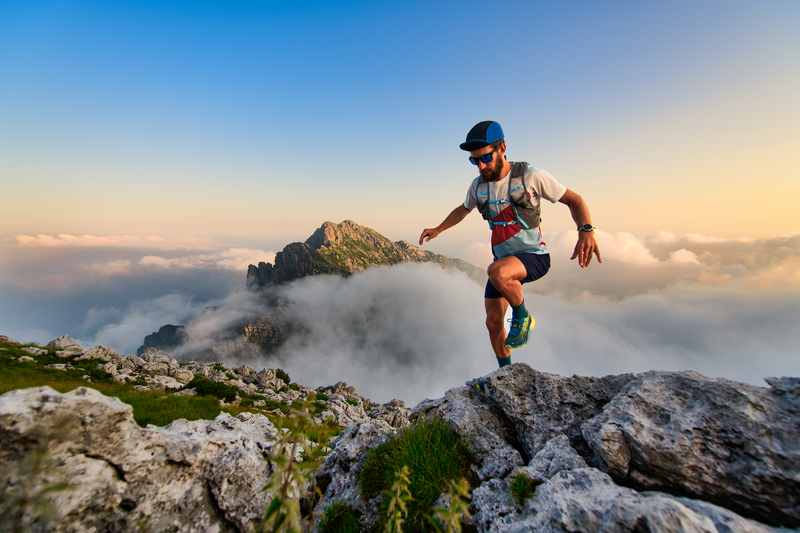
95% of researchers rate our articles as excellent or good
Learn more about the work of our research integrity team to safeguard the quality of each article we publish.
Find out more
REVIEW article
Front. Microbiol. , 14 November 2023
Sec. Antimicrobials, Resistance and Chemotherapy
Volume 14 - 2023 | https://doi.org/10.3389/fmicb.2023.1285543
This article is part of the Research Topic Biocontrol of phytopathogens-Recent progress for improvement in efficacy and understanding action mechanism View all 8 articles
Plant diseases caused by pathogenic microorganisms in agriculture present a considerable obstacle, resulting in approximately 30–40% crop damage. The use of conventional techniques to manage these microorganisms, i.e., applying chemical pesticides and antimicrobials, has been discovered to have adverse effects on human health and the environment. Furthermore, these methods have contributed to the emergence of resistance among phytopathogens. Consequently, it has become imperative to investigate natural alternatives to address this issue. The Streptomyces genus of gram-positive bacteria is a potentially viable natural alternative that has been extensively researched due to its capacity to generate diverse antimicrobial compounds, such as metabolites and organic compounds. Scientists globally use diverse approaches and methodologies to extract new bioactive compounds from these bacteria. The efficacy of bioactive compounds in mitigating various phytopathogens that pose a significant threat to crops and plants has been demonstrated. Hence, the Streptomyces genus exhibits potential as a biological control agent for combating plant pathogens. This review article aims to provide further insight into the Streptomyces genus as a source of antimicrobial compounds that can potentially be a biological control against plant pathogens. The investigation of various bioactive compounds synthesized by this genus can enhance our comprehension of their prospective utilization in agriculture.
Rhizosphere microorganisms play a significant role in producing diverse antimicrobial compounds, including metabolites and volatile organic components, aiding plant development and disease prevention (Gong et al., 2022). These compounds, produced by microorganisms, contain inorganic substances like NH3, CO2, and HCN that can modify the structures of other molecules, such as terpenes, ketones, sulfur-containing, and nitrogen-containing compounds, and aldehydes (Gong et al., 2022). Volatile organic substances possess distinct physical and chemical properties like high vapor pressure, low molecular weight, lipophilic moiety, low boiling point, and ease of movement through the gaseous phase, which facilitate their long-range air and soil diffusion (Gong et al., 2022). Bacteria and fungi have been applied as biofertilizers and biocontrol agents for several years (Qiu et al., 2019). Focusing solely on in vitro inhibition overlooks a range of ecological factors that can influence the establishment and persistence of biological control agents in their natural habitats, as highlighted by Kaminsky et al. (2019). When researching microbial methods for controlling plant diseases, the emphasis often falls on specific pathogen-host pairings, raising questions about how effective and applicable these biological control agents are across various plant pathogens and crop varieties, as discussed by LeBlanc (2022). The primary mechanism associated with the effectiveness of biocontrol is the production of multiple antibiotics. Many of these antimicrobial compounds contain enzymes with antifungal and antibacterial properties, such as proteases, lipases, or chitinases, which break down fungal cells and prevent plant-fungal infections. Among these antifungal enzymes, proteases are the most commonly encountered type, as noted by Vurukonda et al. (2018). Similarly, Streptomyces strains produce antagonistic compounds, including siderophores, to ward off bacterial infections and exhibit antiviral and antibiofilm properties as well. Streptomyces is also responsible for the production of industrially valuable enzymes and a diverse array of biologically active secondary metabolites, which encompass antibiotics, antioxidants, and anticancer agents (Butt et al., 2023). In a broader context, microbial inoculants show great promise as tools for maintaining agricultural sustainability. These microorganisms can enhance plant health and nutrient availability, as emphasized by Qiu et al. (2019). An especially effective approach against M. incognita involves combining abamectin and/or emamectin benzoate with P. lilacinum and rhizobacteria, as demonstrated by El-Ashry et al. (2021). This approach not only significantly reduces the formation of galls and the reproduction of M. incognita but also leads to marked improvements in various tomato growth parameters compared to the control group. The use of various bioagents, including abamectin, holds great promise as a potential antagonistic strategy against phytonematodes in protected agricultural environments, as indicated by El-Ashry et al. (2021).
Plant diseases are classified into two types based on the pathogens that cause them: infectious and non-infectious. Plant diseases that are not infectious do not spread from one plant to another, whereas infectious plant diseases can travel from an infected plant to another healthy plant (Nazarov et al., 2020). Both biotic and abiotic factors can influence plant disease development. Plant illnesses are distinguished by symptoms such as spotting (necrosis), pustules, wilting, rot, hyperplasia (overgrowth) and hypertrophy, mold, deformation, discoloration, mummification, and tissue destruction. Various fungi, bacteria, viruses, and other pathogens spread these diseases (Nazarov et al., 2020).
The potato, scientifically known as Solanum tuberosum L., is the fourth most common crop produced worldwide after maize, wheat, and rice, and it is vital to the economy’s functioning. China, India, and Ukraine are the world’s top three potato exporters. Potatoes are susceptible to various diseases, the most common being early blight, late blight, black scurf, powdery scab, and soft rot (Liu et al., 2020). Most chemical substances are not used in disease control due to stringent regulations regularly managed and implemented globally. As a result, eco-friendly alternatives to traditional disease and pest management techniques are presently being researched and developed (Liu et al., 2020). Some of these techniques also use specific agricultural practices comparable to those used to cultivate products in a controlled setting. The agricultural technique of early harvesting, which entails collecting crops soon after the haulm’s destruction, is occasionally employed as a preventive strategy to curb the spread of black scurf and other potato diseases (Liu et al., 2020).
In addition, biocontrol has been enhanced by implementing “green harvesting” techniques, which involve the utilization of the hyper-parasite Verticillium bigutatum (Ebrahimi-Zarandi et al., 2021). Trichoderma harzianum has demonstrated efficacy as a biological control method for the pathogen Rhizoctonia solani in peas, radishes, and beans under controlled greenhouse conditions. Nonetheless, the predictability of its efficacy in practical scenarios posed a challenge (Ebrahimi-Zarandi et al., 2021). The addition of T. harzianum to the soil decreased the dormant inoculum of Rhizoctonia solani, reducing the quantity of Rhizoctonia solani that accumulated in both greenhouse and field settings. The application of Laetisaria arvalis onto potato seeds and soil modification using this fungus has been found to hinder the contamination of potato effectively stems, stolons, and sclerotial growth caused by R. solani (Ebrahimi-Zarandi et al., 2021). Actinomycetes are a group of unicellular, filamentous bacteria that exhibit branching morphology. They are renowned for their ability to produce antibacterial compounds (Vurukonda et al., 2018).
Actinomycetes are ubiquitous microorganisms that inhabit diverse ecological niches such as soil, air, plant debris, and sedimentary environments (Bhatti et al., 2017). The ability of the Streptomyces genus to produce a diverse array of secondary metabolites is a well-established characteristic within the Actinomycetes order. The secondary metabolites present antibacterial properties against diverse plant and human diseases (Viaene et al., 2016). Using antibiotic-producing bacteria as a biocontrol agent in the soil is a viable alternative to chemical antimicrobials. Streptomyces is known for its ability to synthesize a diverse array of bioactive organic compounds that elicit direct or indirect effects on plant growth and development and its antimicrobial properties (Viaene et al., 2016). For example, an organic compound produced by Streptomyces yanglinensis strain 3–10 can inhibit A. flavus from growing and producing toxins in stored soybeans (Gong et al., 2022). In naturally existing disease-suppressive soils, these bacteria hinder the growth of plant pathogens by generating secondary metabolites and engaging in resource competition, such as for carbon and iron (LeBlanc, 2022). Streptomyces species recently discovered are being seriously considered in the agricultural biocontrol field. Streptomyces is characterized by its capability to synthesize antimicrobial compounds (such as toxins, VOCs, and antibiotics) (Vurukonda et al., 2018). This enables the Streptomyces to help plants against pathogens that might otherwise damage them. Phytopathogen interactions may suppress innate plant responses to pathogens (Vurukonda et al., 2018). Streptomyces bacteria can further alleviate disease pressure by stimulating elements of the plant’s immune system and enhancing plant productivity (Olanrewaju and Babalola, 2019). In addition, it impacts soil fertility through its influence on different factors and its role as a nutrient enhancer (Vurukonda et al., 2018). Despite their proven ability to mitigate fungal plant pathogen-related diseases and their remarkable species diversity, there has been limited commercial development of Streptomyces strains as biological control agents (LeBlanc, 2022). Only Actinovate (containing Streptomyces lydicus WYEC108) and Mycostop (containing Streptomyces griseoviridis K61) are registered as commercial biopesticides for use on multiple continents. The limited availability of such products can be attributed, in part, to the difficulties in formulating them for commercial use and the disparities between results obtained in laboratory studies and those observed in field applications (Vurukonda et al., 2018).
The broadening of international trade, climate change, and the limited availability of plant protection products have contributed to the development and appearance of new plant diseases, leading to substantial losses in agricultural production (Daranas et al., 2019). Understanding the factors that regulate disease emergence and spread has attracted remarkable attention from the world scientific community (Giraud et al., 2010). Plant diseases may express themselves in a variety of distinct manners depends upon type of pathogen i.e., fungal (Figure 1A) and bacterial (Figure 1B). The latest entrance of wheat blast-infected seed into Bangladesh is just one example of how pathogens can spread through damaged plant material, increasing the incidence, geographic range, or host range of the disease. The dispersal of pathogen spores over vast geographical regions can occur due to severe meteorological events like hurricanes (Ristaino et al., 2021).
Southern Mexican indigenous communities initially cultivated maize 10,000 years ago, and today, maize production surpasses that of wheat and rice in many countries. Maize serves various purposes, including human consumption, ethanol production, animal feed, corn starch, and corn syrup. It comes in six primary varieties: dent, pod, flint, popcorn, sweet corn, and flour. Sweet maize is grown for human consumption, while field maize is used for animal feed, maize oil production, whisky and bourbon distillation, and as a chemical feedstock (Chen et al., 2021). However, maize faces numerous threats, including fungal diseases, bacteria, viruses, nematodes, and parasitic plants. There are approximately 112 distinct diseases affecting maize crops, with around 70 transmitted through seeds (Xu et al., 2021). The most damaging seed-borne fungal diseases include Gibberella stalk rots (Gibberella zeae), Fusarium verticillioides, fake head smut (Ustilaginoidea virens), head smut, late wilt (Harpophora maydis), and black bundle (Acremonium maydis) (Lv et al., 2020). Other diseases include charcoal stalk rot, Anthracnose stalk rot (Colletotrichum graminicola), Fusarium, Gibberella, Aspergillus ear rots, charcoal ear rot (Macrophomina phaseolina), Corn smut (Ustilago maydis), Penicillium, common smut, downy mildew, black kernel rot, Horse’s tooth disease, Alternaria leaf blight, and Acremonium zeae stalk rot (Degani, 2021). Major seed-borne and seed-transmitted diseases of maize include black bundle, anthracnose rots, black kernel rot, crazy top downy mildew (Sclerophthora macrospora), brown stripe downy mildew, ear and root rot, Java downy mildew, horse’s tooth, Nigrospora ear rot, Philippine downy mildew disease, and Penicillium ear rot (Chen et al., 2021; Degani, 2021).
