- 1Department of Pathobiology, Ontario Veterinary College, University of Guelph, Guelph, ON, Canada
- 2Bristol Veterinary School, University of Bristol, Langford, Bristol, United Kingdom
Alterations in intestinal microbiota can modulate the developing avian intestinal immune system and, subsequently, may impact on resistance to enteric pathogens. The aim was to demonstrate that early life exposure to Lactococcus lactis, could affect either susceptibility or resistance of broilers to necrotic enteritis (NE). L. lactis NZ9000 (rL. lactis) pre-treatment at 1, 7, 14 and 21 days of age (DOA) led to a significant decrease in NE lesion scores in Clostridium perfringens infected chickens. C. perfringens Infection was associated with spatial and temporal decreases in mononuclear phagocytes and CD4+ αβ T cells. However, rL. Lactis pre-treatment and subsequent C. perfringens infection led to a significant increase in mononuclear phagocytes, CD8α + γδ T, αβ T cells (CD4+ and CD8α+) and B cells (IgM+, IgA+ and IgY+), as well as IL-12p40, IFN-γ and CD40. Differential expression of interleukin (IL)-6, IL-8, IL-10, IL-13, IL-18, IL-22, and transforming growth factor (TGF)-β were observed in L. lactis treated chickens when compared to C. perfringens infected chickens. Microbiota analysis in C. perfringens infected chickens demonstrated an increase in abundance of Bacillota, Bacteroidota, Pseudomonadota and Actinomycetota. These findings suggests that modulation of the chicken intestinal immune system by L. lactis confers partial protection 30 against NE.
1 Introduction
Interactions between the microbiota and intestinal cells play a significant role in shaping the intestinal immune system (Rescigno, 2011). Infections with various enteric pathogens during early life can disrupt the development of normal microbial communities, hence may impact the formation of immune responses (Mowat, 2003; Rescigno, 2011). In broiler chickens, Eimeria mediated enteropathy, facilitates secondary bacterial infections (Park et al., 2008b; Macdonald et al., 2017). Specifically, Clostridium perfringens expressing an array of toxins (α-toxin, necrotic enteritis B-like toxin; NetB and TpeL) exacerbates the primary inflammatory process thereby leading to disruption of intestinal epithelial cells tight junctions (Baba et al., 1997; van Immerseel et al., 2004; Wise and Siragusa, 2005; Uzal et al., 2014; Alizadeh et al., 2021). The resulting effect is loss of intestinal epithelial barrier integrity, and progression of necrotic enteritis (NE) ulcerative lesions (Parish, 1961; Fukata et al., 1988). Presence of C. perfringens cells in combination with various cofactors are usually required to promote overgrowth of C. perfringens in the intestinal tract (Keyburn et al., 2008; Shojadoost et al., 2012; Wang et al., 2019). Modulation of the intestinal mucosa is a determinant of virulent C. perfringens pathogenicity (Shojadoost et al., 2012; Wang et al., 2019).
While there is no clear consensus in the manner in which C. perfringens mediates enteropathy, some contributors to its pathogenesis have already been defined (Keyburn et al., 2008; Park et al., 2008b; Stanley et al., 2012; Huang et al., 2018; Lee et al., 2018). Sensing of C. perfringens pathogen-associated molecular patterns (PAMPs) by Toll-like receptor (TLR)2, TLR4 and TLR9 is thought to mediate the proceeding intestinal inflammatory processes (Lu et al., 2009; Shojadoost et al., 2012; Kamada et al., 2013; Oh et al., 2019). The subsequent inflammatory processes, based on the increased expression of interleukin (IL)-10, IL-13, and IL-17, have guided our early understanding of C. perfringens immune modulatory activity (Park et al., 2008a,b; Lu et al., 2009). Therefore, expression of these cytokines can be used as markers to define any gut microbes that could contribute to or antagonize C. perfringens cellular proliferation and induction of NE.
Beneficial bacteria, including lactic acid producing bacteria (LAB), have been used as probiotics for control of enteric pathogens in chickens, including NE (Shojadoost et al., 2022). Among LAB, Lactococcus lactis strains are of note. In humans, extensive usage of L. lactis expressing nisin as a live probiotic ingredient in yogurt preparations has garnered the designation of generally recognized as safe (GRAS) (Bermúdez-Humarán et al., 2011). Moreover, in chickens, nisin knockout L. lactis strains have been used as a recombinant oral vaccine vector against various respiratory pathogens (Song et al., 2017; Lahiri et al., 2019). Importantly, there is no evidence to suggest that L. lactis cremoris strain (NZ9000), which is increasingly used for oral inoculation and expression of recombinant proteins, could contribute to enteropathy in chickens (Cao et al., 2013; Song et al., 2017).
Since lactic acid producing bacteria can modulate the development and effector function of the intestinal immune system through a variety of mechanisms, including pattern recognition receptors (PRR) (Mowat and Agace, 2014), the present study aimed to evaluate the effects of oral administration of L. lactis on the chicken gut associated lymphoid tissue (GALT). Further, the safety and efficacy of L. lactis was assessed in chickens infected with a virulent C. perfringens strain (CP1 isolate).
2 Materials and method
2.1 Animals and ethics statement
One-day-old mixed sex Cobb broilers (n = 120) purchased from a local producer (Curtis chicks, a division of Maple Lodge hatcheries, Guelph, Ca) were group housed throughout the experiment in specific-pathogen-free filtered air positive pressure rooms on floor pens with wood shaving. All chickens had ad libitum access to water and commercial feed. All animal experiments were approved (028–10,783 – ISOL and AUP 4328) by the Animal Care Committee of the University of Guelph and adhered to the guidelines for the use of animals. Experiments and analyses were performed according to the ARRIVE guidelines.
2.2 Bacterial strains
(i) Lactococcus lactis subsp. cremoris strain (NZ9000 strain from MoBiTec GmbH, Germany), a derivate of L. lactis subsp. cremoris MG1363 with regulatory genes (nisR, nisK) integrated into the pepN gene of MG1363 were cultured (M17 broth; Gibco, Ca) and maintained in anaerobic atmosphere (30°C and no shaking using a gas pack).
(ii) Recombinant L. lactis (rL. lactis): L. lactis were cultured for 2 days in G/L-SGM17B medium (M17-Broth with 0.5 M sucrose, 2.5% glycine and 0.5% glucose), washed and prepared for electroporation. L. lactis NZ9000 cells were transformed with the pNZ8124 plasmid (1 μg/mL; MoBiTec. NICE® expression system for Lactococcus lactis handbook. 20151) by electroporation (0.2 cm Gene Pulser Electroporation Cuvettes; Bio-Rad, On, Canada) as recommended by the manufacturer; 2000 V, 25 μF and 200 Ω with a resulting pulse of 5 msec. Post electroporation, cells were incubated on ice for 5 min and cultured in G/L-M17B (G/L-SGM17B supplemented with 20 mM MgCl2 and 2 mM CaCl2) for 2 h at 30°C. Transformed cells were subsequently streaked on M17 agar supplemented with 3 μg/mL of chloramphenicol and colonies allowed to grow for 48 h. Transformants were confirmed by plasmid DNA extraction and gel electrophoresis. rL. lactis were utilized for subsequent experiments to facilitate bacterial enumeration. rL. lactis starter and expansion cultures were maintained (30°C and no shaking) in M17 broth supplemented with 3 μg/mL of chloramphenicol. The cells were recovered by centrifugation (8,000 × g for 15 min at 4°C) and resuspended in 1 mL of PBS. The rL. lactis was prepared fresh on the day of inoculation.
(iii) The netB positive C. perfringens, CP1 isolate, strain used in this study had been generously provided by Dr. John Prescott (University of Guelph, On, Canada) (Chalmers et al., 2008). Single colonies as selected on blood agar were used to establish stock cultures. Overnight starter cultures (37°C in aerobic condition for 15 h) of the pathogenic C. perfringens, CP1 isolate, strain in Cooked Meat Medium (ThermoFisher Scientific, Canada) were expanded in 3% fluid thioglycollate medium (FTG; Millipore-Sigma, Canada) and incubated at 37°C in anaerobic condition for a further 15 h.
2.3 Experimental design and sampling
(i) rL. lactis treatment: One hundred and twenty-one-day-old chicks were randomly allocated into individual groups and 91-day old chicks were subsequently inoculated by oral gavage with 500 μL of the rL. lactis (1.0 × 108 CFU) in PBS (n = 60) or PBS alone (n = 60). Boiler chicks were again inoculated by oral gavage on day 8, 15 and day 21 of age with 500 μL of the rL. lactis (1.0 × 108 CFU) in PBS or PBS alone. The dosage of L. lactis utilized in this study were optimized in a pilot study which assessed the safety and dosage and frequency of application. No effects were observed at 1.0 × 107 and no additive effects were observed at 10 × 109 CFU against NE lesions.
(ii) Production of NE: One week before challenge, the starter diet was replaced with a wheat-based, high protein diet containing 15% fish meal (30% crude protein). In brief, on the day of challenge, the optical density of propagated C. perfringens cultures was measured by spectrophotometer at 600 nm and inoculum were adjusted to 3.0 × 108 CFU and stored on ice. Sixty chickens that were pre-treated with the rL. lactis (1.0 × 108 CFU) in PBS (n = 30) or PBS alone (n = 30) were infected by oral gavage twice daily for 3 days (21 to 23 days of age: DOA). At 24 DOA, all chickens were euthanized, and lesion scoring, based on the criteria listed in Table 1, was performed as previously described (Shojadoost et al., 2012).
(iii) Sampling: Five to six broiler chicks were euthanized on a weekly basis from both rL. lactis and PBS pre-treated chickens for 4 weeks and intestinal tissue samples were collected from the duodenum, jejunum, ileum and cecal tonsils and stored either in ice cold PBS containing penicillin (10 U/mL), and streptomycin (10 μg/mL) for further processing or in RNAlater (Millipore-Sigma, Canada) and subsequently frozen at –80°C. Intestinal contents from the respective intestinal segments were also collected on a weekly basis and frozen at –80°C.
2.4 Enumeration of Lactococcus lactis
One-hundred milligrams of duodenum, jejunum and ileum intestinal contents, as collected on a weekly basis (24 h post rL. lactis inoculation) from five to six chickens, were dilute in 0.5 mL of ice-cold PBS. Mixtures were thoroughly vortexed and subsequently centrifuged (400 × g for 5 min). Supernatants were collected and tenfold serial dilution were performed in PBS. One hundred microliters of the dilution series were plated in triplicates on M17 agar supplemented with 5 μg/mL of chloramphenicol (Millipore-Sigma, Canada). Colonies were allowed to grow for 24 h in an anaerobic atmosphere (30°C and no shaking using a gas pack). The next day, colonies were counted to estimate recovery and persistence of rL. lactis.
2.5 Intestinal tissue mononuclear cell preparation
Five-centimeter segments of the medial duodenum, jejunum, ileum and whole cecal tonsils were harvested from chickens and stored on ice in PBS containing penicillin (10 U/mL), and streptomycin (10 μg/mL). Each tissue was cut into 0.5 cm2 segments and washed vigorously three times with PBS containing penicillin (10 U/mL), and streptomycin (10 μg/mL). Tissue samples were subsequently digested (collagenase type 1; 80 U/mL at 37°C for 20 min; Millipore-Sigma, Canada) in PBS containing penicillin (10 U/mL), and streptomycin (10 μg/mL). Whole tissue digests were applied onto 40-μm BD cell strainers (BD Biosciences, Canada), and crushed through using the rubber end of a 10 mL syringe plunger (Boodhoo et al., 2016). Duodenum, jejunum, ileum and cecal tonsils cell suspension were prepared by layering (2:1) onto histopaque 1,070 (Millipore-Sigma, Canada) density-gradient centrifugation and centrifuged at 2100 rpm (600 × G) for 20 min to allow the separation of mononuclear cells. Aspirated buffy coats were washed at 1500 rpm (400 × G) for 5 min in RPMI 1640 with penicillin (10 U/mL), and streptomycin (10 μg/mL). Mononuclear cells were suspended in complete RPMI cell culture medium; RPMI 1640 medium containing 10% fetal bovine serum (Millipore-Sigma, Canada), penicillin (10 U/mL), and streptomycin (10 μg/mL). Cell number and viability were calculated using a hemocytometer, and trypan blue exclusion method. Mononuclear cells were suspended in complete RPMI cell culture medium at a density of 5 × 106 cells/ml and kept on ice.
2.6 Flow cytometry
Following a wash in FACS staining buffer (PBS with 0.2% BSA), duodenum, jejunum, ileum and cecal tonsils mononuclear cells, 5.0 × 105 cells per well in triplicates, were counter stained in specific panels as APC (mouse anti-Kul01-FITC and mouse anti-major histocompatibility complex (MHC) II-PE), T cell (mouse anti-CD3ζ-PB, mouse anti-CD4-PE-CY7, mouse anti-CD8α-FITC and mouse anti-γδTCR-PE) and B cell (mouse anti-Bu1-PB, mouse anti-IgA-PE, mouse anti-IgY-FITC, mouse anti-IgM-APC) for 15 min at 4°C in FACS staining buffer. Cells were washed (400 × g for 5 min) with FACS staining buffer and incubated for a further 10 min at 4°C with 7-AAD-PE (BDTM Pharmigen, Canada). Mononuclear cells were washed and stored in 2% paraformaldehyde (PFA) in the fridge (4°C). 5.0 × 105 cells were acquired on a FACS BD Canto II flow cytometer and data were processed by FlowJo V10 software. The full list of antibodies utilized is available in Table 2.
