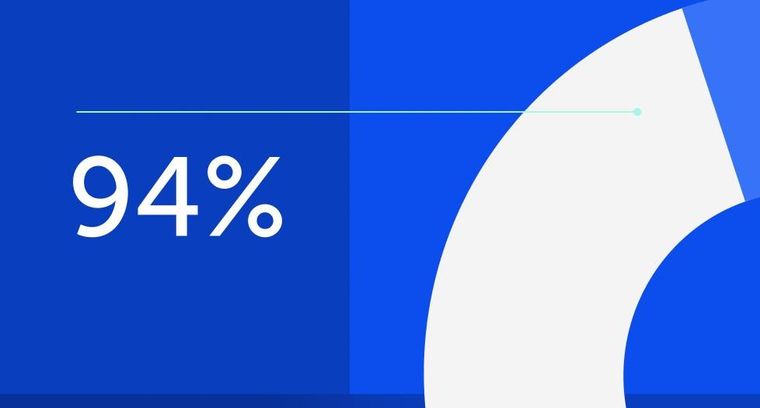
94% of researchers rate our articles as excellent or good
Learn more about the work of our research integrity team to safeguard the quality of each article we publish.
Find out more
ORIGINAL RESEARCH article
Front. Microbiol., 31 October 2023
Sec. Phage Biology
Volume 14 - 2023 | https://doi.org/10.3389/fmicb.2023.1278791
This article is part of the Research TopicBacteriophage and Host InteractionsView all 15 articles
Bacteriophages such as γ and AP50c have been shown to infect strains of Bacillus anthracis with high specificity, and this feature has been exploited in the development of bacterial detection assays. To better understand the emergence of phage resistance, and thus the potential failure of such assays, it is important to identify the host and phage receptors necessary for attachment and entry. Using genetic approaches, the bacterial receptors of AP50c and γ have been identified as sap and GamR, respectively. A second AP50c-like phage, Wip1, also appears to use sap as a receptor. In parallel with this work, the cognate phage-encoded receptor binding proteins (RBPs) have also been identified (Gp14 for γ, P28 for AP50c, and P23 for Wip1); however, the strength of evidence supporting these protein–protein interactions varies, necessitating additional investigation. Here, we present genetic evidence further supporting the interaction between sap and the RBPs of AP50c and Wip1 using fluorescently tagged proteins and a panel of B. anthracis mutants. These results showed that the deletion of the sap gene, as well as the deletion of csaB, whose encoded protein anchors sap to the bacterial S-layer, resulted in the loss of RBP binding. Binding could then be rescued by expressing these genes in trans. We also found that the RBP of the γ-like prophage λBa03 relied on csaB activity for binding, possibly by a different mechanism. RBPλBa03 binding to B. anthracis cells was also unique in that it was not ablated by heat inactivation of vegetative cells, suggesting that its receptor is still functional following incubation at 98°C. These results extend our understanding of the diverse attachment and entry strategies used by B. anthracis phages, enabling future assay development.
Bacillus anthracis, the causative agent of anthrax, is a spore-forming, Gram-positive bacterium that qualifies as a CDC Tier 1 select agent due to its potential use as a bioterrorism agent (Darling et al., 2002). Due to its impact on national biodefense, it is vital to have surveillance strategies in place that are rapid, reliable, and can be performed with limited equipment. (Bacterio)phages that specifically infect B. anthracis represent an appealing set of tools towards this end (Abshire et al., 2005; Sozhamannan et al., 2008; Schuch et al., 2010). Indeed, an engineered lux fusion reporter phage has been developed and tested for rapid detection of viable B. anthracis spores in environmental samples (Sharp et al., 2016). In addition to their potential utility in bacterial identification, there has also been a recent resurgence in use of phages as therapeutics due to the growing threat of multi-drug resistant bacteria (Aranaga et al., 2022). With all of these potential applications, it is critical to understand the genetic mechanisms driving phage resistance, allowing for the intelligent design of both therapeutic phage cocktails and diagnostic/surveillance tools.
An important step towards deciphering these resistance mechanisms is identifying phage receptor binding proteins (RBP) and their cognate bacterial surface receptor(s). The specificity of a phage for its target bacteria is attributed, at least in part, to the strength of this interaction, and receptors can be any of a diverse set of molecules, including proteins, (lipo)polysaccharides, and carbohydrate moieties (Rakhuba et al., 2010; Bertozzi Silva et al., 2016; Hyman and van Raaij, 2018). With respect to B. anthracis-specific phages, the bacterial protein GamR was identified as the bacterial receptor of phage γ almost two decades ago using genetic approaches (Davison et al., 2005). Identification of the bacterial receptors of other phages, including AP50c and Wip1, came later. These efforts started with whole genome sequence analysis of spontaneous AP50c-resistant B. anthracis mutants. Here, mutations in the csaB gene were found to result in phage resistance (Bishop-Lilly et al., 2012). As csaB is known to anchor the surface-array protein (sap) to the bacterial S-layer present on the B. anthracis vegetative cell surface (Mignot et al., 2002), it was postulated that sap, more appropriately the S-layer, could be the receptor for AP50c (Bishop-Lilly et al., 2012). S-layer, which itself provides structural integrity and stability to the cell wall (Fioravanti et al., 2022), was also hypothesized to be the bacterial receptor of Wip1 due to its genetic similarity to AP50c (Kan et al., 2013). More direct evidence in support of the AP50c-sap binding hypothesis was produced when incubation of purified sap protein with intact AP50c phage particles reduced the titer of free phage (Plaut et al., 2014). Ultimately, analysis of various transposon insertions and targeted mutants revealed that not only sap and csaB, but also the sporulation genes spo0A, spo0B, and spo0F all had roles in productive AP50c infection of B. anthracis cells (Plaut et al., 2014). While the direct roles of sap and csaB in the formation of the S-layer are clear, whether the sporulation genes play a direct or indirect role in either S-layer formation or phage attachment and infection is not clear at this time.
