- 1Genomics and Bioinformatics Unit, National Institute of Health Doutor Ricardo Jorge (INSA), Lisbon, Portugal
- 2Reference and Research Laboratory on Special Pathogens, National Centre for Microbiology (CNM), Carlos II Health Institute (ISCIII), Madrid, Spain
- 3Departamento de Ciencias Agroforestales, Instituto Universitario de Investigación en Gestión Forestal Sostenible (iuFOR), E.T.S. Ingenierías Agrarias, Universidad de Valladolid, Palencia, Spain
- 4Technology and Innovation Unit, Department of Human Genetics, National Institute of Health Doutor Ricardo Jorge (INSA), Lisbon, Portugal
- 5Instituto de Investigación en Recursos Cinegéticos (IREC-CSIC, UCLM, JCCM), Ciudad Real, Spain
- 6Área de Microbiología, Facultad de Medicina, Universidad de Catilla-La Mancha (UCLM), Ciudad Real, Spain
- 7Department of Parasitology, Veterinary Faculty, University of Zaragoza, Zaragoza, Spain, Zaragoza, Spain
- 8Departamento de Parasitología, Facultad de Veterinaria, Universidad de Zaragoza, Instituto Agroalimentario de Aragón-IA2 (Universidad de Zaragoza-CITA), Zaragoza, Spain
- 9Veterinary and Animal Research Center (CECAV), Faculty of Veterinary Medicine, Lusófona University, Lisbon, Portugal
- 10Emergency and Biopreparedness Unit, National Institute of Health Doutor Ricardo Jorge, Lisbon, Portugal
Introduction: Francisella tularensis is a highly infectious bacterium that causes the zoonotic disease tularemia. The development of genotyping methods, especially those based on whole-genome sequencing (WGS), has recently increased the knowledge on the epidemiology of this disease. However, due to the difficulties associated with the growth and isolation of this fastidious pathogen in culture, the availability of strains and subsequently WGS data is still limited.
Methods: To surpass these constraints, we aimed to implement a culture-free approach to capture and sequence F. tularensis genomes directly from complex samples. Biological samples obtained from 50 common voles and 13 Iberian hares collected in Spain were confirmed as positive for F. tularensis subsp. holarctica and subjected to a WGS target capture and enrichment protocol, using RNA oligonucleotide baits designed to cover F. tularensis genomic diversity.
Results: We obtained full genome sequences of F. tularensis from 13 animals (20.6%), two of which had mixed infections with distinct genotypes, and achieved a higher success rate when compared with culture-dependent WGS (only successful for two animals). The new genomes belonged to different clades commonly identified in Europe (B.49, B.51 and B.262) and subclades. Despite being phylogenetically closely related to other genomes from Spain, the detected clusters were often found in other countries. A comprehensive phylogenetic analysis, integrating 599 F. tularensis subsp. holarctica genomes, showed that most (sub)clades are found in both humans and animals and that closely related strains are found in different, and often geographically distant, countries.
Discussion: Overall, we show that the implemented culture-free WGS methodology yields timely, complete and high-quality genomic data of F. tularensis, being a highly valuable approach to promote and potentiate the genomic surveillance of F. tularensis and ultimately increase the knowledge on the genomics, ecology and epidemiology of this highly infectious pathogen.
1 Introduction
Francisella tularensis is a facultative intracellular bacterium causing the zoonotic disease tularemia. It is considered a potential bioterrorist agent due to its very low infective dose and considerable stability in aerosols, being classified as a Category A biological agent by Centers for Disease Control and Prevention (CDC) (n.d.). F. tularensis may be transmitted to humans by a number of different routes, including handling infected animals, ingestion of contaminated food or water, inhalation of infective aerosols or arthropod bites (ticks and insects) (Petersen et al., 2009). In nature, F. tularensis has been detected in many wild species including lagomorphs, rodents, insectivores, carnivores, ungulates, marsupials, birds, amphibians, fish, and invertebrates. In Northwest (NW) Spain, the region of Castilla-y-León is an endemic hotspot of the disease in southern Europe, accumulating more than 1,000 human cases since 1997 and its epidemiology is mainly associated with population outbreaks of common voles, Microtus arvalis, in intensive farming landscapes (Luque-Larena et al., 2017; Herrero-Cófreces et al., 2021).
There are two clinically relevant subspecies of F. tularensis: F. tularensis subsp. tularensis and F. tularensis subsp. holarctica. Only the less pathogenic subspecies holarctica has been detected in Europe (Dennis et al., 2001; Carvalho et al., 2014). The diagnosis of tularemia is complex due both to the nonspecific nature of the initial symptoms and to the fact that F. tularensis is difficult to culture (Doern, 2000), showing slow growth rates, with individual colonies on nonselective optimized agar plates usually requiring two to four days of incubation to be visible (Aloni-Grinstein et al., 2017).
Environmental studies on the distribution of Francisella spp. are hampered by the frequency of other species of Francisella such as Francisella-like endosymbionts and other so far not proven pathogenic species that can produce a misleading positive result (Escudero et al., 2008). Efforts have been made to improve methods for the discrimination on Francisella species.
Considering the clonal nature of this species, only molecular methods with high discriminatory power, such as whole genome sequencing (WGS), allow to distinguish between closely related subpopulations at strain level (Johansson and Petersen, 2010; Dwibedi et al., 2016). The genotyping of F. tularensis strains is mostly based on the identification of canonical SNPs defined on a whole-genome level (Kevin et al., 2020). However, despite rapidly accumulating knowledge, the phylogeography of the pathogen is still poorly understood due to the low availability of isolates for WGS and consequent lack of F. tularensis genomic data in many tularemia-endemic countries (Shevtsov et al., 2021).
In this study, we implemented a culture-free approach for capturing and sequencing F. tularensis genomes directly from complex biological samples, passing the constraints associated with the isolation and culture of this fastidious pathogen. Ultimately, we aimed to increase the knowledge on F. tularensis genomic epidemiology not only in Spain but also on a global scale by an integrative analysis with genomes from multiple countries.
2 Methods
2.1 Sample collection and selection
In NW Spain, the common vole is a key host and spillover agent of tularemia (Herrero-Cófreces et al., 2021). Vole samples were obtained from long-term ecological studies in which animals are captured in the field (Rodríguez-Pastor et al., 2017). Voles were live-trapped using Sherman© traps (8 cm × 9 cm × 23 cm, LFAHD Sherman©) baited with carrots and apples, which were set open in the morning and retrieved 24 h later. Captures were performed every four months (March, July and November) between July 2009 and July 2015. Captured voles were taken alive to the laboratory and euthanized using a CO2 cabinet, following a protocol approved by the ethics committee from the University of Valladolid (authorization code: 4801646). Iberian hare samples were obtained from hunters and collected in the field during two hunting seasons (from October 2016 to February 2018). Subsequently, necropsies were performed in a microbiological safety cabinet, collecting different samples in aseptic conditions (mainly liver and spleen) for the detection of F. tularensis. All animals were captured from the provinces of Palencia (42°01′N, 4°42′W), Valladolid (41°34′N, 5°14′W) and Zamora (41°50′N, 5°36′W).
In the present study, the selected biological samples (liver, spleen and lung) were obtained from 50 M. arvalis that had tested positive for F. tularensis by real time-PCR, mostly with low Ct results. Moreover, DNAs extracted from homogenates of spleen and liver from 13 Iberian hares were tested as well, with signs suggestive of tularemia (i.e., hepatomegaly, splenomegaly or foci of necrosis in the selected tissues).
2.2 Culture of the samples
To culture the etiological agent, samples from 50 voles, consisting of lung, liver and spleen of each animal, were inoculated separately in culture media. Each tissue was punctured 10 to 20 times with a sterile wooden stick. Tissue adhering to the stick was transferred to chocolate agar PolyViteX (Biomerieux) and then a 1-μL sterile loop was used to streak the plate for colony isolation. All plates were incubated at 37°C for 5 more days and checked daily for characteristic F. tularensis growth (Petersen et al., 2004).
