- Department of Microbiology and Plant Pathology, University of California, Riverside, Riverside, CA, United States
The horizontal transfer of mobile genetic elements (MGEs) is an essential process determining the functional and genomic diversity of bacterial populations. MGEs facilitate the exchange of fitness determinant genes like antibiotic resistance and virulence factors. Various computational methods exist to identify potential MGEs, but confirming their ability to transfer requires additional experimental approaches. Here, we apply a transposon (Tn) mutagenesis technique for confirming mobilization without the need for targeted mutations. Using this method, we identified two MGEs, including a previously known conjugative transposon (CTn) called BoCTn found in Bacteroides ovatus and a novel CTn, PvCTn, identified in Phocaeicola vulgatus. In addition, Tn mutagenesis and subsequent genetic deletion enabled our characterization of a helix-turn-helix motif gene, BVU3433 which negatively regulates the conjugation efficiency of PvCTn in vitro. Furthermore, our transcriptomics data revealed that BVU3433 plays a crucial role in the repression of PvCTn genes, including genes involved in forming complete conjugation machinery [Type IV Secretion System (T4SS)]. Finally, analysis of individual strain genomes and community metagenomes identified the widespread prevalence of PvCTn-like elements with putative BVU3433 homologs among human gut-associated bacteria. In summary, this Tn mutagenesis mobilization method (TMMM) enables observation of transfer events in vitro and can ultimately be applied in vivo to identify a broader diversity of functional MGEs that may underly the transfer of important fitness determinants.
1 Introduction
Mobile genetic elements (MGEs) are important drivers of bacterial evolution by promoting gene acquisitions that can profoundly affect a bacterial host’s fitness. Known fitness determinants that can be mobilized include those that enable evasion of host immune responses (Rendueles et al., 2018) and antibiotics (Partridge et al., 2018), as well as the ability to intoxicate hosts (Schmidt et al., 1999) and acquire scarce resources in competitive environments (Frye et al., 2021). Furthermore, sequence evidence indicates that extensive interspecies transfer of MGEs among the Bacteroidota, a Gram-negative phylum of bacteria (formerly Bacteroidetes) that is common in the human gut and can represent as much as 80% of the microbiome of some individuals (Wexler, 2007; Smillie et al., 2010; Human Microbiome Project Consortium (HMP), 2012; Coyne et al., 2014; Nayfach et al., 2019). As a result, computational methods to identify, classify, and determine the prevalence of potential MGE activity from DNA sequences have become increasingly sophisticated (Akhter et al., 2012; Ozer et al., 2014; Roux et al., 2015; Johansson et al., 2020). However, given the structural and sequence diversity of MGEs (Osborn and Böltner, 2002), the possibility of inactivating mutations and the presence of cooperative mobilizing elements (Duerkop et al., 2012), confirming the activity of a computationally predicted MGEs generally requires experimental investigation.
Many confirmed MGEs in Bacteroidota have obvious phenotypes that readily enabled the characterization of their functions (e.g., antibiotic resistance) (Schlesinger et al., 2007; Waters and Salyers, 2013; Wood and Gardner, 2015). In contrast, many predicted MGEs lack evidence for obvious functions that would be amenable to a genetic screen (Durrant et al., 2020). Further, the number of predicted MGEs per genome can be quite high, where among Bacteroides spp. 20–50% have at least one plasmid (Wexler, 2007) and 80% encode at least one conjugative transposon (CTn; Shoemaker et al., 2001). As such, strategies that avoid 10s or 100 s of targeted mutations to enable screening for mobilization have the potential to accelerate experimental validation of MGE activity and functions.
One such attempt to implement an untargeted approach to identify functional MGEs in Enterobacteriaceae was developed by Tansirichaiya et al. (2022). The authors constructed a type of entrapment vector (Gay et al., 1985), pBACpAK, that expresses its tetracycline resistance (TetR) allele when a cI repressor gene is disrupted by a MGE (Tansirichaiya et al., 2022). This approach enabled the detection of MGEs integrating into a focal species, or smaller elements replicating within the focal species (e.g., IS elements). However, this system is limited to MGEs that can integrate into the ~0.6 kb cI repressor gene and to species within the Enterobacteriaceae. These limitations suggest the opportunity for additional untargeted approaches to identify and track functional MGEs. Transposon (Tn) mutagenesis is a commonly used method to generate untargeted genomic mutations and characterize gene functions among all domains of life (Lampe et al., 1996; Kiljunen et al., 2017). We propose combining Tn mutagenesis with screens for horizontal gene transfer (HGT) in bacteria to efficiently assess MGE activity.
Here we evaluate the effectiveness of capturing MGEs by combining mariner Tn mutagenesis and HGT screens for a group of human gut-associated bacteria. Through this untargeted Tn mutagenesis mobilization method (TMMM), we successfully tracked the mobilization of two conjugative transposons (CTns), including a novel CTn in P. vulgatus ATCC 8482 that we have named PvCTn. Further, this work provides insight into the regulatory mechanisms of PvCTn, most notably the helix-turn-helix motif encoding gene BVU3433. We also provide computational evidence for the prevalence of PvCTn-like elements among a panel of human gut-associated bacterial genomes and metagenomes from globally distributed patient samples. The observed mobilization of PvCTn and the widespread presence of genetically diverse PvCTn-like elements in Bacteroidota reinforce the importance of MGEs shaping the human microbiome.
2 Materials and methods
2.1 Strains, plasmids, culture conditions
All Bacteroidota cultures were grown on Brain Heart Infusion (BHI) agar supplemented with 10% defibrinated horse blood (BHI-HB; Quad Five, Ryegate, MT) and tryptone-yeast extract-glucose broth (TYG) medium with and without agar using an anaerobic chamber (Coy Laboratory Products, Grass Lake, MI) filled with 73% N2, 20% CO2, and 7% H2. Escherichia coli with the appropriate plasmid(s) (pSAM, pNBU2, pExchange, pLGB13) were grown on LB. All strains and plasmids are listed in Supplementary Table S1. Ampicillin (100 μg/mL), gentamicin (200 μg/mL), erythromycin (25 μg/mL), tetracycline (2 μg/mL), cefoxitin (20 μg/mL), 5′-fluorodeoxyuridine (FUdR; 20 μg/mL), and anhydrotetracycline (aTC; 100 ng/mL) were added to the media when appropriate.
During conjugations, donors with conjugative plasmids (pNBU2, pExchange, pLGB13) or conjugative transposons (PvCTn) and Bacteroidota recipients were grown overnight in 5 mL LB and TYG medium, respectively, with the appropriate antibiotic(s). Overnight stationary phase E. coli strains were then used to inoculate 10 mL subcultures of LB at the following dilutions: 1:200, 1:500, and 1:750 dilutions. Bacteroides thetaiotaomicron, B. ovatus, and P. vulgatus strains were similarly inoculated into TYG but at lower dilutions: 1:25, 1:50, and 1:100. Subcultured E. coli S-17 were shaken aerobically at 250 rpm at 37°C. Bacteroidota were subcultured in an anaerobic chamber, stoppered, removed from the chamber, and incubated statically at 37°C. Growth was monitored and cells were pelleted (4,000 ×g for 5 min) when they reached an OD600 of ~0.4. The supernatants were removed, and cells were resuspended with 1 mL TYG medium and pelleted again. After removing the supernatant, 1 mL TYG medium was used to combine donors and recipients. The combined cells were then spread onto BHI-HB agar. Conjugation plates with E. coli donors were incubated aerobically, while dual-Bacteroidota conjugations were incubated anaerobically. Both aerobic and anaerobic conjugations were incubated at 37°C for 24 h. Conjugation masses were then scraped and resuspended in 5 mL TYG medium. The resuspended conjugation masses were then plated onto LB or BHI-HB agar with serial 10-fold dilutions and antibiotic supplement(s). Conjugation efficiencies were calculated using the following equation:
2.2 Effects of peroxide stress on conjugation
Conjugation experiments were performed as described above, however, once donor and recipient Bacteroidota cells were mixed and pelleted after reaching an OD600 of ~0.4, the cells were plated on TYG agar where 880 μM H2O2 was surface spread 30 min before plating when appropriate. The resuspended conjugation masses were plated on BHI-HB agar with serial 10-fold dilutions and antibiotic supplement(s). Conjugation efficiencies were calculated as described above.
2.3 Transposon mutagenesis based identification of MGEs
2.3.1 Generating transposon (Tn) mutant libraries
Bacteroides thetaiotaomicron, P. vulgatus, and B. ovatus were mutagenized through conjugation with E. coli S17-1 containing the sequencing-adapted mariner transposon plasmid (pSAM) containing an erythromycin resistance cassette (Goodman et al., 2009). Conjugations were carried out as above, however, 100 μL aliquots of the entire conjugation masses were plated on 50 BHI-HB plates supplemented with erythromycin and incubated anaerobically at 37°C for 48 h. Colonies were collected and pooled in TYG + 20% glycerol and stored at −80°C in 500 μL aliquots.
2.3.2 Screening Tn libraries for MGEs
Aliquots of Tn mutant library donors (ErmR) were then conjugated with TetR recipient strains of B. thetaiotaomicron VPI-5482, B. thetaiotaomicron 3731, Parabacteroides merdae ATCC 43184, B. thetaiotaomicron VPI-5482 ∆CPS, and B. uniformis ATCC 8492. To screen for MGE transfers, the conjugation masses were plated onto BHI-HB supplemented with tetracycline and erythromycin. After incubation for 48 h at 37°C, colonies were re-struck for colony purification of the putative transductants or transconjugants. Individual colonies were then grown overnight in liquid TYG supplemented with tetracycline and erythromycin at 37°C for 24 h.
2.3.3 Arbitrary PCR
To map pSAM’s integration sites, arbitrary PCR assay was used as described previously (Goodman et al., 2009). Amplicons were cleaned using a QIAquick PCR Purification Kit (Qiagen, Hilden, Germany) and submitted for Sanger sequencing through the University of California, Riverside Institute for Integrative Genomic Biology (UCR IIGB) core. The sequences were then used as BLASTn search queries against the B. thetaiotaomicron (Refseq:NC_004663), B. ovatus (Refseq:NZ_AAXF00000000), and P. vulgatus (Refseq:NC_009614) reference genomes.
2.4 Cloning and mutagenesis (pExchange, pLGB13, pNBU2)
Multiple vector systems were employed to generate the required strains. First, deletion of BVU3433 mutants was achieved using the P. vulgatus specific thymidine kinase (tdk) allelic exchange system (Campbell et al., 2020). Briefly, the ~1kbp regions flanking BVU3433 were individually amplified, then combined and amplified in the splicing by overlap extension (SOE) reaction. All products were amplified using KAPA HiFi Taq MasterMix (KAPA BIOsystems, Wilmington, MA) with the primers listed in Supplementary Table S1. The purified SOE product was then subjected to restriction digestion (NEB) and ligated into pExchange-tdkBV using T4 DNA Ligase (NEB).
Second, targeted insertional mutations of tetracycline resistance (tetQ) was achieved with pLGB13 which uses erythromycin-aTC counter selection (García-Bayona and Comstock, 2019). We generated a ~ 2 kb SOE product encompassing the intergenic spacer between two co-transcribed hypothetical proteins BVU3417 and BVU3418. However, at the SOE junction within this intergenic spacer, we included a SpeI and a XmaI restriction sites (Supplementary Table S1). In a two-step process, the SOE product was then digested, cleaned and ligated into pLGB13 as described above. Then a tetQ (Martens et al., 2008) cassette was amplified and ligated into the SpeI/XmaI restriction sites in the intergenic spacer.
Third, the BVU3433 complementation construct was generated using pNBU2-bla-CfxA (Campbell et al., 2020). We amplified the gene and its native promoter (351 bp upstream of the start codon) and cloned it as described above into the multiple cloning site of the integrative plasmid pNBU2-bla-CfxA.