2.7 Quantitative real time-PCR (qRT-PCR)
(i) RNA extraction and cDNA synthesis: Total RNA was extracted using Trizol (Trizol®, Life Technologies, Inc.) from duodenum, jejunum and ileum tissue samples and preserved using RNA later. Tissue samples (50–100 mg) were homogenized in a tube containing glass beads using Elite Bead Ruptor (Omni International, Kennesaw GA, USA) with 1 mL of Trizol and RNA was extracted according to the manufacturer’s instructions as described previously (Boodhoo et al., 2022a). RNA quantity and quality were determined using the NanoDrop® ND-1000 spectrophotometer (NanoDrop Technologies, Wilmington, DE) after DNAse treatment. Synthesis of complementary DNAs (cDNA) was carried out by reverse transcription of 1 μg of total RNA using Oligo (dT) 12–18 primers and the Super-ScriptTM First-Strand Synthesis System (ThermoFisher Scientific, Canada.) according to the manufacturer’s instructions. Template cDNA were diluted 1:10 in milliQ water and stored in -20oC until required.
(ii) SYBR green qRT-PCR: qRT-PCR was run in 384-well plates with 5 μL of cDNA (1:10 dilution), 0.25 μM of forward and reverse primers, and 10 μL of SYBR Green (Roche Diagnostic, Laval, QC, Canada) and a balance of water to 20 μL total reaction volume per well. Each reaction involved a pre-incubation at 95°C for 5 min, followed by 40 cycles of 95°C for 20 s, 55°C–64°C (TA as per primer) for 15 s, and elongation at 72°C for 10 s. Subsequent melt curve analysis was performed by heating to 95°C for 10 s, cooling to 65°C for 1 min, and heating to 97°C. Primers sequences and accession numbers are outlined in Table 3. All data for qRT-PCR, where relative expression of each gene was calculated relative to β-actin as a housekeeping gene.
2.8 Bacterial DNA extraction
Duodenum, jejunum and ileum intestinal microbial genomic DNA extraction was performed using QIAamp® Fast DNA Stool Mini Kit (Qiagen, Canada) as recommended by the manufacturer. Briefly, 1 mL of InhibitEX buffer was added to 200 mg of duodenum, jejunum and ileum content. After a brief vortex (1 min) and centrifugation (20,000 × g for 1 min), supernatants were collected and added to tubes containing 25 μL of Proteinase K. AL buffer was added to the mixtures and incubated for 10 min at 70°C. Following the addition of 600 μL of 100% Ethanol, lysate-mixtures were briefly vortexed (1 min). The lysate-mixtures were applied to the spin columns, centrifuged (20,000 × g for 1 min) and washed with AW buffers in 2 steps. Eluted DNA (20,000 × g for 1 min) were quantified using a NanoDrop® ND-1000 spectrophotometer (NanoDrop Technologies, Wilmington, DE) and adjusted to 40 ng/μl for SYBR green qRT-PCR. Plates were read and analyzed by Light Cycler 480 II (Roche Diagnostics GmbH Mannheim, Germany). Total bacterial population was determined relative to the universal primers over control samples. Primer sequences and annealing temperatures are outlined in Table 2.
2.9 Statistical analysis
Graph Pad Prism 8 for windows was utilized to generate graphs and perform statistical analysis. All data are presented as mean + SD and analyzed by Wilcoxon and Mann Whitney non-parametric as well as two-way ANOVA was used to test significance. Results were considered statistically significant at p < 0.05 (*).
3 Results
3.1 Oral inoculation of rL. lactis reduces necrotic enteritis lesions
Lactococcus lactis bacteria were transformed with the pNZ8142 plasmid (rL. lactis) to enable detection and enumeration in intestinal contents post inoculation. The experimental timeline is illustrated in Figure 1A. The result demonstrates that chickens infected with C. perfringens had lower NE lesions if they had been orally inoculated with a rL. lactis (10*8 CFU; n = 10) compared to the PBS-treated infected group (n = 10) (Figure 1B). Lesion scoring, as listed in Table 1, was performed from the proximal end to the distal end of the small intestine (Figure 1C). Inoculation of rL. lactis prior to infection with C. perfringens inhibited fibrin formation, necrotic foci and ulcers. However, erythematous mucosa was still evident in these birds. It should be noted that chickens receiving rL. lactis or PBS without infection with C. perfringens showed no intestinal lesions. The quantification of rL. lactis in intestinal contents, collected from at least 6 chicks from each group (24 h post oral gavage), demonstrated that rL. lactis pass through and can survive in the medial duodenum (Figure 1D), jejunum (Figure 1E) and ileum (Figure 1F). Positive bacterial colonies were considered as the rL. lactis carrying the pNZ8124 plasmid providing resistance to chloramphenicol. Taken together, the results indicate that the presence of rL. lactis in the intestinal contents is associated with a reduction in necrotic lesions induced by C. perfringens.
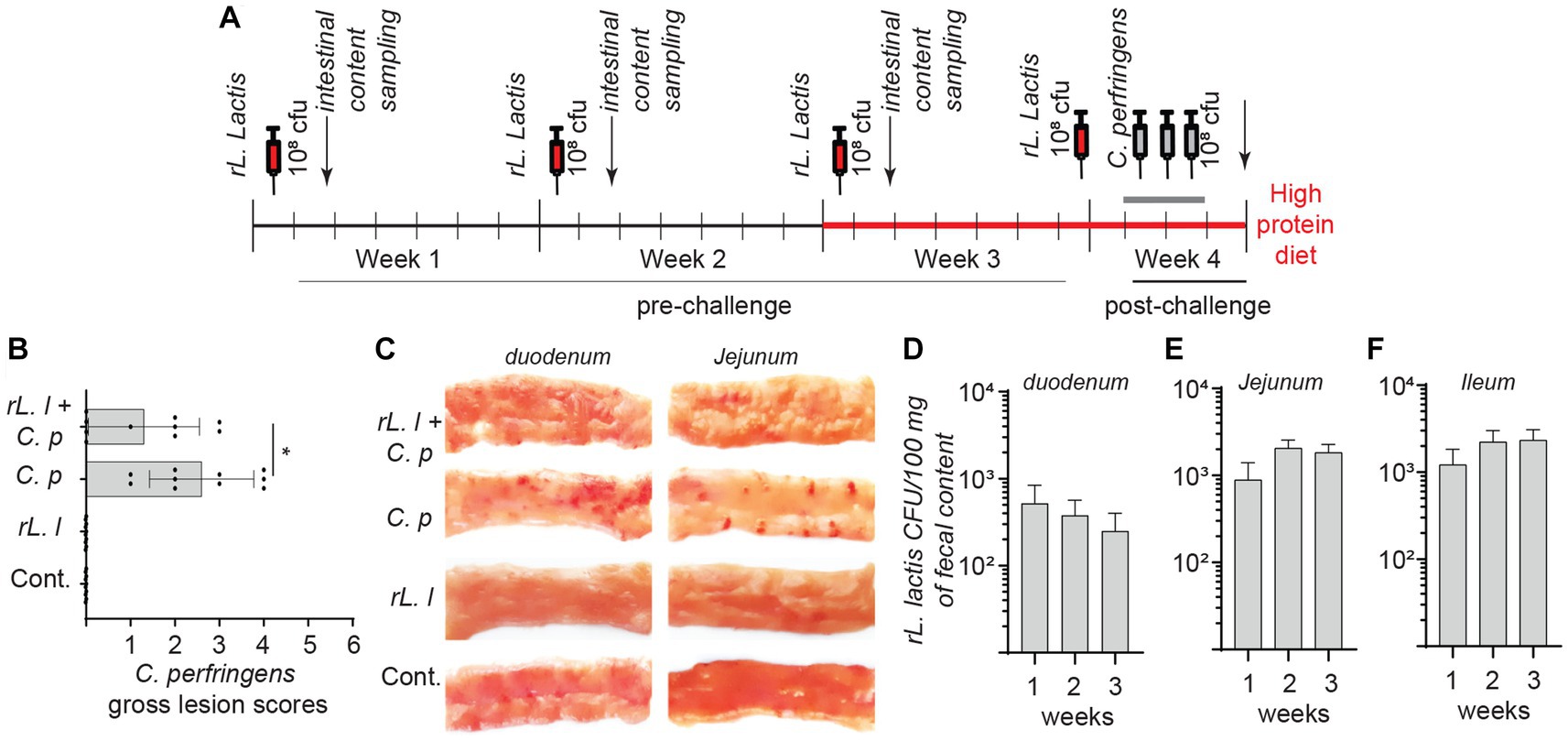
Figure 1. Necrotic lesion in C. perfringens infected boiler chickens. Schematic representation of experimental timeline (A) whereby 60 broiler chickens were orally administered with a recombinant L. lactis (1.0 × 108 CFU) or PBS on day 1, 7, 14 and 21 days of age. Starting week 4 (day 21), 30 broiler chickens were orally infected with C. perfringens (3.0 × 108 CFU) twice daily for 3 days. All chickens were sacrificed (n = 10 per group) on day 24 and (B) necrotic lesion were assessed in all groups and (C) a representative image of the necrotic lesion is shown. Presence of recombinant L. lactis two-days post inoculation (n = 6 weekly per group) was confirmed by serial dilution of intestinal contents collected from (D) duodenum, (E) jejunum and (F) ileum segments. The results are shown as mean ± SD. Non-parametric Wilcoxon tests (Mann–Whitney) was used to test significance. *(p ≤ 0.05) indicates a statistically significant result. rL. L; rL. lactis, C.P; C. perfringens.
3.2 rL. lactis treatment alters microbial genome content in chicken intestine
To examine any possible association between necrotic lesions and the abundance of microbial groups, intestinal contents (n = 6 per time points) were analyzed to determine phylogenetic relations in the rL. lactis treated chickens compared to control/PBS treated chickens prior to and post C. perfringens infection (Figure 2). Temporal changes in microbial relative content were used to estimate the abundance of specific bacterial phylum: Bacillota (Firmicutes), Bacteroidota (Bacteroides), Lactobacillaceae (Lactobacillus), Pseudomonadota (γ-proteobacteria) and Actinomycetota (Actinobacteria) to universal primers at 36 h post oral gavage with rL. lactis. The assessment of intestinal contents indicated that a diverse microbial community was established and could be modified by rL. lactis treatment. The results demonstrate site specific and temporal increases (p ≤ 0.01) in the Bacteroidota, Bacillota, and Lactobacillaceae prior to infection. There was a significant increase in the relative abundance of Bacteroidota (Figure 2A), Bacillota (Figure 2B), and Lactobacillaceae (Figure 2C) in jejunum (p ≤ 0.01) and ileum (p ≤ 0.01) at week 2 and 3 compared to that observed in duodenum. However, Actinomycetota (Figure 2D) relative number was significantly (p ≤ 0.005) more abundant in jejunum and ileum content at week 3 in comparison to that in duodenum. No changes in Pseudomonadota were observed after rL. lactis treatment alone in comparison to the control group (Figure 2E). Post C. perfringens infection, treatment with rL. lactis led to a decrease in the Bacillota but an increase in Bacteroidota, Lactobacillaceae, Pseudomonadota and Actinomycetota. The results also demonstrate that infection alone with C. perfringens (day 24) led to a significant (p ≤ 0.01) increase in duodenum, jejunum and ileum abundance of the Bacillota (Figure 2B), Actinomycetota (Figure 2D) and Pseudomonadota (Figure 2E). However, prior treatment with rL. lactis and subsequent infection with C. perfringens (day 24) resulted in a significant (p ≤ 0.005) decrease in duodenum, jejunum and ileum abundance of the Bacillota (Figure 2B). In contrast, prior treatment with rL. lactis and subsequent infection with C. perfringens (day 24) resulted in a significant (p ≤ 0.005) increase in duodenum, jejunum and ileum intestinal content of the Bacteroidota (Figure 2A), Lactobacillaceae (Figure 2C), Actinomycetota (Figure 2D) and Pseudomonadota (Figure 2E).