2.3 DNA extraction and real-time PCR
For cultures, DNA was extracted and purified using QIAGEN® Genomic-tip 100/G columns and QIAGEN® Genomic DNA Buffer sets from suspensions calibrated to 3 McFarland units according to the manufacturer’s recommendations. For the biological samples, DNA from a homogenized mixture of liver and spleen (≈25 mg) was extracted by standard procedures using the QIAamp DNA Mini Kit (QIAGEN, Valencia, CA, United States).
Samples were tested using a real-time multitarget TaqMan PCR, using tul4 and ISFtu2 assays, and genome equivalents (GEs) concentration was inferred as previously described (Versage et al., 2003). Positive samples were further tested using real-time TaqMan PCR assays which differentiate between F. tularensis subsp. tularensis (type A) and F. tularensis subsp. holarctica (type B) (Kugeler et al., 2006). Moreover, a phylogenetically informative region of lipoprotein A (lpnA) gene (231 bp) was amplified by conventional PCR and hybridized with specific probes by reverse-line blotting (RLB) as previously described (Escudero et al., 2008).
For real-time PCR using tul4, ISFtu2, type A and type B assays, a type B positive control was used, as type A strains are restricted to North America.
In the four positive cultures of F. tularensis, the isolates were subjected to whole-genome sequencing on an Illumina MiSeq platform after DNA extraction and library preparation using the Nextera XT Library Preparation kit (Illumina, San Diego, CA, United States), according to manufacturer’s instruction.
2.4 SureSelectXT HS custom library design for targeted sequencing of Francisella tularensis
RNA oligonucleotide baits (120 bp in size, a total of 54,756 baits) contiguously spanning (i.e., no tiling) the entire genome of Francisella tularensis were designed based on representative publicly available genomes, and plasmids, of the four F. tularensis subspecies (accession numbers NC_010677.1, NZ_CP009694.1, NZ_CP009683.1, NZ_CP010104.1, NZ_CP009352.1, NZ_CP010447.1, NC_006570.2, and NZ_CP009633.1). To ensure specificity, we excluded baits with considerable homology (determined by BLASTn) with the human (Human G + T) or mouse (Mouse G + T) genomes and transcriptomes or with available nucleotide sequences from the genus Microtus (taxonomy ID 10053), Oryctolagus (taxonomy ID 9984) or Lepus (taxonomy ID 9980). Finally, this custom library was uploaded to Agilent’s SureDesign software (settings: Tier 3; Boosting: Balanced; Probe Precedence: Reuse Existing) and synthesized by Agilent Technologies (Santa Clara, CA, United States). The set of RNA baits sequences used in the current Target Enrichment protocol is available at https://doi.org/10.5281/zenodo.8043219.
2.5 SureSelectXT HS targeted whole-genome sequencing of Francisella tularensis directly from biological samples
In parallel to culture attempts, we used the designed RNA oligonucleotide baits in order to capture and sequence F. tularensis genomes directly from the biological samples, similarly to previous studies (Pinto et al., 2016; Borges et al., 2021; Macedo et al., 2023). After DNA extraction (described above), whole-genome capture and sequencing of Francisella tularensis was performed following SureSelect XT HS Target Enrichment System for Illumina Multiplexed Sequencing protocol (G9702-90000, Version E0, November 2020, Agilent Technologies, Santa Clara, CA, United States) using the custom baits library described above, in a 1:5 dilution. Library preparation started with the fragmentation of high quality DNA using the Agilent’s SureSelectXT HS Low input Enzymatic Fragmentation kit (“Method 2: Enzymatic DNA Fragmentation” option described in “Step 2.”), and was further carried out following the manufacturer’s instructions. Libraries fragments size, concentration and molarity were determined on a Fragment Analyzer system (Agilent, Santa Clara, CA, United States). Libraries were then sequenced on an Illumina MiSeq or NextSeq apparatus (Illumina, San Diego, CA, United States) (Supplementary Table 1). To evaluate the success and feasibility of the target capture and enrichment (TCE) on a routine surveillance basis (in terms of both time and costs), a single library was prepared from each sample and sequenced only once (despite the potential gains of resequencing). The sequencing reads (only reads mapping against F. tularensis strain FTNF002-00 genome) generated in the present study were deposited in the European Nucleotide Archive (ENA) (BioProject PRJEB63267). Detailed ENA accession numbers are described in Table 1.
2.6 Sequence data analysis
To assess the efficiency of the whole-genome capture and enrichment, we determined the percentage of reads that corresponded to F. tularensis in each sample (percentage of reads “on-target”) by mapping the reads (before and after quality improvement) against the reference genome Francisella tularensis subsp. holarctica FTNF002-00 (acc. no. NC_009749.1) using bowtie2 v.2.4.2 (Langmead and Salzberg, 2012). The percentage of the reference genome covered and respective mean depth of coverage were evaluated using the getCoverage tool.1
The de novo assembly of the genomes was performed with INNUca v.4.2.2,2 which, after reads’ quality analysis (FastQC v0.11.5)3 and cleaning (Trimmomatic v0.36) (Bolger et al., 2014), performs de novo assembly with SPAdes v3.14.0 (Bankevich et al., 2012) and post-assembly optimization with Pilon v1.23 (Walker et al., 2014). The reads and contigs were taxonomically classified using Kraken 2 v2.0.7-beta (Wood et al., 2019) with the Standard-16 16 GB database (26th September 2022, available at: https://benlangmead.github.io/aws-indexes/k2). For the samples with a flag in the INNUca pipeline indicating that other taxa besides Francisella were identified in the assembly, only the contigs classified within the family Francisellaceae, or at lower taxonomical ranks, were kept in the final assemblies. When needed, genome annotation was performed with Prokka v1.14.6 (Seemann, 2014). The de novo assemblies generated in this study are available at https://doi.org/10.5281/zenodo.8043219.
2.7 Genetic diversity of the captured genomes and phylogenetic analysis
The genomes were genotyped using CanSNPer2,4 which assigns the genomes to genetic clades based on the identification of established canonical single nucleotide polymorphisms (canSNPs) (Lärkeryd et al., 2014). To assess the phylogenetic context of the newly sequenced genomes into the F. tularensis diversity, all the F. tularensis complete genomes available in RefSeq and Genbank (and associated metadata), as of 27th February 2023, were collected and also classified with CanSNPer2. The details of the genomes can be found in Supplementary Table 2, including BioProjects and associated publications (La Scola et al., 2008; Barabote et al., 2009; Antwerpen et al., 2013; Coolen et al., 2013; Alm et al., 2015; Atkins et al., 2015; Dwibedi et al., 2016; Madani et al., 2017; Busch et al., 2018; Koene et al., 2019; Sichtig et al., 2019; Busch et al., 2020; Kevin et al., 2020; Kittl et al., 2020; Myrtennäs et al., 2020; Witt et al., 2020; Neubert et al., 2021; Öhrman et al., 2021; Pisano et al., 2021).
Multi-genome alignments and extraction of single nucleotide variant sites (SNVs) was performed with Parsnp v1.2 (Treangen et al., 2014) with the parameters –c and –C 2000, using the genome of strain FTNF002-00 (acc. no. NC_009749.1) as reference (for increased phylogenetic resolution, other reference genomes were used for some subclades phylogenetic trees; see figures legends for further information). Maximum likelihood trees were obtained with MEGA-CC v10.0.5 (Kumar et al., 2018) with 100 bootstraps. For particular comparative analyses, Snippy v4.5.15 was used for mapping and variant calling directly from the sequencing reads (with default parameters, with exception of –mapqual that was set to 20) and lofreq6 was run over snippy BAM files for the detection of minor variants (Wilm et al., 2012), with indelqual mode with –dindel to assess indel qualities and then call mode, including –call-indels. Only the minor variants at a frequency of ≥5%, supported by at least 4 reads in positions covered by at least 10 reads were considered and further inspected with the Integrative Genomics Viewer (IGV) for confirmation/exclusion (Thorvaldsdottir et al., 2013).
