- 1Department of Plant Pathology, College of Plant Protection, China Agricultural University, Beijing, China
- 2Department of Plant Pathology, University of Georgia, Tifton, GA, United States
Disease outbreaks of bacterial leaf spot and blight of pepper and tomato often occur in both transplant- and field-production systems worldwide. In some cases, the outbreaks are caused by novel bacterial species. Characterization of these novel bacterial species are critical in developing diagnostic assays and identifying management options for pathogen monitoring and sustainable production, respectively. We characterized strains belonging to novel Pseudomonas species that are responsible for outbreaks in pepper and tomato both in transplant-houses and in production fields in Georgia, USA. Phylogenomic analyses and whole genome sequence indices demonstrated that the pepper and tomato strains belonged to P. capsici. The whole-genome comparison revealed that 13 Pseudomonas strains from diverse isolation sources that were curated in NCBI were indeed P. capsici indicating a potential wide-host range for this bacterial species. Our greenhouse-based host-range assay also indicated that P. capsici strains were pathogenic on pepper, tomato, eggplant, cabbage, lettuce, and watermelon corroborating a wide-host-range. A phylogenetic tree inferred from the whole genome sequence data showed that the P. capsici strains from Georgia (pepper and tomato) were genetically diverse, and were closely related to tomato P. capsici strains from Florida. Genomic presence of traditional bacterial virulence factors in P. capsici strains was also determined. Pseudomonascapsici strains encode one set of type I secretion system, two sets of type II secretion systems, one set of type III secretion system, two sets of type V secretion systems, three sets of type VI secretion systems, and various secondary metabolite gene clusters including lipopeptides. In in-vitro assays, it was demonstrated that six out of seven P. capsici strains (pepper and tomato strains from Georgia) were not sensitive to 0.8 mM CuSO4. When the genomes of copper-tolerant strains were compared with the copper-sensitive strains, it was observed that the former strains encode a cluster of genes related to copper tolerance, which were absent in the genomes of copper-sensitive strains. Considering the ability of P. capsici strains to infect a range of vegetable hosts and possession of a wide range of bacterial virulence factors, secondary metabolites, and copper-tolerance genes, we envision that the management of this pathogen might potentially be a challenge.
1. Introduction
Tomato (Solanum lycopersicum) and pepper (Capsicum annuum) are important vegetable crops in the United States. However, they are vulnerable to several Pseudomonas species, including P. corrugata, P. syringae pv. tomato, and P. syringae pv. syringae, P. viridiflava, P. mediterranea, which have been known to cause diseases in these crops in the United States particularly in Georgia and Florida (Jones et al., 1981; McCarter et al., 1983; Bonn et al., 1985; Jones et al., 1986; Gitaitis et al., 1987; Voloudakis et al., 1991; Searcy et al., 2015). In fact, P. syringae pv. syringae and P. syringae pv. tomato strains were routinely recovered from field-grown tomato transplants in Georgia (Gitaitis et al., 1985). Later, the number of outbreaks reduced considerably when the transplant production was shifted completely inside temperature-controlled greenhouses. Nevertheless, sporadic outbreaks on a limited scale still occur in the greenhouse production systems.
In August 2020, two separate bacterial disease outbreaks in tomato were reported in Georgia, USA, one in the greenhouse and another in the commercial production field. In both outbreaks, the affected foliage displayed irregular lesions with distinct yellow margins. Upon bacterial isolation from symptomatic foliage and 16S rRNA sequence analysis, the strains were found to be closely related to P. cichorii. The strains were positive for oxidase activity, were able to rot potato, and were also able to induce a hypersensitive reaction on tobacco leaves. However, the strains were negative for levan production and arginine dihydrolase utilization. It is worth noting that these tomato strains were able to rot potatoes, which was different from the typical P. cichorii LOPAT profile (− + −−+) (Lelliott et al., 1966).
We previously reported a new Pseudomonas species, P. capsici, that caused typical bacterial symptoms on pepper foliage (leaf spots and blights) under greenhouse conditions (Zhao et al., 2021). The pathogen was also able to cause lesions on fruit that turned necrotic and eventually resulted in fruit rot in pepper. Pseudomonas capsici is closely related to P. cichorii, and strains from pepper could also rot potatoes (Zhao et al., 2021). In this manuscript, we determined that tomato strains isolated from two separate outbreaks were P. capsici, not P. cichorii. Furthermore, we also provided a detailed characterization of these tomato and pepper strains from recent outbreaks in Georgia, United States. The characterization included genome analysis for relatedness and the presence of traditional virulence factors, evaluation of host-range under greenhouse conditions, and copper tolerance (phenotypic and genotypic evidence). These results will provide insights into the molecular mechanisms underlying P. capsici virulence, and may prove useful in developing new strategies for managing this destructive pathogen.
2. Materials and methods
2.1. Bacterial isolation and inoculum preparation
In 2020, tomato foliage samples exhibiting symptoms were collected from Cook County and Grady County in Georgia, United States. For bacterial isolation, the margins of healthy and symptomatic tomato leaf tissues were excised using a sterile scalpel and then macerated in sterile distilled water (sdH2O). The resulting tissue macerates were then streaked onto the nutrient agar (NA) medium. The NA plates were incubated at 28°C for 2 days. The dominant colonies on the isolation plates were selected and streaked onto new NA plates to obtain pure cultures. In addition, three P. capsici strains (Pc19-1T, Pc19-2, and Pc19-3) isolated from pepper in Georgia (Zhao et al., 2021), two Pseudomonas strains (GEV417 and GEV1127) isolated from tomato in Florida (Timilsina et al., 2017), and three Pseudomonas strains (NCPPB1511, NCPPB2479, and NCPPB3928) obtained from the National Collection of Plant Pathogenic Bacteria (NCPPB) were also included and characterized in this study. The location, host, and year of isolations for these strains are shown in Table 1.
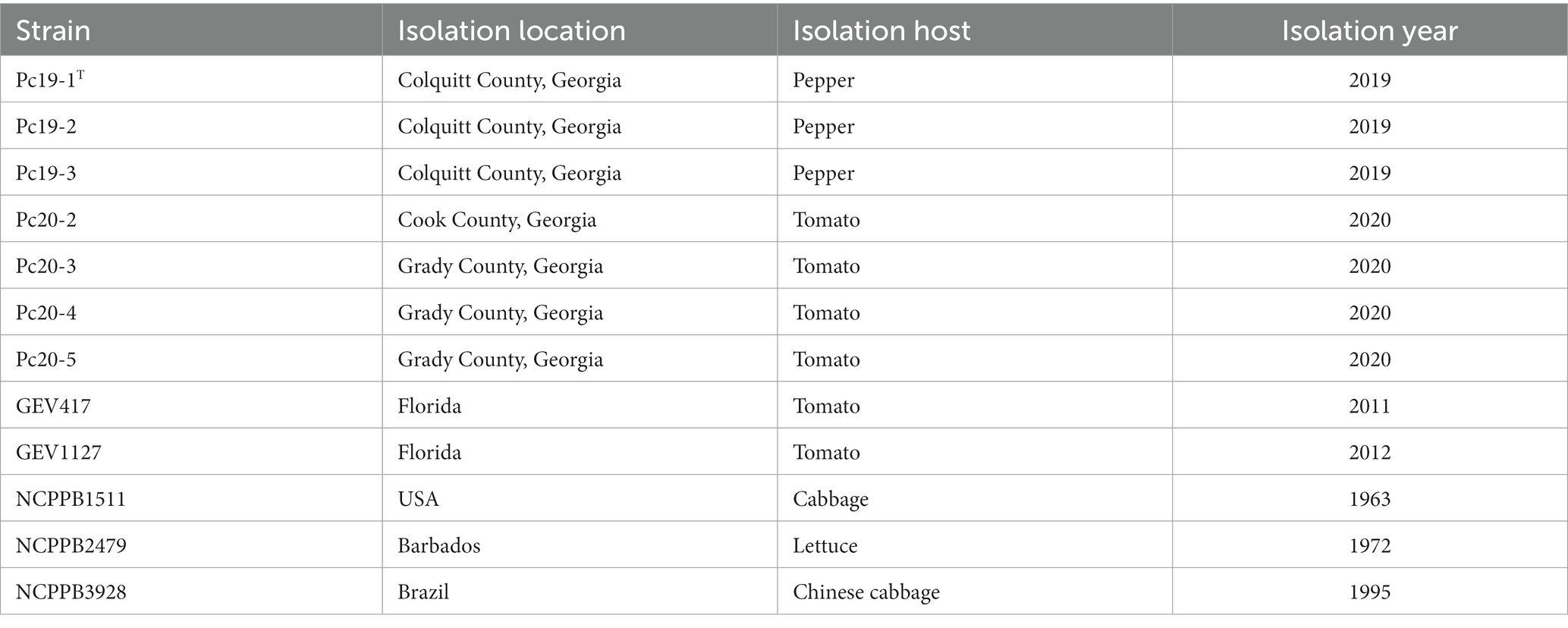
Table 1. List of Pseudomonas capsici strains characterized in this study, isolation location, host, and year.
