- 1Department of Biochemistry, Robert-Cedergren Centre for Bioinformatics and Genomics, Université de Montréal, Montreal, QC, Canada
- 2DTU Bioengineering, Technical University of Denmark, Lyngby, Denmark
- 3Department of Biological Sciences, King Abdulaziz University, Jeddah, Saudi Arabia
Fungi colonizing plants are gaining attention because of their ability to promote plant growth and suppress pathogens. While most studies focus on endosymbionts from grasses and legumes, the large and diverse group of ericaceous plants has been much neglected. We recently described one of the very few fungal endophytes promoting the growth of the Ericaceae Vaccinium macrocarpon (American cranberry), notably the Codinaeella isolate EC4. Here, we show that EC4 also suppresses fungal pathogens, which makes it a promising endophyte for sustainable cranberry cultivation. By dual-culture assays on agar plates, we tested the potential growth suppression (or biocontrol) of EC4 on other microbes, notably 12 pathogenic fungi and one oomycete reported to infect not only cranberry but also blueberry, strawberry, tomato plants, rose bushes and olive trees. Under greenhouse conditions, EC4 protects cranberry plantlets infected with one of the most notorious cranberry-plant pathogens, Diaporthe vaccinii, known to cause upright dieback and berry rot. The nuclear genome sequence of EC4 revealed a large arsenal of genes potentially involved in biocontrol. About ∼60 distinct clusters of genes are homologs of secondary metabolite gene clusters, some of which were shown in other fungi to synthesize nonribosomal peptides and polyketides, but in most cases, the exact compounds these clusters may produce are unknown. The EC4 genome also encodes numerous homologs of hydrolytic enzymes known to degrade fungal cell walls. About half of the nearly 250 distinct glucanases and chitinases are likely involved in biocontrol because they are predicted to be secreted outside the cell. Transcriptome analysis shows that the expression of about a quarter of the predicted secondary-metabolite gene clusters and glucan and chitin-degrading genes of EC4 is stimulated when it is co-cultured with D. vaccinii. Some of the differentially expressed EC4 genes are alternatively spliced exclusively in the presence of the pathogen, altering the proteins’ domain content and subcellular localization signal, thus adding a second level of proteome adaptation in response to habitat competition. To our knowledge, this is the first report of Diaporthe-induced alternative splicing of biocontrol genes.
1 Introduction
Essentially all plants are associated with microbes, either internally colonizing different plant parts or externally present on the surface. Among the microbes residing inside the plant (referred to as endophytes), some engage in a mutualistic relationship with their host by improving the plant’s nutrient uptake (biofertilization) and inhibiting plant pathogens (biocontrol), while others are plant pathogens themselves.
Microorganisms associated with plants have been studied for decades with a focus on grass (Poaceae) and legume (Fabaceae) symbionts (Harrison and Griffin, 2020; Pirttilä et al., 2021), but only little is known about endophytes colonizing the large and diverse group of ericaceous plants. The Ericaceae are one of the most species-rich families of flowering plants, containing 4,250 known species from 124 genera. They are commonly known as the heather family and are often found in nutrient-poor and acidic soil (Stevens et al., 2004). Unexpectedly, Ericaceae do not host the well-known Arbuscular Mycorrhizal fungi (Glomeromycota), which otherwise colonize more than 90% of all land-plant species. Instead, most fungi isolated from Ericaceae belong to the Ascomycota, including Helotiales, Chaetothyriales, and Xylariaceae (Allen et al., 2003; Bougoure and Cairney, 2005; Zhang et al., 2016; Yang et al., 2018), and a few are Basidiomycota from the order Sebacinales (Berch et al., 2002; Allen et al., 2003). Vaccinium macrocarpon (cranberry) is one of the economically most important Ericaceae crop plants. Particularly in milder climates, cranberry is prone to diseases (Hisano et al., 2012; University of Massachusetts Cranberry Station, 2016), which may explain why most of the published work on cranberry-associated microbes bears on pathogens. Only a few reports have focused on the beneficial effects of cranberry symbionts, such as the stimulation of nitrogen influx into the plant (Kosola et al., 2007) and pathogen suppression (Mason et al., 2014).
Recently, we isolated and characterized a large number of bacterial and fungal endosymbionts of cranberry plants and measured their biofertilizing and biocontrol ability (Salhi et al., 2022). The Codinaeella sp. isolate EC4 proved to promote cranberry-plant growth (Thimmappa et al., 2023) and, in addition, to inhibit the growth of two cranberry-plant pathogens, Godronia sp. and Cytospora chrysosperma (Salhi et al., 2022). Here, we aim to unravel the biocontrol mechanism of EC4. To achieve this, we investigated the effect of EC4 on a broader range of plant pathogens, searched for homologs of known biocontrol genes in EC4’s nuclear genome (Thimmappa et al., 2023) and examined their transcriptional expression. We observed that the fungal plant pathogen Diaporthe vaccinii triggers differential expression of EC4 genes tentatively involved in biocontrol and promotes alternative splicing of a subset of these genes. These phenomena indicate that much of the biocontrol activity of EC4 is induced upon the encounter with the pathogen.
2 Materials and methods
2.1 Strains and culture conditions
The ten presumed pathogenic fungal strains (with isolate designation given in parenthesis) were isolated from the following environments: Physalospora vaccinii (C1), Colletotrichum gloeosporioides (EC77), and Godronia cassandrae (EC82) from the surface of diseased cranberries; Rhizopus sp. (F1), Cadophora luteo-olivacea (F4) and Botrytis cinerea (F5) from the surface of diseased strawberries; Alternaria alternata (B1) from the surface of diseased blueberries; and Alternaria alternata (T1 and T2) from the surface of diseased tomatoes. All fruit were grown in Quebec and purchased from Montreal grocery stores. Botrytis cinerea (R1) was isolated from diseased Quebec-grown rose flowers (Supplementary Tables 1, 2).
Alternaria alternata (IS2), Peniophora sp. (IS5), Diaporthe vaccinii (IS7) and Penicillium sp. (IS8) were isolated by Dr. Richard Belanger’s group at Laval University from diseased Quebec-grown cranberries. Verticillium dahliae VD1, Verticillium dahliae VD2, Fusarium graminearum FG5, Fusarium graminearum FG6, Phytophthora infestans PI3, and Phytophthora infestans PI4 were isolated from olive trees and kindly provided by Dr. Konstantinos A. Aliferis, Agricultural University of Athens, Greece. Most of the fungal strains mentioned here grew readily on potato dextrose agar (PDA) and yeast glycerol growth medium except for Verticillium (VD1 and VD2) and Phytophthora (PI3 and PI4), which require a V8 agar medium (Supplementary Tables 1, 2).
2.2 DNA extraction and fungal identification
All the fungal strains were grown in a glycerol yeast extract liquid medium (2% glycerol, 0.4% yeast extract, pH 7). Mycelia were mechanically broken using a mortar and pestle with glass beads (425–600 μm, Sigma). DNA was then extracted using a Qiagen Genomic-tips 20/G (Qiagen, GmbH) following the manufacturer’s recommendation. Fungal ribosomal internal transcribed spacer regions were amplified using BMBC-F and ITS4-R primers as described elsewhere (Thimmappa et al., 2023), and the PCR products were purified using Wizard® SV Gel and PCR Clean-Up System (Promega, USA). Amplicons were sequenced, and reads were assembled using PHRAP v1.090518 (de la Bastide and McCombie, 2007) and visualized with Consed v27.0 (Gordon et al., 1998). Species were identified by BLASTN1 searches against the NCBI non-redundant (nr) database. Multiple matches with the same taxon among the ten best hits were considered correctly identified. Matches with more than one taxon among the top ten were labeled ambiguous.
2.3 Preparation of fungal inoculum
Fungi were grown in a potato dextrose broth at room temperature for 4 days. The mycelia were filtered and ground in a blender for 3 min (Hamilton Beach, model 51109C, 225W). After serial dilution, the titer was plated on a potato dextrose agar plate, and colony forming units (CFUs) were counted. The titer was adjusted to 1 × w6 CFU/ml for inoculation.
2.4 Screening of growth suppression (biocontrol) activity on agar plates
Biocontrol analysis was performed by placing 5 mm of 5-day-old mycelia of EC4 and pathogens on an agar plate at a 30 mm distance. After incubation for 15 days at 25°C, the growth radius of the pathogen was measured. The percentage of radial growth inhibition (PRI) was calculated using the equation: PRI = (r1-r2/r1) × 100, where r1 is the radial colony growth on the control plate, and r2 is the radial colony growth in the respective screening experiment. To test if residual pathogen mycelium was present in the inhibition zone, the dual-culture plate was inspected under a Nikon SMZ stereo microscope. A petri dish colonized by Diaporthe was inoculated with an agar block of 5 mm2 of 15 days-old EC4 inoculum. After 3 weeks, the top and bottom view of the clearance zone was recorded.