Grapetree7 (Zhou et al., 2018) and Microreact8 (Argimón et al., 2016) were used for phylogenies and metadata visualization.
3 Results
3.1 Dataset characterization and culture of samples
A total of 63 samples, including samples from 50 common voles and 13 Iberian hares, that consisted of pooled of spleen and liver homogenates, were subjected to the SureSelectXT HS target capture and enrichment protocol (TCE) after DNA extraction. The counts and concentration of genome equivalents (GE) were assessed for 52 samples (46 voles and 6 hares) based on the screening of tul4 and ISFtu2 (Supplementary Table 1). All samples tested positive for ISFtu2, but 11 were negative for tul4 in the real time PCR, but positive by conventional PCR followed by RLB. This discrepancy is likely due to the fact that ISFtu2 is an insertion element-like sequence present in multiple copies and hence its detection is more sensitive than tul4 (Versage et al., 2003). All samples were confirmed as F. tularensis subsp. holarctica.
In parallel, culture was attempted on different tissues collected from the 50 voles (Supplementary Table 1). It was possible to isolate F. tularensis in two animals (4%), specifically from the spleen and liver of vole FT-MA1953 and from the lung and spleen of vole FT-MA2129. Concordantly, FT-MA1953 and FT-MA2129 were the voles samples with the highest GE counts used for TCE input (Supplementary Table 1). The four isolates were subjected to WGS and included in the downstream analysis.
3.2 Target capture and enrichment success
All 63 samples met the minimum required DNA input (10 ng) for the TCE protocol, with the majority having the maximum quantity of 200 ng of DNA available to use as input. After the TCE protocol, 14/63 samples were excluded due to very low library concentrations and the remaining 49 samples proceeded to NGS (Supplementary Table 1). A mean of 5,888,432 reads was generated per sample, with a high variability between samples (range: 169366–24,726,074 reads), although not correlating with success (Figure 1).
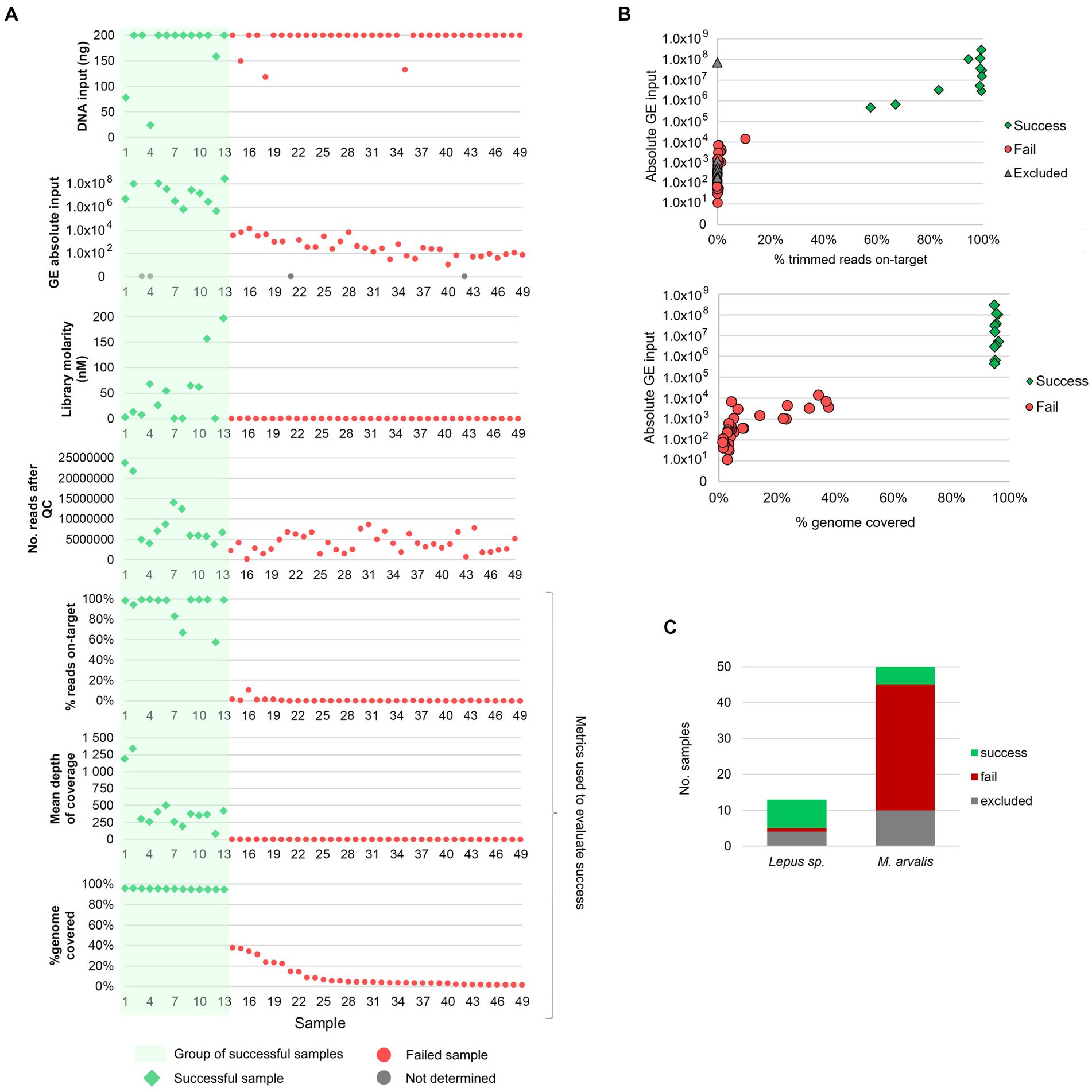
Figure 1. Overview of the success of the target and enrichment sequencing approach and its relation to multiple sample features. (A) Relation between genome coverage and percentage of reads on-target (three bottom panels), and multiple factors: initial DNA input, absolute input of genome equivalents (GE), NGS library molarity and number of reads after quality control (QC). Samples are ordered from 1 to 49 (on the xx-axis) from the sample with the highest to the lowest percentage of horizontal genome coverage (by at least 1-fold). (B) Association between absolute GE input [available for 52 samples (45 sequenced and 7 excluded)] and: (i) the percentage of reads on-target (upper panel) and, (ii) the percentage of genome coverage (lower panel). Gray triangles in the upper panel identifies the samples that were excluded before sequencing due to low library concentration but for which GE counts were available (n = 7). The excluded sample with high GE counts (1×108) corresponds to sample FT-MA1953. (C) Distribution of successful, failed and excluded samples among the samples from common voles (M. arvalis, n = 50) and Iberian hares (L. granatensis, n = 13).
To evaluate the success of the TCE approach, the proportion of reads corresponding to F. tularensis (reads on-target) and the completeness of the genome (percentage of the reference genome covered by at least 1-fold) was determined for each sample. Among the 49 samples sequenced, 13 (26.5%) samples yielded >50% reads on-target (10 of which having >90% of reads on-target) and > 94% of the reference genome covered (Figure 1). Most of the remaining 36 unsuccessful samples had very low percentages of reads on-target (ranging between 0.01 and 10.64%, mean = 0.61%) and a mean of 9.3% of the genome covered (Figure 1). Interestingly, this revealed an “all-or-nothing” scenario where the successful and unsuccessful samples are clearly separated in two distinct groups (Figure 1B), rather than showing a linear trend of the evaluated metrics. In fact, success was obtained for all samples with more than 1 × 105 GE of input, with a minimum of 463,513 GE for sample FT-MA2136, while the highest GE input among the “fail” group was 13,948 GE (Figure 1B). This large “gap” in GE input values between success and fail groups might help explain the “all-or-nothing” scenario with no samples with intermediate success in between.
Among the 50 vole samples that were also subjected to culture, isolation of F. tularensis was possible for two samples (4%), while the culture-free TCE approach allowed obtaining the genome of F. tularensis from five samples (10%). Of note, one of the two successfully cultured samples, FT-MA1953, did not proceed to NGS after the TCE protocol due to a very low library concentration. However, the high GE count (~7×107) of FT-MA1953 falls within the successful GE input range and it is, in fact, the only sample failing within this group (Figure 1B). As such, we speculate that some experimental issue might have occurred during the wet-lab protocol leading to a poor library (e.g., failing to add a reagent) for this sample.