All ligation products were transformed into E. coli S-17 with electroporation. Individual ampicillin resistant colonies were isolated and purified plasmids were confirmed through PCR and Sanger sequencing. The confirmed allelic exchange and complementation vectors were then conjugated into the appropriate parent stains (e.g., P. vulgatus ∆tdk) and recombinant merodiploids were selected for on erythromycin. For the deletion and insertion mutants, merodiploids were allowed to loop out. Resolved merodiploids resistant to 20 μg/mL FUdR or 100 ng/μL aTC were isolated and screened by PCR desired mutations.
2.5 Measuring growth kinetics of Phocaeicola vulgatus
Wild-type and mutant P. vulgatus were grown and washed in TYG media. The cells were normalized and diluted to OD600 of 0.002 in TYG and dispensed in triplicate into a 96-well plate. Cell growth was measured every 30 min, over 36 h using a BMG Labtech CLARIOstar plate reader. Doubling times were calculated using the least-squares method for growth between 0.05 and 0.12 OD600 (n = 3).
2.6 RNA isolation and RNA-Seq
Samples for RNA-Seq of P. vulgatus, P. vulgatus ∆BVU3433, and B. thetaiotaomicron PvCTn:tetQ::∆BVU3433 were grown overnight in 5 mL TYG medium. Overnight cells were used to inoculate cultures at 10 mL TYG at a final dilution of 1:25 in biological triplicate. Cell growth was monitored and harvested at an OD600 of ~0.4. Total RNA was extracted using a lysis buffer (Degnan et al., 2014) and prepared with a Qiagen RNeasy kit and treated with DNA-free™ DNA Removal Kit (Invitrogen).
Total RNA was also extracted from B. thetaiotaomicron and P. vulgatus during conjugation. Conjugation of the two strains was carried out as above. However, after 24 h of growth, the conjugation mass was scraped from the plate surface into TYG and the cells were immediately pelleted and total RNA was extracted as described above.
Library preparation of RNA was completed by following the Illumina (San Diego, CA) Stranded Total RNA Prep, Ligation with Ribo-Zero protocol and using a starting material of 500 ng of total RNA. The only variation from the Illumina protocol is that the volume used for each reagent was reduced by half. The library was submitted to the UCR IIGB core for quality analysis of the multiplexed samples and sequencing on an Illumina NextSeq mid output 75 bp paired end platform.
RNA-Seq read quality was determined through FastQC1 and trimmed using trimmomatic (SLIDINGWINDOW:4:15 LEADING:2 TRAILING:2 MINLEN:70) (Bolger et al., 2014). Transcript expression was calculated using Rockhopper with default parameters (McClure et al., 2013) and trimmed reads were mapped to the P. vulgatus ATCC 8482 reference genome (Refseq: NC_009614.1), B. thetaiotaomicron VPI-5482 (Refseq:NC_004663), and/or PvCTn when appropriate.
2.7 cDNA preparation, qPCR
Phocaeicola vulgatus ∆tdk strains were grown overnight in 5 mL TYG medium. Overnight cells were used to inoculate cultures at 10 mL TYG at a final dilution of 1:25 in biological triplicate. Cell growth was monitored and harvested at an OD600 of ~0.4. When appropriate, cells were perturbed by 880 μM H2O2 and immediately harvested after 30 min of incubation at 37°C. Total RNA was extracted using a lysis buffer (Degnan et al., 2014) and prepared with a Qiagen RNeasy kit and treated with DNA-free™ DNA Removal Kit (Invitrogen).
Complementary DNA (cDNA) was generated from 500 ng total RNA using SUPERase•In™ RNase Inhibitor (Invitrogen) and Superscript-II RT (Invitrogen), where the RNA template was eventually degraded by inoculating 1 N NaOH for 30 min at 65°C and neutralized with 1 N HCl. DNA was isolated using a QIAquick spin column (Qiagen) and eluted in 10 mM Tris-Cl, pH 8.5.
Quantification of BVU3433 gene expression was measured through real-time quantitative PCR (qPCR) using Bio-Rad CFX96 Touch Real-Time PCR Detection System and SYBR Green (KAPA Biosystems) fluorescent dye. The CFX Maestro Software and ∆∆Cq method (Bookout et al., 2006) were used to process and calculate differences in BVU3433 and 16 s rRNA (Supplementary Table S2) expression. BVU3366 and BVU3378 were used as candidate genes to confirm RNA-Seq expression profiles.
2.8 Identification of PvCTn family among gut Bacteroidota
The boundaries of PvCTn were predicted using functional gene annotations of the regions surrounding BVU3433 and alignments of the genomic region with a panel of related P. vulgatus (n = 13) and P. dorei (n = 10) strains using Mauve (Darling et al., 2004; Supplementary Table S3). This included functionally characterizing the genomic region using HMMER v32 with trusted cutoffs to search the PFAM v35 (El-Gebali et al., 2019) and TIGRFAM v15 (Haft et al., 2003) databases (Frye et al., 2021). The CTn attachment sites (attL and attR) were determined using a combination of Mauve alignment inspection, BLASTn and manual sequence alignment.
The putative negative regulator BVU3433 was subsequently used to screen a total of 134 gut Bacteroidota genomes by BLASTp to identify homologs (bit score ratio ≥ 0.3; Supplementary Table S3). The process to identify the boundaries of PvCTn was repeated for each homolog detected as described above, determining its genomic context and if the homolog was a part of an MGE. Identified genomic regions corresponding to putative CTns were then compared using pairwise BLASTn (E value ≤0.0001), filtered for ≥20% percent length aligned (PLA)3 and then clustered using MCL (Inflation = 20) (Enright, 2002). Clusters were visualized in Cytoscape (Shannon et al., 2003). Alignments of individual genes were performed using Muscle v3.8.1551 (Edgar, 2004) and maximum likelihood phylogenies were reconstructed using FastTree v2.1.11 (Price et al., 2010).
2.9 Regulatory protein and promoter conservation analysis
Examination of the CTn clusters identified a conserved three gene regulatory region including BVU3433 and genes BVU3432 and BVU_RS21835. We extracted the intergenic region between BVU_RS21835 and BVU3433 (and homologs) for alignment and assessment for conserved sequence features. In addition, 250 nt upstream regions of differentially regulated operons were retrieved from PvCTn and conserved genes in related CTns. MEME (Bailey et al., 2015) was used to analyze these regions for conserved sequence patterns that may be involved as regulator binding sites. Searches were performed iteratively using different combinations of promoter regions, number of patterns retrieved (n = 5–6), maximum motif widths (n = 20–30), and motif distributions (‘zero or one occurrence per sequence’ or ‘any number of repetitions’).
2.10 Detection of PvCTn in human gut metagenomes
To identify the frequency of PvCTn-like elements in human gut metagenome samples we employed a marker gene approach. First, nucleotide sequences for two sets of species-specific markers were retrieved from 46 representative genomes in our panel (Supplementary Table S3) corresponding to universally conserved 30S ribosomal protein S5 (rpsE) and the attB site in pyruvate phosphate dikinase (ppdK). Second, 500 bp regions centered on the attL and attR of known PvCTn-like elements were retrieved. Finally, the terminal 500 bp of PvCTn-like integrases and the entire sequences of BVU3433 homologs were retrieved. In the end, all markers were ~ 500 bp in size. Then short read metagenomic datasets PRJEB7774 (Feng et al., 2015), PRJEB12449 (Vogtmann et al., 2016) and PRJEB10878 (Yu et al., 2017) from healthy patients and cohorts with colorectal cancer were retrieved from the NCBI SRA database (Supplementary Table S6). The data were quality filtered with Trimommatic as described above and mapped to the 6 marker gene regions using Bowtie2 with the default settings (Langmead and Salzberg, 2012). Conservative estimates of read coverage was measured for each sample based solely on the number of reads spanning the central 24 nt of a given marker gene. This ensured reads spanned the attB, attL and attR. Read coverages were first normalized by the number of reads per sample, then to the read coverage of rpsE. Paired reads were also taxonomically classified using Kraken2 (Wood et al., 2019) using the default parameters and the pluspf database (downloaded 17 May 2021).
To evaluate the robustness of this analysis, simulated short read data were generated from the genomes of P. vulgatus ATCC 8483 and 14 additional strains with wgsim (−1 100 –2 100 –d 300 –s 100 –N 4000000) part of the SamTools v1.16 package (Danecek et al., 2021) Three groups of ten randomized datasets of 30 million pairs of reads were generated with P. vulgatus ATCC 8483 comprising 10, 1% or 0.1% of the sample. The remaining reads in each dataset were randomly selected but divided equally among the other 14 strains. The datasets were then mapped with Bowtie2 against the marker genes and observed and expected read coverages were evaluated as described above.
3 Results
3.1 Tn mutagenesis enabled the identification of functional MGEs
Computationally predicted mobile genetic elements (MGEs) are common among Bacteroidota genomes, with over 97% of genomes examined having 1 or more predicted type of elements (Figure 1A; Frye et al., 2021). To determine the activity and functionality of these predicted MGEs, we employed an untargeted transposon (Tn) mutagenesis approach. Three prominent gut microbe species with computationally predicted MGEs were selected as donors: B. thetaiotaomicron VPI-5482, P. vulgatus ATCC 8482, and B. ovatus ATCC 8483. Each species has a distinct repertoire of predicted MGEs including CTns and integrated prophages (Figure 1B). Some of these MGEs have been demonstrated to be functional, allowing them to act as positive controls (Reyes et al., 2013; Campbell et al., 2020; Frye et al., 2021).
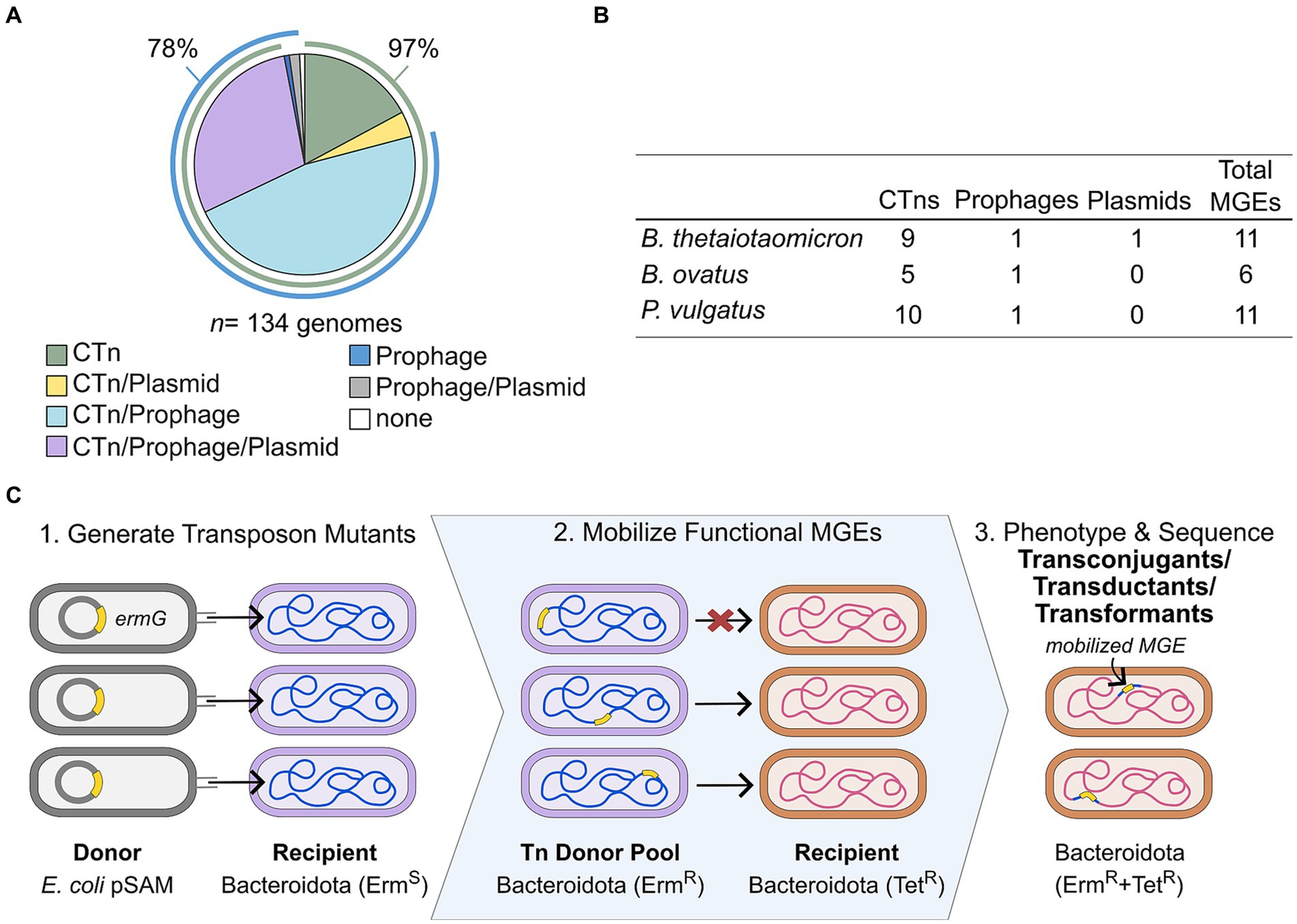
Figure 1. MGEs are common in human gut-associated Bacteroidota. (A) The proportions of 134 human gut-associated Bacteroidota with one or more of the computationally predicted MGE element classes (prophage, CTn and plasmid). (B) Predicted MGEs counts for the three Bacteroidota species used to generate Tn mutant libraries. (C) Schematic of untargeted Tn mutagenesis mobilization method (TMMM) for functional MGE detection.