Glomerella graminicola, responsible for “Anthracnose Leaf Blight,” poses a threat to both wheat and maize crops and can also infect certain GMO cereals. This fungus causes anthracnose stalk rot worldwide, primarily damaging maize plants. The disease can manifest at any point during the growing season, leading to stem rot or leaf blight. Aspergillus flavus, a fungus with saprotrophic and pathogenic tendencies, is commonly found in various environments, especially cereal grains, tree nuts, and legumes (Chen et al., 2021). Post-harvest rot often occurs after harvesting or during storage and transport, with the name “flavus” referring to the yellow spore color. Macrophomina phaseolina, a Botryosphaeriaceae fungal plant disease, affects numerous plant species, including wheat, maize, peanuts, chickpeas, cabbage, tomatoes, soybeans, sweet onions, sunflowers, alfalfa, sesame, potatoes, and sorghum, causing damping-off, charcoal rot, seedling blight, and various stem and root rots (Degani, 2021; Degani and Dor, 2021). Sclerophthora macrospora, an Oomycota-pathogenic protist, infects maize, oats, rice, wheat, and turfgrass, leading to “crazy top” in maize and “yellow tuft” in turfgrass, particularly in Europe. Its lack of host specificity makes it a significant threat to various economically important crops. Gray Leaf Spot (GLS) is a major foliar fungal disease in maize caused by Cercospora zeina and Cercospora zeae, Cercospora maydis, with maize being its exclusive host. Cercospora zeae maydis populations are characterized by geographic distribution, growth rate, molecular analysis, and the presence of cercosporin toxins. Smut, or maize smut, is caused by the fungus Ustilago maydis, resulting in galls on the entire above-ground portion of the maize plant. Finally, southern maize leaf blight, caused by Bipolaris maydis, can be found wherever corn is grown worldwide (Yang et al., 2017). A list of major phytopathogens and corresponding disease caused in maize is listed in Table 1.
Table 1. List of major maize diseases, their causal agents, and yearly losses (Khokhar et al., 2014).
Wheat is the main source of calories and plant-derived protein in human food. According to the UN Food and Agricultural Organization, world wheat supply is sufficient. As the global population is expected to approach nine billion by 2050, production must expand (Figueroa et al., 2018). Wheat production is threatened by climate change, decreasing farmland, and unpredictable abiotic and biotic stresses. Diseases threaten world wheat supplies due to a perfect storm involving the advent of new pathogens and the reduction in wheat’s genetic variety brought on by the pursuit of elite high-performing cultivars. Pathogenic fungus limit wheat production. Rust infections have affected wheat production since its adoption. Wheat rust pathogens cause US$4.3–5.0 billion in annual losses worldwide (Juroszek and von Tiedemann, 2015). Rust species can infect diverse hosts, which is represented in formae speciales classification (ff. spp.). Puccinia graminis f. sp. tritici (Pgt), Puccinia striiformis f. tritici (Pst), and Puccinia triticina (Pt), are all members of the Basidiomycete family and the genus Puccinia (Singh et al., 2016). They are the pathogens that cause stem, stripe, and leaf rust, all diseases that affect wheat (Table 2).
Table 2. List of the most significant diseases of Wheat, their causative pathogens, and the disease’s primary symptoms (Figueroa et al., 2018; Waqar et al., 2018; Degani et al., 2021).
Wheat stem (black) rust, caused by Puccinia graminis f. sp. tritici Ericks and Henn (Pgt), is widespread but less prevalent than the other two wheat rusts. In warm, damp climates, Pgt causes masses of red-brick urediniospores on vulnerable plants’ leaf sheaths, stems, glumes, and awns (Martinelli et al., 2015; Figueroa et al., 2018). Stem rust reduces grain size and plant lodging, reducing yield. Stem rust has been largely managed in many regions of the world, but forecasting models assuming no persistent resistance anticipate global losses of 6.2 million metric tons per year or greater under severe epidemics. P. striiformis Westend. f. sp. tritici (Pst), a pathogen common in temperate regions with cool and wet weather, causes wheat stripe (yellow) rust (Figueroa et al., 2018). In vulnerable cultivars, stripe rust causes 100% yield losses. Pst affects 88% of wheat cultivars worldwide, costing almost US$1 billion annually. Wheat stripe rust has been documented in over 60 countries and Pst has spread globally in the last 50 years. Pst populations in Europe, Australia, and North America are clonal, but some have high genotypic diversity (McIntosh et al., 2018). Western China and Central Asia have polymorphism populations, supporting the Himalayan and adjacent regions as the pathogen diversity hotspot where sexual recombination is widespread (Sharma et al., 2020). Leaf rust, the most widespread of the three wheat rust diseases, is caused by Puccinia triticina Eriks. Mild, damp climates support the pathogen. Kernel weight and grain per head decrease due to illness. Leaf rot causes temporal and regional variance in grain losses, yet it is economically significant. Leaf rust is an issue because the pathogen is diverse, produces new virulence profiles, and adapts to several conditions. The Ascomycete fungus Zymoseptoria tritici, Parastagonospora nodorum, and Pyrenophora tritici-repentis cause STB, SNB, and TS (Moolhuijzen et al., 2022). Blotch illnesses are these disorders. STB, the main leaf disease of temperate wheat, is caused by Zymoseptoria tritici (Zt). The Ascomycete fungus Fusarium graminearum causes Fusarium head blight (FHB), often known as wheat scab or ear blight, which prematurely ages wheat heads (Fg). Many regional species complexes of cereal-infecting Fusarium species create severe FHB epidemics. FHB is the worst wheat floral disease worldwide. Rain during crop anthesis makes wheat harvests susceptible to FHB (Xu et al., 2022). FHB disease reduces grain yield and quality, lowering harvest and marketability and accumulating sesquiterpenoid trichothecene mycotoxins like deoxynivalenol (DON) in the grain, posing a food safety and health risk to humans, animals, and ecosystems (Xu et al., 2022). The pathogenic fungus Magnaporthe oryzae Triticum pathotype (MoT) causes wheat blast (WB) (Paul et al., 2022). WB affects the head. Small elliptical lesions to bleaching and empty spikes are common signs. Yields have dropped 40–100%. MoT-caused foliar lesions have also been reported, but their impact on grain output is unknown. WB development requires 25°C and a 10-h soaking period (Ceresini et al., 2018).
Rice is grown on 161 million acres worldwide, producing 678.7 million tons of rice. Asia produces 612 million tons of rice on 143 million acres (Asibi et al., 2019). With a projected 34% increase in the world population to 9.3 billion by 2050, the goal of more production while losing less seems compelling due to the threat of pathogens and pest introductions due to increased human mobility, global trade, and climate change. Rice plants are infected by many devastating diseases like blast, leaf blights, sheath blight, sheath rot, brown spot, bakanae disease, etc., caused by fungi, bacteria, and viruses as mentioned in Table 3 (Singh et al., 2019; Abade et al., 2021; Ngalimat et al., 2021). These diseases reduce crop yield and quality. Pathogens cause a loss of 15–30% rice yield, costing 33 billion USD yearly. Management and prevention of pathogen-caused illnesses that impair rice yields are major challenges. Due to population booms, rice experts have struggled to find ways to produce nutritious food grains at cheaper costs. These must be done in the face of unforgiving plant diseases. Rice hosts 58 fungal (43 seedborne or seed-transmittable), 12 bacterial, 17 viral and mycoplasma-like, and over 30 nematode species (Chukwu et al., 2019; Ngalimat et al., 2021). Pathogens infect seeds, propagules, roots, nodes, panicles, and leaves. Pathogens may cause local or systemic infections that cause minor to severe crop loss. As rice is grown worldwide, so are its pathogens. Blast, brown spot, bacterial blight, sheath blight, and tungro are still inflicting harm, while new minor diseases, including bakanae, fake smut, grain discoloration, early seedling blight, narrow brown spot, and sheath rot have become important issues (Abade et al., 2021). In many rice-growing countries, Helminthosporium oryzae, Rhizoctonia solani, Gerlachia, Pyricularia, Xanthomonas, Sclerotium, and others have caused foliar diseases and stem, root, or leaf sheath issues (Chukwu et al., 2019). These diseases might cause 1–100% losses depending on growth conditions, varietal sensitivity, etc. Rice sheath rot is a complex disease caused by fungal and bacterial phytopathogens. Sarocladium oryzae and Fusarium fujikuroi species (Fusarium fujikuroi, Fusarium verticillioides, and other Fusarium spp.) complex are the main phytopathogens (Sankaran et al., 2010). Pseudomonas fuscovaginae is a bacterial pathogen (Liu et al., 2014). The main pathogen, Sarocladium oryzae, was first reported from Taiwan in 1922 as Acrocylindrium oryzae. Sarocladium oryzae was renamed in 1975 after the genus was formed. Fusarium fujikuroi (Nirenberg) causes foot rot, or bakanae, in rice. In recent years, north Indian basmati-growing regions have been plagued by the disease (Sunder et al., 2014; Kumar et al., 2016). Punjab, Haryana, eastern UP, Uttarakhand, and New Delhi, notably basmati-growing districts, are plagued by disease. Bakanae disease causes grain sterility, reducing yield. The illness can reduce field yield and quality by 70% (Zhu et al., 2022). Ustilaginoidea virens causes false or green smut, a rice disease in India. False smut disease of rice outbreaks began in Tamil Nadu, India, and spread worldwide (Prasher and Sharma, 2022). Smut balls replace one or more ripe plant kernels. Powdery dark green spores discharge as smut balls burst. U. virens thrives in 25–30°C and >90% relative humidity (Abade et al., 2021). Early seedling blight (Sclerotium oryzae, Sclerotium rolfsii) is a major developing disease in nursery beds during the cold season (winter months) (Gaire et al., 2023). Seedlings in the nursery bed begin withering. Later, fungal mycelia cover the seedlings. Fungal mats cover most roots and branches. Sclerotial bodies evolve into dark brown hard structures from white mustard-shaped ones (Chukwu et al., 2019; Abade et al., 2021). In Asia, high-yielding hybrid varieties have replaced traditional landraces, and chemical fertilizers and plant growth hormones have intensified crop production. Rice pathogens—the clever enemies—have become more relevant under these shifting settings. Management techniques had to include many formerly unimportant diseases. Many new pathotypes have emerged and some have disappeared (Parajuli et al., 2022). A list of major phytopathogens and related diseases caused in rice is listed in Table 3.
Table 3. List of the most significant diseases of rice, their causative pathogens, and the disease’s primary symptoms (Mehta et al., 2019; Singh et al., 2019; Abade et al., 2021; Ngalimat et al., 2021).
The global potato production landscape is vast, with approximately 378 million tons of potatoes cultivated across roughly 19 million hectares of farmland. The majority of potato cultivation takes place in the northern hemisphere’s temperate regions due to the crop’s susceptibility to frost during the summer months. Potatoes exhibit adaptability to various environmental conditions, leading to significant production increases in several countries over the past two decades, particularly in emerging nations, with a notable surge in Asia and Africa Campos and Ortiz (2020). However, potato production faces numerous challenges, primarily from various diseases that affect both the tubers and the plants. More than 40 diseases can affect potatoes, caused by a range of microorganisms such as viruses, fungi, nematodes, insects, and bacteria. These diseases can lead to quality deterioration and yield losses of up to 22% in the potato production system, either directly or indirectly (Tiwari et al., 2020). One of the historically significant diseases is late blight, caused by the fungal pathogen Phytophthora infestans, which led to the devastating Irish potato famine in the 1840s. Late blight continues to pose a significant challenge to potato producers worldwide, with changing characteristics, including an increased affinity for tomato and potato plants (Kumbar et al., 2019). Other notable diseases mentioned include potato silver scurf caused by Helminthosporium solani, Rhizoctonia solani complex disease, and early blight caused by Alternaria solani. Each of these diseases can have a detrimental impact on potato production, leading to reduced yields and compromised tuber quality (Tomilova et al., 2020; Gorai et al., 2021; Tiwari et al., 2022).