As the potential bacterial receptors of these phage were put forth, research was also conducted to identify their respective phage receptors. The RBP of Wip1 was identified as P23 (henceforth referred to as RBPWip1) based on phage adsorption tests and immunofluorescence assays (Kan et al., 2013). The activity of P23 was found to require the translation of the downstream p24 gene (Kan et al., 2013), which shares extensive sequence similarity to p29 gene of AP50c (Braun et al., 2020). Based on its location immediately upstream of p29, along with a short amino acid sequence identity to the Wip1 P23 protein, the AP50c protein P28 (RBPAP50c) was then posited as its RBP (Braun et al., 2020). When the Wip1 p23 and AP50c p28 genes were then cloned into expression vectors with fluorescent protein genes, the resulting fusion proteins were shown to bind to B. anthracis cell surfaces (Braun et al., 2020). Together, this work supported the role of P23 and P28 in phage binding but did not establish their interaction with sap. Here, we describe a set of genetic and molecular experiments to further probe these phage-bacterial interactions and provide evidence that presence of sap, csaB, and the sporulation proteins Spo0A, Spo0B, and Spo0F are likely required for RBPWip1 and RBPAP50c binding to sap on B. anthracis cell surface.
In addition to prototypical phages, there have also been prophages identified in the chromosome of B. anthracis, including the γ-like prophage λBa03 (Sozhamannan et al., 2006). The genome of λBa03 encodes the hypothetical protein BA4079 (henceforth referred to as RBPλBa03) that shares significant amino acid sequence homology to γ phage encoded RBP Gp14, making it an ideal candidate for λBa03 RBP (Braun et al., 2020). Like RBPAP50c and RBPWip1, a domain of RBPλBa03 lacking the first 120 amino acids (designated as RBPλ03Δ1-120) has been shown to bind to the cell surface of vegetative B. anthracis bacterium, but little else is known about its bacterial target for binding. Here, we find that λBa03 represents a different paradigm than Wip1 and AP50c in that it requires csaB activity for binding, but that this phenotype appears to be unrelated to its role in anchoring sap to the S-layer. Intriguingly, RBPλ03Δ1-120 binding to B. anthracis also is maintained following heat treatment, meaning its receptor is not heat labile or protected from heat inactivation. These results underscore the significant diversity in strategies adopted by these phages and corresponding genetic requirements for attachment and entry during infection of their host bacteria.
B. anthracis and Escherichia coli strains, plasmid vectors and recombinant plasmids used in this study are listed in Table 1. Construction of the in-frame mutant B. anthracis strains used in this study is originally described in Plaut et al. (2014).
Glycerol stocks of bacteria were used to inoculate 50 mL of tryptic soy broth (TSB). The cultures were grown overnight at 37°C in 250 mL flasks at 150 rotations per minute (rpm). In experiments using heat-killed cells, a 1 mL aliquot was taken from these cultures, diluted to an optical density (OD600) of 1.0 and incubated at 98°C for 30 min. Serial dilutions of cells were plated on tryptic soy agar (TSA) to verify non-viability.
Spores were produced as described in Braun et al. (2020). Briefly, a colony from a fresh overnight TSA plate was inoculated in 50 mL of sporulation medium containing 0.8% nutrient broth supplemented with 0.05 mM MnCl2, 0.7 mM CaCl2, and 1 mM MgCl2 in a 250 mL flask. After cultivation for 72 h at 37°C with shaking at 150 rpm, 3% Tween-80 was added to each flask and incubated for an additional 24 h under the same conditions. Samples were harvested in a 50 mL conical tube by centrifugation at 2000 × g for 10 min. The supernatant was discarded and the pellet was washed twice with 3% Tween-80. Samples were resuspended in 25 mL of 3% Tween-80 and incubated at 37°C for 24 h with shaking at 150 rpm. Phase contrast microscopy was used to approximate the percentage of spores versus vegetative cells in each suspension. When the spore percentage reached >95%, they were harvested by centrifugation at 2000 × g for 10 min, the supernatant was discarded, and the pellet was resuspended in 3 mL of ice-cold ultrapure water and stored at 4°C. The final purity of a given spore preparation was determined by (i) documenting the size and light refractivity by microscopy and (ii) measuring viability following the heating process described above.
Isolation of DNA from bacterial cultures was performed using the GeneJET Plasmid Miniprep kit (ThermoFisher Scientific) K0502 or the Nanobind High Molecular Weight Extraction kit (Circulomics 102-762-700) according to manufacturer’s recommendations. Extracted nucleic acids were quantified using either the Qubit dsDNA HS Assay (ThermoFisher Scientific Q32851) or the High Sensitivity DNA ScreenTape (Agilent 5,067–5,584).
Whole genome sequencing on the Oxford Nanopore and Illumina platforms and downstream assembly and alignment, were performed as described in Sholes et al. (2023). Single nucleotide polymorphisms (SNPs) and small insertions or deletions were called and filtered as described in Forde et al. (2022). In brief, VarScan v2.4.4 was used with a minimum read depth of 4x, a minimum base quality of 20, and a variant allele frequency ≥ 0.95 (Koboldt et al., 2012). Large structural variations were inspected using sniffles v2.0 (Sedlazeck et al., 2018). All variants were visually validated using IGV (Thorvaldsdóttir et al., 2013).