The first step in the untargeted TMMM (Figure 1C) is to generate Tn libraries using the pSAM INseq vector (Goodman et al., 2009) for the three species. We isolated and pooled ~40,000 independent colonies per Tn library. These ErmR mutant library pools were then used as donors and mated with five TetR Bacteroidota recipients; B. thetaiotaomicron VPI-5482, B. thetaiotaomicron 3731, Parabacteroides merdae ATCC 43184, B. thetaiotaomicron VPI-5482 ∆CPS, and B. uniformis ATCC 8492. After mating and selective plating, dually resistant ErmR and TetR colonies represent putative MGE transfer events (Figure 1C).
The success of the TMMM strategy was mixed. In the mating growth conditions tested here, none of the nine predicted MGEs in B. thetaiotaomicron were found to be transferable. However, one B. ovatus and one P. vulgatus MGE were observed to be mobilizable (Figure 1B). Dually resistant colonies were recovered from matings of B. ovatus Tn with B. thetaiotaomicron VPI-5482 and B. thetaiotaomicron VPI-5482 ∆CPS and colony purified. Using arbitrary PCR (Goodman et al., 2009) we found that all strains screened were transconjugants that acquired BoCTn (16/16), a CTn that encodes a vitamin B12 transport locus (Frye et al., 2021). Thus, confirming this strategy for identifying MGE transfer events. For the P. vulgatus Tn donor library we initially only isolated dually resistant colonies when B. thetaiotaomicron VPI-5482 ∆CPS was used as the recipient. However, all of the isolates (16/16) harbored a single newly acquired and uncharacterized P. vulgatus CTn (PvCTn).
3.2 Fortuitous detection of a putative repressor of PvCTn activity
PvCTn is ~75 kb with 77 total genes including T4SS machinery, a putative ADP-ribosylglycohydrolase, and a Type I restriction-modification system (Figure 2A; Supplementary Table S2). Examination of the Tn insertion locations for the B. thetaiotaomicron VPI-5482 ∆CPS PvCTn transconjugants revealed that they were all inserted into gene BVU3433. This gene encodes a putative 149 amino acid helix-turn-helix (HTH) DNA binding domain protein (PF01381). BVU3433 has extensive Phyre2 predicted structural homology with diverse transcriptional regulators (e.g., PlcR [2QFC] 99.5% confidence, 91% coverage) (Declerck et al., 2007; Kelley et al., 2015). Further, alignments of the 16 arbitrary PCR products identified a total of 4 independent Tn insertion sites within BVU3433, all of which are expected to disrupt its function (Figure 2B). Together, this suggests that the inactivation of BVU3433 through pSAM mutagenesis de-represses PvCTn conjugation genes, thereby increasing the frequency of PvCTn mobilization.
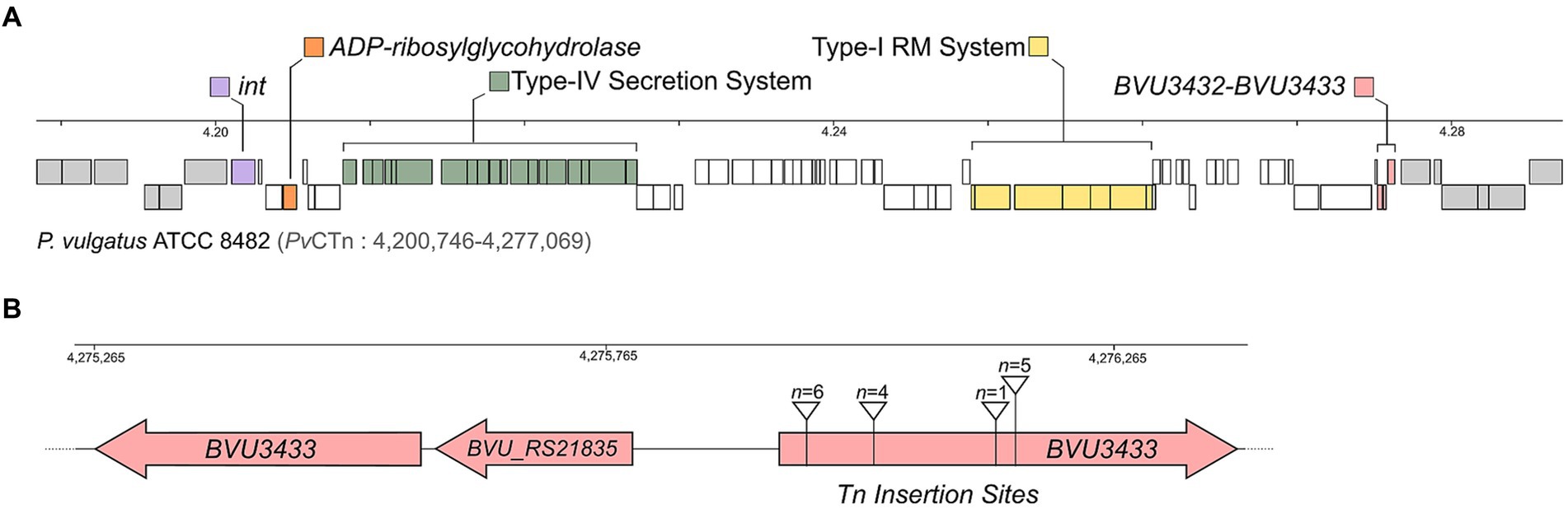
Figure 2. Detection of a mobilizable conjugative transposon PvCTn using Tn mutagenesis. (A) Scaled diagram of Phocaeicola vulgatus ATCC 8482 genomic region encoding PvCTn and its immediate upstream and downstream regions (genes in grey). Key gene regions described in the text are indicated. Scale bar indicated in megabases (Mb). (B) Schematic of the BVU3432–BVU3433 regulatory region and the four Tn cassette insertion locations identified in BVU3433 for the 16 screened transconjugants.
To directly test the function of BVU3433, we hypothesized that deleting BVU3433 from P. vulgatus should increase the conjugation efficiency of PvCTn. We first generated a clean deletion of BVU3433, followed by marking PvCTn in the wildtype and deletion background with an antibiotic resistance cassette using allelic exchange resulting in P. vulgatus ∆BVU3433 PvCTn:tetQ and P. vulgatus ∆tdk PvCTn:tetQ. We then carried out conjugations with these new donor strains using B. thetaiotaomicron VPI-5482 pNBU2_ermG and B. thetaiotaomicron VPI-5482 ∆CPS pNBU2_ermG as the recipient(s). The conjugation efficiencies of PvCTn ranged from 1.71 × 10−8 to 2.11 × 10−7 CFUs/mL for P. vulgatus PvCTn:tetQ and 8.77 × 10−7 – 1.28 × 10−6 CFUs/mL for P. vulgatus PvCTn:tetQ ∆BVU3433 depending on the recipient used (Figure 3A). Even though P. vulgatus ∆BVU3433 has a longer lag phase compared to WT (Supplementary Figure S1A), there were consistently 4.4–51.3-fold higher conjugation efficiencies of the P. vulgatus ∆BVU3433 mutant. We attempted to complement BVU3433 in trans using pNBU2-bla-CfxA however, no significant difference in conjugation efficiency compared to P. vulgatus with only pNBU2-bla-CfxA was detected (p = 0.12; Figure 3B). Regardless, the P. vulgatus ∆BVU3433 results recapitulate the phenotype detected with the initial Tn mutants.
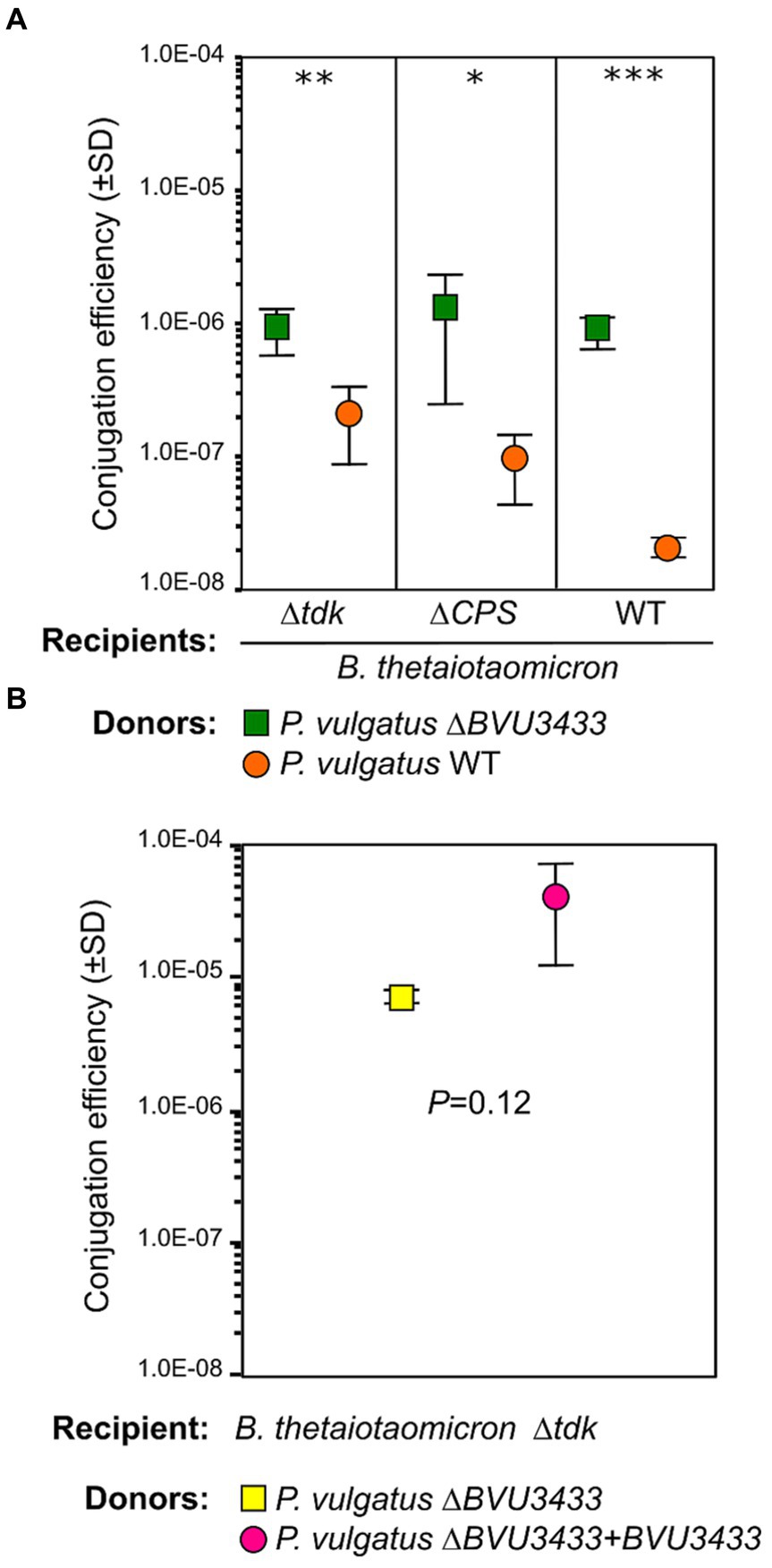
Figure 3. Conjugation efficiency of PvCTn is increased in the BVU3433 mutant. (A) Conjugation efficiencies of PvCTn from WT and ∆BVU3433 P. vulgatus donor strains were calculated using three Bacteroides thetaiotaomicron recipient strains with two technical replicates for each conjugation combination. This included mutant strains B. thetaiotaomicron ∆tdk and B. thetaiotaomicron ∆tdk ∆CPS and their WT parent B. thetaiotaomicron VPI-5482. (B) Complementation of BVU3433 in trans using pNBU2-bla-CfxA did not restore lower conjugation efficiencies to ∆BVU3433 + BVU3433 donors. Conjugation efficiencies of donor P. vulgatus ∆BVU3433 with an empty pNBU2-bla-CfxA vector or P. vulgatus ∆BVU3433 pNBU2-bla-CfxA_BVU3433 were calculated with B. thetaiotaomicron ∆tdk recipient cells. Conjugation efficiencies were compared between relevant strains using a one-tailed homoscedastic t-test significance (***p < 0.001; **p < 0.01; *p < 0.05). All measures represent means ± standard deviation 2–3 technical replicates.
