- 1Key Laboratory of Helicobacter Pylori, Microbiota and Gastrointestinal Cancer of Henan Province, Marshall Medical Research Center, Fifth Affiliated Hospital of Zhengzhou University, Zhengzhou, China
- 2Department of Children Rehabilitation Medicine, Fifth Affiliated Hospital of Zhengzhou University, Zhengzhou, China
- 3Department of Gastroenterology, Fifth Affiliated Hospital of Zhengzhou University, Zhengzhou, China
The development of cancer is not just the growth and proliferation of a single transformed cell, but its tumor microenvironment (TME) also coevolves with it, which is primarily involved in tumor initiation, development, metastasis, and therapeutic responses. Recent years, TME has been emerged as a potential target for cancer diagnosis and treatment. However, the clinical efficacy of treatments targeting the TME, especially its specific components, remains insufficient. In parallel, the gut microbiome is an essential TME component that is crucial in cancer immunotherapy. Thus, assessing and constructing frameworks between the gut microbiota and the TME can significantly enhance the exploration of effective treatment strategies for various tumors. In this review the role of the gut microbiota in human cancers, including its function and relationship with various tumors was summarized. In addition, the interaction between the gut microbiota and the TME as well as its potential applications in cancer therapeutics was described. Furthermore, it was summarized that fecal microbiota transplantation, dietary adjustments, and synthetic biology to introduce gut microbiota-based medical technologies for cancer treatment. This review provides a comprehensive summary for uncovering the mechanism underlying the effects of the gut microbiota on the TME and lays a foundation for the development of personalized medicine in further studies.
1 Introduction
Cancer is one of the significant causes of death, affecting millions of people globally (Siegel et al., 2019). Only 5–10% of cancer cases are associated with genetics, while most are related to environmental factors (Anand et al., 2008). The tumor microenvironment (TME) has been confirmed to play an essential role in tumor initiation and development, with its interactions with cancer cells well-studied (Kise et al., 2016). Most scientists believe that the TME can offer efficient and cost-effective therapeutics for various cancers, including gastric and colon cancers (Merlo et al., 2006). The TME comprises noncellular components and noncancerous host cells, including endothelial cells, fibroblasts, immune cells, and even microbes (Whisner and Athena Aktipis, 2019). The chemopathological qualities of the TME were classified into six categories, contributing to an in-depth understanding of its complexity and heterogeneity and guiding anticancer therapy (Jin and Jin, 2020). Considering the strengthened comprehension of the essential effects of TME on tumor growth and therapeutic resistance, therapeutic benefits in cancer patients have been achieved by targeting components of the TME (Xiao and Yu, 2021). Therefore, a comprehensive understanding of the TME provides a framework for preventing and treating cancers.
Microbiota is one of the cellular components in TME that play an essential and irreplaceable role in human systems as well as other factors like genetic due to the microbiota community can modulate various biological processes including cellular metabolism, physiology, and immune responses (Marsland et al., 2015; Andreeva et al., 2020). Disturbances in the human microbiota have been linked to several diseases, such as inflammatory bowel diseases (IBDs), cardiovascular diseases, and cancer (De Martel et al., 2012). Microbiota can form the TME for tumor initiation and development by regulating mucosal immunity and hormonal elements in humans (De Martel et al., 2012). Modulating host-microbiota interactions, especially in the gut, which hosts the most rich and diverse microbiota, has emerged as a state-of-the-art therapeutic approach for cancer treatment (Schwabe and Jobin, 2013). Previous studies have shown that the gut microbiota can regulate the sensitivity and responses of cancer patients to chemotherapy (Liu et al., 2022; Rahman et al., 2022). Furthermore, alterations in gut microbial structure have been reported to serve as potential indicators for early cancer diagnosis and other diseases (Sepich-Poore et al., 2021). Therefore, a comprehensive understanding of the interaction between the gut microbiota and the TME is beneficial for developing effective, safe, and patient-friendly treatments.
In this review, we (1) investigated the effects of microbiota and the TME on host immunity; (2) introduced their mutual effects on cancer prevention and therapy; and (3) discussed various methods for adjusting the TME to maximize the therapeutic effect of cancer, including fecal microbiota transplantation (FMT), dietary adjustments, and synthetic biology design. This study will provide a foundation for cancer-targeted therapies based on the gut microbiota and the TME in future applications and studies.
2 Role of the gut microbiota in cancer
2.1 Human microbiota
The human body hosts various microbes, with over 100 trillion symbiotic microorganisms (Sender et al., 2016). The human microbiota comprises complicated communities of bacteria, archaea, and viruses (Matson et al., 2021). The primary colonizers in these communities belong to six phyla: Firmicutes, Bacteroidetes, Proteobacteria, Actinobacteria, Fusobacteria, and Cyanobacteria (Ghosh and Pramanik, 2021). However, the relative abundance and load of these phyla, especially the bacterial composition at the genera level, differ significantly among the different communities (Cho and Blaser, 2012). Each anatomical niche, including the skin, gut, vagina, nose, mouth, and conjunctiva, possesses a distinct mixture of microbial populations. Among these, the human microbiota, especially the gut microbiota, has gained more attention due to its significant effect on human health and diseases (O'Hara and Shanahan, 2006). However, the relationship between microbiota and tumorigenesis is complicated as it is influenced by the microbial community and abiotic factors. Studies have reported that changes in the gut microbial community and its homeostasis can influence the development and progression of multiple cancers in humans. Chronic inflammation caused by the gut microbiota is a widely accepted mechanism that promotes tumor development. Furthermore, substances released by gut bacteria have been found to damage DNA, resulting in pathogenic mutations (Kumar et al., 2023). Notably, certain species of gut bacteria exhibited antitumor effects in some animal studies, particularly those involved in short-chain fatty acid (SCFA) synthesis (Yao et al., 2022). Moreover, gut bacteria can enhance the immune response to tumors by activating the immune system (Ge et al., 2021). By studying the tumor-associated gut microbiota, cancer prognosis can be predicted, and thus, stopping the generation of these associated microbes can halt cancer progression. Research on this microbiota would provide a novel and more patient-friendly strategy for cancer treatment.
2.2 Cancer microbiota
The International Association for Cancer Research has identified 11 microbes as human carcinogens or “oncomicrobes,” including Human Papillomaviruses, Hepatitis B virus, Hepatitis C virus, Epstein–Barr virus, Human T-cell lymphotropic virus type I, Human immunodeficiency virus type 1, Human herpesvirus 8, Merkel cell polyomavirus, Helicobacter pylori (H. pylori), Opisthorchis viverrini, and Schistosoma haematobium (Plummer et al., 2016). Among these, H. pylori, regarding gut microbiota modulation, has received significant attention and has been well studied. H. pylori is known to cause chronic inflammation of the gastric mucosa, potentially leading to gastric and duodenal ulcers, and is confirmed to be related to mucosa-associated lymphoid tissue lymphoma (extranodal marginal zone B-cell lymphoma) in the stomach (Wang et al., 2014). Consequently, since gastric cancer caused by H. pylori infection depends significantly on the long-term inflammatory response of the host immune system, understanding the relationship between H. pylori and other gastric bacterial infections and host immune responses at the molecular level during gastric carcinogenesis is of great importance (Kim and Wang, 2021).
The molecular mechanisms underlying the epidemiology of oncomicrobes and their clinical scenarios have been well studied (Sepich-Poore et al., 2021). Although carcinogenic microbiota can colonize various parts of the human body, their detection in microbial-triggered cancer is challenging, mainly due to individual differences in genetic makeup (Ribet and Cossart, 2015). In addition, certain microbiota can cause cancer through genotoxin-mediated mutagenesis, such as colibactin (Wilson et al., 2019) and cytolethal distending toxins, indicating that not all microbiota are carcinogenic or can be conditionally carcinogenic, e.g., prolonged H. pylori infection can trigger gastric cancer (Parsonnet, 1993; Matysiak-Budnik and Mégraud, 2006).
Increasing evidence shows that a significant “complicit” microbiota can trigger carcinogenesis through interactions with other abiotic factors. This category encompasses multiple immunomodulatory roles of microbiota and their bioactive metabolites involved in tumor growth, which might be related to the effect of the immune system on solid tumorigenesis (Bagheri et al., 2022). Tumors located on boundary surfaces-including the oropharynx, skin, and the respiratory, digestive, and genitourinary tracts-contain microbiota, which complicates cancer-microbe causality (Garrett, 2015). The gut microbiota establishes the core factors of the gut microenvironment under healthy and cancerous conditions. Simultaneously, different TMEs show diverse community structures of the central gut microbiota. The different gut microbiota compositions associated with various cancers are summarized in Table 1. Moreover, a decrease in the abundance of specific microorganisms may also increase the cancer risk of the host in areas far from the transfer of such microorganisms (Sears and Garrett, 2014). Therefore, understanding microbes throughout the body is essential for understanding the relationship between the gut microbiota and cancer.
Given the high individual heterogeneity of the gut microbiota due to variations in genetics, diet, and other factors, its performance varies across subtypes of certain cancers. The gut microbiota is highly related to chronic inflammation in multiple organs, which can promote the development and progression of tumorigenesis. However, it is unrelated to cancers resulting from genetic inheritance or mutations (Karki and Kanneganti, 2019). Previous research has found that Enterobacteriaceae exhibits high abundance across all subtypes of gastric tumors, whereas Lachnoclostridium, Bifidobacterium, Parabacteroides, and Barnesiella are found in patients with adenocarcinoma (Zhou et al., 2021). The gut microbial community and biodiversity significantly depend on the types/subtypes of tumors and different tumor stages (Chen et al., 2022). Although the role of the gut microbiota in subtypes of different cancers requires further study and clarification, its potential for tumor diagnosis and treatment has gained widespread recognition.
3 Relationship between the gut microbiota and the TME
3.1 The microbiome as an ingredient of the tumor microenvironment
The TME comprises malignant and nonmalignant cells and the contents of the tumor (Figure 1). The permanent mutual relationship between tumor cells and the TME significantly affects tumor initiation, progression, metastasis, and therapeutic responses (Xiao and Yu, 2021). Recently, the conventional drugs including aspirin, celecoxib, β-adrenergic antagonist, metformin, and statin with antitumor capability that show potential use in combination therapy by targeting TME components (Jin and Jin, 2020). Due to the different layers of microbial niches, the TME is a complex environment in which the microbiota has been recognized as a novel yet essential element (Turroni et al., 2008; Rowland et al., 2018). The microbiota functionally reduces tumor cell metabolism, such as inflammation, genotoxin generation, and production of bacterial metabolites with various characteristics (Kovács et al., 2020). Accumulating evidence has shown that the interactions between the microbiota and their metabolites in the TME can influence host immunity and the intestinal epithelium, ultimately driving or inhibiting tumor growth (Barry et al., 2018).