The pASG-105-TST-eGFP-RBP plasmids expressing the receptor binding proteins of phages Wip1 and AP50C and the soluble domain of prophage λBa03 described in Braun et al. (2020) were used as the basis for construction of the new expression constructs used herein. The sequences for the RBPs and corresponding chaperone proteins (when applicable) and for eGFP were provided kindly by Gregor Grass. Insert sequence synthesis, cloning, and sequence validation were performed by Azenta (Burlington, MA). Briefly, an oligonucleotide encoding a start codon and N-terminal HISTAG-thrombin site-Twin-Strep tag was synthesized upstream of the eGFP sequence. A short -CTCGAG- linker sequence was added, followed immediately by the phage RBP sequence. For those RBPs requiring the co-expression of a second protein (RBPAP50c and RBPWip1), the linker sequence -AAGGAGGGAACTAT- was added, followed by the full coding sequence of respective gene. These inserts were cloned into plasmid pET22 using NdeI and HindIII restriction sites.
Protein expression and purification were performed by Olympic Protein Technologies (Seattle, WA). The pET22-eGFP-RBP expression vectors were used to transform (i) E. coli Arctic Express DE3 cells (Agilent 230,192) for protein production and (ii) E. coli TOP10 (Thermo Fisher C404010) cells for plasmid stock production. Cultures were grown on LB-carbenicillin (100 μg/mL) plates at 37°C overnight prior to single colony selection, outgrowth in liquid culture, and sequence verification using the pET22 entry primers T7 (TAATACGACTCACTATAGGG) and T7 term (GCTAGTTATTGCTCAGCGG).
For protein production, colonies were grown in 25 mL Terrific Broth (TB) with 20 μg/mL gentamicin overnight at 30°C (250 rpm). One liter of autoinduction medium (MagicMedia™ Thermo K6803) was inoculated with 20 mL of the overnight culture and grown at 30°C for 4 hrs before the temperature of the shaken cultures was shifted to 12°C and allowed to proceed overnight. To test for expression, cell pellets were harvested by centrifugation at 10,000 rpm for 10 min at 4°C and lysed using an equal volume of BugbusterHT+ (Millipore Sigma 70,922) and fractionated into soluble and insoluble material. Insoluble fractions were taken up in equal volume as the original culture. All samples were run on a 4–20% Tris-glycine reducing SDS-PAGE gel to ascertain the presence of the tagged protein in the soluble fraction.
For purification, separate cell pellets were resuspended in IMAC breaking buffer (25 mM Tris–HCl, pH 7.9, 500 mM NaCl, 2 mM imidazole, with a complete protease inhibitor tablet and benzonase). The suspensions were lysed via three passages through a microfluidizer. Lysates were centrifuged as above, and, the cleared supernatants were passed through a 0.45 μm filter, and frozen at −80°C.
Thawed lysates were loaded on to a 5 mL Nickel Excel IMAC column (Cytiva) previously equilibrated with IMAC running buffer (50 mM Tris–HCl, pH 8.0, 300 mM NaCl, 2 mM imidazole) using an AKTA instrument. The immobilized proteins were then washed with IMAC running buffer followed by running buffer containing 12 mM imidazole. Once baseline Abs280 readings were reached, the bound proteins were eluted using IMAC elution buffer (50 mM Tris–HCl, pH 8.0, 300 mM NaCl, 250 mM imidazole), and peak protein fractions were collected and pooled by Abs280 measurements.
Pooled IMAC fractions were adjusted to 10 mM CaCl2, and thrombin (Sigma 69,671) was added to a final concentration of 0.24 U/mg of protein. Thrombin reactions were carried out at room temperature for 3 h under conditions of slow rotation. The reaction products were loaded at 3 mL/min on to a 5 mL Strep-Tactin XL Superflow high-capacity column (IBA) previously equilibrated with buffer W (100 mM Tris, pH 8.0, 150 mM NaCl, 1 mM EDTA). Bound samples were washed with buffer W until Abs280 measurements reached a steady baseline value. Proteins were eluted using buffer BXT (100 mM Tris, pH 8.0, 150 mM NaCl, 1 mM EDTA, 50 mM biotin). As above, protein peaks were measured by Abs280 values and pooled. Overall sample purities were quantified by running a 15 μL aliquot run on an HPLC-SEC column (Tosoh G3000SW) in SEC running buffer (1X PBS) at 200 μL/min. Eluents were run on 4–20% Tris-glycine SDS-PAGE gels (ThermoFisher).
A 100 μL aliquot of living or heat-killed vegetative cells (OD600 = 1) was added to a 1.5 mL microcentrifuge tube and centrifuged at 5000 × g for 2 min. For spore preparations, 2 × 106 spores were added to 10 mL of Ringer-HEPES buffer (50 mM HEPES, 1.5 mM CaCl2, 1.5 mM KCl, 100 mM NaCl, 0.6 mM NaHCO3, pH 7.4) and centrifuged at 5000 × g for 2 min. After the supernatant was removed, the pellet was resuspended in 1 mL of Ringer-HEPES buffer then transferred to a 1.5 mL microcentrifuge tube and centrifuged again under the same conditions. The supernatant was removed and the pellet was resuspended in 100 μL of Ringer-HEPES buffer and 5 μg of purified RBP fusion protein was added to the sample. Samples were incubated at room temperature for 5 min then centrifuged and resuspended in 100 μL of Ringer-HEPES buffer. A 3 μL aliquot of the sample was added to a glass microscope slide and imaged by both brightfield and FITC filter. All fluorescence images of samples containing the same phage protein were processed in the same way, with contrast, brightness, and exposure times kept constant. All microscopy images in this manuscript were taken at 100X under oil immersion.
The starting plasmid for the cloning of B. anthracis genes was the E. coli-B. anthracis shuttle vector, pSW4, the construction of which was previously described (Pomerantsev et al., 2003). The coding sequences of the bacterial genes of interest were individually synthesized, inserted into pSW4 linearized using BglII and BlpI, and sequence verified by Azenta Life Sciences (Burlington, MA). Constructs were then transformed into competent dam−/dcm− E. coli cells (NEB C2925H) and purified to yield unmethylated plasmid stocks.
