thereby promoting cell survival (Figure 2A) (Zúñiga et al., 2012).
4.1.3 Asthma
During M. pneumoniae infection, the host can regulate certain lncRNAs to inhibit inflammation and apoptosis. Activation of the transcription factor NF-κB, which regulates various inflammatory response genes, plays a significant role in M. pneumoniae-induced airway inflammation. Under normal conditions, NF-κB is bound to its inhibitor, I-κB, and remains in the cytoplasm. When stimulated by M. pneumoniae lipoproteins, which are recognized by toll-like receptors (TLRs), I-κB is phosphorylated and degraded, releasing NF-κB; activated phosphorylated NF-κB p65 then enters the nucleus and upregulates inflammation-related genes (Zhu et al., 2010). The pro-inflammatory mechanism of action of the lncRNA MALAT1 partially relies on it increasing NF-κB activation (by directly binding to it or indirect regulation, i.e., acting as a competitive endogenous RNA, competing with miRNAs that target NF-κB mRNA, and thus enhancing NF-κB activity). It thereby regulates the M. pneumoniae-induced inflammatory response (Figure 2B) (Dai et al., 2018; Lei et al., 2018). NF-κB upregulates TNF-α, which can damage capillary endothelial cells, thereby promoting microthrombosis and leading to ischemic necrosis, so TNF-α is associated with pneumonia severity (Figure 2B) (Salvatore et al., 2007). MALAT1 knockdown inhibits M. pneumoniae-induced NF-κB p65 phosphorylation in mouse airway epithelial cells and mouse lung tissue. Thus, the regulatory role of MALAT1 in M. pneumoniae infection-induced inflammation is closely related to NF-κB activation (Zhang et al., 2021).
The mechanism by which lower respiratory tract M. pneumoniae infections trigger or worsen asthma in children is not completely clear (Kumar et al., 2019). Following M. pneumoniae infection in children, a small percentage of individuals present with recurrent wheezing episodes, and the prevalence of M. pneumoniae infection in children with acute asthma has been reported to be 46% (Kassisse et al., 2018). M. pneumoniae can induce mucin overproduction by inhibiting the transcription suppressor FOXA2 Lung function is improved by restoring FOXA2’s transcription suppressor function and downregulating goblet cell hyperplasia and metaplasia (GCHM)-promoting pathways in M. pneumoniae-infected airways in asthma patients with abnormal mucin secretion and accumulation in airway lumens, which are clinical markers of asthma (Hao et al., 2014). In addition, asthma is associated with upregulated MALAT1 and downregulated miRNA-216a (which is inhibited by MALAT1, acting as a molecular sponge), while the opposite (MALAT1 downregulation and/or miRNA-216a upregulation) significantly increases apoptosis while significantly decreasing cell proliferation, migration, and invasion (Huang J. et al., 2021).
4.2 Immune-mediated mechanisms of M. pneumoniae extrapulmonary manifestations
M. pneumoniae can cause various extrapulmonary manifestations, including those that affect the cardiovascular system, skin, and liver.
The cardiovascular manifestations of M. pneumoniae infection (Bakshi et al., 2006) include aortic thrombosis (Flateau et al., 2013) and pulmonary thrombosis. M. pneumoniae can directly spread via the blood to distant organs and induce local production of cytokines and chemokines (TNF-α and IL-8), eventually leading to local vasculitis or thrombosis. M. pneumoniae can also indirectly lead to systemic hypercoagulability by activating chemical mediators, complement, and fibrin D-dimer, which increase the risk of thrombotic vascular occlusion (Hu et al., 2022).
The dermatological manifestations of M. pneumoniae infection include erythema nodosum [an immune complex-mediated disease that primarily affects young women (Kakourou et al., 2001)] and cutaneous leucocytic vasculitis [characterized by perivascular neutrophilia reported to be caused by circulating immune complexes (Kakourou et al., 2001; Perez and Montes, 2002)]. Although M. pneumoniae cannot infect the squamous cell epithelium, it may produce inflammatory bullous lesions due to the transfer of cytokines from the respiratory tract to the skin via the blood (Narita, 2016).
The hepatic manifestations of M. pneumoniae infection can arise as a result of modulation of T-cell-mediated immune responses by T cell immunoglobulin and mucin domain-containing proteins (TIMs) expressed on T cells, which can regulate T cell cytokine differentiation (Wang et al., 2008). Liver damage can also be caused by inflammatory cell activation induced by signaling involving TLR2 and TLR4, which are expressed on cell surfaces and can detect and initiate responses to extracellular pathogens (Kawasaki and Kawai, 2014; Shimizu et al., 2014). M. pneumoniae causes acute and severe hepatitis in children, which is likely to be immune-mediated and involve both innate and adaptive immune responses (Poddighe, 2020).
In summary, the detailed mechanisms underlying the three abovementioned types of M. pneumoniae extrapulmonary infection are unclear, but it is clear that they generally involve inflammatory immune responses (Poddighe et al., 2022).
4.3 LncRNAs and immune-mediated mechanisms of M. pneumoniae infection
4.3.1 Intrapulmonary M. pneumoniae manifestation
M. pneumoniae adhesion molecules and metabolites can cause immune damage to respiratory epithelial cells. M. pneumoniae infection decreases CD4+T cell function, which is the primary cause of immune dysfunction in patients with M. pneumoniae infection, impairing antigen presentation, B-cell maturation, and antibody production. M. pneumoniae also disrupts other humoral and cellular immune responses (Hu et al., 2022). During host cells’ non-specific immune defense against M. pneumoniae, lncRNAs regulate reactive oxygen species production by NADPH oxidase to fight M. pneumoniae (Lee et al., 2020). LncRNAs can also be exploited by M. pneumoniae to evade the immune system (Hu et al., 2022).
4.3.2 Extrapulmonary in children infected (neurological) M. pneumoniae manifestations
M. pneumoniae infection-induced neurological diseases are likely to be a result of immune responses to the infection, based on indirect immunofluorescence and PCR analysis of cerebrospinal fluid samples from patients with these neurological diseases (Poddighe, 2018).
The lncRNA NKILA was downregulated while IL-8 and TNF-α were upregulated in children infected with M. pneumoniae. NKILA knockdown in vitro promotes the inflammatory effect of M. pneumoniae on A549 and BEAS-2B respiratory epithelial cells (Zhang et al., 2021). IL-8 and TNF-α are two well-known pro-inflammatory cytokines that play crucial roles in airway inflammation and chemotaxis caused by M. pneumoniae (Martin et al., 1997).