Numerous phytopathogenic microorganisms generate active extracellular proteinases, which, in conjunction with other enzymes, serve a crucial function in their pathogenic processes. These enzymes include polygalacturonases, pectolyases, and xylanases, among others (Valueva and Mosolov, 2004).
Fungi are likely the most diverse group of environmentally and economically important organisms, particularly concerning their plant pathogenicity. Most fungal plant pathogens are classified within the phyla Basidiomycota and Ascomycota. Plant infections are categorized into various classes within the ascomycetes group, including Dothideomycetes (which encompasses Cladosporium spp.), Leotiomycetes (such as Botrytis spp.), and Sordariomycetes (including Magnaporthe spp.). The basidiomycetes, spread among the sub-phylum of Ustilaginomycotina, comprise two significant groups of plant pathogens: rusts (Pucciniomycetes) and smuts (Doehlemann et al., 2017). Crops are susceptible to fungal infections, either individually or in conjunction with other phytopathogens, throughout their growth cycle from seedling to seed maturation under natural conditions (Narayanasamy, 2011). Plant pathogenic fungi are responsible for causing various diseases in plants, including but not limited to Anthracnose, root rot, scab, canker, powdery mildew, dieback, leaf spot, blight, gall, damping off, rust, and wilt. These diseases are widely prevalent and can significantly impact plant health and productivity (Iqbal et al., 2018). Numerous phytopathogenic fungi induce crop diseases, leading to crop damage as mentioned in Table 4.
Table 4. Fungal phytopathogens with their respective host and disease causes (Narayanasamy, 2011; Doehlemann et al., 2017; Möller and Stukenbrock, 2017; Iqbal et al., 2018; Hariharan and Prasannath, 2021).
Bacterial infections have been observed to affect diverse plant species across the globe, causing various plant disease (Table 5). Phytopathogenic bacteria are a group of microorganisms that can inhabit the tissues or surfaces of plants and are of significant interest to the agriculture industry due to their impact on food-producing plants. Some of the symptoms produced by these organisms include cankers, spots, tissue rots, blights, and/or hormonal imbalances. Additionally, these organisms have the potential to induce excessive plant growth, promote root proliferation, trigger leaf epinasty, and interfere with growth (Kannan et al., 2015). Gram-negative bacteria constitute the majority (95%) of pathogenic bacteria, and gram-positive bacteria, which make up less than 5% of pathogenic bacteria, exhibit these differences (Liu et al., 2020). Bacterial infections substantially impact the sustainability of farming practices worldwide, potentially leading to negative consequences (Rubab et al., 2018). Several diseases are detected pre-harvest, and certain ones may continue to affect the quality of the product post-harvest.
Table 5. Bacterial plant pathogens with their host and disease (Kannan et al., 2015; Shafi et al., 2017; El-Sayed et al., 2019; Petersen et al., 2021).
Botrytis cinerea is a fungal pathogen that is responsible for the development of gray mold disease. This pathogen can potentially cause significant damage to plant products and has been reported to infect more than 200 plant species (Buttimer et al., 2017). Other possibly harmful infections include Colletotrichum musae, which causes blossom end rot illnesses and Anthracnose in bananas, Alternata alternata, which causes alternaria rot in cherries; and Penicillium expansum, which causes blue mold in apples. Moreover, due to other novel attempts, many infections can be found before and after harvesting.
Viruses are non-cellular infectious agents with a unique ability to replicate only within living host cells. They can infect a wide range of organisms, including plants, animals, bacteria, and archaea. Viruses can either integrate into the host’s genome as inactive proviruses or actively replicate and manipulate the host’s biological processes. Suppression of viral gene transcription can lead to latent infections. Plants viruses primarily exist as single-stranded (ss) and double-stranded (ds) RNA viruses, as well as single-stranded and DNA-containing retroviruses. To infect plant cells, virions enter the cytoplasm through wounds created by mechanical damage to the cuticle and cell wall since they cannot pass through these barriers on their own. Once inside the cell, the virus un-coats, and DNA-containing viruses must penetrate the nucleus to initiate transcription and mRNA synthesis. Viruses encode at least two types of proteins: replication proteins required for nucleic acid synthesis and structural proteins forming the capsid. Some viruses also possess proteins responsible for virion movement between plant cells. Plant viruses can be transmitted both vertically (from parents to offspring) and horizontally (from diseased plants to healthy ones). They use small intercellular channels called plasmodesmata to penetrate neighboring cells, facilitating local infections. To infect an entire plant, a virus must enter the vascular system, moving passively through the phloem sieve tubes with the flow of substances, allowing it to infect cells distant from the primary site of infection. Some viruses are highly stable and resistant to heat, remaining viable in plant cells and derived products for extended periods. However, many plant viruses actively spread from infected plants to healthy ones through carrier organisms, divided into mechanical vectors (agents that do not propagate the virus) and biological vectors (where part of the viral life cycle occurs). Common vectors include arthropods, nematodes, and plant-feeding fungi.
Plant viruses pose a significant threat to various crops, with economic losses ranking second only to losses caused by other pathogens. They can infect numerous plant species, leading to potential crop losses of up to 98%, especially in subtropical and tropical regions. Notably, some infections may not exhibit obvious symptoms. Virus diseases in plants manifest in various ways, including growth suppression, discoloration (such as mosaic patterns and chlorosis), deformations (wrinkling, corrugation), necrosis, and impaired reproduction (flower sterility, parthenocarpy, shedding of flowers and ovaries).
Apart from viruses, viroids are another group of infectious agents, circular RNAs that cause diseases in plants and animals. They belong to the viral families Pospiviroidae and Avsunviroidae. Viroids lack a protein envelope (capsid) and consist of covalently linked ssRNA molecules, which are significantly shorter than viral genomes. Viroids cannot replicate autonomously and likely utilize host cell enzymes for replication through mechanisms such as rolling-circle replication. The exact molecular mechanisms of viroid pathogenicity are not fully understood but are believed to involve interactions with cellular kinases, gene expression alterations, protein induction, RNA interference, splicing disruption, and rRNA gene demethylation. Small changes in viroid nucleotide sequences can significantly affect their pathogenicity. Common symptoms of viroid diseases in plants include reduced growth, discoloration (chlorosis and anthocyanosis), and deformations in various plant organs.
Phytoplasmosis has a profound negative impact on both crop yield and quality. The extent of crop losses varies across different plants, with eggplants experiencing a 40% reduction, tomatoes suffering a 60% decrease, peppers facing a staggering 93% loss, potatoes encountering losses ranging from 30 to 80%, and cucumbers being particularly hard-hit with a 100% loss (Shafi et al., 2017). Plants affected by phytoplasmosis exhibit various disorders in their reproductive organs, including virescence, which involves the greening of flowers and the loss of normal pigmentation. Additionally, they may develop phyllodia, where parts of a flower transform into leaf-like structures, and proliferation, which leads to the emergence of multiple “pseudo” flowers instead of one. Furthermore, phytoplasmosis can result in symptoms like witches’ broom (excessive bushiness), dwarfism, wilting of plants, and leaf deformities. It’s worth noting that there is only one documented case of positive phytoplasmosis, which has a beneficial effect: phytoplasmosis in poinsettias, a popular seasonal ornamental plant, is known to have economically advantageous outcomes.
Managing plant diseases caused by microorganisms is a crucial aspect of sustainable agriculture, which aims to produce healthy crops while minimizing environmental damage. Biological control is a promising approach that utilizes living organisms as natural enemies of plant pathogens (Bonaterra et al., 2022). The occurrence frequency of phytonematode genera revealed that Meloidogyne (35.41%), Pratylenchus (17.18%), Tylenchorhynchus (15.62%), and Tylenchus (11.45%) were the most prevalent genera among phytonematodes. Meloidogyne had the highest prominence value, followed by Pratylenchus and Tylenchus. The effects of animal manure treatments were sustained for an extended period in protective cucumber plants compared to the control treatment, resulting in the lowest population density of Helicotylenchus spp., Tylenchorhynchus spp., and Pratylenchus spp., as well as a significant reduction in galling and reproduction of Meloidogyne incognita in greenhouse experiment whereas in the field experiment, combinations of test manures and PGPR led to the highest improvement in tomato yield per plant compared to using animal manures alone. This coincided with reduced numbers of M. incognita populations and the maintenance of sustainable levels of beneficial nematodes (FLNs and PNs). Consequently, the application of a mixture of animal manures and PGPR emerges as a promising alternative to chemical pesticides for the biological control of nematode (Ali et al., 2022). Various Streptomyces strains underwent assessment for their potential to lower the population levels of the root-lesion nematode (RLN), Pratylenchus penetrans, within the roots of alfalfa (Medicago sativa) through experiments conducted in growth chambers. These same strains were previously observed to effectively control potato scab disease, which is caused by Streptomyces scabies, during field trials. Moreover, they exhibited the capability to restrain the in vitro proliferation of a diverse array of plant-pathogenic fungi and bacteria (Samac and Kinkel, 2001). An active compound possessing nematicidal properties was extracted from a strain identified as Streptomyces sp. 680560, and its structure was subsequently determined to be teleocidin B4. The nematicidal efficacy of this isolated compound, teleocidin B4, was subsequently verified (Kang et al., 2021). These actinobacteria typically inhabit the rhizosphere and rhizoplane, influencing the composition of the microbial community within the soil-root system. In some instances, they act as endophytes, forming a closer relationship with plant tissues. The mechanisms through which they promote plant growth include biofertilization and biostimulation effects, while their role in bioprotection relies on competitive mechanisms and the production of secondary metabolites. Among these metabolites, several compounds exhibit insecticidal properties, such as antimycin A, flavensomycin, macrotetralides, piericidins, and prasinons. Streptomycetes also produce highly effective and commercially successful metabolites, including avermectins, which are derivatives of macrocyclic lactones that impact the nervous system of insects. Avermectins interact with gamma-aminobutyric acid (GABA) receptors, initiating a series of events that lead to the inhibition of neurotransmission, resulting in neuromuscular paralysis and the eventual demise of the insects (Ruiu, 2020). Spectinabilin, a compound exhibiting nematicidal properties against both C. elegans and the southern root-knot nematode M. incognita, was isolated and characterized from Streptomyces sp. DT10. Spectinabilin demonstrated notable nematicidal activity against C. elegans L1 and L4 larvae, and it significantly impaired the mobility of C. elegans L4 larvae. Subsequent analysis indicated that spectinabilin operates on distinct targets compared to commonly used nematode treatments like abamectin and phosphine thiazole. These discoveries open up new possibilities for the development of more effective and environmentally sustainable strategies to combat parasitic nematodes while mitigating issues associated with resistance in these organisms (Sun et al., 2023). Meeting the growing demand for agricultural products requires optimizing production potential and minimizing crop losses attributed to common plant-parasitic nematodes. While chemical-based nematode management has proven effective in mitigating nematode-induced damage and yield reductions, the improper and irresponsible use of synthetic pesticides can have adverse effects on fauna, biodiversity, and natural predators and parasites. Farmers highly value biocontrol agents as a nematode management approach because it not only ensures safety but also reduces environmental pollution. There is a growing emphasis on the biological control of plant-parasitic nematodes through the utilization of plant growth-promoting rhizobacteria (PGPR) as biopesticides. Additionally, PGPR strains have the capability to enhance plant growth by producing a variety of secondary metabolites (Aioub et al., 2022). This method can be used alone or in conjunction with traditional pesticides, and it is more efficient and less harmful to the ecosystem. The use of biocides ranges from microbes and fungi to viruses and has gained attention from researchers worldwide due to their eco-friendly and effective properties. Agriculture is vital for sustaining the world’s population, and there is a growing need for increased crop production. However, the impact of weeds, plant diseases, and pests can cause significant damage to crops, leading to a decrease in yield and quality. The extensive use of chemical fertilizers and pesticides has resulted in environmental damage, the evolution of pesticide-resistant insects, and potential harm to human health. Therefore, alternative approaches, such as biological control, have been explored to minimize the impact of plant pathogens on crops (Keswani et al., 2019).