2.5 In planta pathogenicity test
Cranberry plantlets were raised aseptically from seeds, as described elsewhere (Thimmappa et al., 2023). In brief, surface-sterilized cranberry seeds were germinated in a culture box containing gelzan, sucrose, NaFe, MgSO4, KNO3, KCL, KH2PO4, Ca(NO3), KI, MnSO4, ZnSO4, CuSO4, and vitamins (for more detailed information on the growth medium, see Thimmappa et al., 2023) and grown under 16 h light and 8 h dark conditions at room temperature. After 3 months, two to three leaves of each plant were inoculated with presumed fungal pathogens (see Section “2 Materials and methods” and Supplementary Table 1). The in planta test was performed in three cultivation boxes, each holding nine plants. Plants were regularly inspected visually for any disease development.
2.6 In planta biocontrol test
Two to three leaves of each of 27 aseptically grown three-month-old cranberry plantlets (again three boxes, each holding nine plants) were inoculated with 10 CFU/leaf with either EC4 alone, Diaporthe alone or EC4 and Diaporthe together. Cranberry plants were inoculated with 3 μl of sterile distilled water as a control. Plants were visually inspected after 10, 40, 70 and 120 days.
2.7 Test for secreted antimicrobial compounds
A liquid yeast glycerol medium in which either Diaporthe or EC4 was grown alone, or EC4 was grown together with Diaporthe for 1 to 4 days was tested for antimicrobial compounds. The medium was concentrated 20× using a speed vac at 4°C and then filtered (0.22 μm pore size). Diaporthe was inoculated at the center of the culture plate, and the 50 μl of filtrates were placed 3 cm from the center, with yeast glycerol medium as a control. Pathogen growth at room temperature was monitored for 15 days.
2.8 Co-culture of fungi and RNA isolation
To test RNA expression of EC4 in the presence of Diaporthe, ∼1 × 106 CFUs of EC4 and the pathogen were inoculated into 50 mL of glycerol yeast extract liquid medium. The control experiments with EC4 grown alone are reported in our previous work (Thimmappa et al., 2023). In both experiments, cultures were grown under shaking at room temperature for 3 days. The RNA was isolated with the RNeasy Plus Universal kit from Qiagen following the manufacturer’s recommendations.
2.9 RNA-Seq library construction, sequencing, and differential gene expression analysis
The construction of stranded poly-A RNA libraries and Illumina paired-end sequencing (100-bp read length) were outsourced to the Genome Quebec Innovation Center in Montreal. Adapter sequences were trimmed using Trimmomatic v0.35 (Bolger et al., 2014) and reads aligned to the genome using STAR v2.7.1 with –quantMode TranscriptomeSAM parameter (Dobin et al., 2013). Gene and isoform expression were estimated using RSEM v1.3.3 (Li and Dewey, 2011). Differential gene expression analysis was performed with three biological replicates using the DESeq2 (Love et al., 2021) tool. Genes were considered up and down-regulated if the log2 fold change was ≥ +1 and ≤ −1, respectively, and using a false-discovery rate cutoff of 0.05. A biosynthetic gene cluster was considered differentially upregulated when the core-biosynthetic gene was differentially upregulated. RNA-Seq data sets are deposited under the NCBI BioProject ID: PRJNA831867.
2.10 Statistical data analysis
For the statistical analysis of growth inhibition, the Kruskal–Wallis rank-sum test was performed using the package FSA (Ogle et al., 2023), followed by the Dunn or pairwise Mann-Whitney test as a multiple comparison post hoc analysis. Differences with p-values of < 0.05 were considered statistically significant.
2.11 Prediction of fungal cell wall degrading enzymes
CAZyme families were identified in the inferred EC4 proteome using the methodology that is applied for the regular updates of the CAZy database,2 along with expert validation (Cantarel et al., 2009; Lombard et al., 2014).
2.12 Prediction of biosynthetic gene clusters
Secondary metabolite biosynthetic gene clusters (BGCs) were identified genome-wide using antiSMASH (antibiotics and Secondary Metabolite Analysis) v.7.0.0 for fungi (Blin et al., 2023), with the following options: detection strictness: strict, KnownClusterBlast, ClusterBlast, SubClusterBlast, minimum information about a biosynthetic gene (MIBiG) cluster comparison, ActiveSiteFinder, RREFinder, Cluster Pfam analysis, Pfam-based GO term annotation and TIGRFam analysis.
2.13 Secretome prediction
The prediction of secreted proteins in the inferred EC4 proteome proceeded in several steps. First, proteins carrying an N-terminal signal peptide were predicted cumulatively using SignalP v5.0 (Bendtsen et al., 2004), Phobius v1.01 (Käll et al., 2004) and TargetP v2.0 (Almagro Armenteros et al., 2019) with default parameters. Then, proteins that potentially attach to membranes or carry signals for retention in certain subcellular locations were identified by TMHMM v2.0 (Krogh et al., 2001) and Phobius v1.01 (Käll et al., 2004) and tested for the presence of a Glycosylphosphatidylinositol(GPI)-anchor predicted by the PredGPI tool (Pierleoni et al., 2008), and the Prosite motif “PS00014” predicted by PS-Scan (de Castro et al., 2006). These proteins were removed from the set carrying a signal peptide determined in Step 1.
2.14 Alternative splicing events
Genome-wide Alternative Splicing (AS) events recorded in the annotation (.gtf) file were classified using SUPPA2 (Trincado et al., 2018). Secretory peptides were predicted as described above. Protein domains were predicted using Pfam-scan.pl v1.6 (Finn et al., 2010). Domains were analyzed manually to pinpoint the consequences of AS events. Graphs were generated using IsoformSwitchAnalyzeR v2.1.2 (Vitting-Seerup and Sandelin, 2019).
3 Results and discussion
3.1 EC4 inhibits the growth of a broad range of plant pathogens
Codinaeella sp. isolate EC4 is a phytostimulating fungal endosymbiont of cranberry, which was shown in on-plate assays to inhibit the growth of two fungal pathogens, Cytospora chrysosperma and Godronia cassandrae (Salhi et al., 2022; Thimmappa et al., 2023). Here, we examined which other pathogens are suppressed by EC4 and validated their pathogenicity in cranberry plants.
To test the biocontrol ability of EC4, the endophyte was inoculated on an agar plate together with several presumed plant pathogens (for details, see “2 Materials and methods”). These microbes, mostly Ascomycota, Basidiomycota, and Mucoromycota, have been reported to infect not only cranberry plants but also blueberry, strawberry, tomato, rose plants and olive trees. A strain of the oomycete Phytophthora infestans isolated from olive trees was also tested (Supplementary Table 1).
EC4 was able to slow down the growth of all the above microbes by 13 to 70%, with the notable exception of Penicillium sp. strain IS8 (Figure 1 and Supplementary Tables 1, 2). The strongest growth suppression was noted for the two fungi, Botrytis cinerea strain R1 and Diaporthe vaccinii strain IS7. B. cinerea is a necrotrophic fungus that causes diseases in various plant species, including gray mold in rose plants (Ha et al., 2021) and yellow rot in cranberry fruit (Sabaratnam et al., 2016), while D. vaccinii is known to cause upright dieback of cranberry plant shoots and viscid rot as well as field and storage rot of the berries (Oudemans et al., 1998; Michalecka et al., 2017).
