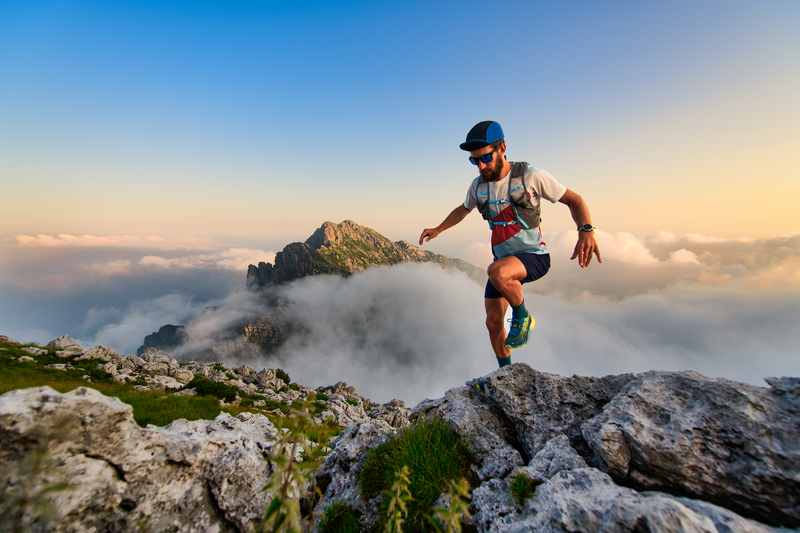
94% of researchers rate our articles as excellent or good
Learn more about the work of our research integrity team to safeguard the quality of each article we publish.
Find out more
REVIEW article
Front. Microbiol. , 03 April 2024
Sec. Infectious Agents and Disease
Volume 15 - 2024 | https://doi.org/10.3389/fmicb.2024.1326369
This article is part of the Research Topic Latest findings on Leishmania parasites for better Vaccine Design and Drug Development View all 7 articles
Leishmaniasis is a vector-borne disease caused by the protozoan parasite of Leishmania genus and is a complex disease affecting mostly tropical regions of the world. Unfortunately, despite the extensive effort made, there is no vaccine available for human use. Undoubtedly, a comprehensive understanding of the host-vector-parasite interaction is substantial for developing an effective prophylactic vaccine. Recently the role of sandfly saliva on disease progression has been uncovered which can make a substantial contribution in vaccine design. In this review we try to focus on the strategies that most probably meet the prerequisites of vaccine development (based on the current understandings) including live attenuated/non-pathogenic and subunit DNA vaccines. Innovative approaches such as reverse genetics, CRISP/R-Cas9 and antibiotic-free selection are now available to promisingly compensate for intrinsic drawbacks associated with these platforms. Our main goal is to call more attention toward the prerequisites of effective vaccine development while controlling the disease outspread is a substantial need.
Neglected tropical diseases (NTDs) are diseases of poverty that impose a high socio-economic burden on more than 1 billion people worldwide, mainly in tropical and subtropical areas. Despite substantial progress in reducing the overall burden, the current road maps toward full eradication still need further improvement. Among the 20 different diseases in this category, leishmaniasis remains an unresolved threat to global health with an estimated annual rate of 700,000 to 1 million new cases leading to 20,000–30,000 deaths each year.1
Leishmaniasis is a vector-borne disease caused by the protozoan parasite of Leishmania genus and is a complex disease affecting mostly tropical regions of the world. The parasite with a dynamic genome has evolved to advantage a vector (sand fly) mediated transmission for successful disease establishment. Decades of labor-intensive hard work has partially deconvoluted the host-pathogen interaction which is partly controlled by different components deposited into the human skin through sand fly proboscis (Serafim et al., 2021). These include sand fly salivary proteins (Lestinova et al., 2017), parasite secretory gel (Sainz and de la Maza, 2014), exosomes carrying virulence factors (Dong et al., 2019, 2021), parasite associated double-stranded RNA viruses including Leishmania RNA Viruses (LRVs) (Rossi and Fasel, 2018) or non-LRV (Grybchuk et al., 2020) viruses inoculated by parasite or parasite exosomes, and even the gut microbiota of sand fly (Omondi and Demir, 2021). Sand fly saliva, is composed of different immunomodulatory components among which chemotactic factors for neutrophils are recently characterized (Guimaraes-Costa et al., 2021), LRVs trigger TLR-3 for pro-inflammatory cytokine production (Hartley et al., 2012), gut microbiota induces IL-1b production via inflammasome activation (Dey et al., 2018) and parasite dependent factors induce Toll-like receptors (TLR) including TLR-2, TLR-4, TLR-7, and TLR-9 (Masoudzadeh et al., 2020a,b). The cumulative consequence of this inflammatory response is the massive neutrophilic recruitment to the bite site early after infection (Peters et al., 2008). While some parasites are killed by neutrophils like Leishmania (L.) amazonensis, others are able to survive by skipping the killing mechanisms like L. major (Passelli et al., 2021). Depending on the parasite species, host related immune response and genetic background, neutrophil recruitment to the bite site can then affect the outcome of the infection (Peters and Sacks, 2009). Although neutrophil function has shown protective in some experimental leishmaniasis (de Souza Carmo et al., 2010; Carlsen et al., 2015), in other experiments, massive neutrophil recruitment has correlated with more prominent lesions by providing a permissive environment for disease establishment (Ronet et al., 2019; Chaves et al., 2020).
Unfortunately, despite the high burden of the disease around the world (more than 1 billion people live in areas endemic for leishmaniasis and are at risk of infection with an annual estimation of 30,000 new cases of VL and more than 1 million new cases of CL), there is no vaccine available for human use. All we know so far is that full protection against Leishmania parasite-induced infection generally requires CD4+-Th1 response. Therefore, extensive effort has been made to generate memory Th1 response advantaging so many different vaccine platforms with promising experimental results (Costa et al., 2011; Dinc, 2022).
It was not until recently when it was realized that vector derived compounds abrogate vaccine efficacy in sandfly-derived infection (Peters et al., 2009). This has been concluded from the comparisons made between leishmanization and different killed or multiprotein subunit formulations (already accepted as promising candidates) taking into account the sand fly versus needle challenge (Seyed et al., 2018). ALM-CpG (Autoclaved Leishmania major adjuvanted with CpG oligonucleotide) as killed vaccine (Peters et al., 2009) in addition to KSAC-GLA.SE [recombinant protein adjuvanted with stable emulsion (SE) formulation of glucopyranosyl lipid A (GLA)] and L110F-GLA.SE as poly-protein vaccines (Peters et al., 2012) have been compared in protection efficacy to leishmanization in natural sand fly challenge of C57BL/6 mice. Although all these formulations potentially protect against needle Leishmania challenge when it comes to parasite load, but none of them are able to emulate the protection conferred by leishmanization after sand fly challenge in C57BL/6 mice. Of note, the same KSAC-GLA-SE formulation examined in BALB/c mice conferred protection against Leishmania major transmitted by sand fly bites (regarding lesion size and parasite burden) due to a strong immune response to Leishmania antigens by memory T cells after sand fly transmission of the parasite (Gomes et al., 2012). The sustained and massive neutrophilic recruitment up to 28 days post sand fly infection in C57BL/6 mice was the major complicating factor compared to needle challenge which inversely dampens the vaccine’s protection potential. It was postulated that leishmanization potentially modulates these early inflammatory events post sand fly challenge by a rapid and robust IFN-γ mediated intervention. It was recently unraveled that this rapid and robust IFN-γ response cannot be provided by memory Th1 cells at the time of infection since they are activated and proliferate at later time points (Seyed and Rafati, 2021). Generally speaking, this means that for achieving a potential vaccine against leishmaniasis, we have to recognize the correlates of protective immunity besides Th1 memory T cells.
Among the different platforms examined so far (Kaye et al., 2021; Volpedo et al., 2021), leishmanization remains the only vaccine formulation effective against Leishmania infection in endemic areas (although not approved for general use in humans) (Pacheco-Fernandez et al., 2021). The lessons learned from leishmanization has led to the “concomitant Immunity” concept which means “ongoing chronic infection” (Sacks, 2014). This primary subclinical chronic infection with life-long persistent parasites at low levels after healing, induces major subsets of effector and memory T cells which play a critical role early after sand fly bite at secondary infection site. The immune correlates associated with “concomitant Immunity” include skin-resident memory T cells (TRM) and effector Ly6C+ T cells (TEFF) besides Th1 central (TCM) and effector memory (TEM) cells (Hohman and Peters, 2019; Seyed and Rafati, 2021). Ly6C+ TEFF cells (Peters et al., 2014), and TRM cells (Glennie and Scott, 2016; Scott, 2020) play an indispensable role in protection against sand fly deposition of the parasite. They promote a non-permissive environment for parasite growth in inflammatory monocytes recruited to the bite site by providing early (within hours) and robust local IFN-γ. This means that timing is everything. Hohman et al. clearly demonstrated that permissive phagocytic host cells’ activation by pre-activated Ly6C+ TEFF cells is the pre-requisite for Th1 induced protection (Hohman et al., 2021). Otherwise, delayed Th1 central memory cell activation does not confer full protection after the permissive environment is established by skin-deposited immunomodulatory sand fly components (Hohman et al., 2021). These cells are well characterized in mouse models and we still need to identify the human counterparts. Mouse TEFF cells which rapidly accumulate at the bite site, are ready to take action without proliferation and produce large amounts of IFN-γ to provide a timely and robust response. These cells are very much antigen dependent and disappear in the absence of antigen. TRM cells instead are non-circulating tissue resident memory cells which mediate CCL2 dependent recruitment of inflammatory monocytes highly expressing MHCII molecules and producing active anti-leishmanial metabolites mainly nitric oxide and reactive oxygen derivatives.