The Pseudomonas strains were routinely cultured on NA at 28°C for 2 days. To prepare the inoculum, the strains were grown in nutrient broth in a shaking incubator (MaxQ 4,450, Thermo Scientific, Waltham, MA) at 28°C and 200 rpm for around 16 h. After centrifugation at 16,100 x g for 1 min, the supernatants were then removed, and the resulting pellets were resuspended in sdH2O. The bacterial concentrations were then adjusted to an optical density of 0.3 at 600 nm, which corresponds to approximately 108 colony-forming units (CFU/mL), using a Biophotometer (Eppendorf, Hamburg, Germany).
2.2. Pathogen identification
2.2.1. Genome sequencing and assembly
In order to identify the species of bacterial isolates, we utilized whole genome sequencing on the twelve Pseudomonas strains. First, single colonies from each strain were transferred from NA plates to 4 mL nutrient broth and cultured overnight in a shaking incubator (200 rpm, 28°C). Then, a Monarch Genomic DNA Purification Kit (New England Biolabs, Ipswich, MA) was used to extract genomic DNA from 1 mL of overnight culture. A NEBNext Ultra II DNA Library Prep Kit for Illumina was used to prepare genomic libraries and the Illumina Novaseq 6,000 platform was used to sequence the libraries by Novogene Co., Ltd. (Beijing, China). Raw sequences were filtered using fastp v 0.20.0 (Chen et al., 2018), and quality checks were conducted using fastqc v 0.11.91. The processed reads were assembled using SPAdes v 3.14 (−-isolate --cov-cutoff auto mode) (Bankevich et al., 2012) and filtered for a minimum contig size of 500 bp. The final assemblies were deposited in the NCBI database under the BioProject PRJNA890938, and uploaded to the Life Identification Number (LIN) platform developed by Tian et al. (2020).
2.2.2. Digital DNA–DNA hybridization (dDDH) and average nucleotide identity (ANI)
To determine the taxonomic classification of the twelve Pseudomonas strains at the species level, we conducted a comparative analysis of their dDDH and ANI values with the P. capsici type strain Pc19-1T. For dDDH values, the recommended settings [formula 2; i.e. GBDP formula d4; sum of all identities found in HSPs (high-scoring segment pairs) divided by overall HSP length] of the genome-to-genome distance calculator 2.1 (Meier-Kolthoff et al., 2013) were utilized for calculation using the Type Strain Genomic Server (TYGS) (Meier-Kolthoff and Göker, 2019). Additionally, the ANI values based on the BLAST algorithm (ANIb) were calculated using jSpeciesWS v1.2.1 (Richter et al., 2016).
2.2.3. Phylogenomic analysis
The species identity of all 49 P. cichorii and 12 P. capsici strains with genome assemblies that were available on NCBI (accessed on Dec 07 2022) were screened using the TYGS. Based on the results, strains that were confirmed as P. capsici, along with P. cichorii ATCC 10857T (RefSeq assembly accession: GCF_900104015.1) as an outgroup, were chosen for phylogenetic analysis based on their core genomes using the M1CR0B1AL1Z3R web server2 (Avram et al., 2019). The default settings (maximal e-value cutoff: 0.01, identity minimal percent cutoff: 80.0%, minimal percentage for core: 100.0%) were employed. Bootstrap analyses were enabled to increase the statistical robustness of the results. Similarly, phylogenomic analysis of the 12 P. capsici strains in Table 1 was also conducted using the M1CR0B1AL1Z3R web server with the default settings and bootstrap analyses enabled. Pseudomonas cichorii ATCC 10857T was included as an outgroup.
2.2.4. Phylogenetic analysis of housekeeping genes gyrB and rpoD
The sequences of housekeeping genes gyrB and rpoD were extracted from the genomes of 12 P. capsici strains and selected strains using BLASTN and analyzed in Geneious Prime (v2019.2.3). The individual gene sequences were aligned using MAFFT (v7.294b) (Katoh and Standley, 2013) and trimmed. The concatenated alignments of gyrB (487 bp) and rpoD (474 bp) were used to construct a neighbor-joining phylogenetic tree. The robustness of the tree topology was estimated using 1,000 bootstrap replicates. The tree was visualized using the tvBOT (Xie et al., 2023).
2.3. Host range assays
The host range assays were performed on pepper cv. Aristotle, tomato cv. Glacier, Chinese cabbage (Brassica rapa ssp. chinensis) cv. Rubicon, eggplant (Solanum melongena) cv. Nadia, lettuce (Lactuca sativa) cv. Dragoon, broccoli (Brassica oleracea var. italica) cv. Arcadia F1, and endive (Cichorium endivia) cv. Curlesi. Seedlings (n = 10 seedlings per host per experiment) were grown in plastic pots filled with commercial potting mix and maintained at a temperature of 28°C in a greenhouse. Two independent experiments were conducted. To inoculate the seedlings, leaves of four to six-week-old seedlings of each host were infiltrated with bacterial suspensions of Pseudomonas strains in Table 1 at a concentration of 106 CFU/mL using a syringe. Seedlings inoculated with sdH2O were used as negative controls. The symptom was evaluated qualitatively (presence/absence) in the inoculated seedlings at 7 days post-inoculation (dpi). The bacteria were re-isolated from the symptomatic tissues and the identities of the isolated bacteria were confirmed by BOX-PCR (Brusetti et al., 2008). Briefly, isolated bacterial strains were inoculated into 3 mL of nutrient broth and incubated on a rotary shaker (Innova; New Brunswick Scientific Co., Edison, NJ) at 250 rpm for 18 h. After incubation, cells were harvested by centrifugation at 6,000 × g (Allegra 25R, Beckman Coulter, Fullerton, CA) for 5 min and DNA was extracted using the UltraClean Microbial DNA Kit (MO BIO, Carlsbad, CA) according to the manufacturer’s instructions. For BOX-PCR, 2 μL of bacterial DNA were amplified using 10 μM of BOXA1R primer (5’-CTA CGG CAA GGC GAC GCT GAC G-3′) according to PCR conditions as described previously (Brusetti et al., 2008). PCR products (10 μL) were separated by electrophoresis at 125 V for 4 h on a 1.5% agarose gel in 1X Trisborate ethylenediaminetetraacetic acid (EDTA) buffer.
2.4. Copper tolerance tests
Nutrient agar supplemented with 0.8 mM copper sulphate (CuSO4· 5H2O) was prepared following published protocols (Walcott et al., 2004; Stice et al., 2018). Ten microliters of each 1 × 108 CFU/mL bacterial suspension in sdH2O were spotted onto the plates, and growth was visually evaluated and recorded as positive or negative after two days of incubation at 28°C. Acidovorax citrulli group I strain M6, and group II strain AAC00-1 were used as copper-tolerant and sensitive controls, respectively. Each strain was tested in triplicate, and the experiment was conducted twice.
2.5. Computational identification of unique copper tolerance genes, protein secretion systems, and secondary metabolite gene clusters
Genome annotation was performed using Rapid Annotation using Subsystem Technology (RAST v2.0) server (Overbeek et al., 2014). The subsystems of the annotated genomes related to copper were further manually reviewed on the RAST server. Copper tolerance gene clusters unique to the copper-tolerant strains were identified and their nucleotide sequences were extracted and aligned using MAFFT in Geneious. Protein secretion systems were predicted using TXSScan (Abby et al., 2014, 2016). Operon maps of the identified copper tolerance clusters and protein secretion systems were generated using Gene Graphics (Harrison et al., 2018) and annotated in PowerPoint. The assembly files were used as input data for in silico secondary metabolite gene cluster analysis using antiSMASH v 6 with default parameters and the ‘knownclusterblast’ flag (Blin et al., 2021).
3. Results
3.1. General features of the genome sequences
The general features of the 12 Pseudomonas sequenced genomes are summarized in Table 2. The genome lengths of the assemblies ranged from 5.82 Mbp (NCPPB2479) to 6.05 Mbp (NCPPB1511) with an average length of 5.91 Mb. The number of contigs in each genome assembly ranged from 40 to 59. The N50 of the assemblies ranged from 201,021 bp (GEV417) to 362,351 bp (NCPPB3928). The total number of genes in each genome ranges from 5,104 (Pc19-2) to 5,367 (NCPPB1511). The predicted total number of protein-coding genes varies from 4,991 (Pc19-2) to 5,248 (NCPPB1511). The GC content of the twelve genomes ranges from 58.37 to 58.58%, with an average of 58.43% (Table 2). The LINs for the twelve Pseudomonas genomes are shown in Table 3.