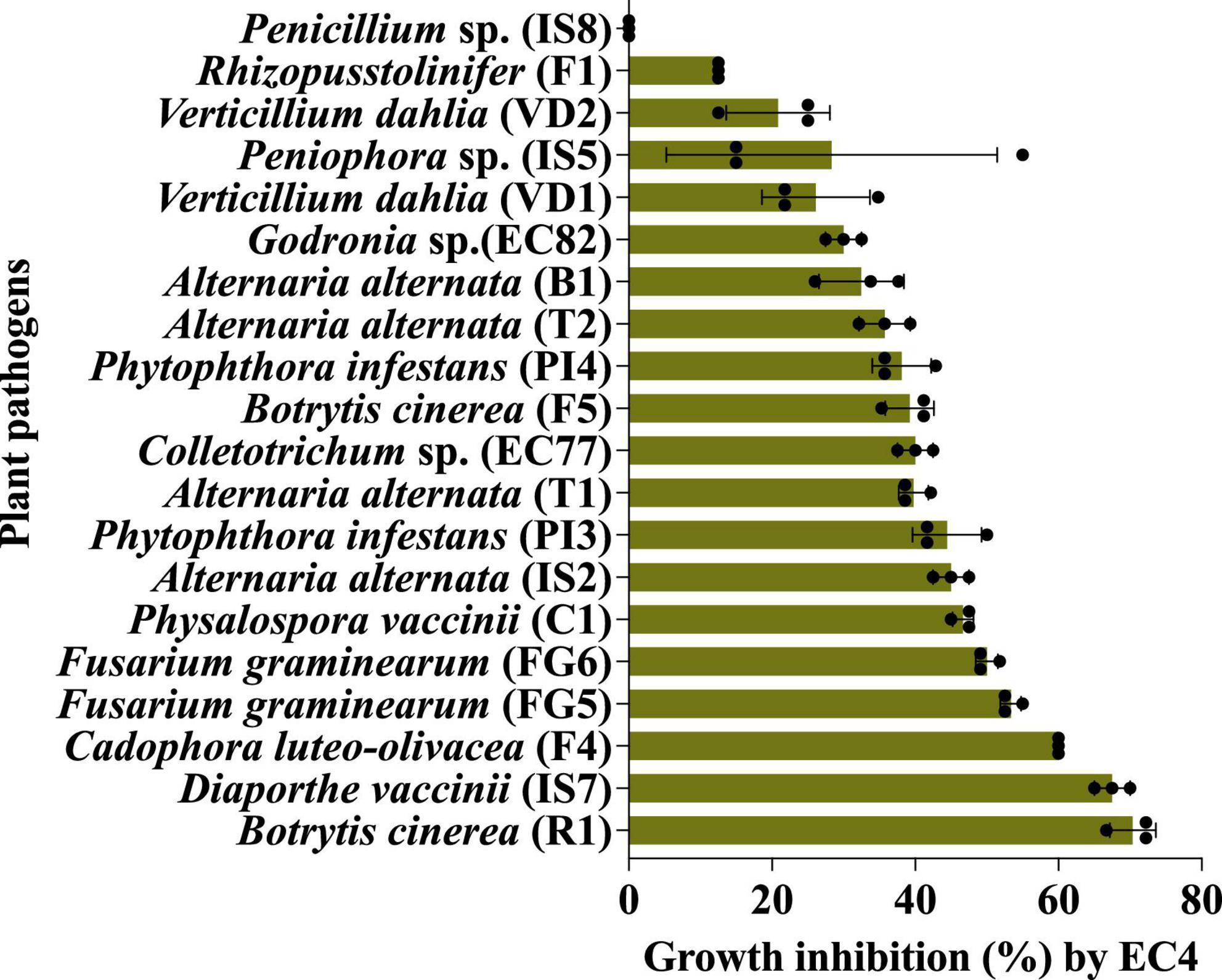
Figure 1. The in vitro biocontrol activity of EC4 against a broad range of other fungi and oomycetes was measured by a confrontation test on agar plates. The statistical support of growth inhibition was P < 0.05, calculated by the Kruskal–Wallis test (Ogle et al., 2023) (for details, see “Materials and methods”). All the tests were done in triplicates and repeated three times. The fungal strains IS2, IS5, IS7, IS8, C1, EC77 and EC82 were isolated from cranberry, B1 from blueberry, R1 from rose flower petals, F1, F4 and F5 from strawberry, T1 and T2 from tomato, VD1, VD2, FG5 and FG6 from olive tree; oomycetes PI3 and PI4 were isolated from olive tree. For details on strains, see Supplementary Table 1. The bars represent the standard error. Dots are individual data points. The growth medium for Phytophthora infestans (PI3 and PI4) and Verticillium dahliae (VD1 and VD2) was V8, whereas all other fungi were grown on yeast-glycerol agar plates.
The effect of EC4 on D. vaccinii was examined in more detail because it was originally isolated from diseased cranberry and is one of the important pathogens to impact cranberry crop. Ten days after simultaneous inoculation of EC4 and Diaporthe on an agar plate, a growth inhibition zone of Diaporthe became clearly visible to the eye (Figure 2A), and microscopic inspection of this zone revealed the absence of any fungal hyphae (Figure 2B). We also tested if EC4 can slow down the growth of Diaporthe on a petri dish already colonized by the pathogen. Indeed, 3 weeks after the inoculation with EC4, a clearance zone appeared and expanded in Diaporthe’s tuft (Figures 2C, D). In this zone, the pathogen’s mycelia did not completely disappear but were reduced considerably.
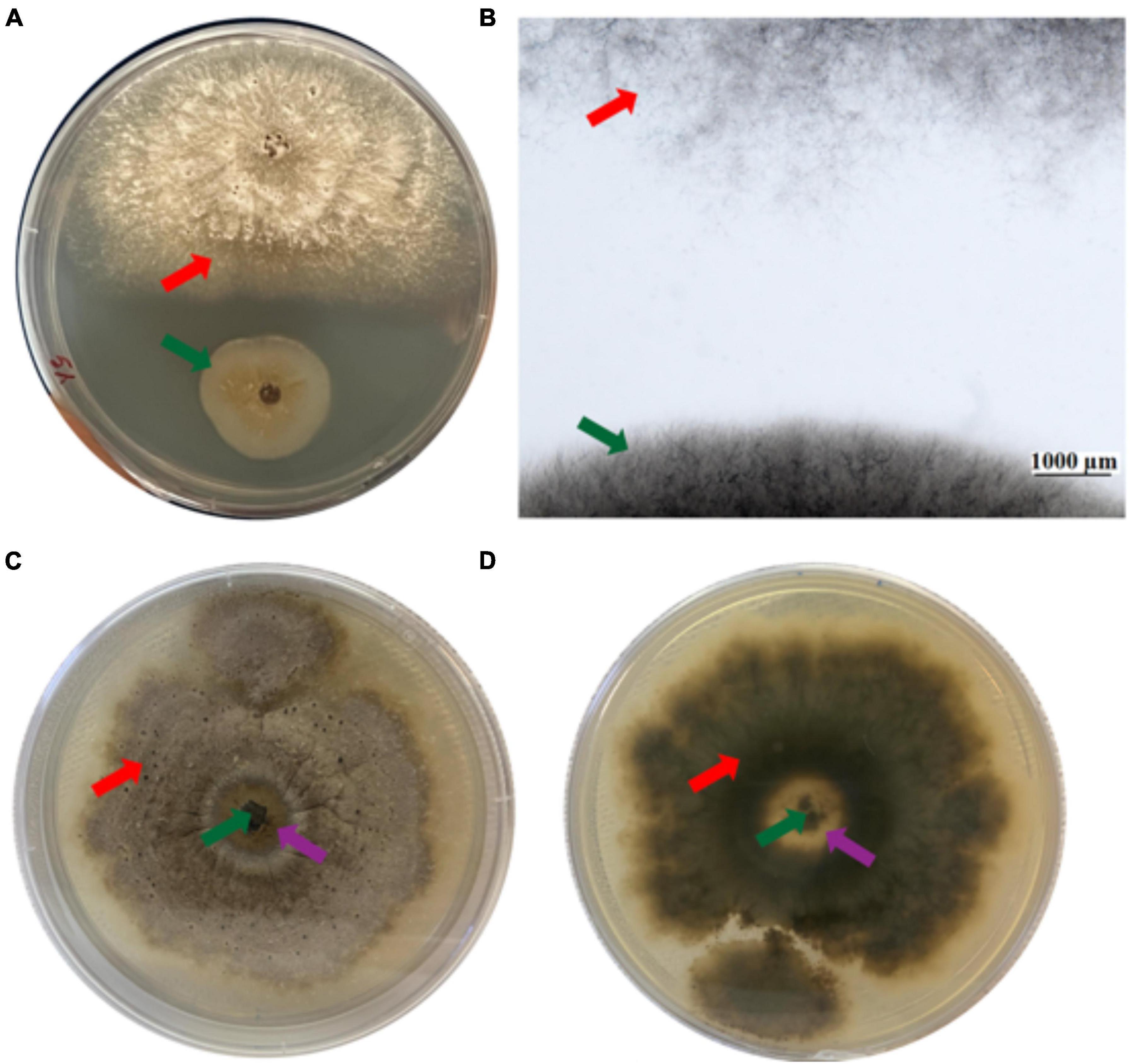
Figure 2. Inhibition of Diaporthe growth by EC4. The red arrow points to Diaporthe and the green arrow to EC4. (A) Dual-culture assay on an agar plate, top view. The tests were conducted in triplicates and repeated three times. (B) Stereo microscopic image of the inhibition zone observed in the dual culture assay. (C,D) A plate colonized by Diaporthe was inoculated with EC4, showing partial clearance of Diaporthe 21 days after inoculation. The purple arrows point to the partial clearance zone. (C) Top view and (D) bottom view of the plate.
3.2 In planta pathogenicity test of presumed pathogens isolated from cranberry plants
The microbial strains tested in this study are considered pathogenic because ribotyping assigned them to species that have been experimentally demonstrated to be plant pathogens, yet experimental data are unavailable for the particular strains. Therefore, we infected aseptically grown cranberry plantlets with either of the seven microbes isolated from cranberry plants (C1, EC77, EC82, IS2, IS5, IS7, and IS8) and monitored plant health. Plantlets inoculated with D. vaccinii (IS7) and Colletotrichum sp. (EC77) died within 40 days (Supplementary Figure 1), but all other tested alleged pathogens showed no phenotypical effect on their host plant. A possible explanation for this observation is that the latter five microbes are latent pathogens under the tested conditions or, alternatively, only attack the berries but not the other plant parts.