As described, the prominent characteristic of leishmanization-induced protection is the persistence of parasite after cure (Belkaid et al., 2001). In other words, long-lasting antigen presentation remains the key challenging point for the alternative platforms other than leishmanization and mainly the subunit vaccines. In the absence of distinguished TRM and Ly6C+ TEFF cells, permissive phagocytic cells lead to parasite propagation and disease establishment early after sand fly bite. Therefore, for a vaccination strategy to succeed, pre-activated Ly6C+ TEFF (Hohman and Peters, 2019) and TRM (Glennie et al., 2015) cells are necessary without which the formulation will most likely fail in field trials even in the presence of memory T cells. Although it still remains elusive how to best generate TRM and Ly6C+ TEFF cells by vaccination, live attenuated parasites and subunit multivalent DNA vaccines are here suggested as alternatives of leishmanization for long term antigen presentation. We have discussed the advantages and have suggested the dendritic-cell based vaccines in another paper (Seyed et al., 2018). Here, we summarize the evidence why live attenuated parasites and subunit multivalent DNA vaccine strategies can be attractive for more intensive investigation. Several innovative approaches including reverse genetics, CRISPR/Cas9 (clustered regularly interspaced palindromic repeats) technology and antibiotic free selection are now available to overcome the intrinsic drawbacks of these platforms and make waves in the future of vaccination against leishmaniasis. The rest of the vaccination platforms, although examined in experimental models or even in clinical trials, remain to address the question whether they can provide a long-lasting antigen presentation or not.
Live attenuated vaccines of different pathogens have successfully eradicated many intracellular pathogens such as poliovirus and smallpox (variola virus) and have controlled many others such as measles, mumps and rubella viruses (Plotkin, 2014). Mimicking the normal course of the infection with natural molecular patterns to induce innate immunity, complete set of antigens delivered and above all, subclinical infection which lasts longer than any protein subunit formulation, are among the major factors which prioritize the live vaccines (Silvestre et al., 2008). These traits are in-line with the Leishmania vaccination goal and has led to the intensive effort for attenuating different parasite species. So far various genes have been explored as targets of attenuation both in cutaneous (Zabala-Penafiel et al., 2020) and visceral (Pandey et al., 2020) leishmaniasis with the aim of generating long-lived non-virulent parasites unable to revert back to the wild type parental parasite. Among others, lpg2−/− L. major has shown a long persistence up to 2 years in BALB/c mice without pathology (Spath et al., 2003). This mutant strain is cleared easily within macrophages and is assumed to persist in cells other than macrophages in vivo. This model increased hopes for a protective vaccine with encouraging results in mouse models (Uzonna et al., 2004) but the dreams were ruined when the compensatory mutants (lpg2−/− REV) were detected (Spath et al., 2004). This means that a major concern for a parasite with a dynamic genome is the ability to compensate for the mutant gene and revert back into the pathogenic parasite which is the top concern of regulatory authorities regarding live attenuated pathogens. Thus, in addition to the persistence without pathology, choosing for virulence genes which cannot be compensated by other genes is of paramount importance in generating live attenuated Leishmania strains.
Recently, genetically modified live attenuated Centrin−/− (Cen−/−) L. donovani (Bhattacharya et al., 2016), L. major (Zhang et al., 2020) and L. mexicana (Karmakar et al., 2022) mutants have generated promising results as vaccine candidates (Volpedo et al., 2022). Centrin, a calcium-binding cytoskeletal protein, is concerned with the basal body duplication and segregation in lower eukaryotes (Salisbury, 2004). The lack of basal body formation and cytokinesis leads to apoptosis and G2/M phase cell arrest. Therefore, Centrin mutation inhibits amastigote proliferation in macrophages leading to immunological clearance as suggested (Selvapandiyan et al., 2001, 2004). LmCen−/− parasites represent an equally effective but safer alternative to leishmanization for the protection induced against L. major transmitted by sand fly bite (Silvestre et al., 2008) and also cross protection against more virulent L. donovani (Karmakar et al., 2022). In particular, mice immunized with LmCen−/− parasites compared to mice healed from L. major leishmanization, have generated a significantly higher pro-inflammatory immune response, characterized by CD4+CD44highLy6C+T-bet+ IFN-γ+ effector T cells (Zhang et al., 2020) and tissue resident memory (TRM) T cell responses (Ismail et al., 2022). This is the first report of a live attenuated formulation that enables Ly6C+ TEFF and TRM induction without a compensatory reversion. Owing to the fast-growing CRISPR/Cas9 gene editing technology, LmCen−/− mutant is now at the front line to initiate human clinical trials since the parasite lacks antibiotic resistance genes which is another top concern of regulatory authorities about genetically modified pathogens (Zhang et al., 2020). CRISPR/Cas9-mediated gene targeting and editing facilitates deletion of essential genes (either single or multigene families) to observe the functional and phenotypic effects on living cells in a time-dependent manner (Zhang and Matlashewski, 2015; Adaui et al., 2020). The fast development of this technology will certainly accelerate the production of knockout mutants in Leishmania instead of homologous recombination-based gene replacement making more live attenuated parasites available in near future (Singh et al., 2022; Moreira et al., 2023).
With the advent of reverse instead of forward genetics and with complete parasite genomes now available due to new technologies for whole genome sequencing, new horizons have appeared. Non-pathogenic lizard-isolated Leishmania (L.) tarentolae is a precious tool with the whole genome sequence now available. In a study by Azizi et al. searching for virulence factors in L. tarentolae, it was realized that the lack of pathogenicity is not LPG3, CPB, GP63 and Amastin dependent (Azizi et al., 2009) which reflected the importance of other genes either unique to L. tarentolae or missing from this species for the nonpathogenic potential of this lizard parasite. Later, the whole genome of this non-pathogenic parasite was sequenced and compared to L. major, L. infantum and L. braziliensis by Reymond et al (Raymond et al., 2012). This “subtractive genomics” study identified 95 predicted coding sequences unique to L. tarentolae and 250 genes present in the pathogenic species but absent in L. tarentolae which are mainly associated with the amastigote stage. This explained in part why L. tarentolae proliferates less well in human macrophages and why these parasites are mostly reported as free organisms in the lizards. Fortunately, reverse genetics tools can unveil new virulence factors out of these unique or missing genes associated with non-pathogenic L. tarentolae. Duncan et al. have described these reverse genetics tools in different categories (Duncan et al., 2017) including tools for assessing if Leishmania genes are essential for cellular proliferation like plasmid shuffle (McCall et al., 2015), DiCre (dimerizable Cre) recombinase (Madeira da Silva and Beverley, 2010; Schindler et al., 2015) and CRISPR/Cas9 (Zhang et al., 2017) or tools for regulating gene expression such as RNAi (de Paiva et al., 2015), Tetracycline inducible gene expression (Ishemgulova et al., 2016) or “DiCre recombinase inducible gene expression” (Santos et al., 2017). Tools for endogenous tagging and regulating protein levels are also introduced such as “endogenous tagging” and “conditional protein destabilization” (Damerow et al., 2015; Dean et al., 2015). Fortunately, all of these new technologies are applicable for manipulating pathogenic Leishmania genomes and/or proteomes with the hope of finding novel virulence factors in comparison to non-pathogenic strains such as L. tarentolae.
Leishmania tarentolae has also been investigated as vaccines against human pathogenic Leishmania species (Bandi et al., 2023). After Breton et al. showed that intra-peritoneal administration of live L. tarentolae in BALB/c mice induces a Th1 pathway with significant protection against L. donovani infectious challenge (Breton et al., 2005), non-engineered live L. tarentolae promastigotes were assayed as candidate vaccines adjuvanted with CpG oligonucleotides (Keshavarzian et al., 2020), or chitin microparticles against L. major (Haghdoust et al., 2022; Noroozbeygi et al., 2023). Others employed genetically modified strains of L. tarentolae, engineered for overexpression of antigens from human pathogenic Leishmanias (Saljoughian et al., 2013; Topuz Ata et al., 2023) or antigens from the vector saliva (Katebi et al., 2015; Lajevardi et al., 2022). These studies generally showed that L. tarentolae induces protection in animal models against pathogenic species, including L. infantum and L. major (Mendoza-Roldan et al., 2022). We still need to further address the induction of Ly6C+ TEFF and/or TRM cells by this platform. L. tarentolae is able to enter human phagocytic cells and differentiate into amastigote like forms. However, there is no clear evidence for their efficient replication within macrophages (Raymond et al., 2012). Therefore, the top priority is to determine whether the parasite persists in vivo and if yes for how long and in which cells. A recently published paper has detected L. tarentolae in blood samples from dogs and lizards in an endemic area of southern Italy and also in sand flies. Amazingly, at the cytology of lizard blood, Leishmania spp. amastigote-like forms were detected in erythrocytes (Mendoza-Roldan et al., 2021).