4.4 LncRNAs/circRNAs in drug-resistant M. pneumoniae infection
Both macrolide-resistant and refractory M. pneumoniae infections complicate the clinical management of M. pneumoniae pneumonia (Tsai et al., 2021). Macrolide-resistant M. pneumoniae harbors a point mutation in 23S rRNA domain V (with substitutions mainly detected at positions 2063 and 2064) (Yang et al., 2017). Circular RNAs (circRNAs), which are like lncRNAs but form a closed loop (Ashekyan et al., 2022), play important roles in gene expression regulation by sequestering miRNA targets (acting as molecular sponges) (Meng et al., 2017). The miRNA targets of circRNAs (detected by high-throughput sequencing) could be utilized as biomarkers for the diagnosis of early-stage refractory M. pneumoniae pneumonia (Huang F. et al., 2021).
5 LncRNAs in other diseases
LncRNAs affect cardiovascular development, including the embryonic development of the heart and vascular system (Kohlmaier et al., 2023). The lncRNA CARMEN can regulate the fate, differentiation, and homeostasis of human cardiac progenitor cells (Ounzain et al., 2015). Additionally, lncRNAs serve as key regulators in cardiovascular diseases such as arterial hypertension, coronary heart disease, and acute myocardial infarction (Correia et al., 2021). For example, overexpression of lnc-Ang362 indirectly activates the (NF-κB) signaling pathway, which promotes vascular smooth muscle cell proliferation and migration, thereby aggravating arterial hypertension (Wang et al., 2020). Additionally, upregulation of the lncRNA cardiac hypertrophy-related factor (CHRF) in cardiomyocytes can upregulate myeloid differentiation primary response 88 (MYD88), inducing cardiomyocyte hypertrophy and apoptosis, leading to heart failure (Wang et al., 2014).
LncRNAs also regulate the development and differentiation of neurons and the nervous system, and they play various pathological roles, leading to various neurodegenerative diseases (Nadhan et al., 2022). In Alzheimer’s disease, the highly upregulated antisense lncRNA BACE1-AS stably binds to BACE1, enhancing the production of β-amyloid plaques (Zeng et al., 2019). In schizophrenia, the reduced expression of the lncRNA MIAT is associated with behavioral changes (Ip et al., 2016). In autism spectrum disorder, the lncRNA SYNGAP-AS1 can downregulate SYNGAP1, causing cortical functional impairment (Velmeshev et al., 2013). In ischemic stroke, the reduced expression of the lncRNA MEG3 activates the Notch signaling pathway and thereby promotes angiogenesis (Yan et al., 2016).
In cancer, some lncRNAs have been identified as oncogenes, while others have been identified as tumor suppressors (Nadhan et al., 2022). The lncRNA HOTTIP acts as an oncogene in acute myeloid leukemia, where it is abnormally elevated and functions as an epigenetic regulator, modulating hematopoietic gene-associated chromatin signatures and transcription (Luo et al., 2019). The p53-dependent lncRNA PVT1 inhibits lung cancer by downregulating c-Myc (Olivero et al., 2020). The abovementioned lncRNA CHRF plays a crucial role in the progression of various tumors, such as prostate cancer, by miRNA binding (Gai et al., 2019). The lncRNA LUCAT1 is associated with various cancers, including cervical cancer, where it exerts oncogenic functions by sequestering miR-181a (Xing et al., 2021). Finally, the highly expressed lncRNA NEAT1 sequesters miR-155 and upregulates TIM3, which promotes CD8 T cell apoptosis and thereby facilitates hepatocellular carcinoma immune evasion and development (Yan et al., 2019).
In endocrine diseases such as diabetes and related conditions (including diabetic nephropathy, diabetic retinopathy, and diabetic neuropathic pain), dysregulated lncRNAs have significant effects (Alipoor et al., 2021). For example, the downregulation of the lncRNA H19 disrupts mitochondrial fatty acid β-oxidation and leads to fatty acid accumulation and insulin resistance (Gui et al., 2020). The lncRNA PVT1 is overexpressed in diabetic nephropathy. PVT1 silences FOXA1 by directly binding to and stabilizing the histone methyltransferase EZH2 to induce trimethylation-based silencing (Liu D. W. et al., 2019). The reduced expression of FOXA1 induces podocyte apoptosis, contributing to the progression of diabetic nephropathy. The lncRNA MALAT1 sequesters miR-125b and thereby upregulates target genes, promotes neovascularization, and impairs vision (Liu P. et al., 2019). The lncRNA NONRATT021972 is upregulated in diabetic neuropathic pain, which it exacerbates by upregulating TNF-α and purinergic receptors (P2X) 3 and 7 It increases the expression of TNFα as well as purinergic receptors (P2X) 3 and 7 (Peng et al., 2017).
5.1 Small molecule response induced by lncRNAs in M. pneumoniae infection
Neutrophils are one of the cells that respond to inflammation sites and play a vital role in killing pathogens (Schenten et al., 2018). The inflammatory response caused by neutrophil activation can be triggered by endogenous ligands called damage-associated molecular patterns (DAMPs) or actively aerated alarmins (Chan et al., 2012). Recently, S100A8/9 proteins have been identified as DAMPs released by neutrophils and monocytes [which has been proposed to be an active process dependent on the microtubule network (Schiopu and Cotoi, 2013) or a process involving NETosis (Ehrchen et al., 2009; Bianchi et al., 2011)]. The elevation of S100A8/9 increases neutrophils in the blood, which can promote the occurrence of atherosclerotic disease due to neutrophil accumulations in artery walls (Schiopu and Cotoi, 2013).