3.3 The cranberry-plant pathogen Diaporthe vaccinii can be controlled by EC4
We examined whether EC4 is able to suppress Diaporthe in planta. For that, leaves of endophyte-free plantlets were inoculated with EC4 alone, Diaporthe alone, and EC4 plus Diaporthe (Figure 3). Controls and EC4-inoculated plantlets grew well over the monitored period of 3 months, while those inoculated with Diaporthe started dying off 10 days after inoculation. However, plantlets inoculated simultaneously with EC4 and Diaporthe remained healthy throughout 120 days (and even longer, not shown), demonstrating the biocontrol ability of EC4. Biocontrol of other Diaporthe species (D. longicolla, D. fukushii, and D. foeniculina) has been described before (Divilov and Walker, 2016; Geiger et al., 2022), but not of D. vaccinii. Thus, EC4 appears to be the first described microbe able to suppress D. vaccinii in planta.
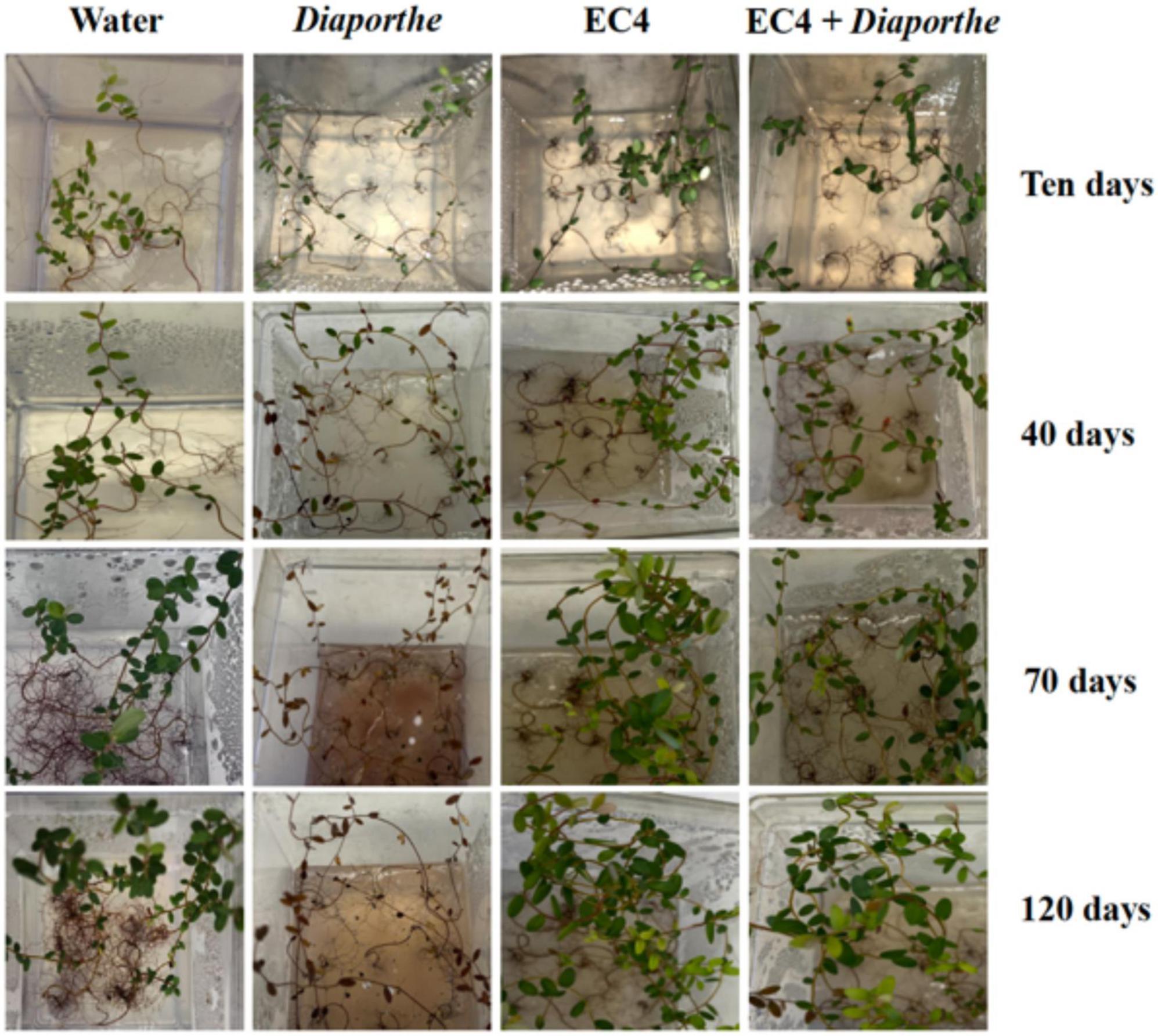
Figure 3. In planta pathogenicity test of Diaporthe and biocontrol test of EC4. Experiments were performed in triplicates and repeated three times for each condition. Cranberry plants were inoculated with sterile distilled water as a control, with Diaporthe alone, EC4 alone, or both, and inspected after 10, 40, 70, and 120 days. After 10 days, the health of Diaporthe-inoculated plants is visibly affected, and they died after 40 days. Plants inoculated with both Diaporthe and EC4 remained vigorous throughout the 3 months.
3.4 D. vaccinii triggers the secretion of antimicrobial compounds by EC4
To investigate if EC4 produced antimicrobial compounds that suppress Diaporthe, we took aliquots of three different liquid media, notably from cultures in which either Diaporthe has grown alone, or EC4 has grown alone, or both have grown together, and tested if these aliquots confer growth inhibition when added to an agar plate inoculated with Diaporthe. The co-culture medium, but not that from EC4 alone or Diaporthe alone, caused an asymmetric radial growth of the pathogen (Figures 4A, B). This indicates that EC4 secretes antimicrobial compounds when in contact with Diaporthe but not in the absence of the pathogen.
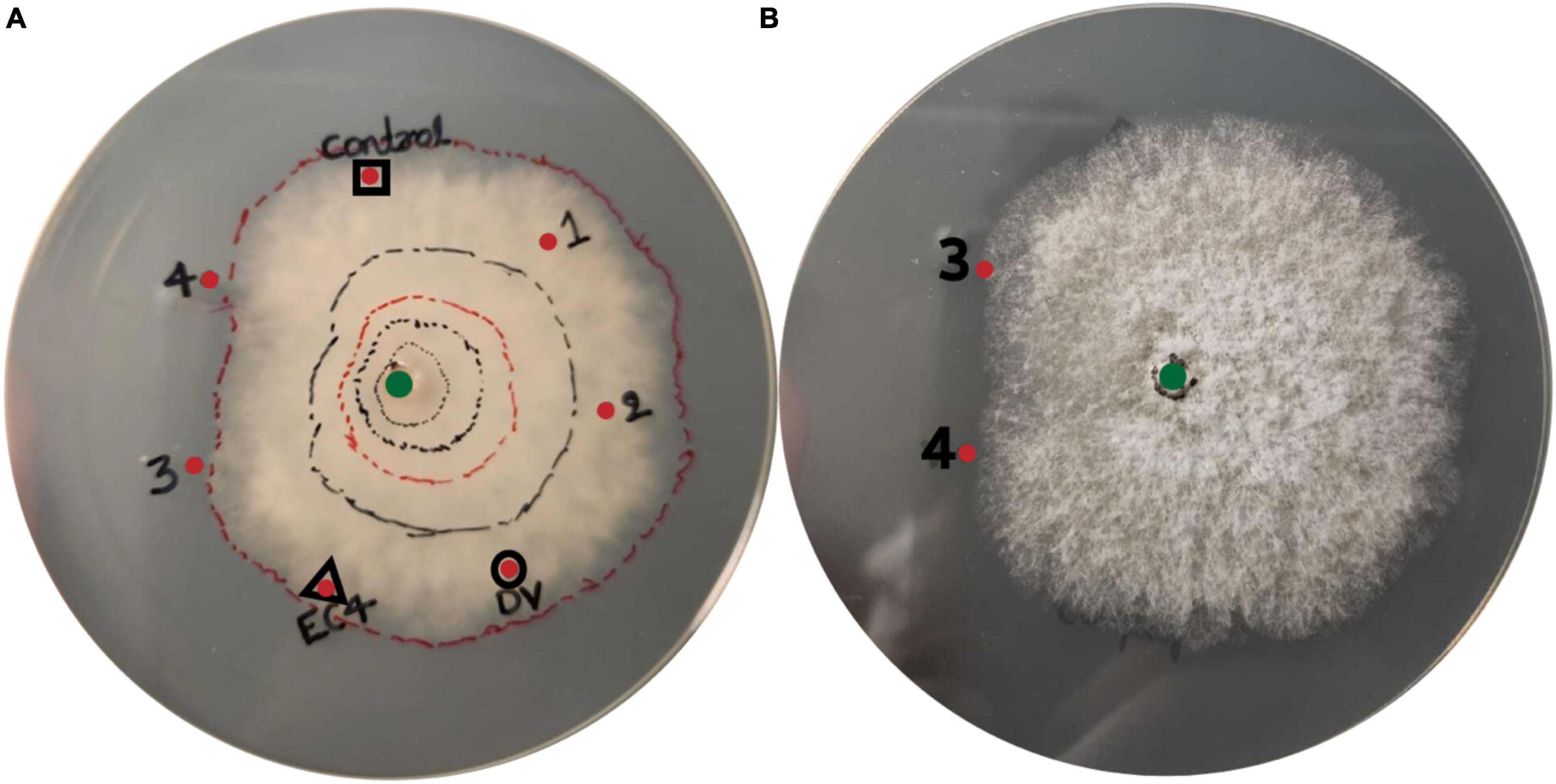
Figure 4. Presence of antimicrobial compounds in the EC4-Diaporthe co-culture medium. Dv, D. vaccinii. (A) Bottom view and (B) top view of the agar plate. Diaporthe has been inoculated at the center of the plate (green dot). Liquid medium from several cultures was tested for growth inhibition of D. vaccinii: a culture of EC4 alone (red dot framed by a triangle), Diaporthe alone (red dot framed by a circle), and a co-culture of EC4 and Diaporthe grown for 1–4 days (red dots labeled 1–4). Culture medium (2 ml) was concentrated 20× and placed 3 cm from the plate’s center. Sterile medium served as a control (red dot framed by a square). The growth of Diaporthe at room temperature was monitored for 15 days. Lines were drawn around the colony’s perimeter to document the growth in time. A conspicuous slow-down of radial growth occurred at dots 3 and 4. The tests were conducted in triplicates and repeated three times.