With the advent of molecular genetics and recombinant technologies, DNA-based vaccines (also called genetic vaccines) which advantage the bacterial plasmid constructs, moved to the frontline to pioneer for vaccine design against infectious diseases (Gary and Weiner, 2020). They are more stable in biological systems and induce strong and long-lasting antigen-specific cell-mediated immune responses due to the sustained in vivo expression of antigen, efficient antigen presentation and the presence of in-built innate immunity-stimulatory CpG motifs as adjuvant (McCluskie et al., 2000; Lee et al., 2018). Plasmids are small circular structures engineered to accommodate genes from different organisms for in vivo production. The plasmid backbone is composed of eukaryotic sequences for protein production in eukaryotes and also prokaryotic sequences for replication and selection in laboratory production steps.
Remarkable advantages of DNA vaccines include induction of a prolonged cellular and humoral immunity, ease of large-scale production, adaptability to encode several antigens and above all self-adjuvanicity. DNA vaccines are currently licensed for several animal diseases (Kutzler and Weiner, 2008). In many cases of cancer, they are also used as therapeutic vaccines and they have even reached the second and third phases of human preventive vaccine in clinical trials (Lopes et al., 2019). However, the potential of DNA vaccines has not been realized due to the poor cellular uptake of DNA in vivo, resulting in poor immunogenicity. Innovative delivery systems are now available to improve cellular uptake and consequently the immunogenicity (Lim et al., 2020; Ho et al., 2021).
In Leishmania vaccine research, DNA vaccines have made an important contribution because of long-lasting Th1 immunity conferred against the parasite. One of the major factors in sustained Th1 response differentiation is IL-12 cytokine produced by dendritic cells which is well provided by DNA vaccination instead of protein plus IL-12 (Gurunathan et al., 1997). In 2001, Mendez et al. compared the DNA vaccination versus protein/recombinant IL-12 conferred protection in low dose infectious challenge with L. major (intradermal inoculation of 100–1,000 metacyclic promastigotes). Three months post-immunization a potential sustained protective immunity due to DNA vaccination versus a partial protection due to protein/IL-12 vaccination was observed (Mendez et al., 2001). The durable protection in mice vaccinated with DNA was associated with the recruitment of both CD8+ and CD4+ T cells to the site of intradermal challenge and with IFN-γ production by CD8+ T cells in challenge draining lymph nodes. This is attributable to the sustained IL-12 production besides low levels of persistent antigen produced by DNA structures (Gurunathan et al., 2000). Although these results might well correspond with the long-lived memory T cells which potentially protect against sand fly components-free challenge, but are invaluable respecting the more sustained antigen presentation by DNA structures compared to naked-recombinant protein formulations.
After introducing the role of sand fly proteins in protection against sand fly transmitted infection (Kamhawi, 2000; Kamhavi et al., 2018), other groups evaluated the durability of protection conferred against more natural infection. Salivary Gland Homogenates (SGH) of the vector was co-injected with intradermal low dose parasite after DNA vaccine immunization encoding Phlebotomus (Ph.) papatasi Sp15 protein (a 15 kilodalton protein). Three months post booster immunization, the protection was quite comparable to that achieved 2 weeks post vaccination with a significant reduction in both lesion size and parasite number (Valenzuela et al., 2001). Although this group never evaluated the biomarkers of long-lasting protection (Ly6C+ T cells and TRM cells) required for protection against SGH-induced inflammatory response, but the results raised the hope.
Recently Davarpanah et al. advantaged the Lactococcus lactis based-DNA transfer technology to evaluate the protection against Ph. papatasi SGH co-injected with L. major parasite. Six months following immunization with Ph. papatasi Sp15 expressing L. lactis, the lesion size and parasite number was significantly lower in Sp15 vaccinated animals as compared to control groups due to a Th1 polarized immune response (Davarpanah et al., 2020). Again, the SGH-required protection biomarkers were not evaluated, however a significant protection almost comparable to the early detected protection (within 2 weeks post challenge) could be a good sign.
Luise et al. recently conducted an experiment to evaluate the claim that intradermal (i.d.) immunization or scarification effectively generates TRM cells in the skin (Sangare et al., 2009). To address this issue, they have compared the generation of skin-resident T cells and protection against L. major induced cutaneous leishmaniasis following intra-muscular and intra-dermal DNA immunizations routes. A plasmid DNA encoding Leishmania phosphoenolpyruvate carboxykinase was injected intradermaly by electroporation and also intramuscularly. As indicated, intradermal immunization better protected against L. major challenge. Of note, the protection level was comparable to the leishmanized-immune mice previously infected with parasites both in lesion size and parasite burden. They concluded that the intradermal route may be most efficient at generating TRM cells and protection against Leishmania parasites (Louis et al., 2019). This robust document indicating the TRM generation following DNA immunization by intradermal route, further pushes the DNA vaccine strategy to the top of the list of candidate vaccine formulations against leishmaniasis.
Despite the fact that specific complications such as DNA insertion into the chromosome and autoimmunity or tolerance have not been reported (Liu, 2011), unfortunately no DNA-based vaccine is approved for human infections including Leishmaniasis. Although the immunogenicity of DNA vaccines as a major concern is under intensive investigation (extensively reviewed elsewhere) and major improvements have been achieved (Li and Petrovsky, 2016; Suschak et al., 2017), but this is not the real reason. In fact, the most important reason is the prokaryotic backbone of the plasmid which are advantaged in plasmid production steps only. Following DNA entry into the eukaryotic host cell, only the eukaryotic regions including promoter, enhancer sequences, and polyadenylation sequences are used, and the prokaryotic region has no use at all. Instead, these prokaryotic sequences can cause serious problems after transfection which are discussed as follows (Li and Petrovsky, 2016; Eusebio et al., 2021):
First, the presence of an antibiotic resistance gene can spread antibiotic resistance to other bacteria in the vaccinated person through horizontal transfer. Many plasmid structures use aminoglycoside resistance genes such as kanamycin and neomycin which have extensive clinical uses. Therefore, there is a risk of developing resistance to these antibiotics. Some other structures use beta-lactam resistance genes, such as ampicillin. Antibiotics remnants after the in vitro purification process, can lead to a severe anaphylactic reaction in susceptible individuals (Williams, 2013). Second, antibiotic resistance in large-scale culture of parasites reduces the plasmid production yield resulting from metabolic pressure on bacterial cells, because the antibiotic in the culture medium induces constitutive expression of the resistance gene which slows down the growth rate of bacteria (Rozkov et al., 2004; Mairhofer et al., 2010). Third, the prokaryotic backbone can interfere with the expression of the gene while inside the eukaryotic cells. After entering the eukaryotic cell, the prokaryotic region becomes predominantly heterochromatin, which can spread to the target gene region. In addition, prokaryotic regions may have cryptic promoters that produce small RNA sequences in eukaryotic cells and block the expression of encoded gene’s mRNA (Lu et al., 2012; Gracey Maniar et al., 2013). Finally, first-generation plasmids used for vaccines or immunotherapy, such as pcDNA, are relatively large in size, and much of this large structure belongs to prokaryotic regions that interfere with the efficiency of transfection. Comparison has evidenced the relation between the smaller DNA structure and the higher transfection efficiency. Although methods such as electroporation, gene guns, or liposomes and lipid nanoparticles help increase transfer from the membrane into the cell, the large size of the plasmid slows down the transfer from the cytoplasm to the nucleus (Lukacs et al., 2000; Yin et al., 2005; Rosazza et al., 2011).
New generation of plasmids devoid of antibiotic resistance marker with shorter prokaryotic backbones are now available to overcome these drawbacks (Vandermeulen et al., 2011). They are either based on complete elimination of the prokaryotic sequences to generate small-sized minicircles (which are not further plasmids) or selection systems other than antibiotic resistance. The latter include complementation of auxotrophic strains by suppressive tRNAs (Marie et al., 2008, 2010; Bakker et al., 2019), toxin–antitoxin systems (Bukowski et al., 2011), operator–repressor titration (Cranenburgh et al., 2001; Mwau et al., 2004; Ramos et al., 2009), RNA markers including RNA out (Luke et al., 2009) and RNA I (Pfaffenzeller et al., 2006) systems, overexpression of a growth essential gene (Goh and Good, 2008) and enzyme-inhibitor ratios (Alcolea et al., 2019). These are altogether known as marker-free plasmids.