6 LncRNAs as targets for treating M. pneumoniae infection
LncRNAs in the nucleus (e.g., CRNDE, HAGLROS, and GAS5) and cytoplasm (e.g., MALAT1 and NKILA) work together to resist M. pneumoniae invasion. Downregulation of CRNDE can upregulate miR-141 and thereby inhibit lipopolysaccharide-induced MRC-5 fibroblast apoptosis and the associated inflammatory response (Meng et al., 2019). HAGLROS downregulation ameliorates lipopolysaccharide-induced PI3K/AKT/NF-κB pathway activation and inflammatory damage in WI-38 cells by causing a lack of HAGLROS to compete with miRNA-100, leading to NF-κB3 downregulation (Torrealba et al., 2020). The HAGLROS/miR-100/NF-κB axis may provide a new target for the treatment of acute-phase M. pneumoniae pneumonia (Fang and Shi, 2022). Highly expressed lncRNA GAS5 reduces the inflammatory response and the viability of LAMP-1-induced human acute monocytic leukemia THP-1 cells by targeting the miR-222-3p/TIMP3 axis (Yang et al., 2021). Downregulated MALAT1 plays a key regulatory role in reducing M. pneumoniae-induced inflammation (Zhao et al., 2016) by downregulating NF-κB signaling (Shimizu et al., 2008). NKILA inhibits the M. pneumoniae-induced inflammatory response of airway epithelial cells by modulating NF-κB (Zhu et al., 2019).
The findings that lncRNAs/circRNAs carried by exosomes in breast cancer (BC) regulate breast cancer-related target genes (Ashekyan et al., 2022) prompt the question of whether the lncRNAs/circRNAs/target genes are related to M. pneumoniae infection and whether they may represent novel targets for the treatment of M. pneumoniae (Tang et al., 2020). LncRNAs have been shown to have broad clinical applications, including cancer diagnosis and prognosis biomarkers (Ashekyan et al., 2022).
7 Perspectives
Although recent lncRNA sequencing analyses have identified potentially key lncRNAs associated with M. pneumoniae pneumonia (Huang et al., 2016), their biological roles and function mechanisms remain largely unknown (Chen et al., 2018). It is important to determine the pivotal molecular mechanisms underlying M. pneumoniae pneumonia in order to develop effective treatment strategies (Chen et al., 2018). Studying lncRNAs may provide an academic foundation for more comprehensive understanding of the molecular mechanisms underlying M. pneumoniae pneumonia and for identifying effective treatment targets, thereby identifying unconventional strategies for the treatment of acute-phase M. pneumoniae pneumonia.
LncRNA regulates cardiovascular development (Correia et al., 2021) and the development and differentiation of neurons and the nervous system (Nadhan et al., 2022). In cancer, some lncRNAs have been identified as oncogenes, while others have been identified as tumor suppressors (Nadhan et al., 2022). In endocrine diseases such as diabetes and related conditions, dysregulated lncRNAs have significant effects (Alipoor et al., 2021). LncRNAs play a variety of roles in these diseases, which may provide insights into the currently unknown roles of lncRNAs in various M. pneumoniae infection states.
8 Conclusion
LncRNAs encompass a wide range of transcripts with significant diversity in terms of the presence of initiation codons, genomic location, and functional roles. They are newly discovered regulators in many diseases, and there is a growing body of literature suggesting a relationship between lncRNAs and M. pneumoniae infection. In this review, we broadly classified lncRNAs’ mechanisms of action as transcriptional regulation, post-transcriptional regulation, and others, and detailed the conventional mechanisms of action of lncRNAs. We also discussed lncRNAs’ roles in the pathogenesis of four major disease types (cardiovascular diseases, neurological disorders, cancers, and the endocrine disease diabetes). Furthermore, we provided insights into lncRNAs’ key protective roles against intrapulmonary, extrapulmonary, and drug-resistant M. pneumoniae infections. This review serves as a succinct overview and indicates avenues for investigating lncRNAs’ roles as novel therapeutic targets.
Author contributions
ZY: Writing – original draft, Project administration. JZ: Writing – original draft. NS: Writing – original draft. ZZ: Writing – original draft. JC: Writing – original draft. PLiu: Writing – review & editing, Writing – original draft, Supervision, Resources. PLin: Supervision, Writing – review & editing.
Funding
The author(s) declare that financial support was received for the research, authorship, and/or publication of this article. This research was supported by the Natural Science Foundation of Hunan Province, China (2023JJ30503); Research Foundation of Education Bureau of Hunan Province, China (22A0297); Research Foundation of University of South China (190XQD015); and Hunan Provincial College Students’ Innovation and Entrepreneurship Training Program (2022X10555197).
Conflict of interest
The authors declare that the research was conducted in the absence of any commercial or financial relationships that could be construed as a potential conflict of interest.
Publisher’s note
All claims expressed in this article are solely those of the authors and do not necessarily represent those of their affiliated organizations, or those of the publisher, the editors and the reviewers. Any product that may be evaluated in this article, or claim that may be made by its manufacturer, is not guaranteed or endorsed by the publisher.