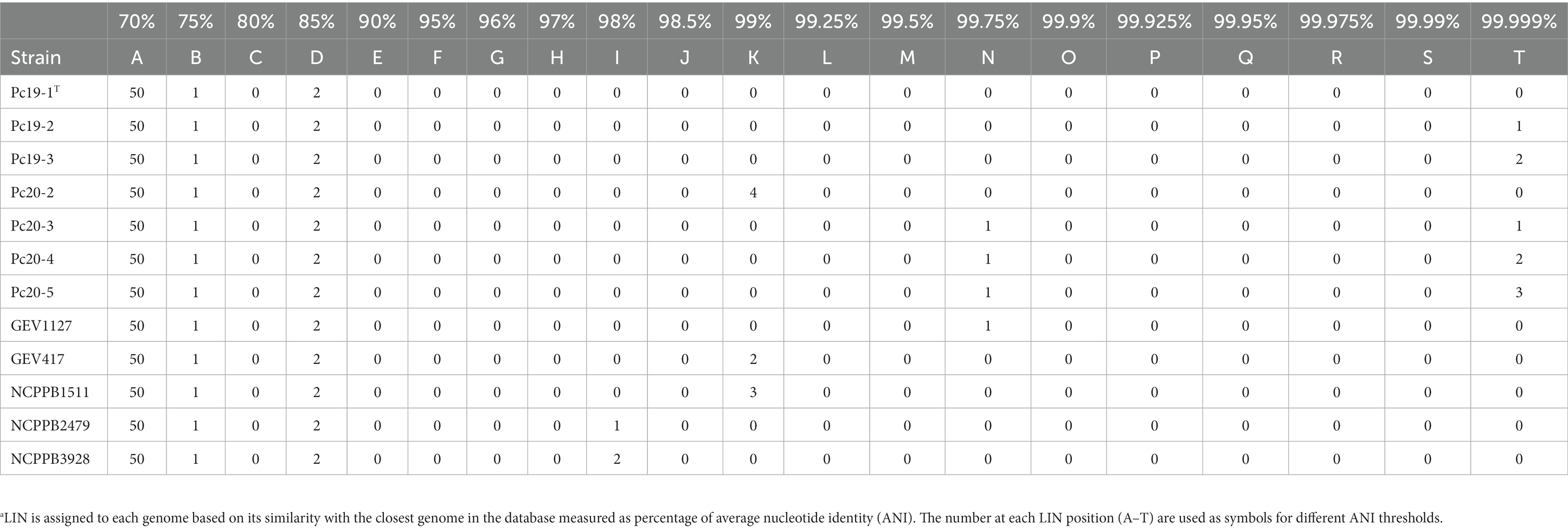
Table 3. Life Identification Number (LIN)a of Pseudomonas capsici strains.
3.2. Pathogen identification
To determine the species identity of the 12 Pseudomonas genomes, their dDDH and ANIb values were calculated by comparing them with the P. capsici type strain Pc19-1T. All strains displayed dDDH values ranging from 77.0 to 100.0% when compared with P. capsici Pc19-1T, exceeding the cut-off value of 70% for species delineation based on dDDH (Table 4). Similarly, all strains exhibited ANIb values ranging from 97.1 to 100.0% when compared with P. capsici Pc19-1T, surpassing the commonly accepted threshold of 95–96% for species delineation based on ANIb (Table 4). These observations indicate that all twelve strains belonged to the same species. Furthermore, 13 genomes listed under the P. cichorii genome list in the NCBI database had dDDH values exceeding 70%, indicating that they are in fact P. capsici. The list of strains that were wrongly speciated as P. cichorri in the NCBI database but were indeed P. capsici based on the above genome-based assays include; 473, MAFF 302698, Ku1409-10-1, NB15027, LCDW06, LCFQ22, 481, 482, 136, 474, WSXC14, s-2-2-1, and ICMP 1649.
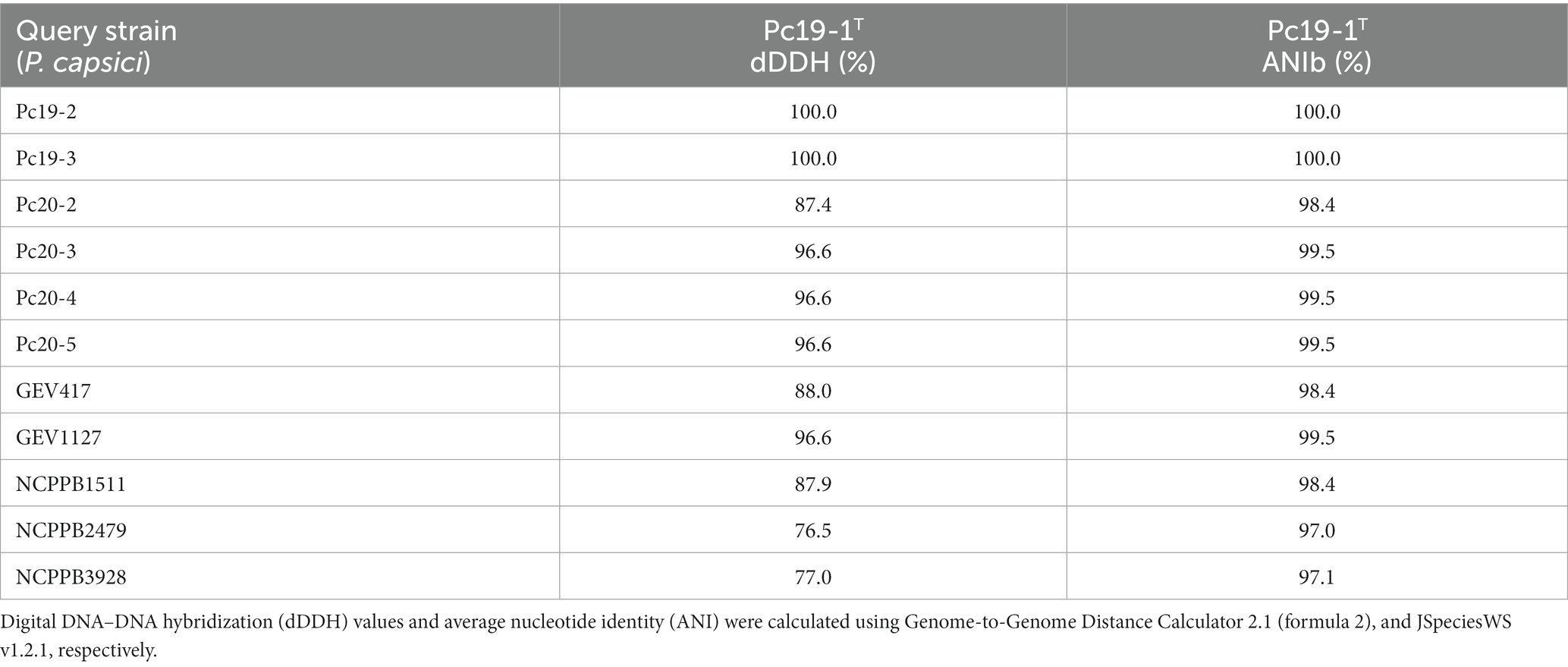
Table 4. Genomic relationship between Pseudomonas capsici strains and the type strain of Pseudomonas capsici Pc19-1T.
The phylogenomic tree, which was generated using the M1CR0B1AL1Z3R web server, is based on the alignment of the core-proteome (4,216 genes) of all 25 P. capsici strains, with P. cichorii ATCC 10857T as an outgroup. This core-proteome tree showed that the Georgia pepper strains Pc19-1T, Pc19-2, and Pc19-3 formed a cluster that was closely related to another cluster consisting of Georgia tomato strains Pc20-3, Pc20-4, Pc20-5, and a tomato strain from Florida GEV1127, with 100% support values (Figure 1). These observations suggest that these strains have a close evolutionary relationship with each other. However, strain Pc20-2, which was isolated from a separate tomato outbreak in Cook County, Georgia, was found to be distinct from other strains, indicating a unique source of introduction and outbreak (Figure 1).