3.3 BVU3433 alters the PvCTn transcriptome
After observing the consistent increase in PvCTn conjugation efficiency of the BVU3433 mutant we investigated differences in PvCTn WT and ∆BVU3433 transcription through RNA-Seq. We hypothesized that the increase in the mutant PvCTn conjugation efficiency is due to BVU3433 no longer repressing the putative conjugation genes in PvCTn. Therefore, we expected the conjugation genes in PvCTn to be upregulated in the mutant due to the lack of BVU3433 repression.
Analysis of the RNA-Seq data revealed that there was indeed an increase in expression throughout PvCTn (Figure 4). In fact, 60% of PvCTn genes (44/73) have a 2-fold or greater upregulation in the mutant. This included operons 4 and 5 that encode the majority of the PvCTn conjugation genes which were expressed an average of 11-fold greater in the BVU3433 mutant. Using qPCR, we confirmed the upregulation of two candidate operon 5 conjugation genes, one predicted to code for a putative DNA partitioning protein (BVU3366) and the other for a conserved protein found in CTns (BVU3378). We measured a significant 42 (p = 1.58 × 10−5) and 23-fold (p = 2.28 × 10−8) increase in expression in BVU3366 and BVU3378, respectively, in the mutant when compared to WT. This validates our RNA-Seq results (Supplementary Figure S2).
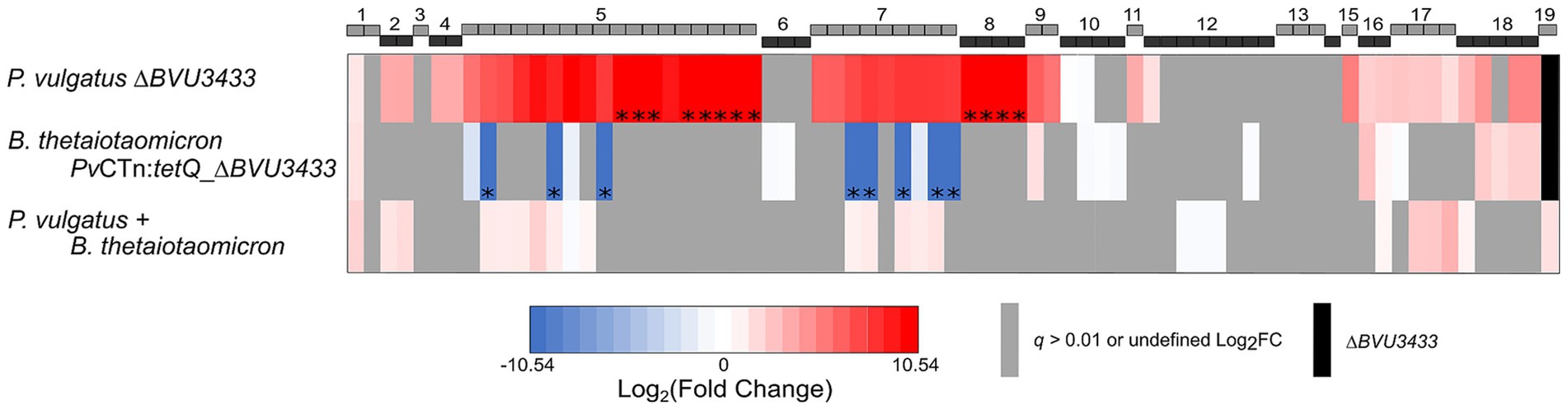
Figure 4. Loss of BVU3433 leads to the upregulation of PvCTn genes. Heatmap represents the log2 fold change (log2FC) of RNA-Seq gene expression values for PvCTn genes from (i) P. vulgatus ∆BVU3433, (ii) B. thetaiotaomicron PvCTn:tetQ_∆BVU3433, and (iii) P. vulgatus WT x B. thetaiotaomicron when compared to P. vulgatus WT (only genes with a q < 0.01 are shown, genes with q > 0.01 and/or undefined log2FC values are gray). Black bars represent gene deletions. RNA-Seq for all samples was carried out in biological triplicate. The corresponding gene names for each numbered operon are listed in Supplementary Table S2.
In addition to genes directly linked to the conjugation apparatus, we identified nine other PvCTn-encoded, putative regulatory genes that were differentially regulated by the absence of BVU3433 (Figure 4; Supplementary Table S4). This includes six putative HTH domain genes: BVU3415, BVU3420, BVU3426, BVU3429, BVU_RS21735, and BVU3432. These genes were expressed ~3.5–31.6 fold greater in the BVU3433 mutant when compared to WT. It is likely that one or more of these HTH genes are involved in the regulation of PvCTn however, the increased expression in the mutant suggests that BVU3433 is the primary regulator repressing these genes. The other three non-HTH regulators include an SOS-response transcriptional repressor (BVU3423), a HNH endonuclease (BVU3427), and a putative bacterial DNA-binding protein (BVU3428). Like the HTH genes, the BVU3433 mutant has increased gene expression for all three, with a fold change increase ranging from 2.6 to 51.3-fold more compared to WT, further indicating the key role of BVU3433 in PvCTn regulation.
To better understand the effect of BVU3433 on conjugation, RNA from two additional samples were sequenced. The first was from B. thetaiotaomicron with a mobilized PvCTn:tetQ::∆BVU3433 from P. vulgatus integrated into its genome. Remarkably, we determined that the expression profile of PvCTn:tetQ::∆BVU3433 in B. thetaiotaomicron was more like that of the WT P. vulgatus than the BVU3433 mutant. If anything, the expression is lower than WT, with 19% of PvCTn genes (14/73) are at least 2-fold more upregulated in than WT. Expression of operons 4, 5, 7, and 8 are not detected in B. thetaiotaomicron PvCTn:tetQ::∆BVU3433 (Figure 4). The lack of conjugation gene expression needed to produce the T4SS structures encoded in operons 4 and 5 suggests that PvCTn:tetQ::∆BVU3433 in a B. thetaiotaomicron recipient likely has reduced conjugation efficiency. Therefore, despite lacking BVU3433, the significant de-repression of genes observed in the mutant PvCTn in one host background (P. vulgatus) does not translate to a different host background (B. thetaiotaomicron). One possible explanation for this change in expression profile based on the host background may be due to other existing chromosomal or MGE-encoded regulators in B. thetaiotaomicron (Figure 1B).
The final sample we sequenced for RNA-Seq, unlike the previous RNA-Seq samples from mid-log phase cells growing in liquid TYG medium, was extracted directly from a mixed lawn of WT P. vulgatus and B. thetaiotaomicron growing on BHI-HB agar. Since conjugation does not generally occur in liquid cultures, we evaluated the expression of PvCTn during relevant conditions on solid medium. We hypothesized that the conjugation genes on operons 4 and 5 will be upregulated due to the increased likelihood of T4SS structures being formed in these conditions. RNA-Seq data of the B. thetaiotaomicron and P. vulgatus conjugation mass shows that even when only a small fraction of cells was likely directly conjugating in the mixed population, a slight upregulation of some operon 4 genes and DNA replication genes in operon 8 is observed. However, unlike the WT and mutant P. vulgatus, there is a slight downregulation of a cluster of restriction-modification genes in operon 17. Aside from the slight differences in the three operons mentioned, the expression values are like WT PvCTn in P. vulgatus (Figure 4A).
3.4 H2O2 decreases BVU3433 expression and increases conjugation efficiency
Due to the observed increase in conjugation and de-repression of PvCTn genes in P. vulgatus ∆BVU3433, we attempted to identify conditions that might influence the expression of BVU3433. We hypothesized that stress may be an important driver for the repression of BVU3433, therefore, we tested BVU3433 expression via qPCR of P. vulgatus exposed to various sub-inhibitory stress conditions including H2O2, salinity, antibiotics, taurocholic acid, heat, and UV. We discovered a significant (p = 0.015) ~4.4-fold decrease in BVU3433 expression of H2O2-exposed P. vulgatus when compared to the control group (Figure 5A). Considering this decrease in BVU3433 expression, we tested how H2O2-exposure affects conjugation efficiency. We observed an insignificant (p = 0.42) 1.73-fold increase in the conjugation efficiency of the H2O2-exposed WT P. vulgatus (Figure 5B) and the P. vulgatus ∆BVU3433 strain followed the same trend with an insignificant (p = 0.36) 1.45-fold increase in conjugation efficiency for the H2O2-exposed mutant (Figure 5B). Together, the qPCR and conjugation efficiency data suggest that subinhibitory H2O2 stress can increase conjugation efficiency. However, since the conjugation efficiency of both WT and ∆BVU3433 P. vulgatus increased with H2O2 stress, it is possible that H2O2 not only influences the expression of BVU3433 but is likely to have additional effects on the cell physiology of the donor and/or recipient cells.