Minicircles were designed in 1997. Using homologous recombinant technology, the prokaryotic parts were removed from the parental plasmid after the completion of the in vitro amplification step (Darquet et al., 1997). The use of these structures with a prokaryotic region below 100 base pairs, manipulated either before inoculation or within the cells after transfection, has generated promising results in vaccine studies of cancer (Pang et al., 2017), Hepatitis B virus (Li et al., 2016), HIV (Wang et al., 2014), Newcastle Disease (Jiang et al., 2019) and Listeria monocytogenes (Dietz et al., 2013). In all cases, an elevated protection level following long-term expression of the antigen has been observed when compared to the first-generation plasmids (Munye et al., 2016). However, the purification of these small-sized constructs is much more labor intensive and complicated than the conventional plasmids. Although newer systems suggest the removal of the prokaryotic part in vivo (Jiang et al., 2019), this can leave a mixture of the parental plasmid and minicircles within the same cell.
Besides Minicircles, marker-free plasmids are also under investigation as vaccine or non-viral gene therapy tools. Among the many different antibiotic-free selection systems, RNA-based methods are currently more attractive. Recently, in a study published by Suschak, the effect of DNA vaccine against Ebola and against Venezuelan Equine Encephalitis Virus (VEEV) was compared using pWRG7077 plasmids and an RNA-OUT system along with immunostimulatory sequences (CpG and Immunostimulatory RNA) and it was determined that an RNA-OUT system as in the case of VEEV, the vaccine had a similar protective effect and in the case of the Ebola vaccine, it had a better effect than the pWRG7077 plasmid (Suschak et al., 2020).
Other selection systems are also under investigation with promising results. Alcolea et al. have examined the Th1 response induction levels against canine leishmaniasis using pPAL-LACK vector (an enzyme-inhibitor system). The protection was comparable to recombinant vaccinia virus in combination with standard mammalian expression plasmid vectors. The pPAL vector (3,899 bp in length) contains the cytomegalovirus enhancer and promoter for expression in mammalian cells and the E. coli fabI chromosomal gene as a selectable marker. The pPAL plasmid contains the essential elements for manipulation and expression of any cloned DNA sequence in prokaryotic and mammalian cells using an E. coli endogenous gene as a selectable marker, which also provides a long CpG island. This antibiotic resistance-free plasmid is a vaccine vector actively participating in protection against canine leishmaniasis, and may be potentially tested as a vaccine vector with other antigens against different pathogens (Alcolea et al., 2019).
Leishmaniasis is a vector-borne parasitic infection with an unresolved complex host-pathogen interaction. That is why a protective vaccine is missing for human use despite labor intensive hard work to reach the point. However, vaccination is still a hope and not hype referring to the field observations like asymptomatic infection in endemic areas. Apparently, the more we understand host pathogen interaction, the closer we get to a protective vaccine.
Knowing that the efficacy of the Th1 memory protection is abrogated following sand fly transmission of parasite, the vaccine options are more restricted particularly in non-endemic areas. Live attenuated, live nonpathogenic and DNA vaccines seem the best choices as long as they persist in the body and continue producing the pertinent antigens. Therefore, we can think of these choices in endemic areas where the immunization occurs within a few months before sand fly biting season. For sure, intradermal immunization and intradermal low dose sand fly challenge are of paramount importance respecting the TEFF and TRM cells for effective vaccine design. Hopefully, novel innovative approaches such as CRISPR/Cas9 technology and antibiotic-free plasmids will improve intrinsic drawbacks associated with live attenuated parasites or DNA vaccines.
Alternatively, raising neutralizing antibodies against sand fly derived factors could be applicable both for endemic and non-endemic areas. Of note some sand fly derived proteins are identified to recruit neutrophils to the bite site. Therefore, neutralization of these proteins by vaccination and antibody generation could be a good choice to restrict sand fly saliva mediated neutrophil recruitment. Albeit, the pre-requisites for the long-lived plasma cell and memory B cell generation remain to be fully addressed and are still under intensive investigation.
NS: Conceptualization, Supervision, Writing – original draft. TT: Writing – review & editing. SR: Writing – review & editing.
The author(s) declare that no financial support was received for the research, authorship, and/or publication of this article.
The authors which to sincerely acknowledge European Union’s H2020 LeiSHield MATI RISE (Research and Innovation Staff Exchange), under the Marie Skłodowska-Curie grant agreement No 778298 for supporting this work.
The authors declare that the research was conducted in the absence of any commercial or financial relationships that could be construed as a potential conflict of interest.
All claims expressed in this article are solely those of the authors and do not necessarily represent those of their affiliated organizations, or those of the publisher, the editors and the reviewers. Any product that may be evaluated in this article, or claim that may be made by its manufacturer, is not guaranteed or endorsed by the publisher.
Adaui, V., Krober-Boncardo, C., Brinker, C., Zirpel, H., Sellau, J., Arevalo, J., et al. (2020). Application of CRISPR/Cas9-based reverse genetics in Leishmania braziliensis: conserved roles for HSP100 and HSP23. Genes (Basel) 11:1159. doi: 10.3390/genes11101159
Alcolea, P. J., Alonso, A., and Larraga, V. (2019). The antibiotic resistance-free mammalian expression plasmid vector pPAL for development of third generation vaccines. Plasmid 101, 35–42. doi: 10.1016/j.plasmid.2018.12.002
Azizi, H., Hassani, K., Taslimi, Y., Najafabadi, H. S., Papadopoulou, B., and Rafati, S. (2009). Searching for virulence factors in the non-pathogenic parasite to humans Leishmania tarentolae. Parasitology 136, 723–735. doi: 10.1017/S0031182009005873
Bakker, N. A. M., de Boer, R., Marie, C., Scherman, D., Haanen, J., Beijnen, J. H., et al. (2019). Small-scale GMP production of plasmid DNA using a simplified and fully disposable production method. J. Biotechnol. 306:100007. doi: 10.1016/j.btecx.2019.100007
Bandi, C., Mendoza-Roldan, J. A., Otranto, D., Alvaro, A., Louzada-Flores, V. N., Pajoro, M., et al. (2023). Leishmania tarentolae: a vaccine platform to target dendritic cells and a surrogate pathogen for next generation vaccine research in leishmaniases and viral infections. Parasit. Vectors 16:35. doi: 10.1186/s13071-023-05651-1
Belkaid, Y., Hoffmann, K. F., Mendez, S., Kamhawi, S., Udey, M. C., Wynn, T. A., et al. (2001). The role of interleukin (IL)-10 in the persistence of Leishmania major in the skin after healing and the therapeutic potential of anti-IL-10 receptor antibody for sterile cure. J. Exp. Med. 194, 1497–1506. doi: 10.1084/jem.194.10.1497
Bhattacharya, P., Dey, R., Dagur, P. K., Joshi, A. B., Ismail, N., Gannavaram, S., et al. (2016). Live attenuated Leishmania donovani Centrin Knock out parasites generate non-inferior protective immune response in aged mice against visceral Leishmaniasis. PLoS Negl. Trop. Dis. 10:e0004963. doi: 10.1371/journal.pntd.0004963
Breton, M., Tremblay, M. J., Ouellette, M., and Papadopoulou, B. (2005). Live nonpathogenic parasitic vector as a candidate vaccine against visceral leishmaniasis. Infect. Immun. 73, 6372–6382. doi: 10.1128/IAI.73.10.6372-6382.2005
Bukowski, M., Rojowska, A., and Wladyka, B. (2011). Prokaryotic toxin-antitoxin systems--the role in bacterial physiology and application in molecular biology. Acta Biochim. Pol. 58, 1–9. doi: 10.18388/abp.2011_2278
Carlsen, E. D., Jie, Z., Liang, Y., Henard, C. A., Hay, C., Sun, J., et al. (2015). Interactions between neutrophils and Leishmania braziliensis amastigotes facilitate cell activation and parasite clearance. J. Innate Immun. 7, 354–363. doi: 10.1159/000373923
Chaves, M. M., Lee, S. H., Kamenyeva, O., Ghosh, K., Peters, N. C., and Sacks, D. (2020). The role of dermis resident macrophages and their interaction with neutrophils in the early establishment of Leishmania major infection transmitted by sand fly bite. PLoS Pathog. 16:e1008674. doi: 10.1371/journal.ppat.1008674