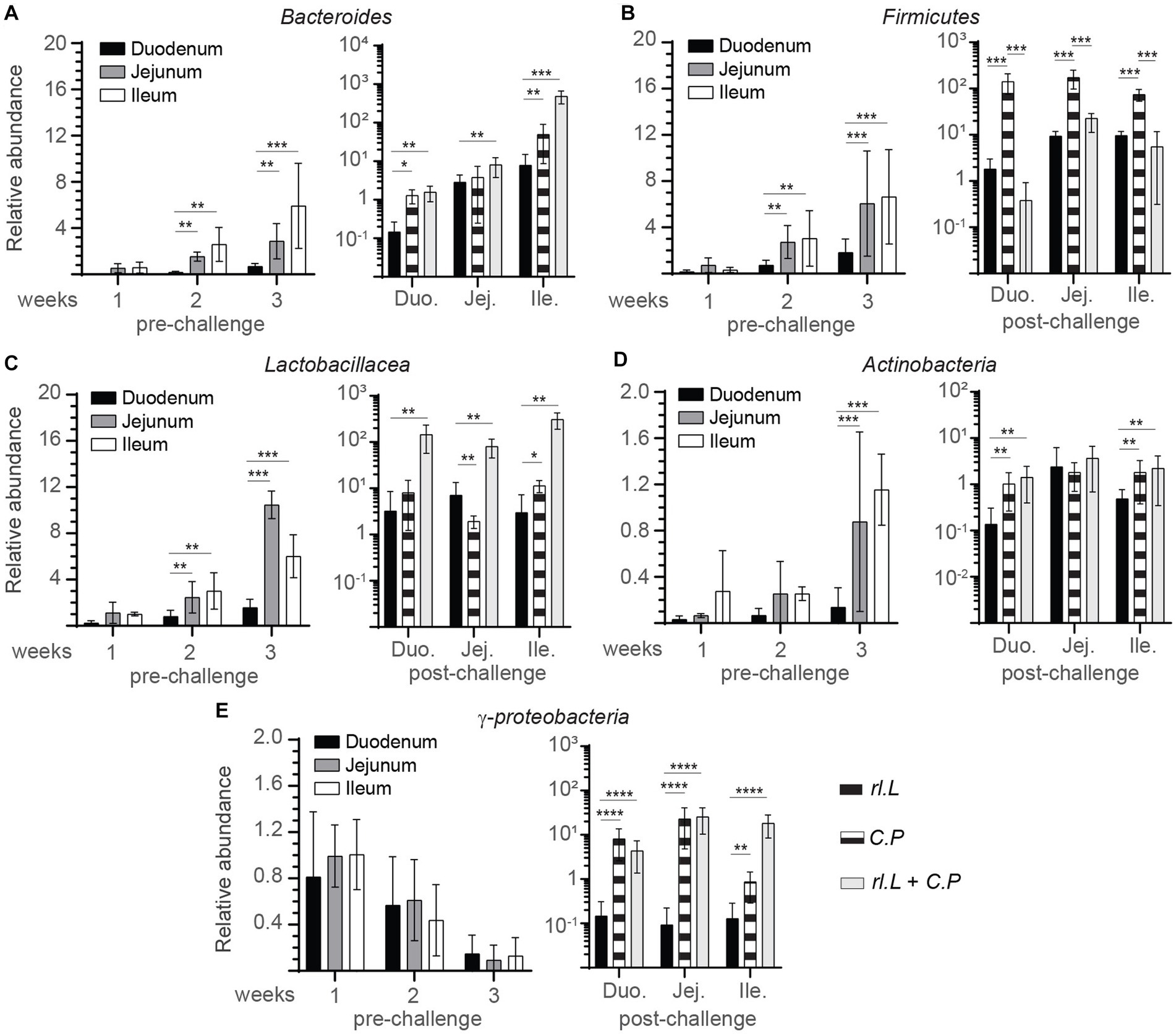
Figure 2. Changes in intestinal microbe genomic content as a result of rL. lactis inoculation. Weekly relative abundance of (A) Bacteroidota, (B) Bacillota, (C) Lactobacillaceae, (D) Actinomycetota, (E) Pseudomonadota to universal primers over control were assessed in intestinal contents collected 2 days post rL. lactis inoculation from the medial duodenum, jejunum and ileum intestinal segments prior to (n = 6 per group) and post C. perfringens infection (n = 10 per group) over control/PBS inoculated chickens. DNA extracted from 100 mg of intestinal contents were utilized for real-time PCR. The results are shown as mean ± SD. Non-parametric Wilcoxon tests (Mann–Whitney) was used to test significance. *(p ≤ 0.05), **(p < 0.01), and ****(p < 0.001) indicates a statistically significant result. Duo, duodenum; Jej, jejunum; Ile, ileum; Cont., control/PBS; rL. L, rL. lactis; C.P, C. perfringens.
3.3 Dynamics of induction of pro-inflammatory cytokines and mucosal innate immune cells
To identify the effects of rL. lactis treatment on modulation of mucosal immune parameters, we analyzed induction of innate responses, such as temporal expression of IL-6, and IL-8 to β-actin as well as frequency of intestinal mononuclear phagocytes. Treatment with rL. lactis prior to infection with C. perfringens led to a time- and site-specific dependent increase in expression of IL-6 (Figure 3A), and IL-8 (Figure 3B). Over time (week 1 vs. week 3), IL-6 expression was significantly decreased in the duodenum and jejunum of rL. lactis treated chickens (Figure 3A). There was a significant (p ≤ 0.05) decrease in expression of IL-6 (Figure 3C), and IL-8 (Figure 3D) in the duodenum and jejunum of chickens that were pre-treated with rL. lactis and infected with C. perfringens in comparison to C. perfringens only infected chickens. In contrast, infection with C. perfringens led to a significant increase (p ≤ 0.05) in expression of IL-8 (Figure 3D) when comparing to rL. lactis treated only and rL. lactis and C. perfringens infected groups in the ileum.
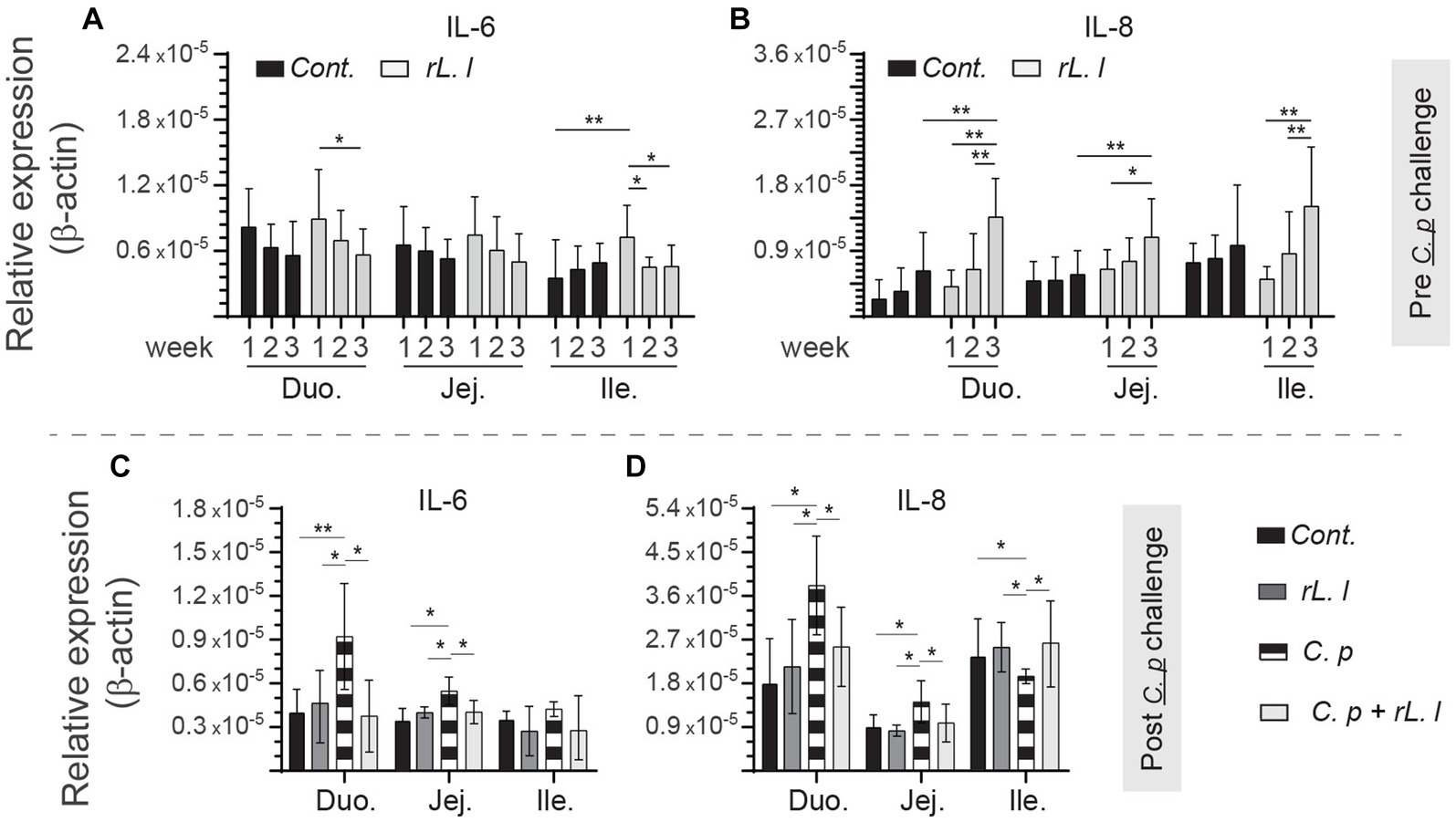
Figure 3. Temporal changes in intestinal IL-6, and IL-8. Weekly relative (A,C) IL-6, and (B,D) IL-8 to β-actin mRNA transcripts in medial duodenum, jejunum and ileum intestinal segments from rL. lactis treated chickens (A,B) prior to (n = 6 per group) and (C,D) post C. perfringens infection (n = 10 per group) over β-actin in control/PBS inoculated chickens. The results are shown as mean ± SD. Non-parametric Wilcoxon tests (Mann–Whitney) or two-way ANOVA was used to test significance. *(p ≤ 0.05), and **(p ≤ 0.01) indicates a statistically significant result. Duo, duodenum; Jej, jejunum; Ile, ileum; Cont., control/PBS; rL. L, rL. Lactis; C.P, C. perfringens.
As IL-6 and IL-8 can be produced by mononuclear phagocytes, we analyzed the frequencies of Kul01 + MHC-II+ cells in different experimental groups using flow cytometry (Figure 4A). The results demonstrate a significant increase in frequency of the Kul01 + MHC-II+ cells, following rL. lactis treatment, in the medial duodenum (p ≤ 0.0001), and jejunum (p ≤ 0.05) but not in the ileum and cecal tonsil when compared to the control chickens (Figure 4B). Infection with C. perfringens led to a significant decrease in frequency of the Kul01 + MHC-II+ cells in duodenum (p ≤ 0.05) and jejunum (p ≤ 0.05) compared to the control or the rL. lactis treated and infected chickens (Figure 4C). There was a significant (p ≤ 0.0001) increase in frequencies of Kul01 + MHCII+ cells in ileum of the C. perfringens infected chickens compared to the control or the rL. lactis treated only groups (Figure 4C). The increase in mucosal Kul01 + MHC-II+ cells was sustained post infection with C. perfringens (Figure 4C).
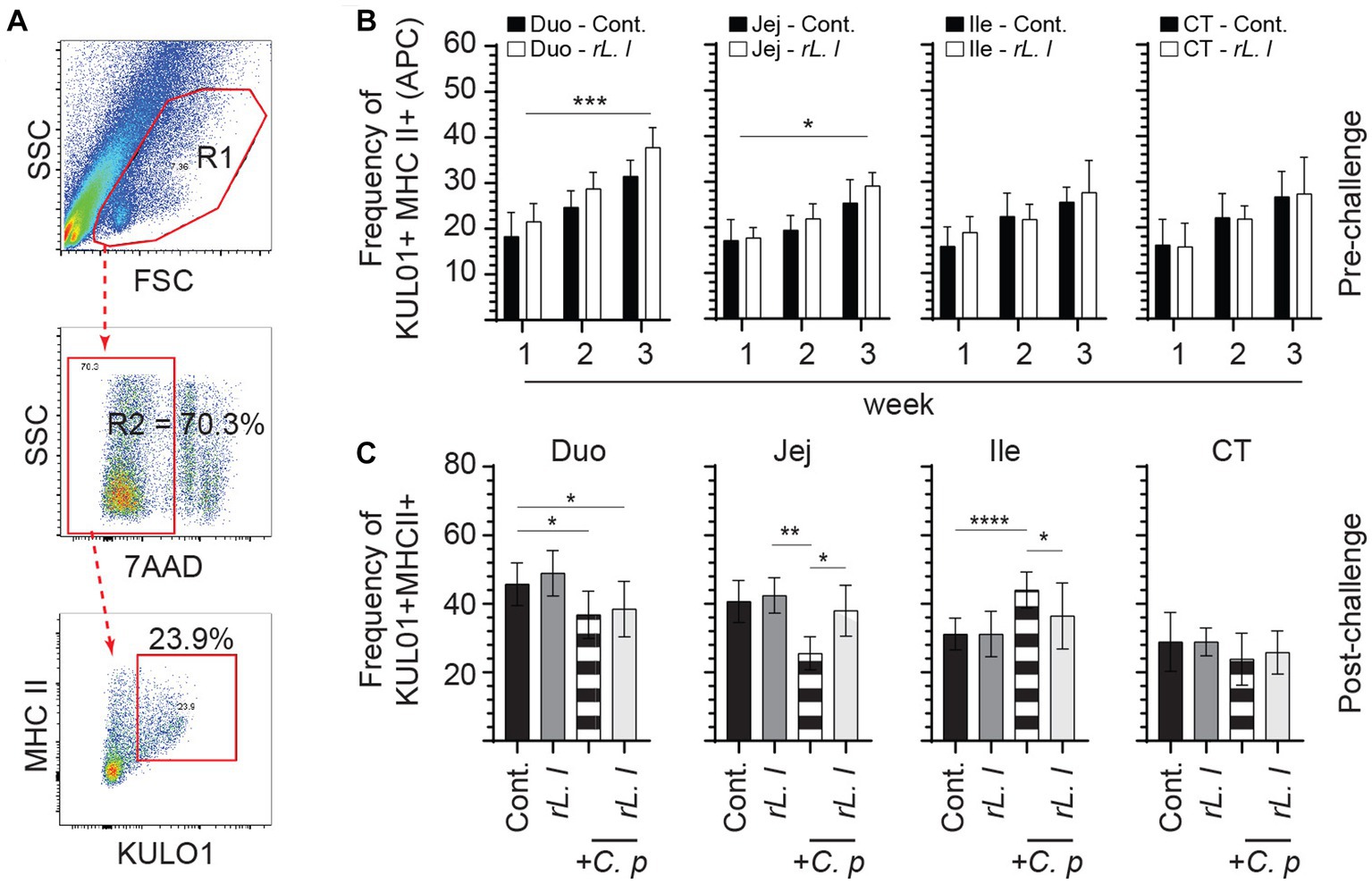
Figure 4. Changes in the frequency of intestinal mononuclear phagocytes. The frequency of duodenum, jejunum, ileum and cecal tonsils mononuclear cells were assessed in rL. lactis inoculated chickens prior to and post challenge with PBS or C. perfringens in comparison C. perfringens infected only and control chickens (n = 6 per group). (A) Dot plot demonstrates gating strategy for detection of macrophages (KUL01 + MHC-II+) are shown. The weekly frequency (7AAD-; dead cell exclusion) of KUL01 + MHC-II+ in the (B) pre-challenge (C) and post C. perfringens challenge are presented. Non-parametric Wilcoxon tests (Mann–Whitney) or two-way ANOVA was used to test significance with the results shown as mean ± SD. *(p ≤ 0.05), **(p ≤ 0.01), ***(p ≤ 0.005), and ****(p ≤ 0.001) indicates a statistically significant result. The mean ± SD value are shown in weekly samples collected from six individual birds for each group. Duo, duodenum; Jej, jejunum; Ile, ileum; CT, cecal tonsils; Cont., control; rL. L, rL. Lactis; C.P, C. perfringens.