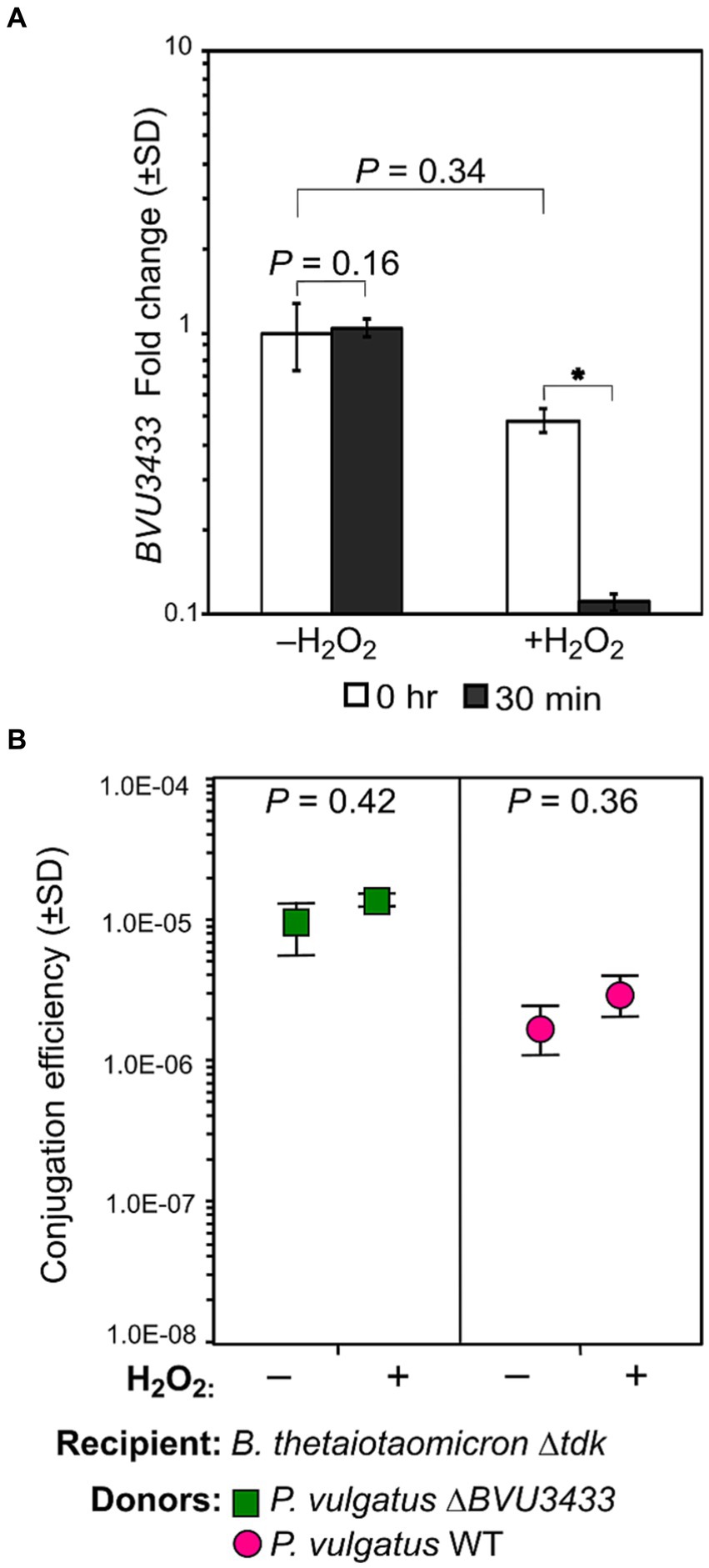
Figure 5. H2O2 exposure downregulates BVU3433 expression and increases conjugation efficiency. (A) Relative fold change of BVU3433 was measured in mid-log phase P. vulgatus WT treated with H2O2 or not immediately prior to exposure and after 30 min of incubation at 37°C. RT-qPCR was performed on RNA extracted from biological triplicate cultures for the two treatment groups. (B) Conjugation efficiencies of PvCTn from WT and ∆BVU3433 P. vulgatus donor strains were calculated using B. thetaiotaomicron ∆tdk recipients while exposed to H2O2. Conjugation efficiencies were compared between relevant strains using a one-tailed homoscedastic t-test significance from 2–3 technical replicates.
3.5 PvCTn-like MGEs are common among other Bacteroidota
To determine the prevalence of BVU3433-mediated regulation among gut microbes, we screened 133 other gut microbial genomes for BVU3433 homologs. This search identified 22 putative BVU3433 homologs, 19 of which were encoded by intact CTns found in eight additional species (Supplementary Table S5). The three remaining homologs reside in genomic loci that appear to have experienced deletions or rearrangements leading to the loss of nearly all genes essential for conjugation. Examination of the shared DNA among the 19 CTns content generated four CTn clusters (Figure 6A; Supplementary Figure S3A). Like PvCTn, most encode restriction-modification systems, while others encode putative metal (tellurite) resistance genes (PF02342, PF05099, PF15616), UV protection genes (PF00817, PF11700) and/or protein phosphatases (PF13672).
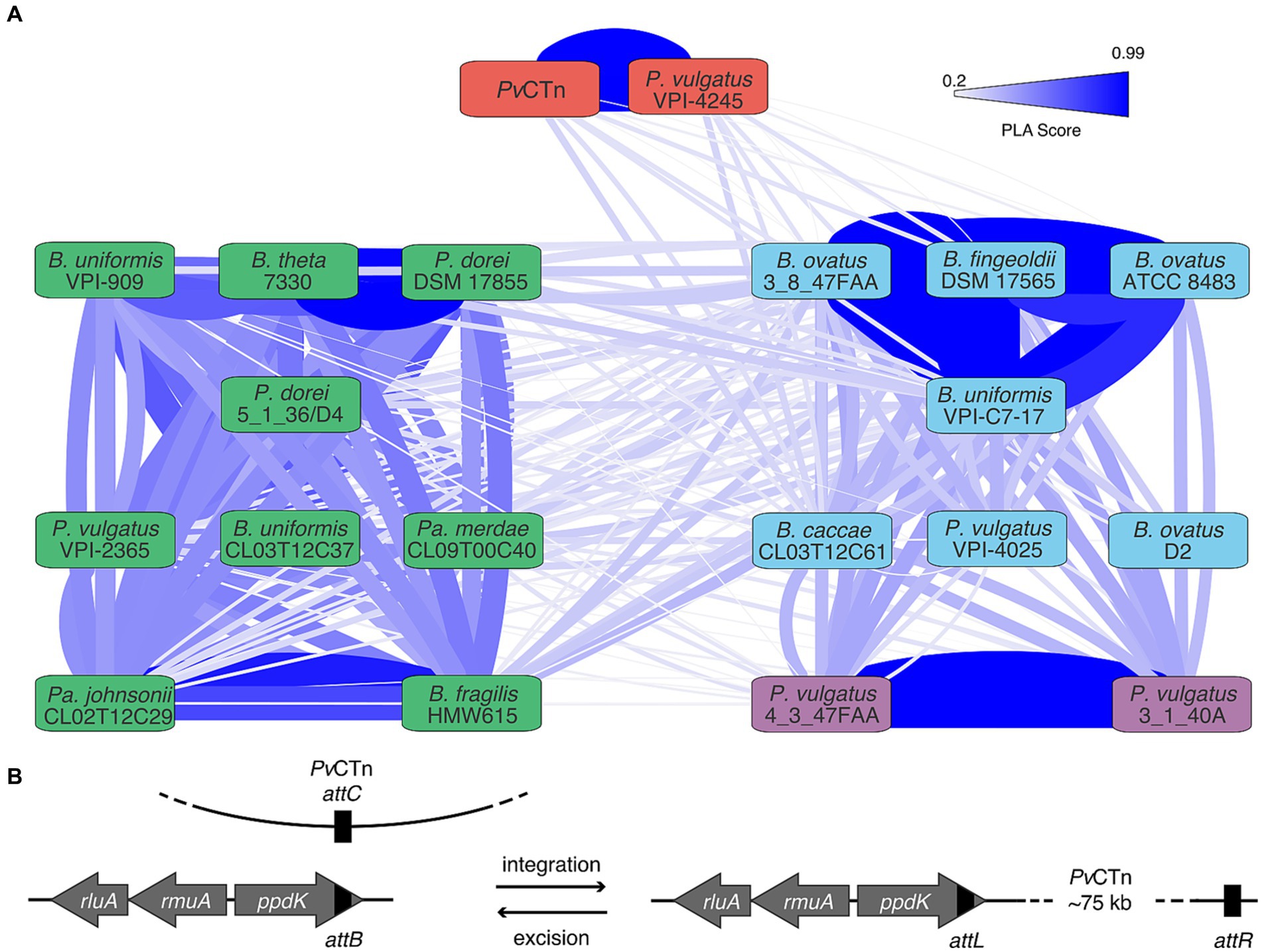
Figure 6. Genetic similarities among PvCTn-like MGEs from human gut microbes. (A) Network analysis of DNA sequence similarity among BVU3433-homolog encoding CTns from the human gut microbiome. Four clusters of genome encoded CTn-like elements were identified using MCL based on the percentage of length aligned (PLA) scores. Clusters are indicated by the colored nodes labeled with the strain of origin and edge thickness and color correspond to PLA scores shown in the key (20–99%). (B) Schematic of site-specific PvCTn-like MGE insertions in the 3′ end of the strain’s ppdK gene. In each case, a stop codon of ppdK is regenerated within 2–3 amino acids of expected location after MGE integration.
Despite the divergence in their overall gene content, all 19 CTns share a common integration site at the 3′ end of a conserved three gene cluster rluA-rmuA-ppdK. Specifically, we identified the attB as the 20 bp motif 5’-GYS GCN CAR GCK GCH RTH GA-3′ within the 3′ end of ppdK itself (Figure 6B). Integration of the CTn produces two imperfect direct repeats (attL and attR) and in each instance regenerates a stop codon within three amino acids of the typical ppdK stop codon. Our re-examination of the RNA-Seq read data from our novel B. thetaiotaomicron PvCTn:tetQ::∆BVU3433 transconjugant detected transcriptional readthrough across both the novel attL and attR sites (Supplementary Figure S3B). These data confirm the preference for this integration location by PvCTn. The conservation of the attB is somewhat surprising due to the sequence diversity observed among the predicted integrases (mean = 78.3% ± 9.9% amino acid identity; Supplementary Figure S3C). On further inspection of the attB site in the panel of gut microbial genomes we identified another five distinct MGEs of various sizes in 10 genomes that lack BVU3433 but do encode related integrases (Supplementary Figure S3C).
In addition, we found that all 22 BVU3433 homologs encode adjacent homologs of BVU3432 (105AA, merR-like HTH domain PF13411) and the small ORF BVU_RS21835 (63AA, no conserved domains) (Figure 7A). The divergent transcriptional organization of these HTH domain proteins is akin to that of cI and cro in phage Lambda and similar phages (Degnan et al., 2007). By examining the RNA-Seq transcriptional coverage we were able to identify putative transcriptional start sites and 5′ untranslated regions (5’UTRs) for BVU3433, BVU_RS21835, and several differentially regulated operons in PvCTn. We subsequently found that the RNA polymerase binding sites (−10 and −35) of BVU3433 and BVU_RS21835 occur within the 5’UTR of the other gene owing to the small size of this intergenic region and are highly conserved among the related CTns (Figure 7A).