Of note, TCE success rate was much higher among hares (61.5%) than voles (10%) samples (Figure 1C), consistent with the higher GE counts observed in the former species (Supplementary Table 1).
3.3 Characterization of Francisella tularensis genomes and integration into the global phylogeny
3.3.1 CanSNP classification
The de novo assemblies of the 13 successful sequenced genomes ranged from 1.72 to 1.78 Mb, which is close to the expected F. tularensis genome size (1.8–1.9 Mb), and had a mean depth of coverage of 505-fold (range 104–1,316-fold) (Table 1). Genomes were typed based on canSNPs classification by canSNPer2 (Lärkeryd et al., 2014). For clarity reasons, the clades are referred to according to their level of discrimination (from D0 to D9) within this dataset (Figure 2), where level D0 corresponds to the level of the clade that is common to all the analyzed sequences and level D1 is the first level where the analyzed sequences start being discriminated by clade.
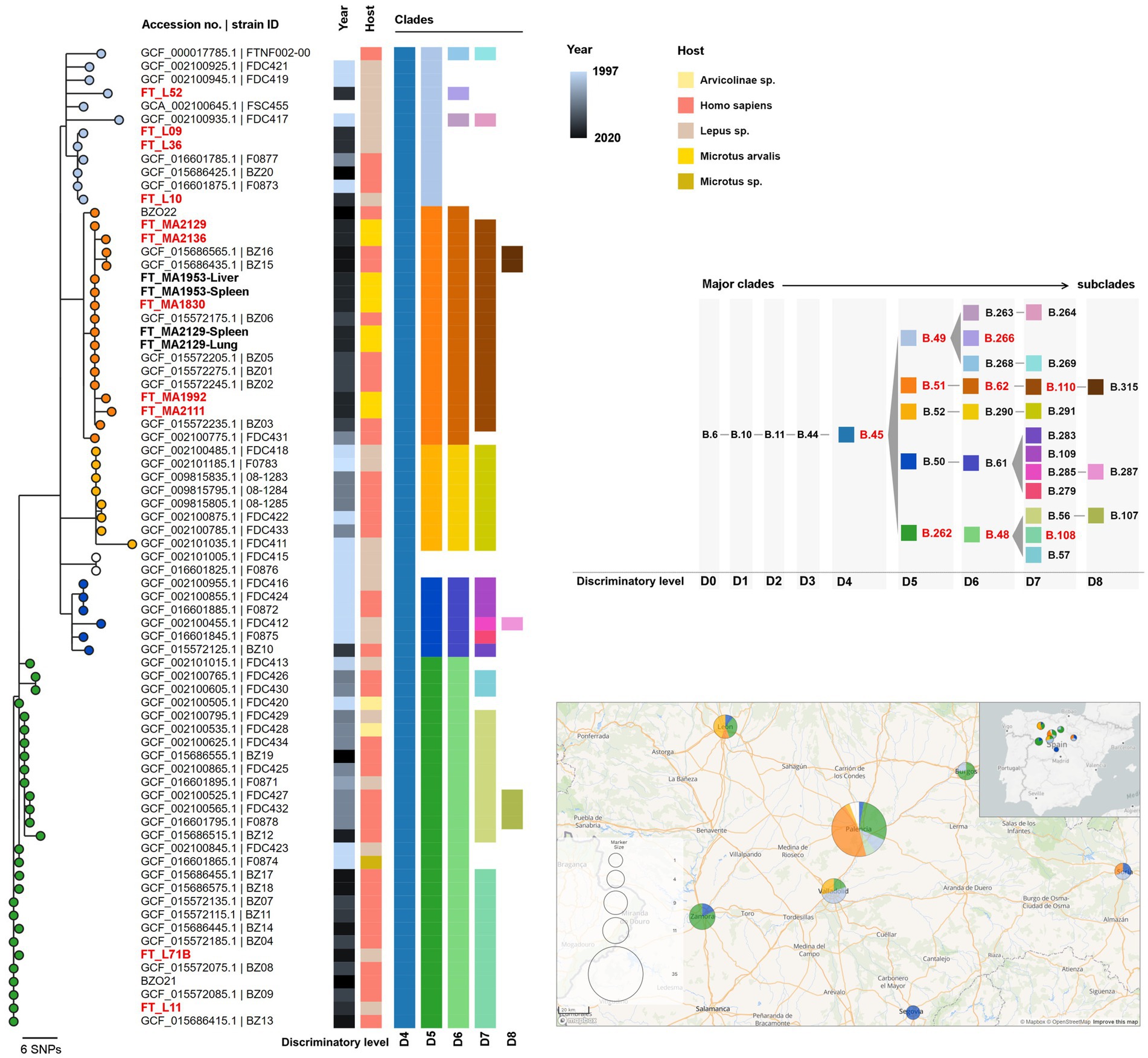
Figure 2. Maximum likelihood phylogenetic tree of the newly sequenced genomes from clade B.10 (D1; n = 17) and all available Francisella tularensis subsp. holarctica genome assemblies from Spain (n = 58; Supplementary Table 2). The phylogenetic tree was generated using MEGA-CC v10.0.5 with 100 bootstraps based on 113 core single nucleotide variant positions (SNVs) extracted from a multiple genome alignment with 1,558,560 bp, generated with parsnp using the genome of strain FTNF002-00 (acc. no. NC_009749.1) as reference. Tree nodes are colored by clade (at level D5) and metadata blocks show the respective year of collection, host and canSNP clades and subclades (from level D4 to D8). The strain IDs from samples generated in this study using the capture and enrichment approach (TCE) (n = 11) are shown in red. Other genomes obtained from strains isolated in culture are shown in black and bold (n = 4). The panel on the right shows the color codes for each clade and subclade identified among the analyzed genomes and the ancestry relationships between them. The clades identified among the TCE genomes are highlighted in red. The ancestral clades (SNP path) of major clade B.45 identified by CanSNPer2 are B.1-B.2-B.3-B.5-B.6-B.10-B.11-B.44-B.45 (not shown). The lower panel on the right shows the geographical distribution of the samples, with the same sample color scheme of the nodes on the phylogenetic tree (clade level D5). A smaller map shows the geographical location of the study area within the Iberian Peninsula.
All 13 genomes belonged to major clade B.6 (level D0) (descendant of ancestral clades B.1-B.2-B.3-B.5), consistent with strains circulating in Europe and North America (Pilo, 2018). Among these, 11 genomes belonged to subclade B.10 (D1), common in Western Europe, and the other two belonged to B.7 (D1), a common clade in the United States and Scandinavia (Supplementary Figure 1) (Pilo, 2018).
3.3.2 Integration in global phylogeny
All the genome assemblies of F. tularensis subs. holartica (or F. tularensis without subspecies described) obtained from public databases (RefSeq and Genbank) were classified with CanSNPer2. All the non-duplicate genomes from subclades B.10 and B.7 (level D1) were selected for the phylogenetic analysis (n = 556, Supplementary Table 2, Supplementary Figure 1).
The 11 B.10 (level D1) genomes belonged to subclade B.45 (level D4) and could be further discriminated into three subclades within level D5: (i) B.49 (n = 4), (ii) B.51 (n = 5) and (iii) B.262 (n = 2) (Figure 2 and Supplementary Figure 2, Table 1).
Within the global phylogeny of subclade B.49 (n = 81) (Supplementary Figure 3), which included mostly genomes from France (n = 45), Germany (n = 21) and Spain (n = 11), the genomes from samples FT_L09, FT_L10, and FT_L36 segregated in a branch together with other strains from Spain (three human isolates) and one from France (isolated from hare) (Figure 2 and Supplementary Figure 3). Strain FT_L52 integrates another phylogenetic branch that is composed of six B.266 (level D6) genomes, four of which are from France (including one human isolate).
