- 1Laboratorio de Microbiología Molecular, Departamento de Biología, Facultad de Química y Biología, Universidad de Santiago de Chile, Santiago, Chile
- 2Laboratory of Food Biotechnology, Department of Health Sciences and Technology, ETH, Zürich, Switzerland
- 3E. & J. Gallo Winery, Modesto, CA, United States
- 4Facultad de Ciencias, Universidad Arturo Prat, Iquique, Chile
Metal(loid) salts were used to treat infectious diseases in the past due to their exceptional biocidal properties at low concentrations. However, the mechanism of their toxicity has yet to be fully elucidated. The production of reactive oxygen species (ROS) has been linked to the toxicity of soft metal(loid)s such as Ag(I), Au(III), As(III), Cd(II), Hg(II), and Te(IV). Nevertheless, few reports have described the direct, or ROS-independent, effects of some of these soft-metal(loid)s on bacteria, including the dismantling of iron–sulfur clusters [4Fe-4S] and the accumulation of porphyrin IX. Here, we used genome-wide genetic, proteomic, and biochemical approaches under anaerobic conditions to evaluate the direct mechanisms of toxicity of these metal(loid)s in Escherichia coli. We found that certain soft-metal(loid)s promote protein aggregation in a ROS-independent manner. This aggregation occurs during translation in the presence of Ag(I), Au(III), Hg(II), or Te(IV) and post-translationally in cells exposed to Cd(II) or As(III). We determined that aggregated proteins were involved in several essential biological processes that could lead to cell death. For instance, several enzymes involved in amino acid biosynthesis were aggregated after soft-metal(loid) exposure, disrupting intracellular amino acid concentration. We also propose a possible mechanism to explain how soft-metal(loid)s act as proteotoxic agents.
1 Introduction
Some metallic elements (e.g., sodium, iron, copper, and cobalt) are essential for life because their unique chemical properties are indispensable for cellular functions. These metals participate in redox reactions, provide structural stability, and enable critical cellular processes such as electron transfer and catalysis (Lemire et al., 2013; Foster et al., 2022). However, in excess, these metals can become toxic (Nies, 1999). On the other hand, non-essential metal(loid) ions such as mercury, arsenic, tellurium, and silver are highly toxic to most organisms, even at micromolar concentrations (Daum et al., 1993; Tchounwou et al., 2012; Kim et al., 2019; Noreen et al., 2019; Zarei et al., 2019; Li et al., 2021). Metal-based molecules were used as treatment against syphilis, such as the As(III)-based drug Arsphenamine (Williams, 2009). Other metal-based drugs or metallodrugs hold great potential as a new generation of antimicrobials, cancer treatment, psychotropics, among others (Mjos and Orvig, 2014).
Ions of these elements, due to their high polarizability, are classified as soft acids or soft-metal(loid)s. They tend to react with intracellular molecules containing soft bases like sulfhydryl, thioesters, phenyl, or imidazole groups, forming covalently bound adducts (Pearson, 1966; Lemire et al., 2013). Conversely, metals such as Mg(II), Na(I), and K(I) are classified as hard metals and primarily interact through ionic bonds with hard bases like sulfate, carboxylate, phosphate, and amine groups (Pearson, 1966; Lemire et al., 2013).
Soft-metal(loid)s toxify cells by producing Reactive Oxygen Species (ROS) and inducing oxidative stress damage (Parvatiyar et al., 2005; Pérez et al., 2007; Tremaroli et al., 2007; Pacheco et al., 2008; Park et al., 2009; Lemire et al., 2013; Muñoz-Villagrán et al., 2020). Some metal(loid)s can produce ROS directly via Fenton chemistry [e.g., Fe(II) and Cu(I)], or indirectly, where Fenton-inactive metal(oid)s such as As(III), Cd(II), and Te(IV) deplete reduced glutathione, compromising the redox state and oxidative stress response of the cell (Valko et al., 2005; Baba and Bhatnagar, 2018; Ouyang et al., 2018). The depletion of major cellular sulfhydryl reserves seems to be a critical indirect mechanism for oxidative stress induced by metal(loid)s (Ouyang et al., 2018). The consequences of cells under oxidative stress include various dysfunctions mediated by direct damage to lipids, proteins, and DNA (Sies et al., 2017; Hawkins and Davies, 2019; Poetsch, 2020). Metals like Cd(II), Cu(I), Hg(II), Te(IV), and Ni(II) can trigger lipid peroxidation in different organisms (Company et al., 2004; Paraszkiewicz et al., 2010; Pradenas et al., 2013). Membrane damage caused by Cu(II), Cd(II) or Ag(I) can produce a loss of membrane potential (Dibrov et al., 2002; Hong et al., 2012). Double-stranded DNA can be damaged by oxidation, thereby inducing cell death and mutagenicity (Sies et al., 2017; Poetsch, 2020), as demonstrated with iron (Nunoshiba et al., 1999) and via genotoxicity assays with Mn, Cr, Cd, and other metals (Lemire et al., 2013). ROS can also induce protein oxidation and dysfunction, forming carbonyl derivatives through the metal-catalyzed oxidation of several amino acid side chains (such as histidine, arginine, lysine, and proline). Hence, carbonyl group levels are often used as a marker of oxidative protein damage (Stadtman and Levine, 2003). In other instances, metal(loid)-induced ROS dismantle Fe-S clusters in some dehydratases (Imlay, 2006; Calderón et al., 2009; Gomez et al., 2014).
The soft or hard acid nature of a specific metal(loid) can explain its toxicity and reactivity toward soft-base ligands within the protein matrix (Medici et al., 2021; Peana et al., 2021). For instance, Ni(II) can replace the structural Zn(II) present in the metal-binding sites of some proteins. These metal-binding sites can be exchanged by other more competitive divalent soft-metals, resulting in mismetallation and protein function loss (Macomber et al., 2011; Quintal et al., 2011). Certain metal(loid)s can damage enzymes that display reactive Cys residues at their active sites (Rajanna et al., 1990; Fadeeva et al., 2011). The soft-metal(loid)s Cu(I), Hg(II), Ag(I), Cd(II), and Te(IV) can dismantle solvent-exposed [4Fe-4S] clusters of Escherichia coli aconitases (Calderón et al., 2009; Macomber and Imlay, 2009; Xu and Imlay, 2012), releasing Fenton-active iron into the cytoplasm, which in turn generates ROS.
Despite the similar chemical reactivity of soft-metal(loid)s, few studies have identified cell targets beyond ROS-mediated oxidative stress. In this study, we conducted a genome-wide screening of an E. coli deletion collection challenged with soft-metal(loid)s under anaerobic conditions to identify ROS-independent targets. Strains lacking genes involved in protein homeostasis showed reduced fitness under these conditions, suggesting that proteins aggregated when exposed to Ag(I), Au(III), As(III), Cd(II), Hg(II), or Te(IV). Protein aggregation induced by Ag(I), Au(III), Hg(II), and Te(IV) required active translation, whereas As(III) and Cd(II) do not, suggesting that the mechanism by which these metals act during protein aggregation is different. In summary, we demonstrated that, in a ROS-independent manner, soft-metal(loid)s act as proteotoxic agents, leading to the accumulation of aggregated proteins, most likely due to at least two distinct mechanisms.
2 Methods
2.1 Bacterial strains
Escherichia coli K-12 BW25113 (National Institute of Genetics, Microbial Genetics Laboratory, NBRP, Japan) was used as the model strain. Unless otherwise indicated, all cultures were grown at 37°C in an anaerobic chamber (Coy Laboratory Products Inc., 100% N2 atmosphere) with constant shaking (150 rpm) in MOPS minimal medium supplemented with 0.2% (w/v) glucose. The barcoded deletion collection was derived from E. coli K-12 BW38028 (Otsuka et al., 2015).
2.2 Chemical genomic profiling of soft-metal(loid)s in Escherichia coli
The pooled E. coli deletion collection was independently inoculated in media supplemented with the following soft-metal(loid) salts (resuspended in H2O) at concentrations that resulted in a 20–30% reduction of OD600 compared to unexposed controls after 24 h: AgNO3 (625 nM), HAuCl4 (2 μM), NaAsO2 (125 μM), CdCl2 (31.25 μM), HgCl2 (375 nM) or K2TeO3 (14.7 μM). For control experiments, salts were replaced with H2O. Each pooled competition experiment was conducted in 200 μL cultures in triplicate at 37°C for 24 h. Genomic DNA was extracted using the Wizard Genomic DNA Purification Kit (Promega, Cat. No. A1120). Strain-specific barcodes from each culture were amplified using indexed primers designed for multiplexed Illumina sequencing. The forward primer contained the Illumina-specific P5 sequence, a 10 bp index tag (x’s), and the 19 bp E. coli deletion collection common priming site: 5-AATGATACGGCGACCACCGAGATCTACACTCTTTCCCTACACGACGCTCTTCCGATCTxxxxxxxxxxAATCTTCGGTAGTCCAGCG-3′. The reverse primer included the Illumina-specific P7 sequence and 20 bp E. coli common priming site: 5-CAAGCAGAAGACGGCATACGAGCTCTTCCGATCTTGTAGGCTGGAGCTGCTTCG-3′.
As previously described, barcodes were amplified by PCR, pooled, gel-purified, and quantified by quantitative PCR (Piotrowski et al., 2015). For barcode sequencing, samples were run on an Illumina HiSeq2500 in rapid run mode for 50 cycles at a loading concentration of 15 pM. The resulting fastq file was used for the analysis of sensitive and resistant mutants. Normalized counts were compared to a control solvent (water) to identify compound-specific responses among gene deletion mutants (chemical-genetic interaction score).
Sequence data were processed using BEANcounter (Simpkins et al., 2019) and EdgeR (Robinson et al., 2010). The chemical-genetic interaction score was computed as z-scores, representing the standardized deviation of each strain in treatments compared to their counterpart strain in the control solvent, for the 3,551 mutants quantified (Piotrowski et al., 2017; Simpkins et al., 2019). In this way, deletion-mutants whose abundance (compared to the mean of the population) was decrease as result of the treatment show a negative CG-score, while those having a higher abundance show positive CG-scores. CG-scores were analyzed using the DAVID database (Huang et al., 2009). Gene functional annotations were retrieved from EcoCyc (Keseler et al., 2017).
2.3 Isolation of aggregated proteins
Protein aggregation was assessed using the method described by Tomoyasu et al. (2001). Briefly, saturated cultures (16 h) were inoculated into 250 mL flasks containing 60 mL of MOPS medium, to a starting OD600 of approximately 0.05, and grown anaerobically at 37°C with constant shaking until they reached an OD600 of approximately 0.3. Cultures were individually exposed to soft-metal(loid) concentrations (as indicated in each figure) and incubated for 2 h at 37°C. Fifty mL of each treated culture were centrifuged at 5,000 × g for 10 min at 4°C. The resulting cell pellets were suspended in 250 μL of 10 mM potassium phosphate buffer pH 6.5 containing 1 mM EDTA (buffer A), supplemented with 20% (w/v) sucrose and 1 mg/mL lysozyme, and incubated on ice for 30 min. Then, 360 μL of buffer A was added, and cells were disrupted by sonication on ice (eight 15-s cycles with 45-s rests at 60% amplitude). Cell debris was removed by centrifugation at 2,000 × g for 15 min at 4°C. Both aggregated and membrane proteins were centrifuged at 15,000 × g for 20 min at 4°C and frozen at −80°C for later processing. Sedimented proteins were suspended in 400 μL of buffer A by brief sonication (one pulse, 5 s at 60% amplitude) and sedimented again at 15,000 × g for 20 min at 4°C. Membrane proteins were removed by suspending the pellet via brief sonication (one pulse, 5 s at 60% amplitude) in 320 μL of buffer A containing 2% (v/v) NP-40 (Abcam, Cat. No. ab142227). The aggregated proteins were sedimented at 15,000 × g for 30 min at 4°C. This washing procedure was repeated four times. The NP-40 insoluble pellet was rinsed with 400 μL of buffer A and finally suspended in 200 μL of the same buffer. Aggregated proteins were quantified by the Bradford method (Bradford, 1976), resolved by SDS-PAGE (12%), and visualized by silver staining.