To make electrocompetent cells, B. anthracis wild type or mutant strains were grown overnight on LB plates containing 1% w/v glucose (LBG agar). Colonies were inoculated into 1 mL of LBG medium and incubated for 10 min at 37°C and 225 rpm before being spiked into a larger 25 mL LBG culture. The culture was incubated and shaken until the OD600 reached 0.15–0.25. Cells were centrifuged at 4000 × g for 5 min and washed three times with electroporation buffer (10% sucrose, 15% glycerol, 2 mM potassium phosphate buffer, pH 8.0–8.4). Cells were resuspended in 200 μL of electroporation buffer and transferred to a 0.2 cm electroporation cuvette. After incubation on ice for 10 min, approximately 0.5 μg of unmethylated plasmid was added into the cuvette and mixed gently with the cells. Cells were pulsed once at 1.77 kV with a mean time constant between 3.5–5 msec, then 1 mL of S.O.C medium was added to the cells. Cells were transferred to a FALCON 14 mL tube and incubated at 37°C for 1 h. A 200 μL aliquot was plated on LBG-kanamycin plates and incubated for 16 h at 37°C.
A transposon insertion screen previously identified several genes in B. anthracis Sterne 7702 that appeared to be necessary for AP50c phage adsorption (Plaut et al., 2014). These included sap, spo0A, and spo0F (discussed in the Introduction section), as well genes encoding the transcriptional regulator BAS0566 and the branched amino-acid ABC transporter BAS1792. Each of these genes, as well as two genes in the same operon as sap, (csaB and eag, the latter of which is an alternate S-layer protein), were then individually deleted using markerless allelic exchange, and a final mutant strain was created that lacked the additional sporulation gene spo0B (Plaut et al., 2014). Recently, the full sequences of the genomes of these strains were published (Sholes et al., 2023). We found that each of these mutant strains contained the expected deletions of the targeted genes (Figure 1), in addition to individual SNPs or deletions present elsewhere in the genome (Supplementary Table S1). The BAP350 mutant (ΔcsaB) also contained two additional deletions in genes BAS26725 and BAS26720 (Figure 1, bottom block). All these “hitch-hiker” mutations were taken into consideration when examining the results of RBP binding experiments described below.
Figure 1. Genomic maps of wild type and mutant strains of B. anthracis Sterne 7702 indicating the presence of deletions in different locations. The top black bar represents the bacterial chromosome with the relative locations of the genes targeted for deletion in the mutants. The bottom blocks zoom in on the individual regions of each deletion with genome positions provided on top, relevant open reading frames in the middle, and sequence coverage of respective locus with flanking regions on the bottom. The mutant bars are color coded to match with the genes indicated on the whole chromosome map on top. Black boxes are to aid in visual differentiation of different strains. The secondary, off-target hitch-hiker deletions in the BAP350 strain (ORFs BAS26725 and BAS26720) are indicated in the bottom-most box.
Earlier work by Braun et al. described the identification, cloning, expression, and purification of the putative receptor binding proteins (RBPs) of phages Wip1 (P23), AP50c (P28), γ (Gp14) and a soluble domain of λBa03 (BA4079 Δ1-120) (Braun et al., 2020). Here, we expanded on this work and developed a plasmid system in which each RBP could be expressed with GFP fused to the N-terminus. These constructs additionally contained a His-thrombin site and Twin-Strep-tag epitope to aid in purification. For the plasmids expressing the Wip1 or AP50c RBP-GFP fusion proteins, a second protein encoded immediately downstream in the phage genome was co-expressed based on previous results suggesting these secondary proteins were required for RBP function (Braun et al., 2020). Proteins were produced in Arctic Express E. coli cells and following autoinduction, lysates were separated into soluble and insoluble fractions. As all proteins of interest were soluble, these samples were fractionated using immobilized nickel affinity chromatography columns. The purity of each fraction was measured by Abs280 and size exclusion chromatography. Final preparations were visualized on a 4–20% Tris-glycine gel (Figure 2). These proteins are henceforth referred to as RBPWip1-GFP, RBPAP50c-GFP, and RBPλ03Δ1-120-GFP.
Figure 2. Production and purification of the three GFP-tagged phage receptor binding proteins and the untagged sap∆SLH domain (not used in current study). Five microgram aliquots of the final preparations of each purified product were run on a denaturing 4–20% Tris-glycine gel and imaged using a LI-COR instrument (LI-COR Biosciences). The <15 kDa bands in the RBPAP50c-GFP (P28) and RBPWip1-GFP (P23) lanes represent the co-expressed chaperone proteins P29 and P24, respectively.
The protein preparations containing the recombinant, tagged phage RBPs were next tested for binding against the panel of sequence-verified mutant strains described in Figure 1. As previous work suggested that phage RBP binding (i) is dependent on the growth phase of the bacteria and (ii) is strong for RBPWip1, RBPAP50c, and RBPλ03Δ1-120 during logarithmic growth (Braun et al., 2020), all experiments were performed using cells harvested during this period. Cells were not otherwise synchronized. As expected, all three phage RBPs bound to wild type Sterne strain 7702, as well as to mutants lacking expression of BAS0566 (BA749), eag (BA751), and BAS1792 (BA752) (Figure 3). In support of earlier work with whole phage AP50c (Plaut et al., 2014), the deletion of sap, csaB, or any one of the three sporulation genes spo0A, spo0B, or spo0F also resulted in the loss of RBPAP50c-GFP binding. The same binding profile was observed with the RBPWip1-GFP. Interestingly, the putative RBPλ03Δ1-120-GFP bound to all mutant strains with the exception of BAP350, which lacks csaB.