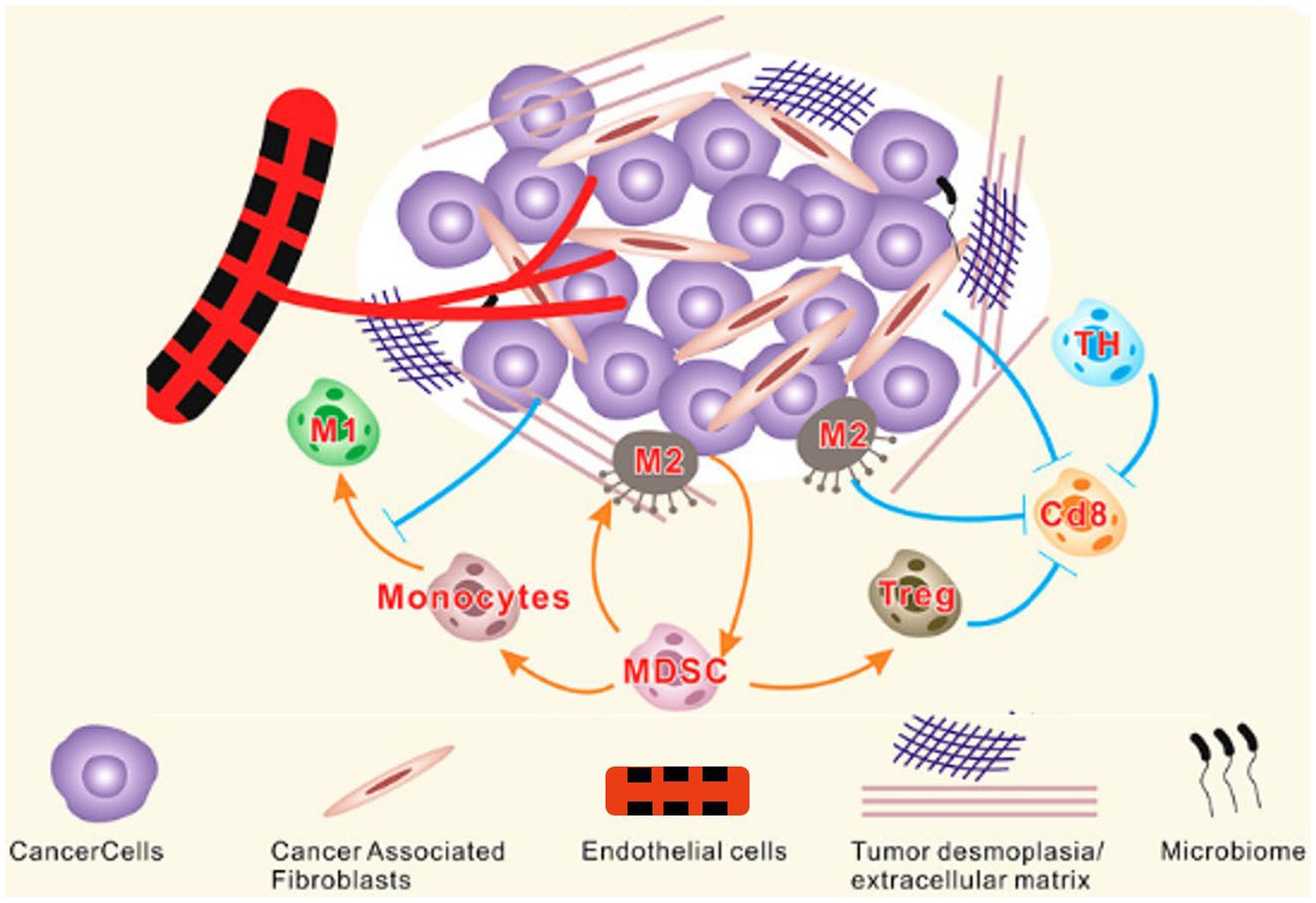
Figure 1. Components of TME. The TME is a complex network of stromal cells, microbiome and other cellular entities surrounding tumor cells. Tumor and stromal cells actively interact to support tumor growth by promoting desmoplasia, angiogenesis, and immune suppression. MDSC, Myeloid-derived suppressor cells; TH, T helper cells; M, Macrophages (Zubair et al., 2022).
The model of the gut microbiota and the TME is complex, including biotic and abiotic drivers from cells, blood vessels, and the extracellular matrix that constitutes the tissues surrounding a tumor (Zhu et al., 2021). Studies have reported that gut bacteria can regulate the activation of human immune cells to migrate to the TME for tumor cell elimination (Buzas, 2023). In addition, the complex interaction between the gut microbiota and the TME can enable tumor cells to evade the immune system and proliferate more efficiently (Kalaora et al., 2022). Understanding this system holds promise for cancer prevention, diagnosis, and treatment.
3.2 Effects of the gut microbiota on the TME
The gut microbiota is crucial in the development, maintenance, and growth of the host immune system (Shi et al., 2017). The intestinal ecosystem can affect local and distant neoplasia by influencing the influx of myeloid, immune context, lymphoid cells, and inflammatory and metabolic patterns (Ma et al., 2019). Thus, the gut microbiota is emerging as a critical modulator of the TME in various cancers, such as colorectal, gastric, and liver cancers (Lakritz et al., 2014). For instance, a previous study reported that bacteria such as Fusobacterium nucleatum can enhance tumor growth by inhibiting human immune responses (Chattopadhyay et al., 2021). Moreover, breast and ovarian cancers are associated with specific biosignatures of the gut microbiota, such as the abundance of Lactobacillus crispatus, which negatively correlates with cancer occurrence (Banerjee et al., 2018).
Furthermore, the secretory components of the gut microbiota are reported to be associated with TME. For example, outer membrane vesicles (OMVs) can reprogram the TME toward a pro-TH1 pattern (CXCL10, IFN-g; Kim et al., 2017). Metabolites produced by the gut microbiota, including butyrate and niacin, can mediate Gpr109a-dependent interleukin (IL)-18 induction in the colonic epithelium, suppressing colitis and colon cancer. Additionally, the TME can regulate tumor development, metastatic progression, and the efficacy of therapeutic interventions (Figure 2; Singh et al., 2014). Studies have found that tumor cells can establish a bidirectional functional relationship with the surrounding stromal cells during malignant progression (Poutahidis et al., 2013). The synthesis and secretion of sonic hedgehog, which selectively reacts with stellate cells, are promoted by the activation of the CXCL12/CXCR4 pathway in pancreatic tumor cells, thereby driving desmoplasia (Singh et al., 2012). The desmoplastic TME affects pancreatic cancer pathobiology and chemoresistance (Özdemir et al., 2014). One study on lung cancer showed that cancer-associated fibroblast (CAF)-derived IL-6 induces epithelial-mesenchymal transition and confers resistance to cisplatin in non-small cell lung carcinoma (Yang et al., 2016). Moreover, CAFs secrete various proinflammatory molecules (IL-6, CCL2, and TGF-β), which enhance immunosuppressive cell recruitment (Dirkx et al., 2006).
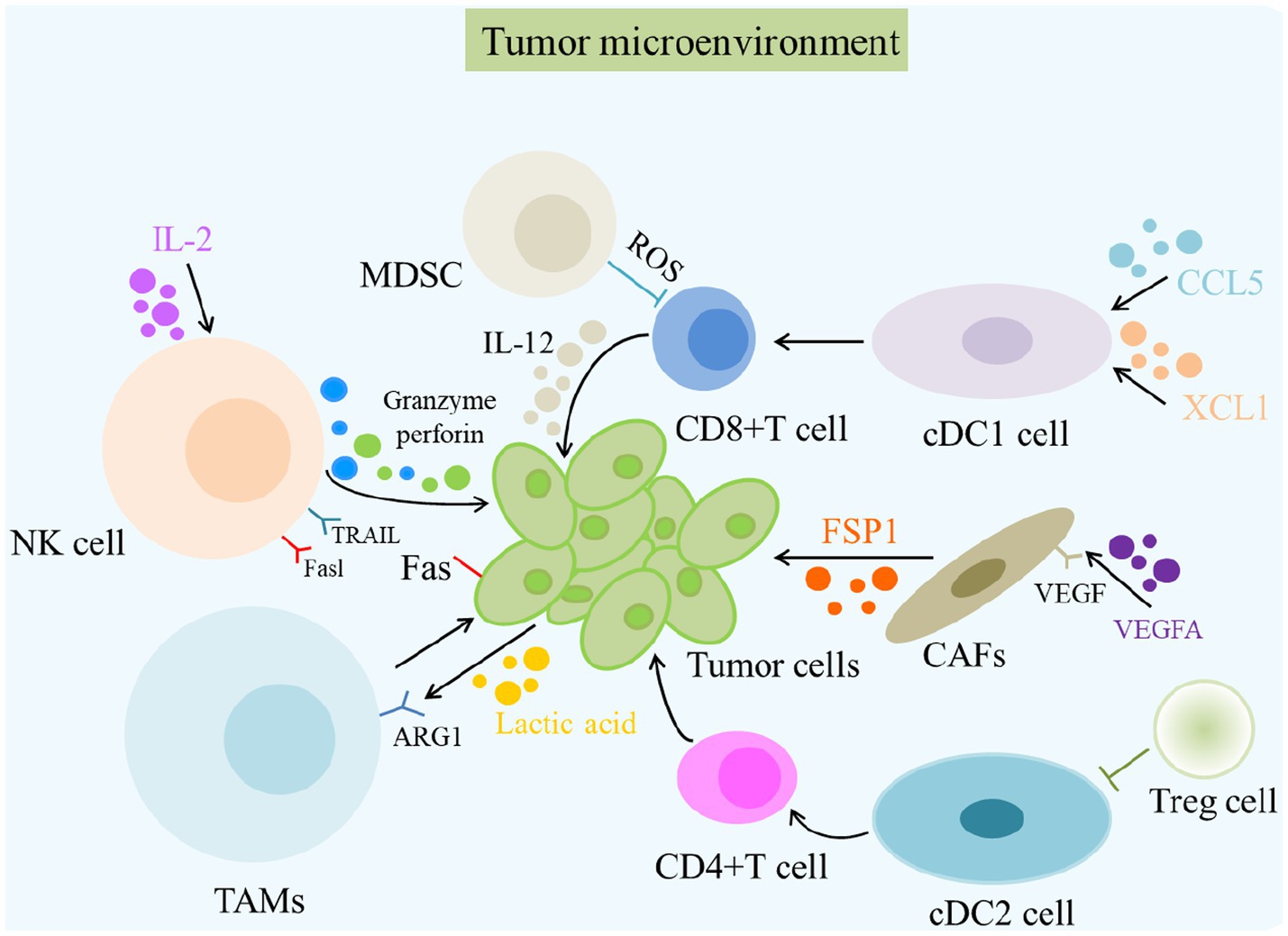
Figure 2. The role of TME in cancer and its immunotherapy. The primary cells of the TME in cancer immunity are NK cells, DC cells, CD8 + T cells, Treg cells, fibroblasts, TAMs, and MDSCs. Different cells induce the death of tumor cells in various ways, such as releasing perforin and granzyme and mediating cytotoxicity by TRAIL and Fasl receptors. MDSC, Myeloid-derived suppressor cells; ROS, Reactive oxygen species; NK cells, Natural killer cells.
