- 1Saneamiento Ecológico, Salud y Medio Ambiente, Universidad El Bosque, Vicerrectoría de Investigaciones, Bogotá, Colombia
- 2Grupo de Virología, Universidad El Bosque, Vicerrectoría de Investigaciones, Bogotá, Colombia
Arboviral infections transmitted by Aedes spp. mosquitoes are a major threat to human health, particularly in tropical regions but are expanding to temperate regions. The ability of Aedes aegypti and Aedes albopictus to transmit multiple arboviruses involves a complex relationship between mosquitoes and the virus, with recent discoveries shedding light on it. Furthermore, this relationship is not solely between mosquitoes and arboviruses, but also involves the mosquito microbiome. Here, we aimed to construct a comprehensive review of the latest information about the arbovirus infection process in A. aegypti and A. albopictus, the source of mosquito microbiota, and its interaction with the arbovirus infection process, in terms of its implications for vectorial competence. First, we summarized studies showing a new mechanism for arbovirus infection at the cellular level, recently described innate immunological pathways, and the mechanism of adaptive response in mosquitoes. Second, we addressed the general sources of the Aedes mosquito microbiota (bacteria, fungi, and viruses) during their life cycle, and the geographical reports of the most common microbiota in adults mosquitoes. How the microbiota interacts directly or indirectly with arbovirus transmission, thereby modifying vectorial competence. We highlight the complexity of this tripartite relationship, influenced by intrinsic and extrinsic conditions at different geographical scales, with many gaps to fill and promising directions for developing strategies to control arbovirus transmission and to gain a better understanding of vectorial competence. The interactions between mosquitoes, arboviruses and their associated microbiota are yet to be investigated in depth.
1 Introduction
Only ~1% of Aedes spp. are involved in transmitting pathogens affecting human health. Aedes aegypti and Aedes albopictus are the main vectors of arboviruses, such as dengue (DENV), Zika (ZIKV), yellow fever (YFV), and chikungunya (CHIKV) (Wilkerson et al., 2015; Houé et al., 2019a). Their role as vectors is linked to their ability to allow viral development, become infected, and then transmit it to a susceptible host, known as vectorial competence, and the ability of the virus to overcome the mosquito's immune response (Moncada et al., 2021). The mosquito microbiota (bacteria, fungi, and viruses) can influence the complex relationship between mosquitoes and arboviruses (Vega-Rua et al., 2014; Pang et al., 2016; Caragata et al., 2019; Houé et al., 2019b; Yin et al., 2020). Understanding this relationship is becoming increasingly important, as it is a great source of information for developing effective strategies to control arbovirus transmission. This review identifies the generalities of arbovirus infection in mosquito's cells and Aedes immune responses to arboviral infections, focusing on the most recent discoveries. We then discuss the presence and sources of their microbiota and how this affects their vectorial competence; we also identify the knowledge gaps and suggest perspectives for future research.
2 Mosquitoes and arbovirus
2.1 Arbovirus infection in mosquitoes and insect cells
Arbovirus acquisition in mosquitoes begins with the ingestion of an infected blood meal. Once in the midgut, the viral particles are exposed to trypsin produced during blood digestion (Noriega and Wells, 1999), which destroys the viral envelope. Despite that the peritrophic matrix (PM) does not act as a barrier for arbovirus infections, a peroxidase that mediates PM formation, enhance arbovirus infections through oxidative stress regulation (Talyuli et al., 2023), to infect the midgut epithelial cells (the first target of infection), the virus must first pass through the cellular mucin layer. Midgut cells and their immunological responses contribute to the establishment of a midgut infection barrier (MIB) (Moncada et al., 2021).
Once the virus escapes the MIB (dissemination capacity), it enters the hemocoel (open circulatory system in the mosquito) and can infect circulatory cells, including granulocytes (the main phagocytic cells), enocytes (involved in melanization), and prohemocytes (stem cells with phagocytic capacity) (Castillo et al., 2006). Prohemocytes are the main targets of DENV infection (Cheng et al., 2022), suggesting their role as viral amplifiers. In addition, the expression of specific lectins by hemocytes favors the infection of other tissue cells (Cardoso-Jaime et al., 2022), leading to their spread to the adipose tissue, nervous system where the viral infection is controlled by the neural factor Hikaru genki (AaHig) to avoid deleterious effects on the mosquito (Xiao et al., 2015), and ovarioles (involved in vertical transmission). Infection of salivary gland epithelial cells and escape to saliva by passing through the salivary gland infection barrier (SGIB) allows for viral inoculation during the next bite (Moncada et al., 2021). Recently, it has been proposed that mosquito bites may inject not only viral particles but also extracellular vesicles containing viral RNAs or proteins that could infect or modulate the infection of vertebrate cells, changing our understanding of the infection process.
2.2 Viral infection in mosquito cells
The viral envelope (E) protein binds to various mosquito cell receptors such as HSC70, laminin union proteins, enolase, and prohibitins, depending on the virus and cell type (Sakoonwatanyoo et al., 2006; Cheng et al., 2010; Kuadkitkan et al., 2010; Liu et al., 2014; Ghosh et al., 2018). Wide tissue expressing C-type lectins may also help the DENV E protein bind to mosquito tyrosine phosphatase receptors (Cheng et al., 2010). After binding, the virus is internalized via clathrin-mediated endocytosis (Figure 1B-2), and the drop in endosome pH triggers E dimer dissociation, exposing the hydrophobic domains and leading to membrane fusion and viral genomic RNA (vgRNA)-capsid release (Figure 1B-3). The A226V mutation in the E1 protein of CHIKV boosts infection of A. albopictus cells by increasing cholesterol affinity (Tsetsarkin et al., 2007).
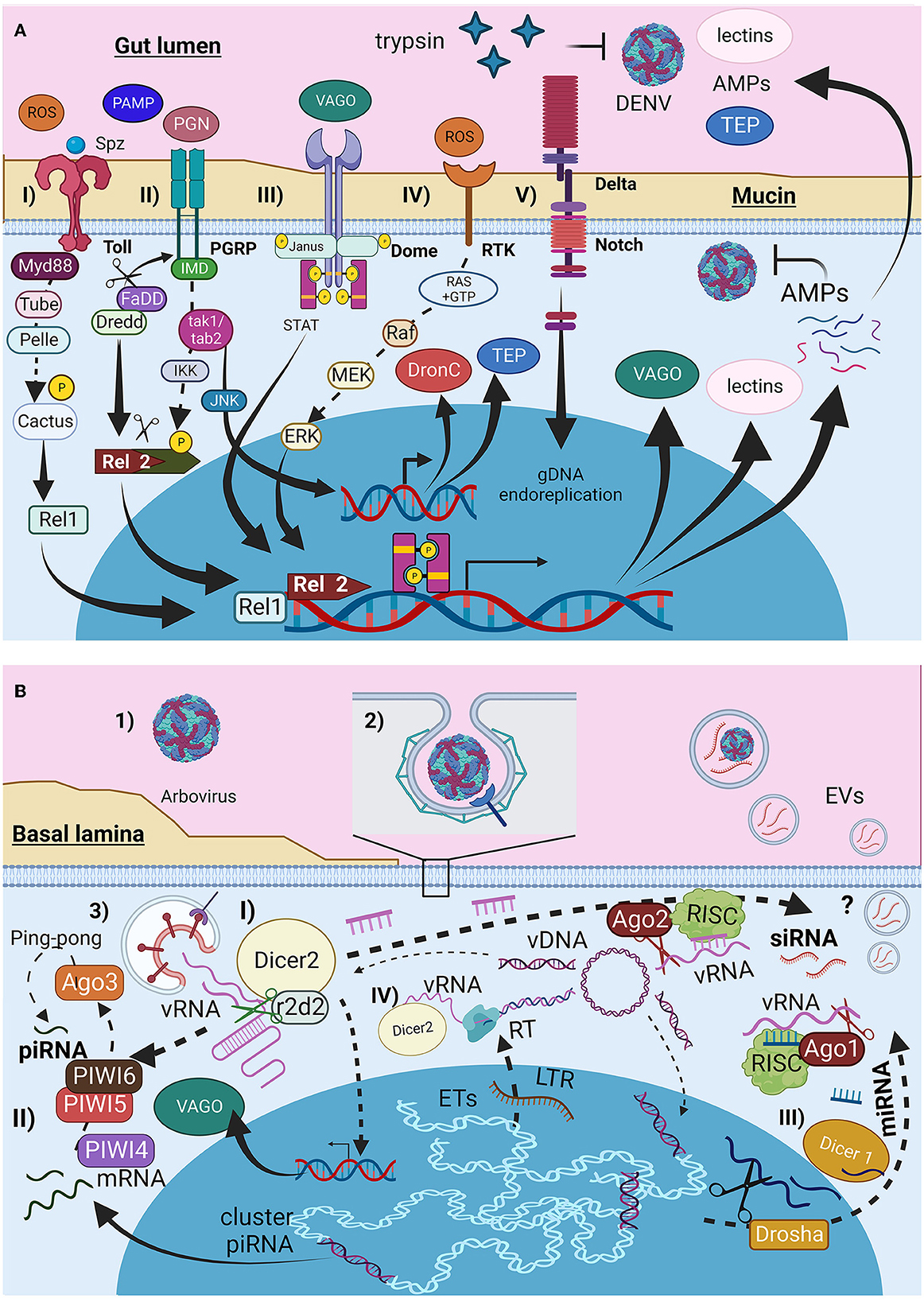
Figure 1. Infection barriers and immunological mosquito cellular response to arbovirus infection. (A) Immunological signal pathways (I) Toll receptors, (II) IMD, (III) Dome (JAK-STAT), (IV) tyrosine-kinase receptors (RTK), and (V) Notch pathway. (B) Viral entry and RNAi responses produced by mosquitoes cells. (I) small interference RNAs siRNA, (II) Piwi RNAs piRNA, and (III) microRNAs miRNA. Figure produced in BioRender.com.
The viral genomic RNA (vgRNA) of DENV, ZIKV, YFV, and CHIKV are positive-stranded and encodes a viral polyprotein, including non-structural and structural proteins. Non-structural proteins with protease activity release themselves, and other non-structural proteins, such as RNA-dependent RNA polymerase, are responsible for synthesizing vgRNA and subgenomic RNA (sgRNA). Post-translational modifications of E-related proteins occur in the endoplasmic reticulum, Golgi complex, and plasma membrane. In the cytoplasm, capsid proteins interact with the vgRNA to package it, and this complex moves to the cell membrane in the presence of E proteins to assemble and release viral particles (Velandia and Castellanos, 2011; Calvo et al., 2021).
Cell-secreted extracellular vesicles (EVs) have been proposed as a complementary mechanism through which arboviruses infect arthropods and vertebrates (Reyes-Ruiz et al., 2020; Sultana and Neelakanta, 2020). EVs are used for cell-to-cell communication and carry DNA, RNA, and proteins (Teng and Fussenegger, 2021), and EVs produced by infected mosquito cells have been reported to carry viral RNA fragments and proteins that can infect mosquitoes or mammalian cells. This infection strategy helps to reduce the cytopathic effect and avoid the immune response in mosquitoes and vertebrate hosts (Reyes-Ruiz et al., 2020). Additionally, the protein cargo of EVs changes in DENV-infected cells, increasing the presence of proteins that can enhance subsequent viral infections (Gold et al., 2020). These vesicles can be transported through cell-cell junctions (Cheng et al., 2020) or released into the extracellular space (Vora et al., 2018).
2.3 The mosquito immune response
The cellular response to viral pathogens involves phagocytosis and encapsulation of pathogens by hemocytes and the production of antimicrobial peptides (AMPs), reactive oxygen species (ROS), complement proteins (CP) such as opsonins, and thioester-containing proteins (TEPs) (Figure 1A). These responses are mediated through the Toll pathway (Figure 1A-I), the immune deficiency (Imd) pathway (Figure 1A-II), and the JAK/STAT signaling pathway (Figure 1A-III); their roles in the mosquito immune response have been well-described and reviewed previously (Shaul and Seger, 2007; Xi et al., 2008; Fragkoudis et al., 2009; Xiao et al., 2014; Cheng et al., 2016; Simões et al., 2018; Wang et al., 2019; Chowdhury et al., 2020; Liu et al., 2020; Rosendo Machado et al., 2021; Prince et al., 2023). It has been reported that these pathways are activated during DENV and ZIKV infections, but not CHIKV infections, in A. aegypti and A. albopictus cell lines (Xi et al., 2008; Houé et al., 2019a; Abduljalil and Abd Al Galil, 2022). Other additional pathways were identified in the Aedes mosquito cells. The first pathway is the mitogen-activated protein kinase (MAPK) pathway (Figure 1A-IV), mediated by receptor tyrosine kinase (RTK), which is activated by ROS (Horton et al., 2011; Plotnikov et al., 2011; Arthur and Ley, 2013), and signals Ras proteins to hydrolyze GTP, leading to Raf, MEK, and ERK activation and AMPs production (Shaul and Seger, 2007; Liu et al., 2020). The delta-Notch pathway (Figure 1A-V) activates Notch receptors in midgut cells, triggering Notch cleavage and endoreplication of genetic material without cell division, thereby increasing antiviral and immune gene expression (Serrato-Salas et al., 2018).
2.4 The interference RNA response
The main antiviral response in insects is mediated by interfering RNA (iRNA) such as small interfering RNA (siRNAs) (Figure 1B-I), PIWI-interacting RNA (piRNAs) (Figure 1B-II), and microRNAs (miRNAs) (Figure 1B-III) (Olson and Blair, 2015; Cheng et al., 2016; Tassetto et al., 2017; Silverman et al., 2019; Rosendo Machado et al., 2021; Dong et al., 2022). These responses are activated by the double-stranded RNA present in the secondary structures of vgRNA, RNA intermediates, or mosquito cell transcripts from specific genomic regions. They can be transferred to other cells via GAP junctions, cytoplasmic bridges, or EVs. EVs produced by Drosophila hemocytes release siRNA during localized tissue infection as an immunological response (Tassetto et al., 2017). Dicer2 expression during siRNA synthesis induces vago transcription (Kingsolver and Hardy, 2012).