Within B.51 (level D5), five genomes obtained through the TCE approach (FT_MA1830, FT_MA1992, FT_MA2111, FT_MA2129, and FT_MA2136) belonged to B.62 (level D6) and B.110 (level D7) subclades. The B.1.110 subclade (total of 19 sequences) also included the four isolates obtained from culture (FT_MA1953-Liver, FT_MA1953-Spleen, FT_MA2129-Lung, and FT_MA2129-Spleen), seven human isolates from Spain and three hare isolates from Germany (Supplementary Figure 4). Interestingly, although B.110 is strongly associated with samples collected in Spain, its ascendant clade B.51 (level D5; n = 59) is mostly associated with genomes from Germany (Supplementary Figure 4). Furthermore, it is noteworthy that all the B.110 strains are very closely related, even when comparing strains from Spain and Germany.
Within B.262 (level D5), both studied samples (FT_L11 and FT_L71B) belonged to subclade B.108 (D7), which involves nine other human isolates from Spain (Figure 2 and Supplementary Figure 5). This subclade is a descendent of B.48 (level D6), which is almost exclusively associated with samples collected in Spain (Supplementary Figure 5), concordant with previous reports (Dwibedi et al., 2016).
The two B.7 (level D1) genomes obtained in this study, FT_L51 and FT_L72B [further classified in subclades B.133 (D2), B.81 (D3), B.136 (D4), and B.153 (D5)], were analyzed together with 26 other genome assemblies available for this major clade (Supplementary Figure 1). These genomes originate from Norway, Germany, United States, Sweden, Finland and Russia, making FT_L51 and FT_L72B the only samples from Spain among this clade. Supplementary Figure 6 shows the phylogenetic relationship of the 22 closest isolates (USA genomes are not shown as they cluster apart at a high genetic distance).
3.3.3 Comparing the genomic information obtained by TCE and culture
Among the studied sample dataset, we could obtain the genome of F. tularensis from both culture and TCE approaches for vole FT_MA2129. No phylogenetic differences were observed between the three genomes (FT_MA2129 obtained by TCE and the culture isolates obtained from the lung FT_MA2129-Lung and spleen FT_MA2129-Spleen) obtained from this vole (Figure 2 and Supplementary Figure 4), which could be confirmed by further fine-tuned mapping-based SNP calling. Furthermore, the TCE-derived de novo assembly of sample FT_MA2129 was only about ~12 kb shorter (with similar number of contigs and N50) than the assemblies generated from the cultured isolates (Table 1). Although no TCE genome was obtained from vole FT_MA1953, a mapping-based SNP analysis was also applied to compare the two culture genomes from this animal (FT_MA1953-Liver and FT_MA1953-Spleen), confirming that they had no differences.
To further access the genomic information obtained with TCE, we explored the minor variants (i.e., low frequency populations) in sample FT_MA2129. For this, we mapped the reads of all three samples FT_MA2129, FT_MA2129-Spleen, and FT_MA2129-Lung against the FT_MA2129-Lung genome assembly and detected the minor variants with ≥5% frequency. We validated 13 minor variants in sample FT_MA2129 (with frequencies ranging between 5.1% and 13.8%) that were not detected in either of the two culture-derived genomes (which had only one minor variant validated each) (Supplementary Table 3). The proteins predicted to be affected by the 13 mutations include several transporters and two proteins potentially involved in host-pathogen interaction (ankyrin repeat domain-containing protein and normocyte binding protein 2b), among others (Supplementary Table 3).
3.3.4 Detection of mixed infections
The screening of minor variants was also performed for the remaining samples and revealed multiple mutations at frequencies between 20 and 50% in samples FT_L09 and FT_L10. To assess if these mutations corresponded to subpopulations of the same strain or to a different strain in the same sample, we artificially inserted the minor mutations in the respective original assemblies. The CanSNPer2 classification of these assemblies revealed that the minor populations belonged to clades B.110 (D7) (descendant of B.51 (D5) and B.62 (D6)) contrarily to the major populations in these samples that belong to genotype B.49 (D5), confirming that the two hares had mixed infections with two different genotypes. Moreover, the integration of these genomes (FT_L09_MINOR and FT_L10_MINOR) in the phylogenetic analysis of the dataset from Spain show that they cluster perfectly within clade B.110 (Supplementary Figure 7), together with the other five TCE genomes already in this clade.
4 Discussion
In this study, we were able to sequence near-complete F. tularensis genomes using a culture-free approach relying on the use of RNA oligonucleotide “baits” to capture and enrich F. tularensis genomic material directly from complex biological samples. The high-quality sequencing data generated allowed the characterization and typing of the newly sequenced genomes and their integration into the global phylogeny of F. tularensis subsp. holarctica. The new genomes belonged to clades B.10 (D1), common in Western Europe, and unexpectedly to B.7 (D1), common in the United States and Scandinavia (Pilo, 2018).
The TCE approach here described had a higher success rate than the conventional culture-based method. A recent study by Wagner et al. (2022) has applied a similar approach with Agilent SureSelect technology (Agilent Technologies, Santa Clara, CA, United States) where RNA probes were designed to detect and characterize the genome of F. tularensis and other diverse species in the family Francisellaceae. Although a high success among samples with low concentration of target DNA (including environmental samples) was reported, there was no information regarding a direct or indirect quantification of the pathogen in the samples, which hampers a direct comparison with the success rate achieved in the present work. Furthermore, Wagner and colleagues processed the samples with two rounds of TCE, considerably increasing processing time and cost (Wagner et al., 2022). Still, as a potential gain in sensitivity may be achieved, the TCE two-round strategy applied by Wagner and colleagues may be a very interesting option. Our approach had an estimated cost of ~250€ per sample (for SureSelect and Illumina reagents only) and a turnaround time of four to five working days (from sample processing to sequence data), presenting a valuable option to recover near-complete genomic information.
The implementation of a TCE protocol also largely benefits from a pre-selection of samples with higher probability of success. Here, we showed that samples with at least 1 × 105 GE of input had a success rate close to 100%. As such, the GE concentration estimated from real-time PCR data provides a straightforward approach for sample pre-selection using this “cut-off” value, increasing the effectiveness of the protocol.
Of note, we observed a higher success rate of TCE sequencing of hares samples (which had much higher GE concentrations) compared to voles samples. One explanation for this might be the capture context of these animals since, unlike voles (that were captured with traps), some of the sampled hares might have died of tularemia (7/13 were found dead) and others were hunted or hit by car, hinting that they might have been debilitated. Thus, in a pure speculative basis, if these animals died (or were sick) with tularemia, it is likely that they had much higher bacterial loads (congruent with our observations).
Comparing the sequence data generated from the same sample with both TCE and culture-based methods (sample FT_MA2129) showed that the designed RNA baits are highly efficient to capture the full genomic information of F. tularensis and that the culture-free generated data is highly reliable and equivalent to that obtained by sequencing of the isolates. This observation indicates that the 13 newly sequenced genomes could be well characterized at phylogenetic level. Importantly, CanSNP-based typing showed that the new genomes belonged to different clades commonly identified in Europe and was highly congruent with the genome-based phylogenies. Further phylogenetic analysis indicated that most of the new genomes were closely related to other genomes from Spain (Figure 2), with the noteworthy exception of the two new genomes from clade B.7, which is more commonly found in Scandinavia and the United States (Supplementary Figure 6). As previously reported, and in accordance with the known low genetic diversity of the subspecies, subclades do not generally correlate with geography or host (Dwibedi et al., 2016; Kevin et al., 2020), as is well illustrated here by the identification of several closely related strains largely dispersed in different countries and different hosts.
Interestingly, the three main clades (at the D5 discriminatory level) found in Spain, B.49, B.51, and B.262 (Supplementary Figure 2), were identified in both human and hares (B.49 and B.52; Figure 2) during the initial outbreaks (1997–2007) and later identified in humans and common voles (B.51; Figure 2) during the most recent vole outbreaks (2014 onwards). As such, our findings are consistent with the important roles of hares and voles outbreaks in the tularemia epidemiology and transmission to humans in this region (e.g., 2014 outbreak; see Ariza-Miguel et al., 2014; Luque-Larena et al., 2015; Herrero-Cófreces et al., 2021). Remarkably, using TCE sequencing, in two hares we were able to identify mixed infections with two distinct genotypes (clades B.49 and B.51), which further highlights the role of this host species in the transmission dynamics of tularemia.