High accumulation of tumor-associated macrophages (TAMs) and other immunosuppressive cells in the TME induces cancer progression and therapy resistance (Xiang et al., 2021; Yan and Wan, 2021). The depletion of CD163+ TAMs, which cause immune suppression, leads to robust infiltration of cytotoxic T cells into the TME, resulting in the control of melanoma development (Etzerodt et al., 2019). High CD163+ TAMs in the TME have been linked to worse clinical outcomes in patients with various myelomas (Omatsu et al., 2014). Pancreatic tumors exhibit a growing infiltration of TAMs and a scarcity of cytotoxic T cells in their TME (Lankadasari et al., 2019). Additionally, the effects of TAMs on angiogenesis have been previously reported (Larionova et al., 2021). For example, TAM depletion can induce a significant decrease in vessel density (Yang et al., 2016). Thus, various factors, such as MMPs, ILs, VEGF, PDGF, and TGF-β secreted by TAMs in the TME, can promote vascularization in tumor tissues (Dirkx et al., 2006). MDSCs are used to heavily infiltrate the TME of glioblastoma, activating B-cell–induced immune suppression by inhibiting CD8+ T-cell activation (Lee-Chang et al., 2019). Furthermore, the high intratumoral burden of F. nucleatum correlates with a poor response to neoadjuvant chemotherapy in patients with esophageal squamous cell carcinoma (Yamamura et al., 2019). Therefore, the TME microbiota significantly influences cancer pathogenesis and therapeutic outcomes.
Noncellular components of the TME are also crucial for cancer progression, aggressiveness, and chemoresistance (Schulz et al., 2019). The stiffness of the extracellular matrix promotes tumor cell survival and proliferation while upregulating integrin signaling (Pickup et al., 2014). Hyaluronic acid, a CD44 receptor, is abundant in the TME of various cancers (Mattheolabakis et al., 2015). Their mutual effects activate cancer-promoting signaling pathways and induce the upregulation of noncoding RNA species, such as miR-10b/miR-302/miR-21 and lncRNAs. In pancreatic cancer, the stroma is highly reactive with different hyaluronic acids, resulting in elevated interstitial fluid pressures that lead to vascular collapse and poor chemotherapy outcomes (Provenzano et al., 2012). Targeting enzymes in pancreatic tumors with recombinant hyaluronidase has been shown to degrade hyaluronic acid and enhance therapy by reducing metastasis and improving survival (Kim et al., 2021). In addition, the secretory components of the gut microbiota are related to the TME. For instance, OMVs can reprogram the TME toward a pro-TH1 pattern (CXCL10, IFN-g; Kim et al., 2017), while metabolites, such as butyrate and niacin, can mediate the Gpr109a-dependent induction of IL-18 in the colonic epithelium, suppressing colitis and colon cancer.
Metabolites from the gut microbiota enter host cells and interact with the human immune response, promoting various tumor-inhibitory and immunomodulatory molecules. They also inhibit inflammation by maintaining the integrity of the epithelial barrier and the intestinal tract (Rooks and Garrett, 2016). A previous study found that the products of the metabolic activities of the gut microbiota significantly affect host metabolic pathways related to adiposity, lipids, and energy homeostasis (Poutahidis et al., 2013). Thus, uncovering how metabolites and submetabolites from the gut microbiota affect immune cells and reshape the TME can strongly contribute to the development of tumor therapeutics.
Gut microbiota metabolites, such as SCFAs and inosine, directly or indirectly interact with the TME to reshape it, thereby affecting the cancer process (Min et al., 2005). SCFAs contribute to maintaining intestinal homeostasis and regulating intestinal barrier function (Wang et al., 2017). Moreover, several fatty and cholic acids are associated with inflammation (Min et al., 2005). Butyrate and SCFAs, which can be generated by Faecalibacterium prausnitzii, control angiogenesis and reduce the expression of proangiogenic factors. Thus, increasing butyrate concentration is believed to slow down and halt cancer growth (Davie, 2003). Conversely, deoxycholic and petrocholic acids can cause DNA damage by increasing the generation of reactive oxygen species (Payne et al., 2007). Recent studies have shown that the intestinal bacteria B. pseudolongum can produce inosine, which drives Th1 cell differentiation in the presence of exogenous IFN-g (Kroemer and Zitvogel, 2020). Moreover, the status of B. pseudolongum has been reported to be associated with the response to ICB therapy, such as anti-CTLA-4 and anti-PD-L1, through its interaction with the adenosine A2A receptor on T cells (Mager and Burkhard, 2020). CTLA-4 and PD-L1 are the primary targets of immune checkpoint therapy, which involves membrane-bound molecules that impede unbounded T-cell responses after initial stimulation (Mager et al., 2020). Thus, cancer cells can avoid immune surveillance by employing this mechanism. However, while reactivating inefficient T cells, immune checkpoint inhibitors (ICIs) can restore the response to tumor antigens (Sharma and Allison, 2015). Clinical research and preclinical trials have revealed that the gut microbiota affects the efficacy of ICIs, thereby explaining significant variations in patients’ responses to ICIs (Vétizou et al., 2015). Hence, gaining an in-depth understanding of how the gut microbiota, their metabolites, and the host immune system interact to reshape and regulate the TME holds promise for advancing cancer immunotherapy.
Overall, the effects of the gut microbiota on the TME are complex and not yet fully understood. However, studies on this system have demonstrated its potential application in manipulating gut microbes to influence the effectiveness of cancer treatment and improve patient outcomes.
4 Gut microbiota modulation and their TME target
4.1 Cancer diagnostics based on microbiota
Cancer is typically diagnosed following the identification of a lump through palpation or imaging techniques, followed by a biopsy to confirm cellular malignancy (Fass, 2008). Tomographic detection techniques, including PET, MRI, and CT, efficiently identify macroscopic lesions in the body (Pokharel et al., 2013). Compared to stable genetic characteristics, the homeostasis of the human gut microbiota is more susceptible to tumorigenesis. Furthermore, studies have confirmed that the gut microbiota dynamics can potentially aid in diagnosing and locating malignancies, such as Streptococcus gallolyticus bacteremia, based on their gastrointestinal origin (Klein et al., 1977). Most microbial-based cancer diagnostics focus on sequencing tumors within the aerodigestive tract, including colorectal (Flemer et al., 2017), pancreatic (Farrell et al., 2012), and lung cancer (Yan et al., 2015). It has been suggested that different cancer types may host microbiota with unique compositions outside the aerodigestive tract, such as in the oral cavity. Nejman et al. investigated intratumoral microbiota from over 30 cancers, applying their blood-based diagnostics and providing visual evidence of microbial intratumoral spatial distributions and intracellular localization in seven different cancers (Nejman and Livyatan, 2020).
Currently, several bacteria-based strategies have been developed for tumor detection (Panteli et al., 2015), including the use of engineered bacteria that combine the specificity of tumor-targeting bacteria with the sensitivity of biomarker assays (Panteli et al., 2020). Attenuated bacteria were engineered to release an exogenous reporter protein, ZsGreen, using a remotely inducible genetic switch (Kaimala et al., 2018). Both in vivo and in vitro experiments showed that these bacteria could identify tumors through systemic measurements of the released ZsGreen (Panteli et al., 2020). Although bacteria-based cancer diagnosis is a promising strategy, it faces several challenges, such as low biomass relative to the host and confounding from reagents or environmental pollutants. Thus, combining gut microbiota-based methods with conventional diagnostic techniques, including genome sequencing, qPCR, immunohistochemistry, and electron microscopy, can offer more accurate and efficient cancer diagnoses.
Microbial-based cancer diagnostics have emerged as a new area that is focused on designing or developing novel strategies based on specific biosignatures of the gut microbiota in various cancers or at different tumor stages (Kim and Lee, 2021). Furthermore, deep learning and machine learning algorithms enable the identification of microbial profiles indicative of cancers, which is the basis of precision medicine. Microbial-based cancer diagnostics also hold the potential to improve cancer screening and early detection efforts, promising the development of more accurate and effective diagnostic tools for various cancers and ultimately improving patient outcomes.
4.2 Microbial-based cancer therapy
The human gut microbiota is recognized as a fundamental component of the immune system (Hooper and Macpherson, 2010; Maynard et al., 2012). Further studies have demonstrated that the gut microbiota can regulate immune responses, thus affecting the efficacy of cancer immunotherapy (Roy and Trinchieri, 2017). Several clinical trials have recently been conducted to alter the gut microbiota for cancer therapy (Table 2). Methods such as FMT, probiotics, dietary interventions (discussed in the subsequent section), and microbial engineering based on synthetic biology offer potential anticancer effects by targeting both tumor cells and the TME (Figure 3).