3.4 Temporal expression profile of cytokines that modulate T cell function
rL. lactis treatment prior to infection with C. perfringens led to temporal and spatial changes in expression of IL-13 (Figure 5A), IL-17 (Figure 5B), IL-18 (Figure 5C), IL-22 (Figure 5D), IL-12p40 (Figure 5E) and IFN-γ (Figure 5F) to β-actin from week 1 onwards in all sites along the intestine. IL-17 (Figure 5B), and IFN-γ (Figure 5F) mRNA transcripts were significantly (p ≤ 0.01) upregulated in the jejunum of rL. lactis treated group but not in the duodenum and ileum. In contrast, IL-13 (Figure 5A) and IL-18 (Figure 5C) mRNA transcripts were significantly (p ≤ 0.05) upregulated in the ileum of rL. lactis treated group. There was a significant (p ≤ 0.05) decrease in expression of intestinal IL-13 (Figure 5G) and IL-18 (Figure 3I) in chickens that were pre-treated with rL. lactis and subsequently infected with C. perfringens in comparison with C. perfringens only infected chickens. Post- C. perfringens infection, there was a significant increase in intestinal IL-13 (p ≤ 0.001) (Figure 5G) and IL-18 (p ≤ 0.05) (Figure 5I) expression in both rL. lactis treated only and rL. lactis treated and subsequently C. perfringens infected chickens compared to control chickens. Expression of IL-17 was significantly decreased (p ≤ 0.001) in the ileum only and not in duodenum and jejunum in both rL. lactis treated only and rL. lactis treated and subsequently C. perfringens infected chickens compared to control chickens (Figure 5H). Both IL-12p40 (Figure 5K) and IFN-γ (Figure 5L) intestinal mRNA transcripts were significantly increased (p ≤ 0.001) in rL. lactis treated and C. perfringens infected chickens when compared to C. perfringens only infected chickens. No change in IL-22 (Figure 5J) was observed post-infection with C. perfringens.
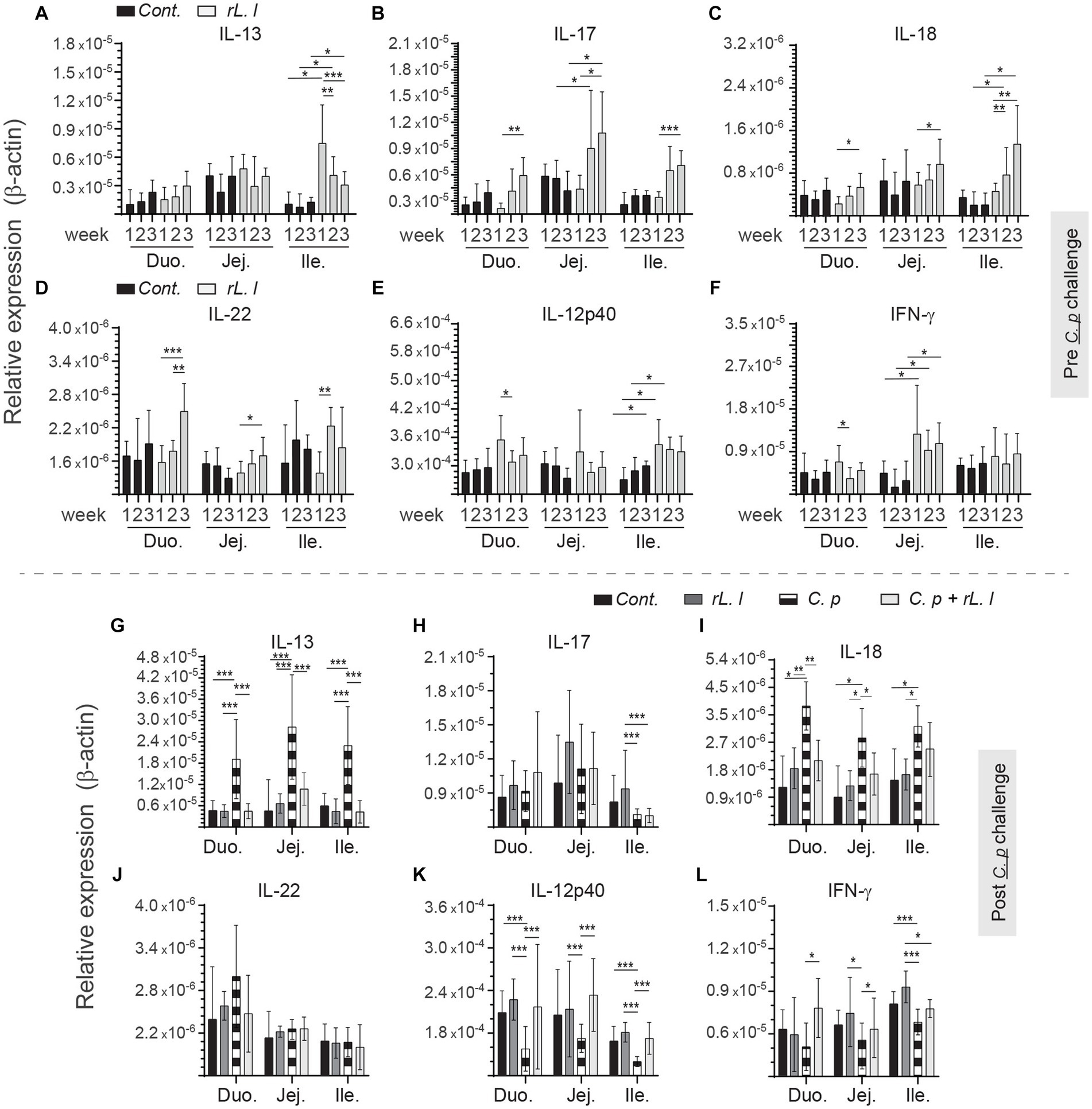
Figure 5. Temporal changes in intestinal IL-13, IL-17, IL-18 IL-22, IL-12p40 and IFN-γ. Weekly relative (A,G) IL-13, (B,H) IL-17, (C,I) IL-18, (D,J) IL-22, (E,K) IL-12p40 and (F,L) IFN-γ to β-actin mRNA transcripts in medial duodenum, jejunum and ileum intestinal segments from rL. Lactis treated chickens (A–F) prior to (n = 6 per group) and (G–L) post C. perfringens infection (n = 10 per group) over β-actin in control/PBS inoculated chickens. The results are shown as mean ± SD. Non-parametric Wilcoxon tests (Mann–Whitney) or two-way ANOVA was used to test significance. *(p ≤ 0.05) **(p ≤ 0.01) ***(p ≤ 0.005) indicates a statistically significant result. Duo, duodenum; Jej, jejunum; Ile, ileum; Cont., control/PBS; rL. L, rL. lactis; C.P, C. perfringens.
3.5 Challenge with Clostridium perfringens led to an increase in intestinal γδ T cells
Alterations in intestinal cytokines can affect intestinal cellular composition, such as abundance of T cells. FACS analysis was used to characterize intestinal mononuclear cells along the intestinal tissue (Figure 6A). The frequencies of CD3ζ + CD8α + γδ T cells within CD3ζ + γδ T cell population are presented. In control chickens, the ileum was found to have higher frequencies of CD3ζ + CD8α + γδ T cells in comparison with the duodenum, jejunum and cecal tonsils. The results also demonstrate that treatment with rL. lactis led to a significant increase in the frequency of CD3ζ + CD8α + γδ T cells in the duodenum (p ≤ 0.05), ileum (p ≤ 0.0001) and cecal tonsils (p ≤ 0.05) as assessed by two-way ANOVA when compared to control chickens (Figure 6B). Infection with C. perfringens also led to a significant increase in the frequency of CD3ζ + CD8α + γδ T cells in duodenum (p ≤ 0.01), ileum (p ≤ 0.05) and cecal tonsils (p ≤ 0.005) (Figure 6C). Similarly, rL. lactis treatment followed by infection with C. perfringens increased the frequency of these cells in duodenum (p ≤ 0.005), jejunum (p ≤ 0.0001) and cecal tonsils (p ≤ 0.01) CD3ζ + CD8α + γδ T cells when compared to control chickens (Figure 6C).
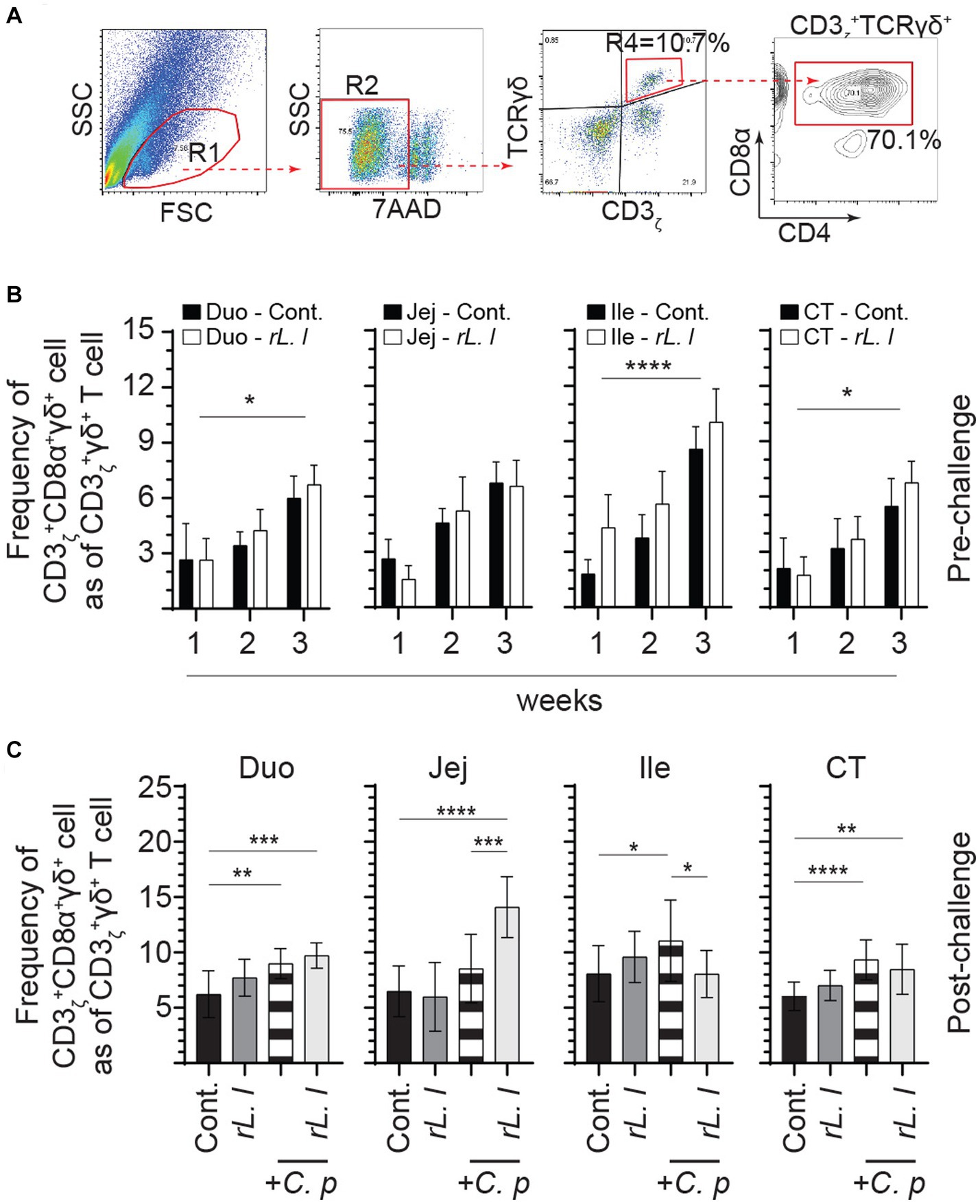
Figure 6. Increase in the frequency of intestinal γδ T cells in response to rL. lactis inoculation. The frequency of duodenum, jejunum, ileum and cecal tonsils mononuclear cells were assessed in rL. lactis inoculated chickens prior to and post challenge with PBS or C. perfringens in comparison to C. perfringens infected only and control chickens (n = 6 per group). (A) Pseudocolor plot demonstrates gating strategy for detection of CD3 + CD8α + γδ T cells are shown. The weekly frequency (7AAD-; dead cell exclusion) of CD3 + CD8α + γδ T in γδ T cells (B) pre-challenge (C) and post C. perfringens challenge are presented. Non-parametric Wilcoxon tests (Mann–Whitney) or two-way ANOVA was used to test significance with the results shown as mean ± SD. *(p ≤ 0.05), **(p ≤ 0.01), ***(p ≤ 0.005), and ****(p ≤ 0.001) indicates a statistically significant result. The mean ± SD value are shown in weekly samples collected from six individual birds for each group. Duo, duodenum; Jej, jejunum; Ile, ileum; CT, cecal tonsils; Cont., control; rL. L, rL. lactis; C.P, C. perfringens.