References
Ahmed Shehata, W., Maraee, A., Abd El Monem Ellaithy, M., Tayel, N., Abo-Ghazala, A., and Mohammed El-Hefnawy, S. (2021). Circulating long noncoding RNA growth arrest-specific transcript 5 as a diagnostic marker and indicator of degree of severity in plaque psoriasis. Int. J. Dermatol. 60, 973–979. doi: 10.1111/ijd.15494
Alipoor, B., Nikouei, S., Rezaeinejad, F., Malakooti-Dehkordi, S. N., Sabati, Z., and Ghasemi, H. (2021). Long non-coding RNAs in metabolic disorders: pathogenetic relevance and potential biomarkers and therapeutic targets. J. Endocrinol. Investig. 44, 2015–2041. doi: 10.1007/s40618-021-01559-8
Ashekyan, O., Abdallah, S., Shoukari, A. A., Chamandi, G., Choubassy, H., Itani, A. R. S., et al. (2022). Spotlight on exosomal non-coding RNAs in breast cancer: an in silico analysis to identify potential lncRNA/circRNA-miRNA-target axis. Int. J. Mol. Sci. 23:8351. doi: 10.3390/ijms23158351
Bakshi, M., Khemani, C., Vishwanathan, V., Anand, R. K., and Khubchandani, R. P. (2006). Mycoplasma pneumonia with antiphospholipid antibodies and a cardiac thrombus. Lupus 15, 105–106. doi: 10.1191/0961203306lu2258cr
Bianchi, M., Niemiec, M. J., Siler, U., Urban, C. F., and Reichenbach, J. (2011). Restoration of anti-Aspergillus defense by neutrophil extracellular traps in human chronic granulomatous disease after gene therapy is calprotectin-dependent. J. Allergy Clin. Immunol. 127, 1243–52.e7. doi: 10.1016/j.jaci.2011.01.021
Cabili, M. N., Dunagin, M. C., McClanahan, P. D., Biaesch, A., Padovan-Merhar, O., Regev, A., et al. (2015). Localization and abundance analysis of human lncRNAs at single-cell and single-molecule resolution. Genome Biol. 16:20. doi: 10.1186/s13059-015-0586-4
Cao, H. L., Liu, Z. J., Huang, P. L., Yue, Y. L., and Xi, J. N. (2019). lncRNA-RMRP promotes proliferation, migration and invasion of bladder cancer via miR-206. Eur. Rev. Med. Pharmacol. Sci. 23, 1012–1021. doi: 10.26355/eurrev_201902_16988
Chan, J. K., Roth, J., Oppenheim, J. J., Tracey, K. J., Vogl, T., Feldmann, M., et al. (2012). Alarmins: awaiting a clinical response. J. Clin. Invest. 122, 2711–2719. doi: 10.1172/JCI62423
Chen, J. F., Wu, P., Xia, R., Yang, J., Huo, X. Y., Gu, D. Y., et al. (2018). STAT3-induced lncRNA HAGLROS overexpression contributes to the malignant progression of gastric cancer cells via mTOR signal-mediated inhibition of autophagy. Mol. Cancer 17:6. doi: 10.1186/s12943-017-0756-y
Chen, L., Xie, Y., Yu, M., and Gou, Q. (2022). Long noncoding RNAs in lung cancer: from disease markers to treatment roles. Cancer Manag. Res. 14, 1771–1782. doi: 10.2147/CMAR.S365762
Chen, P., Yu, J., Luo, Q., Li, J., and Wang, W. (2023). Construction of disulfidptosis-related lncRNA signature for predicting the prognosis and immune escape in colon adenocarcinoma. BMC Gastroenterol. 23:382. doi: 10.1186/s12876-023-03020-x
Combaz-Söhnchen, N., and Kuhn, A. (2017). A systematic review of mycoplasma and ureaplasma in urogynaecology. Geburtshilfe Frauenheilkd. 77, 1299–1303. doi: 10.1055/s-0043-119687
Correia, C. C. M., Rodrigues, L. F., de Avila Pelozin, B. R., Oliveira, E. M., and Fernandes, T. (2021). Long non-coding RNAs in cardiovascular diseases: potential function as biomarkers and therapeutic targets of exercise training. Noncoding RNA 7:65. doi: 10.3390/ncrna7040065
Dai, L., Zhang, G., Cheng, Z., Wang, X., Jia, L., Jing, X., et al. (2018). Knockdown of lncRNA MALAT1 contributes to the suppression of inflammatory responses by up-regulating miR-146a in LPS-induced acute lung injury. Connect. Tissue Res. 59, 581–592. doi: 10.1080/03008207.2018.1439480
de Groot, R. C. A., Meyer Sauteur, P. M., Unger, W. W. J., and van Rossum, A. M. C. (2017). Things that could be Mycoplasma pneumoniae. J. Infect. 74, S95–s100. doi: 10.1016/S0163-4453(17)30198-6
Djebali, S., Davis, C. A., Merkel, A., Dobin, A., Lassmann, T., Mortazavi, A., et al. (2012). Landscape of transcription in human cells. Nature 489, 101–108. doi: 10.1038/nature11233
Dykes, I. M., and Emanueli, C. (2017). Transcriptional and post-transcriptional gene regulation by long non-coding RNA. Genomics Proteomics Bioinformatics 15, 177–186. doi: 10.1016/j.gpb.2016.12.005
Ehrchen, J. M., Sunderkötter, C., Foell, D., Vogl, T., and Roth, J. (2009). The endogenous Toll-like receptor 4 agonist S100A8/S100A9 (calprotectin) as innate amplifier of infection, autoimmunity, and cancer. J. Leukoc. Biol. 86, 557–566. doi: 10.1189/jlb.1008647
Fang, X. L., and Shi, S. G. (2022). lncRNA FGD5-AS1 acts AS a ceRNA to regulate lipopolysaccharide-induced injury via the miR-223-3p-3p/GAS5 axis in cardiomyocytes. Hum. Exp. Toxicol. 41:9603271221138969. doi: 10.1177/09603271221138969
Feng, J., Funk, W. D., Wang, S. S., Weinrich, S. L., Avilion, A. A., Chiu, C. P., et al. (1995). The RNA component of human telomerase. Science 269, 1236–1241. doi: 10.1126/science.7544491
Flateau, C., Asfalou, I., Deman, A. L., Ficko, C., Andriamanantena, D., Fontan, E., et al. (2013). Aortic thrombus and multiple embolisms during a Mycoplasma pneumoniae infection. Infection 41, 867–873. doi: 10.1007/s15010-013-0475-2