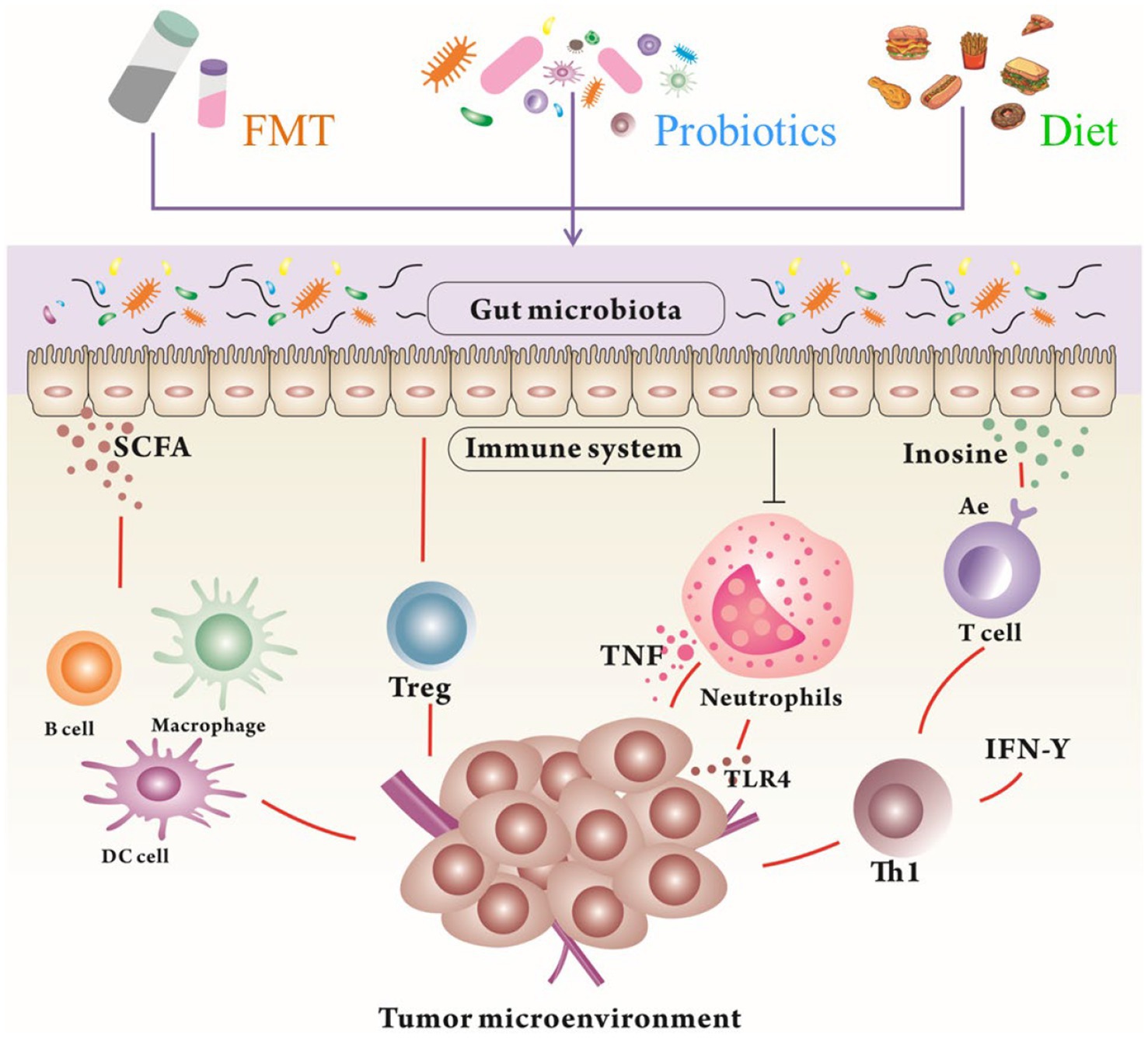
Figure 3. The modulation of the gut microbiota and their metabolites by FMT, probiotics, and diet to reshape TME for tumor therapy. Gut microbiota and their metabolites can promote the immunotherapy in humans through different mechanisms. FMT, Fecal Microbial Transplantation; SCFA, Short Chain Fatty Acids; TNF, Tumor necrosis factor.
FMT is an artificial strategy for manipulating the gut microbiota and the TME (Zhang et al., 2020). Several clinical conditions, such as clostridium difficile infection, ulcerative colitis, and other gastrointestinal conditions, have been successfully treated by transferring fecal material from a donor to a recipient through colonoscopy, enema, or oral administration (Tan and Johnson, 2019). According to ongoing clinical trials, FMT from donors responsive to immunotherapy can enhance antitumor immune responses and potential clinical outcomes (Kang and Cai, 2021). Modifying the gut microbiota through FMT has been found to modulate the composition of the tumor microbiota, antitumor immune responses, and tumor growth kinetics (Matson et al., 2021). However, the long-term effectiveness and stability of FMT remain unclear (McQuade et al., 2019). Although some clinical trials have successfully incorporated the modulation of the gut microbiota using FMT into cancer therapy, the applications of FMT in cancer patients are limited due to antibiotic preconditioning, administration route, and modulation frequency, complicating its clinical use in targeting the gut microbiota (Cheng et al., 2020). Therefore, more specific clinical trials are needed for fecal transplants in cancer patients.
Probiotics are widely used to shift the microbial community (Zaramela et al., 2021). These interventions are being investigated for tumor therapy based on both retrospective studies and prospective clinical trials (Panebianco et al., 2020). Live bacteria are orally administered in probiotics, supplying substrates that stimulate the development or activity of beneficial bacteria in the gut and that further modulate the components of the overall gut microbiota (Markowiak and Śliżewska, 2017). Recent findings have confirmed that the gut microbiota can regulate immune responses, which could potentially affect the efficacy of cancer immunotherapy, indicating that probiotics can reduce the side effects of anticancer therapy (Lu et al., 2021). Several commercially available probiotics have been studied in preclinical models and clinical trials (Helmink et al., 2019). It has been reported that patient outcomes can be influenced by compositional variations in the gut microbiota or the TME (Helmink et al., 2019).
Anaerobic bacteria play a crucial role in the gastrointestinal tract (Zaramela et al., 2021). A functional gut microbiota Bifidobacterium is commonly used to treat IBDs, including ulcerative colitis (Zhang et al., 2021). The TME creates a suitable growth environment for anaerobic bacteria under low-oxygen conditions (Leppäranta et al., 2008). The antitumor effects of anti-CD47 immunotherapy can be significantly improved by accumulating Bifidobacterium in the TME (Sivan et al., 2015). Current clinical trials primarily focus on the effectiveness of probiotic treatment for colorectal, kidney, breast, gynecologic, and lung cancers.
Microbial metabolites also contribute to regulating antitumor immunity (Sipe et al., 2020). SCFAs are crucial for maintaining gut integrity and serve as the primary energy source for intestinal epithelial cells (Parada Venegas et al., 2019). SCFAs, such as acetate, propionate, and butyrate, are absorbed through the intestinal epithelium and transmitted to T cells through G-coupled protein receptors to influence tumor differentiation (Moniri and Farah, 2021). In the colon, SCFAs protect gut integrity from invading foreign microorganisms by inducing Treg cells or IL-10 (Park et al., 2015). A direct interaction exists between SCFAs and CD8+ T cells in the circulation system, enhancing their antitumor effects (Bachem et al., 2019). Overall, SCFA-producing microbiota contribute to the response to ICIs (Huang et al., 2020). In addition, prebiotics and synbiotics (a combination of probiotics and prebiotics) are ideal for cancer prevention (Raman et al., 2013). Prebiotics are defined as fermentable, nondigestible food ingredients that can improve the health of the host (Legesse Bedada et al., 2020).
Dietary fibers resist digestion and absorption in the small intestine but undergo complete or partial fermentation in the large intestine (Buttriss and Stokes, 2008; Mudgil and Barak, 2013). Most fractions of edible plants or their extracts are carbohydrates and are regarded as prebiotics (Hijová et al., 2019). Fermentation of nondigestible compounds is key to proliferation and apoptosis modulation in tumor cells (Cruz-Bravo et al., 2014). Prebiotics protect cells against cancer through fecal bulking, colonic pH change, carcinogen binding to bacteria, xenobiotic-metabolizing enzymes, gene expression modulation in feces and cecum, and immune response modulation (Harris and Ferguson, 1993). Therefore, diet, lifestyle, and gut microbiota composition are related (Shamekhi et al., 2020). Regarding dysbiosis or bacterial imbalance in the intestine due to variations in diet or the environment, tumors can be induced by virulence factors, microbial metabolites, and inflammatory routes (Dos Reis et al., 2017).
4.3 Synthetic biology application on cancer diagnosis and therapy
Synthetic biology has enabled the modification of living cells through sophisticated decision-making processes to achieve user-defined outcomes, such as creating sense-and-respond adaptive therapies (Kitada et al., 2018). Some bacterial species selectively proliferate and accumulate at tumor sites, making them suitable candidates for tumor monitoring and targeted therapy (Figure 4; Kramer et al., 2018). The ability of bacteria to target tumors can be improved through synthetic biology, and therapeutic payloads can be delivered with increased precision. For instance, to decrease off-target effects in healthy tissues, bacteria have been engineered with quorum sensing switches that activate effector gene expression only when the bacterial population reaches a certain threshold density (Anderson et al., 2006). Alternatively, bacteria expressing therapeutic payloads can infiltrate tumor cells or utilize the type III secretory system (T3SS). This syringe-like, protein-based structure injects proteins into target cells (Huh et al., 2013). T3SS has been used to engineer Salmonella to deliver antiangiogenic proteins to tumor cells for controlling tumor growth in vivo or tumor-related antigens to antigen-presenting cells for triggering antitumor immunity (Shi et al., 2016). In addition, bacteria were programmed to degrade adenosine and kynurenine (West et al., 2018), which inhibit antitumor immunity (Siska and Rathmell, 2015) and produce cyclic-di-AMP (Leventhal et al., 2018), thus activating the stimulator of interferon genes pathway to enhance antitumor immunity (Chen et al., 2016).