3.6 Temporal and site-specific changes in adaptive immune system cells (αβ T and B cells)
The frequencies of αβ T cells were also assessed by FACS analysis. A negative gating strategy, CD3ζ + γδ- T cells, was used to identify CD3ζ + αβ + T cells that are either express CD4+ or CD8α + (Figure 7A). CD3ζ + CD8α + αβ T cells (Figure 7D) made up the majority of the αβ T cells and were more abundant (on average 2:1 ratio) than CD3ζ + CD4+ αβ T cells (Figure 7B). The abundance of intestinal tissue CD3ζ + CD8α + αβ T cells was further amplified following a prolonged treatment with rL. lactis. The results demonstrate that treatment with rL. lactis led to a significant increase in the frequency of CD3ζ + CD8α + αβ T cells in the duodenum (p ≤ 0.01) and ileum (p ≤ 0.01) when compared to the control chickens (Figure 7D). In contrast, treatment with rL. lactis led to a significant decrease in the frequency of intestinal CD3ζ + CD4+ αβ T cell when compared to the control chickens (Figure 7B). Infection with C. perfringens also altered the frequency of CD4+ and CD8α + αβ T cells. Infection with C. perfringens resulted in a significant decrease in the frequency of CD3ζ + CD4+ αβ T cells across all segments of the intestine (Figure 7C). Conversely, infection significantly increased the frequency of CD3ζ + CD8α + αβ T cells across all segments of the intestine when compared to the rL. lactis treated and infected chickens (Figure 7E). However, the combination of rL. lactis treatment and subsequent C. perfringens infection resulted in the increase of both CD3ζ + CD4+ (Figure 7C) and CD3ζ + CD8α + (Figure 7E) αβ T across the intestine when compared to the infected or control chickens, respectively.
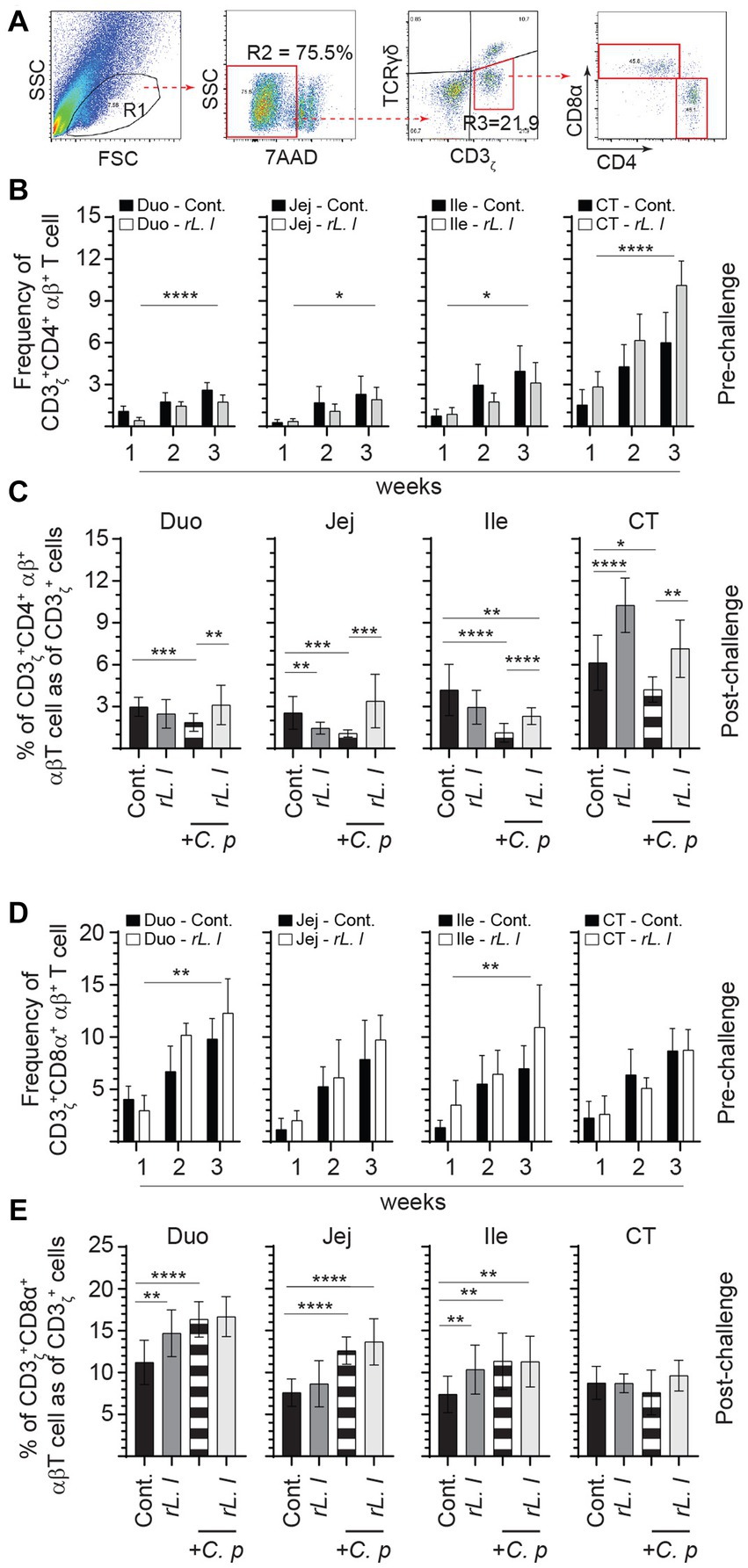
Figure 7. Differential changes in the frequency of intestinal αβ T cells subsets in response to rL. lactis inoculation. The frequency of duodenum, jejunum, ileum and cecal tonsils mononuclear cells were assessed in rL. lactis inoculated chickens prior to and post challenge with PBS or C. perfringens in comparison to C. perfringens infected only and control chickens (n = 6 per group). (A) Pseudocolor plot demonstrates gating strategy for detection of CD3+ αβ T cells that are (B,C) CD4+ or (D,E) CD8α + are shown. The weekly frequency (7AAD-; dead cell exclusion) of CD3 + CD4+ and CD3 + CD8α + αβ T in CD3+ αβ T cells (B,D) pre-challenge (C,E) and post C. perfringens challenge are presented. Non-parametric Wilcoxon tests (Mann–Whitney) or two-way ANOVA was used to test significance with the results shown as mean ± SD. *(p ≤ 0.05), **(p ≤ 0.01), and ***(p ≤ 0.0005) and ****(p ≤ 0.0001) indicates a statistically significant difference compared to control. The mean ± SD value are shown in weekly samples collected from six individual birds for each group. Duo, duodenum; Jej, jejunum; Ile, ileum; CT, cecal tonsils; Cont., control; rL. L, rL. lactis; C.P, C. perfringens.
In addition to T cells, B cells were also characterized in the present study (Figure 8). As expected, three major B cell subsets expressing either IgM, IgY or IgA immunoglobulins were found in the intestine (Figure 8A). The intestine was mainly populated by IgA+ (Figure 8B) followed by IgY+ B cells (Figure 8C) while IgM+ B cells (Figure 8D) made up a minority portion of duodenum, jejunum and ileum Bu1+ B cells. In contrast, the cecal tonsils are mainly populated (p ≤ 0.0001) by IgM+ B cells (Figure 8D) followed by IgY+ (Figure 8C) and IgA+ B cells (Figure 8C). The results demonstrated that repeated treatment with rL. lactis over several weeks resulted in the expansion of B cells that are IgA+ within the duodenum (p ≤ 0.05), jejunum (p ≤ 0.005) and ileum (p ≤ 0.0001) and IgY+ within the jejunum (p ≤ 0.0001) and ileum (p ≤ 0.0001) when compared to control chickens. There were no changes in the frequency of IgM+ B cells within the duodenum, jejunum and ileum as a result of rL. lactis.
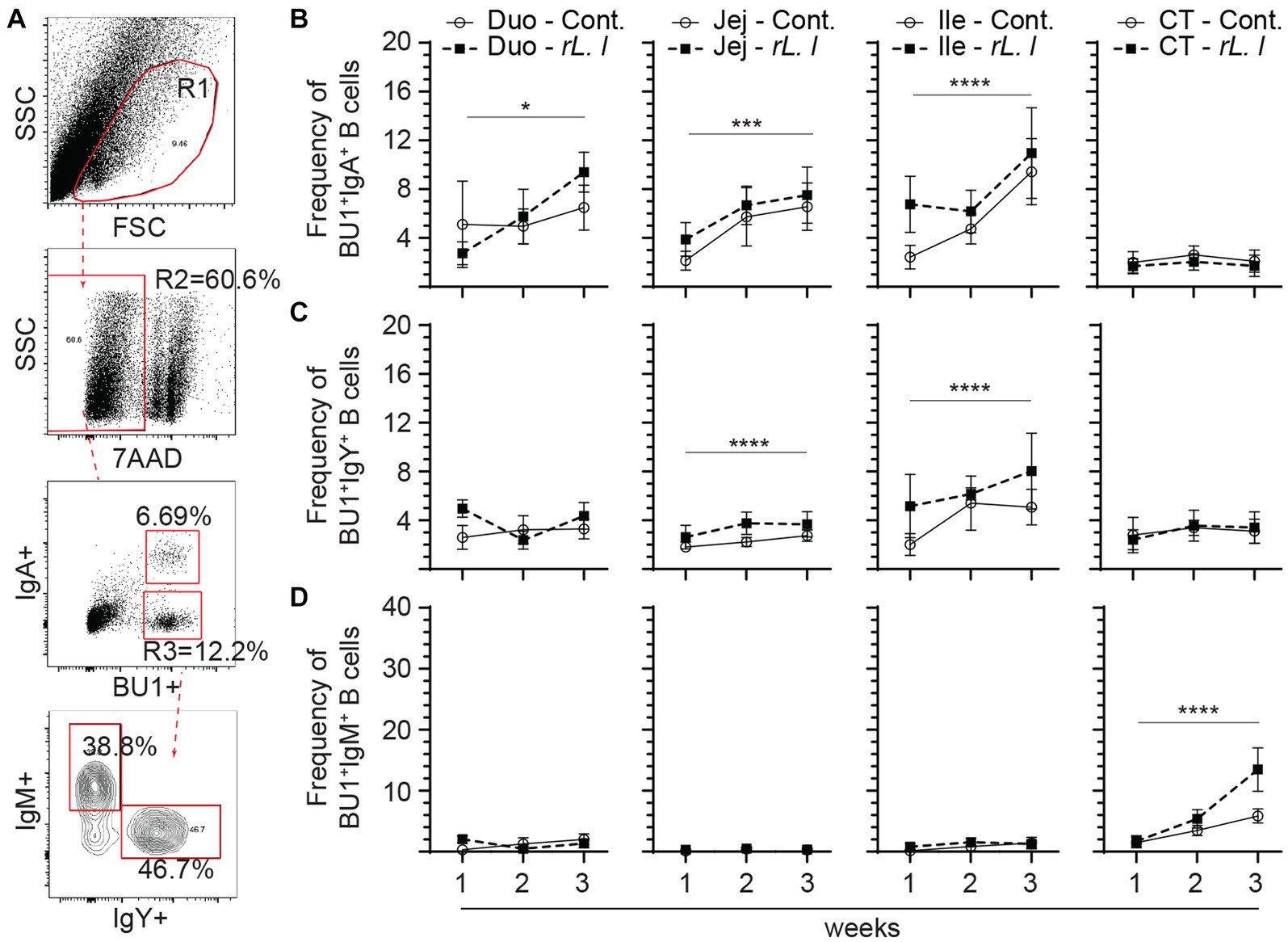
Figure 8. Differential changes in the frequency of intestinal B cell subsets in response to rL. lactis inoculation. The frequency of duodenum, jejunum, ileum and cecal tonsils mononuclear cells were assessed in rL. lactis inoculated chickens in comparison to control chickens (n = 6 per group). (A) Dot plot demonstrates gating strategy for detection of Bu1+ B cells and the various subsets; IgA+, IgY+ and IgM+. The weekly frequencies (7AAD-; dead cell exclusion) of Bu1+ B cells that are (B) IgA+, (C) IgY+ and (D) IgM+ pre-challenge are presented. Non-parametric Wilcoxon tests (Mann–Whitney) or two-way ANOVA was used to test significance with the results shown as mean ± SD. *(p ≤ 0.05), ***(p ≤ 0.0005) and ****(p ≤ 0.0001) indicates a statistically significant difference compared to control. The mean ± SD value are shown in weekly samples collected from six individual birds from each group. Duo, duodenum; Jej, jejunum; Ile, ileum; CT, cecal tonsils; Cont., control; rL. L, rL. lactis.