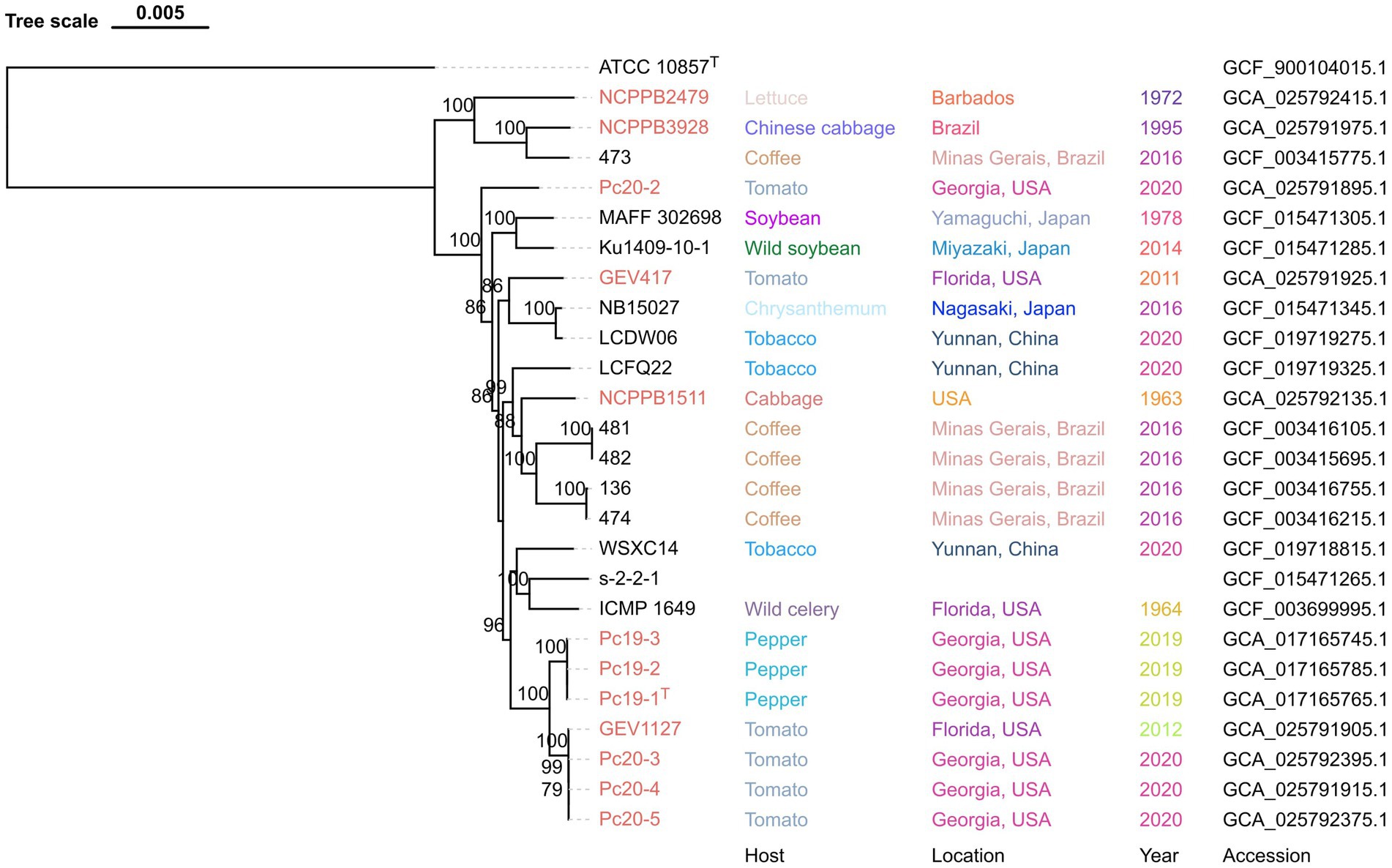
Figure 1. Maximum-likelihood phylogeny of Pseudomonas capsici strains publicly available based on whole genome sequences. Pseudomonas cichorii ATCC 10857T was used as an outgroup. Pseudomonas capsici strains characterized in this study are labeled in red. Bootstrap values >70% are shown as percentages of 1,000 replicates. The scale bar represents nucleotide substitutions per site. Isolation host, location, year, and assembly accession are shown.
3.3. Phylogenetic analysis based on gyrB and rpoD genes
A phylogenetic analysis was conducted using the concatenated sequences of gyrB and rpoD, which had a total length of 961 nucleotides. Fifteen validly described Pseudomonas type strains were included in the analysis, with P. graminis DSM 11363T serving as an outgroup. The resulting phylogenetic tree showed that the strains claimed to be a new phylogroup of P. cichorii, as well as 12 P. capcisi strains whose genomes were sequenced in this project including P. capsici type strain Pc19-1T, clustered together and formed a separate clade from the P. cichorii clade (Figure 2). This clade included P. capsici strains from diverse crops and diverse geographical locations. Additionally, the Georgia tomato strains Pc20-2, Pc20-3, and Pc20-4 were clustered together with the 17 Florida tomato strains isolated in 2012 (Figure 2). Pepper strains from Georgia were clustered together and close to tomato strains.
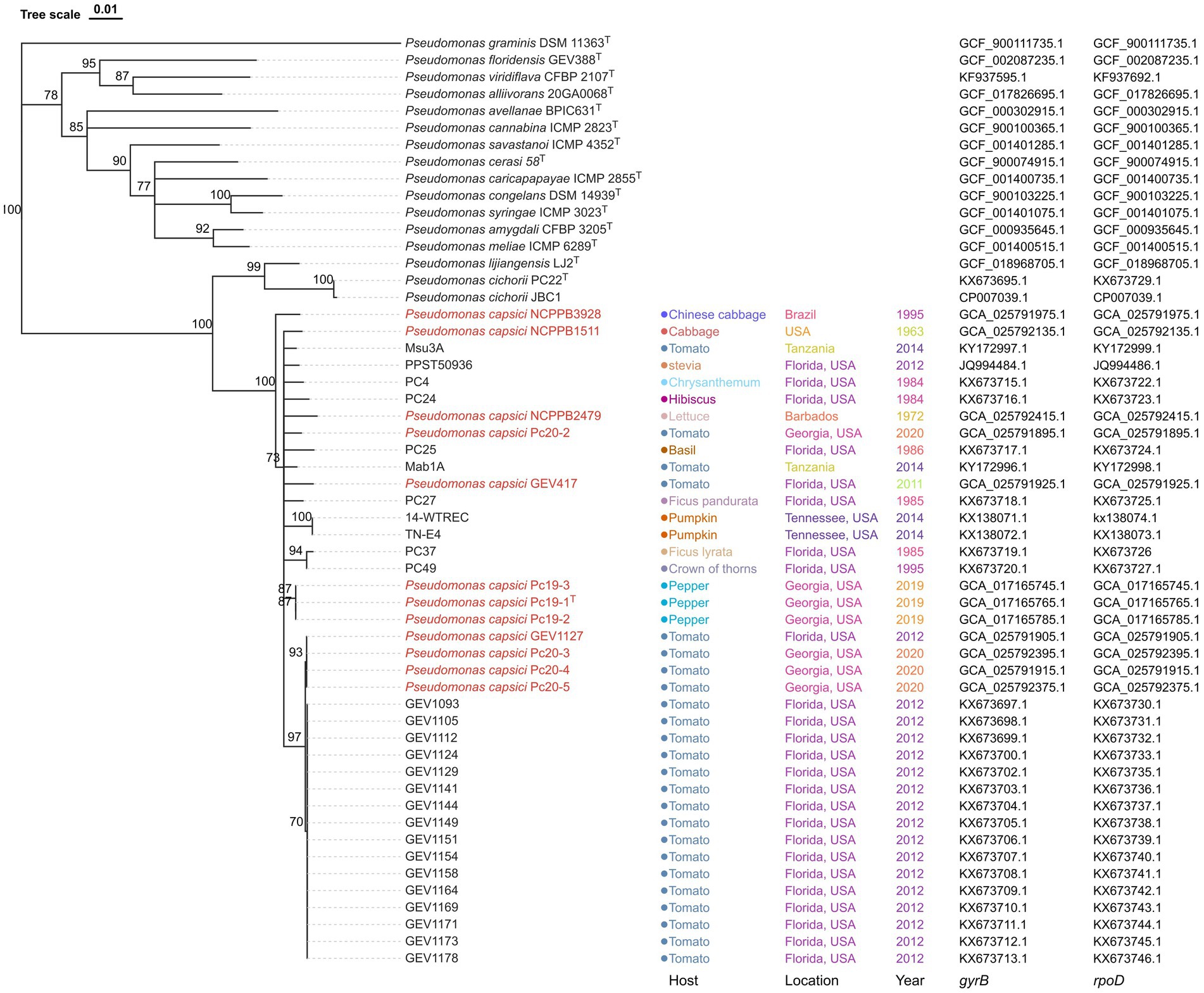
Figure 2. Neighbor-joining phylogeny of Pseudomonas strains using concatenated sequences of gyrB and rpoD genes. Pseudomonas graminis DSM 11363T was used as an outgroup. Pseudomonas capsici strains whose genome has been sequenced are labeled in red. Bootstrap values >70% are shown as percentages of 1,000 replicates. The scale bar represents nucleotide substitutions per site. Isolation host, location, year, gryB accession, and rpoD accession are shown. Pseudomonas capsici strains characterized in this study are labeled in red.
3.4. Host range
The host range of twelve P. capsici strains was evaluated on seven different plant species, including pepper cv. Aristotle, tomato cv. Glacier, Chinese cabbage cv. Rubicon, eggplant cv. Nadia, lettuce cv. Dragoon, broccoli cv. Arcadia F1, and endive cv. Curlesi. All twelve strains induced symptoms on the leaves of pepper, tomato, Chinese cabbage, eggplant, and lettuce, but did not cause any symptoms on broccoli and endive (Table 5). The symptoms observed on the tested hosts were more or less similar with initial water-soaked lesions that gradually turn necrotic. Control plants of each respective host did not exhibit any symptoms. The responses of seedlings were consistent across replicates and experiments. The isolates obtained from artificially inoculated plants were confirmed to be identical to the original inoculated strain through BOX-PCR analysis for DNA fingerprinting comparisons.