2.4 Translation-arrested cells and pulse-chase of aggregated proteins
For experiments involving translation arrest, 100 μg/mL of chloramphenicol (CHL) was added to the cultures 5 min prior to the soft-metal(loid) treatment. For pulse-chase experiments, cultures (with an OD600 of approximately 0.3) were pulse-labeled with 250 μM 4-azido-L-homoalanine (AHA, Jena Bioscience, Cat. No. CLK-AA005) and incubated for 15 min at 37°C. The chase was initiated by adding L-methionine (Sigma-Aldrich, Cat. No. M9625) to a final concentration of 250 μM and incubated for 30 min at 37°C. Cells were then exposed to soft-metal(loid)s at the indicated concentrations for 2 h at the same temperature. Aggregated proteins were isolated as described above, but EDTA was omitted from the buffers to prevent Cu chelation in subsequent reactions. Protein aggregates were washed with phosphate saline buffer (PBS) and suspended through a brief (5-s) sonication in 200 μL of Click reaction buffer. This buffer contained 50 μM acetylene-PEG4-Biotin (Jena Bioscience, Cat. No. CLK-TA105), 1 mM TCEP (Tris(2-carboxyethyl)phosphine hydrochloride Sigma-Aldrich, Cat. No. C4706), 100 μM THPTA (Tris(3-hydroxypropyltriazolylmethyl)amine, Jena Bioscience, Cat. No. CLK-1010), and 1 mM CuSO4 (Sigma-Aldrich, Cat. No. C8027) in PBS, and incubated for 30 min at 37°C. Biotinylated protein aggregates were washed twice with PBS and then analyzed via Western blotting.
2.5 Western blotting of biotinylated aggregated proteins
Five hundred nanograms of aggregated proteins were fractionated using SDS-PAGE (12%). Proteins were then transferred to PVDF membranes (Bio-Rad, Cat. No. 1620177) at 40 mA overnight at 4°C. The membranes were blocked for 1 h in a Tris-buffered saline (TBS) buffer that was supplemented with 1% (v/v) Tween-20 (TBS-T) and 3% (w/v) BSA. After an overnight incubation at 4°C with a 1:10,000 dilution of Streptavidin-HRP (Sigma-Aldrich, Cat. No. S5512) in TBS-T plus 3% (w/v) BSA, the biotinylated proteins were visualized using SuperSignal Western blot FEMTO substrate (Thermo Scientific, Cat. No. 34094).
2.6 Proteomic profiling of aggregated proteins
Samples of aggregated proteins, obtained after a 2 h exposure to metal(loid) concentrations affecting the cell viability in a similar extend (10 μM AgNO3, 5 μM HAuCl4, 200 mM NaAsO2, 500 μM CdCl2, 5 μM HgCl2, or 20 μM K2TeO3), were flash-frozen in an ethanol dry-ice bath and processed for label-free quantification at Bioproximity in Virginia, USA. Only proteins identified with at least two unique peptides were included in the analysis. All proteins identified in the control sample were excluded from the analysis, mainly ribosomal proteins and translation factors. Functional protein associations and enrichment analysis were evaluated using the stringApp in Cytoscape (Doncheva et al., 2018). Enrichment analysis of aggregated proteins in each treatment was conducted using the DAVID database (Huang et al., 2009). All properties and subcellular locations of E. coli proteins were retrieved from EcoCyc (Keseler et al., 2017).
2.7 Cell viability
Cell viability was determined by diluting 20 μL of each treatment with 180 μL of a sterilized 0.9% (w/v) NaCl solution. After diluting up to 10−7, 4 μL of each dilution was spotted on LB (Luria Bertani broth) agar plates and incubated overnight at 37°C in anaerobic conditions.
2.8 Amino acids extraction and quantification
Amino acids were extracted using the method described by Steinfeld et al. (2014). Briefly, 60 mL of anaerobic E. coli cultures at OD600 0.3 in MOPS media were treated for 2 h with defined concentrations of soft-metal(loid)s. Twenty mL from each treatment were centrifuged at 4,000 × g for 10 min at 4°C. Cell pellets were resuspended in 1 mL of ice-cold MOPS media and centrifuged as described above. Then, cells were lysed with 1 mL of 1 N HCl, incubated for 5 min at room temperature, and centrifuged at 19,000 × g for 20 min at 20°C. The supernatant was dried in a Vacuum Concentrator SpeedVac SPD120 for 3 h at 50°C and 1.5 h at 35°C. The resulting pellets were flash-frozen at −80°C until use. Pellets were resuspended in 300 μL of water by sonication for 5 s, and two consecutive extractions with 500 and 300 μL of chloroform were performed. The aqueous layers were combined, filtered through a 0.22 μm PVDF filter (4 mm), and dried for 80 min at 65°C and 80 min at 55°C. The pellets were kept flash-frozen at −80°C until used.
Amino acids were quantified using diethyl ethoxymethylenemalonate (DEEMM) derivatization followed by UPLC-DAD quantification as described in Otaru et al. (2021), with few modifications. Each pellet was resuspended in 50 μL of 0.1 N HCl. After dissolution, 87.5 μL of 1 M borate buffer pH 9.0, 37.5 μL methanol, 2 μL of 2 g/L L-2-aminoadipic acid (in 0.1 M HCl; internal standard), and 1.75 μL DEEMM were added. Analytes were derivatized for 45 min at room temperature in an ultrasonic water bath, and the samples were then heated for 2 h at 70°C to stop the reaction. After that, samples were filtered (0.45 μm membrane) and transferred into glass vials for analysis.
Quantification was carried out using an H-Class Acquity UPLC system (Waters Corp., Milford, MA, USA) equipped with a photodiode array detector (DAD). The derivatized molecules were separated using a gradient of (A) 25 mM acetate buffer pH 6.6, (B) methanol, and (C) acetonitrile in a Waters Acquity UPLC BEH C18 1.7 μm column (2.1 × 100 mm) at 40°C, as described in Otaru et al. (2021). One μL sample was applied to the column and eluted at a flow rate of 0.46 mL/min. Individual compounds were quantified at 280 nm using the internal standard method. Data processing was performed using Empower 3 software (Waters).
2.9 Statistical analysis
Biochemical assays were repeated at least three times, and the data were represented as bar plots (mean with standard deviation or standard error), scatter plots (mean with standard error), or heatmaps (mean). In the case of SDS-PAGE and Western blot, representative gels were shown.
The DAVID platform was used to compute enrichment analysis statistics. Two-sample student’s t-tests, ANOVA, post hoc tests, and normal distribution statistics were calculated using R.
3 Results and discussion
3.1 Chemical genomic analysis of soft-metal(loid) toxicity in Escherichia coli
The toxicity of metal(loid)s in bacteria has been extensively studied and has been mostly related to the production of ROS. These highly reactive molecules rapidly oxidize several macromolecules, including DNA, membranes, and proteins. Moreover, protein aggregation was observed in yeast exposed to As(III) or Cd(II) under aerobic conditions (Jacobson et al., 2012, 2017). As(III) and Cd(II) displayed mutagenic activities dependent on the presence of RecA, suggesting single or double breaks in the DNA molecule (Lemire et al., 2013). However, the presence of oxygen in these experiments makes it difficult to determine whether the observed effect is a direct result of metal(loid) damage or ROS production. To directly identify the direct intracellular targets of soft-metal(loid)s, we challenged E. coli cells under anaerobic conditions where ROS cannot be formed.
We conducted chemical-genomic profiling of E. coli to identify deletions responsive to the soft-metal(loid)s Ag(I), Au(III), As(III), Cd(II), Hg(II), and Te(IV) under anaerobic conditions by using a published barcoded deletion collection (Otsuka et al., 2015; Morales et al., 2017). Each mutant in this barcoded collection has a unique 20-nucleotide barcode that allows quantification of its abundance through next-generation sequencing in a pooled competition experiment. We challenged the pooled collection with soft-metal(loid) concentrations that produced a mild (20–30%) reduction of the optical density (OD600) after 24 h compared to the non-treated control to avoid depletion of mutants involved in the general stress response. The chemical-genetic score (from now on, CG-scores, see Supplementary Table S1) of each knockout strain served as an indicator of the relative abundance after the challenge (Simpkins et al., 2019). A negative CG-score means that the abundance of the deletion mutant, compared to the rest of the population, was negatively affected by the treatment, while a positive score means an increased abundance on those conditions.
Deletion of genes involved in fundamental processes such as cell division, lipopolysaccharide core region synthesis, protein import, homologous recombination, conjugation, and cell shape regulation was detrimental to soft-metal(loid) exposure (Supplementary Figure S1). These results suggest that soft-metal(loid)s have a pleiotropic effect and affect multiple molecular targets, which ultimately leads to cell death.
Deleting prc, nlpI, ompA, galU, and ldcA resulted in reduced tolerance to most treatments (Supplementary Figure S2A,B). For example, the prc and nlpI gene deletions have a very low CG-score in most soft-metal(loid) treatments, except Te(IV). Prc (or Tsp) is a periplasmic protease indispensable for E. coli to survive under low osmolality at 42°C due to a potential role in regulating peptidoglycan biogenesis (Hsu et al., 2020). NlpI is a lipoprotein and adaptor protein for Prc (Tadokoro et al., 2004), targeting the murein endopeptidase MepS for degradation. Other gene deletions related to peptidoglycan synthesis showed a negative CG-score, such as mrcB in treatment with Cd(II) (Sugawara et al., 2021), and ybgF in treatment with Te(IV) (Gray et al., 2015) and ftsN in the treatment with As(III) (Müller et al., 2007) (Supplementary Figure S2B). Therefore, our data suggest that peptidoglycan biosynthesis might be a potential soft-metal(loid)s target.
Strikingly, our analyses revealed that mutants in genes encoding proteins required for homologous recombination-DNA repair and protein aggregation (Figure 1A), were among the most impacted by toxic exposure, strongly suggesting that soft-metal(loid)s could damage DNA and proteins under anoxic conditions. In contrast, no common pathway or biological process could give tolerance to all metal(loid)s under study (Supplementary Figures S2A,C), suggesting that their common chemical properties could explain some toxicity mechanisms, but tolerance mechanisms are rather diverse. Notably, the deletion of members of importers such as CysPUWA or MetNIQ gave resistance to Au(III) or As(III), respectively, implying that these metals could get inside the cell by those amino acid transporters (Supplementary Figure S2A). An alternative explanation is that importing Cys or Met could increase sensitivity to Au(III) or As(III), respectively. However, these experiments were performed in MOPS minimal media without amino acid addition.