Costa, C. H., Peters, N. C., Maruyama, S. R., De Brito, E. C. Jr., and Santos, I. K. (2011). Vaccines for the leishmaniases: proposals for a research agenda. PLoS Negl. Trop. Dis. 5:e943. doi: 10.1371/journal.pntd.0000943
Cranenburgh, R. M., Hanak, J. A., Williams, S. G., and Sherratt, D. J. (2001). Escherichia coli strains that allow antibiotic-free plasmid selection and maintenance by repressor titration. Nucleic Acids Res. 29, 26e–226e. doi: 10.1093/nar/29.5.e26
Damerow, S., Hoppe, C., Bandini, G., Zarnovican, P., Buettner, F. F., Luder, C. G., et al. (2015). Depletion of UDP-glucose and UDP-galactose using a Degron system leads to growth cessation of Leishmania major. PLoS Negl. Trop. Dis. 9:e0004205. doi: 10.1371/journal.pntd.0004205
Darquet, A. M., Cameron, B., Wils, P., Scherman, D., and Crouzet, J. (1997). A new DNA vehicle for nonviral gene delivery: supercoiled minicircle. Gene Ther. 4, 1341–1349. doi: 10.1038/sj.gt.3300540
Davarpanah, E., Seyed, N., Bahrami, F., Rafati, S., Safaralizadeh, R., and Taheri, T. (2020). Lactococcus lactis expressing sand fly PpSP15 salivary protein confers long-term protection against Leishmania major in BALB/c mice. PLoS Negl. Trop. Dis. 14:e0007939. doi: 10.1371/journal.pntd.0007939
de Paiva, R. M., Grazielle-Silva, V., Cardoso, M. S., Nakagaki, B. N., Mendonca-Neto, R. P., Canavaci, A. M., et al. (2015). Amastin knockdown in Leishmania braziliensis affects parasite-macrophage interaction and results in impaired viability of intracellular amastigotes. PLoS Pathog. 11:e1005296. doi: 10.1371/journal.ppat.1005296
de Souza Carmo, E. V., Katz, S., and Barbieri, C. L. (2010). Neutrophils reduce the parasite burden in Leishmania (Leishmania) amazonensis-infected macrophages. PLoS One 5:e13815. doi: 10.1371/journal.pone.0013815
Dean, S., Sunter, J., Wheeler, R. J., Hodkinson, I., Gluenz, E., and Gull, K. (2015). A toolkit enabling efficient, scalable and reproducible gene tagging in trypanosomatids. Open Biol. 5:140197. doi: 10.1098/rsob.140197
Dey, R., Joshi, A. B., Oliveira, F., Pereira, L., Guimaraes-Costa, A. B., Serafim, T. D., et al. (2018). Gut microbes egested during bites of infected sand flies augment severity of Leishmaniasis via Inflammasome-derived IL-1beta. Cell Host Microbe 23, 134–143.e6. doi: 10.1016/j.chom.2017.12.002
Dietz, W. M., Skinner, N. E., Hamilton, S. E., Jund, M. D., Heitfeld, S. M., Litterman, A. J., et al. (2013). Minicircle DNA is superior to plasmid DNA in eliciting antigen-specific CD8+ T-cell responses. Mol. Ther. 21, 1526–1535. doi: 10.1038/mt.2013.85
Dinc, R. (2022). Leishmania vaccines: the current situation with its promising aspect for the future. Korean J. Parasitol. 60, 379–391. doi: 10.3347/kjp.2022.60.6.379
Dong, G., Filho, A. L., and Olivier, M. (2019). Modulation of host-pathogen communication by extracellular vesicles (EVs) of the protozoan parasite Leishmania. Front. Cell. Infect. Microbiol. 9:100. doi: 10.3389/fcimb.2019.00100
Dong, G., Wagner, V., Minguez-Menendez, A., Fernandez-Prada, C., and Olivier, M. (2021). Extracellular vesicles and leishmaniasis: current knowledge and promising avenues for future development. Mol. Immunol. 135, 73–83. doi: 10.1016/j.molimm.2021.04.003
Duncan, S. M., Jones, N. G., and Mottram, J. C. (2017). Recent advances in Leishmania reverse genetics: manipulating a manipulative parasite. Mol. Biochem. Parasitol. 216, 30–38. doi: 10.1016/j.molbiopara.2017.06.005
Eusebio, D., Neves, A. R., Costa, D., Biswas, S., Alves, G., Cui, Z., et al. (2021). Methods to improve the immunogenicity of plasmid DNA vaccines. Drug Discov. Today 26, 2575–2592. doi: 10.1016/j.drudis.2021.06.008
Gary, E. N., and Weiner, D. B. (2020). DNA vaccines: prime time is now. Curr. Opin. Immunol. 65, 21–27. doi: 10.1016/j.coi.2020.01.006
Glennie, N. D., and Scott, P. (2016). Memory T cells in cutaneous leishmaniasis. Cell. Immunol. 309, 50–54. doi: 10.1016/j.cellimm.2016.07.010
Glennie, N. D., Yeramilli, V. A., Beiting, D. P., Volk, S. W., Weaver, C. T., and Scott, P. (2015). Skin-resident memory CD4+ T cells enhance protection against Leishmania major infection. J. Exp. Med. 212, 1405–1414. doi: 10.1084/jem.20142101
Goh, S., and Good, L. (2008). Plasmid selection in Escherichia coli using an endogenous essential gene marker. BMC Biotechnol. 8:61. doi: 10.1186/1472-6750-8-61
Gomes, R., Teixeira, C., Oliveira, F., Lawyer, P. G., Elnaiem, D. E., Meneses, C., et al. (2012). KSAC, a defined Leishmania antigen, plus adjuvant protects against the virulence of L. major transmitted by its natural vector Phlebotomus duboscqi. PLoS Negl. Trop. Dis. 6:e1610. doi: 10.1371/journal.pntd.0001610
Gracey Maniar, L. E., Maniar, J. M., Chen, Z. Y., Lu, J., Fire, A. Z., and Kay, M. A. (2013). Minicircle DNA vectors achieve sustained expression reflected by active chromatin and transcriptional level. Mol. Ther. 21, 131–138. doi: 10.1038/mt.2012.244
Grybchuk, D., Macedo, D. H., Kleschenko, Y., Kraeva, N., Lukashev, A. N., Bates, P. A., et al. (2020). The first non-LRV RNA virus in Leishmania. Viruses 12:168. doi: 10.3390/v12020168
Guimaraes-Costa, A. B., Shannon, J. P., Waclawiak, I., Oliveira, J., Meneses, C., de Castro, W., et al. (2021). A sand fly salivary protein acts as a neutrophil chemoattractant. Nat. Commun. 12:3213. doi: 10.1038/s41467-021-23002-5
Gurunathan, S., Sacks, D. L., Brown, D. R., Reiner, S. L., Charest, H., Glaichenhaus, N., et al. (1997). Vaccination with DNA encoding the immunodominant LACK parasite antigen confers protective immunity to mice infected with Leishmania major. J. Exp. Med. 186, 1137–1147. doi: 10.1084/jem.186.7.1137
Gurunathan, S., Wu, C. Y., Freidag, B. L., and Seder, R. A. (2000). DNA vaccines: a key for inducing long-term cellular immunity. Curr. Opin. Immunol. 12, 442–447. doi: 10.1016/S0952-7915(00)00118-7
Haghdoust, S., Noroozbeygi, M., Hajimollahoseini, M., Masooleh, M. M., and Yeganeh, F. (2022). A candidate vaccine composed of live nonpathogenic Iranian lizard Leishmania mixed with chitin microparticles protects mice against Leishmania major infection. Acta Trop. 227:106298. doi: 10.1016/j.actatropica.2021.106298
Hartley, M.-A., Ronet, C., Zangger, H., Beverley, S. M., and Fasel, N. (2012). Leishmania RNA virus: when the host pays the toll. Front. Cell. Infect. Microbiol. 2:99. doi: 10.3389/fcimb.2012.00099
Ho, W., Gao, M., Li, F., Li, Z., Zhang, X. Q., and Xu, X. (2021). Next-generation vaccines: nanoparticle-mediated DNA and mRNA delivery. Adv. Healthc. Mater. 10:e2001812. doi: 10.1002/adhm.202001812
Hohman, L. S., Carneiro, M. B., Kratofil, R. M., and Peters, N. C. (2021). Effector function prior to establishment of the phagosomal pathogen niche is required for protective CD4+ T cell-mediated immunity against Leishmania. J. Immunol. 206:16.29. doi: 10.4049/jimmunol.206.Supp.16.29
Hohman, L. S., Mou, Z., Carneiro, M. B., Ferland, G., Kratofil, R. M., Kubes, P., et al. (2021). Protective CD4+ Th1 cell-mediated immunity is reliant upon execution of effector function prior to the establishment of the pathogen niche. PLoS Pathog. 17:e1009944. doi: 10.1371/journal.ppat.1009944
Hohman, L. S., and Peters, N. C. (2019). CD4(+) T cell-mediated immunity against the Phagosomal pathogen Leishmania: implications for vaccination. Trends Parasitol. 35, 423–435. doi: 10.1016/j.pt.2019.04.002
Ishemgulova, A., Kraeva, N., Faktorová, D., Podesvova, L., Lukes, J., and Yurchenko, V. (2016). T7 polymerase-driven transcription is downregulated in metacyclic promastigotes and amastigotes of Leishmania mexicana. Folia Parasitol. 63:1. doi: 10.14411/fp.2016.016
Ismail, N., Karmakar, S., Bhattacharya, P., Sepahpour, T., Takeda, K., Hamano, S., et al. (2022). Leishmania major Centrin gene-deleted parasites generate skin resident memory T-cell immune response analogous to Leishmanization. Front. Immunol. 13:864031. doi: 10.3389/fimmu.2022.864031
Jiang, Y., Gao, X., Xu, K., Wang, J., Huang, H., Shi, C., et al. (2019). A novel Cre recombinase-mediated in vivo Minicircle DNA (CRIM) vaccine provides partial protection against Newcastle disease virus. Appl. Environ. Microbiol. 85:e00407-19. doi: 10.1128/AEM.00407-19
Kamhavi, S., Valenzuela, J. G., Coutinho-Abreu, I. V., and Brodskyn, C. I. Role of sand fly saliva on Leishmania-infection and the potential of vector salivary proteins as vaccines. Skin and arthropod vectors. Academic Press, London. (2018), 96–119.