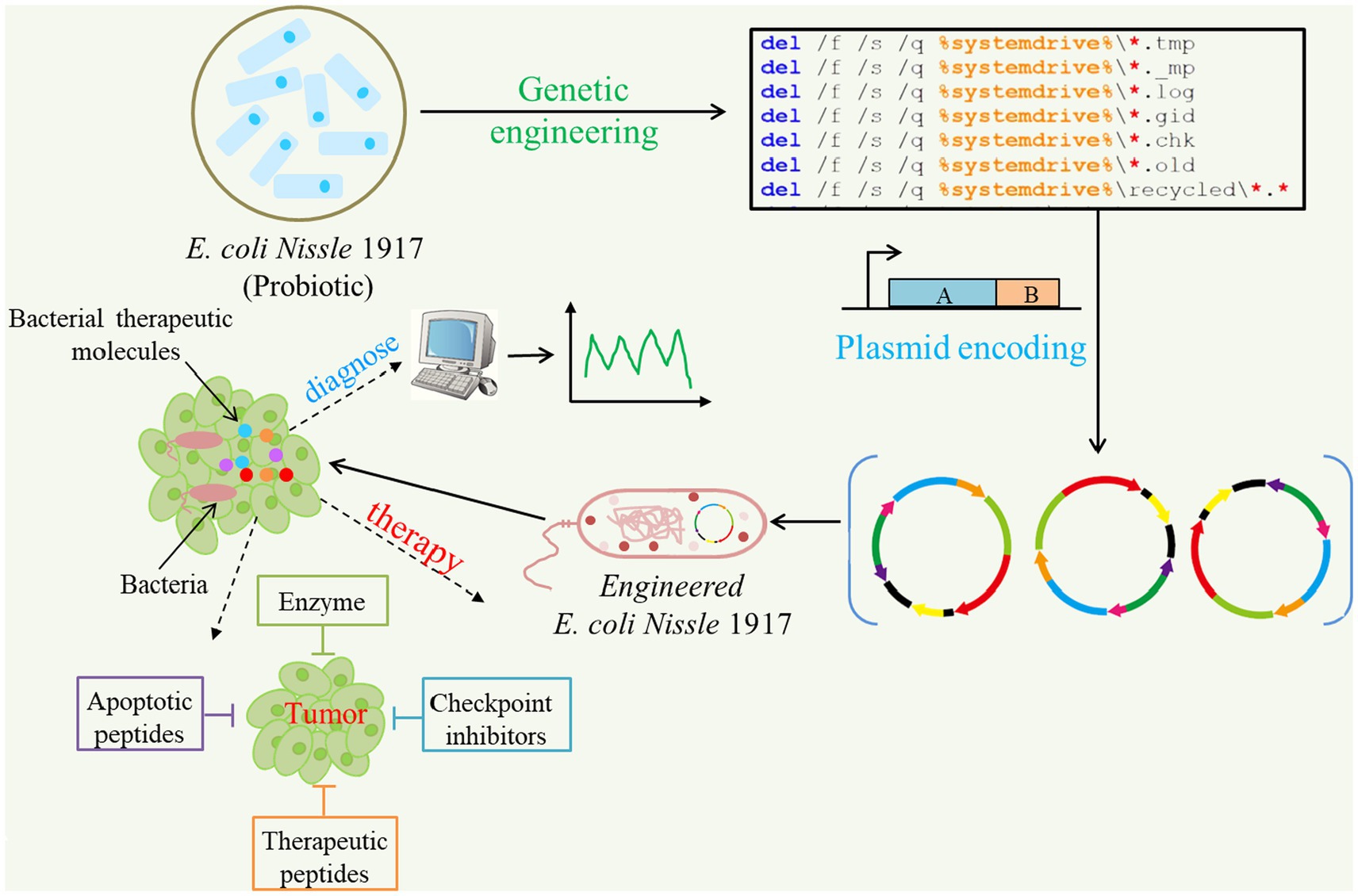
Figure 4. Applications of synthetic biology for cancer diagnosis and targeted therapeutics. Some probiotics like E. coli Nissle 1917 can be programmed to produce and deliver anti-cancer agents in solid tumors. Multiple drug payloads can be encoded by one or more engineered strains against tumors.
Several studies have shown a significant decrease in tumor growth using anticancer-related bacteria in preclinical mouse models (Din et al., 2016). However, bacterial susceptibility was not eliminated by the host’s immune system (Grushkin, 2012). Thus, the use of anticancer-related bacteria may involve sophisticated engineering to ensure their efficient action before they are released by the host’s immune system.
5 Prospective and conclusion
The human gut microbiota plays a critical role in tumor growth, progression, and treatment. The interaction among the gut microbiota, host’s immune system, and tumors can offer valuable insights into adjusting the gut microbiota to optimize the TME and enhance cancer immunotherapy.
The human microbiota significantly impacts the overall health of the human host and contributes to the development of various diseases. However, our current understanding of how human microbiota can confer susceptibility to certain cancers remains incomplete. A significant knowledge gap still exists regarding the underlying mechanisms governing bacterial activity as well as the compositions of microbiota due to limitations in culturing many bacterial species, small clinical sample sizes, and a lack of risk assessment data.
Individual diversity in the gut microbiota is the primary challenge for large-scale validation and further intestinal microecology analysis. Therefore, integrating biological information, extensive data, and artificial intelligence into precision medicine would be aid in the future 10 development of novel drugs. However, these methods are not yet widely employed, and further in-depth investigations are necessary to ensure their adoption. In future, personalized medicine will likely incorporate microbiome-based diagnosis and treatment strategies. Despite the current challenges, a powerful new toolkit has been developed by enhancing our understanding of the roles of microbiota in cancer to improve patient care.
Author contributions
PF: Conceptualization, Writing – original draft. XX: Writing – review & editing. IB: Writing – review & editing. CQ: Data curation, Software, Writing – review & editing. YL: Data curation, Supervision, Writing – review & editing. PZ: Conceptualization, Writing – review & editing. YM: Writing – review & editing.
Funding
The author(s) declare financial support was received for the research, authorship, and/or publication of this article. This work was supported by Zhengzhou Major Collaborative Innovation Project (No. 18XTZX12003); Key projects of discipline construction in Zhengzhou University (No. XKZDJC202001); National Key Research and development program in China (No. 2020YFC2006100); Medical service capacity improvement project of Henan Province in China (Grant number Yu Wei Medicine [2017] No. 66); Natural Science Foundation of Henan Province (No. 212300410399). Key Research and Development and Promotion Special Projects of Henan Province (No. 232102311068).
Conflict of interest
The authors declare that the research was conducted in the absence of any commercial or financial relationships that could be construed as a potential conflict of interest.
Publisher’s note
All claims expressed in this article are solely those of the authors and do not necessarily represent those of their affiliated organizations, or those of the publisher, the editors and the reviewers. Any product that may be evaluated in this article, or claim that may be made by its manufacturer, is not guaranteed or endorsed by the publisher.
References
Anand, P., Kunnumakara, A. B., Sundaram, C., Harikumar, K. B., Tharakan, S. T., Lai, O. S., et al. (2008). Cancer is a preventable disease that requires major lifestyle changes. Pharm. Res. 25, 2097–2116. doi: 10.1007/s11095-008-9661-9
Anderson, J. C., Clarke, E. J., Arkin, A. P., and Voigt, C. A. (2006). Environmentally controlled invasion of cancer cells by engineered bacteria. J. Mol. Biol. 355, 619–627. doi: 10.1016/j.jmb.2005.10.076
Andreeva, N. V., Gabbasova, R. R., and Grivennikov, S. I. (2020). Microbiome in cancer progression and therapy. Curr. Opin. Microbiol. 56, 118–126. doi: 10.1016/j.mib.2020.09.001
Bachem, A., Makhlouf, C., Binger, K. J., de Souza, D. P., Tull, D., Hochheiser, K., et al. (2019). Microbiota-derived short-chain fatty acids promote the memory potential of antigen-activated CD8+ T cells. Immunity 51, 285–297. doi: 10.1016/j.immuni.2019.06.002
Bagheri, Z., Moeinzadeh, L., and Razmkhah, M. (2022). Roles of microbiota in Cancer: From tumor development to treatment. J. Oncol. 2022, 3845104.
Banerjee, S., Tian, T., Wei, Z., Shih, N., Feldman, M. D., Peck, K. N., et al. (2018). Distinct microbial signatures associated with different breast Cancer types. Front. Microbiol. 9:951. doi: 10.3389/fmicb.2018.00951
Barry, K. C., Hsu, J., Broz, M. L., Cueto, F. J., Binnewies, M., Combes, A. J., et al. (2018). A natural killer–dendritic cell axis defines checkpoint therapy–responsive tumor microenvironments. Nat. Med. 24, 1178–1191. doi: 10.1038/s41591-018-0085-8
Buttriss, J., and Stokes, C. (2008). Dietary fibre and health: an overview. Nutr. Bull. 33, 186–200. doi: 10.1111/j.1467-3010.2008.00705.x
Buzas, E. I. (2023). The roles of extracellular vesicles in the immune system. Nat. Rev. Immunol. 23, 236–250. doi: 10.1038/s41577-022-00763-8
Chattopadhyay, I., Dhar, R., Pethusamy, K., Seethy, A., Srivastava, T., Sah, R., et al. Exploring the role of gut microbiome in Colon Cancer. 193 (2021) 1780–1799 London: BioMed Central.