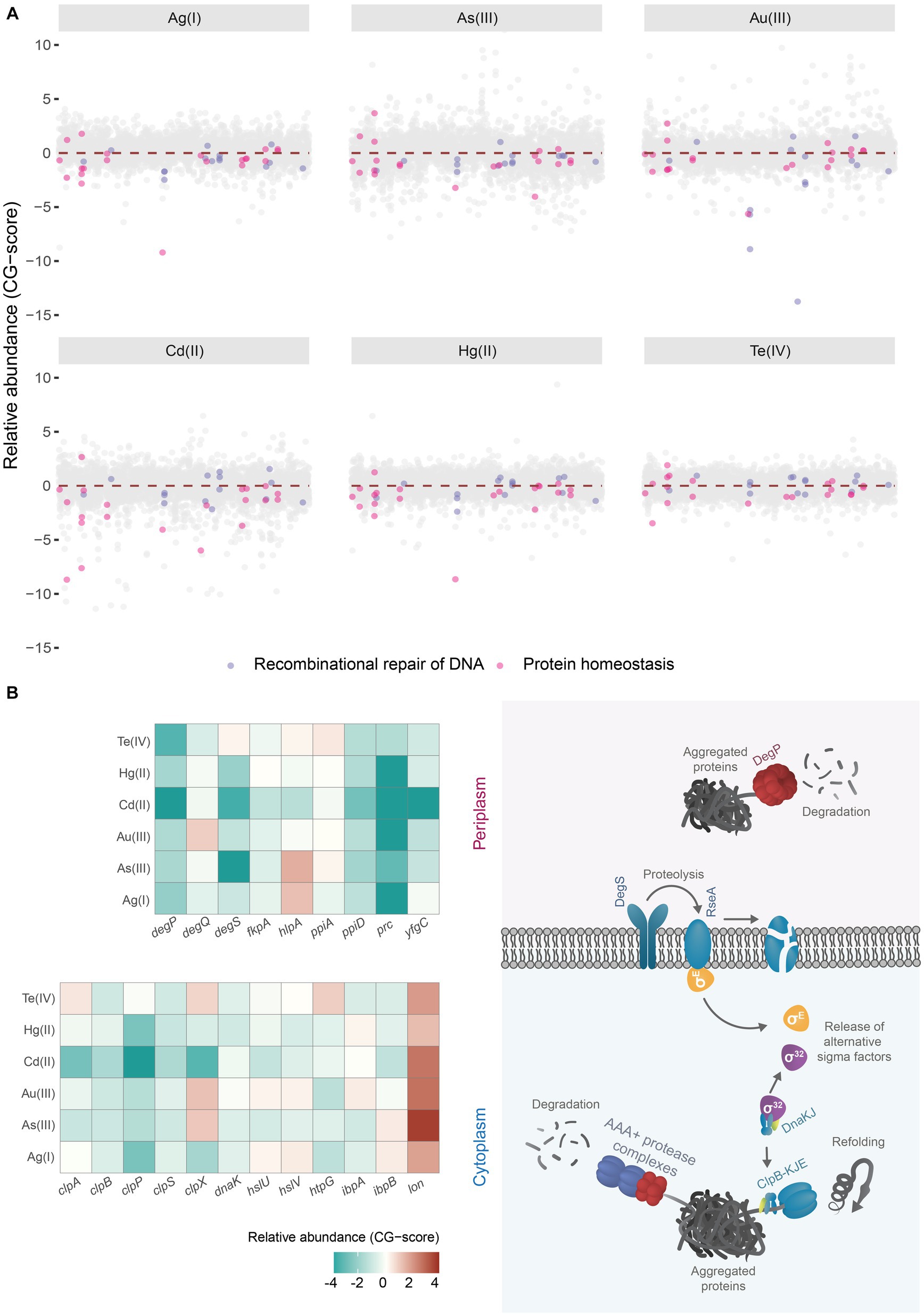
Figure 1. Chemical genomic analysis of soft-metal(loid) toxicity in E. coli. (A) Relative abundance (CG-score) of single gene deletion mutants after soft-metal(loid)s 24 h treatment. Mutants lacking genes involved in the recombinational repair of DNA (lavender dots) and protein homeostasis (pink dots) are highlighted. (B) In the left panel, a heat map of the relative abundance of deletion mutants in genes implicated in proteins homeostasis present in the periplasmic and cytoplasmic compartments after soft metal(loid)s treatments. In the right panel, a simplified model of chaperones/proteases involved in aggregated protein clearance in both periplasm and cytoplasm. ClpAPS and other AAA+ protease complexes and ClpB-DnaKJ-GrpE (refoldase) act in the cytoplasm; meanwhile, DegP (protease) functions in the periplasm. Misfolded proteins in this last compartment are detected by DegS, which catalyzes the degradation of the antisigma factor RseA. In the cytoplasm, on the other hand, sigma 32 is controlled through titration of DnaKJ by protein aggregates.
3.2 Soft-metal(loid)s induce protein aggregation
Previous research has suggested that soft metal(loid)s can induce protein aggregation and damage proteins under aerobic conditions. A prominent example of this is the interaction of Hg(II) and Cd(II) with luciferase during an in vitro refolding process, leading to its inactivation (Sharma et al., 2008). A recent study discovered that the soft-metal Cu(I) can cause protein aggregation in E. coli independently of ROS (Zuily et al., 2022).
Our analysis showed that deletion mutants in genes implicated in the protein quality control (PQC) network had decreased fitness in E. coli exposed to soft-metal(loid)s, suggesting these metal(loid)s can induce protein misfolding and aggregation in a ROS-independent manner (Figure 1A).
Under standard conditions, misfolded proteins can be refolded by chaperones or degraded by various proteolytic systems, preventing their accumulation. However, the protein quality control network can be overwhelmed under stress conditions, leading to an accumulation of protein aggregates (Tyedmers et al., 2010). Once these aggregated proteins are formed in E. coli, they can be refolded by ClpB (assisted by DnaK, DnaJ, and GrpE) or degraded by AAA+ proteases like ClpAP, HslUV, or Lon (Dougan et al., 2002; Haslberger et al., 2007; Tyedmers et al., 2010). DegS and DegP are two critical proteases involved in detecting misfolded and aggregated proteins in the periplasmic compartment. DegS functions as a regulatory protease that detects misfolded proteins through its PDZ domain and catalyzes the proteolysis of the anti-sigma factor RseA (Wilken et al., 2004) (Figure 1B, right panel). This process releases the alternative sigma factor RpoE, initiating a transcriptional response to manage misfolded proteins in the cytoplasmic and periplasmic compartments (Erickson and Gross, 1989; Danese and Silhavy, 1997). Egler et al. (2005) found that a ΔrpoE strain is sensitive to Cu(II), Cd(II), and Zn(II), but the authors did not speculate about a potential metal-mediated proteotoxic effect. DegP is a RpoE-induced serine protease/chaperone that degrades misfolded proteins in the periplasm. In our analysis, mutants lacking cytoplasmic and periplasmic proteases and chaperones, such as DegP, DegQ, the AAA+ protease ClpAP, and its adaptor ClpS (Dougan et al., 2002), displayed negative fitness when exposed to soft-metal(loid)s (Figure 1B). A similar negative fitness was observed for mutants lacking the refoldase ClpB and other cytoplasmic chaperones like DnaK and the small heat shock proteins IbpA and IbpB, all of which form an intricate network for protein disaggregation (Tyedmers et al., 2010). Consistent with this, previous transcriptomic analyses of E. coli exposed to Cd(II), Hg(II), and Te(IV) showed the induction of chaperone and protease systems such as ClpB, DnaK, and ClpP, among others (Wang and Crowley, 2005; Molina-Quiroz et al., 2014; LaVoie and Summers, 2018), supporting a proteotoxic effect of these metal(loid)s.
An intriguing observation was that lon deletion mutants showed improved fitness following soft-metal(loid) treatment. Lon is one of the main AAA+ proteases involved in the degradation of misfolded proteins, thereby preventing aggregation (Rosen et al., 2002). Some researchers have reported that it plays a minor role in protein aggregate clearance (Vera et al., 2005), but this does not explain the increased resistance to soft-metal(loid)s. This phenotype could result from Lon-dependent regulatory proteolysis of an unknown factor that when stabilized, could help combat soft-metal(loid) toxicity. Further studies are required to elucidate the role of Lon in this process.
To directly test if soft-metal(loid)s caused protein misfolding and aggregation under anaerobic conditions, we challenged exponentially growing wild type E. coli BW25113 to increasing concentrations of soft-metal(loid)s for 2 h and immediately quantified aggregated proteins (Figures 2A–C; Supplementary Figure S3). For all soft-metal(loid)s tested, treatment led to protein aggregation in a concentration-dependent manner and started even at low toxic concentrations (Figure 2B; Supplementary Figure S3A).
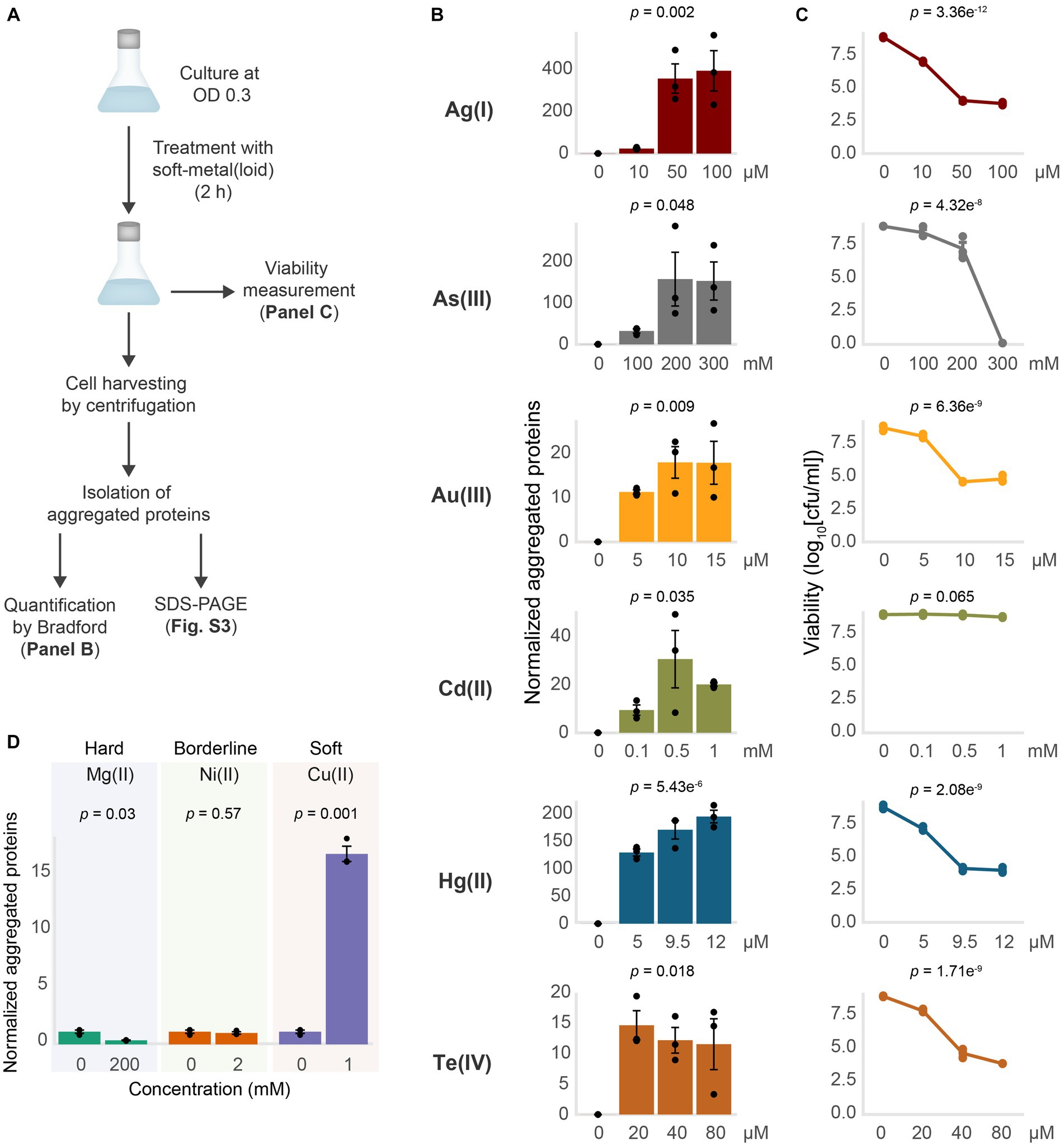
Figure 2. Soft-metal(loid)s induce protein aggregation. (A) Outline of the methodology for soft-metal(loid) treatment and aggregated protein quantification (Bradford) and visualization (SDS-PAGE). (B) Amount of aggregated proteins isolated from E. coli after the treatment with increasing concentrations of soft-metal(loid)s in anaerobic conditions. The amount of protein aggregated (μg) was normalized by the total protein amount in crude extracts (mg). The bars represent the mean and standard deviation of three biological replicates. p values were calculated by one-way ANOVA (C) Viability curve of E. coli treated with increasing concentrations of soft-metal(loid)s. The graph displays the mean and standard deviation of three biological replicates. p values were calculated using one-way ANOVA (D) Amount of aggregated proteins isolated from E. coli exposed to 200 mM of the hard-acid metal Mg(II), 2 mM of the borderline metal Ni(II), or 2 mM of the borderline/soft metal Cu(II). The bars represent the mean and standard deviation of three biological replicates. p values were calculated using the student’s t-test.