Furthermore, we could also identify minor variants in a sample subjected to TCE that were not present in the genomes obtained from the respective cultured isolates. We hypothesize that these subpopulations reflect the within-host pathogen diversity, potentially linked to the ongoing adaptation to different niches/tissues. While RNA “baits” acted upon complex biological samples (in this case, a homogenate of liver and spleen), potentially capturing the in vivo genomic diversity of the strain, sequencing from culture reflects only the diversity of one or a few colonies. This approach has been previously applied for other pathogens, such as Treponema pallidum, revealing substantial within-patient genetic diversity (Pinto et al., 2016), Mycobacterium tuberculosis, as an alternative approach for surveillance and drug susceptibility inferences (Macedo et al., 2023), and Chlamydia trachomatis, where the technique was used to characterize and monitor the transcontinental dissemination of an emergent recombinant strain (Borges et al., 2021). In this context, despite F. tularensis being a highly monomorphic pathogen, the TCE approach allows the exploration of this additional layer of genetic variability of low-frequency populations and potentially distinguish isolates that are otherwise indistinguishable (if only the consensus sequences are considered).
Globally, this study showed that the TCE method can generate complete F. tularensis genomic information in a timely manner, allowing us to carry out highly discriminatory phylogenetic analysis at whole-genome level. We performed CanSNP typing, integration of genomes into global phylogeny (with important observations in regards to clades geographical distribution and hosts) and fine-tuned mutation analysis, showing that TCE can greatly increase the current knowledge on F. tularensis. Although the applicability of target capture and enrichment for sequencing on a routine surveillance basis is still debatable (complexity of protocols, cost), TCE performed with greater success than culture-dependent sequencing and can be further potentiated by the pre-selection of samples based on real-time PCR data, as we propose. Considering the low success rate of F. tularensis culture and the fact that serology, a main diagnosis method, provides no information regarding molecular epidemiology, the present methodology provides a highly valuable approach toward an increased knowledge on the genomics and epidemiology of this highly infectious pathogen.
Data availability statement
Sequencing reads (only reads mapping against F. tularensis strain FTNF002-00 genome) generated in the present study were deposited in the European Nucleotide Archive (ENA) (BioProject PRJEB63267). Detailed ENA accession numbers are described in Table 1. The set of RNA baits sequences used in the current Target Enrichment protocol and the de novo assemblies are available at https://doi.org/10.5281/zenodo.8043219.
Ethics statement
The animal study was approved by the Ethics Committee from the University of Valladolid (authorization code: 4801646). The study was conducted in accordance with the local legislation and institutional requirements.
Author contributions
JI: Investigation, Methodology, Writing – original draft, Data curation, Formal analysis, Validation. RE: Investigation, Methodology, Writing – original draft, Conceptualization, Funding acquisition, Project administration, Resources, Supervision. JL-L: Conceptualization, Funding acquisition, Investigation, Methodology, Resources, Supervision, Writing – review & editing. MP: Investigation, Methodology, Writing – review & editing, Data curation. VB: Data curation, Investigation, Methodology, Writing – review & editing, Validation. RG-M-N: Investigation, Writing – review & editing, Resources. SD: Writing – review & editing, Data curation, Methodology. LV: Data curation, Methodology, Writing – review & editing. FM: Methodology, Writing – review & editing, Conceptualization, Funding acquisition, Investigation, Resources, Supervision. DV: Conceptualization, Investigation, Methodology, Resources, Supervision, Writing – review & editing. DH-R: Investigation, Resources, Writing – review & editing, Data curation, Visualization. RR-P: Data curation, Investigation, Resources, Writing – review & editing. SH-C: Data curation, Investigation, Resources, Writing – review & editing. FJ-T: Resources, Writing – review & editing. JG: Conceptualization, Funding acquisition, Investigation, Methodology, Project administration, Resources, Supervision, Writing – original draft. IL: Conceptualization, Funding acquisition, Investigation, Methodology, Project administration, Resources, Supervision, Writing – original draft.
Funding
The author(s) declare financial support was received for the research, authorship, and/or publication of this article. This work was supported by funding from the European Union EU4Health Programme under grant agreement No 101113460 (GENEO) and by the GenomePT project (POCI-01-0145-FEDER-022184), supported by COMPETE 2020 - Operational Programme for Competitiveness and Internationalisation (POCI), Lisboa Portugal Regional Operational Programme (Lisboa2020), Algarve Portugal Regional Operational Programme (CRESC Algarve2020), under the PORTUGAL 2020 Partnership Agreement, through the European Regional Development Fund (ERDF), and by Fundação para a Ciência e a Tecnologia (FCT). The acquisition of WGS-related reagents was supported by the Ministerio de Economía y Competitividad, Government of Spain/FEDER, RESERTULA project (CLG2015-66962-C2-2-R). Fieldwork for sample collection was funded by the Ministerio de Economía y Competitividad, Government of Spain/FEDER ECOTULA project (CGL2015-66962-C2-1-R) and by the Ministerio de Ciencia, Innovación y Universidades BOOMRAT project (PID2019-109327RB-I00) from Spain.
Acknowledgments
The authors want to thank Carolina Nunes for the support in laboratory work.
Conflict of interest
The authors declare that the research was conducted in the absence of any commercial or financial relationships that could be construed as a potential conflict of interest.
Publisher’s note
All claims expressed in this article are solely those of the authors and do not necessarily represent those of their affiliated organizations, or those of the publisher, the editors and the reviewers. Any product that may be evaluated in this article, or claim that may be made by its manufacturer, is not guaranteed or endorsed by the publisher.