Fungi have gained increased attention as biocontrol agents due to their superior rates of sexual and asexual reproduction, rapid generation, and target-specificity (Thambugala et al., 2020). Although phytopathogenic fungi are a common source of plant disease, many fungal species have developed defense mechanisms to repel these invaders. Only a few species of Trichoderma are cited as biological control agents due to their strong opportunistic traits (Woo et al., 2014). Trichoderma spp. has a diverse metabolism and can use many substrates, producing hundreds of chemicals dispersed in more than 120 secondary metabolite structures (Malmierca et al., 2016). Plant growth-promoting bacteria (PGPB) is a type of bacteria that supports plant growth (Morales-Cedeño et al., 2021). Bacterial 1-aminocyclopropane-1-carboxylic acid (ACC) deaminase converts the ACC molecule to ammonia and -ketobutyrate under stress or pathogen assault, preventing the production of ethylene and promoting plant growth and longevity (Orozco-Mosqueda et al., 2020). Recently, there has been a surge in identifying and characterizing Bacilli strains with enhanced resistance to salty conditions, promotes numerous plant specie’s growth. Bacilli interact with plants in various beneficial ways (Tahir et al., 2019).
Actinomycetes are distributed in various natural ecosystems such as rhizosphere soil, agricultural soil, marine and freshwater habitats, limestone, sponges, volcanic cave, desert, insect gut, goat feces, and endophytic plants, as reported by Selim et al. (2021). These microorganisms can produce secondary metabolites that are not directly linked to their growth, maintenance, or reproduction, making them unique due to their broad metabolic range and potential for generating novel compounds (Elmallah et al., 2020). Marine Actinomycetes, in particular, have been found to produce a diverse array of antibiotics, accounting for over 45% of ecologically significant bioactive metabolites (Jakubiec-krzesniak et al., 2018).
More than 700 species constitute the family Streptomycetaceae (order Actinomycetales), of which the genus Streptomyces is the most prominent. Their DNA has a G + C content greater than 70% (Law et al., 2017; Olanrewaju and Babalola, 2019; Pacios-Michelena et al., 2021) and they are Gram-positive, neutrophilic, facultatively aerobic, mesophilic filamentous bacteria with a growth temperature between 25 and 35°C (Law et al., 2017). Biosynthetic gene clusters (BGC) in Streptomyces bacteria code for the enzymes needed to produce the bacterium’s secondary metabolites (SMs). In most cases, they are governed by complicated and stringent transcriptional regulation. Various nutritional and environmental factors activate distinct regulatory mechanisms that control Streptomyces SM production. Most Streptomyces are successful colonizers of the rhizosphere. Endophytes are a subset that lives within the host plant and colonizes its tissues (Trejo-Estrada et al., 1998; Muangham et al., 2015; Thambugala et al., 2020). The production of cellulases, chitinases, lipases, and beta-1,3-glucanases, as well as the synthesis of siderophores, phytohormones, or amino acids, may be responsible for these traits. There are three basic strategies by which Streptomyces can exert its antagonistic actions against pathogens: compete for space and nutrients, antibiosis, and parasitism (Sadeghi et al., 2017).
Certain Streptomyces strains demonstrate antagonistic and antimicrobial capabilities against pathogens found in aquaculture environments. They achieve this by generating inhibitory substances like bacteriocins, siderophores, hydrogen peroxide, and organic acids, which are used to vie for nutrients and attachment sites within the host, thereby inhibiting the growth of these pathogens (Butt et al., 2023).
Streptomyces many secondary metabolites which adversely affect the growth of pathogens, resulting in antibiosis (de Lima Procópio et al., 2012).
The main genus of Actinomycetes is Streptomyces. Through competition or parasitism, its member species manage plant parasitic nematodes (Jin et al., 2019).
Plant root surfaces are a prime colonization site for Streptomyces spp., which can also thrive in a wide range of soil types and release spores to ensure their survival in harsh environments (Law et al., 2017). Streptomyces strains produce a wide array of antibiotics, volatile organic compounds (VOC), which are effective against diseases and disrupt bacterial cell-cell communication (quorum sensing), as well as a number of enzymes that destroy the cell wall of fungi as shown in Figures 2, 3. During their metabolic activities, Streptomycetes produce a number of lytic enzymes. They significantly contribute to carbon recycling by decomposing various biopolymers derived from dead plants and animal debris. These organic molecules are broken down by exoenzymes; they include xylan, chitin, and cellulose. Carbohydrate importers, which typically recognize mono- and disaccharides, direct the products into the cell. Bentley et al. (2002) sequenced all the genomes of the model organism Streptomyces coelicolor A3 and found 172 genes encoding secreted proteins like hydrolases, chitinases, cellulases, lipases, nucleases, and proteases, as well as 81 ATP-binding cassette (ABC) permeases that may be used to uptake sugars, oligopeptides, nucleosides, and drugs. Streptomycetes have a stronger metabolic capacity than other bacteria by a factor of 5–10, as evidenced by their larger levels of exoenzymes and ABC systems (Vurukonda et al., 2018). Streptomyces strains can be cultivated and nourished in a variety of ways to considerably alter their ability to produce antimicrobials. Lack of nutrients can affect hyphae formation, which in turn leads to the production of these antimicrobial chemicals. Cells perceive these environmental signals as stress, and thus, these compounds are categorized as stress metabolites owing to their function in enabling adaptability. In addition, Streptomyces antibiotic regulatory proteins and lysosomal acid lipase are two examples of species-specific enzymes that play a vital role in controlling metabolic pathways. The metabolites produced by Streptomyces are dependent on the nature of the signals it receives and sends. Invasion by pathogens triggers a signal, food deficiency triggers a signal, etc. Consequently, the ability of the producer organism and the composition of the culture medium are crucial factors in the production of secondary metabolites (Jakubiec-krzesniak et al., 2018; Keswani et al., 2019). Fermentation can be a key step in the production of secondary metabolites. Nutrients (nitrogen, phosphorus source, and carbon), growth rate, enzyme inactivation, and some variable factors (oxygen supply, temperature, light, and pH) are the primary factors that can be modified. Additionally, Actinomycetes’ strain-specific synthesis of important metabolites varies qualitatively and quantitatively (Muangham et al., 2015).
Figure 3. Streptomyces strains as a potential biocontrol agent against various pathogenic organisms and their mechanisms.
Furthermore, the diverse metabolic capabilities of the organism have facilitated their ability to inhabit various ecological niches and utilize a wide range of carbon and nitrogen substrates. While the pH range of the genus is typically between 6.5 and 8, it has been observed that specific strains can thrive in environments with a pH of 9 or greater. Streptomyces can produce mycelia and spores, which serve as a mechanism of spreading and resistance, facilitating survival during prolonged periods of water scarcity and nutrient deprivation (Pacios-Michelena et al., 2021). The genome of Streptomyces harbors over 20 gene clusters that synthesize secondary metabolites of notable antimicrobial significance, that exhibit the potential to address antimicrobial resistance (Hopwood, 2019). Streptomyces is characterized by its DNA-DNA hybridization and 16S rDNA analysis in contrast to other actinobacteria (Kumar et al., 2012). Actinomycetes isolated from hypersaline soils, such as Streptomyces alboflavus, Micromonospora species, Nocardia species, and Streptomyces griseoflavus, have also demonstrated antifungal activity against various fungal species, including Aspergillus niger, Fusarium species, and Cryptococcus species (Karuppiah and Mustaffa, 2013). Hypersaline soils are characterized by a salt content ranging from 9 to 23% and are found in various habitats such as saline soils, saline lakes, and salterns.
Recent studies have emphasized using Streptomyces species as a biocontrol agent to counteract bacterial and fungi diseases that can affect plants (Suárez-Moreno et al., 2019). Multiple studies have shown that Streptomycetes exhibit efficacy against many phytopathogenic fungi, such as Magnaporthe oryzae, Pyrrhoderma noxium, Phytophthora capsici, Rhizoctonia solani, Puccinia triticina, Pythium aphanidermatum, Fusarium verticillioides, Botrytis cinerea, and Pythium ultimum (Panchalingam et al., 2022). Moreover, as numerous investigations indicate, Streptomyces exhibits significant potential for synthesizing secondary metabolites, including antibiotics, growth promoters, and herbicides (Harir et al., 2018). The genus, as mentioned above, is accountable for synthesizing 70% of the antibiotics currently utilized for therapeutic purposes in the treatment of diverse human diseases and constitutes 66.67% of all antibiotics that occur naturally (Sivalingam et al., 2019). The quantity of antimicrobial compounds produced by Streptomyces strains is contingent upon nutritional and environmental factors. The development of fungal hyphae and the production of antimicrobial compounds may be impeded in instances of nutrient deficiency. These compounds are generally identified as stress metabolites due to their adaptation function. The production of antibiotics is facilitated by different species and strains of Streptomyces, as presented in Table 6. The control of metabolic pathways is contingent upon the existence of various enzymes that are limited to specific organisms. These enzymes include lysosomal-acid lipase and the Streptomyces bacteria’s regulating proteins for drugs.
Table 6. Antibiotics produced by Streptomyces species along with their target pathogens and applications.
The metabolites synthesized by Streptomyces depend on the signals the organism receives and transmits. An example of this is the activation of a signal in response to the presence of a pathogen or a deficiency in nutrients. Butyrolactone is a key signaling molecule that promotes intercellular communication among PSPG (Plant Secondary Product Glycosyltransferases) cells via direct cell-to-cell contact (Olanrewaju and Babalola, 2019). The Streptomyces strain C, which produces siderophores, has been found to enhance the growth of wheat and its iron uptake capacity under saltwater conditions (Sadeghi et al., 2017). Siderophores legonoxamine A and B, members of the hydroxamate family, are produced by the strain of Streptomyces known as MA37 (Maglangit et al., 2019). Four strains of Streptomyces, namely S. violaceusniger YCED9, S. lydicus WYEC108, S. saraceticus KH400, and S. griseoviridis K61, are represented by six distinct marketable combinations. These strains are used to biocontrol bacterial and fungal diseases associated with the soil (Palaniyandi et al., 2013).
The agricultural sector commonly engages in the inappropriate disposal of pesticides, resulting in the chemical pollution of the nearby environment’s soil, air, and water. Pesticides that pose a significant risk to human wellbeing are readily available and can be obtained through authorized and unauthorized means, presenting a global concern. The detection of 10.5 metric tons of banned pesticides and/or insecticides with fraudulent labeling being imported into the European Union from China underscores the prevalence of uncontrolled and illicit pesticide application. The circumstance mentioned above presents a noteworthy peril to the wellbeing of the general populace, given that individuals could potentially encounter perilous concentrations of said chemicals, thereby leading to the grave and extensive health ramifications (Abraham and Gajendiran, 2019). Various types of diseases caused by bacteria, viruses, fungi, and nematodes substantially impact agricultural productivity on a global scale. The decrease in global food production caused by plant diseases was attributed to fungal and bacterial pathogens, which accounted for 42 and 27% of the decline, respectively. The potential exacerbation of this trend may be attributed to the impact of climate change. The utilization of biological control has experienced a notable increase due to its various advantages, including minimal residual toxicity, reduced environmental contamination, and more excellent pest resistance compared to chemical control (Le et al., 2022). The abundance of agro-active substances and biological control compounds found in Actinomycetes has captured the interest of researchers in diverse agricultural domains, owing to their considerable potential for practical utilization (Tables 7, 8). The Streptomyces genus has garnered considerable significance in the pharmaceutical sector owing to its capacity to synthesize one or more categories of antibiotics in nearly 75% of its constituent species.
Furthermore, this particular genus has significantly contributed to the advancement of approximately sixty percent of novel insecticides and herbicides within the past 30 years. A significant amount of research is being conducted worldwide to develop efficacious compositions that incorporate Actinomycetes as a bioactive component (Le et al., 2022). Streptomyces is a noteworthy origin of biologically active substances, including vitamins, plant growth hormones, alkaloids, enzymes, and enzyme inhibitors. According to Shojaee et al. (2014), the biological stabilization of soils and the promotion of crop productivity are facilitated by soil Streptomycetes, which aid in the decomposition of organic materials.