Interestingly, while all the tested soft-metal(loid)s caused protein aggregation, their effects on cell viability varied considerably (Figures 2B,C). For instance, 9.5 μM Hg(II) induced approximately 150 μg of aggregated proteins per mg of total protein, significantly decreasing viability by 5 orders of magnitude. In contrast, 200 mM As(III) produced similar number of aggregated proteins to Hg(II), but its impact on cell viability was noticeably less (Figures 2B,C). Others, like Te(IV) and Au(III), strongly affected viability at 20 and 10 μM, respectively; however, they induced less protein aggregation compared to Ag(I), Hg(II), and As(III). This indicates that for each metal(loid) there is no direct correlation between its effect on viability and the amount of protein aggregates (Figures 2B,C).
Recently, Zuily et al. (2022) demonstrated that the soft-metal Cu(I), and to a lesser extent Cu(II), can cause protein aggregation independent of ROS. In agreement with these results, we observed protein aggregation induced by Cu(II) at millimolar concentrations (1 mM), comparable to protein aggregation induced by some other soft-metal(loid)s tested (Figure 2D; Supplementary Figure S3B). Altogether, this suggests that the soft-acid property of these metal(loid)s could explain their reactivity toward proteins and cause aggregation. To test this, we exposed E. coli to the hard-acid metal Mg(II) and the borderline metal Ni(II) (Figure 2D; Supplementary Figure S3B). Exposure to Mg(II) or Ni(II) in the millimolar range did not result in protein aggregation, which is even reduced in the presence of Mg(II), suggesting that the soft-acid property, and thus the covalent nature of the metal–ligand interaction, might be important for inducing protein aggregation.
We next sought to assess whether protein aggregation could happen during or required active translation, as nascent proteins are not fully folded. The exposed soft residues may interact with these metal(loid)s through covalent bond formation, leading to unexpected geometries that could cause protein misfolding. To test this, we halted active translation by using chloramphenicol (CHL) for 5 min prior to toxic exposure and measured aggregated proteins (Figure 3). CHL pre-treatment did not affect protein aggregation mediated by As(III) and Cd(II), suggesting that it does not depend on active translation (Figures 3B,C). Conversely, Au(III)-induced aggregation was completely inhibited when the translation was blocked (Figures 3B,C), while for Ag(I), Hg(II), and Te(IV) it was inhibited by 85, 65, and 80%, respectively (Figure 3C). We hypothesize that the metal(loid) Ag(I), Hg(II), Au(III), and Te(IV) primarily cause protein aggregation by interacting with nascent proteins, while Cd(II) or As(III) can also interact with pre-folded proteins and produce aggregation by different mechanism. To directly test this, we conducted pulse-chase experiments in cells treated with the metalloids As(III) and Te(IV) as models. Proteins were pulse-labeled with 4-azido-L-homoalanine (AHA) for 15 min and chased with excess L-Met for 30 min to produce labeled folded proteins and unlabeled nascent proteins during toxicant exposure (Supplementary Figure S4). As hypothesized, the label was only detected in aggregates from cells treated with As(III), confirming that this oxyanion causes aggregation of proteins that are already folded, while Te(IV) appears to target nascent proteins (Supplementary Figure S4).
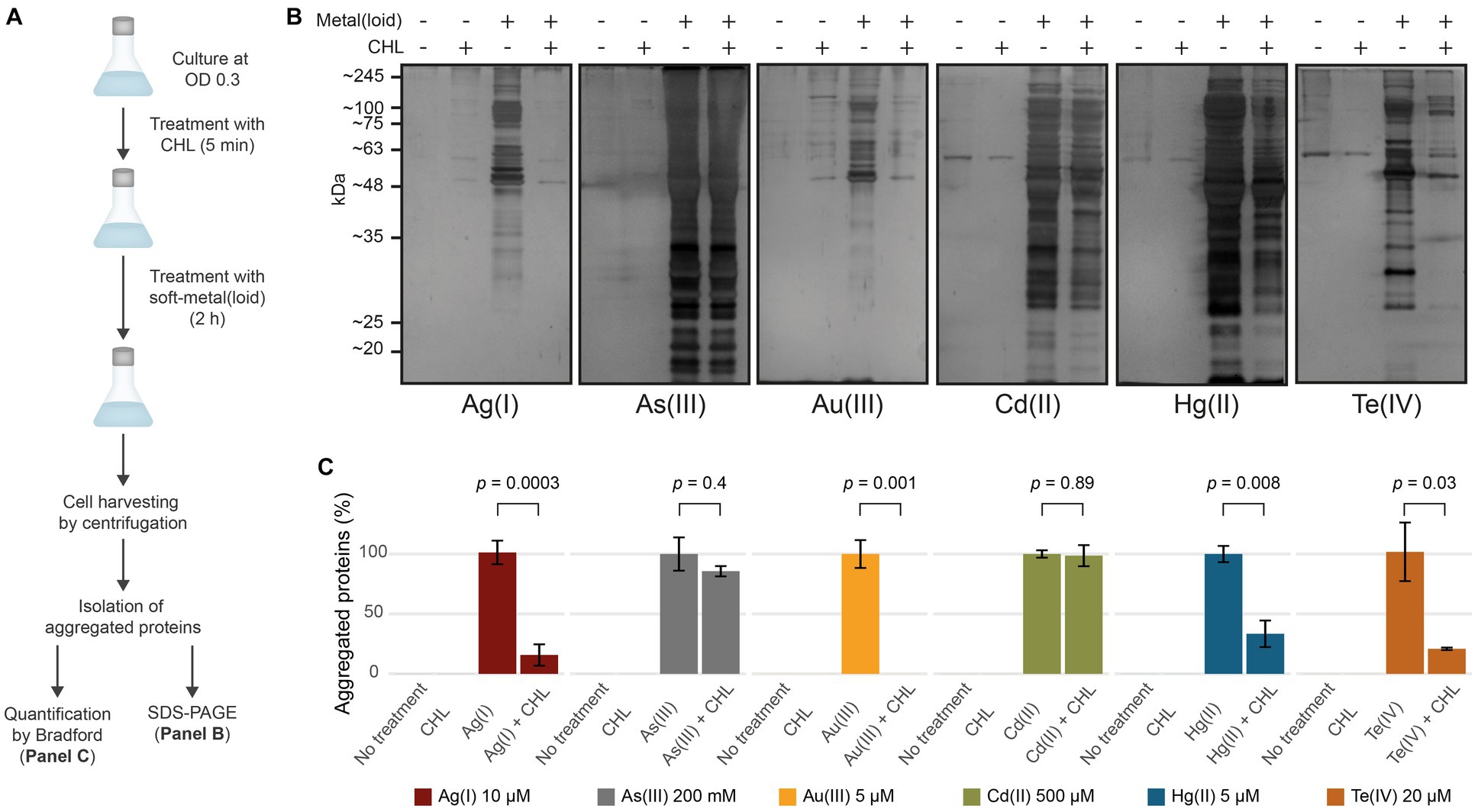
Figure 3. Soft-metal(loid)s induce protein aggregation of nascent and mature proteins. (A) Outline of the methodology used in the CHL treatment. Briefly, cells were grown to OD 0.3 and treated with 100 μg/mL of chloramphenicol (CHL) to stop protein synthesis before soft-metal(loid) treatment. After 2 h, protein aggregates were isolated and resolved on SDS-PAGE and quantified by Bradford. (B,C) Effect of protein synthesis inhibition on soft metal(loid)-induced protein aggregation in E. coli. (B) Ten microliters of the aggregated protein isolation were resolved in a denaturing SDS-PAGE (12%) and silver stained. (C) The amount of aggregated proteins in CHL-treated samples were compared against the respective soft-metal(loid) treatment (100%). Values represent the mean and the standard deviation of at least three biological replicates. p values were calculated using the student’s t-test.
In summary, our results show that exposure to soft-metal(loid)s caused protein aggregation in a ROS-independent manner. In E. coli, soft-metal(loid)s could cause protein aggregation through at least two hypothetically distinct mechanisms: (i) a translational-dependent mechanism where Hg(II), Au(III), Ag(I), and Te(IV) react with nascent proteins, causing misfolding, and (ii) a translational-independent (or post-translational) one, where As(III) and Cd(II) preferentially target mature proteins. Alternatively, the latter could be attributed to the inhibition of disaggregation or protein degradation pathways by Cd(II) and As(III), which might ultimately lead to an imbalance in proteostasis and an accumulation of protein aggregates. In this context, Cd(II), Hg(II), and Pb(II) have been found to inhibit DnaK-, DnaJ-, and GrpE-assisted refolding and disaggregation in vitro (Sharma et al., 2008).
Protein aggregation at the translational level could be explained by the interaction between Hg(II), Au(III), Ag(I), and Te(IV) with soft-side chains such as those from Cys, His, Met, and Phe residues (Stadtman and Levine, 2003). This may hinder proper folding by introducing different coordination geometries and impacting folding rates, leading to misfolding, aggregation, and the sequestration of other proteins. Proteins likely react with these metal(loid)s through metal binding sites as they are being synthesized, resulting in incorrect metallation and misfolding. For instance, the Cu, Zn superoxide dismutase (Cu, Zn - SOD) from S. cerevisiae undergoes the exchange of Cu(I) for Ag(I) when the yeast is exposed to silver nitrate, generating an inactive Ag, Zn - SOD. Interestingly, Ag, Zn - SOD is less immunoreactive to conformational antibodies against Cu, Zn - SOD, suggesting a change in the protein’s folding or conformation (Ciriolo et al., 1994).
3.3 Identification of aggregated proteins
The reduction of soluble proteins and their diversion to the insoluble fraction may account for the toxicity of protein aggregates, consequently impacting normal metabolism and appropriate cell development (Mogk et al., 2018). To gain further insights on how this process impacts the resistance of E. coli against soft-metal(loid)s, we identified the aggregated proteins derived from the exposure to each toxic by mass spectrometry (label-free quantification) (Figure 4; Supplementary Table S2).