Infection with C. perfringens resulted in further expansion of IgA+, IgY+ and IgM+ Bu1+ B cells across the intestine (Figure 9). The results demonstrate that infection with C. perfringens led to a significant increase in the frequency of both IgA+ (p ≤ 0.0001) (Figure 9B) and IgY+ (p ≤ 0.0001) (Figure 9C) Bu1+ B cell within the duodenum, jejunum and cecal tonsils when compared to the control chickens. The combination of prolonged rL. lactis pre-treatment followed by infection with C. perfringens resulted in a significant increase of both IgA+ (p ≤ 0.0001) (Figure 9B) and IgY+ (p ≤ 0.0001) (Figure 9C) Bu1+ B cell frequencies within the duodenum, jejunum and ileum when compared to control chickens. The frequency of IgM+ Bu1+ B cell frequency was significantly increased in the jejunum (p ≤ 0.005) and ileum (p ≤ 0.0001) of chickens that were previously treated with rL. lactis and subsequently infected with C. perfringens or in C. perfringens only infected chickens (Figure 9D).
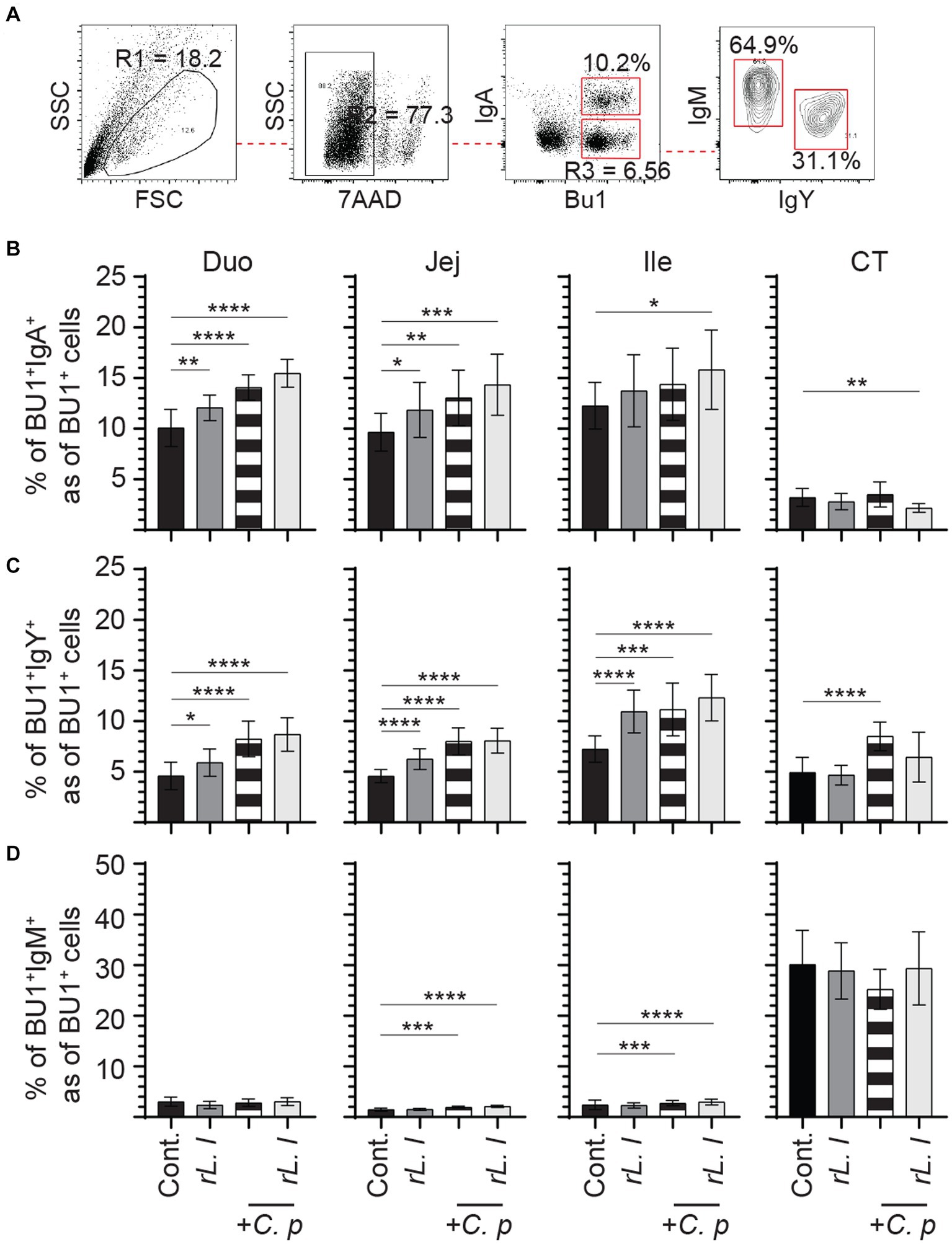
Figure 9. The frequency of intestinal B cell subsets post C. perfringens infection. The frequency of duodenum, jejunum, ileum and cecal tonsils mononuclear cells were assessed in rL. lactis inoculated chickens prior to and post challenge with PBS or C. perfringens in comparison to C. perfringens infected only and control chickens (n = 6 per group). (A) Dot plot demonstrates gating strategy for identification of Bu1+ B cells that are IgA+, IgY+ and IgM+. The weekly frequency (7AAD-; dead cell exclusion) of Bu1+ B cells that are (B) IgA+, (C) IgY+ and (D) IgM+ post C. perfringens infection are presented. Non-parametric Wilcoxon tests (Mann–Whitney) was used to test significance with the results shown as mean ± SD. *(p ≤ 0.05), **(p ≤ 0.01), ***(p ≤ 0.0005) and **** (p ≤ 0.0001) indicates a statistically significant difference compared to control. The mean ± SD value are shown in weekly samples collected from six individual birds for each group. Duo, duodenum; Jej, jejunum; Ile, ileum; CT, cecal tonsils; Cont., control; rL. L, rL. lactis; C.P, C. perfringens.
3.7 Temporal expression of molecules associated with immune regulation
To determine the immune regulatory effects of rL. lactis, temporal expression of IL-10, TGF-β, CTLA-4 and CD40 was assessed to β-actin (Figure 10). Prior treatment with rL. lactis led to an increase in expression of CD40 (Figure 10A), TGF-β (Figure 10B), IL-10 (Figure 10C) and CTLA-4 (Figure 10D) in the duodenum, jejunum and ileum. Infection with C. perfringens in those chickens that had prior treatment with rL. lactis resulted in a significant (p ≤ 0.01) increase in CD40 expression in comparison to C. perfringens infected only chickens (Figure 10E). CD40 (Figure 10E) expression levels were significantly (p ≤ 0.01) decreased in the duodenum, jejunum and ileum post infection with C. perfringens. However, CTLA-4 expression was only decreased in the ileum post infection with C. perfringens (Figure 10H). IL-10 mRNA expression level was significantly (p ≤ 0.01) decreased in chicken pretreated with rL. lactis and subsequently infected with C. perfringens, similar to rL. lactis treated only chickens, when compared to infected only chickens (Figure 10G). In contrast, infection with C. perfringens resulted in a significant (p ≤ 0.01) increase in IL-10 mRNA transcripts (Figure 10G) in all intestinal segments studied. No changes in induction of TGF-β mRNA transcripts was observed (Figure 10F).
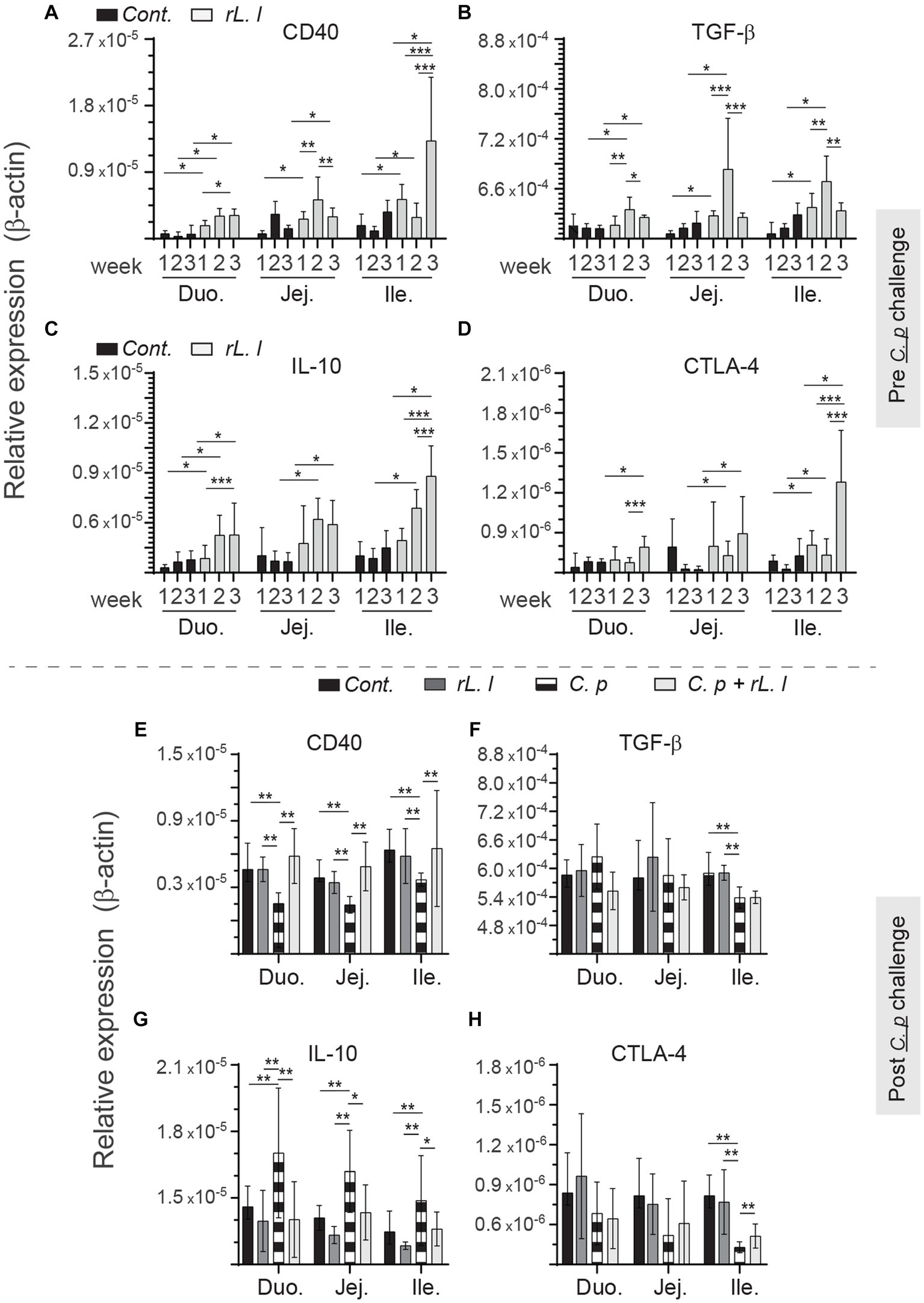
Figure 10. Temporal changes in intestinal CD40, TGF-β, IL-10 and CTLA-4. Weekly relative (A,F) CD40, (B,G) TGF-β, (C,H) IL-10 and (D,I) CTLA-4 to β-actin mRNA transcripts in medial duodenum, jejunum and ileum intestinal segments from rL. Lactis treated chickens (A–C) prior to (n = 6 per group) and (D–F) post C. perfringens infection (n = 10 per group) over β-actin in control/PBS inoculated chickens. The results are shown as mean ± SD. Non-parametric Wilcoxon tests (Mann–Whitney) or two-way ANOVA was used to test significance. *(p ≤ 0.05) **(p ≤ 0.01) ***(p ≤ 0.005) indicates a statistically significant result. Duo, duodenum; Jej, jejunum; Ile, ileum; Cont., control/PBS; rL. L, rL. lactis; C.P, C. perfringens.
4 Discussion
The intestine contains the largest number of immune system cells of any tissue, and it is continually exposed to a wide range of antigens and immune stimuli (Lee and Mazmanian, 2010). The involvement of specific host defenses, processes that take place in the mucosa and underlying lamina propria (LP), differ between microorganisms. Among several factors, pathogenic bacteria and their secretory products contribute to gut barrier impairment and its increased permeability, precisely the key predisposing conditions that facilitates C. perfringens cellular proliferation and progression to NE in chickens (Shojadoost et al., 2012). To counteract the effects of enteric pathogens, beneficial microbes may be employed (Alizadeh et al., 2021). Here, we evaluated the utility of L. lactis as a beneficial microbe whereby treatment led to a reduction in intestinal necrotic lesion scores associated with infection by a virulent C. perfringens strain. This observation might be due to a reduction in inflammatory processes, alteration in the proportion of intestinal immune system cell, and modification of intestinal microbial communities (Figure 11).