Additionally, reverse transcriptase (RT) from autonomous transposable elements (TEs) can reverse transcribe viral RNA (vRNA) into Intermediate Viral DNA (vDNA). Dicer2 and its helicase subunit recognize and facilitate interactions between vRNA and RT. The vDNA is endogenized by the integrase produced by the same autonomous TEs to be integrated into non-retroviral endogenous elements (NERVE) (Figure 1B-IV) for piRNA and miRNA production or remains in the cytoplasmic region as linear or circular vDNA serving as a template for siRNA (Houé et al., 2019a). This adaptive immune mechanism can be inherited (Goic et al., 2016; Mukherjee et al., 2019; Mondotte et al., 2020). The presence of TEs is typically considered deleterious. However, TEs represent almost half of A. aegypti and A. albopictus genomes (Houé et al., 2019b). Furthermore, TEs, especially autonomous TE-like long terminal repeats, appear to be active, as evidenced by the genetic richness of these elements in both mosquito species (de Melo and Wallau, 2020). This evidence suggests that despite the potential cost of TE activity, mosquitoes use it to develop adaptive immunity to tolerate arboviral infections.
2.5 The mosquito microbiota
Mosquitoes are holometabolous insects with differential exposure to microorganisms throughout their lives (Coon et al., 2014, 2016; Correa et al., 2018; Scolari et al., 2019; Tawidian et al., 2021), and their microbiota include bacteria, viruses, fungi, and archaea (Dickson et al., 2017, 2018; Öhlund et al., 2019; Tawidian et al., 2021). A. aegypti and A. albopictus breeding sites are usually small natural or artificial water bodies that can be clean or polluted (Chandrasiri et al., 2020). The physicochemical characteristics of the breeding site influence the richness and diversity of the microbial composition in the water and mosquitoes (Gusmão et al., 2007; Yadav et al., 2015; Bozic et al., 2017; Dickson et al., 2017; Mancini et al., 2018; Bogale et al., 2020; Tawidian et al., 2021).
The microbiota composition varies depending on the sex of the mosquito, developmental stage, larvae diet, blood source, ecology, geographical location, temperature, mosquito genetics, and metabolism (Minard et al., 2018; Parry et al., 2018; Bogale et al., 2020; Onyango et al., 2020; MacLeod et al., 2021; Pérez-Ramos et al., 2022; Sarma et al., 2022; Ratnayake et al., 2023; Rodpai et al., 2023). Water microbiota composition is also influenced by larval feces and the egestion of microorganisms by females during oviposition (Guégan et al., 2018; Scolari et al., 2019, 2021). However, most mosquito larval microbiota are lost in adults owing to meconium ingestion. Maternally transmitted microorganisms create a core microbiota associated with each species regardless of their geographic distribution (Yadav et al., 2015; Mancini et al., 2018; Scolari et al., 2021; Olmo et al., 2023). Wolbachia bacteria, Microsporium fungi, insect-specific viruses, and arboviruses are vertically transmitted microorganisms (Werren, 1997; Duguma et al., 2015; Velandia-Romero et al., 2017; Dickson et al., 2018; Scolari et al., 2019; Sicard et al., 2019).
Bacterial composition in adults are an important part of midgut microbiota with specific and common bacteria genera composition (Supplementary Figure 1) (Yadav et al., 2015; Raharimalala et al., 2016; Dickson et al., 2018; Hegde et al., 2018; Rosso et al., 2018; Thongsripong et al., 2018; Bennett et al., 2019; Arévalo-Cortés et al., 2020; Molina-Henao et al., 2020; Ramos-Nino et al., 2020; Seabourn et al., 2020; Balaji et al., 2021; Díaz et al., 2021; Lin et al., 2021; Scolari et al., 2021; Rau et al., 2022; Sarma et al., 2022; Al-Ghamdi et al., 2023; Baltar et al., 2023; Martinez Villegas et al., 2023; Mosso González et al., 2023; Rodpai et al., 2023). Wolbachia is the most characterized bacteria in Aedes mosquitos (Supplementary Table 1 and Supplementary Figure 1) (Kitrayapong et al., 2002; Ravikumar et al., 2010; Tortosa et al., 2010; de Albuquerque et al., 2011; Wiwatanaratanabutr, 2013; Joanne et al., 2015; Noor Afizah et al., 2015; Raharimalala et al., 2016; Ahmad et al., 2017; Nugapola et al., 2017; Soni et al., 2017; Chuchuy et al., 2018; Goindin et al., 2018; Hegde et al., 2018; Thongsripong et al., 2018; Anderson et al., 2019; Balaji et al., 2019; Carvajal et al., 2019; Kulkarni et al., 2019; Mohanty et al., 2019; Shaikevich et al., 2019; Ding et al., 2020; Hu et al., 2020; Puerta-Guardo et al., 2020; Torres-Monzón et al., 2020; Lin et al., 2021; Duque-Granda et al., 2022; Roslan et al., 2022; Sasaki et al., 2022; Zhang et al., 2022; Bueno-Marí et al., 2023; Chao and Shih, 2023; Li et al., 2023; Ruiz et al., 2023; Somia et al., 2023; Vinayagam et al., 2023). Due to its ability to affects insect reproduction and genetic diversity (Sicard et al., 2019; Sinha et al., 2019).
A. albopictus could have a Wolbachia pipientis prevalence of up to 90% in some regions, and wAlbA and wAlbB are the two prominent strains. Although A. aegypti it has been artificially infected with Drosophila melanogaster (wMel and wMelpop) and A. albopictus Wolbachia strains (Moreira et al., 2009; Walker et al., 2011), with reports of some populations with natural infections (Supplementary Figure 1) (Thongsripong et al., 2018; Balaji et al., 2019; Carvajal et al., 2019; Kulkarni et al., 2019; Zhang et al., 2022; Chao and Shih, 2023; Somia et al., 2023; Vinayagam et al., 2023).
Furthermore, mosquitoes are exposed to several arboviruses and insect-specific viruses (ISVs) (Supplementary Figure 2 and Supplementary Table 1). ISVs have been described in the Birnaviridae, Bunyaviridae, Mesoniviridae, Negeviridae, Nodaviridae, Reoviridae, Rhabdoviridae, Togaviridae, Tymoviridae, and Flaviviridae families. They only infect insects and are vertically transmitted (Bolling et al., 2015; Hoyos-López et al., 2016; Guzman et al., 2018; Salim Mattar and Marco González, 2018; Öhlund et al., 2019; Laiton-Donato et al., 2023).
Flaviviridae has the highest ISV richness, and it has been proposed that the arbovirus originates in ISV adapting to vertebrate hosts with the development of hematophagy by some arthropods (Bolling et al., 2015; Guzman et al., 2018; Öhlund et al., 2019). The specificity of ISV to invertebrate hosts is related to genetic, structural, immunological, and microclimatic conditions (Elrefaey et al., 2020). In addition, multiple infections with arboviruses such as DENV, ZIKV, YFV, and CHIKV have been found in field-collected mosquitoes, with evidence of vertical transmission (Velandia-Romero et al., 2017; Alencar et al., 2021; Mantilla-Granados et al., 2022). Thus, arboviruses are a part of the normal microbiota of these insect vectors, making them reservoirs.
2.6 Arbovirus-mosquito microbiota relationships
The midgut lumen is the mosquito compartment with the highest presence of microbiota, consisting mainly of commensal microorganisms that have developed different strategies to resist chemical and enzymatic activity, and the mosquito immune response mediated by broad-spectrum AMPs expressed to different pathogen-associated molecular patterns (PAMPS) (Caragata et al., 2019), which could favor or limit the development of arboviruses. For example, Talaromyces sp. produces specific metabolites that reduce the transcription of trypsin peptidases, restricting trypsin activity in the gut to ensure their development (Figure 2A-I). This indirectly favors DENV mosquito infections (Angleró-Rodríguez et al., 2017). Other gut mosquitoes fungus like Zancudomyces culisetae also change bacteria composition at midgut (Frankel-Bricker, 2020), probably affecting bacterial effect on arbovirus infection.
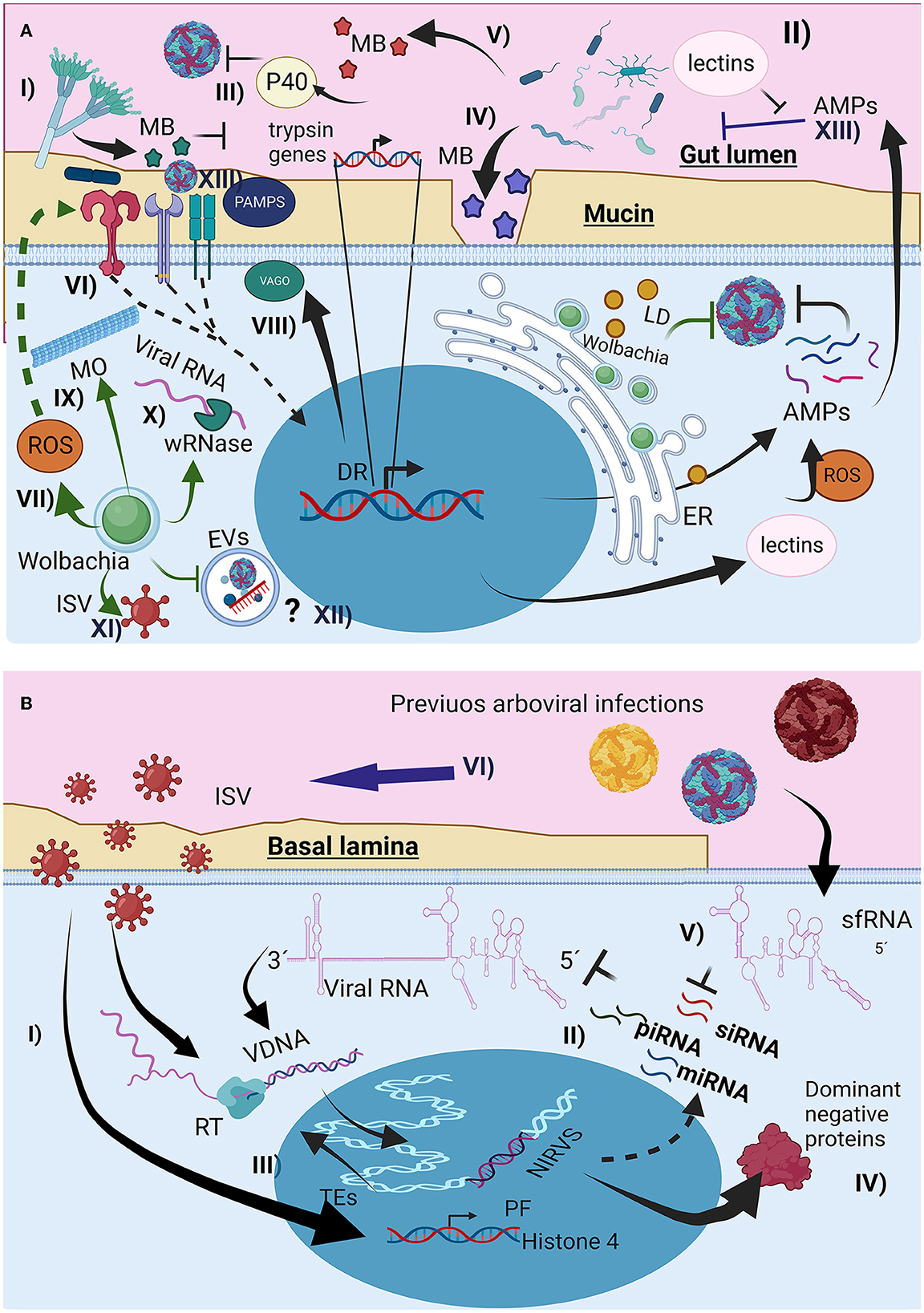
Figure 2. The interaction of the microbiota with the mosquito immunological response affects arboviral infection. (A) Direct and indirect mechanism of the microorganism could interfere with arbovirus infection, (I) through metabolites (MB) lumen fungus could affect trypsin production, favoring arbovirus survival, (II) change in lectin production, affecting AMPs recognition, and arbovirus entrance (III) production of proteins like p40 facilitating arbovirus recognition by host cells, (IV) MB production that destroy arbovirus envelope, (V) MB that degrade mucin layer, (VI) Immune priming through canonical immunological pathways, increasing AMPs and ROS production, (VII) Wolbachia presence increase oxidative stress, activating Toll pathway, (VIII) Microtubules reorganization induced by Wolbachia presence affecting vesicular traffic, (IX) Wolbachia Rnase production, destroying viral RNA, (X) Wolbachia could also compete with arbovirus for resources like lipids inducing its storage as lipid droplets (LD), (XI) Wolbachia infection can change the interaction between the arbovirus and the insect specific viruses (ISV), (XII) potential effect of Wolbachia infection on the extracellular vesicles infection mechanism used by the arboviruses, (XIII) Changes of microbiota composition by the arbovirus infections, associated to the immunological response activation and AMPs production. (B) Interactions of previous viral infections with new arboviral infection, (I), immune priming mediated by interference RNA (iRNAs), (II) Endogenization of non-retroviral elements (NIRVS) from viral RNA and ISV RNA, through the insect autonomous transposable elements (TEs) and its retrotranscriptase (RT), creating viral intermediaries DNA (VDNA) for i RNA production, (III) Overexpression of proviral proteins for ISV infection, (IV) Synthesis of non-functional viral proteins, promoting non-functional viral particles, (V) subgenomic flaviviral RNAs (sfRNA) interfere with iRNAs responses, (VI) arbovirus infection can also change ISV presence (mostly enhance), probably by the changes on mosquito immunological response like interfering with iRNA response.
Mosquito commensal homeostatic bacteria can change after blood digestion, altering the bacterial diversity, fungus susceptibility, ROS, and AMPs production in mosquitoes (Xiao et al., 2017; Wang et al., 2019; Cabral et al., 2020). To protect themselves and restore gut microbiota homeostasis, bacteria alter lectin production through the Imd pathway, involving classical NF-κB immune signaling, to increase lectins, which protect the commensal microbiota from AMPs activity (Figure 2A-II) (Pang et al., 2016). Changes in lectin composition also affect the ability of arboviruses to infect mosquito cells. Similarly, Serratia odorifera infection increased the production of the P40 protein (Figure 2A-III), which mediates the interaction of CHIKV and DENV envelope proteins with cellular prohibitin receptors, favoring viral entry. Presence of ZIKV in blood-meal favors bacteria diversity, favoring some bacteria genera, probably through mosquito-immune regulation and changes on bacteriophage load (Shi et al., 2022).