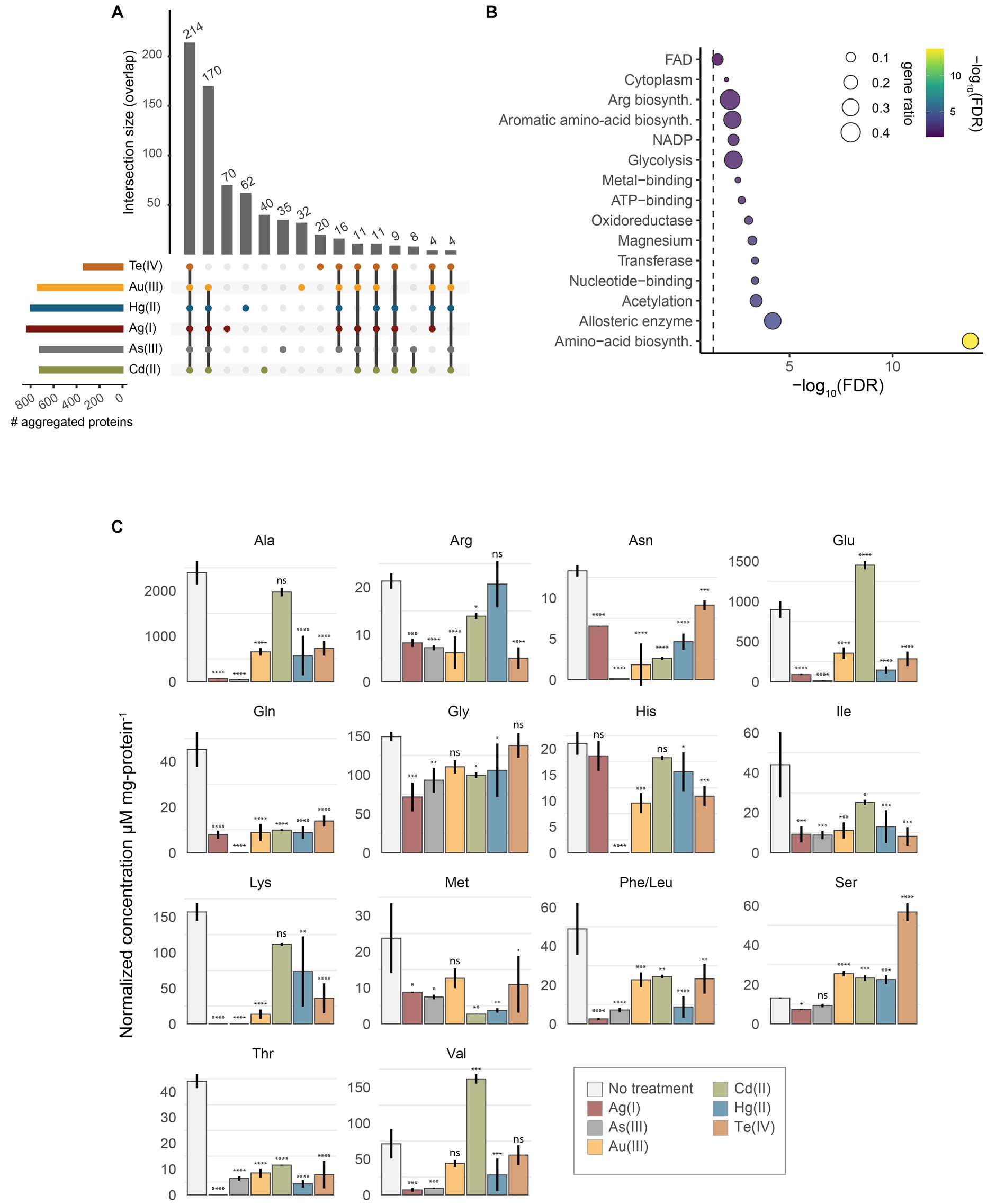
Figure 4. Identification of aggregated proteins. (A) UpSet plot representing the overlap of identified aggregated proteins after treatment with Te(IV), Au(III), Hg(II), Ag(I), As(III), or Cd(II). (B) UniProt keywords enrichment analysis of aggregated proteins by all soft-metal(loid)s. Gene ratio (size) is the fraction of significant genes over the total genes in a given pathway, ranked by FDR-adjusted p value (C) Normalized intracellular concentration of 15 amino acids measured from exponentially-grown E. coli exposed to 10 μM Ag(I), 200 mM As(III), 5 μM Au(III), 500 μM Cd(II), 5 μM Hg(II), or 20 μM Te(IV). Phe/Leu represents the sum of both amino acids. Statistical significance was calculated by one-way ANOVA and Dunnett’s posthoc test using no treatment as the control mean. Values represent the mean of three biological replicates and the standard error. ns: not significant; *: adjusted p value < 0.05; **: adjusted p value < 0.01; ***: adjusted p value < 0.001; ****: adjusted p value < 0.0001.
After treatment with Te(IV), Au(III), As(III), Hg(II), Cd(II), or Ag(I), we identified 335, 737, 718, 798, 719, and 831 insoluble proteins, respectively (Figure 4A). This represents between 7.5 and 18.6% of the proteins encoded by E. coli. Although the removal of periplasmic chaperones and proteases resulted in decreased fitness after toxic exposure, the aggregated proteins that we identified are mainly cytoplasmic. For example, 78.5, 85.7, 87.2, 82.8, 88.9, and 87.1% of the identified proteins in aggregates induced by Te(IV), Au(III), As(III), Ag(I), Cd(II), and Hg(II), respectively, were cytoplasmic proteins.
To assess if aggregated proteins where enriched in residues with a soft-base nature such as Cys (C), Met (M), His (H) or Phe (F), we quantified the frequency of each residue on aggregated proteins compared to the total coding sequences of E. coli (Supplementary Figure S5A). When comparing cytoplasmic proteins, which represent the biggest share of aggregated proteins, a slight increase of frequency of Cys was observed in Ag(I) and Cd(II) treatments. On the other hand, a different effect is observed in aggregated membrane proteins, where the frequency of residues such as Asp (D), Glu (E), Arg (R) was overrepresented in all treatments with all metal(oid)s, and a lower representation of tryptophan (W) was observed in 5 of the metals analyzed (Supplementary Figure S5A). Furthermore, soft-metal(loid)-induced aggregated proteins have a similar hydrophobicity index compared to the E. coli proteome (Supplementary Figure S5B). Aggregated cytoplasmic proteins do not show a bias to specific pI or molecular weight. In contrast, aggregation of membrane proteins seems to mainly affect acidic proteins (Supplementary Figure S5C), which coincides with what was observed in a greater number of acidic amino acids, such as D (in Ag(I), As(III), Au(III), Cd(II) and Hg(II)) and E (in all metal(loid)s). In addition, a slight shift to a higher molecular weight is observed in the aggregated proteins (Supplementary Figure S5C). This suggest that ionic interaction seems to be more relevant for aggregation of membrane proteins than covalent interactions.
The results described above suggest that there is no clear physicochemical property that can explain the aggregation of cytoplasmic proteins induced by soft metal(loid)s. Then, we ought to see if proteins that are highly translated might be targeted by soft metal(loid)s given that we observed a dependency on active translation for Ag(I), Au(III), Hg(II) and Te(IV)-mediated protein aggregation. For this, we took advantage of the already published protein synthesis rate for E. coli in MOPS minimal media (Li et al., 2014). Remarkably, the proteins detected in soft metal(loid)-induced aggregates are among the highly expressed proteins from E. coli (Supplementary Figure S5D). This correlates with the observed translation-dependent protein aggregation for Te(IV), Au(III), Ag(I) and Hg(II); however, protein aggregated by As(III) or Cd(II) show a similar trend.
During their synthesis, proteins can be assisted for correct folding by chaperones such as DnaK, GroE and the trigger factor. In E. coli, deletion of dnaK and tig (trigger factor) leads to disrupted proteostasis, producing aggregation of more than 1,000 proteins (Calloni et al., 2012). Therefore, we examined if proteins aggregated by soft metal(loid) treatments are preferentially clients of one of these chaperone systems (Supplementary Figure S5E). Noticeably, around 50% of the proteins aggregated by soft metal(loid) treatments are known clients of DnaK, according to STEPdb. This effect is not observed for trigger factor or GroE clients. These results agree with Sharma and collaborators (2008) that observed inhibition of DnaKJ-GrpE in vitro refolding of unfolded luciferase by Cd(II); probably by interaction of Cd(II) with the zinc-binding domain of DnaJ.
Next, to assess the presence of essential proteins within the aggregated material, we compared sets of aggregated proteins in each treatment to essential genes identified for a genome-wide deletion collection in MOPS media (Baba et al., 2006). The number of essential proteins in aggregates varied depending on the metal(loid) treatment: aggregates induced by Ag(I), Te(IV), As(III), Au(III), Hg(II), and Cd(II) contained 110, 21, 98, 102, 109, and 96 essential proteins, respectively. This indicates that protein aggregation induced by soft metal(loid)s disrupts crucial cellular processes by decreasing the soluble levels of essential proteins, leading to cell death. An interesting documented example of this phenomenon is MetA from E. coli, an essential thermosensitive protein in minimal media; depletion of this protein from soluble media due to high temperature-dependent aggregation causes a decrease in L-Met biosynthesis, impacting cell growth (Gur et al., 2002).
We collated the results of chemical genomics and protein aggregation to identify if, among the aggregated proteins, we could identify the ones that are important for soft metal(loid) tolerance (Supplementary Figure S6). The rationale was that deleting the gene coding for those proteins would negatively impact strain abundance (CG scores) in the treatments. Overall, there is no clear correlation between proteins identified in the aggregates, observing that deletion of genes of identified aggregated proteins can have favorable, deleterious or no effect on resistance to the metals analyzed. However, in 5 of the 6 metals analyzed (Ag(I), As(III), Au(III), Cd(II) and Hg(II)), the RfaG (WaaG) protein is detected in the aggregates, and its deletion increases sensitivity to them. Also, other proteins part of LPS biosynthesis are found, such as RfaH, RfaF, and RfaQ, confirming that the biosynthesis of liposaccharides is affected by these soft metal(loid)s (Figure 1B). In E. coli, deletion of waaG resulted in a truncated LPS core section lacking most of the negatively charged phosphate groups where the UO22+ cation binds (Thorgersen et al., 2021). In addition, Chromium exposure induces a membrane response, and lipopolysaccharide biosynthesis is a mechanism upregulated in Caulobacter crescentus (Hu et al., 2005).
Among the total number of proteins identified in the aggregates induced by soft metal(loid)s, 214 were shared by all treatments (Figure 4A). A KEGG pathway enrichment analysis revealed that these proteins are primarily involved in metabolic pathways such as amino acid biosynthesis, pyruvate metabolism, central metabolism, and the biosynthesis of secondary metabolites (Figure 4B; Supplementary Table S3). When proteins identified in every soft-metal(loid) treatment are examined individually, they participate in the same cellular processes and metabolic pathways as the aforementioned 214 proteins (Figures 4A,B). A UniProt keywords enrichment analysis of those proteins showed a clear enrichment of proteins involved in amino acid biosynthesis. Notably, metal and magnesium binding proteins were also enriched, suggesting a possible role of mismetallation on protein aggregation. Surprisingly, Cd(II) and As(III) influenced the same processes as the other soft-metal(loid)s, despite inducing aggregation through a potentially different mechanism. Nonetheless, in our analysis, it is challenging to determine which proteins were directly misfolded by the metal(loid) treatment and which were subsequently trapped by the aggregates.
Our results support the observations made by Weids and collaborators (2016) that described that As(III), H2O2 and azetidine-2-carboxylic acid promote the aggregation of highly expressed proteins, which are involved in metabolic processes such as amino acid biosynthesis, and a large fraction interacts with the Hsp70 chaperone (DnaK in E. coli).
3.4 Soft-metal(loid)s perturb intracellular amino acid concentration
Since proteins involved in amino acid biosynthesis were among the most represented in protein aggregates for all treatments (Figure 4B), we speculated that amino acid levels might be affected after toxicant exposure. Furthermore, amino acid biosynthesis is an essential process for E. coli growing in minimal media, and its disruption would explain part of their toxicity mechanisms. We measured the intracellular concentration of 15 amino acids in exponentially growing E. coli exposed to soft-metal(loid)s under the same conditions as in the protein identification analysis. Peaks for phenylalanine and leucine were not resolved, and their concentration represents the sum of both amino acids. Although none of the metal(loid)s depleted all amino acids, they impacted the levels of a significant number of them, ranging from 8 [Cd(II)] to 14 [Ag(I) and As(III)]. All soft-metal(loid) treatments decreased the intracellular concentration of asparagine, glutamine, isoleucine, phenylalanine + leucine, and threonine (Figure 4C). For asparagine, isoleucine, and leucine, we detected proteins involved in their biosynthesis in the aggregates of most of the soft-metal treatments (Figure 4C; Supplementary Table S2). AsnA (aspartate-ammonia ligase (ADP-forming) (EC 6.3.1.4)) and IlvA (threonine ammonia-lyase, EC 4.3.1.19) have been previously detected in aggregates during anaerobic copper treatment (Zuily et al., 2022). LeuB (3-isopropylmalate dehydrogenase, EC 1.1.1.85) has been detected in protein aggregates when the gene was overexpressed in E. coli (Śmigiel et al., 2022) and in yeast deficient in the shock protein Hsp70 of type-Ssa (Amm et al., 2016).