Supplementary material
The Supplementary material for this article can be found online at: https://www.frontiersin.org/articles/10.3389/fmicb.2023.1277468/full#supplementary-material
Footnotes
1. ^https://github.com/monsanto-pinheiro/getCoverage
2. ^https://github.com/B-UMMI/INNUca
3. ^http://www.bioinformatics.babraham.ac.uk/projects/fastqc/
4. ^https://github.com/FOI-Bioinformatics/CanSNPer2
5. ^https://github.com/tseemann/snippy
6. ^https://github.com/CSB5/lofreq
References
Alm, E., Advani, A., Bråve, A., and Wahab, T. (2015). Draft genome sequence of strain R13-38 from a Francisella tularensis outbreak in Sweden. Genome Announc. 3:e01517–14. doi: 10.1128/genomeA.01517-14
Aloni-Grinstein, R., Schuster, O., Yitzhaki, S., Aftalion, M., Maoz, S., Steinberger-Levy, I., et al. (2017). Isolation of Francisella tularensis and Yersinia pestis from blood cultures by plasma purification and Immunomagnetic separation accelerates antibiotic susceptibility determination. Front. Microbiol. 8:312. doi: 10.3389/fmicb.2017.00312
Antwerpen, M. H., Schacht, E., Kaysser, P., and Splettstoesser, W. D. (2013). Complete genome sequence of a Francisella tularensis subsp. holarctica strain from Germany causing lethal infection in common marmosets. Genome Announc. 1:e00135–12. doi: 10.1128/genomeA.00135-12
Argimón, S., Abudahab, K., Goater, R. J. E., Fedosejev, A., Bhai, J., Glasner, C., et al. (2016). Microreact: visualizing and sharing data for genomic epidemiology and phylogeography. Microb. Genom. 2:e000093. doi: 10.1099/mgen.0.000093
Ariza-Miguel, J., Johansson, A., Fernández-Natal, M. I., Martínez-Nistal, C., Orduña, A., Rodríguez-Ferri, E. F., et al. (2014). Molecular investigation of tularemia outbreaks, Spain, 1997–2008. Emerg. Infect. Dis. 20, 754–761. doi: 10.3201/eid2005.130654
Atkins, L. M., Holder, M. E., Ajami, N. J., Metcalf, G. A., Weissenberger, G. M., Wang, M., et al. (2015). High-quality draft genome sequence of Francisella tularensis subsp. holarctica strain OR96-0246. Genome Announc. 3:e00898–15. doi: 10.1128/genomeA.00898-15
Bankevich, A., Nurk, S., Antipov, D., Gurevich, A. A., Dvorkin, M., Kulikov, A. S., et al. (2012). SPAdes: a new genome assembly algorithm and its applications to single-cell sequencing. J. Comput. Biol. 19, 455–477. doi: 10.1089/cmb.2012.0021
Barabote, R. D., Xie, G., Brettin, T. S., Hinrichs, S. H., Fey, P. D., Jay, J. J., et al. (2009). Complete genome sequence of Francisella tularensis subspecies holarctica FTNF002-00. PLoS One 4:e7041. doi: 10.1371/journal.pone.0007041
Bolger, A. M., Lohse, M., and Usadel, B. (2014). Trimmomatic: a flexible trimmer for Illumina sequence data. Bioinformatics 30, 2114–2120. doi: 10.1093/bioinformatics/btu170
Borges, V., Isidro, J., Correia, C., Cordeiro, D., Vieira, L., Lodhia, Z., et al. (2021). Transcontinental dissemination of the L2b/D-Da recombinant chlamydia trachomatis lymphogranuloma venereum (LGV) strain: need of broad multi-country molecular surveillance. Clin. Infect. Dis. 73, e1004–e1007. doi: 10.1093/cid/ciab067
Busch, A., Homeier-Bachmann, T., Abdel-Glil, M. Y., Hackbart, A., Hotzel, H., and Tomaso, H. (2020). Using affinity propagation clustering for identifying bacterial clades and subclades with whole-genome sequences of Francisella tularensis. PLoS Negl. Trop. Dis. 14:e0008018. doi: 10.1371/journal.pntd.0008018
Busch, A., Thomas, P., Zuchantke, E., Brendebach, H., Neubert, K., Gruetzke, J., et al. (2018). Revisiting Francisella tularensis subsp. holarctica, causative agent of tularemia in Germany with bioinformatics: new insights in genome structure, DNA methylation and comparative phylogenetic analysis. Front. Microbiol. 9:344. doi: 10.3389/fmicb.2018.00344
Carvalho, C. L., Lopes de Carvalho, I., Zé-Zé, L., Núncio, M. S., and Duarte, E. L. (2014). Tularaemia: A challenging zoonosis. Comp. Immunol. Microbiol. Infect. Dis. 37, 85–96. doi: 10.1016/j.cimid.2014.01.002
Centers for Disease Control and Prevention (CDC) (n.d.). Bioterrorism agents/diseases. Available at: https://emergency.cdc.gov/agent/agentlist-category.asp#a (accessed July 3, 2023).
Coolen, J. P. M., Sjödin, A., Maraha, B., Hajer, G. F., Forsman, M., Verspui, E., et al. (2013). Draft genome sequence of Francisella tularensis subsp. holarctica BD11-00177. Stand. Genomic Sci. 8, 539–547. doi: 10.4056/sigs.4217923
Dennis, D. T., Inglesby, T. V., Henderson, D. A., Bartlett, J. G., Ascher, M. S., Eitzen, E., et al. (2001). Tularemia as a biological weapon: medical and public health management. JAMA 285, 2763–2773. doi: 10.1001/jama.285.21.2763
Doern, G. V. (2000). Detection of selected fastidious Bacteria. Clin. Infect. Dis. 30, 166–173. doi: 10.1086/313586
Dwibedi, C., Birdsell, D., Lärkeryd, A., Myrtennäs, K., Öhrman, C., Nilsson, E., et al. (2016). Long-range dispersal moved Francisella tularensis into Western Europe from the east. Microb. Genom. 2:e000100. doi: 10.1099/mgen.0.000100
Escudero, R., Toledo, A., Gil, H., Kovácsová, K., Rodríguez-Vargas, M., Jado, I., et al. (2008). Molecular method for discrimination between Francisella tularensis and Francisella -like endosymbionts. J. Clin. Microbiol. 46, 3139–3143. doi: 10.1128/JCM.00275-08
Herrero-Cófreces, S., Mougeot, F., Lambin, X., and Luque-Larena, J. J. (2021). Linking zoonosis emergence to farmland invasion by fluctuating herbivores: common vole populations and tularemia outbreaks in NW Spain. Front. Vet. Sci. 8:454. doi: 10.3389/fvets.2021.698454
Johansson, A., and Petersen, J. M. (2010). Genotyping of Francisella tularensis, the causative agent of tularemia. J. AOAC Int. 93, 1930–1943. doi: 10.1093/jaoac/93.6.1930
Kevin, M., Girault, G., Caspar, Y., Cherfa, M. A., Mendy, C., Tomaso, H., et al. (2020). Phylogeography and genetic diversity of Francisella tularensis subsp. holarctica in France (1947–2018). Front. Microbiol. 11:286. doi: 10.3389/fmicb.2020.00287
Kittl, S., Francey, T., Brodard, I., Origgi, F. C., Borel, S., Ryser-Degiorgis, M.-P., et al. (2020). First European report of Francisella tularensis subsp. holarctica isolation from a domestic cat. Vet. Res. 51:109. doi: 10.1186/s13567-020-00834-5
Koene, M., Rijks, J., Maas, M., Ruuls, R., Engelsma, M., van Tulden, P., et al. (2019). Phylogeographic distribution of human and hare Francisella Tularensis Subsp. Holarctica strains in the Netherlands and its pathology in European Brown hares (Lepus Europaeus). Front. Cell. Infect. Microbiol. 9:11. doi: 10.3389/fcimb.2019.00011
Kugeler, K., Pappert, R., Zhou, Y., and Petersen, J. (2006). Real-time PCR for Francisella tularensis types a and B. Emerg. Infect. Dis. 12, 1799–1801. doi: 10.3201/eid1211.060629
Kumar, S., Stecher, G., Li, M., Knyaz, C., and Tamura, K. (2018). MEGA X: molecular evolutionary genetics analysis across computing platforms. Mol. Biol. Evol. 35, 1547–1549. doi: 10.1093/molbev/msy096
La Scola, B., Elkarkouri, K., Li, W., Wahab, T., Fournous, G., Rolain, J.-M., et al. (2008). Rapid comparative genomic analysis for clinical microbiology: the Francisella tularensis paradigm. Genome Res. 18, 742–750. doi: 10.1101/gr.071266.107
Langmead, B., and Salzberg, S. L. (2012). Fast gapped-read alignment with bowtie 2. Nat. Methods 9, 357–359. doi: 10.1038/nmeth.1923
Lärkeryd, A., Myrtennäs, K., Karlsson, E., Dwibedi, C. K., Forsman, M., Larsson, P., et al. (2014). CanSNPer: a hierarchical genotype classifier of clonal pathogens. Bioinformatics 30, 1762–1764. doi: 10.1093/bioinformatics/btu113