Serratia marcescens gut bacteria secrete SmEnhancin (Figure 2A-IV), which weakens the mucin layer and increases DENV infectivity in A. aegypti (Wu et al., 2019), whereas Chromobacterium spp. aminopeptidases and lipase production (Figure 2A-V) degrade the DENV envelope (Ramirez et al., 2014; Yu et al., 2022), S. marcescens can also affects mosquito larval survival and adult microbiota composition (Heu et al., 2021). Midgut microorganisms limit arboviral development by activating immunological pathways and producing broad-spectrum AMPs, ROS, and opsonins (Xi et al., 2008). For instance, Proteus spp. increase AMPs production by activating the Toll pathway, thus reducing DENV infection (Figure 2A-VI), and Beauveria bassiana fungus in A. aegypti activates the JAK-STAT and Toll pathways, thereby increasing resistance to DENV (Dong et al., 2012). In contrast, DENV can induce AMPs production to reduce gut microbiota (Ramirez et al., 2012), ZIKV has also been reported to modulate microbiota composition (Arévalo-Cortés et al., 2022). Microbiota bacteria also affect the development of Serratia (Kozlova et al., 2021).
The effect of Wolbachia has different mechanism for restrict arbovirus transmission, indirect and mediated by innate immunological priming (Figure 2A-VII) (Pan et al., 2012). Additionally, Wolbachia hijacks the cellular resources from the host cells (e.g., amino acids, nucleotides, cell machinery, phospholipids, and cholesterol), affecting their availability (Frentiu, 2017; Geoghegan et al., 2017; Lindsey et al., 2018; Teramoto et al., 2019), interfering arbovirus infection, replication and assembly. Furthermore, Wolbachia causes endoplasmic reticulum stress, disrupting viral envelope protein processing. The bacteria also alters microtubule organization (Figure 2A-VIII) and vesicular trafficking (Ferree et al., 2005), a key factor in arbovirus entry (Velandia and Castellanos, 2011; Calvo et al., 2021), and Wolbachia wRNase HI degrades DENV RNA, suppressing early infection (Hussain et al., 2023b), Wolbachia could also affects EVs biogenesis and their involvement in arbovirus infection.
Not all Wolbachia strains have the same arboviral restriction. For example, wAlbA does not have an inhibitory effect, whereas wAlbB restricts salivary glands infection in A. albopictus (Mousson et al., 2012). Compared to wMel and wMelPop, wAlbB had a lower suppressive effect on DENV infection in A. aegypti (Flores et al., 2020). Additionally, Wolbachia changes midgut bacterial composition (Audsley et al., 2018), but increase ISV-flavivirus presence (Amuzu et al., 2018; Balaji et al., 2021). The wMel infection seems to be genetically stable in A. aegypti, as well as their levels of infection and their capacity to restrict arbovirus infection at population level (Frentiu et al., 2014; Ford et al., 2019; Ahmad et al., 2021). However, as the Wolbachia tissue infection is not homogeneous, its arbovirus restriction is cell-dependent, and the arbovirus restriction capacity in the mosquito is not complete and change among arbovirus and serotypes (Carrington et al., 2017; Flores et al., 2020; Fraser et al., 2020), this opens the possibility for emerging and selection of Wolbachia-resistant arboviruses. However, the likelihood of this event is low but not impossible (Edenborough et al., 2021), as Wolbachia use different mechanism to interfere with arbovirus development, it makes difficult for the virus to overcome all. At population level probably if Wolbachia-resistant arbovirus emerge, the performance of Wolbachia strategy to reduce arbovirus transmission will be compromise.
A. aegypti and A. albopictus are commonly infected with ISVs (Supplementary Figure 2 and Supplementary Table 1) (Hoshino et al., 2009; Rizzo et al., 2014; Vasilakis et al., 2014; Chandler et al., 2015; Fan et al., 2016; Fernandes et al., 2016; Sadeghi et al., 2017; Ajamma et al., 2018; Fang et al., 2018, 2021a,b; Iwashita et al., 2018; Parry et al., 2018, 2021; Zakrzewski et al., 2018; Zhang et al., 2018; Gravina et al., 2019; Martin et al., 2019, 2020; Shi et al., 2019; Baidaliuk et al., 2020; da Silva Ferreira et al., 2020; Diagne et al., 2020; Jeffries et al., 2020; Kubacki et al., 2020; Ramos-Nino et al., 2020; Ribeiro et al., 2020; Supriyono et al., 2020; Thannesberger et al., 2020; Batson et al., 2021; Chiuya et al., 2021; Hameed et al., 2021; He et al., 2021; Munivenkatappa et al., 2021; Nebbak et al., 2021; Yezli et al., 2021; Calle-Tobón et al., 2022; Coatsworth et al., 2022; Duarte et al., 2022; Oguzie et al., 2022; Palatini et al., 2022; Aragão et al., 2023; Bennouna et al., 2023) that may alter their vectorial competence (Bolling et al., 2015; Öhlund et al., 2019). Phasi Charoen-like virus (PCLV) and Humaita Tubiacanga virus (HTV) are common ISVs found in A. aegypti, and A. albopictus (Supplementary Figure 2). Furthermore, their cocirculation is linked to DENV endemic zones, and in vitro PCLV and HTV infection in mosquito cells increases the expression of histone 4, a proviral factor (Olmo et al., 2023). However, another study found that PCLV infection of Aag2 cells conferred a DENV infection-resistant phenotype after wAlbB was cleared, highlighting a complex relationship between ISV and Wolbachia (Hussain et al., 2023a). Mosquito densoviruses also decrease DENV2 vectorial competence in A. albopictus mosquitoes (Kong et al., 2023).
Previous exposure to inactivated DENV during the larval stage has been shown to confer protection against subsequent DENV infections in A. aegypti females, and their generations can inherit resistance (Mondotte et al., 2020; Vargas et al., 2020). The mechanism of this adaptive immunity appears to be mediated by siRNA, piRNA, and miRNA (Figure 2B-II) via viral DNA (vDNA) intermediates produced by RT from TE (Figure 1B-IV, Figure 2B-III) (Houé et al., 2019a). vDNA endogenization can also occur in ISV RNAs, some of which are genetically related to arboviruses and serve as templates for iRNAs against conserved arboviral RNA sequences for degradation. Another mechanism by which vDNA endogenization affects viral development is the production of defective viral proteins from endogenous vDNA (Houé et al., 2019a), which compete with normal viral proteins during viral assembly (Figure 2B-IV).
Arbovirus co-infections, even from different arbovirus families, such as Flaviviridae (DENV, ZIKV, and YFV) and Togaviridae (CHIKV), are possible in wild Aedes spp. mosquitoes (Mantilla-Granados et al., 2022). Arboviruses can exhibit different strategies to evade the immunological response of mosquitoes. Flaviviruses can limits the iRNA-mediated through subgenomic flaviviral RNAs (sfRNAs; Yeh and Pompon, 2018). These non-coding regions have complex secondary structures that interfere with helicases from the host cell, recruiting and kidnapping the machinery for the recognition and degradation of vRNA, thus helping the virus spread (Pompon et al., 2017; Yeh and Pompon, 2018; Göertz et al., 2019). This evasion mechanism is conserved in ISV flaviviruses (Slonchak and Khromykh, 2018; Slonchak et al., 2022). It is possible that co-infection with ISV could also facilitate subsequent arbovirus infections by reducing the activity of iRNA with their sfRNA, this could also help to evade response through vDNA. On the other hand experimental co-infections with two arbovirus (DENV2 and ZIKV) have shown mutual enhancement, mediated by the non-structural protein NS5 (Lin et al., 2023).
3 Perspectives
3.1 In vitro and laboratory models
To get a better understanding of the factors involving on vectorial competence in order to design better control strategies, it is important to clarify the role of EVs in arbovirus infections, immunological response and arbovirus transmission to the vertebrate hosts, using a comprehensive evaluation and in vitro experimentation (Théry et al., 2018). Studies on mosquito cell lines, as well as primary cultures of midgut epithelial cells, salivary glands, and circulatory cells, can help us understand EVs function in arbovirus transmission to mosquitoes of mammal cells, as well as in the immune response as was demonstrate for Drosophila (Tassetto et al., 2017). In addition, it is important to characterize the potential role of Wolbachia and other microorganism on EVs biogenesis and trafficking, as this could be other mechanism to interfere arbovirus transmission. Also, a deeply characterization of the mosquito adaptive immune response involving endogenous TEs and DNA endoreplication, are key pieces to understand the vectorial competence. Another point to take into account for in-vitro models is the presence of ISV in insect cell lines or primary cell cultures (Fujita et al., 2018). Finally, for in-vivo experiments, the use of gnotobiotic or axenic mosquitoes is a powerful strategy to understand the effect of single and multiple microorganisms in the whole mosquito and it's the vectorial competence, helping to validate in vitro and fieldwork results (Romoli et al., 2021; Wu et al., 2023).
3.2 Field characterization
The characterization of mosquitoes microbiota in terms of fungi, bacteria, and viruses from different regions is important (Supplementary Figures 1–3), to get a better knowledge of the common microbiota presence, geographical patterns and to detected associations with arbovirus transmission (Olmo et al., 2023), being important to use different molecular markers (Frankel-Bricker and Frankel, 2022; Schrieke et al., 2022), with special focus on fungus since its characterization remains scarce (Angleró-Rodríguez et al., 2017; Zakrzewski et al., 2018; Luis et al., 2019; Guégan et al., 2020; Ramos-Nino et al., 2020) and go further characterizing the transcriptomic, proteomic and metabolomic networks, that are driving the interactions among the microbiota, with the mosquito and arboviruses. Another important topic is how the mosquitoes exposure to antibiotics could change their microbioma affecting its vectorial competence (Garrigós et al., 2023).
3.3 Microbiota as biocontrol strategies
The use of Wolbachia (wMel) is a successful and promising strategy for reducing arbovirus transmission (Aliota et al., 2016; Flores and O'Neill, 2018; Velez et al., 2020). However, it is important to characterize the interference mechanism with arbovirus development, as well as the fitness costs of the bacteria in their new host, interaction with the mosquito microbiota at the local level, the effect of local climatic conditions on Wolbachia infection, and interaction with other components of the mosquito microbiota. As A. albopictus is naturally infected, a meticulous characterization of Wolbachia strains in global populations is required. Furthermore, it is extremely important to characterize the potential mechanisms of the arbovirus to overcome the microbiota restriction pathways, since information is still scarce, not only for Wolbachia but also for other bacteria, ISV, and fungi, to guarantee the long-term success of microbiota use as transmission control strategies. Another microorganism like the ISV PCLV and densovirus, as well as the fungus Beauveria bassiana, or Serratia bacteria, have the potential to be used as control strategies to reduce arbovirus transmission, but more studies have to be conducted to prove their feasibility. The ISVs are also a promising biocontrol strategy for developing mosquito-mediated vaccines, not only for humans but also for wildlife or for changing vectorial competence. For example, by modifying the flaviviral ISV Chaoyang virus and replacing its premembrane and envelope proteins with those of ZIKV, a mosquito-delivered vaccine was developed to induce protective immunity against ZIKV in female mice (Wen et al., 2022), also mosquito cell-derived CHIKV-like particles have shown potential for vaccine development (Tsai et al., 2023).
Author contributions
JM-G: Conceptualization, Funding acquisition, Investigation, Methodology, Writing–original draft. JC: Conceptualization, Funding acquisition, Methodology, Supervision, Writing–review & editing. MV-R: Conceptualization, Funding acquisition, Methodology, Supervision, Writing–review & editing.
Funding
The author(s) declare financial support was received for the research, authorship, and/or publication of this article. This project was funded by Minciencias and Universidad El Bosque, contract 891-2019. The funding sources had no role in information review or preparation of the manuscript.
Acknowledgments
We want to thank to Ligia Moncada from Universidad Nacional de Colombia and Javier Escobar from Universidad El Bosque, for their comments during the conception of the manuscript. We would like to thank Editage (www.editage.com) for English language editing and the reviewers for their comments to improve the original manuscript.
Conflict of interest
The authors declare that the research was conducted in the absence of any commercial or financial relationships that could be construed as a potential conflict of interest.
Publisher's note
All claims expressed in this article are solely those of the authors and do not necessarily represent those of their affiliated organizations, or those of the publisher, the editors and the reviewers. Any product that may be evaluated in this article, or claim that may be made by its manufacturer, is not guaranteed or endorsed by the publisher.
Supplementary material
The Supplementary Material for this article can be found online at: https://www.frontiersin.org/articles/10.3389/fmicb.2023.1287519/full#supplementary-material
Supplementary Figure 1. Reported studies by august 2023, of bacterial microbiota in Aedes aegypti and Aedes albopictus adults, for database construction (Supplementary Table 1), only studies with well-established mosquitoes collection origin, adult characterization microbiota and bacteria genus level characterization were chosen. Maps were constructed using ArcGis Pro 2.8.3. The maps shows, the number studies reporting samples from each country. The most reported bacteria or the ones previously reported with arbovirus effect were chosen to be displayed at the map, for easily visual identification Aedes aegypti was marked green and Aedes albopictus pink.
Supplementary Figure 2. Reported studies by august 2023, of insect specific virus (ISV) microbiota in Aedes aegypti and Aedes albopictus adults, for database construction (Supplementary Table 1), only studies with well-established mosquitoes collection origin, adult characterization microbiota. Maps were constructed using ArcGis Pro 2.8.3. The maps shows, the number studies reporting samples from each country. The most reported ISV or the ones previously reported with arbovirus effect were chosen to be displayed at the map, for easily visual identification Aedes aegypti was marked green and Aedes albopictus pink.
Supplementary Figure 3. Reported studies by august 2023, of fungi microbiota in Aedes aegypti and Aedes albopictus adults, for database construction (Supplementary Table 1), only studies with well-established mosquitoes collection origin, adult characterization microbiota and fungi genus level characterization were chosen. Maps were constructed using ArcGis Pro 2.8.3. The maps shows, the number studies reporting samples from each country. The most reported fungi or the ones previously reported with arbovirus effect were chosen to be displayed at the map, for easily visual identification Aedes aegypti was marked green and Aedes albopictus pink.