3.5 The EC4 nuclear genome contains homologs of genes encoding hydrolytic enzymes
One of the mechanisms through which fungi suppress the growth of other fungi is by secreting cell-wall degrading enzymes (Jadhav et al., 2017). The targeted compounds are chitin, a β-(1,4)-linked N-acetylglucosamine polymer and β-(1,3)-glucan, galactomannans, α-glucans, and other carbohydrate polymers (Latgé, 2007). The nuclear genome of EC4 (Thimmappa et al., 2023) contains counterparts of 953 genes encoding Carbohydrate-Active enZymes (CAZymes) (Supplementary File 1). We predicted which of these enzymes remain inside the cell to serve the formation and maintenance of its own cell wall and which are likely secreted outside the cell and thus may degrade the cell wall of competing fungi. The CAZymes predicted to be secreted comprise between 20 and 66 homologs each of chitin, glucan, and mannan-degrading enzymes (Table 1 and Supplementary File 1). Further, EC4 encodes 46 likely secreted glucose–methanol–choline (GMC) oxidoreductases (Table 1 and Supplementary File 1), known to be involved in the biocontrol of fungi by fungi (Kawabe et al., 2011).
As mentioned above, EC4 inhibited the growth of the oomycete Phytophthora, the cell wall of which contains cellulose instead of chitin (Thines, 2018). Indeed, the EC4 genome encodes 42 homologs of cellulase enzymes predicted to be secreted (Table 1 and Supplementary File 1). Such genes are also present in the genomes of fungi and bacteria, for which the ability to suppress Phytophthora has been demonstrated experimentally (Admassie et al., 2022; Frascella et al., 2022).
3.6 The EC4 nuclear genome encodes genes predicted to participate in secondary metabolite synthesis
Another important biocontrol mechanism, besides cell-wall hydrolysis, is the production of secondary metabolites. Among the well-known compound classes are nonribosomal peptides, polyketides, hybrid nonribosomal peptides/polyketides, terpenes, and indoles, many of which act as toxins, antibiotics, or cytostatics. Secondary metabolites are assembled by specialized enzymes, the genes of which are organized in biosynthetic gene clusters, together with genes encoding tailoring enzymes that further modify the molecules, and genes involved in regulation and transport (Medema et al., 2011). The EC4 nuclear genome contains 58 putative secondary metabolite gene clusters, about half of which are type-1 polyketide synthase (PKS) clusters, nonribosomal peptide synthetase (NRPS) clusters and hybrid clusters (Table 2 and Supplementary Table 3). Nineteen of these share substantial similarities with clusters from other organisms and for which the exact product is known, whereas no information is available for the compounds synthesized by the remaining 39 clusters.
3.7 Gene expression of EC4 when co-cultured with the pathogen
Of the 17,582 protein-coding genes encoded by the EC4 nuclear genome, about 89% are transcriptionally expressed under four conditions tested (Supplementary File 2), suggesting that essentially all genes are expressed under one or more of the various metabolic, physiological, and environmental conditions the fungus encounters during its life. To get insight into the genes involved in biocontrol, we investigated which EC4 genes change their expression when the organism is co-cultured with Diaporthe.
Table 3 compiles the transcriptional behavior of EC4 genes in the presence of the pathogen, with a focus on the genes coding for secondary metabolites and secreted hydrolytic enzymes. We refer to this subset of EC4 genes as “Diaporthe-growth-quelling candidates” or short “quelling candidates.” About ∼11% of these genes were upregulated when EC4 was cultured in the presence of the pathogen (for details, see Supplementary File 2). More specifically, the upregulated quelling candidates are predicted to encode 16 chitinases, 17 glucanases, and seven mannanases (Table 1, and Supplementary File 1) that were demonstrated in the biocontrol agent Trichoderma to be involved in inhibiting the growth of phytopathogens (Lopes et al., 2012; Geraldine et al., 2013; Vos et al., 2015). Of the ten differentially upregulated biosynthetic gene clusters, seven are predicted to produce antifungal compounds such as (-)-Mellein, Chaetoglobosin, and Oxaleimide (Chen et al., 2015; Perlatti et al., 2020; Reveglia et al., 2020), and putative cytotoxic compounds such as Sansalvamide, Communesin, Squalestatin S1, and Wortmanamide (see Table 2; Belofsky et al., 1999; Trost and Osipov, 2015; Bonsch et al., 2016; Hai and Tang, 2018). The compounds synthesized by the remaining three clusters are unknown but are assumed to act as antifungals as well.
3.8 The role of mRNA splicing isoforms in EC4
Previous transcriptome analysis showed that of the 17,582 EC4 nuclear genes for which we detected transcripts, nearly 20% produce mRNA splicing isoforms (Thimmappa et al., 2023). Here, we analyzed the types of alternative splicing and the function of isoforms, first globally and then with a focus on Diaporthe-quelling candidates. Globally, five distinct modes of alternative splicing were observed in EC4: intron retention (the most frequent event), exon skipping, use of alternative 5′ and 3′ splice sites, and alternative use of last exon (Table 4 and Supplementary File 3). Mutually exclusive exons and alternative first exon usage were not observed. Among the genes with isoforms, those differing in their coding regions amounted to 80%, while changes in their UTRs were observed in nearly all instances (Table 3). Distinct UTRs of alternative transcripts are thought to influence mRNA expression by changing the binding platform of regulatory microRNAs (Preussner et al., 2020). A similar pattern of alternative splicing, as seen in EC4, has been reported for other fungi (Fang et al., 2020; Ibrahim et al., 2021).
In EC4, 61 of the quelling candidates have isoforms, summing up to a total of 170. Of these isoforms, 16 (9%) were upregulated when EC4 was cultivated in the presence of Diaporthe (Supplementary File 3). The corresponding genes include chitinase, GMC oxidoreductase and others. Interestingly, alternative splicing events of 34 quelling candidates resulted in a change of conserved protein domains or targeting signals, changes that are bound to entail functional consequences for the translation product (Supplementary File 3).
One remarkable example of a quelling-candidate gene with isoforms encoding different protein domains is FUNEC_09200 (Figure 5A). The protein product was annotated by our pipeline as a chitinase that is secreted outside the cell; secretion was corroborated by an additional InterPro scan analysis (Quevillon et al., 2005). Both isoforms are transcriptionally expressed in the absence as well as in the presence of Diaporthe (Figure 5B), but only isoform 1 is differentially upregulated under the latter condition (Figure 5C). While both isoforms comprise a chitinase domain belonging to the GH18 family of glycoside hydrolases (Cantarel et al., 2009), isoform 1 carries, in addition, a family-1 carbohydrate-binding module (CBM), which is non-catalytic. Members of this latter family bind cellulose, and some were shown to bind chitin as well (Linder et al., 1996). CBMs present in CAZymes were demonstrated to enhance the cell-wall degrading activity of the enzyme’s catalytic domain by binding to polymers that are in close proximity to the cognate substrate of the catalytic domain (Hervé et al., 2010). Further, in Trichoderma reesei, a family-1 CBM (designated Cel7A), when fused by genetic engineering to a chitinase-only enzyme, strongly improved the degradation of insoluble chitin (Neeraja et al., 2010; Kowsari et al., 2014). This indicates that co-cultivation with Diaporthe triggers differential up-regulation of isoform 1, augmenting the efficiency of a secreted EC4 chitinase that most likely participates in growth inhibition of the pathogen.