Luque-Larena, J. J., Mougeot, F., Arroyo, B., Vidal, M. D., Rodríguez-Pastor, R., Escudero, R., et al. (2017). Irruptive mammal host populations shape tularemia epidemiology. PLoS Pathog. 13:e1006622. doi: 10.1371/journal.ppat.1006622
Luque-Larena, J. J., Mougeot, F., Roig, D. V., Lambin, X., Rodríguez-Pastor, R., Rodríguez-Valín, E., et al. (2015). Tularemia outbreaks and common vole (Microtus arvalis) irruptive population dynamics in northwestern Spain, 1997–2014. Vector-Borne Zoonotic Dis. 15, 568–570. doi: 10.1089/vbz.2015.1770
Macedo, R., Isidro, J., Ferreira, R., Pinto, M., Borges, V., Duarte, S., et al. (2023). Molecular capture of Mycobacterium tuberculosis genomes directly from clinical samples: a potential backup approach for epidemiological and drug susceptibility inferences. Int. J. Mol. Sci. 24:2912. doi: 10.3390/ijms24032912
Madani, N., Giraud, P., Mendy, C., Colaneri, C., Cherchame, E., Cherfa, M.-A., et al. (2017). First draft genome sequences of three strains of Francisella tularensis subsp. holarctica, isolated from hares and a tick in France. Genome Announc. 5:e00993–17. doi: 10.1128/genomeA.00993-17
Myrtennäs, K., Escudero, R., Zaballos, Á., González-Martín-Niño, R., Gyuranecz, M., and Johansson, A. (2020). Genetic traces of the Francisella tularensis colonization of Spain, 1998–2020. Microorganisms 8:1784. doi: 10.3390/microorganisms8111784
Neubert, K., Zuchantke, E., Leidenfrost, R. M., Wünschiers, R., Grützke, J., Malorny, B., et al. (2021). Testing assembly strategies of Francisella tularensis genomes to infer an evolutionary conservation analysis of genomic structures. BMC Genomics 22:822. doi: 10.1186/s12864-021-08115-x
Öhrman, C., Sahl, J. W., Sjödin, A., Uneklint, I., Ballard, R., Karlsson, L., et al. (2021). Reorganized genomic taxonomy of Francisellaceae enables Design of Robust Environmental PCR assays for detection of Francisella tularensis. Microorganisms 9:146. doi: 10.3390/microorganisms9010146
Petersen, J. M., Mead, P. S., and Schriefer, M. E. (2009). Francisella tularensis: an arthropod-borne pathogen. Vet. Res. 40:07. doi: 10.1051/vetres:2008045
Petersen, J. M., Schriefer, M. E., Gage, K. L., Montenieri, J. A., Carter, L. G., Stanley, M., et al. (2004). Methods forenhanced culture recovery of Francisella tularensis. Appl. Environ. Microbiol. 70, 3733–3735. doi: 10.1128/AEM.70.6.3733-3735.2004
Pilo, P. (2018). Phylogenetic lineages of Francisella tularensis in animals. Front. Cell. Infect. Microbiol. 8:258. doi: 10.3389/fcimb.2018.00258
Pinto, M., Borges, V., Antelo, M., Pinheiro, M., Nunes, A., Azevedo, J., et al. (2016). Genome-scale analysis of the non-cultivable Treponema pallidum reveals extensive within-patient genetic variation. Nat. Microbiol. 2:16190. doi: 10.1038/nmicrobiol.2016.190
Pisano, S. R. R., Kittl, S., Eulenberger, U., Jores, J., and Origgi, F. C. (2021). Natural infection of a European red squirrel (Sciurus vulgaris) with Francisella tularensis subsp. Holarctica. J. Wildl. Dis. 57, 970–973. doi: 10.7589/JWD-D-20-00182
Rodríguez-Pastor, R., Escudero, R., Vidal, D., Mougeot, F., Arroyo, B., Lambin, X., et al. (2017). Density-dependent prevalence of Francisella tularensis in fluctuating vole populations, northwestern Spain. Emerg. Infect. Dis. 23, 1377–1379. doi: 10.3201/eid2308.161194
Seemann, T. (2014). Prokka: rapid prokaryotic genome annotation. Bioinformatics 30, 2068–2069. doi: 10.1093/bioinformatics/btu153
Shevtsov, V., Kairzhanova, A., Shevtsov, A., Shustov, A., Kalendar, R., Abdrakhmanov, S., et al. (2021). Genetic diversity of Francisella tularensis subsp. holarctica in Kazakhstan. PLoS Negl. Trop. Dis. 15:e0009419. doi: 10.1371/journal.pntd.0009419
Sichtig, H., Minogue, T., Yan, Y., Stefan, C., Hall, A., Tallon, L., et al. (2019). FDA-ARGOS is a database with public quality-controlled reference genomes for diagnostic use and regulatory science. Nat. Commun. 10:3313. doi: 10.1038/s41467-019-11306-6
Thorvaldsdottir, H., Robinson, J. T., and Mesirov, J. P. (2013). Integrative genomics viewer (IGV): high-performance genomics data visualization and exploration. Brief. Bioinform. 14, 178–192. doi: 10.1093/bib/bbs017
Treangen, T. J., Ondov, B. D., Koren, S., and Phillippy, A. M. (2014). The harvest suite for rapid core-genome alignment and visualization of thousands of intraspecific microbial genomes. Genome Biol. 15:524. doi: 10.1186/s13059-014-0524-x
Versage, J. L., Severin, D. D. M., Chu, M. C., and Petersen, J. M. (2003). Development of a multitarget Real-time TaqMan PCR assay for enhanced detection of Francisella tularensis in complex specimens. J. Clin. Microbiol. 41, 5492–5499. doi: 10.1128/JCM.41.12.5492-5499.2003
Wagner, D. M., Birdsell, D. N., McDonough, R. F., Nottingham, R., Kocos, K., Celona, K., et al. (2022). Genomic characterization of Francisella tularensis and other diverse Francisella species from complex samples. PLoS One 17:e0273273. doi: 10.1371/journal.pone.0273273
Walker, B. J., Abeel, T., Shea, T., Priest, M., Abouelliel, A., Sakthikumar, S., et al. (2014). Pilon: an integrated tool for comprehensive microbial variant detection and genome assembly improvement. PLoS One 9:e112963. doi: 10.1371/journal.pone.0112963
Wilm, A., Aw, P. P. K., Bertrand, D., Yeo, G. H. T., Ong, S. H., Wong, C. H., et al. (2012). LoFreq: a sequence-quality aware, ultra-sensitive variant caller for uncovering cell-population heterogeneity from high-throughput sequencing datasets. Nucleic Acids Res. 40, 11189–11201. doi: 10.1093/nar/gks918
Witt, N., Andreotti, S., Busch, A., Neubert, K., Reinert, K., Tomaso, H., et al. (2020). Rapid and culture free identification of Francisella in hare carcasses by high-resolution tandem mass spectrometry Proteotyping. Front. Microbiol. 11:636. doi: 10.3389/fmicb.2020.00636
Wood, D. E., Lu, J., and Langmead, B. (2019). Improved metagenomic analysis with kraken 2. Genome Biol. 20:257. doi: 10.1186/s13059-019-1891-0
Keywords: Francisella tularensis , WGS, RNA oligonucleotide baits, Microtus arvalis , SureSelect
Citation: Isidro J, Escudero R, Luque-Larena JJ, Pinto M, Borges V, González-Martín-Niño R, Duarte S, Vieira L, Mougeot F, Vidal D, Herrera-Rodríguez D, Rodríguez-Pastor R, Herrero-Cófreces S, Jubete-Tazo F, Gomes JP and Lopes de Carvalho I (2024) Strengthening the genomic surveillance of Francisella tularensis by using culture-free whole-genome sequencing from biological samples. Front. Microbiol. 14:1277468. doi: 10.3389/fmicb.2023.1277468
Edited by:
Augusto Simoes-Barbosa, The University of Auckland, New ZealandReviewed by:
Siva Uppalapati, University of Colorado Anschutz Medical Campus, United StatesMikeljon P. Nikolich, Walter Reed Army Institute of Research, United States
Copyright © 2024 Isidro, Escudero, Luque-Larena, Pinto, Borges, González-Martín-Niño, Duarte, Vieira, Mougeot, Vidal, Herrera-Rodríguez, Rodríguez-Pastor, Herrero-Cófreces, Jubete-Tazo, Gomes and Lopes de Carvalho. This is an open-access article distributed under the terms of the Creative Commons Attribution License (CC BY). The use, distribution or reproduction in other forums is permitted, provided the original author(s) and the copyright owner(s) are credited and that the original publication in this journal is cited, in accordance with accepted academic practice. No use, distribution or reproduction is permitted which does not comply with these terms.
*Correspondence: Isabel Lopes de Carvalho, aXNhYmVsLmNhcnZhbGhvQGluc2EubWluLXNhdWRlLnB0
†These authors share first authorship