References
Abduljalil, J. M., and Abd Al Galil, F. M. (2022). Molecular pathogenesis of dengue virus infection in Aedes mosquitoes. J. Insect Physiol. 138, 104367. doi: 10.1016/j.jinsphys.2022.104367
Ahmad, N. A., Mancini, M. V., Ant, T. H., Martinez, J., Kamarul, G. M. R., Nazni, W. A., et al. (2021). Wolbachia strain wAlbB maintains high density and dengue inhibition following introduction into a field population of Aedes aegypti. Philos. Trans. R. Soc. London. Ser. B, Biol. Sci. 376, 20190809. doi: 10.1098/rstb.2019.0809
Ahmad, N. A., Vythilingam, I., Lim, Y. A. L., Zabari, N. Z. A. M., and Lee, H. L. (2017). Detection of Wolbachia in Aedes albopictus and their effects on chikungunya virus. Am. J. Trop. Med. Hyg. 96, 148–156. doi: 10.4269/ajtmh.16-0516
Ajamma, Y. U., Onchuru, T. O., Ouso, D. O., Omondi, D., Masiga, D. K., and Villinger, J. (2018). Vertical transmission of naturally occurring Bunyamwera and insect-specific flavivirus infections in mosquitoes from islands and mainland shores of Lakes Victoria and Baringo in Kenya. PLoS Negl. Trop. Dis. 12, e0006949. doi: 10.1371/journal.pntd.0006949
Alencar, J., de Mello, C. F., Marcondes, C. B., Guimarães, A. É., Toma, H. K., Bastos, A. Q., et al. (2021). Natural infection and vertical transmission of Zika virus in sylvatic mosquitoes Aedes albopictus and Haemagogus leucocelaenus from Rio de Janeiro, Brazil. Trop. Med. Infect. Dis. 6, 99. doi: 10.3390/TROPICALMED6020099
Al-Ghamdi, S., Farrag, M. A., Abdel-Gaber, R., Alrajeh, A., El-Ashram, S., Dafalla, O., et al. (2023). Screening of the normal bacterial flora in the gut of Aedes aegypti Mosquito in Saudi Arabia. J. King Saud Univ. Sci. 35, 102715. doi: 10.1016/j.jksus.2023.102715
Aliota, M. T., Peinado, S. A., Velez, I. D., and Osorio, J. E. (2016). The wMel strain of Wolbachia reduces transmission of Zika virus by Aedes aegypti. Sci. Rep. 6, 28792. doi: 10.1038/srep28792
Amuzu, H. E., Tsyganov, K., Koh, C., Herbert, R. I., Powell, D. R., and McGraw, E. A. (2018). Wolbachia enhances insect-specific flavivirus infection in Aedes aegypti mosquitoes. Ecol. Evol. 8, 5441–5454. doi: 10.1002/ece3.4066
Anderson, M. L., Rustin, R. C., and Eremeeva, M. E. (2019). Pilot survey of mosquitoes (Diptera: Culicidae) from southeastern Georgia, USA for Wolbachia and Rickettsia felis (Rickettsiales: Rickettsiaceae). J. Vector Borne Dis. 56, 92–97. doi: 10.4103/0972-9062.263714
Angleró-Rodríguez, Y. I., Talyuli, O. A. C., Blumberg, B. J., Kang, S., Demby, C., Shields, A., et al. (2017). An Aedes aegypti-associated fungus increases susceptibility to dengue virus by modulating gut trypsin activity. Elife 6, 1–20. doi: 10.7554/eLife.28844
Aragão, C. F., da Silva, S. P., do Nascimento, B. L. S., da Silva, F. S., Nunes Neto, J. P., Pinheiro, V. C. S., et al. (2023). Shotgun metagenomic sequencing reveals virome composition of mosquitoes from a transition ecosystem of North-Northeast Brazil. Genes 14, 443. doi: 10.3390/genes14071443
Arévalo-Cortés, A., Damania, A., Granada, Y., Zuluaga, S., Mejia, R., and Triana-Chavez, O. (2022). Association of midgut bacteria and their metabolic pathways with Zika infection and insecticide resistance in Colombian Aedes aegypti populations. Viruses 14, 2197. doi: 10.3390/v14102197
Arévalo-Cortés, A., Mejia-Jaramillo, A. M., Granada, Y., Coatsworth, H., Lowenberger, C., and Triana-Chavez, O. (2020). The midgut microbiota of colombian aedes aegypti populations with different levels of resistance to the insecticide lambda-cyhalothrin. Insects 11, 1–19. doi: 10.3390/insects11090584
Arthur, J. S. C., and Ley, S. C. (2013). Mitogen-activated protein kinases in innate immunity. Nat. Rev. Immunol. 13, 679–692. doi: 10.1038/nri3495
Audsley, M. D., Seleznev, A., Joubert, D. A., Woolfit, M., O'Neill, S. L., and McGraw, E. A. (2018). Wolbachia infection alters the relative abundance of resident bacteria in adult Aedes aegypti mosquitoes, but not larvae. Mol. Ecol. 27, 297–309. doi: 10.1111/mec.14436
Baidaliuk, A., Lequime, S., Moltini-Conclois, I., Dabo, S., Dickson, L. B., Prot, M., et al. (2020). Novel genome sequences of cell-fusing agent virus allow comparison of virus phylogeny with the genetic structure of Aedes aegypti populations. Virus Evol. 6, veaa018. doi: 10.1093/ve/veaa018
Balaji, S., Deepthi, K. N. G., and Prabagaran, S. R. (2021). Native Wolbachia influence bacterial composition in the major vector mosquito Aedes aegypti. Arch. Microbiol. 203, 5225–5240. doi: 10.1007/s00203-021-02506-0
Balaji, S., Jayachandran, S., and Prabagaran, S. R. (2019). Evidence for the natural occurrence of Wolbachia in Aedes aegypti mosquitoes. FEMS Microbiol. Lett. 366. doi: 10.1093/femsle/fnz055
Baltar, J. M. C., Pavan, M. G., Corrêa-Antônio, J., Couto-Lima, D., Maciel-de-Freitas, R., and David, M. R. (2023). Gut bacterial diversity of field and laboratory-reared Aedes albopictus populations of Rio de Janeiro, Brazil. Viruses 15, 1309. doi: 10.3390/v15061309
Batson, J., Dudas, G., Haas-Stapleton, E., Kistler, A. L., Li, L. M., Logan, P., et al. (2021). Single mosquito metatranscriptomics identifies vectors, emerging pathogens and reservoirs in one assay. Elife 10, 68353. doi: 10.7554/ELIFE.68353
Bennett, K. L., Gómez-Martínez, C., Chin, Y., Saltonstall, K., McMillan, W. O., Rovira, J. R., et al. (2019). Dynamics and diversity of bacteria associated with the disease vectors Aedes aegypti and Aedes albopictus. Sci. Rep. 9, 12160. doi: 10.1038/s41598-019-48414-8
Bennouna, A., Tantely, M. L., Raharinosy, V., Andriamandimby, S. F., Bigot, T., Chrétien, D., et al. (2023). Comprehensive characterization of viral diversity of female mosquitoes in Madagascar. Viruses 15, 91852. doi: 10.3390/v15091852
Bogale, H. N., Cannon, M. V., Keita, K., Camara, D., Barry, Y., Keita, M., et al. (2020). Relative contributions of various endogenous and exogenous factors to the mosquito microbiota. Parasit Vect. 13, 1–13. doi: 10.1186/S13071-020-04491-7/TABLES/3
Bolling, B. G., Weaver, S. C., Tesh, R. B., and Vasilakis, N. (2015). Insect-specific virus discovery: significance for the arbovirus community. Viruses 7, 4911–4928. doi: 10.3390/V7092851
Bozic, J., Capone, A., Pediconi, D., Mensah, P., Cappelli, A., Valzano, M., et al. (2017). Mosquitoes can harbour yeasts of clinical significance and contribute to their environmental dissemination. Environ. Microbiol. Rep. 9, 642–648. doi: 10.1111/1758-2229.12569
Bueno-Marí, R., Domínguez-Santos, R., Trelis, M., Garrote-Sánchez, E., Cholvi, M., Quero de Lera, F., et al. (2023). Wolbachia pipientis infections in populations of Aedes albopictus in the city of València (Spain): implications for mosquito control. Rev Esp Salud Publica. 97, e202303017es
Cabral, S., de Paula, A., Samuels, R., da Fonseca, R., Gomes, S., Silva, J. R., et al. (2020). Aedes aegypti (Diptera: Culicidae) immune responses with different feeding regimes following infection by the entomopathogenic fungus Metarhizium anisopliae. Insects 11, 95. doi: 10.3390/insects11020095
Calle-Tobón, A., Pérez-Pérez, J., Forero-Pineda, N., Chávez, O. T., Rojas-Montoya, W., Rúa-Uribe, G., et al. (2022). Local-scale virome depiction in Medellín, Colombia, supports significant differences between Aedes aegypti and Aedes albopictus. PLoS ONE 17, e0263143. doi: 10.1371/journal.pone.0263143
Calvo, E. P., Archila, E. D., López, L., and Castellanos, J. E. (2021). Reconociendo el virus del chikunguña. Biomédica 41, 353–373. doi: 10.7705/biomedica.5797
Caragata, E. P., Tikhe, C. V., and Dimopoulos, G. (2019). Curious entanglements: interactions between mosquitoes, their microbiota, and arboviruses. Curr. Opin. Virol. 37, 26–36. doi: 10.1016/J.COVIRO.2019.05.005
Cardoso-Jaime, V., Tikhe, C. V., Dong, S., and Dimopoulos, G. (2022). The role of mosquito hemocytes in viral infections. Viruses 14, 102088. doi: 10.3390/v14102088
Carrington, L. B., Tran, B. C. N., Le, N. T. H., Luong, T. T. H., Nguyen, T. T., Nguyen, P. T., et al. (2017). Field- and clinically derived estimates of Wolbachia-mediated blocking of dengue virus transmission potential in Aedes aegypti mosquitoes. Proc. Natl. Acad. Sci. U. S. A. 115, 361–366. doi: 10.1073/pnas.1715788115
Carvajal, T. M., Hashimoto, K., Harnandika, R. K., Amalin, D. M., and Watanabe, K. (2019). Detection of Wolbachia in field-collected Aedes aegypti mosquitoes in metropolitan Manila, Philippines. Parasit. Vectors 12, 361. doi: 10.1186/s13071-019-3629-y
Castillo, J. C., Robertson, A. E., and Strand, M. R. (2006). Characterization of hemocytes from the mosquitoes Anopheles gambiae and Aedes aegypti. Insect Biochem. Mol. Biol. 36, 891–903. doi: 10.1016/j.ibmb.2006.08.010
Chandler, J. A., Liu, R. M., and Bennett, S. N. (2015). RNA shotgun metagenomic sequencing of Northern California (USA) mosquitoes uncovers viruses, bacteria, and fungi. Front. Microbiol. 6, 1–16. doi: 10.3389/fmicb.2015.00185
Chandrasiri, P. K. G. K., Fernando, H. S. D., and De Silva, B. G. D. N. (2020). Pre-imaginal development of Aedes aegypti in drains containing polluted water in urban cities in Sri Lanka. Int. J. Trop. Insect Sci. 40, 467–471. doi: 10.1007/s42690-019-00079-6
Chao, L.-L., and Shih, C.-M. (2023). First detection and genetic identification of Wolbachia endosymbiont in field-caught Aedes aegypti (Diptera: Culicidae) mosquitoes collected from southern Taiwan. Microorganisms 11, 11. doi: 10.3390/microorganisms11081911
Cheng, C. C., Yang, C. F., Lo, Y. P., Chiang, Y. H., Sofiyatun, E., Wang, L. C., et al. (2020). Cell-to-cell spread of dengue viral RNA in mosquito cells. Biomed Res. Int. 2020, 2452409. doi: 10.1155/2020/2452409
Cheng, G., Cox, J., Wang, P., Krishnan, M. N., Dai, J., Qian, F., et al. (2010). A C-type lectin collaborates with a CD45 phosphatase homolog to facilitate West Nile virus infection of mosquitoes. Cell 142, 714–725. doi: 10.1016/j.cell.2010.07.038
Cheng, G., Liu, Y., Wang, P., and Xiao, X. (2016). Mosquito defense strategies against viral infection. Trends Parasitol. 32, 177–186. doi: 10.1016/J.PT.2015.09.009
Cheng, L., Liu, W. L., Su, M. P., Huang, S. C., Wang, J. R., and Chen, C. H. (2022). Prohemocytes are the main cells infected by dengue virus in Aedes aegypti and Aedes albopictus. Parasit. Vectors 15, 137. doi: 10.1186/s13071-022-05276-w
Chiuya, T., Masiga, D. K., Falzon, L. C., Bastos, A. D. S., Fèvre, E. M., and Villinger, J. (2021). A survey of mosquito-borne and insect-specific viruses in hospitals and livestock markets in western Kenya. PLoS ONE 16, e0252369. doi: 10.1371/journal.pone.0252369
Chowdhury, A., Modahl, C. M., Tan, S. T., Wong Wei Xiang, B., Missé, D., Vial, T., et al. (2020). JNK pathway restricts DENV2, ZIKV and CHIKV infection by activating complement and apoptosis in mosquito salivary glands. PLoS Pathog. 16, e1008754. doi: 10.1371/journal.ppat.1008754
Chuchuy, A., Rodriguero, M. S., Ferrari, W., Ciota, A. T., Kramer, L. D., and Micieli, M. V. (2018). Biological characterization of Aedes albopictus (Diptera: Culicidae) in Argentina: implications for arbovirus transmission. Sci. Rep. 8, 5041. doi: 10.1038/s41598-018-23401-7