Kamhawi, S. (2000). The biological and immunomodulatory properties of sand fly saliva and its role in the establishment of Leishmania infections. Microbes Infect. 2, 1765–1773. doi: 10.1016/S1286-4579(00)01331-9
Karmakar, S., Volpedo, G., Zhang, W. W., Lypaczewski, P., Ismail, N., Oliveira, F., et al. (2022). Centrin-deficient Leishmania mexicana confers protection against Old World visceral leishmaniasis. NPJ Vaccines. 7:157. doi: 10.1038/s41541-022-00574-x
Katebi, A., Gholami, E., Taheri, T., Zahedifard, F., Habibzadeh, S., Taslimi, Y., et al. (2015). Leishmania tarentolae secreting the sand fly salivary antigen PpSP15 confers protection against Leishmania major infection in a susceptible BALB/c mice model. Mol. Immunol. 67, 501–511. doi: 10.1016/j.molimm.2015.08.001
Kaye, P. M., Mohan, S., Mantel, C., Malhame, M., Revill, P., Le Rutte, E., et al. (2021). Overcoming roadblocks in the development of vaccines for leishmaniasis. Expert Rev. Vaccines 20, 1419–1430. doi: 10.1080/14760584.2021.1990043
Keshavarzian, N., Noroozbeygi, M., Haji Molla Hoseini, M., and Yeganeh, F. (2020). Evaluation of Leishmanization using Iranian lizard Leishmania mixed with CpG-ODN as a candidate vaccine against experimental murine Leishmaniasis. Front. Immunol. 11:1725. doi: 10.3389/fimmu.2020.01725
Kutzler, M. A., and Weiner, D. B. (2008). DNA vaccines: ready for prime time? Nat. Rev. Genet. 9, 776–788. doi: 10.1038/nrg2432
Lajevardi, M. S., Gholami, E., Taheri, T., Sarvnaz, H., Habibzadeh, S., Seyed, N., et al. (2022). Leishmania tarentolae as potential live vaccine co-expressing distinct salivary gland proteins against experimental cutaneous leishmaniasis in BALB/c mice model. Front. Immunol. 13:895234. doi: 10.3389/fimmu.2022.895234
Lee, J., Kumar, S. A., Jhan, Y. Y., and Bishop, C. J. (2018). Engineering DNA vaccines against infectious diseases. Acta Biomater. 80, 31–47. doi: 10.1016/j.actbio.2018.08.033
Lestinova, T., Rohousova, I., Sima, M., de Oliveira, C. I., and Volf, P. (2017). Insights into the sand fly saliva: blood-feeding and immune interactions between sand flies, hosts, and Leishmania. PLoS Negl. Trop. Dis. 11:e0005600. doi: 10.1371/journal.pntd.0005600
Li, F., Cheng, L., Murphy, C. M., Reszka-Blanco, N. J., Wu, Y., Chi, L., et al. (2016). Minicircle HBV cccDNA with a Gaussia luciferase reporter for investigating HBV cccDNA biology and developing cccDNA-targeting drugs. Sci. Rep. 6:36483. doi: 10.1038/srep36483
Li, L., and Petrovsky, N. (2016). Molecular mechanisms for enhanced DNA vaccine immunogenicity. Expert Rev. Vaccines 15, 313–329. doi: 10.1586/14760584.2016.1124762
Lim, M., Badruddoza, A. Z. M., Firdous, J., Azad, M., Mannan, A., Al-Hilal, T. A., et al. (2020). Engineered Nanodelivery systems to improve DNA vaccine technologies. Pharmaceutics 12:30. doi: 10.3390/pharmaceutics12010030
Liu, M. A. (2011). DNA vaccines: an historical perspective and view to the future. Immunol. Rev. 239, 62–84. doi: 10.1111/j.1600-065X.2010.00980.x
Lopes, A., Vandermeulen, G., and Preat, V. (2019). Cancer DNA vaccines: current preclinical and clinical developments and future perspectives. J. Exp. Clin. Cancer Res. 38:146. doi: 10.1186/s13046-019-1154-7
Louis, L., Clark, M., Wise, M. C., Glennie, N., Wong, A., Broderick, K., et al. (2019). Intradermal synthetic DNA vaccination generates Leishmania-specific T cells in the skin and protection against Leishmania major. Infect. Immun. 87:e00227-19. doi: 10.1128/IAI.00227-19
Lu, J., Zhang, F., Xu, S., Fire, A. Z., and Kay, M. A. (2012). The extragenic spacer length between the 5′ and 3′ ends of the transgene expression cassette affects transgene silencing from plasmid-based vectors. Mol. Ther. 20, 2111–2119. doi: 10.1038/mt.2012.65
Lukacs, G. L., Haggie, P., Seksek, O., Lechardeur, D., Freedman, N., and Verkman, A. S. (2000). Size-dependent DNA mobility in cytoplasm and nucleus. J. Biol. Chem. 275, 1625–1629. doi: 10.1074/jbc.275.3.1625
Luke, J., Carnes, A. E., Hodgson, C. P., and Williams, J. A. (2009). Improved antibiotic-free DNA vaccine vectors utilizing a novel RNA based plasmid selection system. Vaccine 27, 6454–6459. doi: 10.1016/j.vaccine.2009.06.017
Madeira da Silva, L., and Beverley, S. M. (2010). Expansion of the target of rapamycin (TOR) kinase family and function in Leishmania shows that TOR3 is required for acidocalcisome biogenesis and animal infectivity. Proc. Natl. Acad. Sci. USA 107, 11965–11970. doi: 10.1073/pnas.1004599107
Mairhofer, J., Cserjan-Puschmann, M., Striedner, G., Nobauer, K., Razzazi-Fazeli, E., and Grabherr, R. (2010). Marker-free plasmids for gene therapeutic applications--lack of antibiotic resistance gene substantially improves the manufacturing process. J. Biotechnol. 146, 130–137. doi: 10.1016/j.jbiotec.2010.01.025
Marie, C., Richard, M., Vandermeulen, G., Quiviger, M., Préat, V., and Scherman, D. (2008). pFAR plasmids: new eukaryotic expression vectors for gene therapy, devoid of antibiotic resistance markers. Nat. Preced. :1. doi: 10.1038/npre.2008.2395.1
Marie, C., Vandermeulen, G., Quiviger, M., Richard, M., Preat, V., and Scherman, D. (2010). pFARs, plasmids free of antibiotic resistance markers, display high-level transgene expression in muscle, skin and tumour cells. J. Gene Med. 12, 323–332. doi: 10.1002/jgm.1441
Masoudzadeh, N., Mizbani, A., and Rafati, S. (2020a). Transcriptomic profiling in cutaneous Leishmaniasis patients. Expert Rev. Proteomics 17, 533–541. doi: 10.1080/14789450.2020.1812390
Masoudzadeh, N., Ostensson, M., Persson, J., Mashayekhi Goyonlo, V., Agbajogu, C., Taslimi, Y., et al. (2020b). Molecular signatures of anthroponotic cutaneous leishmaniasis in the lesions of patients infected with Leishmania tropica. Sci. Rep. 10:16198. doi: 10.1038/s41598-020-72671-7
McCall, L. I., El Aroussi, A., Choi, J. Y., Vieira, D. F., De Muylder, G., Johnston, J. B., et al. (2015). Targeting Ergosterol biosynthesis in Leishmania donovani: essentiality of sterol 14 alpha-demethylase. PLoS Negl. Trop. Dis. 9:e0003588. doi: 10.1371/journal.pntd.0003588
McCluskie, M. J., Weeratna, R. D., and Davis, H. L. (2000). The role of CpG in DNA vaccines. Springer Semin. Immunopathol. 22, 125–132. doi: 10.1007/s002810000014
Mendez, S., Gurunathan, S., Kamhawi, S., Belkaid, Y., Moga, M. A., Skeiky, Y. A., et al. (2001). The potency and durability of DNA-and protein-based vaccines against Leishmania major evaluated using low-dose, intradermal challenge. J. Immunol. 166, 5122–5128. doi: 10.4049/jimmunol.166.8.5122