Gai, H. Y., Wu, C., Zhang, Y., and Wang, D. (2019). Long non-coding RNA CHRF modulates the progression of cerebral ischemia/reperfusion injury via miR-126/SOX6 signaling pathway. Biochem. Biophys. Res. Commun. 514, 550–557. doi: 10.1016/j.bbrc.2019.04.161
García-Andrade, F., Vigueras-Villaseñor, R. M., Chávez-Saldaña, M. D., Rojas-Castañeda, J. C., Bahena-Ocampo, I. U., Aréchaga-Ocampo, E., et al. (2022). The role of microRNAs in the gonocyte theory as target of malignancy: looking for potential diagnostic biomarkers. Int. J. Mol. Sci. 23:10526. doi: 10.3390/ijms231810526
Gómez Rufo, D., García Sánchez, E., García Sánchez, J. E., and García Moro, M. (2021). Clinical implications of the genus Mycoplasma. Rev. Esp. Quimioter. 34, 169–184. doi: 10.37201/req/014.2021
Gu, H., Zhu, Y., Zhou, Y., Huang, T., Zhang, S., Zhao, D., et al. (2020). LncRNA MALAT1 affects Mycoplasma pneumoniae pneumonia via NF-κB regulation. Front. Cell Dev. Biol. 8:563693. doi: 10.3389/fcell.2020.563693
Gui, W., Zhu, W. F., Zhu, Y., Tang, S., Zheng, F., Yin, X., et al. (2020). LncRNAH19 improves insulin resistance in skeletal muscle by regulating heterogeneous nuclear ribonucleoprotein A1. Cell Commun. Signal 18:173. doi: 10.1186/s12964-020-00654-2
Gutschner, T., Hämmerle, M., Eißmann, M., Hsu, J., Kim, Y., Hung, G., et al. (2013). The noncoding RNA MALAT1 is a critical regulator of the metastasis phenotype of lung cancer cells. Cancer Res. 73, 1180–1189. doi: 10.1158/0008-5472.CAN-12-2850
Hao, Y., Kuang, Z., Jing, J., Miao, J., Mei, L. Y., Lee, R. J., et al. (2014). Mycoplasma pneumoniae modulates STAT3-STAT6/EGFR-FOXA2 signaling to induce overexpression of airway mucins. Infect. Immun. 82, 5246–5255. doi: 10.1128/IAI.01989-14
Hellwig, S., and Bass, B. L. (2008). A starvation-induced noncoding RNA modulates expression of dicer-regulated genes. Proc. Natl. Acad. Sci. U.S.A. 105, 12897–12902. doi: 10.1073/pnas.0805118105
Hu, J., Ye, Y., Chen, X., Xiong, L., Xie, W., and Liu, P. (2022). Insight into the pathogenic mechanism of Mycoplasma pneumoniae. Curr. Microbiol. 80:14. doi: 10.1007/s00284-022-03103-0
Huang, F., Fan, H., Yang, D., Zhang, J., Shi, T., Zhang, D., et al. (2021). Ribosomal RNA-depleted RNA sequencing reveals the pathogenesis of refractory Mycoplasma pneumoniae pneumonia in children. Mol. Med. Rep. 24:761. doi: 10.3892/mmr.2021.12401
Huang, S., Feng, C., Chen, L., Huang, Z., Zhou, X., Li, B., et al. (2016). Identification of potential key long non-coding RNAs and target genes associated with pneumonia using long non-coding RNA sequencing (lncRNA-Seq): a preliminary study. Med. Sci. Monit. 22, 3394–3408. doi: 10.12659/MSM.900783
Huang, J., Wang, F. H., Wang, L., Li, Y., Lu, J., and Chen, J. Y. (2021). LncRNA MALAT1 promotes proliferation and migration of airway smooth muscle cells in asthma by downregulating microRNA-216a. Saudi J. Biol. Sci. 28, 4124–4131. doi: 10.1016/j.sjbs.2021.03.076
Ip, J. Y., Sone, M., Nashiki, C., Pan, Q., Kitaichi, K., Yanaka, K., et al. (2016). Gomafu lncRNA knockout mice exhibit mild hyperactivity with enhanced responsiveness to the psychostimulant methamphetamine. Sci. Rep. 6:27204. doi: 10.1038/srep27204
Jiang, Z., Li, S., Zhu, C., Zhou, R., and Leung, P. H. M. (2021). Mycoplasma pneumoniae infections: pathogenesis and vaccine development. Pathogens 10:119. doi: 10.3390/pathogens10020119
Kakourou, T., Drosatoua, P., Psychou, F., Aroni, K., and Nicolaidou, P. (2001). Erythema nodosum in children: a prospective study. J. Am. Acad. Dermatol. 44, 17–21. doi: 10.1067/mjd.2001.110877
Karger, A., Mansouri, S., Leisegang, M. S., Weigert, A., Günther, S., Kuenne, C., et al. (2023). ADPGK-AS1 long noncoding RNA switches macrophage metabolic and phenotypic state to promote lung cancer growth. EMBO J. 42:e111620. doi: 10.15252/embj.2022111620
Kassisse, E., García, H., Prada, L., Salazar, I., and Kassisse, J. (2018). Prevalence of Mycoplasma pneumoniae infection in pediatric patients with acute asthma exacerbation. Arch. Argent. Pediatr. 116, 179–185. doi: 10.5546/aap.2018.eng.179
Kawasaki, T., and Kawai, T. (2014). Toll-like receptor signaling pathways. Front. Immunol. 5:461. doi: 10.3389/fimmu.2014.00461
Ke, S., Li, R. C., Meng, F. K., and Fang, M. H. (2018). NKILA inhibits NF-κB signaling and suppresses tumor metastasis. Aging 10, 56–71. doi: 10.18632/aging.101359
Kohlmaier, A., Holdt, L. M., and Teupser, D. (2023). Long noncoding RNAs in cardiovascular disease. Curr. Opin. Cardiol. 38, 179–192. doi: 10.1097/HCO.0000000000001041
Kumar, S., Roy, R. D., Sethi, G. R., and Saigal, S. R. (2019). Mycoplasma pneumoniae infection and asthma in children. Trop. Dr. 49, 117–119. doi: 10.1177/0049475518816591
Lee, D., Lal, N. K., Lin, Z. J. D., Ma, S., Liu, J., Castro, B., et al. (2020). Regulation of reactive oxygen species during plant immunity through phosphorylation and ubiquitination of RBOHD. Nat. Commun. 11:1838. doi: 10.1038/s41467-020-15601-5
Lei, L., Chen, J., Huang, J., Lu, J., Pei, S., Ding, S., et al. (2018). Functions and regulatory mechanisms of metastasis-associated lung adenocarcinoma transcript 1. J. Cell. Physiol. 234, 134–151. doi: 10.1002/jcp.26759