Coatsworth, H., Bozic, J., Carrillo, J., Buckner, E. A., Rivers, A. R., Dinglasan, R. R., et al. (2022). Intrinsic variation in the vertically transmitted core virome of the mosquito Aedes aegypti. Mol. Ecol. 31, 2545–2561. doi: 10.1111/mec.16412
Coon, K. L., Brown, M. R., and Strand, M. R. (2016). Gut bacteria differentially affect egg production in the anautogenous mosquito Aedes aegypti and facultatively autogenous mosquito Aedes atropalpus (Diptera: Culicidae). Parasit. Vectors 9, 1–12. doi: 10.1186/S13071-016-1660-9/FIGURES/5
Coon, K. L., Vogel, K. J., Brown, M. R., and Strand, M. R. (2014). Mosquitoes rely on their gut microbiota for development. Mol. Ecol. 23, 2727–2739. doi: 10.1111/MEC.12771
Correa, M. A., Matusovsky, B., Brackney, D. E., and Steven, B. (2018). Generation of axenic Aedes aegypti demonstrate live bacteria are not required for mosquito development. Nat. Commun. 9, 1–10. doi: 10.1038/s41467-018-07014-2
da Silva Ferreira, R., de Toni Aquino da Cruz, L. C., de Souza, V. J., da Silva Neves, N. A., de Souza, V. C., Filho, L. C. F., et al. (2020). Insect-specific viruses and arboviruses in adult male culicids from Midwestern Brazil. Infect. Genet. Evol. 85, 104561. doi: 10.1016/j.meegid.2020.104561
de Albuquerque, A. L., Magalhães, T., and Ayres, C. F. J. (2011). High prevalence and lack of diversity of Wolbachia pipientis in Aedes albopictus populations from Northeast Brazil. Mem. Inst. Oswaldo Cruz 106, 773–776. doi: 10.1590/S0074-02762011000600021
de Melo, E. S., and Wallau, G. L. (2020). Mosquito genomes are frequently invaded by transposable elements through horizontal transfer. PLoS Genet. 16, e1008946. doi: 10.1371/JOURNAL.PGEN.1008946
Diagne, M. M., Gaye, A., Ndione, M. H. D., Faye, M., Fall, G., Dieng, I., et al. (2020). Dianke virus: a new mesonivirus species isolated from mosquitoes in Eastern Senegal. Virus Res. 275, 197802. doi: 10.1016/j.virusres.2019.197802
Díaz, S., Camargo, C., and Avila, F. W. (2021). Characterization of the reproductive tract bacterial microbiota of virgin, mated, and blood-fed Aedes aegypti and Aedes albopictus females. Parasit. Vectors 14, 592. doi: 10.1186/s13071-021-05093-7
Dickson, L. B., Ghozlane, A., Volant, S., Bouchier, C., Ma, L., Vega-Rúa, A., et al. (2018). Diverse laboratory colonies of Aedes aegypti harbor the same adult midgut bacterial microbiome. Parasit. Vectors 11, 1. doi: 10.1186/s13071-018-2780-1
Dickson, L. B., Jiolle, D., Minard, G., Moltini-Conclois, I., Volant, S., Ghozlane, A., et al. (2017). Carryover effects of larval exposure to different environmental bacteria drive adult trait variation in a mosquito vector. Sci. Adv. 3, 1700585. doi: 10.1126/SCIADV.1700585
Ding, H., Yeo, H., and Puniamoorthy, N. (2020). Wolbachia infection in wild mosquitoes (Diptera: Culicidae): implications for transmission modes and host-endosymbiont associations in Singapore. Parasit. Vectors 13, 612. doi: 10.1186/s13071-020-04466-8
Dong, Y., Dong, S., Dizaji, N. B., Rutkowski, N., Pohlenz, T., Myles, K., et al. (2022). The Aedes aegypti siRNA pathway mediates broad-spectrum defense against human pathogenic viruses and modulates antibacterial and antifungal defenses. PLoS Biol. 20, e3001668. doi: 10.1371/journal.pbio.3001668
Dong, Y., Morton, J. C., Ramirez, J. L., Souza-Neto, J. A., and Dimopoulos, G. (2012). The entomopathogenic fungus Beauveria bassiana activate Toll and JAK-STAT pathway controlled effector genes and anti-dengue activity in Aedes aegypti. Insect Biochem. Mol. Biol. 42, 126. doi: 10.1016/J.IBMB.2011.11.005
Duarte, M. A., Campos, F. S., Araújo Neto, O. F., Silva, L. A., Silva, A. B., Aguiar, T. C., et al. (2022). Identification of potential new mosquito-associated viruses of adult Aedes aegypti mosquitoes from Tocantins state, Brazil. Brazilian J. Microbiol. 53, 51–62. doi: 10.1007/s42770-021-00632-x
Duguma, D., Hall, M. W., Rugman-Jones, P., Stouthamer, R., Terenius, O., Neufeld, J. D., et al. (2015). Developmental succession of the microbiome of Culex mosquitoes Ecological and evolutionary microbiology. BMC Microbiol. 15, 1–13. doi: 10.1186/s12866-015-0475-8
Duque-Granda, D., Posada-Mejía, J. A., Velez-Quiroz, M. A., Cadavid Restrepo, G., Moreno-Herrera, C. X., and Vivero-Gómez, R. J. (2022). Temperature preferences and detection of Wolbachia in Aedes albopictus from Santander, Colombia. SSRN Electron. J. 2022, 4247936. doi: 10.2139/ssrn.4247936
Edenborough, K. M., Flores, H. A., Simmons, C. P., and Fraser, J. E. (2021). Using Wolbachia to eliminate dengue: will the virus fight back? J. Virol. 95, e0220320. doi: 10.1128/JVI.02203-20
Elrefaey, A. M., Abdelnabi, R., Rosales Rosas, A. L., Wang, L., Basu, S., and Delang, L. (2020). Understanding the mechanisms underlying host restriction of insect-specific viruses. Viruses 12, 964. doi: 10.3390/v12090964
Fan, H., Zhao, Q., Guo, X., Sun, Q., Zuo, S., Wu, C., et al. (2016). Complete genome sequence of Xishuangbanna flavivirus, a novel mosquito-specific flavivirus from China. Arch. Virol. 161, 1723–1727. doi: 10.1007/s00705-016-2827-6
Fang, Y., Tambo, E., Xue, J.-B., Zhang, Y., Zhou, X.-N., and Khater, E. I. M. (2021a). Detection of DENV-2 and insect-specific flaviviruses in mosquitoes collected from Jeddah, Saudi Arabia. Front. Cell. Infect. Microbiol. 11, 626368. doi: 10.3389/fcimb.2021.626368
Fang, Y., Zhang, W., Xue, J.-B., and Zhang, Y. (2021b). Monitoring mosquito-borne arbovirus in various insect regions in China in 2018. Front. Cell. Infect. Microbiol. 11, 640993. doi: 10.3389/fcimb.2021.640993
Fang, Y., Zhang, Y., Zhou, Z.-B., Shi, W.-Q., Xia, S., Li, Y.-Y., et al. (2018). Co-circulation of Aedes flavivirus, Culex flavivirus, and Quang Binh virus in Shanghai, China. Infect. Dis. Poverty 7, 75. doi: 10.1186/s40249-018-0457-9
Fernandes, L. N., Paula, M. B., de Araújo, A. B., Gonçalves, E. F. B., Romano, C. M., Natal, D., et al. (2016). Detection of Culex flavivirus and Aedes flavivirus nucleotide sequences in mosquitoes from parks in the city of São Paulo, Brazil. Acta Trop. 157, 73–83. doi: 10.1016/j.actatropica.2016.01.026
Ferree, P. M., Frydman, H. M., Li, J. M., Cao, J., Wieschaus, E., and Sullivan, W. (2005). Wolbachia utilizes host microtubules and Dynein for anterior localization in the Drosophila oocyte. PLoS Pathog. 1, e14. doi: 10.1371/journal.ppat.0010014
Flores, H. A., and O'Neill, S. L. (2018). Controlling vector-borne diseases by releasing modified mosquitoes. Nat. Rev. Microbiol. 16, 508–518. doi: 10.1038/s41579-018-0025-0
Flores, H. A., Taneja de Bruyne, J., O'Donnell, T. B., Tuyet Nhu, V., Thi Giang, N., Thi Xuan Trang, H., et al. (2020). Multiple Wolbachia strains provide comparative levels of protection against dengue virus infection in Aedes aegypti. PLoS Pathog. 16, e1008433. doi: 10.1371/journal.ppat.1008433
Ford, S. A., Allen, S. L., Ohm, J. R., Sigle, L. T., Sebastian, A., Albert, I., et al. (2019). Selection on Aedes aegypti alters Wolbachia-mediated dengue virus blocking and fitness. Nat. Microbiol. 4, 1832–1839. doi: 10.1038/s41564-019-0533-3
Fragkoudis, R., Attarzadeh-Yazdi, G., Nash, A. A., Fazakerley, J. K., and Kohl, A. (2009). Advances in dissecting mosquito innate immune responses to arbovirus infection. J. Gen. Virol. 90, 2061–2072. doi: 10.1099/vir.0.013201-0
Frankel-Bricker, J. (2020). Shifts in the microbiota associated with male mosquitoes (Aedes aegypti) exposed to an obligate gut fungal symbiont (Zancudomyces culisetae). Sci. Rep. 10, 1–11. doi: 10.1038/s41598-020-69828-9
Frankel-Bricker, J., and Frankel, L. K. (2022). Re-analysis of 16S rRNA gene sequence data sets uncovers disparate laboratory-specific microbiomes associated with the yellow fever mosquito (Aedes aegypti). Microb. Ecol. 83, 167–181. doi: 10.1007/s00248-021-01739-2
Fraser, J. E., O'Donnell, T. B., Duyvestyn, J. M., O'Neill, S. L., Simmons, C. P., and Flores, H. A. (2020). Novel phenotype of Wolbachia strain wPip in Aedes aegypti challenges assumptions on mechanisms of Wolbachia-mediated dengue virus inhibition. PLoS Pathog. 16, e1008410. doi: 10.1371/journal.ppat.1008410
Frentiu, F. D. (2017). Lipids and pathogen blocking by Wolbachia. Trends Parasitol. 33, 916–917. doi: 10.1016/j.pt.2017.10.007
Frentiu, F. D., Zakir, T., Walker, T., Popovici, J., Pyke, A. T., van den Hurk, A., et al. (2014). Limited dengue virus replication in field-collected Aedes aegypti mosquitoes infected with Wolbachia. PLoS Negl. Trop. Dis. 8, e2688. doi: 10.1371/journal.pntd.0002688
Fujita, R., Kato, F., Kobayashi, D., Murota, K., Takasaki, T., Tajima, S., et al. (2018). Persistent viruses in mosquito cultured cell line suppress multiplication of flaviviruses. Heliyon 4, e00736. doi: 10.1016/j.heliyon.2018.e00736
Garrigós, M., Garrido, M., Morales-Yuste, M., Martínez-de la Puente, J., and Veiga, J. (2023). Survival effects of antibiotic exposure during the larval and adult stages in the West Nile virus vector Culex pipiens. Insect Sci. doi: 10.1111/1744-7917.13259. [Epub ahead of print].
Geoghegan, V., Stainton, K., Rainey, S. M., Ant, T. H., Dowle, A. A., Larson, T., et al. (2017). Perturbed cholesterol and vesicular trafficking associated with dengue blocking in Wolbachia-infected Aedes aegypti cells. Nat. Commun. 8, 8. doi: 10.1038/s41467-017-00610-8
Ghosh, A., Desai, A., Ravi, V., Narayanappa, G., and Tyagi, B. K. (2018). Chikungunya virus interacts with heat shock cognate 70 protein to facilitate its entry into mosquito cell line. Intervirology 60, 247–262. doi: 10.1159/000489308
Göertz, G. P., van Bree, J. W. M., Hiralal, A., Fernhout, B. M., Steffens, C., Boeren, S., et al. (2019). Subgenomic flavivirus RNA binds the mosquito DEAD/H-box helicase ME31B and determines Zika virus transmission by Aedes aegypti. Proc. Natl. Acad. Sci. U. S. A. 116, 19136–19144. doi: 10.1073/pnas.1905617116
Goic, B., Stapleford, K. A., Frangeul, L., Doucet, A. J., Gausson, V., Blanc, H., et al. (2016). Virus-derived DNA drives mosquito vector tolerance to arboviral infection. Nat. Commun. 7, 12410. doi: 10.1038/ncomms12410
Goindin, D., Cannet, A., Delannay, C., Ramdini, C., Gustave, J., Atyame, C., et al. (2018). Screening of natural Wolbachia infection in Aedes aegypti, Aedes taeniorhynchus and Culex quinquefasciatus from Guadeloupe (French West Indies). Acta Trop. 185, 314–317. doi: 10.1016/j.actatropica.2018.06.011
Gold, A. S., Feitosa-Suntheimer, F., Araujo, R. V., Hekman, R. M., Asad, S., Londono-Renteria, B., et al. (2020). Dengue virus infection of Aedes aegypti alters extracellular vesicle protein cargo to enhance virus transmission. Int. J. Mol. Sci. 21, 1–17. doi: 10.3390/ijms21186609
Gravina, H. D., Suzukawa, A. A., Zanluca, C., Cardozo Segovia, F. M., Tschá, M. K., Martins da Silva, A., et al. (2019). Identification of insect-specific flaviviruses in areas of Brazil and Paraguay experiencing endemic arbovirus transmission and the description of a novel flavivirus infecting Sabethes belisarioi. Virology 527, 98–106. doi: 10.1016/j.virol.2018.11.008
Guégan, M., Martin, E., and Moro, C. V. (2020). Comparative analysis of the bacterial and fungal communities in the gut and the crop of aedes albopictus mosquitoes: a preliminary study. Pathogens 9, 1–10. doi: 10.3390/pathogens9080628