Figure 3. Representative fluorescence and brightfield images showing binding of RBPAP50c-GFP, RBPWip1-GFP, and RBPλ03Δ1-120-GFP to cultures of vegetative B. anthracis Sterne mutants. All slides were prepared using cultures harvested at OD600 = 1.0 following a five-minute incubation with 5 μg of purified phage proteins. Note the filamentous morphology of the BAP350 strain lacking csaB.
We next looked to determine whether the binding of the phage RBPs would be affected by heating of the bacterial culture. Aliquots of mid-log cultures were heated for 30 min at 98°C and plated to verify inactivation prior to incubation with each tagged phage RBP. This process resulted in the loss of RBPAP50c-GFP and RBPWip1-GFP binding to all strains (Supplementary Figure S1). The binding of RBPλ03Δ1-120-GFP to bacterial cells was not affected by heating, with the exception of the csaB mutant.
As B. anthracis cells show significant changes in surface protein expression following sporulation when compared to vegetative cells (Chateau et al., 2020), we were interested to determine whether the bacterial receptors for the RBPs were present on spore surfaces. To this end, tagged RBPs were incubated with spores prepared from each mutant strain. Of those strains that successfully sporulated, no phage RBP binding was observed (Supplementary Figure S2). A summary of the binding profiles for each RBP to each mutant strain under all three conditions can be seen in Table 2.
Table 2. Binding phenotypes observed for each of the B. anthracis Sterne mutant cultures when incubated with RBPs-GFP fusion proteins.
The only mutant strain that resulted in the loss of binding for all three phage RBPs with live vegetative cells was BAP350 (∆csaB). To ensure that this phenotype was due only to this mutation and not to the two hitch-hiker mutations and/or SNPs present in this strain, we expressed csaB in trans from a complementation plasmid. The coding sequence for csaB was cloned into the pSW4 shuttle expression vector as described in the Materials and Methods (Figure 4A) and introduced into BAP350 (ΔcsaB) or the wild type 7702 strain by electroporation. The presence of the plasmid in electroporated cells was verified by PCR (Figure 4B), and binding studies were repeated as described above. Supplying csaB protein in trans in BAP350 indeed rescued wild-type binding profiles of all three RBPs (Figures 4C,D), suggesting that the phenotype seen with the csaB mutant strain in earlier experiments was due to the lack of csaB expression alone. It should additionally be noted that expression of csaB in trans also reversed the mucoid/filamentous morphology phenotype observed in BAP350. It appears that the presence of pSW4 vector by itself, is somewhat partially reverting the mucoid phenotype as observed by the fluffy nature of the culture in the tube (Supplementary Figure S3).
Figure 4. Expression of csaB in trans rescues (i) RBPAP50c-GFP and RBPWip1-GFP binding to live cultures of ∆csaB mutant strain BAP350 and (ii) RBPλ03Δ1-120-GFP binding to heat-killed cultures. (A) Plasmid map for pSW4-csaB. (B) PCR amplicon products show the presence of the expression vector/complementation plasmid in strain BAP350. Lane 1: base pair ladder. Lanes 2 and 4: Stock plasmid preparations. Lane 3 and 5: Plasmids isolated from electroporated BAP350 cultures. NTC indicates no template control. Representative fluorescent microcopy images show binding patterns of tagged phage RBPs to (C) vegetative, or (D) heat-killed preparations of BAP350 with and without csaB complementation. Small white notations in upper right-hand corner of each image indicate the presence (+) or absence (−) of binding.
In addition to the role of csaB in the binding of all 3 phage RBPs, the RBPWip1-GFP and RBPAP50c-GFP proteins also required the expression of sap and the sporulation genes spo0A, spo0B, and spo0F for successful binding (Figure 3). This had been observed previously with whole phage particles (Plaut et al., 2014). A similar set of complementation experiments was carried out for all of these mutant strains, each of which were electroporated with either the empty pSW4 vector or the appropriate complementation construct. The successful electroporation of each plasmid was verified both by PCR (Supplementary Figure S4) and by the restoration of sporulation in mutant strains (Supplementary Figure S5). In the bacteria containing the complementation plasmids expressing sap (complementation in strain BA750) (Figure 5), spo0A (BA754, Figure 6), and spo0B (DP-B-5747, Figure 7), the wild-type binding profile was restored. In experiments with the spo0F mutant (BA755), however, complementation rescued RBPAP50c-GFP binding to live vegetative cells but did not rescue the binding of the RBPWip1-GFP (Figure 8). RBPλ03Δ1-120-GFP binding was unaffected in these mutants or the corresponding complemented strains.
Figure 5. Expression of sap in trans rescues RBPAP50c-GFP and RBPWip1-GFP binding to ∆sap mutant strain BA750. Representative fluorescent microcopy images show binding patterns of tagged phage RBPs to (A) vegetative, or (B) heat-killed preparations of BA750 with and without sap complementation. Small white notations in upper right-hand corner of each image indicate the presence (+) or absence (−) of binding.
Figure 6. Expression of spo0A in trans rescues RBPAP50c-GFP and RBPWip1-GFP binding to ∆spo0A mutant strain BA754. Representative fluorescent microcopy images show binding patterns of tagged phage RBPs to (A) vegetative, or (B) heat-killed preparations of BA754 with and without spo0A complementation. Small white notations in upper right-hand corner of each image indicate the presence (+) or absence (−) of binding.
Figure 7. Expression of spo0B in trans rescues RBPAP50c-GFP and RBPWip1-GFP binding to ∆spo0B mutant strain DP-B-5747. Representative fluorescent microcopy images show binding patterns of tagged phage RBPs to (A) vegetative, or (B) heat-killed preparations of DP-B-5747with and without spo0B complementation. Small white notations in upper right-hand corner of each image indicate the presence (+) or absence (−) of binding.