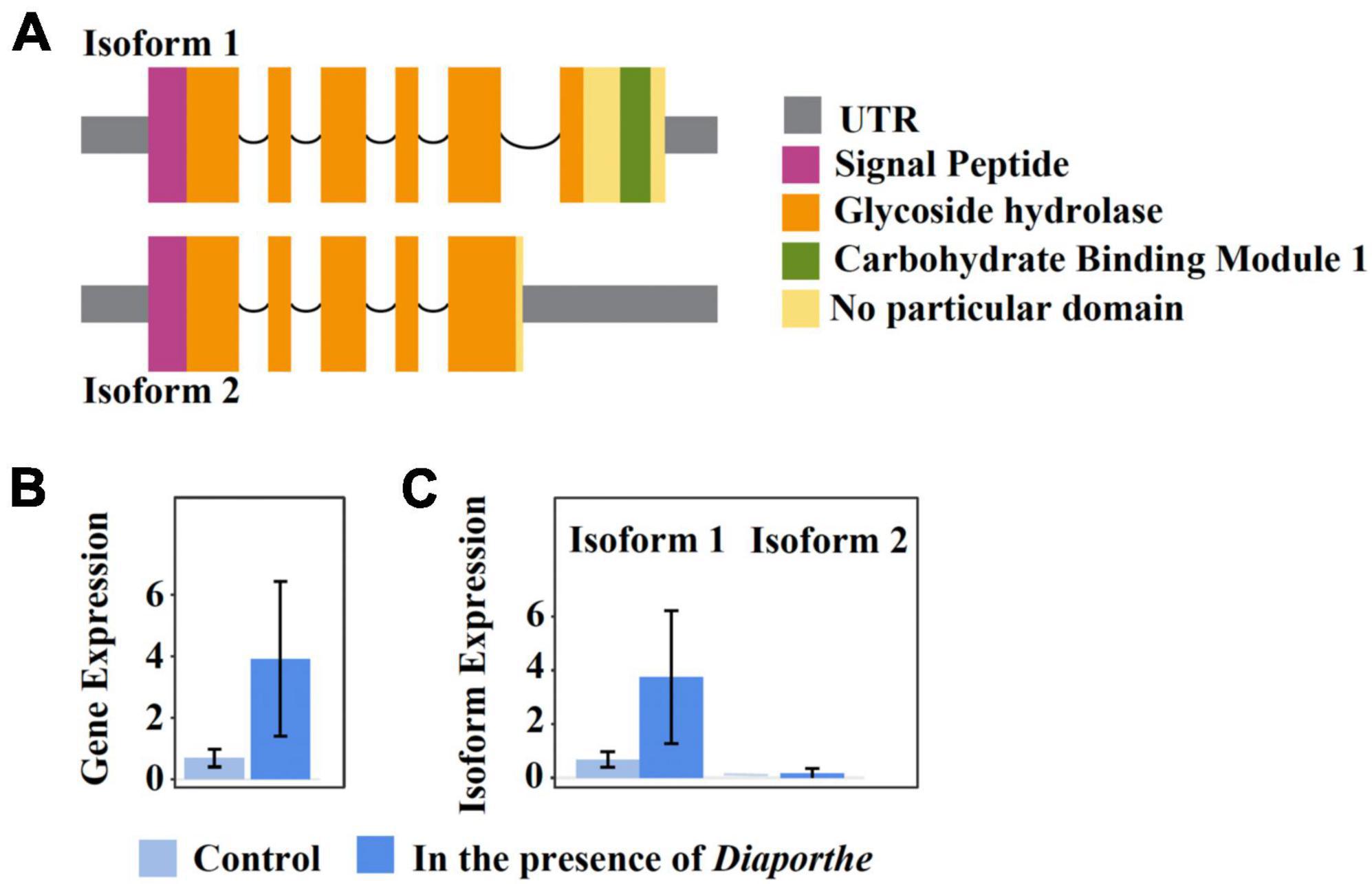
Figure 5. The isoform expression of the EC4 gene FUNEC_09200 gene when the organism was cultured alone or together with D. vaccinii. Isoform 1 is differentially upregulated in the presence of the pathogen. (A) Domain content of the two transcript isoforms. Boxes indicate UTRs and exons, and arcs symbolize introns. Protein domains were predicted using Pfam (Mistry et al., 2021). (B,C), light blue and darker blue boxes represent transcriptional gene expression when EC4 was cultured alone or together with D. vaccinii, respectively. (B) Cumulative expression of all transcripts of the gene. (C) Expression of individual isoforms. Error bars indicate a 95% confidence interval. Plots were created using IsoformSwitchAnalyzeR v2.1.2 (Vitting-Seerup and Sandelin, 2019).
The question arises how EC4 protects itself against the chitinases it secreted into the culture medium. One possibility is that EC4 camouflages its exposed chitin molecules by secreting proteins exclusively consisting of CBMs with a high affinity to chitin. The EC4 genome encodes a number of genes that could serve this purpose. The mechanism of fungal camouflage is well described in pathogenic model fungi as a strategy employed during the colonization of plants to interfere with the activation of chitin-triggered plant immunity (Pusztahelyi, 2018). It would be interesting to examine by proteomics, metabolomics and enzymatics the molecular underpinning of EC4’s chitinase-mediated suppression of Diaporthe and the means of self-protection against these potent antifungal enzymes.
4 Conclusion and outlook
EC4, an endosymbiont of Vaccinium macrocarpon, is one of the few fungal endophytes from the chaetosphaeriaceae family uniting the capacities to promote plant growth (Thimmappa et al., 2023) and to suppress plant pathogens. EC4 protects cranberry plantlets from Diaporthe infection and, in addition, inhibits various other pathogens from taxonomically diverse host plants. Furthermore, when in contact with Diaporthe vaccinii, EC4 expresses hydrolytic enzymes and secondary metabolites recognized for their roles in biocontrol. The described variation in transcription behavior when in contact with this pathogen makes EC4 a prime organism for the investigation of the molecular mechanisms involved in dynamic interactions between microbe species sharing the same plant host as their habitat. At the same time, EC4 is a promising candidate for large-scale sustainable application in industrial cranberry farming, particularly in North America, where it is an economically important crop plant.
Data availability statement
The datasets presented in this study can be found in online repositories. The names of the repository/repositories and accession number(s) can be found in this article/Supplementary material.
Author contributions
BCT: Investigation, Writing – original draft. LS: Investigation, Writing – review & editing. LF: Investigation, Writing – review & editing. MS: Investigation, Writing – review & editing. PB: Investigation, Writing – review & editing. BH: Investigation, Writing – review & editing. BFL: Conceptualization, Funding acquisition, Writing – review & editing. GB: Conceptualization, Funding acquisition, Supervision, Writing – review & editing.
Funding
The authors declare financial support was received for the research, authorship, and/or publication of this article. This research was funded by a collaborative research and development grant from the Natural Sciences and Engineering Research Council of Canada (NSERC), project title “MycAtok Project: innovating cranberry farming with beneficial symbiotic microbes” (Project/funding number: CRDPJ 514188-2017), awarded to GB and BFL, and a J. A. DeSève Excellence Scholarship awarded to BCT.
Acknowledgments
We would like to thank all collaborators of the MycAtok project, notably the cranberry producers M. Bieler, P. Michel, and P.-L. Leblanc (Canneberges Bieler Inc.), D. Landreville (Transport Gaston Nadeau Inc.), V. Godin (Pampev Inc.), K. Lachance (Enterprises Gillivert Inc.); the agronomic and strategic consultants J. Painchaud, S. Chauvette (MAPAQ), and J.-P. Deland (Oceanspray); P. Fortier (les Atocas De L’Erable) for field access to collect endophytes; and the academic co-investigators of the NSERC-CRD project, B. Findlay (Concordia University) and M. Dorais (Université Laval). We thank Dr. Richard Bélanger (Centre de recherche et d’innovation sur les végétaux, Université Laval) for providing four cranberry pathogenic strains. We thank Dr. Konstantinos A. Aliferis (Agricultural University of Athens, Greece) for providing olive-tree pathogenic strains. We also thank M. Turcotte (University of Ottawa) for critical discussions.
Conflict of interest
The authors declare that the research was conducted in the absence of any commercial or financial relationships that could be construed as a potential conflict of interest.
The authors declared that they were an editorial board member of Frontiers, at the time of submission. This had no impact on the peer review process and the final decision.
Publisher’s note
All claims expressed in this article are solely those of the authors and do not necessarily represent those of their affiliated organizations, or those of the publisher, the editors and the reviewers. Any product that may be evaluated in this article, or claim that may be made by its manufacturer, is not guaranteed or endorsed by the publisher.
Supplementary material
The Supplementary Material for this article can be found online at: https://www.frontiersin.org/articles/10.3389/fmicb.2024.1327392/full#supplementary-material
Supplementary File 1 CAZymes.
Supplementary File 2 EC4 gene expression in the presence of Diaporthe.
Supplementary File 3 Isoform analysis.