Guégan, M., Zouache, K., Démichel, C., Minard, G., Tran Van, V., Potier, P., et al. (2018). The mosquito holobiont: fresh insight into mosquito-microbiota interactions. Microbiome 6, 1–17. doi: 10.1186/S40168-018-0435-2
Gusmão, D. S., Santos, A. V., Marini, D. C., Russo, É. D. S., Peixoto, A. M. D., Bacci, M., et al. (2007). First isolation of microorganisms from the gut diverticulum of Aedes aegypti (Diptera: Culicidae): new perspectives for an insect-bacteria association. Mem. Inst. Oswaldo Cruz 102, 919–924. doi: 10.1590/S0074-02762007000800005
Guzman, H., Contreras-Gutierrez, M. A., Travassos da Rosa, A. P. A., Nunes, M. R. T., Cardoso, J. F., Popov, V. L., et al. (2018). Characterization of three new insect-specific flaviviruses: their relationship to the mosquito-borne flavivirus pathogens. Am. J. Trop. Med. Hyg. 98, 410. doi: 10.4269/AJTMH.17-0350
Hameed, M., Wahaab, A., Shan, T., Wang, X., Khan, S., Di, D., et al. (2021). A metagenomic analysis of mosquito virome collected from different animal farms at Yunnan–Myanmar Border of China. Front. Microbiol. 11, 591478. doi: 10.3389/fmicb.2020.591478
He, X., Yin, Q., Zhou, L., Meng, L., Hu, W., Li, F., et al. (2021). Metagenomic sequencing reveals viral abundance and diversity in mosquitoes from the Shaanxi-Gansu-Ningxia region, China. PLoS Negl. Trop. Dis. 15, e0009381. doi: 10.1371/journal.pntd.0009381
Hegde, S., Khanipov, K., Albayrak, L., Golovko, G., Pimenova, M., Saldaña, M. A., et al. (2018). Microbiome interaction networks and community structure from laboratory-reared and field-collected Aedes aegypti, Aedes albopictus, and Culex quinquefasciatus mosquito vectors. Front. Microbiol. 9, 1–16. doi: 10.3389/fmicb.2018.02160
Heu, K., Romoli, O., Schönbeck, J. C., Ajenoe, R., Epelboin, Y., Kircher, V., et al. (2021). The effect of secondary metabolites produced by Serratia marcescens on Aedes aegypti and its microbiota. Front. Microbiol. 12, 645701. doi: 10.3389/fmicb.2021.645701
Horton, A. A., Wang, B., Camp, L., Price, M. S., Arshi, A., Nagy, M., et al. (2011). The mitogen-activated protein kinome from Anopheles gambiae: identification, phylogeny and functional characterization of the ERK, JNK and p38 MAP kinases. BMC Genom. 12, 574. doi: 10.1186/1471-2164-12-574
Hoshino, K., Isawa, H., Tsuda, Y., Sawabe, K., and Kobayashi, M. (2009). Isolation and characterization of a new insect flavivirus from Aedes albopictus and Aedes flavopictus mosquitoes in Japan. Virology 391, 119–129. doi: 10.1016/j.virol.2009.06.025
Houé, V., Bonizzoni, M., and Failloux, A. B. (2019a). Endogenous non-retroviral elements in genomes of Aedes mosquitoes and vector competence. Emerg. Microbes Infect. 8, 542–555. doi: 10.1080/22221751.2019.1599302
Houé, V., Gabiane, G., Dauga, C., Suez, M., Madec, Y., Mousson, L., et al. (2019b). Evolution and biological significance of flaviviral elements in the genome of the arboviral vector Aedes albopictus. Emerg. Microbes Infect. 8, 1265–1279. doi: 10.1080/22221751.2019.1657785
Hoyos-López, R., Suaza-Vasco, J., Rúa-Uribe, G., Uribe, S., and Gallego-Gómez, J. C. (2016). Molecular detection of flaviviruses and alphaviruses in mosquitoes (Diptera: Culicidae) from coastal ecosystems in the Colombian Caribbean. Mem. Inst. Oswaldo Cruz 111, 625–634. doi: 10.1590/0074-02760160096
Hu, Y., Xi, Z., Liu, X., Wang, J., Guo, Y., Ren, D., et al. (2020). Identification and molecular characterization of Wolbachia strains in natural populations of Aedes albopictus in China. Parasit. Vectors 13, 28. doi: 10.1186/s13071-020-3899-4
Hussain, M., Etebari, K., and Asgari, S. (2023a). Analysing inhibition of dengue virus in Wolbachia-infected mosquito cells following the removal of Wolbachia. Virology 581, 48–55. doi: 10.1016/j.virol.2023.02.017
Hussain, M., Zhang, G., Leitner, M., Hedges, L. M., and Asgari, S. (2023b). Wolbachia RNase HI contributes to virus blocking in the mosquito Aedes aegypti. iScience 26, 105836. doi: 10.1016/j.isci.2022.105836
Iwashita, H., Higa, Y., Futami, K., Lutiali, P. A., Njenga, S. M., Nabeshima, T., et al. (2018). Mosquito arbovirus survey in selected areas of Kenya: detection of insect-specific virus. Trop. Med. Health 46, 19. doi: 10.1186/s41182-018-0095-8
Jeffries, C. L., White, M., Wilson, L., Yakob, L., and Walker, T. (2020). Detection of Cell-Fusing Agent virus across ecologically diverse populations of Aedes aegypti on the Caribbean island of Saint Lucia. Wellcome Open Res. 5, 149. doi: 10.12688/wellcomeopenres.16030.2
Joanne, S., Vythilingam, I., Yugavathy, N., Leong, C.-S., Wong, M.-L., and AbuBakar, S. (2015). Distribution and dynamics of Wolbachia infection in Malaysian Aedes albopictus. Acta Trop. 148, 38–45. doi: 10.1016/j.actatropica.2015.04.003
Kingsolver, M. B., and Hardy, R. W. (2012). Making connections in insect innate immunity. Proc. Natl. Acad. Sci. U. S. A. 109, 18639–18640. doi: 10.1073/PNAS.1216736109
Kitrayapong, P., Baimai, V., and O'Neill, S. L. (2002). Field prevalence of Wolbachia in the mosquito vector Aedes albopictus. Am. J. Trop. Med. Hyg. 66, 108–111. doi: 10.4269/ajtmh.2002.66.108
Kong, L., Xiao, J., Yang, L., Sui, Y., Wang, D., Chen, S., et al. (2023). Mosquito densovirus significantly reduces the vector susceptibility to dengue virus serotype 2 in Aedes albopictus mosquitoes (Diptera: Culicidae). Infect. Dis. Poverty 12, 48. doi: 10.1186/s40249-023-01099-8
Kozlova, E. V., Hegde, S., Roundy, C. M., Golovko, G., Saldaña, M. A., Hart, C. E., et al. (2021). Microbial interactions in the mosquito gut determine Serratia colonization and blood-feeding propensity. ISME J. 15, 93–108. doi: 10.1038/s41396-020-00763-3
Kuadkitkan, A., Wikan, N., Fongsaran, C., and Smith, D. R. (2010). Identification and characterization of prohibitin as a receptor protein mediating DENV-2 entry into insect cells. Virology 406, 149–161. doi: 10.1016/j.virol.2010.07.015
Kubacki, J., Flacio, E., Qi, W., Guidi, V., Tonolla, M., and Fraefel, C. (2020). Viral metagenomic analysis of Aedes albopictus mosquitos from southern Switzerland. Viruses 12. doi: 10.3390/v12090929
Kulkarni, A., Yu, W., Jiang, J., Sanchez, C., Karna, A. K., Martinez, K. J. L., et al. (2019). Wolbachia pipientis occurs in Aedes aegypti populations in New Mexico and Florida, USA. Ecol. Evol. 9, 6148–6156. doi: 10.1002/ece3.5198
Laiton-Donato, K., Guzmán, C., Perdomo-Balaguera, E., Sarmiento, L., Torres-Fernandez, O., Ruiz, H. A., et al. (2023). Novel putative tymoviridae-like virus isolated from culex mosquitoes in Colombia. Viruses 15, 40953. doi: 10.3390/v15040953
Li, Y., Sun, Y., Zou, J., Zhong, D., Liu, R., Zhu, C., et al. (2023). Characterizing the Wolbachia infection in field-collected Culicidae mosquitoes from Hainan Province, China. Parasit. Vectors 16, 128. doi: 10.1186/s13071-023-05719-y
Lin, D., Zheng, X., Sanogo, B., Ding, T., Sun, X., and Wu, Z. (2021). Bacterial composition of midgut and entire body of laboratory colonies of Aedes aegypti and Aedes albopictus from southern China. Parasit. Vectors 14, 586. doi: 10.1186/s13071-021-05050-4
Lin, D. C. D., Weng, S. C., Tsao, P. N., Chu, J. J. H., and Shiao, S. H. (2023). Co-infection of dengue and Zika viruses mutually enhances viral replication in the mosquito Aedes aegypti. Parasit. Vectors 16, 160. doi: 10.1186/s13071-023-05778-1
Lindsey, A. R. I., Bhattacharya, T., Newton, I. L. G., and Hardy, R. W. (2018). Conflict in the intracellular lives of endosymbionts and viruses: a mechanistic look at Wolbachia-mediated pathogen-blocking. Viruses 10, 141. doi: 10.3390/v10040141
Liu, W. Q., Chen, S. Q., Bai, H. Q., Wei, Q. M., Zhang, S. N., Chen, C., et al. (2020). The Ras/ERK signaling pathway couples antimicrobial peptides to mediate resistance to dengue virus in Aedes mosquitoes. PLoS Negl. Trop. Dis. 14, e0008660. doi: 10.1371/journal.pntd.0008660
Liu, Y., Zhang, F., Liu, J., Xiao, X., Zhang, S., Qin, C., et al. (2014). Transmission-blocking antibodies against mosquito C-type lectins for dengue prevention. PLoS Pathog. 10, e1003931. doi: 10.1371/journal.ppat.1003931
Luis, P., Vallon, L., Tran, F. H., Hugoni, M., Tran-Van, V., Mavingui, P., et al. (2019). Aedes albopictus mosquitoes host a locally structured mycobiota with evidence of reduced fungal diversity in invasive populations. Fungal Ecol. 39, 257–266. doi: 10.1016/j.funeco.2019.02.004
MacLeod, H. J., Dimopoulos, G., and Short, S. M. (2021). Larval diet abundance influences size and composition of the midgut microbiota of Aedes aegypti mosquitoes. Front. Microbiol. 12, 645362. doi: 10.3389/fmicb.2021.645362
Mancini, M. V., Damiani, C., Accoti, A., Tallarita, M., Nunzi, E., Cappelli, A., et al. (2018). Estimating bacteria diversity in different organs of nine species of mosquito by next generation sequencing. BMC Microbiol. 18, 1–10. doi: 10.1186/S12866-018-1266-9
Mantilla-Granados, J. S., Sarmiento-Senior, D., Manzano, J., Calderón-Peláez, M. A., Velandia-Romero, M. L., Buitrago, L. S., et al. (2022). Multidisciplinary approach for surveillance and risk identification of yellow fever and other arboviruses in Colombia. One Heal. 15, 100438. doi: 10.1016/j.onehlt.2022.100438
Martin, E., Borucki, M. K., Thissen, J., Garcia-Luna, S., Hwang, M., Wise de Valdez, M., et al. (2019). Mosquito-borne viruses and insect-specific viruses revealed in field-collected mosquitoes by a monitoring tool adapted from a microbial detection array. Appl. Environ. Microbiol. 85, 19. doi: 10.1128/AEM.01202-19
Martin, E., Tang, W., Briggs, C., Hopson, H., Juarez, J. G., Garcia-Luna, S. M., et al. (2020). Cell fusing agent virus (Flavivirus) infection in Aedes aegypti in Texas: seasonality, comparison by trap type, and individual viral loads. Arch. Virol. 165, 1769–1776. doi: 10.1007/s00705-020-04652-0
Martinez Villegas, L. E., Radl, J., Dimopoulos, G., and Short, S. M. (2023). Bacterial communities of Aedes aegypti mosquitoes differ between crop and midgut tissues. PLoS Negl. Trop. Dis. 17, 1–26. doi: 10.1371/journal.pntd.0011218
Minard, G., Tran, F.-H., Tran Van, V., Fournier, C., Potier, P., Roiz, D., et al. (2018). Shared larval rearing environment, sex, female size and genetic diversity shape Ae. albopictus bacterial microbiota. PLoS ONE 13, e0194521. doi: 10.1371/journal.pone.0194521
Mohanty, I., Rath, A., Swain, S. P., Pradhan, N., and Hazra, R. K. (2019). Wolbachia population in vectors and non-vectors: a sustainable approach towards dengue control. Curr. Microbiol. 76, 133–143. doi: 10.1007/s00284-018-1596-8
Molina-Henao, E. H., Graffe, M. Y., De La Cadena, E. P., Serrato, I. M., Correa, A., Romero, L. V., et al. (2020). Culturable microbial composition in the midgut of Aedes aegypti strains with different susceptibility to dengue-2 virus infection. Symbiosis 80, 85–93. doi: 10.1007/s13199-019-00646-y
Moncada, L. L. I., Adler, P., and Lucía, Q. M. (2021). Introducción al estudio de insectos de interés en salud pública, de Otros autores - Libros en Google Play. 1st ed. Facultad de Medicina. Universidad Nacional de Colombia Bogotá: Universidad Nacional de Colombia. Available online at: https://play.google.com/store/books/details/Introducción_al_estudio_de_insectos_de_interés_en_?id=HMg4EAAAQBAJ&hl=es_CO&gl=US (accessed November 22, 2021).