Li, H. B., Zi, P. P., Shi, H. J., Gao, M., and Sun, R. Q. (2018). Role of signaling pathway of long non-coding RNA growth arrest-specific transcript 5/microRNA-200c-3p/angiotensin converting enzyme 2 in the apoptosis of human lung epithelial cell A549 in acute respiratory distress syndrome. Zhonghua Yi Xue Za Zhi 98, 3354–3359. doi: 10.3760/cma.j.issn.0376-2491.2018.41.013
Liu, M., Han, T., Shi, S., and Chen, E. (2018). Long noncoding RNA HAGLROS regulates cell apoptosis and autophagy in lipopolysaccharides-induced WI-38 cells via modulating miR-100/NF-κB axis. Biochem. Biophys. Res. Commun. 500, 589–596. doi: 10.1016/j.bbrc.2018.04.109
Liu, P., Jia, S.-B., Shi, J.-M., Li, W.-J., Tang, L.-S., Zhu, X.-H., et al. (2019). LncRNA-MALAT1 promotes neovascularization in diabetic retinopathy through regulating miR-125b/VE-cadherin axis. Biosci. Rep. 39:BSR20181469. doi: 10.1042/BSR20181469
Liu, B., Sun, L., Liu, Q., Gong, C., Yao, Y., Lv, X., et al. (2015). A cytoplasmic NF-κB interacting long noncoding RNA blocks IκB phosphorylation and suppresses breast cancer metastasis. Cancer Cell 27, 370–381. doi: 10.1016/j.ccell.2015.02.004
Liu, D. W., Zhang, J. H., Liu, F. X., Wang, X. T., Pan, S. K., Jiang, D. K., et al. (2019). Silencing of long noncoding RNA PVT1 inhibits podocyte damage and apoptosis in diabetic nephropathy by upregulating FOXA1. Exp. Mol. Med. 51, 1–15. doi: 10.1038/s12276-019-0259-6
Luo, H., Zhu, G., Xu, J., Lai, Q., Yan, B., Guo, Y., et al. (2019). HOTTIP lncRNA promotes hematopoietic stem cell self-renewal leading to AML-like disease in mice. Cancer Cell 36, 645–659.e8. doi: 10.1016/j.ccell.2019.10.011
Ma, L., Bajic, V. B., and Zhang, Z. (2013). On the classification of long non-coding RNAs. RNA Biol. 10, 925–933. doi: 10.4161/rna.24604
Martin, L. D., Rochelle, L. G., Fischer, B. M., Krunkosky, T. M., and Adler, K. B. (1997). Airway epithelium as an effector of inflammation: molecular regulation of secondary mediators. Eur. Respir. J. 10, 2139–2146. doi: 10.1183/09031936.97.10092139
Mattick, J. S., Amaral, P. P., Carninci, P., Carpenter, S., Chang, H. Y., Chen, L. L., et al. (2023). Long non-coding RNAs: definitions, functions, challenges and recommendations. Nat. Rev. Mol. Cell Biol. 24, 430–447. doi: 10.1038/s41580-022-00566-8
Medina, J. L., Coalson, J. J., Brooks, E. G., Winter, V. T., Chaparro, A., Principe, M. F. R., et al. (2012). Mycoplasma pneumoniae CARDS toxin induces pulmonary eosinophilic and lymphocytic inflammation. Am. J. Respir. Cell Mol. Biol. 46, 815–822. doi: 10.1165/rcmb.2011-0135OC
Meng, J., Chen, Y., and Zhang, C. (2019). Protective impacts of long noncoding RNA taurine-upregulated 1 against lipopolysaccharide-evoked injury in MRC-5 cells through inhibition of microRNA-127. J. Cell. Biochem. 120, 14928–14935. doi: 10.1002/jcb.28755
Meng, S., Zhou, H., Feng, Z., Xu, Z., Tang, Y., Li, P., et al. (2017). CircRNA: functions and properties of a novel potential biomarker for cancer. Mol. Cancer 16:94. doi: 10.1186/s12943-017-0663-2
Nadhan, R., Isidoro, C., Song, Y. S., and Dhanasekaran, D. N. (2022). Signaling by lncRNAs: structure, cellular homeostasis, and disease pathology. Cells 11:2517. doi: 10.3390/cells11162517
Narita, M. (2016). Classification of Extrapulmonary manifestations due to Mycoplasma pneumoniae infection on the basis of possible pathogenesis. Front. Microbiol. 7:23. doi: 10.3389/fmicb.2016.00023
Olivero, C. E., Martínez-Terroba, E., Zimmer, J., Liao, C., Tesfaye, E., Hooshdaran, N., et al. (2020). p53 activates the long noncoding RNA Pvt1b to inhibit myc and suppress tumorigenesis. Mol. Cell 77, 761–774.e8. doi: 10.1016/j.molcel.2019.12.014
Ounzain, S., Micheletti, R., Arnan, C., Plaisance, I., Cecchi, D., Schroen, B., et al. (2015). CARMEN, a human super enhancer-associated long noncoding RNA controlling cardiac specification, differentiation and homeostasis. J. Mol. Cell. Cardiol. 89, 98–112. doi: 10.1016/j.yjmcc.2015.09.016
Peng, H., Zou, L., Xie, J., Wu, H., Wu, B., Zhu, G., et al. (2017). lncRNA NONRATT021972 siRNA decreases diabetic neuropathic pain mediated by the P2X3 receptor in dorsal root ganglia. Mol. Neurobiol. 54, 511–523. doi: 10.1007/s12035-015-9632-1
Perez, C., and Montes, M. (2002). Cutaneous leukocytoclastic vasculitis and encephalitis associated with Mycoplasma pneumoniae infection. Arch. Intern. Med. 162, 352–354. doi: 10.1001/archinte.162.3.352
Peschke, K., Weitzmann, A., Heger, K., Behrendt, R., Schubert, N., Scholten, J., et al. (2014). IκB kinase 2 is essential for IgE-induced mast cell de novo cytokine production but not for degranulation. Cell Rep. 8, 1300–1307. doi: 10.1016/j.celrep.2014.07.046
Poddighe, D. (2018). Extra-pulmonary diseases related to Mycoplasma pneumoniae in children: recent insights into the pathogenesis. Curr. Opin. Rheumatol. 30, 380–387. doi: 10.1097/BOR.0000000000000494
Poddighe, D. (2020). Mycoplasma pneumoniae-related hepatitis in children. Microb. Pathog. 139:103863. doi: 10.1016/j.micpath.2019.103863
Poddighe, D., Demirkaya, E., Sazonov, V., and Romano, M. (2022). Mycoplasma pneumoniae infections and primary immune deficiencies. Int. J. Clin. Pract. 2022:6343818. doi: 10.1155/2022/6343818