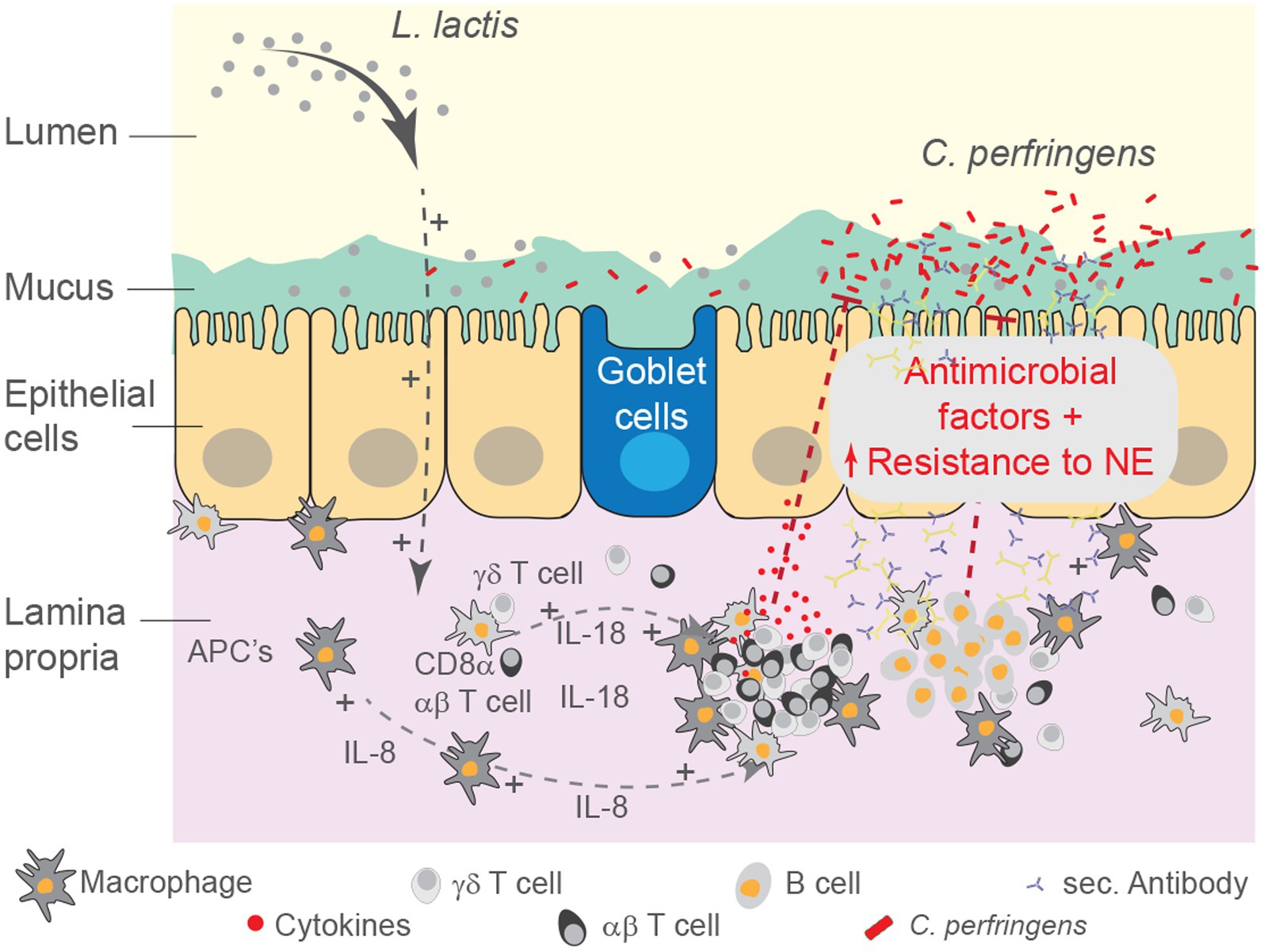
Figure 11. Schematic summary for the effect of L. lactis treatment on the intestinal mucosa. Schematic representation of the study demonstrating systematic modulation of the intestinal mucosa by rL. lactis. Alteration in luminal microbial content as well as intestinal immune system cells indirectly limited the severity of necrotic foci formation as a result of pathogenic C. perfringens infection. We speculate that rL. lactis promotes recruitment of monocyte-macrophages, expansion of CD8α + γδ and αβ T cells as well as IgA+ and IgY+ B cells in the lamina propria of the duodenum, jejunum and ileum. Immune cell activation, as demonstrated by changes in cytokines and chemokines, support the intestinal epithelial barrier to insult from various cytolytic toxins secreted by the pathogenic C. perfringens.
Indeed, the intestinal microbiota acts as a primary barrier to prevent direct contact between invading pathogens such as C. perfringens and the intestinal epithelium. Sequencing efforts have demonstrated that Bacillota, Bacteroidota, Pseudomonadota, Lactobacillaceae and Actinomycetota are the most abundant phyla in the human intestine with similar relative proportions found in the chicken ceca (Tap et al., 2009; Oakley et al., 2014; Clavijo and Flórez, 2018). Firstly, while L. lactis does not colonize the chicken intestine, treatment increased the abundance of Gram-positive (Bacillota, Lactobacillaceae and Actinomycetota) bacteria with limited changes in Gram-negative (Bacteroidota and Pseudomonadota) bacteria, in contrast to C. perfringens infected chickens. The latter was also observed in a mouse model whereby C. difficile infection increased the proportion of Proteobacteria and decreased Bacillota populations which correlated with more severe disease (Reeves et al., 2011). Secondly, dysbiosis can modulate TLR signaling, likely promoting C. perfringens mediated inflammatory processes, partly reversed by treatment with L. lactis. Mononuclear phagocytes active sampling of the gut microbiota modulates the GALT (Balic et al., 2014). This study demonstrates that mononuclear phagocytes make up more than one-third of all immune system cells that populate the chicken intestine which is decreased in C. perfringens infected chickens. Upon detecting endogenous danger signals through TLRs, mononuclear phagocytes in turn regulate B cells, and αβ or γδ T cells activity (Kono and Rock, 2008). TLR mediated and co-stimulatory signaling promote transcription of antimicrobial effector molecules (IL-17a and IL-22), regulatory factors (CTLA-4, CD40, IL-10 and TGF-β), as well as Th1 (IL-12p40, IL-18, IFN-γ) and Th2 (IL-4, IL-10 and IL-13) cytokines. TLR2 signaling has been suggested as a mechanism for dynamic feedback regulating inflammation and anti-microbial activity. Treatment with probiotic Lactobacillus in chickens also modulates TLR expression (Shojadoost et al., 2022). In an ex vivo experiment, competitive interaction by L. lactis has been suggested to be effective at negating TLR4-mediated modulatory effects of C. perfringens toxins and PAMPs on chicken intestinal immune system cells (Boodhoo et al., 2022b). Mouse studies support a protective role played by microbes that contain polysaccharide rich surface capsule that stimulate TLR2 signaling. The commensal microbiota Bacteroides fragilis drives differentiation of FOXP3+ T cells with regulatory function and ability to produce regulatory factors (Round and Mazmanian, 2010). The L. lactis NZ9000 strain used in this study also possesses a surface capsule rich in LTA motifs (TLR2 ligand) (Song et al., 2017). L. lactis treated chickens had higher expression of IL-10 and TGF-β. In line with these finding, the results suggested that L. lactis treatment can modulate intestinal immune response thereby limiting virulence associated factors of C. perfringens.
Selective induction of cytokines in combination with TLR stimulation modulates functions of T cells and antibody producing plasma B cells in the LP (Coffman et al., 1989). Based on FACS analysis, the chicken small intestine is populated by both CD8α + and CD4+ αβ T cell but has proportionally more CD8α + T cells at a ratio of 2.5:1. In humans, a similar ratio has been observed along the length of the small intestine (Mowat and Agace, 2014). However, C. perfringens infection resulted in a significant reduction in intestinal CD4+ αβ T cell while an increase in CD8α + αβ T cell was observed thereby shifting this ratio to 5:1, proportionally more in favor of CD8α + αβ T cells. L. lactis treatment was essential to overcome the depleting effects caused by C. perfringens infection on CD4+ αβ T cells populations. CTLA-4, an immune checkpoint expressed by regulatory T cells, was downregulated in C. perfringens infected chicken. In this study, the method of mononuclear cell isolation does not allow for differentiation between intraepithelial lymphocytes and LP lymphocytes. While these two cell types are well adapted to intestinal stimuli and perform their functions in protecting the delicate epithelial layer, the role of CD8α + αβ T cell in mucosal tissues against C. perfringens infection, an extracellular pathogen, is not understood.
The proportion of intestinal CD4+ to that of CD8α + αβ T cells could be a key factor to limit progression of NE. In mice, monocolonization with segmented filamentous bacteria (SFB), of the order Clostridiales, can induce the development of LP-resident CD4+ αβ T cells, regulatory T cells and T helper 17 (Th17) cells secreting various cytokines including TGF-β, IL-10, IL-13, IL-17a and IL-23 (Ivanov et al., 2009). Loss of mouse Th17 cells, which originate from CD4+ αβ T cells, has been implicated in microbial translocation into the intestinal epithelium and disease progression. Moreover, SFB treatment stimulates local expansion of LP-plasma cells, which secrete IgA antibodies (Ivanov et al., 2009). In addition, Firmicutes, which include the Clostridia order, are essential for induction and maintenance of tissue regulatory T cells (CD4 + CD25 + TGF-β+) during the early stages of development (Lathrop et al., 2011). The distribution and function of these T cell subsets in chickens are still unclear. However, NE severity is thought to be a result of excessive production of IL-1β, IL-13 and IL-17 cytokines during infection, a mechanism mediated via Th2 and Th17 cells (Lillehoj and Trout, 1996; Fasina and Lillehoj, 2019). Furthermore, no change in IFN-γ expression but a decrease in TGF-β expression has been observed in C. perfringens infected chickens (Fasina and Lillehoj, 2019; Zaytsoff et al., 2020). Expression of IFN-γ, IL-13 and IL-17 cytokines could be used as markers to define associations with NE lesion severity. This is because C. perfringens PAMPs and toxins likely have a dual role in activating and inhibiting specific mononuclear cells. Ex vivo stimulation with C. perfringens could effectively inhibit IFN-γ expression but upregulated expression of CD25 in intestinal T cell subsets (Boodhoo et al., 2022b). Our results are further supported based on observations whereby IFN-γ and TGF-β expression were both upregulated in Lactobacillus treated chickens before infection with C. perfringens (Brisbin et al., 2008; Shojadoost et al., 2022). IL-12p40 and IL-18 are inducers of IFN-γ which itself modulates αβ and γδ T cells, and mononuclear phagocyte functions. In the absence of IL-12p40, presence of IL-18 can stimulate Th2 cells to produce pro-inflammatory cytokines such as IL-13 (Hoshino et al., 1999; Nakahira and Nakanishi, 2011). This notion is also in agreement with our observation that infection with C. perfringens increased expression of IL-18 and IL-13 and not IL-12p40. Furthermore, immune mediators including leukotrienes, IFN-γ, IL-13 and IL-17a regulate production of mucus, a highly charged gel that acts as a physical barrier with direct toxic activity against many bacteria (Mowat and Agace, 2014). Overall, presence of antibacterial peptides (β-defensins and cathelicidins) and secretory antibodies (IgA and IgY) in the mucus layer serves to kill and promote their expulsion from the intestine (Mowat and Agace, 2014). Defects in mucus synthesis can lead to increased penetration of intestinal microbes into the epithelial surface. TLR2-deficient mice show impaired barrier function and more severe colitis when infected with Campylobacter jejuni (Stahl et al., 2014). Although the recovery of CD4+ T cell in L. lactis treated chickens likely contributed to improved resistance to C. perfringens infection, an association between IL-17/IL-13, IL-12p40/IL-18/IFN-γ, and TGF-β/IL-10 and stage of C. perfringens infection, before and during infection, requires further studies.
The results of the present study suggest that treatment with L. lactis can promote an intestinal environment which is more resistant to intestinal damage. Intestinal B cells expressing TLRs can directly sense commensal microbial associated molecular pattern (MAMPs) which results in antibody production. In addition to inducing differentiation of T cells into regulatory cells, stimulation by regulatory cytokines and co-stimulation supported by MHC class II-dependent presentation of antigens by mononuclear phagocytes promote IgA secretion by intestinal plasma B cells. The IgA isotype is the predominant immunoglobulin synthesized and secreted by LP-plasma B cells (Bos et al., 2001). Irrespective of IgA antibody specificity, active transcytosis of secretory antibodies into the mucus layer acts as an active immune barrier to prevent bacteria from penetrating into the LP (Kroese et al., 1996; Koskinen et al., 1998; Macpherson and Uhr, 2004). The former was observed in Lactobacillus treated chickens thereby demonstrating a conserved function to elicit a protective intestinal mucosal immune response (Haghighi et al., 2006). In this study, both IgA+ and IgY+ B cells were detected predominantly in the chicken duodenum, jejunum and ileum. The increase in both IgA+ and IgY+ B cells alone was not sufficient to limit NE progression during infection with C. perfringens. These results suggest that L. lactis treatment increased local IgA+ and IgY+ B cells frequency within the duodenum, jejunum and ileum similar to SFB and L. lactis treated mice (Norton et al., 1994; Ivanov et al., 2009) likely resulting in secretion of polyreactive antibodies prior to C. perfringens infection.
Taken together, the causal relationships and underlying mechanisms of L. lactis against C. perfringens needs to be further defined, as TLR sensing and antibody-mediated protection might be an important contributor to protective immunity.
Data availability statement
The datasets presented in this study can be found in online repositories. The names of the repository/repositories and accession number(s) can be found in the article/supplementary material.
Ethics statement
All animal experiments were approved (028–10,783 – ISOL and AUP 4328) by the Animal Care Committee of the University of Guelph and adhered to the guidelines for the use of animals. The study was conducted in accordance with the local legislation and institutional requirements.
Author contributions
NB: Conceptualization, Formal analysis, Investigation, Methodology, Writing – original draft, Writing – review & editing, Data curation. BS: Data curation, Investigation, Validation, Visualization, Writing – review & editing, Methodology. MA: Formal analysis, Investigation, Writing – review & editing, Methodology. JA: Investigation, Methodology, Writing – review & editing. SB: Writing – review & editing. SS: Conceptualization, Data curation, Funding acquisition, Resources, Supervision, Validation, Writing – review & editing, Project administration, Visualization.