Mondotte, J. A., Gausson, V., Frangeul, L., Suzuki, Y., Vazeille, M., Mongelli, V., et al. (2020). Evidence for long-lasting transgenerational antiviral immunity in insects. Cell Rep. 33, 108506. doi: 10.1016/j.celrep.2020.108506
Moreira, L. A., Iturbe-Ormaetxe, I., Jeffery, J. A., Lu, G., Pyke, A. T., Hedges, L. M., et al. (2009). A Wolbachia symbiont in Aedes aegypti limits infection with Dengue, Chikungunya, and Plasmodium. Cell 139, 1268–1278. doi: 10.1016/j.cell.2009.11.042
Mosso González, C., Lozano-Sardaneta, Y. N., Viveros-Santos, V., and Torres Monzon, J. (2023). Aedes albopictus infection by the native bacterium Elizabethkingia anophelis in Southern Mexico. Salud Publica Mex. 65, 14122. doi: 10.21149/14122
Mousson, L., Zouache, K., Arias-Goeta, C., Raquin, V., Mavingui, P., and Failloux, A.-B. (2012). The native Wolbachia symbionts limit transmission of dengue virus in Aedes albopictus. PLoS Negl. Trop. Dis. 6, e1989. doi: 10.1371/journal.pntd.0001989
Mukherjee, D., Das, S., Begum, F., Mal, S., and Ray, U. (2019). The mosquito immune system and the life of dengue virus: what we know and do not know. Pathogens 8, 77. doi: 10.3390/pathogens8020077
Munivenkatappa, A., Nyayanit, D. A., Yadav, P. D., Rangappa, M., Patil, S., Majumdar, T., et al. (2021). Identification of phasi charoen-like phasivirus in field collected Aedes aegypti from Karnataka State, India. Vector Borne Zoonotic Dis. 21, 900–909. doi: 10.1089/vbz.2021.0011
Nebbak, A., Monteil-Bouchard, S., Berenger, J.-M., Almeras, L., Parola, P., and Desnues, C. (2021). Virome diversity among mosquito populations in a sub-urban region of Marseille, France. Viruses 13, 768. doi: 10.3390/v13050768
Noor Afizah, A., Roziah, A., Nazni, W. A., and Lee, H. L. (2015). Detection of Wolbachia from field collected Aedes albopictus Skuse in Malaysia. Indian J. Med. Res. 142, 205–210. doi: 10.4103/0971-5916.164259
Noriega, F. G., and Wells, M. A. (1999). A molecular view of trypsin synthesis in the midgut of Aedes aegypti. J. Insect Physiol. 45, 613–620. doi: 10.1016/s0022-1910(99)00052-9
Nugapola, N. W. N. P., De Silva, W. A. P. P., and Karunaratne, S. H. P. P. (2017). Distribution and phylogeny of Wolbachia strains in wild mosquito populations in Sri Lanka. Parasit. Vectors 10, 230. doi: 10.1186/s13071-017-2174-9
Oguzie, J. U., Nwangwu, U. C., Oluniyi, P. E., Olumade, T. J., George, U. E., Kazeem, A., et al. (2022). Metagenomic sequencing characterizes a wide diversity of viruses in field mosquito samples in Nigeria. Sci. Rep. 12, 7616. doi: 10.1038/s41598-022-11797-2
Öhlund, P., Lundén, H., and Blomström, A. L. (2019). Insect-specific virus evolution and potential effects on vector competence. Virus Genes 55, 127–137. doi: 10.1007/S11262-018-01629-9
Olmo, R. P., Todjro, Y. M. H., Aguiar, E. R. G. R., de Almeida, J. P. P., Ferreira, F. V., Armache, J. N., et al. (2023). Mosquito vector competence for dengue is modulated by insect-specific viruses. Nat. Microbiol. 8, 135–149. doi: 10.1038/s41564-022-01289-4
Olson, K. E., and Blair, C. D. (2015). Arbovirus-mosquito interactions: RNAi pathway. Curr. Opin. Virol. 15, 119–126. doi: 10.1016/j.coviro.2015.10.001
Onyango, G. M., Bialosuknia, M. S., Payne, F. A., Mathias, N., Ciota, T. A., and Kramer, D. L. (2020). Increase in temperature enriches heat tolerant taxa in Aedes aegypti midguts. Sci. Rep. 10, 19135. doi: 10.1038/s41598-020-76188-x
Palatini, U., Alfano, N., Carballar, R. L., Chen, X. G., Delatte, H., and Bonizzoni, M. (2022). Virome and nrEVEome diversity of Aedes albopictus mosquitoes from La Reunion Island and China. Virol. J. 19, 1–12. doi: 10.1186/s12985-022-01918-8
Pan, X., Zhou, G., Wu, J., Bian, G., Lu, P., Raikhel, A. S., et al. (2012). Wolbachia induces reactive oxygen species (ROS)-dependent activation of the Toll pathway to control dengue virus in the mosquito Aedes aegypti. Proc. Natl. Acad. Sci. U. S. A. 109, E23. doi: 10.1073/PNAS.1116932108/-/DCSUPPLEMENTAL
Pang, X., Xiao, X., Liu, Y., Zhang, R., Liu, J., Liu, Q., et al. (2016). Mosquito C-type lectins maintain gut microbiome homeostasis. Nat. Microbiol. 1, 1–11. doi: 10.1038/nmicrobiol.2016.23
Parry, R., Asgari, S., and Williams, B. R. G. (2018). Aedes anphevirus: an insect-specific virus distributed worldwide in Aedes aegypti mosquitoes that has complex interplays with Wolbachia and dengue virus infection in cells. Virus-Cell Interactions 92, 224–242. doi: 10.1128/JVI
Parry, R., James, M. E., and Asgari, S. (2021). Uncovering the worldwide diversity and evolution of the virome of the mosquitoes Aedes aegypti and Aedes albopictus. Microorganisms 9, 1–28. doi: 10.3390/microorganisms9081653
Pérez-Ramos, D. W., Ramos, M. M., Payne, K. C., Giordano, B. V., and Caragata, E. P. (2022). Collection time, location, and mosquito species have distinct impacts on the mosquito microbiota. Front. Trop. Dis. 3, 896289. doi: 10.3389/fitd.2022.896289
Plotnikov, A., Zehorai, E., Procaccia, S., and Seger, R. (2011). The MAPK cascades: signaling components, nuclear roles and mechanisms of nuclear translocation. Biochim. Biophys. Acta Mol. Cell Res. 1813, 1619–1633. doi: 10.1016/j.bbamcr.2010.12.012
Pompon, J., Manuel, M., Ng, G. K., Wong, B., Shan, C., Manokaran, G., et al. (2017). Dengue subgenomic flaviviral RNA disrupts immunity in mosquito salivary glands to increase virus transmission. PLoS Pathog. 13, e1006535. doi: 10.1371/journal.ppat.1006535
Prince, B. C., Walsh, E., Torres, T. Z. B., and Rückert, C. (2023). Recognition of arboviruses by the mosquito immune system. Biomolecules 13, 71159. doi: 10.3390/biom13071159
Puerta-Guardo, H., Contreras-Perera, Y., Perez-Carrillo, S., Che-Mendoza, A., Ayora-Talavera, G., Vazquez-Prokopec, G., et al. (2020). Wolbachia in native populations of Aedes albopictus (Diptera: Culicidae) from Yucatan Peninsula, Mexico. J. Insect Sci. 20, 16. doi: 10.1093/jisesa/ieaa096
Raharimalala, F. N., Boukraa, S., Bawin, T., Boyer, S., and Francis, F. (2016). Molecular detection of six (endo-) symbiotic bacteria in Belgian mosquitoes: first step towards the selection of appropriate paratransgenesis candidates. Parasitol. Res. 115, 1391–1399. doi: 10.1007/s00436-015-4873-5
Ramirez, J. L., Short, S. M., Bahia, A. C., Saraiva, R. G., Dong, Y., Kang, S., et al. (2014). Chromobacterium Csp_P reduces malaria and dengue infection in vector mosquitoes and has entomopathogenic and in vitro anti-pathogen activities. PLoS Pathog. 10, 4398. doi: 10.1371/journal.ppat.1004398
Ramirez, J. L., Souza-Neto, J., Cosme, R. T., Rovira, J., Ortiz, A., Pascale, J. M., et al. (2012). Reciprocal tripartite interactions between the Aedes aegypti midgut microbiota, innate immune system and dengue virus influences vector competence. PLoS Negl. Trop. Dis. 6, 1561. doi: 10.1371/JOURNAL.PNTD.0001561
Ramos-Nino, M. E., Fitzpatrick, D. M., Eckstrom, K. M., Tighe, S., Hattaway, L. M., Hsueh, A. N., et al. (2020). Metagenomic analysis of Aedes aegypti and Culex quinquefasciatus mosquitoes from Grenada, West Indies. PLoS ONE 15, e0231047. doi: 10.1371/journal.pone.0231047
Ratnayake, O. C., Chotiwan, N., Saavedra-Rodriguez, K., and Perera, R. (2023). The buzz in the field: the interaction between viruses, mosquitoes, and metabolism. Front. Cell. Infect. Microbiol. 13, 1125877. doi: 10.3389/fcimb.2023.1128577
Rau, J., Werner, D., Beer, M., Höper, D., and Kampen, H. (2022). The microbial RNA metagenome of Aedes albopictus (Diptera: Culicidae) from Germany. Parasitol. Res. 121, 2587–2599. doi: 10.1007/s00436-022-07576-7
Ravikumar, H., Ramachandraswamy, N., Sampathkumar, S., Prakash, B. M., Huchesh, H. C., Uday, J., et al. (2010). A preliminary survey for Wolbachia and bacteriophage WO infections in Indian mosquitoes (Diptera: Culicidae). Trop. Biomed. 27, 384–393.
Reyes-Ruiz, J. M., Osuna-Ramos, J. F., de Jesús-González, L. A., Palacios-Rápalo, S. N., Cordero-Rivera, C. D., Farfan-Morales, C. N., et al. (2020). The regulation of flavivirus infection by hijacking exosome-mediated cell-cell communication: new insights on virus-host interactions. Viruses 12, 70765. doi: 10.3390/v12070765
Ribeiro, G. O., Morais, V. S., Monteiro, F. J. C., Ribeiro, E. S. D., Rego, M. O. S., Souto, R. N. P., et al. (2020). Aedes aegypti from amazon basin harbor high diversity of novel viral species. Viruses 12, 866. doi: 10.3390/v12080866
Rizzo, F., Cerutti, F., Ballardini, M., Mosca, A., Vitale, N., Radaelli, M. C., et al. (2014). Molecular characterization of flaviviruses from field-collected mosquitoes in northwestern Italy, 2011-2012. Parasit. Vectors 7, 395. doi: 10.1186/1756-3305-7-395
Rodpai, R., Boonroumkaew, P., Sadaow, L., Sanpool, O., Janwan, P., Thanchomnang, T., et al. (2023). Microbiome composition and microbial community structure in mosquito vectors Aedes aegypti and Aedes albopictus in Northeastern Thailand, a dengue-endemic area. Insects 14, 20184. doi: 10.3390/insects14020184
Romoli, O., Schönbeck, J. C., Hapfelmeier, S., and Gendrin, M. (2021). Production of germ-free mosquitoes via transient colonisation allows stage-specific investigation of host–microbiota interactions. Nat. Commun. 12, 942. doi: 10.1038/s41467-021-21195-3
Rosendo Machado, S., van der Most, T., and Miesen, P. (2021). Genetic determinants of antiviral immunity in dipteran insects—compiling the experimental evidence. Dev. Comp. Immunol. 119, 104010. doi: 10.1016/j.dci.2021.104010
Roslan, M. A., Ngui, R., Vythilingam, I., and Sulaiman, W. Y. W. (2022). Community surveillance of Aedes albopictus associated with Wolbachia detection in low-rise residential areas in Selangor, Malaysia. J. Vector Ecol. 47, 142–152. doi: 10.52707/1081-1710-47.2.142
Rosso, F., Tagliapietra, V., Albanese, D., Pindo, M., Baldacchino, F., Arnoldi, D., et al. (2018). Reduced diversity of gut microbiota in two Aedes mosquitoes species in areas of recent invasion. Sci. Rep. 8, 1–11. doi: 10.1038/s41598-018-34640-z
Ruiz, A., Gutiérrez-Bugallo, G., Rodríguez-Roche, R., Pérez, L., González-Broche, R., Piedra, L. A., et al. (2023). First report of natural Wolbachia infections in mosquitoes from Cuba. Acta Trop. 242, 106891. doi: 10.1016/j.actatropica.2023.106891
Sadeghi, M., Popov, V., Guzman, H., Phan, T. G., Vasilakis, N., Tesh, R., et al. (2017). Genomes of viral isolates derived from different mosquitos species. Virus Res. 242, 49–57. doi: 10.1016/j.virusres.2017.08.012
Sakoonwatanyoo, P., Boonsanay, V., and Smith, D. R. (2006). Growth and production of the dengue virus in C6/36 cells and identification of a laminin-binding protein as a candidate serotype 3 and 4 receptor protein. Intervirology 49, 161–172. doi: 10.1159/000089377
Salim Mattar, V., and Marco González, T. (2018). Insect microbiomes and insect- specific viruses a new key for understanding the arboviruses diseases? Rev. MVZ Cordoba 23, 6595–6597. doi: 10.21897/rmvz.1359
Sarma, D. K., Kumar, M., Dhurve, J., Pal, N., Sharma, P., James, M. M., et al. (2022). Influence of host blood meal source on gut microbiota of wild caught Aedes aegypti, a dominant arboviral disease vector. Microorganisms 10, 20332. doi: 10.3390/microorganisms10020332
Sasaki, T., Moi, M. L., Saito, K., Isawa, H., Takasaki, T., and Sawabe, K. (2022). Aedes albopictus strain and dengue virus serotype in the dengue fever outbreaks in Japan: implications of Wolbachia infection. Jpn. J. Infect. Dis. 75, 140–143. doi: 10.7883/yoken.JJID.2021.376
Schrieke, H., Maignien, L., Constancias, F., Trigodet, F., Chakloute, S., Rakotoarivony, I., et al. (2022). The mosquito microbiome includes habitat-specific but rare symbionts. Comput. Struct. Biotechnol. J. 20, 410–420. doi: 10.1016/j.csbj.2021.12.019
Scolari, F., Casiraghi, M., and Bonizzoni, M. (2019). Aedes spp. and their microbiota: a review. Front. Microbiol. 10, 2036. doi: 10.3389/FMICB.2019.02036/BIBTEX
Scolari, F., Sandionigi, A., Carlassara, M., Bruno, A., Casiraghi, M., and Bonizzoni, M. (2021). Exploring changes in the microbiota of Aedes albopictus: comparison among breeding site water, larvae, and adults. Front. Microbiol. 12, 23. doi: 10.3389/FMICB.2021.624170/BIBTEX
Seabourn, P., Spafford, H., Yoneishi, N., and Medeiros, M. (2020). The Aedes albopictus (Diptera: Culicidae) microbiome varies spatially and with Ascogregarine infection. PLoS Negl. Trop. Dis. 14, e0008615. doi: 10.1371/journal.pntd.0008615
Serrato-Salas, J., Hernández-Martínez, S., Martínez-Barnetche, J., Condé, R., Alvarado-Delgado, A., Zumaya-Estrada, F., et al. (2018). De novo DNA synthesis in Aedes aegypti midgut cells as a complementary strategy to limit dengue viral replication. Front. Microbiol. 9, 801. doi: 10.3389/fmicb.2018.00801
Shaikevich, E., Bogacheva, A., and Ganushkina, L. (2019). Dirofilaria and Wolbachia in mosquitoes (Diptera: Culicidae) in central European Russia and on the Black Sea coast. Parasite 26, 2. doi: 10.1051/parasite/2019002
Shaul, Y. D., and Seger, R. (2007). The MEK/ERK cascade: from signaling specificity to diverse functions. Biochim. Biophys. Acta 1773, 1213–1226. doi: 10.1016/j.bbamcr.2006.10.005
Shi, C., Beller, L., Deboutte, W., Yinda, K. C., Delang, L., Vega-Rúa, A., et al. (2019). Stable distinct core eukaryotic viromes in different mosquito species from Guadeloupe, using single mosquito viral metagenomics. Microbiome 7, 121. doi: 10.1186/s40168-019-0734-2
Shi, C., Beller, L., Wang, L., Rosales Rosas, A., De Coninck, L., Héry, L., et al. (2022). Bidirectional interactions between arboviruses and the bacterial and viral microbiota in Aedes aegypti and Culex quinquefasciatus. MBio 13, e0102122. doi: 10.1128/mbio.01021-22