The genus Streptomyces, which is included in the phylum Actinobacteria, exhibits a wide variety of processes, including the production of antibiotics, the degrading of fungal cell walls, engaging in competitive interactions, and hyper-parasitism. The effectiveness of these mechanisms of operation has been exhibited as biological control compounds, either autonomously or in combination with other biological control compounds. Numerous Streptomyces species, including S. lividans, S. plicatus, S. humidus, S. scabies, S. violaceusniger, S. aureofaciens, S. hygroscopicus, S. olivaceoviridis, S. lydicus, S. avermitilis, and S. roseflavus, have been identified for their capacity to produce highly effective bioactive substances that demonstrate effectiveness against diverse pathogenic fungi. Additionally, certain strains of this genus have been carefully chosen for their effectiveness as biological agents in managing diverse plant diseases (Kanini et al., 2013). The efficacy of products obtained from diverse Streptomyces species has been successfully demonstrated through production and experimentation, specifically in managing select plant diseases. One instance of pathogen inhibition entails the utilization of Streptomyces griseoviridis strain K61 to inhibit Ceratocystis radicicola, the etiological agent accountable for inducing black burns on date palms (MycostopR®). In addition, the bacterial strain S. lydicus WYEC108 was utilized to produce Micro108® and Actinovate® commercially used biocontrol products. Similarly, the strain S. saraceticus KH400, in combination with iron, was employed in the manufacturing process of YAN TEN® biocontrol product (Bubici, 2018).
Streptomyces hold promise in agriculture, however, there are several challenges associated with its use in controlling plant diseases that need to be addressed for its effective deployment (Solanki et al., 2016; Le et al., 2021).
Streptomyces strains vary widely in their effectiveness against different plant pathogens. Finding the right strain that targets a particular disease without harming beneficial microorganisms can be challenging. Ensuring specificity is crucial to prevent unintended ecological disruptions in the soil (Kasuga et al., 2017).
Streptomyces performance can be greatly influenced by environmental factors such as temperature, humidity, and soil pH. Maintaining optimal conditions for its growth and activity can be demanding, limiting its effectiveness in diverse agricultural settings (Le et al., 2021).
When introduced into the soil, Streptomyces must compete with the native microbial community for resources and niche space. This competition can reduce the survival and establishment of Streptomyces, undermining its ability to suppress pathogens effectively (Nguyen et al., 2015).
Streptomyces can have limited persistence in the soil. Its beneficial effects may diminish over time, necessitating repeated applications, which can be costly and labor-intensive for farmers.
Just as with chemical pesticides, there is a risk of pathogens developing resistance to Streptomyces-based biocontrol agents. The rapid evolution of resistance can render these agents ineffective over time, necessitating the development of new strains or strategies (Muangham et al., 2015).
Regulatory approval for the use of Streptomyces-based biocontrol agents can be a lengthy and costly process. Ensuring that these agents meet safety and efficacy standards is essential but can impede their widespread adoption (Sun et al., 2023).
Mass production and formulation of Streptomyces-based biocontrol agents can be complex and expensive. Achieving consistency in terms of viable spore count and product quality is crucial for their commercial viability (Sun et al., 2023).
Incorporating Streptomyces-based biocontrol into existing agricultural practices can be challenging. Farmers may need education and support to understand how to effectively integrate these agents with their current pest management strategies.
Streptomyces’ effectiveness can vary from season to season and between different crop types. Predicting its performance accurately can be difficult, making it less reliable than some chemical alternatives (Zheng et al., 2019).
There can be resistance from consumers who are unfamiliar with or skeptical of biological control methods. Public perception and acceptance are essential factors in the adoption of Streptomyces-based solutions (Wu et al., 2015).
In conclusion, while Streptomyces shows promise as a biocontrol agent for plant diseases, it is not without its challenges. Addressing these challenges requires ongoing research and development efforts, as well as collaboration between scientists, regulators, and farmers. By overcoming these obstacles, Streptomyces-based biocontrol agents could play a more prominent role in sustainable agriculture, reducing our reliance on chemical pesticides and promoting healthier ecosystems.
It is of utmost importance to identify antimicrobial compounds that are both ecologically sustainable and derived from natural sources in order to effectively manage plant diseases caused by microorganisms. The Streptomyces genus has been extensively researched and utilized as a biological control strategy against plant pathogenic bacteria. Streptomyces spp. show significant potential in current agricultural practices, such as their use as biofertilizers and for biological control, which may contribute to their continued dominance in the market economy. The biotechnological potential exhibited by these bacteria makes them highly promising microorganism for agricultural applications. However, it is crucial to carefully consider various unresolved issues in order to replicate the outcomes observed in controlled laboratory environments during extensive commercialization and field experiments. Therefore, the cultivation of Streptomyces-derived biological compounds is imperative for enhancing crop health and promoting sustainable agriculture.
Streptomyces spp. have shown significant promise as biological control agents for plant pathogens. These filamentous, Gram-positive bacteria are known for their ability to produce a wide range of secondary metabolites, including antibiotics and antifungal compounds, which can inhibit the growth of plant pathogens. Here are some future perspectives:
a. Understanding Diversity: Researchers are likely to continue exploring the diversity within Streptomyces species. Different strains may possess varying abilities to produce bioactive compounds, and understanding this diversity can lead to the discovery of more potent biological control agents.
b. Metagenomics and Genomics: Advances in metagenomics and genomics can aid in the identification and characterization of novel Streptomyces strains with enhanced biocontrol properties. These techniques can also help in understanding the genetic basis of antibiotic production in Streptomyces.
c. Synthetic Biology: Synthetic biology approaches may be employed to engineer Streptomyces strains for improved biocontrol capabilities. This could involve enhancing the production of specific antifungal or antibacterial compounds or optimizing their environmental survival and competitiveness.
d. Eco-Friendly Formulations: Researchers will likely work on developing eco-friendly formulations of Streptomyces-based biopesticides. This could involve creating stable formulations, improving shelf life, and ensuring ease of application.
e. Integrated Pest Management (IPM): Streptomyces-based biocontrol agents may become an integral part of IPM strategies. Combining biological control with other pest management approaches like crop rotation, organic farming practices, and resistant crop varieties can provide a more comprehensive solution.
f. Regulatory Framework: As Streptomyces-based products gain prominence, regulatory agencies may establish clearer guidelines and standards for their registration and use. Ensuring safety and efficacy will be crucial.
g. Field Trials and Commercialization: Large-scale field trials and commercialization efforts will be essential to bring Streptomyces-based biopesticides to the market. This may involve partnerships between research institutions, agribusinesses, and governmental agencies.
h. Environmental Impact Assessment: Continual assessment of the environmental impact of using Streptomyces-based biocontrol agents will be necessary. This includes evaluating their effects on non-target organisms and assessing their long-term sustainability.
i. Education and Outreach: Raising awareness among farmers and agricultural stakeholders about the benefits and proper use of Streptomyces-based biopesticides will be crucial for their adoption.
j. Global Collaboration: Given the global nature of agriculture and the spread of plant pathogens, international collaboration in research, development, and sharing of best practices will be important.
Streptomyces species hold great potential as biological control agents for plant pathogens, and ongoing research is likely to enhance their effectiveness, safety, and practicality for sustainable agriculture in the future.
SK: Investigation, Methodology, Validation, Writing – original draft. SS: Investigation, Methodology, Data curation, Project administration, Writing – review and editing. AK: Conceptualization, Resources, Supervision, Visualization, Writing – review and editing. TM: Writing – review and editing, Data curation, Formal analysis.
The author(s) declare that no financial support was received for the research, authorship, and/or publication of this article.
We obliged to Lovely Professional University, Phagwara, to conduct my research.
The authors declare that the research was conducted in the absence of any commercial or financial relationships that could be construed as a potential conflict of interest.
All claims expressed in this article are solely those of the authors and do not necessarily represent those of their affiliated organizations, or those of the publisher, the editors and the reviewers. Any product that may be evaluated in this article, or claim that may be made by its manufacturer, is not guaranteed or endorsed by the publisher.
Abade, A., Ferreira, P. A., and de Barros Vidal, F. (2021). Plant diseases recognition on images using convolutional neural networks: A systematic review. Comput. Electron. Agric. 185:106125.
Abraham, J., and Gajendiran, A. (2019). Biodegradation of fipronil and its metabolite fipronil sulfone by Streptomyces rochei strain AJAG7 and its use in bioremediation of contaminated soil. Pesticide Biochem. Physiol. 155, 90–100. doi: 10.1016/j.pestbp.2019.01.011
Aioub, A. A. A., Elesawy, A. E., and Ammar, E. E. (2022). Plant Growth Promoting Rhizobacteria (PGPR) and their role in plant-parasitic nematodes control: A fresh look at an old issue. J. Plant Dis. Protect. 129, 1305–1321. doi: 10.1007/s41348-022-00642-3
Ali, A. A. I., El-Ashry, R. M., and Aioub, A. A. A. (2022). Animal manure rhizobacteria co-fertilization suppresses phytonematodes and enhances plant production: Evidence from field and greenhouse. J. Plant Dis. Protect. 129, 155–169. doi: 10.1007/s41348-021-00529-9
Asibi, A. E., Chai, Q., and Coulter, J. A. (2019). Rice blast: A disease with implications for global food security. Agronomy 9:451.
Bentley, S. D., Chater, K. F., Cerdeno-Tarraga, A. M., Challis, G. L., Thomson, N. R., James, K. D., et al. (2002). Complete genome sequence of the model actinomycete Streptomyces coelicolor A3(2). Nature 417, 141–147. doi: 10.1038/417141a
Betancur, L. A., Forero, A. M., Romero-Otero, A., Sepúlveda, L. Y., Moreno-Sarmiento, N. C., Castellanos, L., et al. (2019). Cyclic tetrapeptides from the marine strain Streptomyces sp. PNM-161a with activity against rice and yam phytopathogens. J. Antibiot. 72, 744–751. doi: 10.1038/s41429-019-0201-0
Bhatti, A. A., Haq, S., and Bhat, R. A. (2017). Actinomycetes benefaction role in soil and plant health. Microb. Pathog. 111, 458–467. doi: 10.1016/j.micpath.2017.09.036
Bonaterra, A., Badosa, E., Daranas, N., Francés, J., Roselló, G., and Montesinos, E. (2022). Bacteria as biological control agents of plant diseases. Microorganisms 10:1759. doi: 10.3390/microorganisms10091759
Bubici, G. (2018). Streptomyces spp. as biocontrol agents against Fusarium species. CABI Rev. 2018, 1–15. doi: 10.1079/PAVSNNR201813050
Butt, U. D., Khan, S., Liu, X., Sharma, A., Zhang, X., Wu, B., et al. (2023). Present status, limitations, and prospects of using Streptomyces bacteria as a potential probiotic agent in aquaculture. Probiot. Antimicrob. Prot. doi: 10.1007/s12602-023-10053-x [Epub ahead of print].
Buttimer, C., McAuliffe, O., Ross, R. P., Hill, C., O’Mahony, J., and Coffey, A. (2017). Bacteriophages and bacterial plant diseases. Front. Microbiol. 8:34. doi: 10.3389/fmicb.2017.00034
Campos, H., and Ortiz, O. (eds) (2020). The Potato Crop. Cham: Springer International Publishing, doi: 10.1007/978-3-030-28683-5
Ceresini, P. C., Castroagudín, V. L., Rodrigues, F. Á, Rios, J. A., Eduardo Aucique-Pérez, C., Moreira, S. I., et al. (2018). Wheat blast: Past, present, and future. Annu. Rev. Phytopathol. 56, 427–456.
Chen, J., Zhou, L., Din, I. U., Arafat, Y., Li, Q., Wang, J., et al. (2021). Antagonistic activity of trichoderma spp. against fusarium oxysporum in rhizosphere of radix pseudostellariae triggers the expression of host defense genes and improves its growth under long-term monoculture system. Front. Microbiol. 12:579920. doi: 10.3389/FMICB.2021.579920/BIBTEX
Chukwu, S. C., Rafii, M. Y., Ramlee, S. I, Ismail, S. I, Hasan, M. M., Oladosu, Y. A., et al. (2019). Bacterial leaf blight resistance in rice: A review of conventional breeding to molecular approach. Mol. Biol. Rep. 46, 1519–1532.