Figure 8. Expression of spo0F in trans does rescue RBPAP50c-GFP binding and does not rescue RBPWip1-GFP binding to ∆spo0F mutant strain BA755. Representative fluorescent microcopy images show binding patterns of tagged phage RBPs to (A) vegetative, or (B) heat-killed preparations of BA755 with and without spo0F complementation small white notations in upper right-hand corner of each image indicate the presence (+) or absence (−) of binding.
In this study, we have investigated the genetic requirements for the binding of bacteria and phage in a B. anthracis model system. We assessed the binding of three different phage RBPs with a collection of B. anthracis mutant strains with the goal of better understanding the underlying biology driving these interactions. While there has been previous work on the binding of these 3 phage RBPs to wild type bacteria (Braun et al., 2020), two questions remained unanswered; namely, (i) is there genetic evidence for these binding pairs? and (ii) is this binding affected by mutations in a specific set of bacterial genes? These questions are critical as they relate directly to the potential for development of phage resistance and the failure of assays that rely on this binding. Here we provide experimental evidence that answers these questions.
Using a set of GFP-tagged recombinant proteins, we recapitulated earlier results derived from whole phage particle experiments. These results indicated that: (i) the RBPAP50c likely binds the bacterial receptor sap, (ii) this binding interaction requires the expression of csaB and the sporulation genes spo0A, spo0B, and spo0F, and (iii) the binding is ablated following heat inactivation. While the putative RBPWip1-GFP does not share extensive sequence similarity to that of RBPAP50c-GFP, it does contain a small region of identical amino acids at its C-terminal, and there does exist a striking similarity in the product of genes immediately downstream of the RBPs in both phage genomes (Braun et al., 2020). Our study here suggests that Wip1 likely uses the same bacterial protein, sap, as its receptor, and that csaB and the same three sporulation genes are necessary for this interaction. The role of csaB in this binding interaction is not surprising, as it has a known role in anchoring sap to the S-layer (Mesnage et al., 2000). The role of the sporulation genes, however, is less intuitive. We hypothesize that the activity of Spo0A, a major transcriptional regulator (Burbulys et al., 1991), has a role in the expression or stability of sap or the S-layer in general. This concept has been introduced in previous work (Plaut et al., 2014), and our results support an expansive role for the sporulation phosphorelay in overall cell state. The successful rescue of wild-type binding profiles, except spo0F with RBPWip1-GFP, upon complementation with the protein absent in a given mutant strain suggests that the minor additional “hitch-hiker” mutations play no role in the phenotypes seen in each of these strains. Additionally, we could find no evidence in the literature supporting the potential involvement of these genetic loci in phage binding, S-layer function, or sporulation. The anomaly with the failure of rescue of spo0F complementation with RBPWip1-GFP binding is not clear at this time.
Our results showing a lack of binding of RBPAP50c-GFP and RBPWip1-GFP to heat-inactivated vegetative cells is somewhat unexpected based on earlier published results (Braun et al., 2020). We hypothesize that this difference could be attributed to strain- and experiment-specific differences. Even in the previously published studies, binding of each RBP to heat-inactivated cells was somewhat diminished compared to wild type cells, and it varied based both on the method of inactivation and the RBP in question (Braun et al., 2020).
Similar to previous works, and unlike the results observed with RBPAP50c-GFP and RBPWip1-GFP, we found that prophage RBPλ03Δ1-120-GFPbinding was not affected by heat inactivation. However, while earlier work also indicated that csaB was not involved in the growth of phage γ, which shows high sequence homology to λBa03 (Davison et al., 2005), we find here that the absence of csaB ablated RBPλ03Δ1-120-GFP binding. The sequence similarity between phage γ and prophage λBa03 suggests that λBa03 shared the same bacterial host receptor as gamma (GamR). Our results, however, suggest that either (i) GamR is not accessible to the RBPλ03Δ1-120-GFP in the absence of csaB (but it is accessible to the whole γ phage particle). The csaB mutants are known to secrete an extracellular flocculent material and in fact, scanning electron microscopic analysis of this mutant revealed the presence of a thin coating of an extracellular material on the outer cell surface (Sozhamannan et al., 2008); it is possible that this layer prevents access of RBPλ03Δ1-120-GFP to the bacterial receptor, or (ii) GamR is not the bacterial receptor for λBa03. If the former is true, GamR may be somehow shielded from heat denaturation, while if the latter is true, it may be that the receptor for RBPλ03Δ1-120-GFP is not proteinaceous in nature, as most proteins lose secondary structure following high temperature exposure. Recent work has in fact suggested that other γ-like phages utilize sugar moieties as part of their binding process (Nakonieczna et al., 2022) and we postulate that this may be the case for λBa03. Our future studies will examine this possibility, and further probe direct protein–protein interactions between phage RBPs and bacterial receptor proteins.
The data presented in the study are deposited in GenBank, accession numbers CP110279, JAOZJJ000000000, CP110281, JAOZJK000000000, JAOZJL000000000, JAOZJM000000000, CP110283, CP110285, and CP110287.
SF: Conceptualization, Formal analysis, Investigation, Writing – review & editing. ST: Conceptualization, Formal analysis, Investigation, Writing – review & editing. SaS: Conceptualization, Formal analysis, Investigation, Writing – review & editing. SH: Conceptualization, Formal analysis, Investigation, Writing – review & editing. RP: Investigation, Writing – review & editing. KV: Project administration, Writing – review & editing. MW: Investigation, Writing – review & editing. EE: Investigation, Writing – review & editing. BN: Funding acquisition, Writing – review & editing. ShS: Conceptualization, Data curation, Formal analysis, Funding acquisition, Project administration, Writing – original draft, Writing – review & editing. SG: Conceptualization, Formal analysis, Investigation, Writing – original draft, Writing – review & editing.