3.5. Copper tolerance determination
All 12 tested P. capsici strains were able to grow on NA medium that was not amended with CuSO4· 5H2O. Out of the 12 strains, seven strains were able to grow on NA medium amended with 0.8 mM CuSO4· 5H2O. However, the remaining five P. capsici strains were not able to grow on the same copper amended medium. Analysis of the copper-tolerance related subsystems in RAST revealed that copper tolerance gene clusters are present only in seven copper-tolerant strains (Pc19-1, Pc19-2, Pc19-3, GEV1127, Pc20-3, Pc20-4, and Pc20-5), but are absent in the remaining five strains (NCPPB3928, NCPPB2479, Pc20-2, GEV417, and NCPPB1511). Interestingly, two types of copper tolerance gene clusters were observed in the seven copper-tolerant strains listed above (Figure 3). Three strains (Pc19-1, Pc19-2, and Pc19-3) had identical sequences, while the other four strains (GEV1127, Pc20-3, Pc20-4, and Pc20-5) had identical sequences for their respective aligned region (Figure 3). The pairwise identity of the copper tolerance gene clusters between Pc19-1T and GEV1127 was 77.3%. The copper tolerance gene cluster in strain GEV1127 included four genes that were absent in the Pc19-1T cluster, including a DUF1289 domain-containing protein, a hypothetical protein, a CDF family Co (II) / Ni (II) efflux transporter DmeF, and a metal/formaldehyde-sensitive transcriptional repressor (Figure 3). These copper tolerance gene clusters were not found in other publicly available P. capsici genomes.
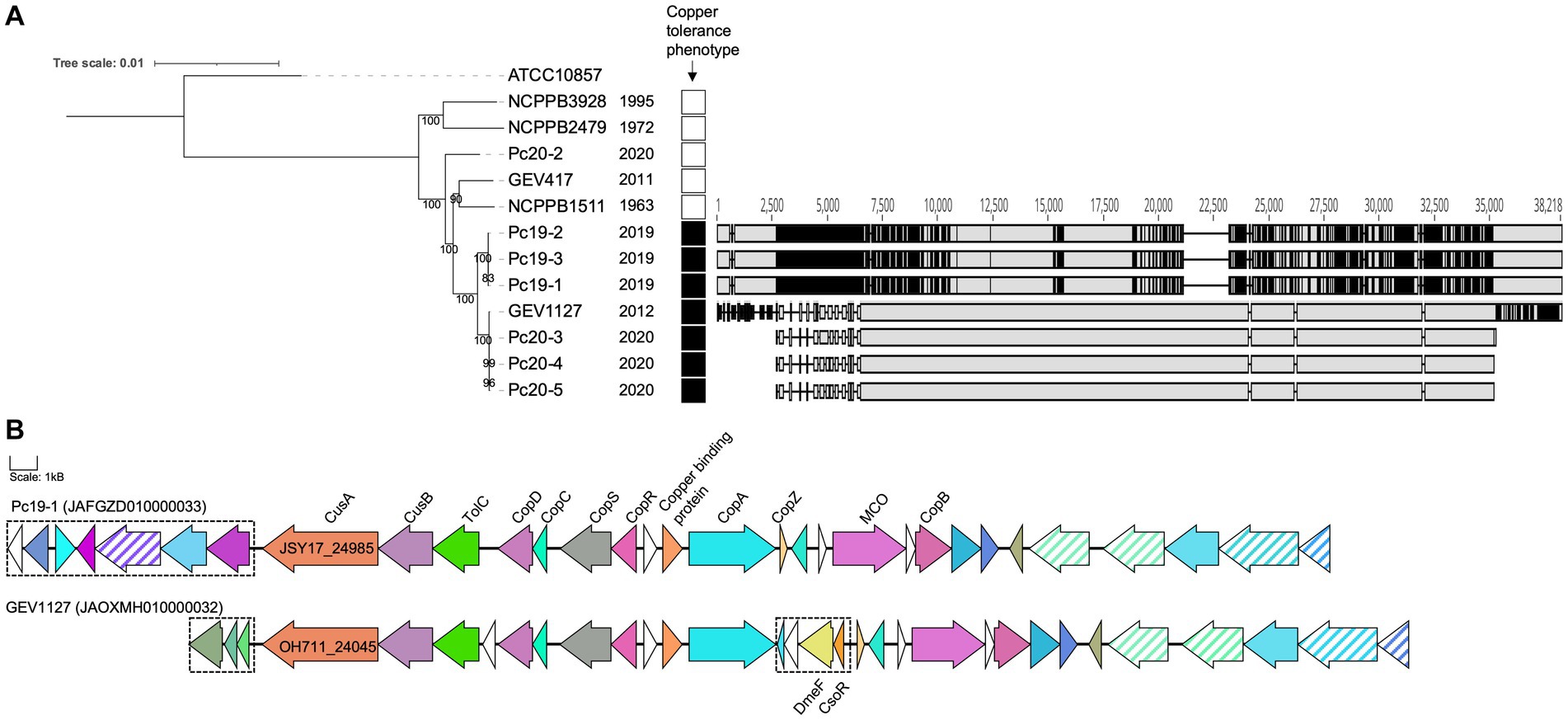
Figure 3. Pseudomonas capsici strains from 2012–2020 are copper tolerant. (A) A maximum likelihood phylogenetic tree based on whole-genome sequences of P. capsici strains and P. cichorii ATCC10857T (as an outgroup). The bootstrap values are shown at the node. Isolation years are shown next to the strain name. Strains that showed growth on medium amended with 0.8 mM CuSO4 were annotated with black squares. The nucleotide alignment of copper-tolerant gene clusters unique in the copper tolerant strains was made using MAFFT in Geneious and shown on the right. Grey color indicates homology, and black color indicates single nucleotide polymorphism positions. (B) The operon maps of the copper tolerant gene clusters unique in P. capsici copper-tolerant strains. Copper tolerance-related gene annotations are labeled. The same colors represent homologous proteins. Transposase genes are in stripes. Differential genes between the clusters of Pc19-1 and GEV1127 are in dashed boxes. The scale represents 1 kB.
3.6. Genome-enabled identification of putative virulence factors and secondary metabolite gene clusters
The P. capsici strains possess an array of secretion systems, including one set of type I secretion system, two sets of type II secretion systems, one set of type III secretion system, two sets of type V secretion systems, three sets of type VI secretion systems (Figure 4). In addition, 10 gene clusters associated with secondary metabolites were identified using antiSMASH and are listed in Table 6. These gene clusters are linked to a variety of products, including a non-ribosomal peptide synthetase (NRPS) and type I polyketide synthase product (similar to yersiniabactin), an NRPS product (similar to cichopeptin), an NRPS product (similar to pyoverdine), an NRPS beta-lactam product (similar to thanamycin), an aryl polyene product, an N-acetylglutaminylglutamine amide product, an NRPS lipopeptide product (similar to cichofactin), a type III polyketide synthase product (similar to fisherindole), a redox-cofactor type product (similar to lankacidin C), and an NRPS product (similar to fragin).