Daranas, N., Roselló, G., Cabrefiga, J., Donati, I., Francés, J., Badosa, E., et al. (2019). Biological control of bacterial plant diseases with Lactobacillus plantarum strains selected for their broad-spectrum activity. Ann. Appl. Biol. 174, 92–105. doi: 10.1111/aab.12476
de Lima Procópio, R. E., da Silva, I. R., Martins, M. K., de Azevedo, J. L., and de Araújo, J. M. (2012). Antibiotics produced by Streptomyces. Braz. J. Infect. Dis. 16, 466–471. doi: 10.1016/j.bjid.2012.08.014
Degani, O. (2021). A review: Late wilt of maize—the pathogen, the disease, current status, and future perspective. J. Fungi 7:989.
Degani, O., and Dor, S. (2021). Trichoderma biological control to protect sensitive maize hybrids against late wilt disease in the field. J. Fungi 7:315.
Degani, O., Regev, D., and Dor, S. (2021). The microflora of maize grains as a biological barrier against the late wilt causal agent, Magnaporthiopsis maydis. Agronomy 11:965.
Díaz-Cruz, G. A., Liu, J., Tahlan, K., and Bignell, D. R. D. (2022). Nigericin and geldanamycin are phytotoxic specialized metabolites produced by the plant pathogen Streptomyces sp. 11-1-2. Microbiol. Spectr. 10:e0231421. doi: 10.1128/spectrum.02314-21
Doehlemann, G., Ökmen, B., Zhu, W., and Sharon, A. (2017). Plant pathogenic fungi. Microbiol. Spectr. 5. doi: 10.1128/microbiolspec.FUNK-0023-2016
Doolotkeldieva, T., Bobusheva, S., and Suleymankisi, A. (2016). Biological control of erwinia carotovora ssp. carotovora by streptomyces species. Adv. Microbiol. 06, 104–114. doi: 10.4236/aim.2016.62011
Ebrahimi-Zarandi, M., Bonjar, G., Riseh, R., El-Shetehy, M., Saadoun, I., and Barka, E. (2021). Exploring two Streptomyces species to control Rhizoctonia solani in tomato. Agronomy 11:1384. doi: 10.3390/agronomy11071384
El-Ashry, R. M., Ali, M. A. S., Elsobki, A. E. A., and Aioub, A. (2021). Integrated management of Meloidogyne incognita on tomato using combinations of abamectin, Purpureocillium lilacinum, rhizobacteria, and botanicals compared with nematicide. Egypt J. Biol. Pest Control 31:93. doi: 10.1186/s41938-021-00438-x
Elmallah, M. I. Y., Cogo, S., Constantinescu, A. A., Elifio-Esposito, S., Abdelfattah, M. S., and Micheau, O. (2020). Marine actinomycetes-derived secondary metabolites overcome TRAIL-resistance via the intrinsic pathway through downregulation of survivin and XIAP. Cells 9:1760. doi: 10.3390/cells9081760
El-Sayed, A. K. A., Abou-Dobara, M. I., Abdel-Malak, C. A., and El-Badaly, A. A. E. (2019). Taqman hydrolysis probe application for Escherichia coli, Salmonella enterica, and Vibrio cholerae detection in surface and drinking water. J. Water Sanitat. Hygiene Dev. 9, 492–499. doi: 10.2166/washdev.2019.137
Evangelista-Martínez, Z. (2014). Isolation and characterization of soil Streptomyces species as potential biological control agents against fungal plant pathogens. World J. Microbiol. Biotechnol. 30, 1639–1647. doi: 10.1007/s11274-013-1568-x
Figueroa, M., Hammond-Kosack, K. E., and Solomon, P. S. (2018). A review of wheat diseases—a field perspective. Mol. Plant Pathol. 19, 1523–1536.
Gaire, S. P., Zhou, X. G., Zhou, Y., Shi, J., and Jo, Y. K. (2023). Identification and distribution of fungal pathogens associated with seedling blight of rice in the southern United States. Plant Pathology. 72, 76–88.
Giraud, T., Gladieux, P., and Gavrilets, S. (2010). Linking the emergence of fungal plant diseases with ecological speciation. Trends Ecol. Evol. 25, 387–395. doi: 10.1016/j.tree.2010.03.006
Gong, Y., Liu, J.-Q., Xu, M.-J., Zhang, C.-M., Gao, J., Li, C.-G., et al. (2022). Antifungal volatile organic compounds from Streptomyces setonii WY228 control black spot disease of sweet potato. Appl. Environ. Microbiol. 88:e0231721. doi: 10.1128/aem.02317-21
Gorai, P. S., Ghosh, R., Konra, S., and Mandal, N. C. (2021). Biological control of early blight disease of potato caused by Alternaria alternata EBP3 by an endophytic bacterial strain Bacillus velezensis SEB1. Biol. Control 156:104551. doi: 10.1016/j.biocontrol.2021.104551
Hariharan, G., and Prasannath, K. (2021). Recent advances in molecular diagnostics of fungal plant pathogens: A mini review. Front. Cell. Infect. Microbiol. 10:600234. doi: 10.3389/fcimb.2020.600234
Harir, M., Bendif, H., Bellahcene, M., Fortas, Z., and Rebecca Pogni, Z. (2018). “Streptomyces secondary metabolites,” in Basic biology and applications of actinobacteria, ed. S. Enany (London: IntechOpen), doi: 10.5772/intechopen.79890
Himmelstein, J. C., Maul, J. E., and Everts, K. L. (2014). Impact of five cover crop green manures and actinovate on fusarium wilt of watermelon. Plant Dis. 98, 965–972. doi: 10.1094/PDIS-06-13-0585-RE
Hopwood, D. A. (2019). Highlights of Streptomyces genetics. Heredity 123, 23–32. doi: 10.1038/s41437-019-0196-0
Huang, J.-W., Shih, H.-D., Huang, H.-C., and Chung, W.-C. (2007). Effects of nutrients on production of fungichromin by Streptomyces padanus PMS-702 and efficacy of control of Phytophthora infestans. Can. J. Plant Pathol. 29, 261–267. doi: 10.1080/07060660709507468
Hwang, B. K., Ahn, S. J., and Moon, S. S. (1994). Production, purification, and antifungal activity of the antibiotic nucleoside, tubercidin, produced by Streptomyces violaceoniger. Can. J. Bot. 72, 480–485. doi: 10.1139/b94-064
Hwang, B. K., Lee, J. Y., Kim, B. S., and Moon, S. S. (1996). Isolation, structure elucidation, and antifungal activity of a manumycin-type antibiotic from Streptomyces flaveus. J. Agric. Food Chem. 44, 3653–3657. doi: 10.1021/jf960084o
Hwang, B. K., Lim, S. W., Kim, B. S., Lee, J. Y., and Moon, S. S. (2001). Isolation and In Vivo and In Vitro antifungal activity of phenylacetic acid and sodium phenylacetate from Streptomyces humidus. Appl. Environ. Microbiol. 67, 3739–3745. doi: 10.1128/AEM.67.8.3739-3745.2001
Iqbal, Z., Khan, M. A., Sharif, M., Shah, J. H., ur Rehman, M. H., and Javed, K. (2018). An automated detection and classification of citrus plant diseases using image processing techniques: A review. Comput. Electron. Agric. 153, 12–32. doi: 10.1016/j.compag.2018.07.032
Jakubiec-krzesniak, K., Rajnisz-mateusiak, A., Guspiel, A., Ziemska, J., and Solecka, J. (2018). Secondary metabolites of actinomycetes and their antibacterial, antifungal and antiviral properties. Polish J. Microbiol. 67, 259–272. doi: 10.21307/pjm-2018-048
Jin, N., Lu, X., Wang, X., Liu, Q., Peng, D., and Jian, H. (2019). The effect of combined application of Streptomyces rubrogriseus HDZ-9-47 with soil biofumigation on soil microbial and nematode communities. Sci. Rep. 9:16886. doi: 10.1038/s41598-019-52941-9
Juroszek, P., and von Tiedemann, A. (2015). Linking plant disease models to climate change scenarios to project future risks of crop diseases: A review. J. Plant Dis. Prot. 122, 3–15.
Kaminsky, L. M., Trexler, R. V., Malik, R. J., Hockett, K. L., and Bell, T. H. (2019). The inherent conflicts in developing soil microbial inoculants. Trends Biotechnol. 37, 140–151. doi: 10.1016/J.TIBTECH.2018.11.011
Kang, M., Kim, M., Liu, M., Jin, C. Z., Park, S. H., Lee, J. M., et al. (2021). Nematicidal activity of teleocidin B4 isolated from Streptomyces sp. against pine wood nematode, Bursaphelenchus xylophilus. Pest Manag. Sci. 77, 1607–1615. doi: 10.1002/ps.6095
Kanini, G. S., Katsifas, E. A., Savvides, A. L., and Karagouni, A. D. (2013). Streptomyces rochei ACTA1551, an Indigenous Greek isolate studied as a potential biocontrol agent against Fusarium oxysporum f.sp. lycopersici. Biomed Res. Int. 2013:387230. doi: 10.1155/2013/387230
Kannan, V., Bastas, K., and Devi, R. (2015). “Scientific and economic impact of plant pathogenic bacteria,” in Sustainable Approaches to Controlling Plant Pathogenic Bacteria, eds R. V. Kannan and K. K. Bastas (Boca Raton, FL: CRC Press), 369–392. doi: 10.1201/b18892-21
Karuppiah, P., and Mustaffa, M. (2013). Antibacterial and antioxidant activities of Musa sp. leaf extracts against multidrug resistant clinical pathogens causing nosocomial infection. Asian Pacific J. Trop. Biomed. 3, 737–742. doi: 10.1016/S2221-1691(13)60148-3
Kasuga, K., Sasaki, A., Matsuo, T., Yamamoto, C., Minato, Y., Kuwahara, N., et al. (2017). Heterologous production of kasugamycin, an aminoglycoside antibiotic from Streptomyces kasugaensis, in Streptomyces lividans and Rhodococcus erythropolis L-88 by constitutive expression of the biosynthetic gene cluster. Appl. Microbiol. Biotechnol. 101, 4259–4268. doi: 10.1007/s00253-017-8189-5
Keswani, C., Singh, H. B., Hermosa, R., García-Estrada, C., Caradus, J., He, Y.-W., et al. (2019). Antimicrobial secondary metabolites from agriculturally important fungi as next biocontrol agents. Appl. Microbiol. Biotechnol. 103, 9287–9303. doi: 10.1007/s00253-019-10209-2
Khokhar, M. K., Hooda, K. S., Sharma, S. S., and Singh, V. (2014). Post flowering stalk rot complex of maize-present status and future prospects. Maydica 59, 226–242.
Kim, J. D., Han, J. W., Lee, S. C., Lee, D., Hwang, I. C., and Kim, B. S. (2011). Disease control effect of strevertenes produced by Streptomyces psammoticus against tomato fusarium wilt. J. Agric. Food Chem. 59, 1893–1899. doi: 10.1021/jf1038585
Kim, Y. H., Park, B. S., Bhatia, S. K., Seo, H.-M., Jeon, J.-M., Kim, H.-J., et al. (2014). Production of rapamycin in Streptomyces hygroscopicus from glycerol-based media optimized by systemic methodology. J. Microbiol. Biotechnol. 24, 1319–1326. doi: 10.4014/jmb.1403.03024
Kishimoto, K., Park, Y. S., Okabe, M., and AKiYAMA, S.-I. (1996). Effect of ferrous ion on mildiomycin production by Streptoverticillium rimofaciens. J. Antibiot. 49, 770–774. doi: 10.7164/antibiotics.49.770
Kobinata, K., Koshino, H., Kudo, T., Isono, K., and Osada, H. (1993). RK-397, a new oxo pentaene antihiotic. J. Antibiot. 46, 1616–1618. doi: 10.7164/antibiotics.46.1616
Kulkarni, M., Gorthi, S., Banerjee, G., and Chattopadhyay, P. (2017). Production, characterization and optimization of actinomycin D from Streptomyces hydrogenans IB310, a(n antagonistic bacterium against phytopathogens. Biocatal. Agric. Biotechnol. 10, 69–74. doi: 10.1016/j.bcab.2017.02.009
Kumar, K. V. K., Reddy, M. S., Kloepper, J. W., Lawrence, K. S., Groth, D. E., and Miller, M. E. (2016). Sheath blight disease of rice (Oryza sativa L.)–an overview. Biosci. Biotechnol. Res. Asia 6, 465–480.