Sicard, M., Bonneau, M., and Weill, M. (2019). Wolbachia prevalence, diversity, and ability to induce cytoplasmic incompatibility in mosquitoes. Curr. Opin. Insect Sci. 34, 12–20. doi: 10.1016/j.cois.2019.02.005
Silverman, N., Tassetto, M., Kunitomi, M., Whitfield, Z. J., Dolan, P. T., Sá nchez-Vargas, I., et al. (2019). Control of RNA viruses in mosquito cells through the acquisition of vDNA and endogenous viral elements. eLife 2019, 1. doi: 10.7554/eLife.41244.001
Simões, M. L., Caragata, E. P., and Dimopoulos, G. (2018). Diverse host and restriction factors regulate mosquito–pathogen interactions. Trends Parasitol. 34, 603–616. doi: 10.1016/j.pt.2018.04.011
Sinha, A., Li, Z., Sun, L., Carlow, C. K. S., and Cordaux, R. (2019). Complete genome sequence of the Wolbachia wAlbB endosymbiont of Aedes albopictus. Genome Biol. Evol. 11, 706–720. doi: 10.1093/gbe/evz025
Slonchak, A., and Khromykh, A. A. (2018). Subgenomic flaviviral RNAs: what do we know after the first decade of research. Antiviral Res. 159, 13–25. doi: 10.1016/j.antiviral.2018.09.006
Slonchak, A., Parry, R., Pullinger, B., Sng, J. D. J., Wang, X., Buck, T. F., et al. (2022). Structural analysis of 3′UTRs in insect flaviviruses reveals novel determinants of sfRNA biogenesis and provides new insights into flavivirus evolution. Nat. Commun. 13, 1279. doi: 10.1038/s41467-022-28977-3
Somia, E. S., Ullah, I., Alyahya, H. S., and Mahyoub, J. A. (2023). Identification of Wolbachia new strains from Aedes aegypti mosquitoes, the vector of dengue fever in Jeddah Province. BMC Microbiol. 23, 287. doi: 10.1186/s12866-023-03010-9
Soni, M., Bhattacharya, C., Sharma, J., Khan, S. A., and Dutta, P. (2017). Molecular typing and phylogeny of Wolbachia: a study from Assam, North-Eastern part of India. Acta Trop. 176, 421–426. doi: 10.1016/j.actatropica.2017.09.005
Sultana, H., and Neelakanta, G. (2020). Arthropod exosomes as bubbles with message(s) to transmit vector-borne diseases. Curr. Opin. Insect Sci. 40, 39–47. doi: 10.1016/j.cois.2020.05.017
Supriyono, O., Kuwata, R., Torii, S., Shimoda, H., Ishijima, K., Yonemitsu, K., et al. (2020). Mosquito-borne viruses, insect-specific flaviviruses (family Flaviviridae, genus Flavivirus), Banna virus (family Reoviridae, genus Seadornavirus), Bogor virus (unassigned member of family Permutotetraviridae), and alphamesoniviruses 2 and 3 (family Mesoniviridae, genus Alphamesonivirus) isolated from Indonesian mosquitoes. J. Vet. Med. Sci. 82, 1030–1041. doi: 10.1292/jvms.20-0261
Talyuli, O. A. C., Oliveira, J. H. M., Bottino-Rojas, V., Silveira, G. O., Alvarenga, P. H., Barletta, A. B. F., et al. (2023). The Aedes aegypti peritrophic matrix controls arbovirus vector competence through HPx1, a heme-induced peroxidase. PLoS Pathog. 19, e1011149. doi: 10.1371/journal.ppat.1011149
Tassetto, M., Kunitomi, M., and Andino, R. (2017). Circulating immune cells mediate a systemic RNAi-based adaptive antiviral response in Drosophila. Cell 169 314–325.e13. doi: 10.1016/j.cell.2017.03.033
Tawidian, P., Coon, K. L., Jumpponen, A., Cohnstaedt, L. W., and Michel, K. (2021). Host-environment interplay shapes fungal diversity in mosquitoes. mSphere 6, 1–14. doi: 10.1128/msphere.00646-21
Teng, F., and Fussenegger, M. (2021). Shedding light on extracellular vesicle biogenesis and bioengineering. Adv. Sci. 8, 3505. doi: 10.1002/advs.202003505
Teramoto, T., Huang, X., Armbruster, P. A., and Padmanabhan, R. (2019). Infection of Aedes albopictus mosquito C6/36 cells with the wMelpop strain of Wolbachia modulates dengue virus-induced host cellular transcripts and induces critical sequence alterations in the dengue viral genome. J. Virol. 93, e00581–e00519. doi: 10.1128/JVI.00581-19
Thannesberger, J., Rascovan, N., Eisenmann, A., Klymiuk, I., Zittra, C., Fuehrer, H.-P., et al. (2020). Highly sensitive virome characterization of Aedes aegypti and Culex pipiens complex from Central Europe and the caribbean reveals potential for interspecies viral transmission. Pathog 9, 686. doi: 10.3390/pathogens9090686
Théry, C., Witwer, K. W., Aikawa, E., Alcaraz, M. J., Anderson, J. D., Andriantsitohaina, R., et al. (2018). Minimal information for studies of extracellular vesicles 2018 (MISEV2018): a position statement of the International Society for Extracellular Vesicles and update of the MISEV2014 guidelines. J. Extracell. Vesicles 7, 1535750. doi: 10.1080/20013078.2018.1535750
Thongsripong, P., Chandler, J. A., Green, A. B., Kittayapong, P., Wilcox, B. A., Kapan, D. D., et al. (2018). Mosquito vector-associated microbiota: metabarcoding bacteria and eukaryotic symbionts across habitat types in Thailand endemic for dengue and other arthropod-borne diseases. Ecol. Evol. 8, 1352–1368. doi: 10.1002/ece3.3676
Torres-Monzón, J. A., Casas-Martínez, M., and López-Ordóñez, T. (2020). Infection of Aedes mosquitoesbynative Wolbachia in urbancemeteriesof Southern Mexico. Salud Publica Mex. 62, 447–449. doi: 10.21149/10163
Tortosa, P., Charlat, S., Labbé, P., Dehecq, J. S., Barré, H., and Weill, M. (2010). Wolbachia age-sex-specific density in Aedes albopictus: a host evolutionary response to cytoplasmic incompatibility? PLoS ONE 5, e9700. doi: 10.1371/journal.pone.0009700
Tsai, S. K., Hsu, Y. L., Chiao, D. J., Shu, P. Y., Lin, H. T., Chang, S. F., et al. (2023). Antigenicity and immunogenicity of chikungunya virus-like particles from mosquito cells. Appl. Microbiol. Biotechnol. 107, 219–232. doi: 10.1007/s00253-022-12280-8
Tsetsarkin, K. A., Vanlandingham, D. L., McGee, C. E., and Higgs, S. (2007). A single mutation in chikungunya virus affects vector specificity and epidemic potential. PLoS Pathog. 3, e201. doi: 10.1371/journal.ppat.0030201
Vargas, V., Cime-Castillo, J., and Lanz-Mendoza, H. (2020). Immune priming with inactive dengue virus during the larval stage of Aedes aegypti protects against the infection in adult mosquitoes. Sci. Rep. 10, 63402. doi: 10.1038/s41598-020-63402-z
Vasilakis, N., Guzman, H., Firth, C., Forrester, N. L., Widen, S. G., Wood, T. G., et al. (2014). Mesoniviruses are mosquito-specific viruses with extensive geographic distribution and host range. Virol. J. 11, 97. doi: 10.1186/1743-422X-11-97
Vega-Rua, A., Zouache, K., Girod, R., Failloux, A.-B., and Lourenco-de-Oliveira, R. (2014). High level of vector competence of Aedes aegypti and Aedes albopictus from ten American countries as a crucial factor in the spread of Chikungunya virus. J. Virol. 88, 6294–6306. doi: 10.1128/jvi.00370-14
Velandia, M. L., and Castellanos, J. E. (2011). Virus del dengue: estructura y ciclo viral. Infectio 15, 33–43. doi: 10.1016/s0123-9392(11)70074-1
Velandia-Romero, M. L., Olano, V. A., Coronel-Ruiz, C., Cabezas, L., Calderón-Peláez, M. A., Castellanos, J. E., et al. (2017). Detección del virus dengue en larvas y pupas de Aedes aegypti recolectadas en áreas rurales del municipio de Anapoima, Cundinamarca, Colombia. Biomedica 37, 1–24. doi: 10.7705/biomedica.v34i2.3584
Velez, I. D., Santacruz, E., Kutcher, S. C., Duque, S. L., Uribe, A., Barajas, J., et al. (2020). The impact of city-wide deployment of Wolbachia-carrying mosquitoes on arboviral disease incidence in Medellín and Bello, Colombia: study protocol for an interrupted time-series analysis and a test-negative design study. F1000Research 8, 1327. doi: 10.12688/f1000research.19858.2
Vinayagam, S., Nirmolia, T., Chetry, S., Kumar, N. P., Saini, P., Bhattacharyya, D. R., et al. (2023). Molecular evidence of Wolbachia species in wild-caught Aedes albopictus and Aedes aegypti Mosquitoes in Four States of Northeast India. J. Trop. Med. 2023, 6678627. doi: 10.1155/2023/6678627
Vora, A., Zhou, W., Londono-Renteria, B., Woodson, M., Sherman, M. B., Colpitts, T. M., et al. (2018). Arthropod EVs mediate dengue virus transmission through interaction with a tetraspanin domain containing glycoprotein Tsp29Fb. Proc. Natl. Acad. Sci. U. S. A. 115, E6604–E6613. doi: 10.1073/pnas.1720125115
Walker, T., Johnson, P. H., Moreira, L. A., Iturbe-Ormaetxe, I., Frentiu, F. D., McMeniman, C. J., et al. (2011). The wMel Wolbachia strain blocks dengue and invades caged Aedes aegypti populations. Nature 476, 450–455. doi: 10.1038/NATURE10355
Wang, J.-M., Cheng, Y., Shi, Z.-K., Li, X.-F., Xing, L.-S., Jiang, H., et al. (2019). Aedes aegypti HPX8C modulates immune responses against viral infection. PLoS Negl. Trop. Dis. 13, e0007287. doi: 10.1371/journal.pntd.0007287
Wen, D., Ding, L. S., Zhang, Y., Li, X., Zhang, X., Yuan, F., et al. (2022). Suppression of flavivirus transmission from animal hosts to mosquitoes with a mosquito-delivered vaccine. Nat. Commun. 13, 35407. doi: 10.1038/s41467-022-35407-x
Werren, J. H. (1997). Biology of Wolbachia. Annu. Rev. Entomol. 42, 587–609. doi: 10.1146/annurev.ento.42.1.587
Wilkerson, R. C., Linton, Y. M., Fonseca, D. M., Schultz, T. R., Price, D. C., and Strickman, D. A. (2015). Making mosquito taxonomy useful: a stable classification of tribe aedini that balances utility with current knowledge of evolutionary relationships. PLoS ONE 10, e0133602. doi: 10.1371/JOURNAL.PONE.0133602
Wiwatanaratanabutr, I. (2013). Geographic distribution of wolbachial infections in mosquitoes from Thailand. J. Invertebr. Pathol. 114, 337–340. doi: 10.1016/j.jip.2013.04.011
Wu, J., Wang, Q., Wang, D., Wong, A. C. N., and Wang, G.-H. (2023). Axenic and gnotobiotic insect technologies in research on host–microbiota interactions. Trends Microbiol. 31, 858–871. doi: 10.1016/j.tim.2023.02.007
Wu, P., Sun, P., Nie, K., Zhu, Y., Shi, M., Xiao, C., et al. (2019). A gut commensal bacterium promotes mosquito permissiveness to arboviruses. Cell Host Microbe 25 101–112.e5. doi: 10.1016/J.CHOM.2018.11.004
Xi, Z., Ramirez, J. L., and Dimopoulos, G. (2008). The Aedes aegypti toll pathway controls dengue virus infection. PLoS Pathog. 4, 98. doi: 10.1371/JOURNAL.PPAT.1000098
Xiao, X., Liu, Y., Zhang, X., Wang, J., Li, Z., Pang, X., et al. (2014). Complement-related proteins control the flavivirus infection of Aedes aegypti by inducing antimicrobial peptides. PLoS Pathog. 10, e1004027. doi: 10.1371/journal.ppat.1004027
Xiao, X., Yang, L., Pang, X., Zhang, R., Zhu, Y., Wang, P., et al. (2017). A Mesh-Duox pathway regulates homeostasis in the insect gut. Nat. Microbiol. 2, 17020. doi: 10.1038/nmicrobiol.2017.20
Xiao, X., Zhang, R., Pang, X., Liang, G., Wang, P., and Cheng, G. (2015). A neuron-specific antiviral mechanism prevents lethal flaviviral infection of mosquitoes. PLoS Pathog. 11, e1004848. doi: 10.1371/journal.ppat.1004848
Yadav, K. K., Bora, A., Datta, S., Chandel, K., Gogoi, H. K., Prasad, G. B. K. S., et al. (2015). Molecular characterization of midgut microbiota of Aedes albopictus and Aedes aegypti from Arunachal Pradesh, India. Parasites and Vectors 8, 1–8. doi: 10.1186/s13071-015-1252-0
Yeh, S.-C., and Pompon, J. (2018). Flaviviruses produce a subgenomic flaviviral RNA that enhances mosquito transmission. DNA Cell Biol. 37, 154–159. doi: 10.1089/dna.2017.4059
Yezli, S., Yasir, M., Yassin, Y., Almazrua, A., Al-Subhi, T., Othman, N., et al. (2021). Lack of zika virus and other recognized flaviviruses among the mosquito vectors during and post the hajj mass gathering. Int. J. Environ. Res. Public Health 18, 126275. doi: 10.3390/ijerph18126275
Yin, C., Sun, P., Yu, X., Wang, P., and Cheng, G. (2020). Roles of symbiotic microorganisms in arboviral infection of arthropod vectors. Trends Parasitol. 36, 607–615. doi: 10.1016/J.PT.2020.04.009
Yu, X., Tong, L., Zhang, L., Yang, Y., Xiao, X., Zhu, Y., et al. (2022). Lipases secreted by a gut bacterium inhibit arbovirus transmission in mosquitoes. PLoS Pathog. 18, e1010552. doi: 10.1371/journal.ppat.1010552
Zakrzewski, M., Rašić, G., Darbro, J., Krause, L., Poo, Y. S., Filipović, I., et al. (2018). Mapping the virome in wild-caught Aedes aegypti from Cairns and Bangkok. Sci. Rep. 8, 1–12. doi: 10.1038/s41598-018-22945-y
Zhang, H., Gao, J., Ma, Z., Liu, Y., Wang, G., Liu, Q., et al. (2022). Wolbachia infection in field-collected Aedes aegypti in Yunnan Province, southwestern China. Front. Cell. Infect. Microbiol. 12, 809. doi: 10.3389/fcimb.2022.1082809
Keywords: Aedes aegypti, Aedes albopictus, vectorial competence, extracellular vesicles, RNA interference, insect specific virus, mosquito-borne disease, Wolbachia
Citation: Mantilla-Granados JS, Castellanos JE and Velandia-Romero ML (2024) A tangled threesome: understanding arbovirus infection in Aedes spp. and the effect of the mosquito microbiota. Front. Microbiol. 14:1287519. doi: 10.3389/fmicb.2023.1287519
Received: 01 September 2023; Accepted: 05 December 2023;
Published: 03 January 2024.
Edited by:
Marcos Rogério André, São Paulo State University, BrazilReviewed by:
Penghua Wang, UCONN Health, United StatesHumberto Lanz-Mendoza, National Institute of Public Health, Mexico
Copyright © 2024 Mantilla-Granados, Castellanos and Velandia-Romero. This is an open-access article distributed under the terms of the Creative Commons Attribution License (CC BY). The use, distribution or reproduction in other forums is permitted, provided the original author(s) and the copyright owner(s) are credited and that the original publication in this journal is cited, in accordance with accepted academic practice. No use, distribution or reproduction is permitted which does not comply with these terms.
*Correspondence: Juan S. Mantilla-Granados, am1hbnRpbGxhZ0B1bmJvc3F1ZS5lZHUuY28=