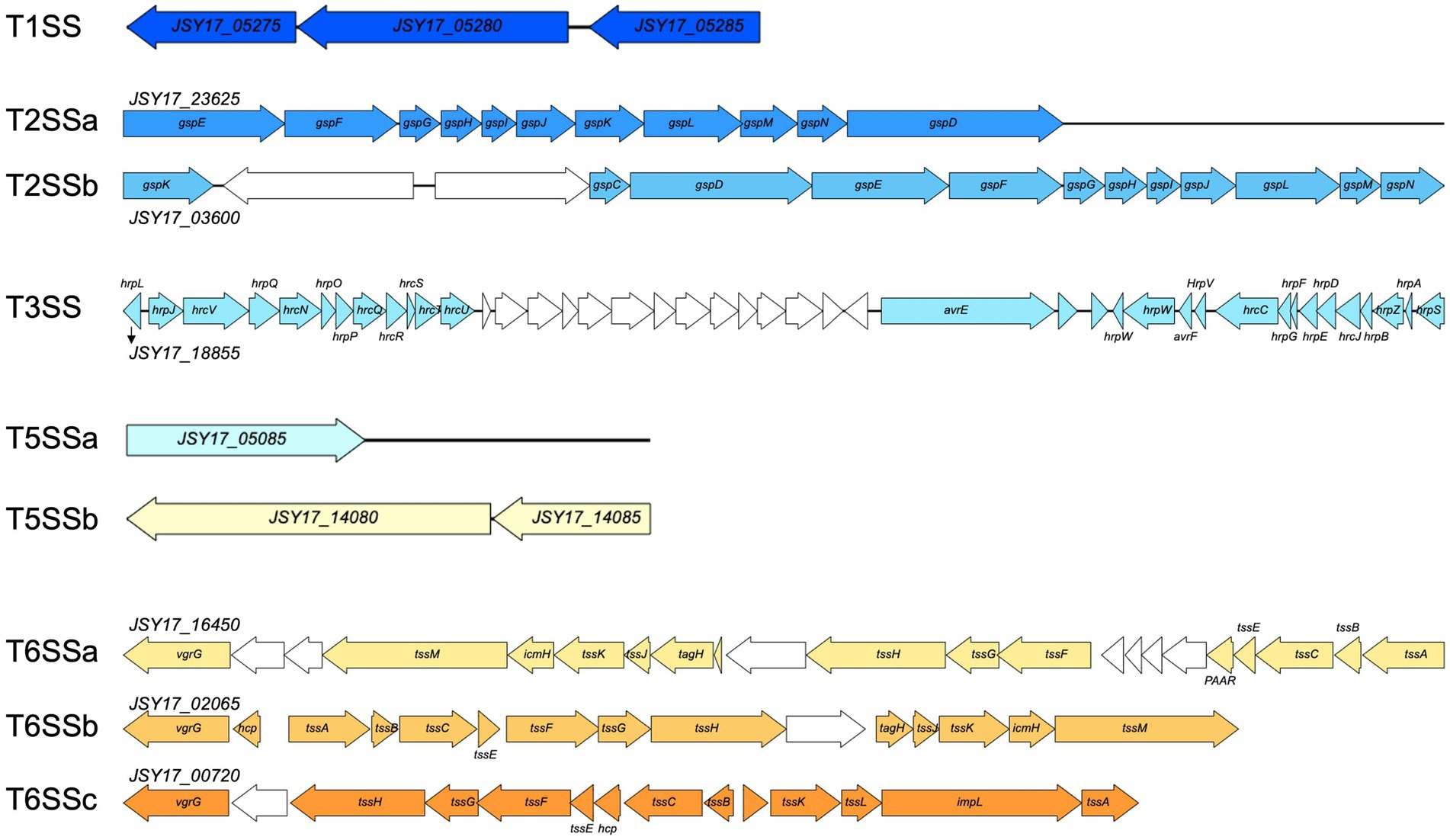
Figure 4. Gene synteny of secretion systems identified in Pseudomonas capsici strain Pc19-1T genome. Colored arrows indicate secretion system related genes, and white arrows indicate genes annotated with hypothetical proteins or other functions.
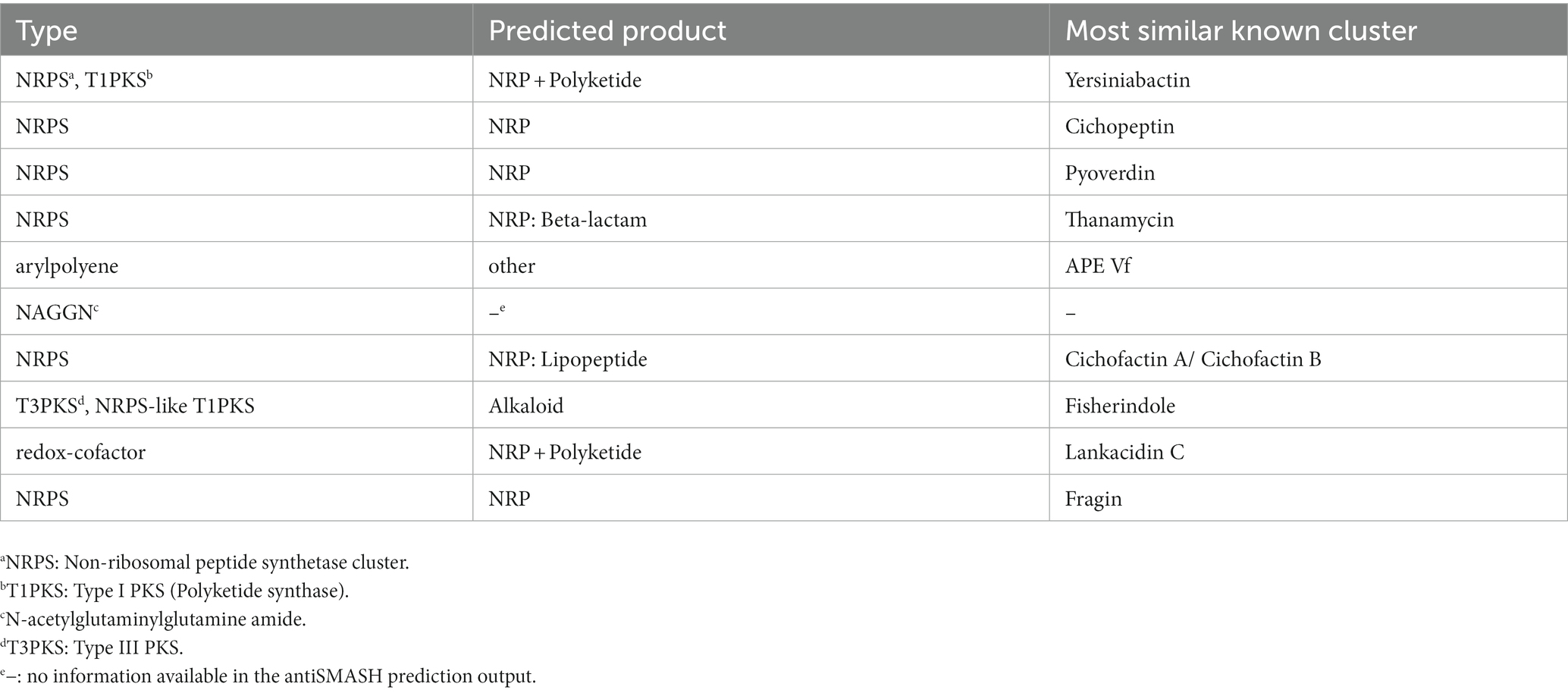
Table 6. Secondary metabolite biosynthetic gene clusters in Pseudomonas capsici strains predicted by antiSMASH v6.
4. Discussion
The genus Pseudomonas is highly diverse, and its taxonomy has undergone many changes over the years. Within the P. syringae species complex, 13 distinct phylogroups have been identified based on the use of multilocus sequence analysis. Pseudomonas cichorii is a bacterial pathogen that can infect a wide range of crops, leading to significant yield losses in commercial agriculture. This pathogen is known to cause leaf spot, blight, and wilting in infected plants (Wilkie and Dye, 2012; Gappa-Adachi et al., 2014; Wang et al., 2022). Phylogenetic analysis based on gyrB and rpoD has placed P. cichorii within the P. syringae complex (Yamamoto et al., 2000) and was later grouped in phylogroup 11 based on multilocus sequence analysis of four housekeeping genes (Berge et al., 2014). In a study by Timilsina et al. (2017), tomato strains from Florida were identified as a novel phylogenetic group of P. cichorii. However, our analysis based on dDDH and ANIb values, as well as phylogenomic analysis, revealed that the previously reported Florida P. cichorii strains GEV417 and GEV1127 were actually P. capsici. Recent advances in high-throughput sequencing technologies have greatly facilitated the analysis of whole-genome sequences of bacterial strains, enabling researchers to infer evolutionary and taxonomic relationships (McAdam et al., 2014). Our analysis has also revealed inaccuracies in the classification of 13 strains in NCBI (473, MAFF 302698, Ku1409-10-1, NB15027, LCDW06, LCFQ22, 481, 482, 136, 474, WSXC14, s-2-2-1, and ICMP 1649), underscoring the importance of prudent species identification. Other examples of error in species designation of Pseudomonas strains are described here. The strain PPST 50936 from stevia (Stevia rebaudiana) in Florida was initially identified as P. cichorii based on the LOPAT scheme and sequence similarity (Strayer et al., 2012). Similarly, strains (14-WTREC and TN-E4) from pumpkin (Cucurbita pepo L. var. pepo) in Tennessee were identified as P. cichorii based on the LOPAT scheme (Newberry et al., 2016). However, our gyrB-rpoD tree analysis indicated that these strains were more closely related to P. capsici than P. cichorii (Figure 2). Therefore, it is likely that these strains may potentially be P. capsici. However, without the whole genome-based dDDH and ANIb analysis, it is difficult to deduce species designation.
Through phylogenomic and phylogenetic analyses (Figures 1, 2), it was discovered that P. capsici strains, despite being newly described, exhibited a broad host range. This was evidenced by their isolation from various sources such as lettuce, Chinese cabbage, coffee (Coffea arabica), tomato, soybean (Glycine max), wild soybean (Glycine soja), chrysanthemum (Chrysanthemum sp.), tobacco (Nicotiana tabacum), wild celery (Apium graveolens), pepper, cabbage (Brassica oleracea), stevia, hibiscus (Hibiscus sp.), basil (Ocimum basilicum), Ficus pandurata, pumpkin, F. lyrata, and crown of thorns (Euphorbia milii). Furthermore, the results of our artificial inoculation assays revealed that 12 P. capsici strains tested were pathogenic on pepper, tomato, Chinese cabbage, eggplant, and lettuce, but non-pathogenic on broccoli and endive. These observations indicate a diverse host range for P. capsici strains across different plant families, which may have practical significance in choosing crops for rotation or for companion cropping systems. However, it is interesting to note that although P. capsici is closely related to P. cichorii it is not pathogenic on endive, which is an original source of isolation for the P. cichorii type strain (ATCC 10857T).