Kumar, P. S., Raj, J. P. P., Duraipandiyan, V., and Ignacimuthu, S. (2012). Antibacterial activity of some actinomycetes from Tamil Nadu, India. Asian Pacific J. Trop. Biomed. 2, 936–943. doi: 10.1016/S2221-1691(13)60003-9
Kumbar, B., Mahmood, R., Nagesha, S. N., Nagaraja, M. S., Prashant, D. G., Kerima, O. Z., et al. (2019). Field application of Bacillus subtilis isolates for controlling late blight disease of potato caused by Phytophthora infestans. Biocatalysis Agric. Biotechnol. 22:101366. doi: 10.1016/j.bcab.2019.101366
Law, J. W.-F., Ser, H.-L., Khan, T. M., Chuah, L.-H., Pusparajah, P., Chan, K.-G., et al. (2017). The potential of Streptomyces as biocontrol agents against the rice blast fungus, Magnaporthe oryzae (Pyricularia oryzae). Front. Microbiol. 8:3. doi: 10.3389/fmicb.2017.00003
Le, K. D., Kim, J., Nguyen, H. T., Yu, N. H., Park, A. R., Lee, C. W., et al. (2021). Streptomyces sp. JCK-6131 protects plants against bacterial and fungal diseases via two mechanisms. Front. Plant Sci. 12:726266. doi: 10.3389/fpls.2021.726266
Le, K. D., Yu, N. H., Park, A. R., Park, D.-J., Kim, C.-J., and Kim, J.-C. (2022). Streptomyces sp. AN090126 as a biocontrol agent against bacterial and fungal plant diseases. Microorganisms 10:791. doi: 10.3390/microorganisms10040791
LeBlanc, N. (2022). Bacteria in the genus Streptomyces are effective biological control agents for management of fungal plant pathogens: A meta-analysis. Biocontrol 67, 111–121. doi: 10.1007/s10526-021-10123-5
Lee, J. Y., Lee, J. Y., Jung, H. W., and Hwang, B. K. (2005). Streptomyces koyangensis sp. nov., a novel actinomycete that produces 4-phenyl-3-butenoic acid. Int. J. Syst. Evol. Microbiol. 55, 257–262. doi: 10.1099/ijs.0.63168-0
Leščić, I., Vukelić, B., Majerić-Elenkov, M., Saenger, W., and Abramić, M. (2001). Substrate specificity and effects of water-miscible solvents on the activity and stability of extracellular lipase from Streptomyces rimosus. Enzyme Microb. Technol. 29, 548–553. doi: 10.1016/S0141-0229(01)00433-1
Li, W., Csukai, M., Corran, A., Crowley, P., Solomon, P. S., and Oliver, R. P. (2008). Malayamycin, a new streptomycete antifungal compound, specifically inhibits sporulation of Stagonospora nodorum (Berk) Castell and Germano, the cause of wheat glume blotch disease. Pest Manag. Sci. 64, 1294–1302. doi: 10.1002/ps.1632
Liu, J.-M., Wang, S.-S., Zheng, X., Jin, N., Lu, J., Huang, Y.-T., et al. (2020). Antimicrobial activity against phytopathogens and inhibitory activity on solanine in potatoes of the endophytic bacteria isolated from potato tubers. Front. Microbiol. 11:570926. doi: 10.3389/fmicb.2020.570926
Liu, W., Liu, J., Triplett, L., Leach, J. E., and Wang, G. L. (2014). Novel insights into rice innate immunity against bacterial and fungal pathogens. Annu. Rev. Phytopathol. 52, 213–241.
Lv, M., Zhou, G., He, M., Chen, A., Zhang, W., and Hu, Y. (2020). Maize leaf disease identification based on feature enhancement and DMS-robust alexnet. IEEE Access 8, 57952–57966.
Maglangit, F., Tong, M. H., Jaspars, M., Kyeremeh, K., and Deng, H. (2019). Legonoxamines A-B, two new hydroxamate siderophores from the soil bacterium, Streptomyces sp. MA37. Tetrahedron Lett. 60, 75–79. doi: 10.1016/j.tetlet.2018.11.063
Malmierca, M. G., Izquierdo-Bueno, I., Mccormick, S. P., Cardoza, R. E., Alexander, N. J., Moraga, J., et al. (2016). Botrydial and botcinins produced by B otrytis cinerea regulate the expression of T richoderma arundinaceum genes involved in trichothecene biosynthesis. Mol. Plant Pathol. 17, 1017–1031. doi: 10.1111/mpp.12343
Martinelli, F., Scalenghe, R., Davino, S., Panno, S., Scuderi, G., Ruisi, P., et al. (2015). Advanced methods of plant disease detection. A review. Agron. Sustain. Dev. 35, 1–25.
Matsuyama, N. (1991). Purification and characterization of antifungal substance Ac-1 produced by a Streptomyces sp. AB-88M. Japan. J. Phytopathol. 57, 591–594. doi: 10.3186/jjphytopath.57.591
McIntosh, R., Mu, J., Han, D., and Kang, Z. (2018). Wheat stripe rust resistance gene Yr24/Yr26: A retrospective review. Crop J. 6, 321–329.
Mehta, S., Singh, B., Dhakate, P., Rahman, M., and Islam, M. A. (2019). “Rice, marker-assisted breeding, and disease resistance,” in Disease Resistance in Crop Plants: Molecular, Genetic and Genomic Perspectives, ed. S. H. Wani (Cham: Springer International Publishing), 83–111.
Minuto, A., Spadaro, D., Garibaldi, A., and Gullino, M. L. (2006). Control of soilborne pathogens of tomato using a commercial formulation of Streptomyces griseoviridis and solarization. Crop Protect. 25, 468–475. doi: 10.1016/j.cropro.2005.08.001
Möller, M., and Stukenbrock, E. H. (2017). Evolution and genome architecture in fungal plant pathogens. Nat. Rev. Microbiol. 15, 756–771. doi: 10.1038/nrmicro.2017.76
Moolhuijzen, P., See, P. T., Shi, G., Powell, H. R., Cockram, J., Jørgensen, L. N., et al. (2022). A global pangenome for the wheat fungal pathogen Pyrenophora tritici-repentis and prediction of effector protein structural homology. bioRxiv [Preprint]. doi: 10.1101/2022.03.07.482416
Morales-Cedeño, L. R., Orozco-Mosqueda, M. C., Loeza-Lara, P. D., Parra-Cota, F. I., de los Santos-Villalobos, S., and Santoyo, G. (2021). Plant growth-promoting bacterial endophytes as biocontrol agents of pre- and post-harvest diseases: Fundamentals, methods of application and future perspectives. Microbiol. Res. 242:126612. doi: 10.1016/j.micres.2020.126612
Muangham, S., Pathom-aree, W., and Duangmal, K. (2015). Melanogenic actinomycetes from rhizosphere soil — antagonistic activity against Xanthomonas oryzae and plant-growth-promoting traits. Can. J. Microbiol. 61, 164–170. doi: 10.1139/cjm-2014-0645
Nakajima, M., Itoi, K., Takamatsu, Y., Kinoshita, T., Okazaki, T., Kawakubo, K., et al. (1991). Hydantocidin: A new compound with herbicidal activity from Streptomyces hygroscopicus. J. Antibiot. 44, 293–300. doi: 10.7164/antibiotics.44.293
Narayanasamy, P. (2011). “Diagnosis of fungal diseases of plants,” in Microbial plant pathogens-detection and disease diagnosis, ed. P. Narayanasamy (Springer: Dordrecht), 273–284. doi: 10.1007/978-90-481-9735-4_5
Nazarov, P. A., Baleev, D. N., Ivanova, M. I, Sokolova, L. M., and Karakozova, M. V. (2020). Infectious plant diseases: Etiology, current status, problems and prospects in plant protection. Acta Naturae 12, 46–59. doi: 10.32607/actanaturae.11026
Ngalimat, M. S., Mohd Hata, E., Zulperi, D., Ismail, S. I., Ismail, M. R., Mohd Zainudin, N. A. I., et al. (2021). Plant growth-promoting bacteria as an emerging tool to manage bacterial rice pathogens. Microorganisms 9:682.
Nguyen, X. H., Naing, K. W., Lee, Y. S., Kim, Y. H., Moon, J. H., and Kim, K. Y. (2015). Antagonism of antifungal metabolites from Streptomyces griseus H7602 against Phytophthora capsici. J. Basic Microbiol. 55, 45–53. doi: 10.1002/jobm.201300820
Olanrewaju, O. S., and Babalola, O. O. (2019). Streptomyces: Implications and interactions in plant growth promotion. Appl. Microbiol. Biotechnol. 103, 1179–1188. doi: 10.1007/s00253-018-09577-y
Orozco-Mosqueda, M. C., Glick, B. R., and Santoyo, G. (2020). ACC deaminase in plant growth-promoting bacteria (PGPB): An efficient mechanism to counter salt stress in crops. Microbiol. Res. 235:126439. doi: 10.1016/j.micres.2020.126439
Pacios-Michelena, S., Aguilar González, C. N., Alvarez-Perez, O. B., Rodriguez-Herrera, R., Chávez-González, M., Arredondo Valdés, R., et al. (2021). Application of streptomyces antimicrobial compounds for the control of phytopathogens. Front. Sustain. Food Syst. 5:696518. doi: 10.3389/fsufs.2021.696518
Palaniyandi, S. A., Yang, S. H., Zhang, L., and Suh, J.-W. (2013). Effects of actinobacteria on plant disease suppression and growth promotion. Appl. Microbiol. Biotechnol. 97, 9621–9636. doi: 10.1007/s00253-013-5206-1
Panchalingam, H., Ashfield-Crook, N., Naik, V., Frenken, R., Foster, K., Tomlin, R., et al. (2022). Testing the biocontrol ability of a trichoderma-streptomycetes consortium against pyrrhoderma noxium (Corner) L.W. Zhou and Y.C. Dai in Soil. J. Fungi 9:67. doi: 10.3390/jof9010067
Parajuli, M., Khadka, G. B., and Chaurasia, J. (2022). A review on comparative effect of chemicals and botanicals in management of brown spot diseases of rice (Oryza sativa L.). Arch. Agric. Environ. Sci. 7, 127–131.
Paul, S. K., Chakraborty, M., Rahman, M., Gupta, D. R., Mahmud, N. U., Rahat, A. A. M., et al. (2022). Marine natural product antimycin A suppresses wheat blast disease caused by Magnaporthe oryzae Triticum. J. Fungi 8:618.
Petersen, M., Ma, L., and Lu, X. (2021). Rapid determination of viable but non-culturable Campylobacter jejuni in food products by loop-mediated isothermal amplification coupling propidium monoazide treatment. Int. J. Food Microbiol. 351:109263. doi: 10.1016/j.ijfoodmicro.2021.109263
Prasher, P., and Sharma, M. (2022). “An introduction to rice diseases,” in Cereal Diseases: Nanobiotechnological Approaches for Diagnosis and Management, eds K. A. Abd-Elsalam and H. I. Mohamed (Singapore: Springer Nature Singapore), 3–15.
Qiu, Z., Egidi, E., Liu, H., Kaur, S., and Singh, B. K. (2019). New frontiers in agriculture productivity: Optimised microbial inoculants and in situ microbiome engineering. Biotechnol. Adv. 37:107371. doi: 10.1016/j.biotechadv.2019.03.010
Rashad, Y. M., Al-Askar, A. A., Ghoneem, K. M., Saber, W. I. A., and Hafez, E. E. (2017). Chitinolytic Streptomyces griseorubens E44G enhances the biocontrol efficacy against Fusarium wilt disease of tomato. Phytoparasitica 45, 227–237. doi: 10.1007/s12600-017-0580-3
Ristaino, J. B., Anderson, P. K., Bebber, D. P., Brauman, K. A., Cunniffe, N. J., Fedoroff, N. V., et al. (2021). The persistent threat of emerging plant disease pandemics to global food security. Proc. Natl. Acad. Sci. U.S.A. 118:e2022239118. doi: 10.1073/pnas.2022239118
Rubab, M., Shahbaz, H. M., Olaimat, A. N., and Oh, D.-H. (2018). Biosensors for rapid and sensitive detection of Staphylococcus aureus in food. Biosens. Bioelectron. 105, 49–57. doi: 10.1016/j.bios.2018.01.023
Ruiu, L. (2020). Plant-Growth-Promoting Bacteria (PGPB) against insects and other agricultural pests. Agronomy 10:861. doi: 10.3390/agronomy10060861
Sadeghi, A., Koobaz, P., Azimi, H., Karimi, E., and Akbari, A. R. (2017). Plant growth promotion and suppression of Phytophthora drechsleri damping-off in cucumber by cellulase-producing Streptomyces. Biocontrol 62, 805–819. doi: 10.1007/s10526-017-9838-4
Samac, D. A., and Kinkel, L. L. (2001). Suppression of the root-lesion nematode (Pratylenchus penetrans) in alfalfa (Medicago sativa) by Streptomyces spp. Plant Soil 235, 35–44. doi: 10.1023/A:1011820002779
Sankaran, S., Mishra, A., Ehsani, R., and Davis, C. (2010). A review of advanced techniques for detecting plant diseases. Comput. Electron. Agric. 72, 1–13.