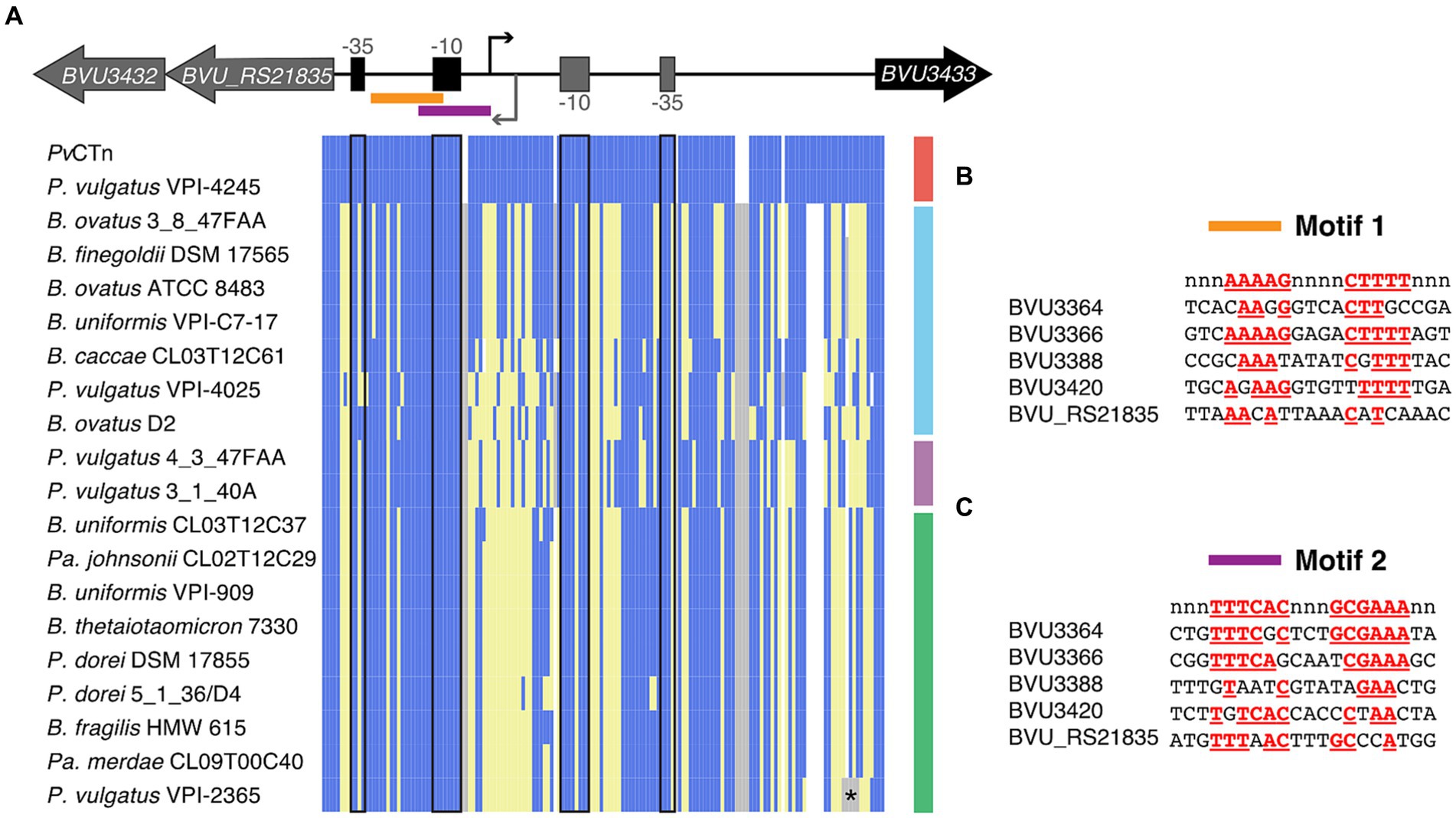
Figure 7. PvCTn-like MGEs encode conserved regulatory regions. (A) Sequence alignment of BVU_RS21835–BVU3433 intergenic region from all 20 PvCTn-like MGEs starting and stopping with the first codon of the indicated gene. The overlapping and conserved RNA polymerase binding sites and transcription start sites are indicated for each divergently transcribed gene. Sites in blue correspond to nucleotides identical to PvCTn, yellow represents alternate nucleotides, white indicates gaps in the alignment, and gray represents inserted nucleotides. The asterisk indicates that P. vulgatus VPI-2365 has a hypothetical gene inserted at this location and encoded in the same orientation as BVU3433. (B,C) Alignments of two conserved motifs with dyad symmetry identified by MEME and found in the upstream regions of five PvCTn operons that are differentially expressed in the ∆BVU3433 strain and broadly conserved in PvCTn-like MGEs. Majority rule consensus sequence is shown on the top line in red and matching positions are shown for each upstream region.
Given the broad conservation of the BVU3432 – BVU3433 regulatory region among the CTns as well as genes involved in the conjugative apparatus, we computationally searched for conserved motifs that may be involved in regulating conjugation activity. We examined the upstream regions of five genes differentially expressed in ∆BVU3433 that represent the first gene in the operon and are conserved in ≥16 of the 19 related CTns. This analysis identified conserved features including RNA polymerase binding sites for all genes as well as potential hairpin structures that might act as transcriptional terminators (Figure 7A; Supplementary Figure S3D). The analysis also identified 2 conserved motifs with imperfect dyad symmetry that we observed in all five upstream regions (Figures 7B,C; Supplementary Figure S3D). It is possible that one or both of these motifs act as binding sites for BVU3433 or another PvCTn encoded regulator (e.g., BVU3432) and be responsible for activation or repression of the conjugative apparatus.
3.6 Detection of PvCTn in human gut metagenomes
Using a marker gene approach, evidence for PvCTn-like elements was detected among Bacteroidota species from both healthy patients and those with colorectal cancer (CRC) in 3 geographically distinct studies (Austria, United States, China) (Figure 8A; Supplementary Figures S4A,B). We found sequence evidence for PvCTn-like elements in ~90% of all the patient samples analyzed (300/335). Metagenome sequence reads semi-quantitatively represent both the diversity and abundance of species and strains present in a community sample of isolated cells. As such we can conservatively estimate that ~5–14% of Bacteroidota cells encode a BVU3433 homolog among the patient sample groups screened. We detected similar coverage ranges indicating the integration of PvCTn-like elements (e.g., ~7–12% attL and ~ 2–7% attR; Figure 8A; Supplementary Figures S4A,B). The higher prevalence of the integrase (~14–19% BVU3359) than BVU3433 is consistent with our identification of MGEs that share a BVU3359-like integrase, but do not encode the BVU3432–BVU3433 regulatory region (Supplementary Figure S4C). Further, despite the previously described differences in community composition including a greater proportion of Bacteroidota cells (Figure 8B) and immunological status between the healthy patients and those with colorectal cancer (Feng et al., 2015; Vogtmann et al., 2016; Yu et al., 2017) we saw no difference in the frequency of PvCTn-like elements after normalizing for Bacteroidota abundance (Figure 8A).
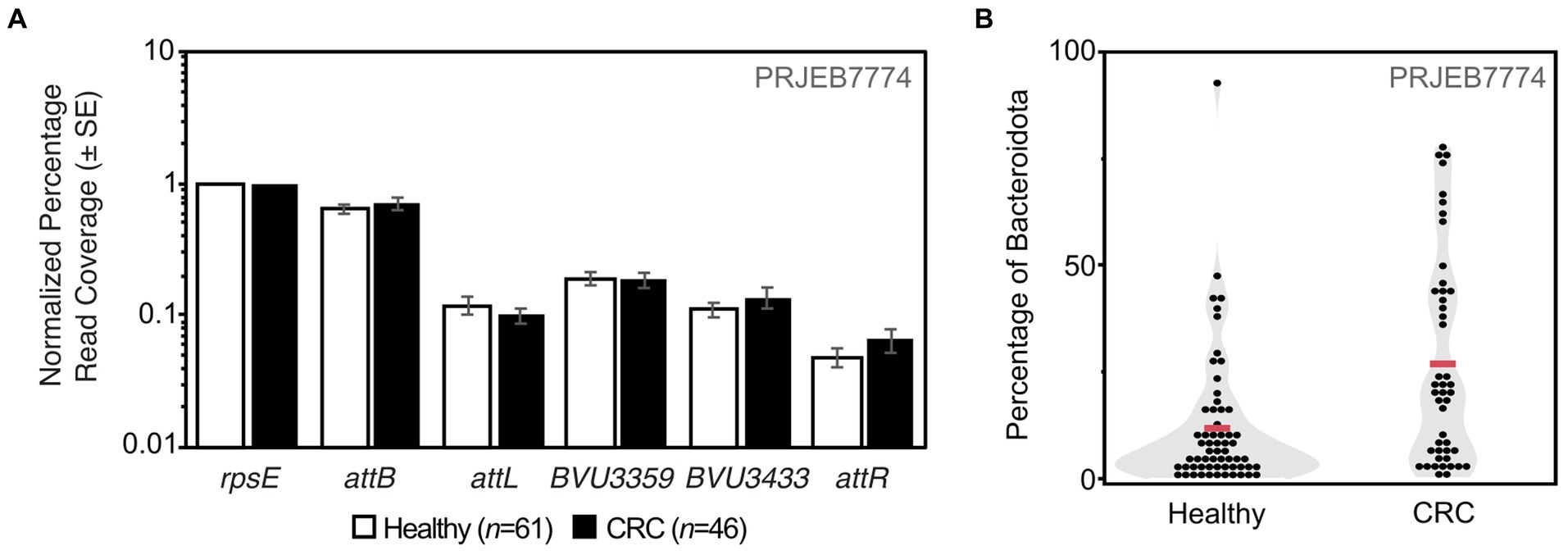
Figure 8. Metagenome detection of PvCTn-like elements. (A) Metagenomic short reads from a panel of healthy patients and those with colorectal cancer (CRC) were mapped to the indicated marker genes. Graph shows the average read coverage among samples and error bars represent standard error of the mean (SE). Read coverage was normalized for each sample first by the number of quality filtered short reads and then normalized to gut Bacteroidota rpsE gene coverage. (B) The same data were also subjected to taxonomic classification using Kraken2 to determine the proportion of Bacteroidota per sample. Individual black dots represent proportion of reads classified as Bacteroidota from the total number of reads that were classified per sample. The average proportion of Bacteroidota among each patient cohort is indicated with the red line (Healthy = 11.7% ± 2.0% SE, CRC = 26.8% ± 3.6% SE).
Analysis of simulated datasets suggests reliable detection of PvCTn when strains with integrated elements account for ≥1% of the population, however, when using the described marker genes and PvCTn encoding strains approach 0.1% detection becomes unpredictable (~50:50; Supplementary Figure S4C). It is likely that such unpredictability could be ameliorated by deeper sequencing coverage. The simulated data, like the actual patient data, also detected a slightly lower than expected coverage of attL and attR sequences (Supplementary Figure S4C). This is possibly due to a read mapping conflict with the sequence similar attB region, but in the patient data it may also be affected by the existence of novel attL and attR junctions not represented in our marker gene dataset (e.g., novel PvCTn-like MGE integration events).
4 Discussion
Bacterial MGEs are known to be significant drivers of bacterial evolution. Therefore, identifying functional MGEs is important for understanding the distribution and exchange of fitness determinants in bacteria. Our study used an untargeted TMMM as a novel approach for tracking functional MGEs in Bacteroidota (Figure 1C). Moreover, this method can be applied to non-Bacteroidota models with established means of Tn mutagenesis including well-known human pathogens (e.g., Pseudomonas aeruginosa, Mycobacterium tuberculosis, Vibrio cholerae) and symbionts (E. coli, Bifidobacterium breve) (Cain et al., 2020; Dempwolff et al., 2020).
Previous research has established the importance of MGEs in altering bacterial phenotypes, which can have considerable effects on human health (Durrant et al., 2020; Panwar et al., 2023). For instance, the emergence and dissemination of a single carbapenem-hydrolyzing gene (blaNDM) carried by diverse transposable elements have put the effectiveness of carbapenems, broad-spectrum antibiotics used for treating various bacterial infections, at risk on a global scale (Acman et al., 2022). Moreover, virulence genes have been observed to mobilize from pathogenic to non-pathogenic bacteria (Messerer et al., 2017), underscoring the potential of MGEs to enhance pathogenicity. As the acquisition of MGEs can immediately impact bacterial fitness, discovering novel MGEs could aid the development of techniques to modify microbial communities for improved human health.
4.1 Constraints of existing approaches for identifying functional MGEs
Computational methods are effective at identifying potential MGEs in bacterial genomes (Akhter et al., 2012; Ozer et al., 2014; Roux et al., 2015; Johansson et al., 2020). However, predictions alone cannot confirm the ability of an MGE to mobilize, which often requires targeted mutations to confirm or the reliance on capturing chance transfer events in genomic data (Coyne et al., 2014). Our untargeted MGE capture method bypasses the need for directed mutations (Saak et al., 2020). Although a previous study developed transposon-aided capture (TRACA), which allows for the preferential capture of circular extrachromosomal MGEs (i.e., plasmids) (Jones and Marchesi, 2006), it is unlikely to capture elements like PvCTn. On the other hand, entrapment vectors like pBACpAK (Tansirichaiya et al., 2022), if adapted for Bacteroidota hosts, may be able to capture PvCTn. However, this method is reliant upon MGEs inserting into a small region of the recipient pBACpAK vector, which may or may not have sufficient sequence homology for site-specific integrases. In contrast, our method mutagenizes the donor and enables MGEs to integrate at a preferred attachment site within recipient cells.
4.2 Tn mutagenesis mobilization method provides insight into CTn regulation
In this study, we successfully identified the mobilization of BoCTn and PvCTn using this Tn mutagenesis method. Curiously, all the PvCTn transconjugants harbored pSAM-mediated disruptions of BVU3433. Our subsequent analyses demonstrated a consistent increase in the conjugation efficiency of P. vulgatus ∆BVU3433 mutants compared to WT. While additional Tn insertions likely occurred in T4SS genes or activators of PvCTn mobilization the data suggest that our method may be able to preferentially capture MGEs by disrupting negative regulators of mobilization. However, this is clearly not always the case, as we successfully captured BoCTn which encodes a vitamin B12 transporter (Frye et al., 2021) and the Tn cassette integrated into an uncharacterized gene (BACOVA0479). Regardless, the range of MGEs captured for each strain will vary based on the functional and regulatory differences in genes essential for mobilization.