Copper-based sprays have been extensively utilized in agriculture for many years for the control of bacterial and fungal diseases. As a result copper-tolerance have been reported in bacterial pathogens affecting multiple agricultural crops including pepper, tomato, and apple. The presence of these copper-tolerant bacterial strains reduces the efficacy of copper-based bactericides (Marco and Stall, 1983; Martin et al., 2004; Strayer-Scherer et al., 2018). Our research shows that all Georgia P. capsici strains from pepper and three out of four Georgia P. capsici strains from tomato exhibited copper tolerance in-vitro. The copper-tolerant strains possess at least two different types of copper-tolerant gene clusters, which were absent in the copper-sensitive P. capsici strains. The copper-tolerant strains were predominantly from the recent outbreaks of pepper and tomato in Georgia (2012, 2019, and 2020), but not from outbreaks in or before 2011. The acquisition of these clusters probably provided a selective advantage to these strains resulting in recent outbreaks that were difficult to manage with a standard copper-based spray program.
We also observed two distinct copper-tolerant gene clusters in seven copper-tolerant P. capsici strains. This may suggest that the sources of the copper tolerance in tomato and pepper strains are distinct. The genes in both clusters encode for efflux RND transporter permease subunit, efflux RND transporter periplasmic adaptor subunit, copper homeostasis membrane protein CopD, copper homeostasis periplasmic binding protein CopC, heavy metal sensor histidine kinase, heavy metal response regulator transcription factor, heavy metal translocating P-type ATPase, cation transporter, copper resistance system multicopper oxidase, and copper resistance protein B. The specific contributions of the individual genes within these clusters remain unclear. Further research is also necessary to understand the regulation of these genes and the distribution of these genes in general P. capsici populations.
The analysis of the whole genomes of P. capsici strains has revealed the presence of several virulence factors and secondary metabolites that may enable them to infect plant tissues and evade plant defense mechanisms. However, mere presence of these factors does not associate them with virulence unless they are validated using mutational and functional analysis. Future studies will focus on characterizing these potential virulence factors in pepper and tomato. The genus Pseudomonas is widely distributed and plays a crucial role in environmental processes (Cornelis, 2008). In addition to their ecological significance, Pseudomonas species are renowned for their ability to produce a vast array of biologically active secondary metabolites (Gross and Loper, 2009). The production of phytotoxins by members of the P. syringae species complex has been shown to contribute to virulence, including phytotoxins like syringomycin, syringopeptin, and cichofactin. We hypothesize that multiple virulence factors including the type III secretion system and cichofactin product may provide a competitive advantage for infecting diverse hosts. Future studies will focus on this aspect as well. Overall, this is the first study to characterize P. capsici strains genotypically and phenotypically from tomato and compared them with strains isolated from diverse hosts. Also, host-range, copper-tolerance and presence and absence of potential virulence factors in P. capsici were among some of the novel findings in this manuscript.
5. Conclusions
Our study experimentally demonstrated that P. capsici strains were pathogenic on pepper and tomato. The strains studied were pathogenic on multiple hosts under experimental conditions. Our findings indicate that this bacterium can potentially be a challenging plant pathogen to manage as it can infect a wide range of host plants and can potentially cause significant damage to agricultural crops. Although the potential mechanism of pathogen survival and dissemination are yet to be determined, it is imperative that future studies should focus on this aspect to assess risks of wide-spread outbreaks. To minimize the impact of P. capsici, it is essential that measures are taken to identify and exclude sources of inoculum, and monitor and control their reoccurrences. Furthermore, it is imperative that we continue to investigate the biology and disease cycle of this pathogen. This knowledge will help us to develop sustainable and effective management strategies that will aid in reducing losses due to this bacterial pathogen.
Data availability statement
The datasets presented in this study can be found in online repositories. The names of the repository/repositories and accession number(s) can be found at: https://www.ncbi.nlm.nih.gov/genbank/, BioProject PRJNA890938.
Author contributions
MZ: Conceptualization, Data curation, Formal analysis, Investigation, Methodology, Validation, Writing – original draft, Writing – review & editing. RG: Conceptualization, Writing – review & editing. BD: Conceptualization, Writing – review & editing, Data curation, Formal analysis, Funding acquisition, Investigation, Methodology, Project administration, Resources, Supervision, Validation, Visualization, Writing – original draft.
Funding
The author(s) declare financial support was received for the research, authorship, and/or publication of this article. This work was supported by GACCV AWD0001296901, and the Specialty Crops Research Initiative Awards 2019–51181-30010 and 2022–51181-38242 and from the USDA National Institute of Food and Agriculture. Any opinions, findings, conclusions, or recommendations expressed in this publication are those of the author(s) and do not necessarily reflect the view of the U.S. Department of Agriculture. The University of Georgia is an equal opportunity provider and employer. This study was supported in part by resources and technical expertise from the Georgia Advanced Computing Resource Center, a partnership between the University of Georgia Office of the Vice President for Research and Office of the Vice President for Information Technology.
Acknowledgments
The authors would like to thank Gary Vallad (University of Florida) for providing strains GEV417 and GEV1127.
Conflict of interest
The authors declare that the research was conducted in the absence of any commercial or financial relationships that could be construed as a potential conflict of interest.
The author(s) declared that they were an editorial board member of Frontiers, at the time of submission. This had no impact on the peer review process and the final decision.
Publisher’s note
All claims expressed in this article are solely those of the authors and do not necessarily represent those of their affiliated organizations, or those of the publisher, the editors and the reviewers. Any product that may be evaluated in this article, or claim that may be made by its manufacturer, is not guaranteed or endorsed by the publisher.
Footnotes
References
Abby, S. S., Cury, J., Guglielmini, J., Néron, B., Touchon, M., and Rocha, E. P. (2016). Identification of protein secretion systems in bacterial genomes. Sci. Rep. 6:23080. doi: 10.1038/srep23080
Abby, S. S., Neron, B., Menager, H., Touchon, M., and Rocha, E. P. (2014). MacSyFinder: a program to mine genomes for molecular systems with an application to CRISPR-Cas systems. PLoS One 9:e110726. doi: 10.1371/journal.pone.0110726
Avram, O., Rapoport, D., Portugez, S., and Pupko, T. (2019). M1CR0B1AL1Z3R—a user-friendly web server for the analysis of large-scale microbial genomics data. Nucleic Acids Res. 47, 88–92. doi: 10.1093/nar/gkz423
Bankevich, A., Nurk, S., Antipov, D., Gurevich, A. A., Dvorkin, M., Kulikov, A. S., et al. (2012). SPAdes: a new genome assembly algorithm and its applications to single-cell sequencing. J. Comput. Biol. 19, 455–477. doi: 10.1089/cmb.2012.0021
Berge, O., Monteil, C. L., Bartoli, C., Chandeysson, C., Guilbaud, C., Sands, D. C., et al. (2014). A user’s guide to a data base of the diversity of Pseudomonas syringae and its application to classifying strains in this phylogenetic complex. PLoS One 9:e105547. doi: 10.1371/journal.pone.0105547
Blin, K., Shaw, S., Kloosterman, A. M., Charlop-Powers, Z., van Wezel, G. P., Medema, M. H., et al. (2021). antiSMASH 6.0: improving cluster detection and comparison capabilities. Nucleic Acids Res. 49, 29–35. doi: 10.1093/nar/gkab335
Bonn, W., Gitaitis, R., and MacNeil, B. (1985). Epiphytic survival of Pseudomonas syringae pv. Tomato on tomato transplants shipped from Georgia. Plant Dis. 69, 58–60. doi: 10.1094/PD-69-58
Brusetti, L., Malkhazova, I., Gtari, M., Tamagnini, I., Borin, S., Merabishvili, M., et al. (2008). Fluorescent-BOX-PCR for resolving bacterial genetic diversity, endemism and biogeography. BMC Microbiol. 8:220. doi: 10.1186/1471-2180-8-220
Chen, S., Zhou, Y., Chen, Y., and Gu, J. (2018). fastp: an ultra-fast all-in-one FASTQ preprocessor. Bioinformatics 34, 884–890. doi: 10.1093/bioinformatics/bty560
Cornelis, P. (2008). Pseudomonas: Genomics and molecular biology. Caister Academic Press, Norwich, United Kingdom.
Gappa-Adachi, R., Morita, Y., Shimomoto, Y., and Shigeharu, T. (2014). Bacterial leaf blight of sweet pepper (Capsicum annuum) caused by Pseudomonas cichorii in Japan. J. Gen. Plant Pathol. 80, 103–107. doi: 10.1007/s10327-013-0491-1
Gitaitis, R., Jones, J., Jaworski, C., and Phatak, S. (1985). Incidence and development of Pseudomonas syringae pv. Syringae on tomato transplants in Georgia. Plant Dis. 69, 32–35.