The author(s) declare financial support was received for the research, authorship, and/or publication of this article. Funding for this work was provided by the Department of Defense (DoD) Joint Program Executive Office for Chemical, Biological, Radiological and Nuclear Defense (JPEO-CBRND) under NAVSEA contract number N00024-13-D-6400. The views expressed in this article are those of the authors and do not necessarily reflect the official policy or position of the JPEO-CBRND, the Departments of the Army, Navy, or Defense, nor the U.S. Government. References to non-federal entities do not constitute or imply Department of Defense or Army endorsement of any company or organization.
The authors would like to acknowledge Andrei Pomerantsev, Steve Leppla, and Gregor Grass for their kind provision of plasmids, sequences, reagents, and expertise. We also appreciate the many useful comments provided by the reviewers.
ShS was employed by Joint Research and Development, Inc. MW and EE were employed by Olympic Protein Technologies.
The remaining authors declare that the research was conducted in the absence of any commercial or financial relationships that could be construed as a potential conflict of interest.
All claims expressed in this article are solely those of the authors and do not necessarily represent those of their affiliated organizations, or those of the publisher, the editors and the reviewers. Any product that may be evaluated in this article, or claim that may be made by its manufacturer, is not guaranteed or endorsed by the publisher.
The Supplementary material for this article can be found online at: https://www.frontiersin.org/articles/10.3389/fmicb.2023.1278791/full#supplementary-material
Abshire, T. G., Brown, J. E., and Ezzell, J. W. (2005). Production and validation of the use of gamma phage for identification of Bacillus anthracis. J. Clin. Microbiol. 43, 4780–4788. doi: 10.1128/JCM.43.9.4780-4788.2005
Aranaga, C., Pantoja, L. D., Martínez, E. A., and Falco, A. (2022). Phage therapy in the era of multidrug resistance in bacteria: a systematic review. Int. J. Mol. Sci. 23:4577. doi: 10.3390/ijms23094577
Bertozzi Silva, J., Storms, Z., and Sauvageau, D. (2016). Host receptors for bacteriophage adsorption. FEMS Microbiol. Lett. 363:fnw002. doi: 10.1093/femsle/fnw002
Bishop-Lilly, K. A., Plaut, R. D., Chen, P. E., Akmal, A., Willner, K. M., Butani, A., et al. (2012). Whole genome sequencing of phage resistant Bacillus anthracis mutants reveals an essential role for cell surface anchoring protein CsaB in phage AP50c adsorption. Virol. J. 9:246. doi: 10.1186/1743-422X-9-246
Braun, P., Wolfschläger, I., Reetz, L., Bachstein, L., Jacinto, A. C., Tocantins, C., et al. (2020). Rapid microscopic detection of Bacillus anthracis by fluorescent receptor binding proteins of bacteriophages. Microorganisms 8:934. doi: 10.3390/microorganisms8060934
Burbulys, D., Trach, K. A., and Hoch, J. A. (1991). Initiation of sporulation in B. subtilis is controlled by a multicomponent phosphorelay. Cells 64, 545–552. doi: 10.1016/0092-8674(91)90238-t
Chateau, A., Van der Verren, S. E., Remaut, H., and Fioravanti, A. (2020). The Bacillus anthracis cell envelope: composition, physiological role, and clinical relevance. Microorganisms 8:1864. doi: 10.3390/microorganisms8121864
Darling, R. G., Catlett, C. L., Huebner, K. D., and Jarrett, D. G. (2002). Threats in bioterrorism. I: CDC category a agents. Emerg. Med. Clin. North Am. 20, 273–309. doi: 10.1016/s0733-8627(02)00005-6
Davison, S., Couture-Tosi, E., Candela, T., Mock, M., and Fouet, A. (2005). Identification of the Bacillus anthracis (gamma) phage receptor. J. Bacteriol. 187, 6742–6749. doi: 10.1128/JB.187.19.6742-6749.2005
Fioravanti, A., Mathelie-Guinlet, M., Dufrêne, Y. F., and Remaut, H. (2022). The Bacillus anthracis S-layer is an exoskeleton-like structure that imparts mechanical and osmotic stabilization to the cell wall. PNAS Nexus 1:pgac121. doi: 10.1093/pnasnexus/pgac121
Forde, T. L., Dennis, T. P. W., Aminu, O. R., Harvey, W. T., Hassim, A., Kiwelu, I., et al. (2022). Population genomics of Bacillus anthracis from an anthrax hyperendemic area reveals transmission processes across spatial scales and unexpected within-host diversity. Microb Genom 8:000759. doi: 10.1099/mgen.0.000759
Hyman, P., and van Raaij, M. (2018). Bacteriophage T4 long tail fiber domains. Biophys. Rev. 10, 463–471. doi: 10.1007/s12551-017-0348-5
Kan, S., Fornelos, N., Schuch, R., and Fischetti, V. A. (2013). Identification of a ligand on the Wip1 bacteriophage highly specific for a receptor on Bacillus anthracis. J. Bacteriol. 195, 4355–4364. doi: 10.1128/JB.00655-13
Koboldt, D. C., Zhang, Q., Larson, D. E., Shen, D., McLellan, M. D., Lin, L., et al. (2012). VarScan 2: somatic mutation and copy number alteration discovery in cancer by exome sequencing. Genome Res. 22, 568–576. doi: 10.1101/gr.129684.111