Regulation of mobilization of CTns is frequently subject to muti-layer, tight regulation (Salyers et al., 1995; Johnson and Grossman, 2015). CTnDOT, a well-characterized CTn in Bacteroidota, requires tetracycline exposure to trigger a four-step process. This process involves seven regulatory proteins and RNAs in its regulatory cascade for the excision and mobilization of CTnDOT to complete (Waters and Salyers, 2013). Our results identify the critical role of BVU3433 in repressing many PvCTn genes and as a result significantly reducing mobilization. Yet our findings also indicate that BVU3433 is likely only the first component of the PvCTn regulatory cascade, as nine other PvCTn-encoded regulatory-related genes were upregulated in the absence of BVU3433. However, the specific regulatory mechanisms connecting these proteins and the DNA-binding sites of BVU3433 on PvCTn remain to be elucidated. Given the broad upregulation of PvCTn ∆BVU3433 genes compared to WT PvCTn (Figure 4), we hypothesize that BVU3433 binds to one or more PvCTn intergenic spacers. Further, we propose that the conserved sequence motifs we identified in the upstream regions of five of the differentially regulated operons represent possible binding sites.
Finally, adding to the complexity of possible regulatory systems within PvCTn, operon 2 of PvCTn contains BVU3362, a gene predicted to encode ADP-ribosylglycohydrolase, a class of enzymes commonly involved in post-translational modifications (Mikolčević et al., 2021). In Bacteria, ADP-ribosylation is often linked to the post-translational modification of eukaryotic proteins (Simon et al., 2014). For instance, the Diphtheria toxin produced in Corynebacterium diphtheriae inhibits cellular protein synthesis in the host and promotes pathogenicity through the ADP-ribosylation of eukaryotic elongation factor 2 (Bachran et al., 2007). Presently, the regulatory mechanism(s) of BVU3362 within PvCTn is unknown. However, given that ADP-ribosylation has been shown to be involved in Legionella pneumophila T4SS effector translocation, it is plausible that BVU3362 may also be involved in the post-translational regulation of the PvCTn T4SS (Amor et al., 2005). This hypothesis will require further investigation to fully understand the role and targets of BVU3362. Overall, this study provides a preliminary characterization of PvCTn’s regulatory mechanism, which is likely to be complex and involve additional layers of transcriptional and translational regulation.
4.3 PvCTn-like elements are diverse and globally distributed
Our characterization of PvCTn and BVU3433 enabled our detection of a diverse group of PvCTn-like elements in human gut-associated bacterial genomes. These conjugative transposons all share a common site-specific integrase and attachment site (attB) along with the BVU3432-BVU3433 regulatory region, and genes involved in the T4SS. While none encode known antibiotic resistance genes, several encode metal and UV resistance genes that may contribute to the fitness of their hosts. Further, virtually all the CTns encode restriction modification systems which may have consequences for further mobile DNA acquisitions by their hosts.Individual bacterial genomes are generally crucial for identifying host-MGE pairs, but they represent only a fraction of species and strain diversity that exists in human guts globally. As such we interrogated the prevalence of PvCTn-like elements in human gut metagenomes (Feng et al., 2015; Vogtmann et al., 2016; Yu et al., 2017), and found they were widespread in ~90% of patient samples. Together these results suggest PvCTn-like elements contribute to genetic and functional diversity of human gut microbes.
5 Conclusion
In this study, we have successfully demonstrated a new and effective method for capturing MGEs from gut microbes. Although our approach did not detect mobilization of all the predicted MGEs, it expands the currently available methods for MGE identification and offers a potential strategy for numerous other bacteria. Our method enabled us to confirm PvCTn functionality and identify a conserved conjugation repressor protein. Further, PvCTn represents one member of a diverse family of elements that can be detected in patient samples from around the globe. Further, these PvCTn-like elements may indirectly contribute to human health as studies have highlighted the distribution of P. vulgatus and its sister species P. dorei in the gut microbiome as a critical determinant in the efficacy of immune checkpoint blockade therapy in advanced melanoma patients (Usyk et al., 2021) and the development of coronary artery disease (Yoshida et al., 2018). And strains of these two species encode 8 of the 20 PvCTn-like elements we identified. Overall, our findings demonstrate the potential of our method for the discovery of novel MGEs and provide insights into the prevalence and distribution of PvCTn-like elements in human gut-associated bacteria.
Data availability statement
The original contributions presented in the study are publicly available. This data can be found here: NCBI BioProject, accession PRJNA983822.
Author contributions
JO: conceptualization, methodology, lab work, manuscript writing and reviewing, and data analysis. PD: conceptualization, methodology, developed software, manuscript writing and reviewing, and data analysis. All authors contributed to the article and approved the submitted version.
Funding
This research was supported by initial complement funding to PD from UCR. JO was supported in part by a Higher Education Emergency Relief Fund (HEERF) Fellowship.
Acknowledgments
We thank Ansel Hsiao and Jason Stajich for useful feedback on the research involved in this manuscript. We would also like to acknowledge Danielle Campbell for the PLA code and BvCTn clustering strategy.
Conflict of interest
The authors declare that the research was conducted in the absence of any commercial or financial relationships that could be construed as a potential conflict of interest.
Publisher’s note
All claims expressed in this article are solely those of the authors and do not necessarily represent those of their affiliated organizations, or those of the publisher, the editors and the reviewers. Any product that may be evaluated in this article, or claim that may be made by its manufacturer, is not guaranteed or endorsed by the publisher.
Supplementary material
The Supplementary material for this article can be found online at: https://www.frontiersin.org/articles/10.3389/fmicb.2024.1241582/full#supplementary-material
Footnotes
References
Acman, M., Wang, R., van Dorp, L., Shaw, L. P., Wang, Q., Luhmann, N., et al. (2022). Role of mobile genetic elements in the global dissemination of the carbapenem resistance gene blaNDM. Nat. Commun. 13:1131. doi: 10.1038/s41467-022-28819-2
Akhter, S., Aziz, R. K., and Edwards, R. A. (2012). PhiSpy: a novel algorithm for finding prophages in bacterial genomes that combines similarity- and composition-based strategies. Nucleic Acids Res. 40:e126. doi: 10.1093/nar/gks406
Amor, J. C., Swails, J., Zhu, X., Roy, C. R., Nagai, H., Ingmundson, A., et al. (2005). The structure of RalF, an ADP-ribosylation factor guanine nucleotide exchange factor from Legionella pneumophila, reveals the presence of a cap over the active site. J. Biol. Chem. 280, 1392–1400. doi: 10.1074/jbc.M410820200
Bachran, C., Sutherland, M., Bachran, D., and Fuchs, H. (2007). Quantification of diphtheria toxin mediated ADP-ribosylation in a solid-phase assay. Clin. Chem. 53, 1676–1683. doi: 10.1373/clinchem.2007.085365
Bailey, T. L., Johnson, J., Grant, C. E., and Noble, W. S. (2015). The MEME suite. Nucleic Acids Res. 43, W39–W49. doi: 10.1093/nar/gkv416
Bolger, A. M., Lohse, M., and Usadel, B. (2014). Trimmomatic: a flexible trimmer for Illumina sequence data. Bioinformatics 30, 2114–2120. doi: 10.1093/bioinformatics/btu170
Bookout, A. L., Cummins, C. L., Mangelsdorf, D. J., Pesola, J. M., and Kramer, M. F. (2006). High-throughput real-time quantitative reverse transcription PCR. Curr. Protoc. Mol. Biol. 73, 15.8.1–15.8.28. doi: 10.1002/0471142727.mb1508s73
Cain, A. K., Barquist, L., Goodman, A. L., Paulsen, I. T., Parkhill, J., and van Opijnen, T. (2020). A decade of advances in transposon-insertion sequencing. Nat. Rev. Genet. 21, 526–540. doi: 10.1038/s41576-020-0244-x
Campbell, D. E., Ly, L. K., Ridlon, J. M., Hsiao, A., Whitaker, R. J., and Degnan, P. H. (2020). Infection with Bacteroides phage BV01 alters the host transcriptome and bile acid metabolism in a common human gut microbe. Cell Rep. 32:108142. doi: 10.1016/j.celrep.2020.108142
Coyne, M. J., Zitomersky, N. L., McGuire, A. M., Earl, A. M., and Comstock, L. E. (2014). Evidence of extensive DNA transfer between Bacteroidales species within the human gut. mBio 5, e01305–e01314. doi: 10.1128/mBio.01305-14
Danecek, P., Bonfield, J. K., Liddle, J., Marshall, J., Ohan, V., Pollard, M. O., et al. (2021). Twelve years of SAMtools and BCFtools. Gigascience 10:giab008. doi: 10.1093/gigascience/giab008
Darling, A. C. E., Mau, B., Blattner, F. R., and Perna, N. T. (2004). Mauve: multiple alignment of conserved genomic sequence with rearrangements. Genome Res. 14, 1394–1403. doi: 10.1101/gr.2289704
Declerck, N., Bouillaut, L., Chaix, D., Rugani, N., Slamti, L., Hoh, F., et al. (2007). Structure of PlcR: insights into virulence regulation and evolution of quorum sensing in gram-positive bacteria. Proc. Natl. Acad. Sci. USA 104, 18490–18495. doi: 10.1073/pnas.0704501104
Degnan, P. H., Michalowski, C. B., Babić, A. C., Cordes, M. H. J., and Little, J. W. (2007). Conservation and diversity in the immunity regions of wild phages with the immunity specificity of phage lambda. Mol. Microbiol. 64, 232–244. doi: 10.1111/j.1365-2958.2007.05650.x
Degnan, P. H., Barry, N. A., Mok, K.C., Taga, M. E., and Goodman, A. L. (2014). Human gut microbes use multiple transporters to distinguish Vitamin B12 analogs and compete in the gut. Cell Host Microbe. 15, 47–57. doi: 10.1016/j.chom.2013.12.007
Dempwolff, F., Sanchez, S., and Kearns, D. B. (2020). TnFLX: a third-generation mariner-based transposon system for Bacillus subtilis. Appl. Environ. Microbiol. 86:e02893-19. doi: 10.1128/AEM.02893-19
Duerkop, B. A., Clements, C. V., Rollins, D., Rodrigues, J. L. M., and Hooper, L. V. (2012). A composite bacteriophage alters colonization by an intestinal commensal bacterium. Proc. Natl. Acad. Sci. USA 109, 17621–17626. doi: 10.1073/pnas.1206136109
Durrant, M. G., Li, M. M., Siranosian, B. A., Montgomery, S. B., and Bhatt, A. S. (2020). A Bioinformatic analysis of integrative Mobile genetic elements highlights their role in bacterial adaptation. Cell Host Microbe 27, 140–153.e9. doi: 10.1016/j.chom.2019.10.022