Mendoza-Roldan, J. A., Latrofa, M. S., Iatta, R., Manoj R, R. S., Panarese, R., Annoscia, G., et al. (2021). Detection of Leishmania tarentolae in lizards, sand flies and dogs in southern Italy, where Leishmania infantum is endemic: hindrances and opportunities. Parasit. Vectors 14, 1–12. doi: 10.1186/s13071-021-04973-2
Mendoza-Roldan, J. A., Votypka, J., Bandi, C., Epis, S., Modry, D., Ticha, L., et al. (2022). Leishmania tarentolae: a new frontier in the epidemiology and control of the leishmaniases. Transbound. Emerg. Dis. 69, e1326–e1337. doi: 10.1111/tbed.14660
Moreira, P. O. L., Nogueira, P. M., and Monte-Neto, R. L. (2023). Next-generation Leishmanization: revisiting molecular targets for selecting genetically engineered live-attenuated Leishmania. Microorganisms. 11:1043. doi: 10.3390/microorganisms11041043
Munye, M. M., Tagalakis, A. D., Barnes, J. L., Brown, R. E., McAnulty, R. J., Howe, S. J., et al. (2016). Minicircle DNA provides enhanced and prolonged transgene expression following airway gene transfer. Sci. Rep. 6:23125. doi: 10.1038/srep23125
Mwau, M., Cebere, I., Sutton, J., Chikoti, P., Winstone, N., Wee, E. G., et al. (2004). A human immunodeficiency virus 1 (HIV-1) clade a vaccine in clinical trials: stimulation of HIV-specific T-cell responses by DNA and recombinant modified vaccinia virus Ankara (MVA) vaccines in humans. J. Gen. Virol. 85, 911–919. doi: 10.1099/vir.0.19701-0
Noroozbeygi, M., Keshavarzian, N., Haji Molla Hoseini, M., Haghdoust, S., and Yeganeh, F. (2023). Comparison of the long-term and short-term protection in mouse model of Leishmania major infection following vaccination with live Iranian lizard Leishmania mixed with chitin microparticles. Parasite Immunol. 46:e13018. doi: 10.1111/pim.13018
Omondi, Z. N., and Demir, S. (2021). Bacteria composition and diversity in the gut of sand fly: impact on Leishmania and sand fly development. Int. J. Trop. Insect. Sci. 41, 25–32. doi: 10.1007/s42690-020-00184-x
Pacheco-Fernandez, T., Volpedo, G., Gannavaram, S., Bhattacharya, P., Dey, R., Satoskar, A., et al. (2021). Revival of Leishmanization and Leishmanin. Front. Cell. Infect. Microbiol. 11:639801. doi: 10.3389/fcimb.2021.639801
Pandey, S. C., Kumar, A., and Samant, M. (2020). Genetically modified live attenuated vaccine: a potential strategy to combat visceral leishmaniasis. Parasite Immunol. 42:e12732. doi: 10.1111/pim.12732
Pang, X., Ma, F., Zhang, P., Zhong, Y., Zhang, J., Wang, T., et al. (2017). Treatment of human B-cell lymphomas using Minicircle DNA vector expressing anti-CD3/CD20 in a mouse model. Hum. Gene Ther. 28, 216–225. doi: 10.1089/hum.2016.122
Passelli, K., Billion, O., and Tacchini-Cottier, F. (2021). The impact of neutrophil recruitment to the skin on the pathology induced by Leishmania infection. Front. Immunol. 12:649348. doi: 10.3389/fimmu.2021.649348
Peters, N. C., Bertholet, S., Lawyer, P. G., Charmoy, M., Romano, A., Ribeiro-Gomes, F. L., et al. (2012). Evaluation of recombinant Leishmania polyprotein plus glucopyranosyl lipid a stable emulsion vaccines against sand fly-transmitted Leishmania major in C57BL/6 mice. J. Immunol. 189, 4832–4841. doi: 10.4049/jimmunol.1201676
Peters, N. C., Egen, J. G., Secundino, N., Debrabant, A., Kimblin, N., Kamhawi, S., et al. (2008). In vivo imaging reveals an essential role for neutrophils in leishmaniasis transmitted by sand flies. Science 321, 970–974. doi: 10.1126/science.1159194
Peters, N. C., Kimblin, N., Secundino, N., Kamhawi, S., Lawyer, P., and Sacks, D. L. (2009). Vector transmission of leishmania abrogates vaccine-induced protective immunity. PLoS Pathog. 5:e1000484. doi: 10.1371/journal.ppat.1000484
Peters, N. C., Pagan, A. J., Lawyer, P. G., Hand, T. W., Henrique Roma, E., Stamper, L. W., et al. (2014). Chronic parasitic infection maintains high frequencies of short-lived Ly6C+CD4+ effector T cells that are required for protection against re-infection. PLoS Pathog. 10:e1004538. doi: 10.1371/journal.ppat.1004538
Peters, N. C., and Sacks, D. L. (2009). The impact of vector-mediated neutrophil recruitment on cutaneous leishmaniasis. Cell. Microbiol. 11, 1290–1296. doi: 10.1111/j.1462-5822.2009.01348.x
Pfaffenzeller, I., Mairhofer, J., Striedner, G., Bayer, K., and Grabherr, R. (2006). Using ColE1-derived RNA I for suppression of a bacterially encoded gene: implication for a novel plasmid addiction system. Biotechnol. J. 1, 675–681. doi: 10.1002/biot.200600017
Plotkin, S. (2014). History of vaccination. Proc. Natl. Acad. Sci. USA 111, 12283–12287. doi: 10.1073/pnas.1400472111
Ramos, I., Alonso, A., Peris, A., Marcen, J. M., Abengozar, M. A., Alcolea, P. J., et al. (2009). Antibiotic resistance free plasmid DNA expressing LACK protein leads towards a protective Th1 response against Leishmania infantum infection. Vaccine 27, 6695–6703. doi: 10.1016/j.vaccine.2009.08.091
Raymond, F., Boisvert, S., Roy, G., Ritt, J. F., Legare, D., Isnard, A., et al. (2012). Genome sequencing of the lizard parasite Leishmania tarentolae reveals loss of genes associated to the intracellular stage of human pathogenic species. Nucleic Acids Res. 40, 1131–1147. doi: 10.1093/nar/gkr834
Ronet, C., Passelli, K., Charmoy, M., Scarpellino, L., Myburgh, E., Hauyon La Torre, Y., et al. (2019). TLR2 signaling in skin nonhematopoietic cells induces early neutrophil recruitment in response to Leishmania major infection. J. Invest. Dermatol. 139, 1318–1328. doi: 10.1016/j.jid.2018.12.012
Rosazza, C., Escoffre, J.-M., Zumbusch, A., and Rols, M.-P. (2011). The actin cytoskeleton has an active role in the electrotransfer of plasmid DNA in mammalian cells. Mol. Ther. 19, 913–921. doi: 10.1038/mt.2010.303
Rossi, M., and Fasel, N. (2018). The criminal association of Leishmania parasites and viruses. Curr. Opin. Microbiol. 46, 65–72. doi: 10.1016/j.mib.2018.07.005
Rozkov, A., Avignone-Rossa, C. A., Ertl, P. F., Jones, P., O'Kennedy, R. D., Smith, J. J., et al. (2004). Characterization of the metabolic burden on Escherichia coli DH1 cells imposed by the presence of a plasmid containing a gene therapy sequence. Biotechnol. Bioeng. 88, 909–915. doi: 10.1002/bit.20327
Sacks, D. L. (2014). Vaccines against tropical parasitic diseases: a persisting answer to a persisting problem. Nat. Immunol. 15, 403–405. doi: 10.1038/ni.2853
Sainz, M., and de la Maza, O. (2014). Leishmaniasis transmission biology: Role of promastigote secretory gel as a transmission determinant London School of Hygiene & Tropical Medicine.