Decreased intracellular amino acid concentrations may result in numerous deleterious effects on bacterial physiology. These could include deficiencies in protein and other cell structure synthesis (e.g., cell wall), metabolism of various cell pathway intermediates, cellular buffering, and osmotolerance, among several others (Nelson and Cox, 2017; Xiao et al., 2017; Aliashkevich et al., 2018; Idrees et al., 2020). Interestingly, this metabolic condition could be somewhat beneficial; under physiological conditions of anaerobic amino acid limitation, the efflux system pump CusCFBA is induced to protect iron–sulfur cluster proteins on E. coli cells exposed to Cu(I) (Fung et al., 2013).
We also observed the accumulation of certain amino acids after metal(loid) exposure. For instance, serine accumulates after Au(III), Cd(II), Hg(II), and Te(IV) treatments, with the latter inducing the highest accumulation (4.3-fold) (Figure 4C). Similarly, Cd(II) treatment also resulted in an accumulation of glutamic acid and valine. The excess of intracellular serine is toxic for E. coli as it inhibits the biosynthesis of isoleucine and aromatic amino acids (Hama et al., 1990; Tazuya-Murayama et al., 2006), disrupts cell division (Zhang and Newman, 2008), and generates the misincorporation of serine instead of alanine in peptidoglycan crosslinks (Zhang et al., 2010). Serine excess is removed to the extracellular medium by SdaC or can be deaminated to pyruvate and ammonia by SdaA, SdaB, TdcG, TdcB, or Thr/Ser dehydrogenase IlvA (Shizuta et al., 1969; Su et al., 1989; Su and Newman, 1991; Burman et al., 2004; Borchert and Downs, 2018; Kriner and Subramaniam, 2019). Mutants lacking these proteins did not display significant changes in fitness (CG z-scores) after soft-metal(loid) exposure, and only IlvA was detected in the aggregated material produced by Te(IV) (Supplementary Table S1). IlvA was also aggregated in treatments that did not induce serine accumulation, so it may not solely explain the phenomena observed with Te(IV). Our data do not suggest that serine accumulation is a direct result of protein aggregation. Altogether, our results demonstrate that soft-metal(loid)s result in the aggregation of proteins required for amino acid biosynthesis, which in turn leads to perturbations in the intracellular levels of amino acids.
In summary, we demonstrate that proteins can be aggregated in a ROS-independent manner by soft-metal(loid)s, mainly affecting proteins involved in anabolic pathways. Protein aggregation might be primarily induced at the translational level by Ag(I), Au(III), Hg(II), and Te(IV), or at the post-translational level by Cd(II) and As(III). Initial protein aggregates might sequester other proteins by exposing hydrophobic or disordered regions to the surface, leading to secondary loss of function (Mogk et al., 2018). Regardless of the mechanism, it can be expected that amino acid biosynthesis is hindered as a cellular response under our experimental conditions, also impacting the tRNA charging process, ribosome stalling and limited translation, induction of the stringent response, and altered synthesis of various metabolites that use amino acids as precursors (Traxler et al., 2008; Nedialkova and Leidel, 2015; Wang et al., 2020). The fact that we did not observe a direct correlation between protein aggregation and cell viability suggests that soft-metal(loid) toxicity is a multifactorial phenomenon, an observation further confirmed by the wide variety of gene deletions that can lead to decreased fitness upon soft-metal(loid) challenges. These various targets could explain why these types of metal(loid)s are effective biocides.
Data availability statement
The original contributions presented in the study are included in the article/Supplementary material, further inquiries can be directed to the corresponding author.
Author contributions
FC: Conceptualization, Formal analysis, Investigation, Methodology, Writing – original draft. CM-V: Conceptualization, Data curation, Formal analysis, Methodology, Investigation, Writing – original draft. RL: Data curation, Formal analysis, Investigation, Writing – original draft. MS-D: Conceptualization, Data curation, Investigation, Methodology, Writing – original draft. CC: Conceptualization, Data curation, Formal analysis, Project administration, Writing – original draft. BP: Conceptualization, Investigation, Methodology, Writing – original draft. EM: Conceptualization, Data curation, Methodology, Writing – original draft. JP: Data curation, Formal analysis, Writing – original draft. JS: Conceptualization, Data curation, Formal analysis, Writing – original draft. CV: Conceptualization, Writing – original draft. FA: Conceptualization, Funding acquisition, Investigation, Resources, Writing – original draft.
In memoriam
This paper is dedicated to the memory of CV. We report with great sadness that CV died on 17th July 2020. He had seen and approved a previous version of this manuscript.
Funding
The author(s) declare financial support was received for the research, authorship, and/or publication of this article. This work received financial support from Fondecyt Regular 1230724 (FA), USA1799 Vridei 021943FA_GO Universidad de Santiago de Chile (FC and FA), Beca Doctorado Nacional 21150690 (FC), and Dicyt (FA and CV).
Acknowledgments
We thank Dr. Roberto Molina-Quiroz (CECS, Valdivia, Chile) and Dr. James Imlay (Department of Microbiology, University of Illinois Urbana-Champaign) for critically reading the manuscript.
Conflict of interest
EM and JP were employed by E. & J. Gallo Winery.
The remaining authors declare that the research was conducted in the absence of any commercial or financial relationships that could be construed as a potential conflict of interest.
Publisher’s note
All claims expressed in this article are solely those of the authors and do not necessarily represent those of their affiliated organizations, or those of the publisher, the editors and the reviewers. Any product that may be evaluated in this article, or claim that may be made by its manufacturer, is not guaranteed or endorsed by the publisher.
Supplementary material
The Supplementary material for this article can be found online at: https://www.frontiersin.org/articles/10.3389/fmicb.2023.1281058/full#supplementary-material
References
Aliashkevich, A., Alvarez, L., and Cava, F. (2018). New insights into the mechanisms and biological roles of D-amino acids in complex eco-systems. Front. Microbiol. 9:683. doi: 10.3389/fmicb.2018.00683
Amm, I., Kawan, M., and Wolf, D. H. (2016). Characterization of protein quality control components via dual reporter-containing misfolded cytosolic model substrates. Anal. Biochem. 515, 14–21. doi: 10.1016/j.ab.2016.09.012
Baba, T., Ara, T., Hasegawa, M., Takai, Y., Okumura, Y., Baba, M., et al. (2006). Construction of Escherichia coli K-12 in-frame, single-gene knockout mutants: the Keio collection. Mol. Syst. Biol. 2:0008. doi: 10.1038/msb4100050
Baba, S. P., and Bhatnagar, A. (2018). Role of thiols in oxidative stress. Curr. Opin. Toxicol. 7, 133–139. doi: 10.1016/j.cotox.2018.03.005
Borchert, A. J., and Downs, D. M. (2018). Analyses of variants of the Ser/Thr dehydratase IlvA provide insight into 2-aminoacrylate metabolism in Salmonella enterica. J. Biol. Chem. 293, 19240–19249. doi: 10.1074/jbc.RA118.005626
Bradford, M. M. (1976). A rapid and sensitive method for the quantitation of microgram quantities of protein utilizing the principle of protein-dye binding. Anal. Biochem. 72, 248–254. doi: 10.1016/0003-2697(76)90527-3
Burman, J. D., Harris, R. L., Hauton, K. A., Lawson, D. M., and Sawers, R. G. (2004). The iron–sulfur cluster in the l-serine dehydratase TdcG from Escherichia coli is required for enzyme activity. FEBS Lett. 576, 442–444. doi: 10.1016/j.febslet.2004.09.058
Calderón, I. L., Elías, A. O., Fuentes, E. L., Pradenas, G. A., Castro, M. E., Arenas, F. A., et al. (2009). Tellurite-mediated disabling of [4Fe-4S] clusters of Escherichia coli dehydratases. Microbiology 155, 1840–1846. doi: 10.1099/mic.0.026260-0
Calloni, G., Chen, T., Schermann, S. M., Chang, H. C., Genevaux, P., Agostini, F., et al. (2012). DnaK functions as a central hub in the E. coli chaperone network. Cell Rep. 1, 251–264. doi: 10.1016/j.celrep.2011.12.007
Ciriolo, M. R., Civitareale, P., Carrì, M. T., De Martino, A., Galiazzo, F., and Rotilio, G. (1994). Purification and characterization of ag,Zn-superoxide dismutase from Saccharomyces cerevisiae exposed to silver. J. Biol. Chem. 269, 25783–25787. doi: 10.1016/S0021-9258(18)47316-4
Company, R., Serafim, A., Bebianno, M. J., Cosson, R., Shillito, B., and Fiala-Medioni, A. (2004). Effect of cadmium, copper and mercury on antioxidant enzyme activities and lipid peroxidation in the gills of the hydrothermal vent mussel Bathymodiolus azoricus. Mar. Environ. Res. 58, 377–381. doi: 10.1016/j.marenvres.2004.03.083
Danese, P. N., and Silhavy, T. J. (1997). The sigma (E) and the Cpx signal transduction systems control the synthesis of periplasmic protein-folding enzymes in Escherichia coli. Genes Dev. 11, 1183–1193. doi: 10.1101/gad.11.9.1183
Daum, J. R., Shepherd, D. M., and Noelle, R. J. (1993). Immunotoxicology of cadmium and mercury on B-lymphocytes--I. Effects on lymphocyte function. Int. J. Immunopharmacol. 15, 383–394. doi: 10.1016/0192-0561(93)90049-5
Dibrov, P., Dzioba, J., Gosink, K. K., and Häse, C. C. (2002). Chemiosmotic mechanism of antimicrobial activity of ag+ in Vibrio cholerae. Antimicrob. Agents Chemother. 46, 2668–2670. doi: 10.1128/AAC.46.8.2668-2670.2002
Doncheva, N. T., Morris, J. H., Gorodkin, J., and Jensen, L. J. (2018). Cytoscape StringApp: network analysis and visualization of proteomics data. J. Proteome Res. 18, 623–632. doi: 10.1021/acs.jproteome.8b00702
Dougan, D. A., Reid, B. G., Horwich, A. L., and Bukau, B. (2002). ClpS, a substrate modulator of the ClpAP machine. Mol. Cell 9, 673–683. doi: 10.1016/S1097-2765(02)00485-9
Egler, M., Grosse, C., Grass, G., and Nies, D. H. (2005). Role of the extracytoplasmic function protein family sigma factor RpoE in metal resistance of Escherichia coli. J. Bacteriol. 187, 2297–2307. doi: 10.1128/JB.187.7.2297-2307.2005