Chen, Y., Huang, Y., Ding, X., Yang, Z., He, L., Ning, M., et al. (2022). A multi-omics study of familial lung Cancer: microbiome and host gene expression patterns. Front. Immunol. 13:827953. doi: 10.3389/fimmu.2022.1101130
Chen, Q., Sun, L., and Chen, Z. J. (2016). Regulation and function of the cGAS–STING pathway of cytosolic DNA sensing. Nat. Immunol. 17, 1142–1149. doi: 10.1038/ni.3558
Cheng, Y.-W., Alhaffar, D., Saha, S., Khanna, S., Bohm, M., Phelps, E., et al. (2020). Fecal microbiota transplantation is safe and effective in patients with Clostridioides difficile infection and cirrhosis. Clin. Gastroenterol. Hepatol. 18, 2234–2243.e1. doi: 10.1016/j.cgh.2019.12.029
Cho, I., and Blaser, M. J. (2012). The human microbiome: at the interface of health and disease. Nat. Rev. Genet. 13, 260–270. doi: 10.1038/nrg3182
Cruz-Bravo, R. K., Guevara-González, R. G., Ramos-Gómez, M., Oomah, B. D., Wiersma, P., Campos-Vega, R., et al. (2014). The fermented non-digestible fraction of common bean (Phaseolus vulgaris L.) triggers cell cycle arrest and apoptosis in human colon adenocarcinoma cells. Genes Nutr. 9:359. doi: 10.1007/s12263-013-0359-1
Cuevas-Ramos, G., Petit, C. R., Marcq, I., Boury, M., Oswald, E., and Nougayrède, J.-P. (2010). Escherichia coli induces DNA damage in vivo and triggers genomic instability in mammalian cells. Proc. Natl. Acad. Sci. 107, 11537–11542. doi: 10.1073/pnas.1001261107
Davie, J. R. (2003). Inhibition of histone deacetylase activity by butyrate. J. Nutr. 133, 2485S–2493S. doi: 10.1093/jn/133.7.2485S
De Martel, C., Ferlay, J., Franceschi, S., Vignat, J., Bray, F., Forman, D., et al. (2012). Global burden of cancers attributable to infections in 2008: a review and synthetic analysis. Lancet Oncol. 13, 607–615. doi: 10.1016/S1470-2045(12)70137-7
Din, M. O., Danino, T., Prindle, A., Skalak, M., Selimkhanov, J., Allen, K., et al. (2016). Synchronized cycles of bacterial lysis for in vivo delivery. Nature 536, 81–85. doi: 10.1038/nature18930
Dirkx, A. E., Egbrink, M. G. O., Wagstaff, J., and Griffioen, A. W. (2006). Monocyte/macrophage infiltration in tumors: modulators of angiogenesis. J. Leukoc. Biol. 80, 1183–1196. doi: 10.1189/jlb.0905495
Dos Reis, S. A., da Conceição, L. L., Siqueira, N. P., Rosa, D. D., da Silva, L. L., and do Carmo, G. P. M. (2017). Review of the mechanisms of probiotic actions in the prevention of colorectal cancer. Nutr. Res. 37, 1–19. doi: 10.1016/j.nutres.2016.11.009
Etzerodt, A., TsaLkitzi, K., Maniecki, M., Damsky, W., Delfini, M., Baudoin, E., et al. (2019). Specific targeting of CD163(+) TAMs mobilizes inflammatory monocytes and promotes T cell-mediated tumor regression. J. Exp. Med. 216, 2394–2411. doi: 10.1084/jem.20182124
Farrell, J. J., Zhang, L., Zhou, H., Chia, D., Elashoff, D., Akin, D., et al. (2012). Variations of oral microbiota are associated with pancreatic diseases including pancreatic cancer. Gut 61, 582–588. doi: 10.1136/gutjnl-2011-300784
Fass, L. (2008). Imaging and cancer: a review. Mol. Oncol. 2, 115–152. doi: 10.1016/j.molonc.2008.04.001
Flemer, B., Lynch, D. B., Brown, J. M., Jeffery, I. B., Ryan, F. J., Claesson, M. J., et al. (2017). Tumour-associated and non-tumour-associated microbiota in colorectal cancer. Gut 66, 633–643. doi: 10.1136/gutjnl-2015-309595
Ge, Y., Wang, X., Guo, Y., Yan, J., Abuduwaili, A., Aximujiang, K., et al. (2021). Gut microbiota influence tumor development and Alter interactions with the human immune system. J. Experimental & Clin. Cancer Res.: CR 40:42. doi: 10.1186/s13046-021-01845-6
Ghosh, S., and Pramanik, S. (2021). Structural diversity, functional aspects and future therapeutic applications of human gut microbiome. Arch. Microbiol. 203, 5281–5308. doi: 10.1007/s00203-021-02516-y
Harris, P. J., and Ferguson, L. R. (1993). Dietary fibre: its composition and role in protection against colorectal cancer. Mutation Research/Fundamental and Molecular Mechanisms of Mutagenesis 290, 97–110. doi: 10.1016/0027-5107(93)90037-G
Helmink, B., Khan, M., Hermann, A., Gopalakrishnan, V., and Wargo, J. (2019). The microbiome, cancer, and cancer therapy. Nat. Med. 25, 377–388. doi: 10.1038/s41591-019-0377-7
Hijová, E., Bertková, I., and Štofilová, J. (2019). Dietary fibre as prebiotics in nutrition. Cent. Eur. J. Public Health 27, 251–255. doi: 10.21101/cejph.a5313
Hooper, L. V., and Macpherson, A. J. (2010). Immune adaptations that maintain homeostasis with the intestinal microbiota. Nat. Rev. Immunol. 10, 159–169. doi: 10.1038/nri2710
Huang, J., Jiang, Z., Wang, Y., Fan, X., Cai, J., Yao, X., et al. (2020). Modulation of gut microbiota to overcome resistance to immune checkpoint blockade in cancer immunotherapy. Curr. Opin. Pharmacol. 54, 1–10. doi: 10.1016/j.coph.2020.06.004
Huck, O., Al-Hashemi, J., Poidevin, L., Poch, O., Davideau, J.-L., Tenenbaum, H., et al. (2017). Identification and characterization of micro RNA differentially expressed in macrophages exposed to Porphyromonas gingivalis infection. Infect. Immun. 85, e00771–e00716. doi: 10.1128/IAI.00771-16
Huh, J. H., Kittleson, J. T., Arkin, A. P., and Anderson, J. C. (2013). Modular Design of a Synthetic Payload Delivery Device. ACS Synth. Biol. 2, 418–424. doi: 10.1021/sb300107h
Jin, M. Z., and Jin, W. L. (2020). The updated landscape of tumor microenvironment and drug repurposing. Signal Transduc. Tar. Ther. 5:166. doi: 10.1038/s41392-020-00280-x
Kaimala, S., Al-Sbiei, A., Cabral-Marques, O., Fernandez-Cabezudo, M. J., and Al-Ramadi, B. K. (2018). Attenuated Bacteria as immunotherapeutic tools for Cancer treatment. Front. Oncol. 8:136. doi: 10.3389/fonc.2018.00136
Kalaora, S., Nagler, A., and Wargo, J. A. (2022). Mechanisms of immune activation and regulation: Lessons from melanoma. Nat. Rev. Cancer. 22, 195–207.
Kang, Y. B., and Cai, Y. (2021). Faecal microbiota transplantation enhances efficacy of immune checkpoint inhibitors therapy against cancer. World J. Gastroenterol. 27, 5362–5375. doi: 10.3748/wjg.v27.i32.5362
Karki, R., and Kanneganti, T.D., Diverging inflammasome signals in tumorigenesis and potential targeting. 19 (2019). 197–214 Washington, DC: American Society For Microbiology.
Kim, P. K., Halbrook, C. J., Kerk, S. A., Radyk, M., Wisner, S., Kremer, D. M., et al. (2021). Hyaluronic acid fuels pancreatic cancer cell growth. elife 10:645. doi: 10.7554/eLife.62645
Kim, J., and Lee, H. K. (2021). Potential role of the gut microbiome in colorectal Cancer progression. Front. Immunol. 12:807648. doi: 10.3389/fimmu.2021.807648
Kim, O. Y., Park, H. T., Dinh, N. T. H., Choi, S. J., Lee, J., Kim, J. H., et al. (2017). Bacterial outer membrane vesicles suppress tumor by interferon-γ-mediated antitumor response. Nat. Commun. 8:626. doi: 10.1038/s41467-017-00729-8
Kim, J., and Wang, T. C. (2021). Helicobacter pylori and gastric Cancer. Gastrointest. Endosc. Clin. N. Am. 31, 451–465. doi: 10.1016/j.giec.2021.03.003
Kise, K., Kinugasa-Katayama, Y., and Takakura, N. (2016). Tumor microenvironment for cancer stem cells. Adv. Drug Deliv. Rev. 99, 197–205. doi: 10.1016/j.addr.2015.08.005
Kitada, T., DiAndreth, B., Teague, B., and Weiss, R. (2018). Programming gene and engineered-cell therapies with synthetic biology. Science 359:eaad1067. doi: 10.1126/science.aad1067
Klein, R. S., Recco, R. A., Catalano, M. T., Edberg, S. C., Casey, J. I., and Steigbigel, N. H. (1977). Association of Streptococcus bovis with carcinoma of the Colon. N. Engl. J. Med. 297, 800–802. doi: 10.1056/NEJM197710132971503
Kovács, T., Mikó, E., Ujlaki, G., Sári, Z., and Bai, P. (2020). The microbiome as a component of the tumor microenvironment. Tumor Microenviron. 1225, 137–153. doi: 10.1007/978-3-030-35727-6_10
Kramer, M. G., Masner, M., Ferreira, F. A., and Hoffman, R. M. (2018). Bacterial therapy of Cancer: promises, limitations, and insights for future directions. Front. Microbiol. 9. doi: 10.3389/fmicb.2018.00016
Kroemer, G., and Zitvogel, L. (2020). Inosine: novel microbiota-derived immunostimulatory metabolite. Cell Res. 30, 942–943. doi: 10.1038/s41422-020-00417-1
Kumar, A., Ali, A., Kapardar, R. K., Dar, G. M., Nimisha, A., Sharma, A. K., et al. (2023). Implication of gut microbes and its metabolites in colorectal cancer. J. Cancer Res. Clin. Oncol. 149, 441–465. doi: 10.1007/s00432-022-04422-2
Lakritz, J. R., Poutahidis, T., Levkovich, T., Varian, B. J., Ibrahim, Y. M., Chatzigiagkos, A., et al. (2014). Beneficial bacteria stimulate host immune cells to counteract dietary and genetic predisposition to mammary cancer in mice. Int. J. Cancer 135, 529–540. doi: 10.1002/ijc.28702
Lankadasari, M. B., Mukhopadhyay, P., Mohammed, S., and Harikumar, K. B. (2019). TAMing pancreatic cancer: Combat with a double edged sword. Mol. Cancer. 18, 48.
Larionova, I., Kazakova, E., Gerashchenko, T., and Kzhyshkowska, J. (2021). New angiogenic regulators produced by TAMs: perspective for targeting tumor angiogenesis. Cancer 13:3253. doi: 10.3390/cancers13133253
Lee-Chang, C., Rashidi, A., Miska, J., Zhang, P., Pituch, K. C., Hou, D., et al. (2019). Myeloid-derived suppressive cells promote B cell–mediated immunosuppression via transfer of PD-L1 in GlioblastomaIdentification of regulatory B cells in GBM. Cancer Immunol. Res. 7, 1928–1943. doi: 10.1158/2326-6066.CIR-19-0240
Legesse Bedada, T., Feto, T. K., Awoke, K. S., Garedew, A. D., Yifat, F. T., and Birri, D. J. (2020). Probiotics for cancer alternative prevention and treatment. Biomed. Pharmacother. 129:110409. doi: 10.1016/j.biopha.2020.110409
Leppäranta, O., Vaahtio, M., Peltola, T., Zhang, D., Hupa, L., Hupa, M., et al. (2008). Antibacterial effect of bioactive glasses on clinically important anaerobic bacteria in vitro. J. Mater. Sci. Mater. Med. 19, 547–551. doi: 10.1007/s10856-007-3018-5
Leventhal, D., West, K., Fisher, A., Sokolovska, A., Kolodziej, S., Li, N., et al. (2018). Abstract LB-131: activation of innate and adaptive immunity via combinatorial immunotherapy using synthetic biotic<sup>™</sup> medicines. Cancer Res. 78:LB-131. doi: 10.1158/1538-7445.AM2018-LB-131
Liu, L., Bai, Y., Xiang, L., Qi, W., Gao, L., Li, X., et al. (2022). Interaction between gut microbiota and tumour chemotherapy. Clin. Transl. Oncol. 24, 2330–2341. doi: 10.1007/s12094-022-02919-3
Lu, K., Dong, S., Wu, X., Jin, R., and Chen, H. (2021). Probiotics in Cancer. Front. Oncol. 11:148. doi: 10.3389/fonc.2021.638148
Ma, W., Mao, Q., Xia, W., Dong, G., Yu, C., and Jiang, F. (2019). Gut microbiota shapes the efficiency of Cancer therapy. Front. Microbiol. 10. doi: 10.3389/fmicb.2019.01050
Mager, L. F., and Burkhard, R. (2020). Microbiome-derived inosine modulates response to checkpoint inhibitor immunotherapy. Sci. 369, 1481–1489.