Gitaitis, R. D., Sasser, M., Beaver, R. W., Mcinnes, T. B., and Stall, R. E. (1987). Pectolytic Xanthomonads in mixed infections with Pseudomonas syringae pv. Syringae, P. syringae pv. Tomato, and Xanthomonas compestris pv. Vesicatoria in tomato and pepper transplants. Phytopathology 77, 611–615. doi: 10.1094/Phyto-77-611
Gross, H., and Loper, J. E. (2009). Genomics of secondary metabolite production by Pseudomonas spp. Nat. Prod. Rep. 26, 1408–1446. doi: 10.1039/b817075b
Harrison, K. J., Crécy-Lagard, V. D., and Zallot, R. (2018). Gene graphics: a genomic neighborhood data visualization web application. Bioinformatics 34, 1406–1408. doi: 10.1093/bioinformatics/btx793
Jones, J., Gitaitis, R., and McCarter, S. (1986). Fluorescence on single-carbon sources for separation of Pseudomonas syringae pv. Syringae, P. syringae pv. Tomato, and P. viridiflava on tomato transplants. Plant Dis. 70, 151–153. doi: 10.1094/PD-70-151
Jones, J., McCarter, S. M., and Gitaitis, R. (1981). Association of Pseudomonas syringae with a leaf spot disease of tomato transplants in southern Georgia. Phytopathology 71, 1281–1285.
Katoh, K., and Standley, D. M. (2013). MAFFT multiple sequence alignment software version 7: improvements in performance and usability. Mol. Biol. Evol. 30, 772–780. doi: 10.1093/molbev/mst010
Lelliott, R. A., Billing, E., and Hayward, A. C. (1966). A determinative scheme for the fluorescent plant pathogenic pseudomonads. J. Appl. Bacteriol. 29, 470–489. doi: 10.1111/j.1365-2672.1966.tb03499.x
Marco, G. M., and Stall, R. E. (1983). Control of bacterial spot of pepper initiated by strains of Xanthomonas campestris pv. Vesicatoria that differ in sensitivity to copper. Plant Dis. 67, 779–781. doi: 10.1094/PD-67-779
Martin, H. L., Hamilton, V. A., and Kopittke, R. A. (2004). Copper tolerance in Australian populations of Xanthomonas campestris pv. Vesicatoria contributes to poor field control of bacterial spot of pepper. Plant Dis. 88, 921–924. doi: 10.1094/PDIS.2004.88.9.921
McAdam, R. P., Richardson, J. E., and Fitzgerald, R. J. (2014). High-throughput sequencing for the study of bacterial pathogen biology. Curr. Opin. Microbiol. 19, 106–113. doi: 10.1016/j.mib.2014.06.002
McCarter, S. M., Jones, J. B., Gitaitis, R. D., and Smitley, D. R. (1983). Survival of Pseudomonas syringae pv. Tomato in association with tomato seeds, soil, host tissue, and epiphytic weed hosts in Georgia. Phytopathology 73, 1393–1398. doi: 10.1094/Phyto-73-1393
Meier-Kolthoff, J. P., Auch, A. F., Klenk, H.-P., and Göker, M. (2013). Genome sequence-based species delimitation with confidence intervals and improved distance functions. BMC Bioinformatics 14, 1–14. doi: 10.1186/1471-2105-14-60
Meier-Kolthoff, J. P., and Göker, M. (2019). TYGS is an automated high-throughput platform for state-of-the-art genome-based taxonomy. Nat. Commun. 10, 1–10. doi: 10.1038/s41467-019-10210-3
Newberry, E. A., Paret, M. L., Jones, J. B., and Bost, S. C. (2016). First report of leaf spot of pumpkin caused by Pseudomonas cichorii in Tennessee. Plant Dis. 100:2159. doi: 10.1094/PDIS-11-15-1387-PDN
Overbeek, R., Olson, R., Pusch, G. D., Olsen, G. J., Davis, J. J., Disz, T., et al. (2014). The SEED and the rapid annotation of microbial genomes using subsystems technology (RAST). Nucleic Acids Res. 42, 206–D214. doi: 10.1093/nar/gkt1226
Richter, M., Rosselló-Móra, R., Oliver Glöckner, F., and Peplies, J. (2016). JSpeciesWS: a web server for prokaryotic species circumscription based on pairwise genome comparison. Bioinformatics 32, 929–931. doi: 10.1093/bioinformatics/btv681
Searcy, J. V. K., Smith, S., Gitaitis, R. D., and Dutta, B. (2015). First report of tomato pith necrosis caused by Pseudomonas mediterranea in Georgia, USA. Plant Dis. 100:518. doi: 10.1094/PDIS-07-21-1434-PDN
Stice, S., Stumpf, S., Gitaitis, R. D., Kvitko, B. H., and Dutta, B. (2018). Pantoea ananatis genetic diversity analysis reveals low core genome diversity and accessory genes correlated with onion pathogenicity. Front. Microbiol. 9:184. doi: 10.3389/fmicb.2018.00184
Strayer, A., Garcia-Maruniak, A., Sun, X., Schubert, T., and Sutton, B. (2012). First report of Pseudomonas cichorii causing leaf spot of Stevia detected in Florida. Plant Dis. 96:1690. doi: 10.1094/PDIS-06-12-0575-PDN
Strayer-Scherer, A., Liao, Y. Y., Young, M., and Paret, M. L. (2018). Advanced copper composites against copper-tolerant Xanthomonas perforans and tomato bacterial spot. Phytopathology 2, 196–205. doi: 10.1094/PHYTO-06-17-0221-R
Tian, L., Huang, C., Mazloom, R., Heath, L. S., and Vinatzer, B. A. (2020). LINbase: a web server for genome-based identification of prokaryotes as members of crowdsourced taxa. Nucleic Acids Res. 48, 529–537. doi: 10.1093/nar/gkaa190
Timilsina, S., Adkison, H., Testen, A. L., Newberry, E. A., Miller, S. A., Paret, M. L., et al. (2017). A novel phylogroup of Pseudomonas cichorii identified following an unusual disease outbreak on tomato. Phytopathology 107, 1298–1304. doi: 10.1094/PHYTO-05-17-0178-R
Voloudakis, A. E., Gitaitis, R. D., Westbrook, J. K., Phatak, S. C., and Mccarter, S. M. (1991). Epiphytic survival of Pseudomonas syringae pv. Syringae and P. s. tomato on tomato transplants in southern Georgia. Plant Dis. 75, 672–675. doi: 10.1094/PD-75-0672
Walcott, R. R., Fessehaie, A., and Castro, A. C. (2004). Differences in pathogenicity between two genetically distinct groups of Acidovorax avenae subsp. citrulli on cucurbit hosts. J. Phytopathol. 152, 277–285. doi: 10.1111/j.1439-0434.2004.00841.x
Wang, F. F., Tang, T., Mao, T., Guo, X. L., Duan, Y. Y., and You, J. M. (2022). Pseudomonas cichorii causing leaf spot disease on Banxia (Pinellia ternata) in China. Plant Dis. 106:3198. doi: 10.1094/PDIS-01-22-0060-PDN
Wilkie, J. P., and Dye, D. W. (2012). Pseudomonas cichorii causing tomato and celery diseases in New Zealand. New Zealand J. Agric. Res. 17, 123–130. doi: 10.1094/PD-69-32
Xie, J. M., Chen, Y. R., Cai, G. J., Cai, R. L., Hu, Z., and Wang, H. (2023). Tree visualization by one table (tvBOT): a web application for visualizing, modifying and annotating phylogenetic trees. Nucleic Acids Res. 51, 587–592. doi: 10.1093/nar/gkad359
Yamamoto, S., Kasai, H., Arnold, D. L., Jackson, R. W., Vivian, A., and Harayama, S. (2000). Phylogeny of the genus Pseudomonas: intrageneric structure reconstructed from the nucleotide sequences of gyrB and rpoD genes. Microbiology 146, 2385–2394. doi: 10.1099/00221287-146-10-2385
Keywords: Pseudomonas capsici , ANI, dDDH, copper, host range, effector
Citation: Zhao M, Gitaitis R and Dutta B (2023) Characterization of Pseudomonas capsici strains from pepper and tomato. Front. Microbiol. 14:1267395. doi: 10.3389/fmicb.2023.1267395
Edited by:
Mohammad Arif, University of Hawaii at Manoa, United StatesReviewed by:
Nagaraju Yalavarthi, National Bureau of Agriculturally Important Microorganisms (ICAR), IndiaRolf Kümmerli, University of Zurich, Switzerland
Copyright © 2023 Zhao, Gitaitis and Dutta. This is an open-access article distributed under the terms of the Creative Commons Attribution License (CC BY). The use, distribution or reproduction in other forums is permitted, provided the original author(s) and the copyright owner(s) are credited and that the original publication in this journal is cited, in accordance with accepted academic practice. No use, distribution or reproduction is permitted which does not comply with these terms.
*Correspondence: Bhabesh Dutta, YmhhYmVzaEB1Z2EuZWR1