Rintala-Maki, N. D., and Sutherland, L. C. (2009). Identification and characterisation of a novel antisense non-coding RNA from the RBM5 gene locus. Gene 445, 7–16. doi: 10.1016/j.gene.2009.06.009
Salvatore, C. M., Fonseca-Aten, M., Katz-Gaynor, K., Gomez, A. M., Mejias, A., Somers, C., et al. (2007). Respiratory tract infection with Mycoplasma pneumoniae in interleukin-12 knockout mice results in improved bacterial clearance and reduced pulmonary inflammation. Infect. Immun. 75, 236–242. doi: 10.1128/IAI.01249-06
Schenten, V., Plançon, S., Jung, N., Hann, J., Bueb, J. L., Bréchard, S., et al. (2018). Secretion of the phosphorylated form of S100A9 from neutrophils is essential for the proinflammatory functions of extracellular S100A8/A9. Front. Immunol. 9:447. doi: 10.3389/fimmu.2018.00447
Schiopu, A., and Cotoi, O. S. (2013). S100A8 and S100A9: DAMPs at the crossroads between innate immunity, traditional risk factors, and cardiovascular disease. Mediat. Inflamm. 2013:828354. doi: 10.1155/2013/828354
Shimizu, T. (2015). Pathogenic factors of mycoplasma. Nihon Saikingaku Zasshi 70, 369–374. doi: 10.3412/jsb.70.369
Shimizu, T. (2016). Inflammation-inducing factors of Mycoplasma pneumoniae. Front. Microbiol. 7:414. doi: 10.3389/fmicb.2016.00414
Shimizu, T., Kida, Y., and Kuwano, K. (2008). Mycoplasma pneumoniae-derived lipopeptides induce acute inflammatory responses in the lungs of mice. Infect. Immun. 76, 270–277. doi: 10.1128/IAI.00955-07
Shimizu, T., Kimura, Y., Kida, Y., Kuwano, K., Tachibana, M., Hashino, M., et al. (2014). Cytadherence of Mycoplasma pneumoniae induces inflammatory responses through autophagy and toll-like receptor 4. Infect. Immun. 82, 3076–3086. doi: 10.1128/IAI.01961-14
Smekalova, E. M., Kotelevtsev, Y. V., Leboeuf, D., Shcherbinina, E. Y., Fefilova, A. S., Zatsepin, T. S., et al. (2016). lncRNA in the liver: prospects for fundamental research and therapy by RNA interference. Biochimie 131, 159–172. doi: 10.1016/j.biochi.2016.06.007
Statello, L., Guo, C. J., Chen, L. L., and Huarte, M. (2021a). Gene regulation by long non-coding RNAs and its biological functions. Nat. Rev. Mol. Cell Biol. 22, 96–118. doi: 10.1038/s41580-020-00315-9
Statello, L., Guo, C. J., Chen, L. L., and Huarte, M. (2021b). Author correction: gene regulation by long non-coding RNAs and its biological functions. Nat. Rev. Mol. Cell Biol. 22:159. doi: 10.1038/s41580-021-00330-4
Sun, Y., Wang, Y., Zou, M., Wang, T., Wang, L., and Peng, X. (2022). Lnc90386 sponges miR-33-5p to mediate Mycoplasma gallisepticum-induced inflammation and apoptosis in chickens via the JNK pathway. Front. Immunol. 13:887602. doi: 10.3389/fimmu.2022.887602
Tang, X., Wang, T., Qiu, C., Zheng, F., Xu, J., and Zhong, B. (2020). Long non-coding RNA (lncRNA) CRNDE regulated lipopolysaccharides (LPS)-induced MRC-5 inflammation injury through targeting MiR-141. Med. Sci. Monit. 26:e920928. doi: 10.12659/MSM.920928
Torrealba, N., Vera, R., Fraile, B., Martínez-Onsurbe, P., Paniagua, R., and Royuela, M. (2020). TGF-β/PI3K/AKT/mTOR/NF-kB pathway. clinicopathological features in prostate cancer. Aging Male 23, 801–811. doi: 10.1080/13685538.2019.1597840
Tripathi, V., Ellis, J. D., Shen, Z., Song, D. Y., Pan, Q., Watt, A. T., et al. (2010). The nuclear-retained noncoding RNA MALAT1 regulates alternative splicing by modulating SR splicing factor phosphorylation. Mol. Cell 39, 925–938. doi: 10.1016/j.molcel.2010.08.011
Tsai, T. A., Tsai, C. K., Kuo, K. C., and Yu, H. R. (2021). Rational stepwise approach for Mycoplasma pneumoniae pneumonia in children. J. Microbiol. Immunol. Infect. 54, 557–565. doi: 10.1016/j.jmii.2020.10.002
Velmeshev, D., Magistri, M., and Faghihi, M. A. (2013). Expression of non-protein-coding antisense RNAs in genomic regions related to autism spectrum disorders. Mol. Autism. 4:32. doi: 10.1186/2040-2392-4-32
Waites, K. B., Xiao, L., Liu, Y., Balish, M. F., and Atkinson, T. P. (2017). Mycoplasma pneumoniae from the respiratory tract and beyond. Clin. Microbiol. Rev. 30, 747–809. doi: 10.1128/CMR.00114-16
Wang, M., Jiang, Y. M., Xia, L. Y., Wang, Y., Li, W. Y., and Jin, T. (2018). LncRNA NKILA upregulation mediates oxygen glucose deprivation/re-oxygenation-induced neuronal cell death by inhibiting NF-κB signaling. Biochem. Biophys. Res. Commun. 503, 2524–2530. doi: 10.1016/j.bbrc.2018.07.010
Wang, K., Liu, F., Zhou, L. Y., Long, B., Yuan, S. M., Wang, Y., et al. (2014). The long noncoding RNA CHRF regulates cardiac hypertrophy by targeting miR-489. Circ. Res. 114, 1377–1388. doi: 10.1161/CIRCRESAHA.114.302476
Wang, Y., Meng, J., Wang, X., Liu, S., Shu, Q., Gao, L., et al. (2008). Expression of human TIM-1 and TIM-3 on lymphocytes from systemic lupus erythematosus patients. Scand. J. Immunol. 67, 63–70. doi: 10.1111/j.1365-3083.2007.02038.x
Wang, H., Qin, R., and Cheng, Y. (2020). LncRNA-Ang362 promotes pulmonary arterial hypertension by regulating miR-221 and miR-222. Shock 53, 723–729. doi: 10.1097/SHK.0000000000001410
Watanabe, Y., and Yamamoto, M. (1994). S. pombe mei2+ encodes an RNA-binding protein essential for premeiotic DNA synthesis and meiosis I, which cooperates with a novel RNA species meiRNA. Cell 78, 487–498. doi: 10.1016/0092-8674(94)90426-X