Mager, L. F., Burkhard, R., Pett, N., Cooke, N. C., Brown, K., Ramay, H., et al. (2020). Microbiome-derived inosine modulates response to checkpoint inhibitor immunotherapy. Science 369, 1481–1489. doi: 10.1126/science.abc3421
Markowiak, P., and Śliżewska, K. (2017). Effects of probiotics, prebiotics, and Synbiotics on human health. Nutrients 9:1021. doi: 10.3390/nu9091021
Marsland, B. J., Trompette, A., and Gollwitzer, E. S. (2015). The gut-lung Axis in respiratory disease. Ann. Am. Thorac. Soc. 12, S150–S156. doi: 10.1513/AnnalsATS.201503-133AW
Matson, V., Chervin, C. S., and Gajewski, T. F. (2021). Cancer and the microbiome-influence of the commensal microbiota on Cancer, immune responses, and immunotherapy. Gastroenterology 160, 600–613. doi: 10.1053/j.gastro.2020.11.041
Mattheolabakis, G., Milane, L., Singh, A., and Amiji, M. M. (2015). Hyaluronic acid targeting of CD44 for cancer therapy: from receptor biology to nanomedicine. J. Drug Target. 23, 605–618. doi: 10.3109/1061186X.2015.1052072
Matysiak-Budnik, T., and Mégraud, F. (2006). Helicobacter pylori infection and gastric cancer. Eur. J. Cancer 42, 708–716. doi: 10.1016/j.ejca.2006.01.020
Maynard, C. L., Elson, C. O., Hatton, R. D., and Weaver, C. T. (2012). Reciprocal interactions of the intestinal microbiota and immune system. Nature 489, 231–241. doi: 10.1038/nature11551
McQuade, J. L., Daniel, C. R., Helmink, B. A., and Wargo, J. A. (2019). Modulating the microbiome to improve therapeutic response in cancer. Lancet Oncol. 20, e77–e91. doi: 10.1016/S1470-2045(18)30952-5
Meng, C., Bai, C., Brown, T. D., Hood, L. E., and Tian, Q. (2018). Human gut microbiota and gastrointestinal cancer. Genomics Proteomics Bioinfo. 16, 33–49. doi: 10.1016/j.gpb.2017.06.002
Merlo, L. M. F., Pepper, J. W., Reid, B. J., and Maley, C. C. (2006). Cancer as an evolutionary and ecological process. Nat. Rev. Cancer 6, 924–935. doi: 10.1038/nrc2013
Min, H., Yao, J., Allinen, M., Cai, L., and Polyak, K. (2005). The role of the tumor microenvironment in breast cancer progression. Breast Cancer Res. 7:1. doi: 10.1186/bcr1060
Moniri, N. H., and Farah, Q. (2021). Short-chain free-fatty acid G protein-coupled receptors in colon cancer. Biochem. Pharmacol. 186:114483. doi: 10.1016/j.bcp.2021.114483
Mudgil, D., and Barak, S. (2013). Composition, properties and health benefits of indigestible carbohydrate polymers as dietary fiber: a review. Int. J. Biol. Macromol. 61, 1–6. doi: 10.1016/j.ijbiomac.2013.06.044
Nejman, D., and Livyatan, I. (2020). The human tumor microbiome is composed of tumor type-specific intracellular bacteria. Sci. 368, 973–980.
O'Hara, A. M., and Shanahan, F. (2006). The gut flora as a forgotten organ. EMBO Rep. 7, 688–693. doi: 10.1038/sj.embor.7400731
Omatsu, M., Kunimura, T., Mikogami, T., Shiokawa, A., Nagai, T., Masunaga, A., et al. (2014). Difference in distribution profiles between CD163+ tumor-associated macrophages and S100+ dendritic cells in thymic epithelial tumors. Diagn. Pathol. 9:215. doi: 10.1186/s13000-014-0215-7
Özdemir, B. C., Pentcheva-Hoang, T., Carstens, J. L., Zheng, X., Wu, C.-C., Simpson, T. R., et al. (2014). Depletion of carcinoma-associated fibroblasts and fibrosis induces immunosuppression and accelerates pancreas Cancer with reduced survival. Cancer Cell 25, 719–734. doi: 10.1016/j.ccr.2014.04.005
Panebianco, C., Latiano, T., and Pazienza, V. (2020). Microbiota manipulation by probiotics administration as emerging tool in Cancer prevention and therapy. Front. Oncol. 10. doi: 10.3389/fonc.2020.00679
Panteli, J. T., Forkus, B. A., Van Dessel, N., and Forbes, N. S. (2015). Genetically modified bacteria as a tool to detect microscopic solid tumor masses with triggered release of a recombinant biomarker. Integr. Biol. 7, 423–434. doi: 10.1039/c5ib00047e
Panteli, J. T., Van Dessel, N., and Forbes, N. S. (2020). Detection of tumors with fluoromarker-releasing bacteria. Int. J. Cancer 146, 137–149. doi: 10.1002/ijc.32414
Parada Venegas, D., De la Fuente, M. K., Landskron, G., González, M. J., Quera, R., Dijkstra, G., et al. (2019). Short chain fatty acids (SCFAs)-mediated gut epithelial and immune regulation and its relevance for inflammatory bowel diseases. Front. Immunol. 10. doi: 10.3389/fimmu.2019.01486
Park, J., Kim, M., Kang, S. G., Jannasch, A. H., Cooper, B., Patterson, J., et al. (2015). Short-chain fatty acids induce both effector and regulatory T cells by suppression of histone deacetylases and regulation of the mTOR–S6K pathway. Mucosal Immunol. 8, 80–93. doi: 10.1038/mi.2014.44
Parsonnet, J. (1993). Helicobacter pylori and gastric cancer. Gastroenterol. Clin. N. Am. 22, 89–104. doi: 10.1016/S0889-8553(21)00265-X
Payne, C. M., Weber, C., Crowley-Skillicorn, C., Dvorak, K., Bernstein, H., Bernstein, C., et al. (2007). Deoxycholate induces mitochondrial oxidative stress and activates NF-κB through multiple mechanisms in HCT-116 colon epithelial cells. Carcinogenesis 28, 215–222. doi: 10.1093/carcin/bgl139
Péré-Védrenne, C., Cardinaud, B., Varon, C., Mocan, I., Buissonnière, A., Izotte, J., et al. (2016). The cytolethal distending toxin subunit CdtB of Helicobacter induces a Th17-related and antimicrobial signature in intestinal and hepatic cells in vitro. J. Infect. Dis. 213, 1979–1989. doi: 10.1093/infdis/jiw042
Pickup, M., Mouw, J., and Weaver, V. (2014). The extracellular matrix modulates the hallmarks of cancer. EMBO Rep. 15, 1243–1253. doi: 10.15252/embr.201439246
Plummer, M., de Martel, C., Vignat, J., Ferlay, J., Bray, F., and Franceschi, S. (2016). Global burden of cancers attributable to infections in 2012: a synthetic analysis. Lancet Glob. Health 4, e609–e616. doi: 10.1016/S2214-109X(16)30143-7
Pokharel, S. S., Macura, K. J., Kamel, I. R., and Zaheer, A. (2013). Current MR imaging lipid detection techniques for diagnosis of lesions in the abdomen and pelvis. Radio Graphics 33, 681–702. doi: 10.1148/rg.333125068
Poutahidis, T., Kearney, S. M., Levkovich, T., Qi, P., Varian, B. J., Lakritz, J. R., et al. (2013). Microbial symbionts accelerate wound healing via the neuropeptide hormone oxytocin. PLoS One 8:e78898. doi: 10.1371/journal.pone.0078898
Provenzano, P. P., Cuevas, C., Chang, A. E., Goel, V. K., Von Hoff, D. D., and Hingorani, S. R. (2012). Enzymatic targeting of the stroma ablates physical barriers to treatment of pancreatic ductal adenocarcinoma. Cancer Cell 21, 418–429. doi: 10.1016/j.ccr.2012.01.007
Rahman, M. M., Islam, M. R., Shohag, S., Ahasan, M. T., Sarkar, N., Khan, H., et al. (2022). Microbiome in cancer: role in carcinogenesis and impact in therapeutic strategies. Biomed. Pharmacother. 149:112898. doi: 10.1016/j.biopha.2022.112898
Raman, M., Ambalam, P., Kondepudi, K. K., Pithva, S., Kothari, C., Patel, A. T., et al. (2013). Potential of probiotics, prebiotics and synbiotics for management of colorectal cancer. Gut Microbes 4, 181–192. doi: 10.4161/gmic.23919
Ribet, D., and Cossart, P. (2015). How bacterial pathogens colonize their hosts and invade deeper tissues. Microbes Infect. 17, 173–183. doi: 10.1016/j.micinf.2015.01.004
Rooks, M. G., and Garrett, W. S. (2016). Gut microbiota, metabolites and host immunity. Nat. Rev. Immunol. 16, 341–352. doi: 10.1038/nri.2016.42
Rowland, I., Gibson, G., Heinken, A., Scott, K., Swann, J., Thiele, I., et al. (2018). Gut microbiota functions: metabolism of nutrients and other food components. Eur. J. Nutr. 57, 1–24. doi: 10.1007/s00394-017-1445-8
Roy, S., and Trinchieri, G. (2017). Microbiota: a key orchestrator of cancer therapy. Nat. Rev. Cancer 17, 271–285. doi: 10.1038/nrc.2017.13
Rubinstein, M. R., Wang, X., Liu, W., Hao, Y., Cai, G., and Han, Y. W. (2013). Fusobacterium nucleatum promotes colorectal carcinogenesis by modulating E-cadherin/β-catenin signaling via its FadA Adhesin. Cell Host Microbe 14, 195–206. doi: 10.1016/j.chom.2013.07.012
Schulz, M., Salamero-Boix, A., Niesel, K., Alekseeva, T., and Sevenich, L. (2019). Microenvironmental regulation of tumor progression and therapeutic response in brain metastasis. Front. Immunol. 10:1713. doi: 10.3389/fimmu.2019.01713
Schwabe, R. F., and Jobin, C. (2013). The microbiome and cancer. Nat. Rev. Cancer 13, 800–812. doi: 10.1038/nrc3610
Sears, C. L., and Garrett, W. S. (2014). Microbes, microbiota, and colon cancer. Cell Host Microbe 15, 317–328. doi: 10.1016/j.chom.2014.02.007
Sender, R., Fuchs, S., and Milo, R. (2016). Are we really vastly outnumbered? Revisiting the ratio of bacterial to host cells in humans. Cell 164, 337–340. doi: 10.1016/j.cell.2016.01.013
Sepich-Poore, G. D., Zitvogel, L., Straussman, R., Hasty, J., Wargo, J. A., and Knight, R. (2021). The microbiome and human cancer. Science 371:552. doi: 10.1126/science.abc4552
Shamekhi, S., Lotfi, H., Abdolalizadeh, J., Bonabi, E., and Zarghami, N. (2020). An overview of yeast probiotics as cancer biotherapeutics: Possible clinical application in colorectal cancer. Clin. Transl. Oncol. 22, 1227–1239.