Salisbury, J. L. (2004). Centrosomes: Sfi1p and centrin unravel a structural riddle. Curr. Biol. 14, R27–R29. doi: 10.1016/j.cub.2003.12.019
Saljoughian, N., Taheri, T., Zahedifard, F., Taslimi, Y., Doustdari, F., Bolhassani, A., et al. (2013). Development of novel prime-boost strategies based on a tri-gene fusion recombinant L. tarentolae vaccine against experimental murine visceral leishmaniasis. PLoS Negl. Trop. Dis. 7:e2174. doi: 10.1371/journal.pntd.0002174
Sangare, L., Manhart, L., Zehrung, D., and Wang, C. C. (2009). Intradermal hepatitis B vaccination: a systematic review and meta-analysis. Vaccine 27, 1777–1786. doi: 10.1016/j.vaccine.2009.01.043
Santos, R., Silva, G. L. A., Santos, E. V., Duncan, S. M., Mottram, J. C., Damasceno, J. D., et al. (2017). A DiCre recombinase-based system for inducible expression in Leishmania major. Mol. Biochem. Parasitol. 216, 45–48. doi: 10.1016/j.molbiopara.2017.06.006
Schindler, S. E., McCall, J. G., Yan, P., Hyrc, K. L., Li, M., Tucker, C. L., et al. (2015). Photo-activatable Cre recombinase regulates gene expression in vivo. Sci. Rep. 5:13627. doi: 10.1038/srep13627
Scott, P. (2020). Long-lived skin-resident memory T cells contribute to concomitant immunity in cutaneous Leishmaniasis. Cold Spring Harb. Perspect. Biol. 12:a038059. doi: 10.1101/cshperspect.a038059
Selvapandiyan, A., Debrabant, A., Duncan, R., Muller, J., Salotra, P., Sreenivas, G., et al. (2004). Centrin gene disruption impairs stage-specific basal body duplication and cell cycle progression in Leishmania. J. Biol. Chem. 279, 25703–25710. doi: 10.1074/jbc.M402794200
Selvapandiyan, A., Duncan, R., Debrabant, A., Bertholet, S., Sreenivas, G., Negi, N. S., et al. (2001). Expression of a mutant form of Leishmania donovani centrin reduces the growth of the parasite. J. Biol. Chem. 276, 43253–43261. doi: 10.1074/jbc.M106806200
Serafim, T. D., Coutinho-Abreu, I. V., Dey, R., Kissinger, R., Valenzuela, J. G., Oliveira, F., et al. (2021). Leishmaniasis: the act of transmission. Trends Parasitol. 37, 976–987. doi: 10.1016/j.pt.2021.07.003
Seyed, N., Peters, N. C., and Rafati, S. (2018). Translating observations from Leishmanization into non-living vaccines: the potential of dendritic cell-based vaccination strategies against Leishmania. Front. Immunol. 9:1227. doi: 10.3389/fimmu.2018.01227
Seyed, N., and Rafati, S. (2021). Th1 concomitant immune response mediated by IFN-gamma protects against sand fly delivered Leishmania infection: implications for vaccine design. Cytokine 147:155247. doi: 10.1016/j.cyto.2020.155247
Silvestre, R., Cordeiro-da-Silva, A., and Ouaissi, A. (2008). Live attenuated Leishmania vaccines: a potential strategic alternative. Arch. Immunol. Ther. Exp. 56, 123–126. doi: 10.1007/s00005-008-0010-9
Singh, K., Kaviraj, S., Karmakar, S., and Singh, S. (2022). Vaccine for leishmaniasis: new era of CRISPR generated live attenuated dermotropic Leishmania. J Cell Mol Immunol. 1, 12–16. doi: 10.46439/immunol.1.004
Spath, G. F., Lye, L.-F., Segawa, H., Sacks, D. L., Turco, S. J., and Beverley, S. M. (2003). Persistence without pathology in phosphoglycan-deficient Leishmania major. Science 301, 1241–1243. doi: 10.1126/science.1087499
Spath, G. F., Lye, L. F., Segawa, H., Turco, S. J., and Beverley, S. M. (2004). Identification of a compensatory mutant (lpg2-REV) of Leishmania major able to survive as amastigotes within macrophages without LPG2-dependent glycoconjugates and its significance to virulence and immunization strategies. Infect. Immun. 72, 3622–3627. doi: 10.1128/IAI.72.6.3622-3627.2004
Suschak, J. J., Dupuy, L. C., Shoemaker, C. J., Six, C., Kwilas, S. A., Spik, K. W., et al. (2020). Nanoplasmid vectors co-expressing innate immune agonists enhance DNA vaccines for Venezuelan equine encephalitis virus and Ebola virus. Mol. Ther. Methods Clin. Dev. 17, 810–821. doi: 10.1016/j.omtm.2020.04.009
Suschak, J. J., Williams, J. A., and Schmaljohn, C. S. (2017). Advancements in DNA vaccine vectors, non-mechanical delivery methods, and molecular adjuvants to increase immunogenicity. Hum. Vaccin. Immunother. 13, 2837–2848. doi: 10.1080/21645515.2017.1330236
Topuz Ata, D., Hussain, M., Jones, M., Best, J., Wiese, M., and Carter, K. C. (2023). Immunisation with transgenic L. tarentolae expressing gamma glutamyl cysteine synthetase from pathogenic leishmania species protected against L. major and L. donovani infection in a murine model. Microorganisms. 11:1322. doi: 10.3390/microorganisms11051322
Uzonna, J. E., Spath, G. F., Beverley, S. M., and Scott, P. (2004). Vaccination with phosphoglycan-deficient Leishmania major protects highly susceptible mice from virulent challenge without inducing a strong Th1 response. J. Immunol. 172, 3793–3797. doi: 10.4049/jimmunol.172.6.3793
Valenzuela, J. G., Belkaid, Y., Garfield, M. K., Mendez, S., Kamhawi, S., Rowton, E. D., et al. (2001). Toward a defined anti-Leishmania vaccine targeting vector antigens: characterization of a protective salivary protein. J. Exp. Med. 194, 331–342. doi: 10.1084/jem.194.3.331
Vandermeulen, G., Marie, C., Scherman, D., and Preat, V. (2011). New generation of plasmid backbones devoid of antibiotic resistance marker for gene therapy trials. Mol. Ther. 19, 1942–1949. doi: 10.1038/mt.2011.182
Volpedo, G., Bhattacharya, P., Gannavaram, S., Pacheco-Fernandez, T., Oljuskin, T., Dey, R., et al. (2022). The history of live attenuated Centrin gene-deleted Leishmania vaccine candidates. Pathogens 11:431. doi: 10.3390/pathogens11040431
Volpedo, G., Huston, R. H., Holcomb, E. A., Pacheco-Fernandez, T., Gannavaram, S., Bhattacharya, P., et al. (2021). From infection to vaccination: reviewing the global burden, history of vaccine development, and recurring challenges in global leishmaniasis protection. Expert Rev. Vaccines 20, 1431–1446. doi: 10.1080/14760584.2021.1969231
Wang, Q., Jiang, W., Chen, Y., Liu, P., Sheng, C., Chen, S., et al. (2014). In vivo electroporation of minicircle DNA as a novel method of vaccine delivery to enhance HIV-1-specific immune responses. J. Virol. 88, 1924–1934. doi: 10.1128/JVI.02757-13
Williams, J. A. (2013). Vector design for improved DNA vaccine efficacy. Safety Product. Vaccines (Basel) 1, 225–249. doi: 10.3390/vaccines1030225
Yin, W., Xiang, P., and Li, Q. (2005). Investigations of the effect of DNA size in transient transfection assay using dual luciferase system. Anal. Biochem. 346, 289–294. doi: 10.1016/j.ab.2005.08.029
Zabala-Penafiel, A., Todd, D., Daneshvar, H., and Burchmore, R. (2020). The potential of live attenuated vaccines against cutaneous Leishmaniasis. Exp. Parasitol. 210:107849. doi: 10.1016/j.exppara.2020.107849
Zhang, W. W., Karmakar, S., Gannavaram, S., Dey, R., Lypaczewski, P., Ismail, N., et al. (2020). A second generation leishmanization vaccine with a markerless attenuated Leishmania major strain using CRISPR gene editing. Nat. Commun. 11:3461. doi: 10.1038/s41467-020-17154-z
Zhang, W. W., Lypaczewski, P., and Matlashewski, G. (2017). Optimized CRISPR-Cas9 genome editing for Leishmania and its use to target a multigene family, induce chromosomal translocation, and study DNA break repair mechanisms. mSphere 2:e00340-16. doi: 10.1128/mSphere.00340-16
Keywords: Leishmaniasis, vaccine, concomitant immunity, live attenuated vaccine, Leishmania tarentolae , antibiotic-free DNA vaccine
Citation: Seyed N, Taheri T and Rafati S (2024) Live attenuated-nonpathogenic Leishmania and DNA structures as promising vaccine platforms against leishmaniasis: innovations can make waves. Front. Microbiol. 15:1326369. doi: 10.3389/fmicb.2024.1326369
Received: 28 October 2023; Accepted: 12 March 2024;
Published: 03 April 2024.
Edited by:
Manas R. Dikhit, Rajendra Memorial Research Institute of Medical Sciences, IndiaReviewed by:
Abhik Sen, Indian Council of Research, IndiaCopyright © 2024 Seyed, Taheri and Rafati. This is an open-access article distributed under the terms of the Creative Commons Attribution License (CC BY). The use, distribution or reproduction in other forums is permitted, provided the original author(s) and the copyright owner(s) are credited and that the original publication in this journal is cited, in accordance with accepted academic practice. No use, distribution or reproduction is permitted which does not comply with these terms.
*Correspondence: Negar Seyed, bmVnYXJzZUBnbWFpbC5jb20=
Disclaimer: All claims expressed in this article are solely those of the authors and do not necessarily represent those of their affiliated organizations, or those of the publisher, the editors and the reviewers. Any product that may be evaluated in this article or claim that may be made by its manufacturer is not guaranteed or endorsed by the publisher.
Research integrity at Frontiers
Learn more about the work of our research integrity team to safeguard the quality of each article we publish.