Erickson, J. W., and Gross, C. A. (1989). Identification of the sigma E subunit of Escherichia coli RNA polymerase: a second alternate sigma factor involved in high-temperature gene expression. Genes Dev. 3, 1462–1471. doi: 10.1101/gad.3.9.1462
Fadeeva, M. S., Bertsova, Y. V., Euro, L., and Bogachev, A. V. (2011). Cys377 residue in NqrF subunit confers ag(+) sensitivity of Na+-translocating NADH:quinone oxidoreductase from Vibrio harveyi. Biochemistry 76, 186–195. doi: 10.1134/s0006297911020040
Foster, A. W., Young, T. R., Chivers, P. T., and Robinson, N. J. (2022). Protein metalation in biology. Curr. Opin. Chem. Biol. 66:102095. doi: 10.1016/j.cbpa.2021.102095
Fung, D. K., Lau, W. Y., Chan, W. T., and Yan, A. (2013). Copper efflux is induced during anaerobic amino acid limitation in Escherichia coli to protect iron-sulfur cluster enzymes and biogenesis. J. Bacteriol. 195, 4556–4568. doi: 10.1128/JB.00543-13
Gomez, M., Pérez-Gallardo, R. V., Sánchez, L. A., Díaz-Pérez, A. L., Cortés-Rojo, C., Meza Carmen, V., et al. (2014). Malfunctioning of the iron–sulfur cluster assembly machinery in Saccharomyces cerevisiae produces oxidative stress via an iron-dependent mechanism, causing dysfunction in respiratory complexes. PLoS One 9:e111585. doi: 10.1371/journal.pone.0111585
Gray, A. N., Egan, A. J. F., Van’t Veer, I., Verheul, J., Colavin, A., Koumoutsi, A., et al. (2015). Coordination of peptidoglycan synthesis and outer membrane constriction during Escherichia coli cell division. eLife 4:e07118. doi: 10.7554/eLife.07118
Gur, E., Biran, D., Gazit, E., and Ron, E. Z. (2002). In vivo aggregation of a single enzyme limits growth of Escherichia coli at elevated temperatures. Mol. Microbiol. 46, 1391–1397. doi: 10.1046/j.1365-2958.2002.03257.x
Hama, H., Sumita, Y., Kakutani, Y., Tsuda, M., and Tsuchiya, T. (1990). Target of serine inhibition in Escherichia coli. Biochem. Biophys. Res. Commun. 168, 1211–1216. doi: 10.1016/0006-291X(90)91157-N
Haslberger, T., Weibezahn, J., Zahn, R., Lee, S., Tsai, F. T., Bukau, B., et al. (2007). M domains couple the ClpB threading motor with the DnaK chaperone activity. Mol. Cell 25, 247–260. doi: 10.1016/j.molcel.2006.11.008
Hawkins, C. L., and Davies, M. J. (2019). Detection, identification, and quantification of oxidative protein modifications. J. Biol. Chem. 294, 19683–19708. doi: 10.1074/jbc.REV119.006217
Hong, R., Kang, T. Y., Michels, C. A., and Gadura, N. (2012). Membrane lipid peroxidation in copper alloy-mediated contact killing of Escherichia coli. Appl. Environ. Microbiol. 78, 1776–1784. doi: 10.1128/AEM.07068-11
Hsu, P., Chen, C., Wang, S., Hashimoto, M., Huang, W., and Teng, C. (2020). Identification of Mlt G as a Prc protease substrate whose dysregulation contributes to the conditional growth defect of prc-deficiente Escherichia coli. Front. Microbiol. 11:2000. doi: 10.3389/fmicb.2020.02000
Hu, P., Brodie, E. L., Suzuki, Y., McAdams, H. H., and Andersen, G. L. (2005). Whole-genome transcriptional analysis of heavy metal stresses in Caulobacter crescentus. J. Bacteriol. 187, 8437–8449. doi: 10.1128/JB.187.24.8437-8449.2005
Huang, d. W., Sherman, B. T., and Lempicki, R. A. (2009). Systematic and integrative analysis of large gene lists using DAVID bioinformatics resources. Nat. Protoc. 4, 44–57. doi: 10.1038/nprot.2008.211
Idrees, M., Mohammad, A. R., Karodia, N., and Rahman, A. (2020). Multimodal role of amino acids in microbial control and drug development. Antibiotics 9:330. doi: 10.3390/antibiotics9060330
Imlay, J. A. (2006). Iron-sulphur clusters and the problem with oxygen. Mol. Microbiol. 59, 1073–1082. doi: 10.1111/j.1365-2958.2006.05028.x
Jacobson, T., Navarrete, C., Sharma, S. K., Sideri, T. C., Ibstedt, S., Priya, S., et al. (2012). Arsenite interferes with protein folding and triggers formation of protein aggregates in yeast. J. Cell Sci. 125, 5073–5083. doi: 10.1242/jcs.107029
Jacobson, T., Priya, S., Sharma, S. K., Andersson, S., Jakobsson, S., Tanghe, R., et al. (2017). Cadmium causes misfolding and aggregation of cytosolic proteins in yeast. Mol. Cell. Biol. 37:e00490-16. doi: 10.1128/MCB.00490-16
Keseler, I. M., Mackie, A., Santos-Zavaleta, A., Billington, R., Bonavides-Martínez, C., Caspi, R., et al. (2017). The EcoCyc database: reflecting new knowledge about Escherichia coli K-12. Nucleic Acids Res. 45, D543–D550. doi: 10.1093/nar/gkw1003
Kim, J. J., Kim, Y. S., and Kumar, V. (2019). Heavy metal toxicity: an update of chelating therapeutic strategies. J. Trace Elem. Med. Biol. 54, 226–231. doi: 10.1016/j.jtemb.2019.05.003
Kriner, M. A., and Subramaniam, A. R. (2019). The serine transporter SdaC prevents cell lysis upon glucose depletion in Escherichia coli. Microbiology 9:e960. doi: 10.1002/mbo3.960
LaVoie, S. P., and Summers, A. O. (2018). Transcriptional responses of Escherichia coli during recovery from inorganic or organic mercury exposure. BMC Genomics 19:52. doi: 10.1186/s12864-017-4413-z
Lemire, J. A., Harrison, J. J., and Turner, R. J. (2013). Antimicrobial activity of metals: mechanisms, molecular targets and applications. Nat. Rev. Microbiol. 11, 371–384. doi: 10.1038/nrmicro3028
Li, Y. P., Ben Fekih, I., Chi Fru, E., Moraleda-Munoz, A., Li, X., Rosen, B. P., et al. (2021). Antimicrobial activity of metals and metalloids. Annu. Rev. Microbiol. 75, 175–197. doi: 10.1146/annurev-micro-032921-123231
Li, G. W., Burkhardt, D., Gross, C., and Weissman, J. S. (2014). Quantifying absolute protein synthesis rates reveals principles underlying allocation of cellular resources. Cells 157, 624–635. doi: 10.1016/j.cell.2014.02.033
Macomber, L., Elsey, S. P., and Hausinger, R. P. (2011). Fructose-1,6-bisphosphate aldose (class II) is the primary site of nickel toxicity in Escherichia coli. Mol. Microbiol. 82, 1291–1300. doi: 10.1111/j.1365-2958.2011.07891.x
Macomber, L., and Imlay, J. A. (2009). The iron-sulfur clusters of dehydratases are primary intracellular targets of copper toxicity. Proc. Natl. Acad. Sci. U. S. A. 106, 8344–8349. doi: 10.1073/pnas.0812808106
Medici, S., Peana, M., Pelucelli, A., and Zoroddu, M. A. (2021). An updated overview on metal nanoparticles toxicity. Semin. Cancer Biol. 76, 17–26. doi: 10.1016/j.semcancer.2021.06.020
Mjos, K. D., and Orvig, C. (2014). Metallodrugs in medicinal inorganic chemistry. Chem. Rev. 114, 4540–4563. doi: 10.1021/cr400460s
Mogk, A., Bukau, B., and Kampinga, H. H. (2018). Cellular handling of protein aggregates by disaggregation machines. Mol. Cell 69, 214–226. doi: 10.1016/j.molcel.2018.01.004
Molina-Quiroz, R. C., Loyola, D. E., Díaz-Vásquez, W. A., Arenas, F. A., Urzúa, U., Pérez-Donoso, J. M., et al. (2014). Global transcriptomic analysis uncovers a switch to anaerobic metabolism in tellurite-exposed Escherichia coli. Res. Microbiol. 165, 566–570. doi: 10.1016/j.resmic.2014.07.003
Morales, E. H., Pinto, C. A., Luraschi, R., Muñoz-Villagrán, C. M., Cornejo, F. A., Simpkins, S. W., et al. (2017). Accumulation of heme biosynthesis intermediates contributes to the antibacterial action of the metalloid tellurite. Nat. Commun. 8:15320. doi: 10.1038/ncomms15320
Müller, P., Ewers, C., Bertsche, U., Anstett, M., Kallis, T., Breukink, E., et al. (2007). The essential cell division protein FtsN interacts with the murein (peptidoglycan) synthase PBP1B in Escherichia coli. J. Biol. Chem. 282, 36394–36402. doi: 10.1074/jbc.M706390200
Muñoz-Villagrán, C., Contreras, F., Cornejo, F., Figueroa, M., Valenzuela-Bezanilla, D., Luraschi, R., et al. (2020). Understanding gold toxicity in aerobically-grown Escherichia coli. Biol. Res. 53:26. doi: 10.1186/s40659-020-00292-5
Nedialkova, D. D., and Leidel, S. A. (2015). Optimization of codon translation rates via tRNA modifications maintains proteome integrity. Cells 161, 1606–1618. doi: 10.1016/j.cell.2015.05.022
Nelson, D. L., and Cox, M. M. (2017). Lehninger: Principles of biochemistry (7th ed.). Hamilton, NJ. American Metro Center.
Nies, D. H. (1999). Microbial heavy-metal resistance. Appl. Microbiol. Biotechnol. 51, 730–750. doi: 10.1007/s002530051457
Noreen, A., Rehman, A., Aftab, S., and Shakoori, A. R. (2019). Antiproliferative effect of oxidative stress induced by tellurite in breast carcinoma cells. J. Cancer Res. Prac. 6, 68–75. doi: 10.4103/JCRP.JCRP_5_19
Nunoshiba, T., Obata, F., Boss, A. C., Oikawa, S., Mori, T., Kawanishi, S., et al. (1999). Role of iron and superoxide for generation of hydroxyl radical, oxidative DNA lesions, and mutagenesis in Escherichia coli. J. Biol. Chem. 274, 34832–34837. doi: 10.1074/jbc.274.49.34832
Otaru, N., Ye, K., Mujezinovic, D., Berchtold, L., Constancias, F., Cornejo, F. A., et al. (2021). GABA production by human intestinal Bacteroides spp.: prevalence, regulation, and role in acid stress tolerance. Front. Microbiol. 12:656895. doi: 10.3389/fmicb.2021.656895
Otsuka, Y., Muto, A., Takeuchi, R., Okada, C., Ishikawa, M., Nakamura, K., et al. (2015). GenoBase: comprehensive resource database of Escherichia coli K-12. Nucleic Acids Res. 43, D606–D617. doi: 10.1093/nar/gku1164
Ouyang, Y., Peng, Y., Li, J., Holmgren, A., and Lu, J. (2018). Modulation of thiol-dependent redox system by metal ions via thioredoxin and glutaredoxin systems. Metallomics 10, 218–228. doi: 10.1039/C7MT00327G
Pacheco, C. C., Passos, J. F., Castro, A. R., Moradas-Ferreira, P., and De Marco, P. (2008). Role of respiration and glutathione in cadmium-induced oxidative stress in Escherichia coli K-12. Arch. Microbiol. 189, 271–278. doi: 10.1007/s00203-007-0316-8
Paraszkiewicz, K., Bernat, P., Naliwajski, M., and Długoński, J. (2010). Lipid peroxidation in the fungus curvularia lunata exposed to nickel. Arch. Microbiol. 192, 135–141. doi: 10.1007/s00203-009-0542-3
Park, H. J., Kim, J. Y., Kim, J., Lee, J. H., Hahn, J. S., Gu, M. B., et al. (2009). Silver-ion-mediated reactive oxygen species generation affecting bactericidal activity. Water Res. 43, 1027–1032. doi: 10.1016/j.watres.2008.12.002
Parvatiyar, K., Alsabbagh, E. M., Ochsner, U. A., Stegemeyer, M. A., Smulian, A. G., Hwang, S. H., et al. (2005). Global analysis of cellular factors and responses involved in Pseudomonas aeruginosa resistance to arsenite. J. Bacteriol. 187, 4853–4864. doi: 10.1128/JB.187.14.4853-4864.2005