Mesnage, S., Fontaine, T., Mignot, T., Delepierre, M., Mock, M., and Fouet, A. (2000). Bacterial SLH domain proteins are non-covalently anchored to the cell surface via a conserved mechanism involving wall polysaccharide pyruvylation. EMBO J. 19, 4473–4484. doi: 10.1093/emboj/19.17.4473
Mignot, T., Mesnage, S., Couture-Tosi, E., Mock, M., and Fouet, A. (2002). Developmental switch of S-layer protein synthesis in Bacillus anthracis. Mol. Microbiol. 43, 1615–1627. doi: 10.1046/j.1365-2958.2002.02852.x
Nakonieczna, A., Rutyna, P., Fedorowicz, M., Kwiatek, M., Mizak, L., and Łobocka, M. (2022). Three novel bacteriophages, J5a, F16Ba, and z1a, specific for Bacillus anthracis, define a new clade of historical Wbeta phage relatives. Viruses 14:213. doi: 10.3390/v14020213
Plaut, R. D., Beaber, J. W., Zemansky, J., Kaur, A. P., George, M., Biswas, B., et al. (2014). Genetic evidence for the involvement of the S-layer protein gene sap and the sporulation genes spo0A, spo0B, and spo0F in phage AP50c infection of Bacillus anthracis. J. Bacteriol. 196, 1143–1154. doi: 10.1128/JB.00739-13
Pomerantsev, A. P., Kalnin, K. V., Osorio, M., and Leppla, S. H. (2003). Phosphatidylcholine-specific phospholipase C and sphingomyelinase activities in bacteria of the Bacillus cereus group. Infect. Immun. 71, 6591–6606. doi: 10.1128/IAI.71.11.6591-6606.2003
Rakhuba, D. V., Kolomiets, E. I., Dey, E. S., and Novik, G. I. (2010). Bacteriophage receptors, mechanisms of phage adsorption and penetration into host cell. Pol. J. Microbiol. 59, 145–155. doi: 10.33073/pjm-2010-023
Schuch, R., Pelzek, A. J., Kan, S., and Fischetti, V. A. (2010). Prevalence of Bacillus anthracis-like organisms and bacteriophages in the intestinal tract of the earthworm Eisenia fetida. Appl. Environ. Microbiol. 76, 2286–2294. doi: 10.1128/AEM.02518-09
Sedlazeck, F. J., Rescheneder, P., Smolka, M., Fang, H., Nattestad, M., von Haeseler, A., et al. (2018). Accurate detection of complex structural variations using single-molecule sequencing. Nat. Methods 15, 461–468. doi: 10.1038/s41592-018-0001-7
Sharp, N. J., Molineux, I. J., Page, M. A., and Schofield, D. A. (2016). Rapid detection of viable Bacillus anthracis spores in environmental samples by using engineered reporter phages. Appl. Environ. Microbiol. 82, 2380–2387. doi: 10.1128/AEM.03772-15
Sholes, S. L., Harrison, S., Forrest, S., Ton, S., Grady, S. L., Verratti, K., et al. (2023). Draft genome assemblies of phage AP50c-resistant derivatives of Bacillus anthracis Sterne strain 7702 lacking plasmid pXO2. Microbiol Resour Announc 12:e0131322. doi: 10.1128/mra.01313-22
Sozhamannan, S., Chute, M. D., McAfee, F. D., Fouts, D. E., Akmal, A., Galloway, D. R., et al. (2006). The Bacillus anthracis chromosome contains four conserved, excision-proficient, putative prophages. BMC Microbiol. 6:34. doi: 10.1186/1471-2180-6-34
Sozhamannan, S., McKinstry, M., Lentz, S. M., Jalasvuori, M., McAfee, F., Smith, A., et al. (2008). Molecular characterization of a variant of Bacillus anthracis-specific phage AP50 with improved bacteriolytic activity. Appl. Environ. Microbiol. 74, 6792–6796. doi: 10.1128/AEM.01124-08
Keywords: Bacillus anthracis, phages, phage resistance, receptor binding proteins, bacterial receptors, S-layer, fluorescence detection
Citation: Forrest S, Ton S, Sholes SL, Harrison S, Plaut RD, Verratti K, Wittekind M, Ettehadieh E, Necciai B, Sozhamannan S and Grady SL (2023) Genetic evidence for the interaction between Bacillus anthracis-encoded phage receptors and their cognate phage-encoded receptor binding proteins. Front. Microbiol. 14:1278791. doi: 10.3389/fmicb.2023.1278791
Received: 16 August 2023; Accepted: 11 October 2023;
Published: 31 October 2023.
Edited by:
Alicja Wegrzyn, Polish Academy of Sciences, PolandReviewed by:
Malgorzata Barbara Lobocka, Polish Academy of Sciences, PolandCopyright © 2023 Forrest, Ton, Sholes, Harrison, Plaut, Verratti, Wittekind, Ettehadieh, Necciai, Sozhamannan and Grady. This is an open-access article distributed under the terms of the Creative Commons Attribution License (CC BY). The use, distribution or reproduction in other forums is permitted, provided the original author(s) and the copyright owner(s) are credited and that the original publication in this journal is cited, in accordance with accepted academic practice. No use, distribution or reproduction is permitted which does not comply with these terms.
*Correspondence: Sarah L. Grady, c2FyYWguZ3JhZHlAamh1YXBsLmVkdQ==; Shanmuga Sozhamannan, c2hhbm11Z2Euc296aGFtYW5uYW4uY3RyQGFybXkubWls
Disclaimer: All claims expressed in this article are solely those of the authors and do not necessarily represent those of their affiliated organizations, or those of the publisher, the editors and the reviewers. Any product that may be evaluated in this article or claim that may be made by its manufacturer is not guaranteed or endorsed by the publisher.
Research integrity at Frontiers
Learn more about the work of our research integrity team to safeguard the quality of each article we publish.