Satomi, T., Kusakabe, H., Nakamura, G., Nishio, T., Uramoto, M., and Isono, K. (1982). Neopeptins A and B, new antifungal antibiotics. Agric. Biol. Chem. 46, 2621–2623. doi: 10.1080/00021369.1982.10865486
Schumacher, D. P., and Hall, S. S. (1982). An efficient stereospecific total synthesis of (.+-.)-anisomycin and related new synthetic antibiotics. J. Am. Chem. Soc. 104, 6076–6080. doi: 10.1021/ja00386a039
Selim, M. S. M., Abdelhamid, S. A., and Mohamed, S. S. (2021). Secondary metabolites and biodiversity of actinomycetes. J. Genet. Eng. Biotechnol. 19:72. doi: 10.1186/s43141-021-00156-9
Shafi, J., Tian, H., and Ji, M. (2017). Bacillus species as versatile weapons for plant pathogens: A review. Biotechnol. Biotechnol. Equip. 31, 446–459. doi: 10.1080/13102818.2017.1286950
Sharma, N., Bhatia, S., Chunduri, V., Kaur, S., Sharma, S., Kapoor, P., et al. (2020). Pathogenesis of celiac disease and other gluten related disorders in wheat and strategies for mitigating them. Front. Nutr. 7:6. doi: 10.3389/fnut.2020.00006
Sheng, Y., Lam, P. W., Shahab, S., Santosa, D. A., Proteau, P. J., Zabriskie, T. M., et al. (2015). Identification of elaiophylin skeletal variants from the Indonesian Streptomyces sp. ICBB 9297. J. Nat. Prod. 78, 2768–2775. doi: 10.1021/acs.jnatprod.5b00752
Shojaee, N., Shahidi, B. G. H., Shahdaei, S., and Leyla, S. B. (2014). Biological control of citrus green mould, Penicillium digitatum, by antifungal activities of Streptomyces isolates from agricultural soils. Afric. J. Microbiol. Res. 8, 1501–1509. doi: 10.5897/AJMR2013.6289
Singh, P., Mazumdar, P., Harikrishna, J. A., and Babu, S. (2019). Sheath blight of rice: A review and identification of priorities for future research. Planta 250, 1387–1407.
Singh, R. P., Singh, P. K., Rutkoski, J., Hodson, D. P., He, X., Jørgensen, L. N., et al. (2016). Disease impact on wheat yield potential and prospects of genetic control. Annu. Rev. Phytopathol. 54, 303–322.
Sivalingam, P., Hong, K., Pote, J., and Prabakar, K. (2019). Extreme environment Streptomyces: Potential sources for new antibacterial and anti-cancer drug leads? Int. J. Microbiol. 2019, 1–20. doi: 10.1155/2019/5283948
Solanki, M. K., Malviya, M. K., and Wang, Z. (2016). “Actinomycetes bio-inoculants: A modern prospectus for plant disease management,” in Plant Growth Promoting Actinobacteria, eds G. Subramaniam, S. Arumugam, and V. Rajendran (Singapore: Springer), 63–81. doi: 10.1007/978-981-10-0707-1_5
Suárez-Moreno, Z. R., Vinchira-Villarraga, D. M., Vergara-Morales, D. I., Castellanos, L., Ramos, F. A., Guarnaccia, C., et al. (2019). Plant-growth promotion and biocontrol properties of three Streptomyces spp. isolates to control bacterial rice pathogens. Front. Microbiol. 10:290. doi: 10.3389/fmicb.2019.00290
Sun, Y., Xie, J., Tang, L., Odiba, A. S., Chen, Y., Fang, W., et al. (2023). Isolation, identification and molecular mechanism analysis of the nematicidal compound spectinabilin from newly isolated Streptomyces sp. DT10. Molecules 28:4365. doi: 10.3390/molecules28114365
Sunder, S., Singh, R. A. M., and Agarwal, R. (2014). Brown spot of rice: An overview. Indian Phytopathol. 67, 201–215.
Tahir, M., Ahmad, I., Shahid, M., Shah, G. M., Farooq, A. B. U., Akram, M., et al. (2019). Regulation of antioxidant production, ion uptake and productivity in potato (Solanum tuberosum L.) plant inoculated with growth promoting salt tolerant Bacillus strains. Ecotoxicol. Environ. Saf. 178, 33–42. doi: 10.1016/j.ecoenv.2019.04.027
Thambugala, K., Daranagama, D., Phillips, A., Kannangara, S., and Promputtha, I. (2020). Fungi vs. fungi in biocontrol: An overview of fungal antagonists applied against fungal plant pathogens. Front. Cell Infect. Microbiol. 10:604923. doi: 10.3389/fcimb.2020.604923
Tiwari, R. K., Kumar, R., Sharma, S., Naga, K. C., Subhash, S., and Sagar, V. (2022). Continuous and emerging challenges of silver scurf disease in potato. Int. J. Pest Manag. 68, 89–101. doi: 10.1080/09670874.2020.1795302
Tiwari, R. K., Kumar, R., Sharma, S., Sagar, V., Aggarwal, R., Naga, K. C., et al. (2020). Potato dry rot disease: Current status, pathogenomics and management. 3 Biotech 10:503. doi: 10.1007/s13205-020-02496-8
Tomilova, O. G., Shaldyaeva, E. M., Kryukova, N. A., Pilipova, Y. V., Schmidt, N. S., Danilov, V. P., et al. (2020). Entomopathogenic fungi decrease Rhizoctonia disease in potato in field conditions. PeerJ 8:e9895. doi: 10.7717/peerj.9895
Trejo-Estrada, S. R., Sepulveda, I. R., and Crawford, D. L. (1998). In vitro and in vivo antagonism of Streptomyces violaceusniger YCED9 against fungal pathogens of turfgrass. World J. Microbiol. Biotechnol. 14, 865–872. doi: 10.1023/A:1008877224089
Valueva, T. A., and Mosolov, V. V. (2004). Role of inhibitors of proteolytic enzymes in plant defense against phytopathogenic microorganisms. Biochemistry 69, 1305–1309. doi: 10.1007/s10541-005-0015-5
Viaene, T., Langendries, S., Beirinckx, S., Maes, M., and Goormachtig, S. (2016). Streptomyces as a plant’s best friend? FEMS Microbiol. Ecol. 92, fiw119. doi: 10.1093/femsec/fiw119
Vurukonda, S. S. K. P., Giovanardi, D., and Stefani, E. (2018). Plant growth promoting and biocontrol activity of streptomyces spp. as endophytes. Int. J. Mol. Sci. 19:952. doi: 10.3390/ijms19040952
Waqar, A., Khattak, S. H., Begum, S., Rehman, T., Shehzad, A., Ajmal, W., et al. (2018). Stripe rust: A review of the disease, Yr genes and its molecular markers. Sarhad J. Agric. 34, 188–201.
Wattana-Amorn, P., Charoenwongsa, W., Williams, C., Crump, M. P., and Apichaisataienchote, B. (2016). Antibacterial activity of cyclo(L -Pro- L -Tyr) and cyclo(D -Pro- L -Tyr) from Streptomyces sp. strain 22-4 against phytopathogenic bacteria. Nat. Prod. Res. 30, 1980–1983. doi: 10.1080/14786419.2015.1095747
Wonglom, P., Suwannarach, N., Lumyong, S., Ito, S., Matsui, K., and Sunpapao, A. (2019). Streptomyces angustmyceticus NR8-2 as a potential microorganism for the biological control of leaf spots of Brassica rapa subsp. pekinensis caused by Colletotrichum sp. and Curvularia lunata. Biol. Control 138:104046. doi: 10.1016/j.biocontrol.2019.104046
Woo, S. L., Ruocco, M., Vinale, F., Nigro, M., Marra, R., Lombardi, N., et al. (2014). Trichoderma-based products and their widespread use in agriculture. Open Mycol. J. 8, 71–126. doi: 10.2174/1874437001408010071
Wu, Y., Yuan, J., E, Y., Raza, W., Shen, Q., and Huang, Q. (2015). Effects of volatile organic compounds from Streptomyces albulus NJZJSA2 on growth of two fungal pathogens. J. Basic Microbiol. 55, 1104–1117. doi: 10.1002/jobm.201400906
Xu, M., Wang, Q., Wang, G., Zhang, X., Liu, H., and Jiang, C. (2022). Combatting Fusarium head blight: Advances in molecular interactions between Fusarium graminearum and wheat. Phytopathol. Res. 4, 1–16.
Xu, Y., Zhao, B., Zhai, Y., Chen, Q., and Zhou, Y. (2021). Maize diseases identification method based on multi-scale convolutional global pooling neural network. IEEE Access 9, 27959–27970.
Yang, Q., Balint-Kurti, P., and Xu, M. (2017). Quantitative disease resistance: Dissection and adoption in maize. Mol. Plant 10, 402–413.
Yuan, W. M., and Crawford, D. L. (1995). Characterization of streptomyces lydicus WYEC108 as a potential biocontrol agent against fungal root and seed rots. Appl. Environ. Microbiol. 61, 3119–3128. doi: 10.1128/aem.61.8.3119-3128.1995
Zhang, X., Li, S., and Xiao, J. (2018). “Screening and fermentation of Jinggang-mycin high-yielding strains,” in Proceedings of the 2018 7th International Conference on Energy and Environmental Protection (ICEEP 2018), (Paris: Atlantis Press). doi: 10.2991/iceep-18.2018.90
Zheng, X., Wang, J., Chen, Z., Zhang, H., Wang, Z., Zhu, Y., et al. (2019). A Streptomyces sp. strain: Isolation, identification, and potential as a biocontrol agent against soilborne diseases of tomato plants. Biol. Control 136:104004. doi: 10.1016/j.biocontrol.2019.104004
Keywords: agricultural industry, antimicrobial compounds, biocontrol, crop protection, Streptomyces, phytopathogens, natural alternatives
Citation: Khan S, Srivastava S, Karnwal A and Malik T (2023) Streptomyces as a promising biological control agents for plant pathogens. Front. Microbiol. 14:1285543. doi: 10.3389/fmicb.2023.1285543
Received: 30 August 2023; Accepted: 16 October 2023;
Published: 14 November 2023.
Edited by:
Musharaf Ahmad, University of Agriculture, Peshawar, PakistanReviewed by:
Modupe Stella Ayilara, North-West University, South AfricaCopyright © 2023 Khan, Srivastava, Karnwal and Malik. This is an open-access article distributed under the terms of the Creative Commons Attribution License (CC BY). The use, distribution or reproduction in other forums is permitted, provided the original author(s) and the copyright owner(s) are credited and that the original publication in this journal is cited, in accordance with accepted academic practice. No use, distribution or reproduction is permitted which does not comply with these terms.
*Correspondence: Tabarak Malik, bWFsaWtpdHJjQGdtYWlsLmNvbQ==; Arun Karnwal, YXJ1bmthcm53YWxAZ21haWwuY29t
†These authors have contributed equally to this work
Disclaimer: All claims expressed in this article are solely those of the authors and do not necessarily represent those of their affiliated organizations, or those of the publisher, the editors and the reviewers. Any product that may be evaluated in this article or claim that may be made by its manufacturer is not guaranteed or endorsed by the publisher.
Research integrity at Frontiers
Learn more about the work of our research integrity team to safeguard the quality of each article we publish.