Footnotes
References
Admassie, M., Woldehawariat, Y., and Alemu, T. (2022). In vitro evaluation of extracellular enzyme activity and its biocontrol efficacy of bacterial isolates from pepper plants for the management of Phytophthora capsici. BioMed Res. Int. 2022:6778352.
Allen, T. R., Millar, T., Berch, S. M., and Berbee, M. L. (2003). Culturing and direct DNA extraction find different fungi from the same ericoid Mycorrhizal roots. New Phytol. 160, 255–272.
Almagro Armenteros, J. J., Salvatore, M., Emanuelsson, O., Winther, O., von Heijne, G., Elofsson, A., et al. (2019). Detecting sequence signals in targeting peptides using deep learning. Life Sci. Alliance 2. doi: 10.26508/lsa.201900429
Belofsky, G. N., Jensen, P. R., and Fenical, W. (1999). Sansalvamide: a new cytotoxic cyclic depsipeptide produced by a marine fungus of the genus Fusarium. Tetrahedron Lett. 40, 2913–2916.
Bendtsen, J. D., Nielsen, H., von Heijne, G., and Brunak, S. (2004). Improved prediction of signal peptides: SignalP 3.0. J. Mol. Biol. 340, 783–795.
Berch, S. M., Allen, T. R., and Berbee, M. L. (2002). “Molecular detection, community structure and phylogeny of ericoid mycorrhizal fungi,” in Diversity and Integration in Mycorrhizas: Proceedings of the 3rd International Conference on Mycorrhizas (ICOM3) Adelaide, Australia, 8–13 July 2001, Developments in Plant and Soil Sciences, eds S. E. Smith and F. A. Smith (Dordrecht: Springer).
Blin, K., Shaw, S., Augustijn, H. E., Reitz, Z. L., Biermann, F., and Alanjary, M. (2023). antiSMASH 7.0: new and improved predictions for detection, regulation, chemical structures and visualisation. Nucleic Acids Res. 51, W46–W50.
Blin, K., Shaw, S., Kloosterman, A. M., Charlop-Powers, Z., van Wezel, G. P., Medema, M. H., et al. (2021). antiSMASH 6.0: improving cluster detection and comparison capabilities. Nucleic Acids Res. 49, W29–W35.
Bolger, A. M., Lohse, M., and Usadel, B. (2014). Trimmomatic: a flexible trimmer for Illumina sequence data. Bioinforma. Oxf. Engl. 30, 2114–2120.
Bonsch, B., Belt, V., Bartel, C., Duensing, N., Koziol, M., Lazarus, C. M., et al. (2016). Identification of genes encoding squalestatin S1 biosynthesis and in vitro production of new squalestatin analogues. Chem. Commun. 52, 6777–6780.
Bougoure, D. S., and Cairney, J. W. G. (2005). Fungi associated with hair roots of Rhododendron lochiae (Ericaceae) in an Australian tropical cloud forest revealed by culturing and culture-independent molecular methods. Environ. Microbiol. 7, 1743–1754.
Cantarel, B. L., Coutinho, P. M., Rancurel, C., Bernard, T., Lombard, V., and Henrissat, B. (2009). The Carbohydrate-Active EnZymes database (CAZy): an expert resource for Glycogenomics. Nucleic Acids Res. 37, D233–D238.
Chen, X.-L., Zhang, L.-J., Li, F.-G., Fan, Y.-X., Wang, W.-P., Li, B.-J., et al. (2015). Synthesis and antifungal evaluation of a series of maleimides. Pest Manag. Sci. 71, 433–440.
de Castro, E., Sigrist, C. J. A., Gattiker, A., Bulliard, V., Langendijk-Genevaux, P. S., Gasteiger, E., et al. (2006). ScanProsite: detection of PROSITE signature matches and ProRule-associated functional and structural residues in proteins. Nucleic Acids Res. 34, W362–W365.
de la Bastide, M., and McCombie, W. R. (2007). Assembling genomic DNA sequences with PHRAP. Curr. Protoc. Bioinforma. doi: 10.1002/0471250953.bi1104s17
Divilov, K., and Walker, D. R. (2016). Reaction of Diaporthe longicolla to a strain of Sarocladium kiliense. Biocontrol Sci. Technol. 26, 938–950.
Dobin, A., Davis, C. A., Schlesinger, F., Drenkow, J., Zaleski, C., Jha, S., et al. (2013). STAR: ultrafast universal RNA-seq aligner. Bioinforma. Oxf. Engl. 29, 15–21.
Fang, S., Hou, X., Qiu, K., He, R., Feng, X., and Liang, X. (2020). The occurrence and function of alternative splicing in fungi. Fungal Biol. Rev. 34, 178–188.
Finn, R. D., Mistry, J., Tate, J., Coggill, P., Heger, A., Pollington, J. E., et al. (2010). The Pfam protein families database. Nucleic Acids Res. 38, D211–D222.
Frascella, A., Sarrocco, S., Mello, A., Venice, F., Salvatici, C., Danti, R., et al. (2022). Biocontrol of Phytophthora xcambivora on Castanea sativa: selection of local Trichoderma spp. isolates for the management of ink disease. Forests 13:1065.
Geiger, A., Karácsony, Z., Geml, J., and Váczy, K. Z. (2022). Mycoparasitism capability and growth inhibition activity of Clonostachys rosea isolates against fungal pathogens of grapevine trunk diseases suggest potential for biocontrol. PLoS One 17:e0273985. doi: 10.1371/journal.pone.0273985
Geraldine, A. M., Lopes, F. A. C., Carvalho, D. D. C., Barbosa, E. T., Rodrigues, A. R., Brandão, R. S., et al. (2013). Cell wall-degrading enzymes and parasitism of sclerotia are key factors on field biocontrol of white mold by Trichoderma spp. Biol. Control 67, 308–316.
Gordon, D., Abajian, C., and Green, P. (1998). Consed: a graphical tool for sequence finishing. Genome Res. 8, 195–202.
Ha, S. T. T., Choi, B., and In, B.-C. (2021). Nature and regulation of Botrytis cinerea in Rosa hybrida. Flower Res. J. 29, 129–137.
Hai, Y., and Tang, Y. (2018). Biosynthesis of long-chain N-acyl amide by a truncated PKS-NRPS hybrid megasynthase in fungi. J. Am. Chem. Soc. 140, 1271–1274.
Harrison, J. G., and Griffin, E. A. (2020). The diversity and distribution of endophytes across biomes, plant phylogeny and host tissues: how far have we come and where do we go from here? Environ. Microbiol. 22, 2107–2123.
Hervé, C., Rogowski, A., Blake, A. W., Marcus, S. E., Gilbert, H. J., and Knox, J. P. (2010). Carbohydrate-binding modules promote the enzymatic deconstruction of intact plant cell walls by targeting and proximity effects. Proc. Natl. Acad. Sci. 107, 15293–15298.
Hisano, M., Bruschini, H., Nicodemo, A. C., and Srougi, M. (2012). Cranberries and lower urinary tract infection prevention. Clinics 67, 661–667.
Ibrahim, H. M. M., Kusch, S., Didelon, M., and Raffaele, S. (2021). Genome-wide alternative splicing profiling in the fungal plant pathogen Sclerotinia sclerotiorum during the colonization of diverse host families. Mol. Plant Pathol. 22, 31–47.
Jadhav, H. P., Shaikh, S. S., and Sayyed, R. Z. (2017). “Role of hydrolytic enzymes of rhizoflora in biocontrol of fungal phytopathogens: an overview,” in Rhizotrophs: Plant Growth Promotion to Bioremediation, Microorganisms for Sustainability, ed. S. Mehnaz (Singapore: Springer).
Käll, L., Krogh, A., and Sonnhammer, E. L. L. (2004). A combined transmembrane topology and signal peptide prediction method. J. Mol. Biol. 338, 1027–1036.
Kawabe, M., Okabe Onokubo, A., Arimoto, Y., Yoshida, T., Azegami, K., Teraoka, T., et al. (2011). GMC oxidoreductase, a highly expressed protein in a potent biocontrol agent Fusarium oxysporum Cong:1-2, is dispensable for biocontrol activity. J. Gen. Appl. Microbiol. 57, 207–217.
Kosola, K. R., Workmaster, B. A. A., and Spada, P. A. (2007). Inoculation of cranberry (Vaccinium macrocarpon) with the ericoid mycorrhizal fungus Rhizoscyphus ericae increases nitrate influx. New Phytol. 176, 184–196.
Kowsari, M., Motallebi, M., and Zamani, M. (2014). Protein engineering of chit42 towards improvement of chitinase and antifungal activities. Curr. Microbiol. 68, 495–502.
Krogh, A., Larsson, B., von Heijne, G., and Sonnhammer, E. L. (2001). Predicting transmembrane protein topology with a hidden Markov model: application to complete genomes. J. Mol. Biol. 305, 567–580.
Latgé, J.-P. (2007). The cell wall: a carbohydrate armour for the fungal cell. Mol. Microbiol. 66, 279–290.