Wen, Y., Chen, H., Luo, F., Zhou, H., and Li, Z. (2020). Roles of long noncoding RNAs in bacterial infection. Life Sci. 263:118579. doi: 10.1016/j.lfs.2020.118579
Wright, A. A., Howitt, B. E., Myers, A. P., Dahlberg, S. E., Palescandolo, E., van Hummelen, P., et al. (2013). Oncogenic mutations in cervical cancer: genomic differences between adenocarcinomas and squamous cell carcinomas of the cervix. Cancer 119, 3776–3783. doi: 10.1002/cncr.28288
Xing, C., Sun, S. G., Yue, Z. Q., and Bai, F. (2021). Role of lncRNA LUCAT1 in cancer. Biomed. Pharmacother. 134:111158. doi: 10.1016/j.biopha.2020.111158
Yamamoto, K., Ferrari, J. D., Cao, Y., Ramirez, M. I., Jones, M. R., Quinton, L. J., et al. (2012). Type I alveolar epithelial cells mount innate immune responses during pneumococcal pneumonia. J. Immunol. 189, 2450–2459. doi: 10.4049/jimmunol.1200634
Yan, K., Fu, Y., Zhu, N., Wang, Z., Hong, J. L., Li, Y., et al. (2019). Repression of lncRNA NEAT1 enhances the antitumor activity of CD8+T cells against hepatocellular carcinoma via regulating miR-155/Tim-3. Int. J. Biochem. Cell Biol. 110, 1–8. doi: 10.1016/j.biocel.2019.01.019
Yan, H., Yuan, J., Gao, L., Rao, J., and Hu, J. (2016). Long noncoding RNA MEG3 activation of p53 mediates ischemic neuronal death in stroke. Neuroscience 337, 191–199. doi: 10.1016/j.neuroscience.2016.09.017
Yang, F. (2022). Promoter antisense RNAs: beyond transcription by-products of active promoters. RNA Biol. 19, 533–540. doi: 10.1080/15476286.2022.2062177
Yang, L., Lin, C., Liu, W., Zhang, J., Ohgi, K. A., Grinstein, J. D., et al. (2011). ncRNA- and Pc2 methylation-dependent gene relocation between nuclear structures mediates gene activation programs. Cell 147, 773–788. doi: 10.1016/j.cell.2011.08.054
Yang, H. J., Song, D. J., and Shim, J. Y. (2017). Mechanism of resistance acquisition and treatment of macrolide-resistant Mycoplasma pneumoniae pneumonia in children. Korean J. Pediatr. 60, 167–174. doi: 10.3345/kjp.2017.60.6.167
Yang, L., Zhang, X., and Liu, X. (2021). Long non-coding RNA GAS5 protects against Mycoplasma pneumoniae pneumonia by regulating the microRNA-222-3p/TIMP3 axis. Mol. Med. Rep. 23:380. doi: 10.3892/mmr.2021.12019
Yu, X., Tang, W., Yang, Y., Tang, L., Dai, R., Pu, B., et al. (2018). Long noncoding RNA NKILA enhances the anti-cancer effects of baicalein in hepatocellular carcinoma via the regulation of NF-κB signaling. Chem. Biol. Interact. 285, 48–58. doi: 10.1016/j.cbi.2018.02.027
Zeng, T., Ni, H., Yu, Y., Zhang, M., Wu, M., Wang, Q., et al. (2019). BACE1-AS prevents BACE1 mRNA degradation through the sequestration of BACE1-targeting miRNAs. J. Chem. Neuroanat. 98, 87–96. doi: 10.1016/j.jchemneu.2019.04.001
Zhang, X., Wang, W., Zhu, W., Dong, J., Cheng, Y., Yin, Z., et al. (2019). Mechanisms and functions of long non-coding RNAs at multiple regulatory levels. Int. J. Mol. Sci. 20:5573. doi: 10.3390/ijms20225573
Zhang, F., Zhang, J., Liu, F., Zhou, Y., Guo, Y., Duan, Q., et al. (2021). Attenuated lncRNA NKILA enhances the secretory function of airway epithelial cells stimulated by Mycoplasma pneumoniae via NF-κB. Biomed. Res. Int. 2021:6656298. doi: 10.1155/2021/6656298
Zhao, G., Su, Z., Song, D., Mao, Y., and Mao, X. (2016). The long noncoding RNA MALAT1 regulates the lipopolysaccharide-induced inflammatory response through its interaction with NF-κB. FEBS Lett. 590, 2884–2895. doi: 10.1002/1873-3468.12315
Zhou, M., Diao, Z., Yue, X., Chen, Y., Zhao, H., Cheng, L., et al. (2016). Construction and analysis of dysregulated lncRNA-associated ceRNA network identified novel lncRNA biomarkers for early diagnosis of human pancreatic cancer. Oncotarget 7, 56383–56394. doi: 10.18632/oncotarget.10891
Zhu, X., du, J., Yu, J., Guo, R., Feng, Y., Qiao, L., et al. (2019). LncRNA NKILA regulates endothelium inflammation by controlling a NF-κB/KLF4 positive feedback loop. J. Mol. Cell. Cardiol. 126, 60–69. doi: 10.1016/j.yjmcc.2018.11.001
Zhu, C., Zhang, A., Huang, S., Ding, G., Pan, X., and Chen, R. (2010). Interleukin-13 inhibits cytokines synthesis by blocking nuclear factor-κB and c-Jun N-terminal kinase in human mesangial cells. J. Biomed. Res. 24, 308–316. doi: 10.1016/S1674-8301(10)60043-7
Keywords: lncRNAs, Mycoplasma pneumoniae, Mycoplasma pneumoniae infection, lncRNAs functions, regulatory mechanism of lncRNAs
Citation: Yang Z, Zhou J, Su N, Zhang Z, Chen J, Liu P and Ling P (2024) Insights into the defensive roles of lncRNAs during Mycoplasma pneumoniae infection. Front. Microbiol. 15:1330660. doi: 10.3389/fmicb.2024.1330660
Edited by:
Michal Letek, University of León, SpainReviewed by:
Dimitri Poddighe, Nazarbayev University, KazakhstanCopyright © 2024 Yang, Zhou, Su, Zhang, Chen, Liu and Ling. This is an open-access article distributed under the terms of the Creative Commons Attribution License (CC BY). The use, distribution or reproduction in other forums is permitted, provided the original author(s) and the copyright owner(s) are credited and that the original publication in this journal is cited, in accordance with accepted academic practice. No use, distribution or reproduction is permitted which does not comply with these terms.
*Correspondence: Peng Ling, bHA1NTAxMjExQDEzOS5jb20=