Sharma, P., and Allison, J. P. (2015). The future of immune checkpoint therapy. Science 348:56. doi: 10.1126/science.aaa8172
Shi, N., Li, N., Duan, X., and Niu, H. (2017). Interaction between the gut microbiome and mucosal immune system. Mil. Med. Res. 4:14. doi: 10.1186/s40779-017-0122-9
Shi, L., Yu, B., Cai, C.-H., and Huang, J.-D. (2016). Angiogenic inhibitors delivered by the type III secretion system of tumor-targeting Salmonella typhimurium safely shrink tumors in mice. AMB Express 6, 1–10. doi: 10.1186/s13568-016-0226-8
Siegel, R. L., Miller, K. D., and Jemal, A. (2019). Cancer statistics. CA Cancer J. Clin. 69, 7–34. doi: 10.3322/caac.21551
Singh, A. P., Arora, S., Bhardwaj, A., Srivastava, S. K., Kadakia, M. P., Wang, B., et al. (2012). CXCL12/CXCR4 protein signaling Axis induces sonic hedgehog expression in pancreatic Cancer cells via extracellular regulated kinase-and Akt kinase-mediated activation of nuclear factor κB: IMPLICATIONS FOR BIDIRECTIONAL TUMOR-STROMAL INTERACTIONS*. J. Biol. Chem. 287, 39115–39124. doi: 10.1074/jbc.M112.409581
Singh, N., Gurav, A., Sivaprakasam, S., Brady, E., Padia, R., Shi, H., et al. (2014). Activation of Gpr109a, receptor for niacin and the commensal metabolite butyrate, suppresses colonic inflammation and carcinogenesis. Immunity 40, 128–139. doi: 10.1016/j.immuni.2013.12.007
Sipe, L. M., Chaib, M., Pingili, A. K., Pierre, J. F., and Makowski, L. (2020). Microbiome, bile acids, and obesity: how microbially modified metabolites shape anti-tumor immunity. Immunol. Rev. 295, 220–239. doi: 10.1111/imr.12856
Siska, P. J., and Rathmell, J. C. (2015). T cell metabolic fitness in antitumor immunity. Trends Immunol. 36, 257–264. doi: 10.1016/j.it.2015.02.007
Sivan, A., Corrales, L., Hubert, N., Williams, J. B., Aquino-Michaels, K., Earley, Z. M., et al. (2015). Commensal Bifidobacterium promotes antitumor immunity and facilitates anti–PD-L1 efficacy. Science 350, 1084–1089. doi: 10.1126/science.aac4255
Tan, X., and Johnson, S. (2019). Fecal microbiota transplantation (FMT) for C. difficile infection, just say ‘no’. Anaerobe 60:102092. doi: 10.1016/j.anaerobe.2019.102092
Tsoi, H., Chu, E. S. H., Zhang, X., Sheng, J., Nakatsu, G., Ng, S. C., et al. (2017). Peptostreptococcus anaerobius induces intracellular cholesterol biosynthesis in Colon cells to induce proliferation and causes dysplasia in mice. Gastroenterology 152, 1419–1433.e5. doi: 10.1053/j.gastro.2017.01.009
Turroni, F., Ribbera, A., Foroni, E., van Sinderen, D., and Ventura, M. (2008). Human gut microbiota and bifidobacteria: from composition to functionality. Antonie Van Leeuwenhoek 94, 35–50. doi: 10.1007/s10482-008-9232-4
Ulger Toprak, N., Yagci, A., Gulluoglu, B. M., Akin, M. L., Demirkalem, P., Celenk, T., et al. (2006). A possible role of Bacteroides fragilis enterotoxin in the aetiology of colorectal cancer. Clin. Microbiol. Infect. 12, 782–786. doi: 10.1111/j.1469-0691.2006.01494.x
Vétizou, M., Pitt, J. M., Daillère, R., Lepage, P., Waldschmitt, N., Flament, C., et al. (2015). Anticancer immunotherapy by CTLA-4 blockade relies on the gut microbiota. Science 350, 1079–1084. doi: 10.1126/science.aad1329
Wang, F., Meng, W., Wang, B., and Qiao, L. (2014). Helicobacter pylori-induced gastric inflammation and gastric cancer. Cancer Lett. 345, 196–202. doi: 10.1016/j.canlet.2013.08.016
Wang, Y., Mumm, J. B., and Herbst, R. (2017). IL-22 increases permeability of intestinal epithelial tight junctions by enhancing Claudin-2 expression, J. Immunology. 199, 3316–3325.
West, K. A., Fisher, A., Leventhal, D., Sokolovska, A., Li, N., Plescia, C., et al. (2018). Abstract 2920: metabolic modulation of the tumor microenvironment using synthetic biotic™ medicines. Cancer Res. 78:2920. doi: 10.1158/1538-7445.AM2018-2920
Whisner, C. M., and Athena Aktipis, C. (2019). The role of the microbiome in Cancer initiation and progression: how microbes and Cancer cells utilize excess energy and promote one Another’s growth. Current Nutrit. Reports 8, 42–51. doi: 10.1007/s13668-019-0257-2
Wilson, M. R., Jiang, Y., Villalta, P. W., Stornetta, A., Boudreau, P. D., Carrá, A., et al. (2019). The human gut bacterial genotoxin colibactin alkylates DNA. Science 363:7785. doi: 10.1126/science.aar7785
Xiang, X., Wang, J., Lu, D., and Xu, X. (2021). Targeting tumor-associated macrophages to synergize tumor immunotherapy. Signal Transduct. Target. Ther. 6:75. doi: 10.1038/s41392-021-00484-9
Xiao, Y., and Yu, D. (2021). Tumor microenvironment as a therapeutic target in cancer. Pharmacol. Ther. 221:107753. doi: 10.1016/j.pharmthera.2020.107753
Yamamura, K., Izumi, D., Kandimalla, R., Sonohara, F., Baba, Y., Yoshida, N., et al. (2019). Intratumoral <em>fusobacterium Nucleatum</em> levels predict therapeutic response to neoadjuvant chemotherapy in esophageal squamous cell carcinoma. Clin. Cancer Res. 25:6170. doi: 10.1158/1078-0432.CCR-19-0318
Yan, S., and Wan, G. (2021). Tumor-associated macrophages in immunotherapy. FEBS J. 288, 6174–6186. doi: 10.1111/febs.15726
Yan, X., Yang, M., Liu, J., Gao, R., Hu, J., Li, J., et al. (2015). Discovery and validation of potential bacterial biomarkers for lung cancer. Am. J. Cancer Res. 5, 3111–3122.
Yang, X., Lin, Y., Shi, Y., Li, B., Liu, W., Yin, W., et al. (2016). FAP promotes immunosuppression by Cancer-associated fibroblasts in the tumor microenvironment via STAT3–CCL2 SignalingFAP via STAT3–CCL2 promote tumor immunosuppression. Cancer Res. 76, 4124–4135. doi: 10.1158/0008-5472.CAN-15-2973
Yang, M., Ma, B., Shao, H., Clark, A. M., and Wells, A. (2016). Macrophage phenotypic subtypes diametrically regulate epithelial-mesenchymal plasticity in breast cancer cells. BMC Cancer 16:419. doi: 10.1186/s12885-016-2411-1
Yao, Y., Cai, X., Fei, W., Ye, Y., Zhao, M., and Zheng, C. (2022). The role of short-chain fatty acids in immunity, inflammation and metabolism. Crit. Rev. Food Sci. Nutr. 62, 1–12. doi: 10.1080/10408398.2020.1854675
Zaramela, L. S., Moyne, O., Kumar, M., Zuniga, C., Tibocha-Bonilla, J. D., and Zengler, K. (2021). The sum is greater than the parts: exploiting microbial communities to achieve complex functions. Curr. Opin. Biotechnol. 67, 149–157. doi: 10.1016/j.copbio.2021.01.013
Zhang, P.-P., Li, L.-L., Han, X., Li, Q.-W., Zhang, X.-H., Liu, J. J., et al. (2020). Fecal microbiota transplantation improves metabolism and gut microbiome composition in db/db mice. Acta Pharmacol. Sin. 41, 678–685. doi: 10.1038/s41401-019-0330-9
Zhang, C., Zhao, Y., Jiang, J., Yu, L., Tian, F., Zhao, J., et al. (2021). Identification of the key characteristics of Bifidobacterium longum strains for the alleviation of ulcerative colitis. Food Funct. 12, 3476–3492. doi: 10.1039/D1FO00017A
Zhou, C. B., Zhou, Y. L., and Fang, J. Y. (2021). Gut microbiota in Cancer immune response and immunotherapy. Trends Cancer 7, 647–660. doi: 10.1016/j.trecan.2021.01.010
Zhu, R., Lang, T., Yan, W., Zhu, X., Huang, X., Yin, Q., et al. (2021). Gut microbiota: Influence on carcinogenesis and modulation strategies by drug delivery systems to improve Cancer therapy. Adv. Sci. 8, 2003542.
Keywords: cancer therapy, gut microbiota, microbiota tumor microenvironment, synthetic biology, therapeutic target
Citation: Feng P, Xue X, Bukhari I, Qiu C, Li Y, Zheng P and Mi Y (2024) Gut microbiota and its therapeutic implications in tumor microenvironment interactions. Front. Microbiol. 15:1287077. doi: 10.3389/fmicb.2024.1287077
Edited by:
William K. K. Wu, The Chinese University of Hong Kong, ChinaReviewed by:
Yuting Ke, Massachusetts Institute of Technology, United StatesJesús Adonai Maguey-González, National Autonomous University of Mexico, Mexico
Gaofeng Wu, Xi’an Jiaotong University, China
Aman Khan, Lanzhou University, China
Copyright © 2024 Feng, Xue, Bukhari, Qiu, Li, Zheng and Mi. This is an open-access article distributed under the terms of the Creative Commons Attribution License (CC BY). The use, distribution or reproduction in other forums is permitted, provided the original author(s) and the copyright owner(s) are credited and that the original publication in this journal is cited, in accordance with accepted academic practice. No use, distribution or reproduction is permitted which does not comply with these terms.
*Correspondence: Pengyuan Zheng, cHl6aGVuZ0B6enUuZWR1LmNu; Yang Mi, eWFuZ21pMTk4QHp6dS5lZHUuY24=
†These authors have contributed equally to this work