Li, B., and Dewey, C. N. (2011). RSEM: accurate transcript quantification from RNA-Seq data with or without a reference genome. BMC Bioinformatics 12:323. doi: 10.1186/1471-2105-12-323
Linder, M., Salovuori, I., Ruohonen, L., and Teeri, T. T. (1996). Characterization of a double cellulose-binding domain. synergistic high affinity binding to crystalline cellulose. J. Biol. Chem. 271, 21268–21272.
Lombard, V., Golaconda Ramulu, H., Drula, E., Coutinho, P. M., and Henrissat, B. (2014). The carbohydrate-active enzymes database (CAZy) in 2013. Nucleic Acids Res. 42, D490–D495.
Lopes, F. A. C., Steindorff, A. S., Geraldine, A. M., Brandão, R. S., Monteiro, V. N., Júnior, M. L., et al. (2012). Biochemical and metabolic profiles of Trichoderma strains isolated from common bean crops in the Brazilian Cerrado, and potential antagonism against Sclerotinia sclerotiorum. Fungal Biol. 116, 815–824.
Love, M., Ahlmann-Eltze, C., Forbes, K., Anders, S., and Huber, W. (2021). DESeq2: Differential Gene Expression Analysis Based on the Negative Binomial Distribution. Available Online at: https://doi.org/10.18129/B9.bioc.DESeq2.
Medema, M. H., Blin, K., Cimermancic, P. de Jager, V., Zakrzewski, P., Fischbach, M. A., et al. (2011). antiSMASH: rapid identification, annotation and analysis of secondary metabolite biosynthesis gene clusters in bacterial and fungal genome sequences. Nucleic Acids Res. 39, W339–W346. doi: 10.1093/nar/gkr466
Mason, C. J., Zeldin, E. L., Currie, C. R., Raffa, K. F., and McCown, B. H. (2014). Populations of uncultivated American cranberry in sphagnum bog communities harbor novel assemblages of Actinobacteria with antifungal properties. Botany 92, 589–595.
Michalecka, M., Bryk, H., and Seliga, P. (2017). Identification and characterization of Diaporthe vaccinii Shear causing upright dieback and viscid rot of cranberry in Poland. Eur. J. Plant Pathol. 148, 595–605.
Mistry, J., Chuguransky, S., Williams, L., Qureshi, M., Salazar, G. A., Sonnhammer, E. L. L., et al. (2021). Pfam: the protein families database in 2021. Nucleic Acids Res. 49, D412–D419.
Neeraja, C., Anil, K., Purushotham, P., Suma, K., Sarma, P., Moerschbacher, B. M., et al. (2010). Biotechnological approaches to develop bacterial chitinases as a bioshield against fungal diseases of plants. Crit. Rev. Biotechnol. 30, 231–241.
Ogle, D. H., Doll, J. C., Wheeler, A. P., and Dinno, A. (2023). FSA: Simple Fisheries Stock Assessment Methods. R Package version 0.9.5.
Oudemans, P. V., Caruso, F. L., and Stretch, A. W. (1998). Cranberry fruit rot in the Northeast: a complex disease. Plant Dis. 82, 1176–1184.
Perlatti, B., Nichols, C. B., Lan, N., Wiemann, P., Harvey, C. J. B., Alspaugh, J. A., et al. (2020). Identification of the antifungal metabolite chaetoglobosin P from Discosia rubi using a Cryptococcus neoformans inhibition assay: insights into mode of action and biosynthesis. Front. Microbiol. 11:1766. doi: 10.3389/fmicb.2020.01766
Pierleoni, A., Martelli, P. L., and Casadio, R. (2008). PredGPI: a GPI-anchor predictor. BMC Bioinformatics 9:392. doi: 10.1186/1471-2105-9-392
Pirttilä, A. M., Mohammad Parast Tabas, H., Baruah, N., and Koskimäki, J. J. (2021). Biofertilizers and biocontrol agents for agriculture: how to identify and develop new potent microbial strains and traits. Microorganisms 9:817.
Preussner, M., Gao, Q., Morrison, E., Herdt, O., Finkernagel, F., Schumann, M., et al. (2020). Splicing-accessible coding 3’UTRs control protein stability and interaction networks. Genome Biol. 21:186.
Pusztahelyi, T. (2018). Chitin and chitin-related compounds in plant-fungal interactions. Mycology 9, 189–201.
Quevillon, E., Silventoinen, V., Pillai, S., Harte, N., Mulder, N., Apweiler, R., et al. (2005). InterProScan: protein domains identifier. Nucleic Acids Res. 33, W116–W120.
Reveglia, P., Masi, M., and Evidente, A. (2020). Melleins—intriguing natural compounds. Biomolecules 10:772.
Sabaratnam, S., Wood, B., and Nabetani, K. (2016). Fruit rot pathogens and their impact on cranberry production in British Columbia [2014 Study], 14. Available online at: https://www.bccranberries.com/pdfs/researchreports/2014/Sabaratnam-Impact-Fruit-Rot-Pathogens-2014.pdf (accessed May 19, 2023).
Salhi, L. N., Bustamante Villalobos, P., Forget, L., Burger, G., and Lang, B. F. (2022). Endosymbionts in cranberry: diversity, effect on plant growth, and pathogen biocontrol. Plants People Planet 4, 511–522.
Stevens, P. F., Luteyn, J., Oliver, E. G. H., Bell, T. L., Brown, E. A., and Crowden, R. K. (2004). “Ericaceae,” in Flowering Plants Dicotyledons: Celastrales, Oxalidales, Rosales, Cornales, Ericales, The Families and Genera of Vascular Plants, ed. K. Kubitzki (Berlin: Springer).
Thimmappa, B. C., Salhi, L. N., Forget, L., Sarrasin, M., Bustamante Villalobos, P., Lang, B. F., et al. (2023). Nuclear genome sequence and gene expression of an intracellular fungal endophyte stimulating the growth of cranberry plants. J. Fungi 9:126.
Trincado, J. L., Entizne, J. C., Hysenaj, G., Singh, B., Skalic, M., Elliott, D. J., et al. (2018). SUPPA2: fast, accurate, and uncertainty-aware differential splicing analysis across multiple conditions. Genome Biol. 19:40.
Trost, B. M., and Osipov, M. (2015). Recent advances on the total syntheses of the communesin alkaloids and perophoramidine. Chem. Weinh. Bergstr. Ger. 21, 16318–16343.
University of Massachusetts Cranberry Station (2016). Cent. Agric. Food Environ. Available Online at: https://ag.umass.edu/news-events/highlights/university-of-massachusetts-cranberry-station (accessed 8 Jun, 2023).
Vitting-Seerup, K., and Sandelin, A. (2019). IsoformSwitchAnalyzeR: analysis of changes in genome-wide patterns of alternative splicing and its functional consequences. Bioinforma. Oxf. Engl. 35, 4469–4471.
Vos, C. M. F., De Cremer, K., Cammue, B. P. A., and De Coninck, B. (2015). The toolbox of Trichoderma spp. in the biocontrol of Botrytis cinerea disease. Mol. Plant Pathol. 16, 400–412.
Yang, H., Zhao, X., Liu, C., Bai, L., Zhao, M., and Li, L. (2018). Diversity and characteristics of colonization of root-associated fungi of Vaccinium uliginosum. Sci. Rep. 8:15283.
Keywords: alternative splicing, biocontrol, Codinaeella sp., Diaporthe vaccinii, hydrolytic enzymes, secondary metabolites, sustainable agriculture, Vaccinium macrocarpon Aiton (American cranberry)
Citation: Thimmappa BC, Salhi LN, Forget L, Sarrasin M, Bustamante Villalobos P, Henrissat B, Lang BF and Burger G (2024) A biofertilizing fungal endophyte of cranberry plants suppresses the plant pathogen Diaporthe. Front. Microbiol. 15:1327392. doi: 10.3389/fmicb.2024.1327392
Received: 24 October 2023; Accepted: 15 January 2024;
Published: 02 February 2024.
Edited by:
Manoj Kumar Solanki, University of Silesia in Katowice, PolandReviewed by:
Monica L. Gerth, Victoria University of Wellington, New ZealandSakineh Abbasi, Institut National de Recherche Pour L’agriculture, L’alimentation et L’environnement (INRAE), France
Copyright © 2024 Thimmappa, Salhi, Forget, Sarrasin, Bustamante Villalobos, Henrissat, Lang and Burger. This is an open-access article distributed under the terms of the Creative Commons Attribution License (CC BY). The use, distribution or reproduction in other forums is permitted, provided the original author(s) and the copyright owner(s) are credited and that the original publication in this journal is cited, in accordance with accepted academic practice. No use, distribution or reproduction is permitted which does not comply with these terms.
*Correspondence: Bhagya C. Thimmappa, YmhhZ3lhLmMudGhpbW1hcHBhQHVtb250cmVhbC5jYQ==
†Present address: Lila Naouelle Salhi, Laboratoire de santé publique du Quebec, Institut national de santé publique du Quebec, Sainte-Anne-de-Bellevue, QC, Canada