Peana, M., Pelucelli, A., Medici, S., Cappai, R., Nurchi, V. M., and Zoroddu, M. A. (2021). Metal toxicity and speciation: a review. Curr. Med. Chem. 28, 7190–7208. doi: 10.2174/0929867328666210324161205
Pérez, J. M., Calderón, I. L., Arenas, F. A., Fuentes, D. E., Pradenas, G. A., Fuentes, E. L., et al. (2007). Bacterial toxicity of potassium tellurite: unveiling an ancient enigma. PLoS One 2:e211. doi: 10.1371/journal.pone.0000211
Piotrowski, J. S., Li, S. C., Deshpande, R., Simpkins, S. W., Nelson, J., Yashiroda, Y., et al. (2017). Functional annotation of chemical libraries across diverse biological processes. Nat. Chem. Biol. 13, 982–993. doi: 10.1038/nchembio.2436
Piotrowski, J. S., Simpkins, S. W., Li, S. C., Deshpande, R., McIlwain, S. J., Ong, I. M., et al. (2015). Chemical genomic profiling via barcode sequencing to predict compound mode of action. Methods Mol. Biol. 1263, 299–318. doi: 10.1007/978-1-4939-2269-7_23
Poetsch, A. R. (2020). The genomics of oxidative DNA damage, repair, and resulting mutagenesis. Comput. Struct. Biotechnol. J. 18, 207–219. doi: 10.1016/j.csbj.2019.12.013
Pradenas, G. A., Díaz-Vásquez, W. A., Pérez-Donoso, J. M., and Vásquez, C. C. (2013). Monounsaturated fatty acids are substrates for aldehyde generation in tellurite-exposed Escherichia coli. Biomed. Res. Int. 2013:563756. doi: 10.1155/2013/563756
Quintal, S. M., dePaula, Q. A., and Farrell, N. P. (2011). Zinc finger proteins as templates for metal ion exchange and ligand reactivity. Chemical and biological consequences. Metallomics 3, 121–139. doi: 10.1039/c0mt00070a
Rajanna, B., Hobson, M., Harris, L., Ware, L., and Chetty, C. S. (1990). Effects of cadmium and mercury on Na+-K+ ATPase and uptake of 3H-dopamine in rat brain synaptosomes. Arch. Int. Phys. Bioch. 98, 291–296. doi: 10.3109/13813459009113989
Robinson, M. D., McCarthy, D. J., and Smyth, G. K. (2010). edgeR: a bioconductor package for differential expression analysis of digital gene expression data. Bioinformatics 26, 139–140. doi: 10.1093/bioinformatics/btp616
Rosen, R., Biran, D., Gur, E., Becher, D., Hecker, M., and Ron, E. Z. (2002). Protein aggregation in Escherichia coli: role of proteases. FEMS Microbiol. Lett. 207, 9–12. doi: 10.1111/j.1574-6968.2002.tb11020.x
Sharma, S. K., Goloubinoff, P., and Christen, P. (2008). Heavy metal ions are potent inhibitors of protein folding. Biochem. Biophys. Res. Commun. 372, 341–345. doi: 10.1016/j.bbrc.2008.05.052
Shizuta, Y., Nakazawa, A., Tokushige, M., and Hayaishi, O. (1969). Studies on the interaction between regulatory enzymes and effectors. III. Crystallization and characterization of adenosine 5′-monophosphate-dependent threonine deaminase from Escherichia coli. J. Biol. Chem. 244, 1883–1889. doi: 10.1016/S0021-9258(18)91763-1
Sies, H., Berndt, C., and Jones, D. P. (2017). Oxidative stress. Annu. Rev. Biochem. 86, 715–748. doi: 10.1146/annurev-biochem-061516-045037
Simpkins, S. W., Deshpande, R., Nelson, J., Li, S. C., Piotrowski, J. S., Ward, H. N., et al. (2019). Using BEAN-counter to quantify genetic interactions from multiplexed barcode sequencing experiments. Nat. Protoc. 14, 415–440. doi: 10.1038/s41596-018-0099-1
Śmigiel, W. M., Mantovanelli, L., Linnik, D. S., Punter, M., Silberberg, J., Xiang, L., et al. (2022). Protein diffusion in Escherichia coli cytoplasm scales with the mass of the complexes and is location dependent. Sci. Adv. 8:eabo5387. doi: 10.1126/sciadv.abo5387
Stadtman, E. R., and Levine, R. L. (2003). Free radical-mediated oxidation of free amino acids and amino acid residues in proteins. Amino Acids 25, 207–218. doi: 10.1007/s00726-003-0011-2
Steinfeld, J. B., Aerni, H. R., Rogulina, S., Liu, Y., and Rinehart, J. (2014). Expanded cellular amino acid pools containing phosphoserine, phosphothreonine, and phosphotyrosine. ACS Chem. Biol. 9, 1104–1112. doi: 10.1021/cb5000532
Su, H. S., Lang, B. F., and Newman, E. B. (1989). L-serine degradation in Escherichia coli K-12: cloning and sequencing of the sdaA gene. J. Bacteriol. 171, 5095–5102. doi: 10.1128/jb.171.9.5095-5102.1989
Su, H., and Newman, E. B. (1991). A novel L-serine deaminase activity in Escherichia coli K-12. J. Bacteriol. 173, 2473–2480. doi: 10.1128/jb.173.8.2473-2480.1991
Sugawara, K., Toyoda, H., Kimura, M., Hayasaka, S., Saito, H., Kobayashi, H., et al. (2021). Loss of cell wall integrity genes cpxA and mrcB causes flocculation in Escherichia coli. Biochem. J. 478, 41–59. doi: 10.1042/BCJ20200723
Tadokoro, A., Hayashi, H., Kishimoto, T., Makino, Y., Fujisaki, S., and Nishimura, Y. (2004). Interacción of the Escherichia coli lipoportein NlpI with periplasmic Prc (tsp) protease. J. Biochem. 135, 185–191. doi: 10.1093/jb/mvh022
Tazuya-Murayama, K., Aramaki, H., Mishima, M., Saito, K., Ishida, S., and Yamada, K. (2006). Effect of L-serine on the biosynthesis of aromatic amino acids in Escherichia coli. J. Nutr. Sci. Vitaminol. 52, 256–260. doi: 10.3177/jnsv.52.256
Tchounwou, P. B., Yedjou, C. G., Patlolla, A. K., and Sutton, D. J. (2012). Heavy metal toxicity and the environment. EXS 101, 133–164. doi: 10.1007/978-3-7643-8340-4_6
Thorgersen, M. P., Xue, J., Majumder, E., Trotter, V. V., Ge, X., Poole, F., et al. (2021). Deciphering microbial metal toxicity responses via random bar code transposon site sequencing and activity-based metabolomics. Appl. Environ. Microbiol. 87:e0103721. doi: 10.1128/AEM.01037-21
Tomoyasu, T., Mogk, A., Langen, H., Goloubinoff, P., and Bukau, B. (2001). Genetic dissection of the roles of chaperones and proteases in protein folding and degradation in the Escherichia coli cytosol. Mol. Microbiol. 40, 397–413. doi: 10.1046/j.1365-2958.2001.02383.x
Traxler, M. F., Summers, S. M., Nguyen, H. T., Zacharia, V. M., Hightower, G. A., Smith, J. T., et al. (2008). The global, ppGpp-mediated stringent response to amino acid starvation in Escherichia coli. Mol. Microbiol. 68, 1128–1148. doi: 10.1111/j.1365-2958.2008.06229.x
Tremaroli, V., Fedi, S., and Zannoni, D. (2007). Evidence for a tellurite-dependent generation of reactive oxygen species and absence of a tellurite-mediated adaptive response to oxidative stress in cells of Pseudomonas pseudoalcaligenes KF707. Arch. Microbiol. 187, 127–135. doi: 10.1007/s00203-006-0179-4
Tyedmers, J., Mogk, A., and Bukau, B. (2010). Cellular strategies for controlling protein aggregation. Nat. Rev. Mol. Cell Biol. 11, 777–788. doi: 10.1038/nrm2993
Valko, M., Morris, H., and Cronin, M. T. (2005). Metals, toxicity and oxidative stress. Curr. Med. Chem. 12, 1161–1208. doi: 10.2174/0929867053764635
Vera, A., Arís, A., Carrió, M., González-Montalbán, N., and Villaverde, A. (2005). Lon and ClpP proteases participate in the physiological disintegration of bacterial inclusion bodies. J. Biotechnol. 119, 163–171. doi: 10.1016/j.jbiotec.2005.04.006
Wang, A., and Crowley, D. E. (2005). Global gene expression responses to cadmium toxicity in Escherichia coli. J. Bacteriol. 187, 3259–3266. doi: 10.1128/JB.187.9.3259-3266.2005
Wang, B., Grant, R. A., and Laub, M. T. (2020). ppGpp coordinates nucleotide and amino-acid synthesis in E. coli during starvation. Mol. Cell 80, 29–42.e10. doi: 10.1016/j.molcel.2020.08.005
Weids, A. J., Ibstedt, S., Tamás, M. J., and Grant, C. M. (2016). Distinct stress conditions result in aggregation of proteins with similar properties. Sci. Rep. 6: 24554.
Wilken, C., Kitzing, K., Kurzbauer, R., Ehrmann, M., and Clausen, T. (2004). Crystal structure of the DegS stress sensor: how a PDZ domain recognizes misfolded protein and activates a protease. Cells 117, 483–494. doi: 10.1016/S0092-8674(04)00454-4
Williams, K. J. (2009). The introduction of chemotherapy’using arsphenamine the first magic bullet. J. R. Soc. Med. 102, 343–348. doi: 10.1258/jrsm.2009.09k036
Xiao, M., Zhu, X., Fan, F., Xu, H., Tang, J., Qin, Y., et al. (2017). Osmotolerance in Escherichia coli is improved by activation of copper efflux genes or supplementation with sulfur-containing amino acids. Appl. Environ. Microbiol. 83, e03050–e03016. doi: 10.1128/AEM.03050-16
Xu, F. F., and Imlay, J. A. (2012). Silver(I), mercury(II), cadmium(II), and zinc(II) target exposed enzymic iron-sulfur clusters when they toxify Escherichia coli. Appl. Environ. Microbiol. 78, 3614–3621. doi: 10.1128/AEM.07368-11
Zarei, M. H., Pourahmad, J., and Nassireslami, E. (2019). Toxicity of arsenic on isolated human lymphocytes: the key role of cytokines and intracellular calcium enhancement in arsenic-induced cell death. Main Group Met. Chem. 42, 125–134. doi: 10.1515/mgmc-2019-0014
Zhang, X., El-Hajj, Z. W., and Newman, E. (2010). Deficiency in L-serine deaminase interferes with one-carbon metabolism and cell wall synthesis in Escherichia coli K-12. J. Bacteriol. 192, 5515–5525. doi: 10.1128/JB.00748-10
Zhang, X., and Newman, E. (2008). Deficiency in l-serine deaminase results in abnormal growth and cell division of Escherichia coli K-12. Mol. Microbiol. 69, 870–881. doi: 10.1111/j.1365-2958.2008.06315.x
Keywords: Escherichia coli, soft-metal(loid)s, anaerobiosis, protein aggregation, proteotoxicity, amino acid metabolism
Citation: Cornejo FA, Muñoz-Villagrán C, Luraschi RA, Sandoval-Díaz MP, Cancino CA, Pugin B, Morales EH, Piotrowski JS, Sandoval JM, Vásquez CC and Arenas FA (2023) Soft-metal(loid)s induce protein aggregation in Escherichia coli. Front. Microbiol. 14:1281058. doi: 10.3389/fmicb.2023.1281058
Edited by:
Jose Echenique, National University of Cordoba, ArgentinaReviewed by:
Jan-Ulrik Dahl, Illinois State University, United StatesMarkus J. Tamás, University of Gothenburg, Sweden
Copyright © 2023 Cornejo, Muñoz-Villagrán, Luraschi, Sandoval-Díaz, Cancino, Pugin, Morales, Piotrowski, Sandoval, Vásquez and Arenas. This is an open-access article distributed under the terms of the Creative Commons Attribution License (CC BY). The use, distribution or reproduction in other forums is permitted, provided the original author(s) and the copyright owner(s) are credited and that the original publication in this journal is cited, in accordance with accepted academic practice. No use, distribution or reproduction is permitted which does not comply with these terms.
*Correspondence: Felipe A. Arenas, ZmVsaXBlLmFyZW5hc3NAdXNhY2guY2w=
†Deceased
‡Present address: Fabián A. Cornejo, Max Planck Unit for the Science of Pathogens, Berlin, Germany