Edgar, R. C. (2004). MUSCLE: multiple sequence alignment with high accuracy and high throughput. Nucleic Acids Res. 32:1792–1797. doi: 10.1093/nar/gkh340
El-Gebali, S., Mistry, J., Bateman, A., Eddy, S. R., Luciani, A., Potter, S. C., et al. (2019). The Pfam protein families database in 2019. Nucleic Acids Res. 47, D427–D432. doi: 10.1093/nar/gky995
Enright, A. J. (2002). An efficient algorithm for large-scale detection of protein families. Nucleic Acids Res. 30:1575–1584. doi: 10.1093/nar/30.7.1575
Feng, Q., Liang, S., Jia, H., Stadlmayr, A., Tang, L., Lan, Z., et al. (2015). Gut microbiome development along the colorectal adenoma-carcinoma sequence. Nat. Commun. 6:6528. doi: 10.1038/ncomms7528
Frye, K. A., Piamthai, V., Hsiao, A., and Degnan, P. H. (2021). Mobilization of vitamin B12 transporters alters competitive dynamics in a human gut microbe. Cell Rep. 37:110164. doi: 10.1016/j.celrep.2021.110164
García-Bayona, L., and Comstock, L. E. (2019). Streamlined genetic manipulation of diverse Bacteroides and Parabacteroides isolates from the human gut microbiota. mBio 10, e01762–e01719. doi: 10.1128/mBio.01762-19
Gay, P., Le Coq, D., Steinmetz, M., Berkelman, T., and Kado, C. I. (1985). Positive selection procedure for entrapment of insertion sequence elements in gram-negative bacteria. J. Bacteriol. 164, 918–921. doi: 10.1128/jb.164.2.918-921.1985
Goodman, A. L., McNulty, N. P., Zhao, Y., Leip, D., Mitra, R. D., Lozupone, C. A., et al. (2009). Identifying genetic determinants needed to establish a human gut symbiont in its habitat. Cell Host Microbe 6, 279–289. doi: 10.1016/j.chom.2009.08.003
Haft, D. H., Selengut, J. D., and White, O. (2003). The TIGRFAMs database of protein families. Nucleic Acids Res. 31:371–373. doi: 10.1093/nar/gkg128
Human Microbiome Project Consortium (HMP) (2012). Structure, function and diversity of the healthy human microbiome. Nature 486, 207–214. doi: 10.1038/nature11234
Johansson, M. H. K., Bortolaia, V., Tansirichaiya, S., Aarestrup, F. M., Roberts, A. P., and Petersen, T. N. (2020). Detection of mobile genetic elements associated with antibiotic resistance in Salmonella enterica using a newly developed web tool: MobileElementFinder. J. Antimicrob. Chemother. 76, 101–109. doi: 10.1093/jac/dkaa390
Johnson, C. M., and Grossman, A. D. (2015). Integrative and conjugative elements (ICEs): what they do and how they work. Annu. Rev. Genet. 49, 577–601. doi: 10.1146/annurev-genet-112414-055018
Jones, B. V., and Marchesi, J. R. (2006). Transposon-aided capture (TRACA) of plasmids resident in the human gut mobile metagenome. Nat. Methods 4, 55–61. doi: 10.1038/nmeth964
Kelley, L. A., Mezulis, S., Yates, C. M., Wass, M. N., and Sternberg, M. J. E. (2015). The Phyre2 web portal for protein modeling, prediction and analysis. Nat. Protoc. 10, 845–858. doi: 10.1038/nprot.2015.053
Kiljunen, S., Pajunen, M. I., and Savilahti, H. (2017). Transposon insertion mutagenesis for archaeal gene discovery. Methods Mol. Biol. 1498, 309–320. doi: 10.1007/978-1-4939-6472-7_20
Lampe, D. J., Churchill, M. E., and Robertson, H. M. (1996). A purified mariner transposase is sufficient to mediate transposition in vitro. EMBO J. 15, 5470–5479. doi: 10.1002/j.1460-2075.1996.tb00930.x
Langmead, B., and Salzberg, S. L. (2012). Fast gapped-read alignment with bowtie 2. Nat. Methods 9, 357–359. doi: 10.1038/nmeth.1923
Martens, E. C., Chiang, H. C., and Gordon, J. I. (2008). Mucosal glycan foraging enhances fitness and transmission of a saccharolytic human gut bacterial symbiont. Cell Host Microbe. 4:447–457. doi: 10.1016/j.chom.2008.09.007
McClure, R., Balasubramanian, D., Sun, Y., Bobrovskyy, M., Sumby, P., Genco, C. A., et al. (2013). Computational analysis of bacterial RNA-Seq data. Nucleic Acids Res. 41:e140. doi: 10.1093/nar/gkt444
Messerer, M., Fischer, W., and Schubert, S. (2017). Investigation of horizontal gene transfer of pathogenicity islands in Escherichia coli using next-generation sequencing. PLoS One 12:e0179880. doi: 10.1371/journal.pone.0179880
Mikolčević, P., Hloušek-Kasun, A., Ahel, I., and Mikoč, A. (2021). ADP-ribosylation systems in bacteria and viruses. Comput. Struct. Biotechnol. J. 19, 2366–2383. doi: 10.1016/j.csbj.2021.04.023
Nayfach, S., Shi, Z. J., Seshadri, R., Pollard, K. S., and Kyrpides, N. C. (2019). New insights from uncultivated genomes of the global human gut microbiome. Nature 568, 505–510. doi: 10.1038/s41586-019-1058-x
Osborn, A. M., and Böltner, D. (2002). When phage, plasmids, and transposons collide: genomic islands, and conjugative- and mobilizable-transposons as a mosaic continuum. Plasmid 48, 202–212. doi: 10.1016/s0147-619x(02)00117-8
Ozer, E. A., Allen, J. P., and Hauser, A. R. (2014). Characterization of the core and accessory genomes of Pseudomonas aeruginosa using bioinformatic tools spine and AGEnt. BMC Genomics 15:737. doi: 10.1186/1471-2164-15-737
Panwar, S., Kumari, S., Verma, J., Bakshi, S., Narendrakumar, L., Paul, D., et al. (2023). Toxin-linked mobile genetic elements in major enteric bacterial pathogens. Gut Microbiome 4:E5. doi: 10.1017/gmb.2023.2
Partridge, S. R., Kwong, S. M., Neville, F., and Jensen, S. O. (2018). Mobile genetic elements associated with antimicrobial resistance. Clin. Microbiol. Rev. 31:e00088-17. doi: 10.1128/CMR.00088-17
Price, M. N., Dehal, P. S., and Arkin, A. P. (2010). FastTree 2--approximately maximum-likelihood trees for large alignments. PLoS One 5:e9490. doi: 10.1371/journal.pone.0009490
Rendueles, O., de Sousa, J. A. M., Bernheim, A., Touchon, M., and Rocha, E. P. C. (2018). Genetic exchanges are more frequent in bacteria encoding capsules. PLoS Genet. 14:e1007862. doi: 10.1371/journal.pgen.1007862
Reyes, A., Wu, M., McNulty, N. P., Rohwer, F. L., and Gordon, J. I. (2013). Gnotobiotic mouse model of phage -bacterial host dynamics in the human gut. Proc Natl Acad Sci USA 110:20236–20241. doi: 10.1073/pnas.1319470110
Roux, S., Enault, F., Hurwitz, B. L., and Sullivan, M. B. (2015). VirSorter: mining viral signal from microbial genomic data. PeerJ 3:e985. doi: 10.7717/peerj.985
Saak, C. C., Dinh, C. B., and Dutton, R. J. (2020). Experimental approaches to tracking mobile genetic elements in microbial communities. FEMS Microbiol. Rev. 44, 606–630. doi: 10.1093/femsre/fuaa025
Salyers, A. A., Shoemaker, N. B., Stevens, A. M., and Li, L. Y. (1995). Conjugative transposons: an unusual and diverse set of integrated gene transfer elements. Microbiol. Rev. 59, 579–590. doi: 10.1128/mr.59.4.579-590.1995
Schlesinger, D. J., Shoemaker, N. B., and Salyers, A. A. (2007). Possible origins of CTnBST, a conjugative transposon found recently in a human colonic Bacteroides strain. Appl. Environ. Microbiol. 73, 4226–4233. doi: 10.1128/AEM.00455-07
Schmidt, H., Bielaszewska, M., and Karch, H. (1999). Transduction of enteric Escherichia coli isolates with a derivative of Shiga toxin 2-encoding bacteriophage phi3538 isolated from Escherichia coli O157:H7. Appl. Environ. Microbiol. 65, 3855–3861. doi: 10.1128/AEM.65.9.3855-3861.1999
Shannon, P., Markiel, A., Ozier, O., Baliga, N. S., Wang, J. T., Ramage, D., et al. (2003). Cytoscape: a software environment for integrated models of biomolecular interaction networks. Genome Res. 13, 2498–2504. doi: 10.1101/gr.1239303
Shoemaker, N. B., Vlamakis, H., Hayes, K., and Salyers, A. A. (2001). Evidence for extensive resistance gene transfer among Bacteroides spp. and among Bacteroides and other genera in the human Colon. Appl. Environ. Microbiol. 67, 561–568. doi: 10.1128/AEM.67.2.561-568.2001
Simon, N. C., Aktories, K., and Barbieri, J. T. (2014). Novel bacterial ADP-ribosylating toxins: structure and function. Nat. Rev. Microbiol. 12, 599–611. doi: 10.1038/nrmicro3310
Smillie, C., Garcillán-Barcia, M. P., Francia, M. V., Rocha, E. P. C., and de la Cruz, F. (2010). Mobility of plasmids. Microbiol. Mol. Biol. Rev. 74, 434–452. doi: 10.1128/MMBR.00020-10
Tansirichaiya, S., Goodman, R. N., Guo, X., Bulgasim, I., Samuelsen, Ø., Al-Haroni, M., et al. (2022). Intracellular transposition and capture of Mobile genetic elements following intercellular conjugation of multidrug resistance conjugative plasmids from clinical Enterobacteriaceae isolates. Microbiol. Spectr. 10, e02140–e02121. doi: 10.1128/spectrum.02140-21
Usyk, M., Pandey, A., Hayes, R. B., Moran, U., Pavlick, A., Osman, I., et al. (2021). Bacteroides vulgatus and Bacteroides dorei predict immune-related adverse events in immune checkpoint blockade treatment of metastatic melanoma. Genome Med. 13:160. doi: 10.1186/s13073-021-00974-z
Vogtmann, E., Hua, X., Zeller, G., Sunagawa, S., Voigt, A. Y., Hercog, R., et al. (2016). Colorectal Cancer and the human gut microbiome: reproducibility with whole-genome shotgun sequencing. PLoS One 11:e0155362. doi: 10.1371/journal.pone.0155362
Waters, J. L., and Salyers, A. A. (2013). Regulation of CTnDOT conjugative transfer is a complex and highly coordinated series of events. mBio 4, e00569–e00513. doi: 10.1128/mBio.00569-13
Wexler, H. M. (2007). Bacteroides: the good, the bad, and the nitty-gritty. Clin. Microbiol. Rev. 20, 593–621. doi: 10.1128/CMR.00008-07
Wood, D. E., Lu, J., and Langmead, B. (2019). Improved metagenomic analysis with kraken 2. Genome Biol. 20:257. doi: 10.1186/s13059-019-1891-0
Wood, M. M., and Gardner, J. F. (2015). The integration and excision of CTnDOT. Microbiol. Spectr. 3:MDNA3–0020–2014. doi: 10.1128/microbiolspec.MDNA3-0020-2014
Yoshida, N., Emoto, T., Yamashita, T., Watanabe, H., Hayashi, T., Tabata, T., et al. (2018). Bacteroides vulgatus and Bacteroides dorei reduce gut microbial lipopolysaccharide production and inhibit atherosclerosis. Circulation 138, 2486–2498. doi: 10.1161/CIRCULATIONAHA.118.033714
Keywords: Phocaeicola vulgatus , Bacteroidota, conjugative transposon, horizontal gene transfer, helix-turn-helix motif, ADP-ribosylglycohydrolase, Tn mutagenesis mobilization method
Citation: Ortañez J and Degnan PH (2024) Tracking and characterization of a novel conjugative transposon identified by shotgun transposon mutagenesis. Front. Microbiol. 15:1241582. doi: 10.3389/fmicb.2024.1241582
Edited by:
Vasco Ariston De Carvalho Azevedo, Federal University of Minas Gerais, BrazilReviewed by:
Susu He, Dartmouth College, United StatesSilas Onyango Awuor, MOH-KISUMU County, Kenya
Copyright © 2024 Ortañez and Degnan. This is an open-access article distributed under the terms of the Creative Commons Attribution License (CC BY). The use, distribution or reproduction in other forums is permitted, provided the original author(s) and the copyright owner(s) are credited and that the original publication in this journal is cited, in accordance with accepted academic practice. No use, distribution or reproduction is permitted which does not comply with these terms.
*Correspondence: Patrick H. Degnan, cGF0cmljay5kZWduYW5AdWNyLmVkdQ==