Funding
The author(s) declare financial support was received for the research, authorship, and/or publication of this article. This research was supported with funds from the Canadian Poultry Research Council, Natural Sciences and Engineering Research Council, Ontario Research Fund-Research Excellence, the Ontario Ministry of Agriculture, Food and Rural Affairs, University of Guelph’s Food from Thought initiative, thanks to funding from the Canada First Research Excellence Fund. SB was supported by funding from the Biotechnology and Biological Sciences Research Council (award no. BBS/E/I/00001825).
Acknowledgments
We acknowledge the isolation facility team at Ontario Veterinary College, University of Guelph for providing constant support with the regular checks and managerial practices for the experimental chickens.
Conflict of interest
The authors declare that the research was conducted in the absence of any commercial or financial relationships that could be construed as a potential conflict of interest.
Publisher’s note
All claims expressed in this article are solely those of the authors and do not necessarily represent those of their affiliated organizations, or those of the publisher, the editors and the reviewers. Any product that may be evaluated in this article, or claim that may be made by its manufacturer, is not guaranteed or endorsed by the publisher.
Footnotes
References
Alizadeh, M., Shojadoost, B., Boodhoo, N., Astill, J., Taha-Abdelaziz, K., Hodgins, D. C., et al. (2021). Necrotic enteritis in chickens: a review of pathogenesis, immune responses and prevention, focusing on probiotics and vaccination. Anim. Health Res. Rev. 22, 147–162. doi: 10.1017/S146625232100013X
Baba, E., Ikemoto, T., Fukata, T., Sasai, K., Arakawa, A., and McDougald, L. R. (1997). Clostridial population and the intestinal lesions in chickens infected with clostridium perfringens and Eimeria necatrix. Vet. Microbiol. 54, 301–308. doi: 10.1016/S0378-1135(96)01289-8
Bacchetti De Gregoris, T., Aldred, N., Clare, A. S., and Burgess, J. G. (2011). Improvement of phylum- and class-specific primers for real-time PCR quantification of bacterial taxa. J. Microbiol. Methods 86, 351–356. doi: 10.1016/j.mimet.2011.06.010
Balic, A., Garcia-Morales, C., Vervelde, L., Gilhooley, H., Sherman, A., Garceau, V., et al. (2014). Visualisation of chicken macrophages using transgenic reporter genes: insights into the development of the avian macrophage lineage. Development 141, 3255–3265. doi: 10.1242/dev.105593
Bermúdez-Humarán, L. G., Kharrat, P., Chatel, J. M., and Langella, P. (2011). Lactococci and lactobacilli as mucosal delivery vectors for therapeutic proteins and DNA vaccines. Microb. Cell Factories 10:S4. doi: 10.1186/1475-2859-10-S1-S4
Boodhoo, N., Gurung, A., and Sharif, S. (2016). Marek’s disease in chickens: a review with focus on immunology. Vet. Res. 47:119. doi: 10.1186/s13567-016-0404-3
Boodhoo, N., Matsuyama-kato, A., Shojadoost, B., Behboudi, S., and Sharif, S. (2022a). The severe acute respiratory syndrome coronavirus 2 non-structural proteins 1 and 15 proteins mediate antiviral immune evasion. Curr. Res. Virol. Sci. 3:100021. doi: 10.1016/j.crviro.2022.100021
Boodhoo, N., Shojadoost, B., Alizadeh, M., Kulkarni, R. R., and Sharif, S. (2022b). Ex vivo differential responsiveness to Clostridium perfringens and Lactococcus lactis by avian small intestine macrophages and T cells. Front. Immunol. 13:807343. doi: 10.3389/fimmu.2022.807343
Bos, N. A., Jiang, H. Q., and Cebra, J. J. (2001). T cell control of the gut IgA response against commensal bacteria. Gut 48, 762–764. doi: 10.1136/gut.48.6.762
Brisbin, J. T., Gong, J., Orouji, S., Esufali, J., Mallick, A. I., Parvizi, P., et al. (2011). Oral treatment of chickens with lactobacilli influences elicitation of immune responses. Clin. Vaccine Immunol. 18, 1447–1455. doi: 10.1128/CVI.05100-11
Brisbin, J. T., Gong, J., Parvizi, P., and Sharif, S. (2010). Effects of lactobacilli on cytokine expression by chicken spleen and cecal tonsil cells. Clin. Vaccine Immunol. 17, 1337–1343. doi: 10.1128/CVI.00143-10
Brisbin, J. T., Gong, J., and Sharif, S. (2008). Interactions between commensal bacteria and the gut-associated immune system of the chicken. Anim. Health Res. Rev. 9, 101–110. doi: 10.1017/S146625230800145X
Cao, H. P., Wang, H. N., Yang, X., Zhang, A. Y., Li, X., Ding, M. D., et al. (2013). Lactococcus lactis anchoring avian infectious bronchitis virus multi-epitope peptide EpiC induced specific immune responses in chickens. Biosci. Biotechnol. Biochem. 77, 1499–1504. doi: 10.1271/bbb.130157
Chalmers, G., Bruce, H. L., Hunter, D. B., Parreira, V. R., Kulkarni, R. R., Jiang, Y. F., et al. (2008). Multilocus sequence typing analysis of Clostridium perfringens isolates from necrotic enteritis outbreaks in broiler chicken populations. J. Clin. Microbiol. 46, 3957–3964. doi: 10.1128/JCM.01548-08
Clavijo, V., and Flórez, M. J. V. (2018). The gastrointestinal microbiome and its association with the control of pathogens in broiler chicken production: a review. Poult. Sci. 97, 1006–1021. doi: 10.3382/ps/pex359
Coffman, R. L., Lebman, D. A., and Shrader, B. (1989). Transforming growth factor beta specifically enhances IgA production by lipopolysaccharide-stimulated murine B lymphocytes. J. Exp. Med. 170, 1039–1044. doi: 10.1084/jem.170.3.1039
Fasina, Y. O., and Lillehoj, H. S. (2019). Characterization of intestinal immune response to Clostridium perfringens infection in broiler chickens. Poult. Sci. 98, 188–198. doi: 10.3382/ps/pey390
Fukata, T., Hadate, Y., Baba, E., Uemura, T., and Arakawa, A. (1988). Influence of Clostridium perfringens and its toxin in germ-free chickens. Res. Vet. Sci. 44, 68–70. doi: 10.1016/0034-5288(88)90015-x
Haghighi, H. R., Gong, J., Gyles, C. L., Hayes, M. A., Zhou, H., Sanei, B., et al. (2006). Probiotics stimulate production of natural antibodies in chickens. Clin. Vaccine Immunol. 13, 975–980. doi: 10.1128/CVI.00161-06
Hoshino, T., Wiltrout, R. H., and Young, H. A. (1999). IL-18 is a potent coinducer of IL-13 in NK and T cells: a new potential role for IL-18 in modulating the immune response. J. Immunol. 162, 5070–5077. doi: 10.4049/jimmunol.162.9.5070
Huang, T., Gao, B., Chen, W. L., Xiang, R., Yuan, M. G., Xu, Z. H., et al. (2018). Temporal effects of high fishmeal diet on gut microbiota and immune response in clostridium perfringens-challenged chickens. Front. Microbiol. 9:2754. doi: 10.3389/fmicb.2018.02754
Ivanov, I. I., Atarashi, K., Manel, N., Brodie, E. L., Shima, T., Karaoz, U., et al. (2009). Induction of intestinal Th17 cells by segmented filamentous Bacteria. Cells 139, 485–498. doi: 10.1016/j.cell.2009.09.033
Kamada, N., Chen, G. Y., Inohara, N., and Núñez, G. (2013). Control of pathogens and pathobionts by the gut microbiota. Nat. Immunol. 14, 685–690. doi: 10.1038/ni.2608
Keyburn, A. L., Boyce, J. D., Vaz, P., Bannam, T. L., Ford, M. E., Parker, D., et al. (2008). NetB, a new toxin that is associated with avian necrotic enteritis caused by Clostridium perfringens. PLoS Pathog. 4:e26. doi: 10.1371/journal.ppat.0040026
Kim, S., Faris, L., Cox, C. M., Sumners, L. H., Jenkins, M. C., Fetterer, R. H., et al. (2012). Molecular characterization and immunological roles of avian IL-22 and its soluble receptor IL-22 binding protein. Cytokine 60, 815–827. doi: 10.1016/j.cyto.2012.08.005
Kono, H., and Rock, K. L. (2008). How dying cells alert the immune system to danger. Nat. Rev. Immunol. 8, 279–289. doi: 10.1038/nri2215
Koskinen, R., Göbel, T. W., Tregaskes, C. A., Young, J. R., and Vainio, O. (1998). The structure of avian CD5 implies a conserved function. J. Immunol. 160, 4943–4950. doi: 10.4049/jimmunol.160.10.4943
Kroese, F. G. M., de Waard, R., and Bos, N. A. (1996). B-1 cells and their reactivity with the murine intestinal microflora. Semin. Immunol. 8, 11–18. doi: 10.1006/smim.1996.0003
Lahiri, A., Sharif, S., and Mallick, A. I. (2019). Intragastric delivery of recombinant Lactococcus lactis displaying ectodomain of influenza matrix protein 2 (M2e) and neuraminidase (NA) induced focused mucosal and systemic immune responses in chickens. Mol. Immunol. 114, 497–512. doi: 10.1016/j.molimm.2019.08.015
Lathrop, S. K., Bloom, S. M., Rao, S. M., Nutsch, K., Lio, C. W., Santacruz, N., et al. (2011). Peripheral education of the immune system by colonic commensal microbiota. Nature 478, 250–254. doi: 10.1038/nature10434
Lee, Y., Kim, W. H., Lee, S., and Lillehoj, H. S. (2018). Detection of chicken interleukin-10 production in intestinal epithelial cells and necrotic enteritis induced by Clostridium perfringens using capture ELISA. Vet. Immunol. Immunopathol. 204, 52–58. doi: 10.1016/j.vetimm.2018.10.001
Lee, Y. K., and Mazmanian, S. K. (2010). Has the microbiota played a critical role in the evolution of the adaptive immune system? Science 330, 1768–1773. doi: 10.1126/science.1195568
Lillehoj, H. S., and Trout, J. M. (1996). Avian gut-associated lymphoid tissues and intestinal immune responses to Eimeria parasites. Clin. Microbiol. Rev. 9, 349–360. doi: 10.1128/cmr.9.3.349
Lu, Y., Sarson, A. J., Gong, J., Zhou, H., Zhu, W., Kang, Z., et al. (2009). Expression profiles of genes in toll-like receptor-mediated signaling of broilers infected with Clostridium perfringens. Clin. Vaccine Immunol. 16, 1639–1647. doi: 10.1128/CVI.00254-09
Macdonald, S. E., Nolan, M. J., Harman, K., Boulton, K., Hume, D. A., Tomley, F. M., et al. (2017). Effects of Eimeria tenella infection on chicken caecal microbiome diversity, exploring variation associated with severity of pathology. PLoS One 12:e0184890. doi: 10.1371/journal.pone.0184890
Macpherson, A. J., and Uhr, T. (2004). Induction of protective IgA by intestinal dendritic cells carrying commensal Bacteria. Science 303, 1662–1665. doi: 10.1126/science.1091334
Mowat, A. M. I. (2003). Anatomical basis of tolerance and immunity to intestinal antigens. Nat. Rev. Immunol. 3, 331–341. doi: 10.1038/nri1057
Mowat, A. M., and Agace, W. W. (2014). Regional specialization within the intestinal immune system. Nat. Rev. Immunol. 14, 667–685. doi: 10.1038/nri3738
Nakahira, M., and Nakanishi, K. (2011). Requirement of GATA-binding protein 3 for Il13 gene expression in IL-18-stimulated T H1 cells. Int. Immunol. 23, 761–772. doi: 10.1093/intimm/dxr087
Norton, P. M., Brown, H. W. G., and le Page, R. W. F. (1994). The immune response to Lactococcus lactis: implications for its use as a vaccine delivery vehicle. FEMS Microbiol. Lett. 120, 249–256. doi: 10.1111/j.1574-6968.1994.tb07041.x
Oakley, B. B., Lillehoj, H. S., Kogut, M. H., Kim, W. K., Maurer, J. J., Pedroso, A., et al. (2014). The chicken gastrointestinal microbiome. FEMS Microbiol. Lett. 360, 100–112. doi: 10.1111/1574-6968.12608
Oh, S., Lillehoj, H. S., Lee, Y., Bravo, D., and Lillehoj, E. P. (2019). Dietary antibiotic growth promoters Down-regulate intestinal inflammatory cytokine expression in chickens challenged with LPS or co-infected with Eimeria maxima and Clostridium perfringens. Front. Vet. Sci. 6:420. doi: 10.3389/fvets.2019.00420
Parish, W. E. (1961). Necrotic enteritis in the fowl (Gallus gallus domesticus). I. Histopathology of the disease and isolation of a strain of Clostridium welchii. J. Comp. Pathol. 71, 377–393.
Park, S. S., Lillehoj, H. S., Allen, P. C., Dong, W. P., FitzCoy, S., Bautista, D. A., et al. (2008a). Immunopathology and cytokine responses in broiler chickens coinfected with Eimeria maxima and Clostridium perfringens with the use of an animal model of necrotic enteritis. Avian Dis. 52, 14–22. doi: 10.1637/7997-041707-Reg
Park, S. S., Lillehoj, H. S., Allen, P. C., Park, D. W., FitzCoy, S., Bautista, D. A., et al. (2008b). Immunopathology and cytokine responses in broiler chickens Coinfected with Eimeria maxima and Clostridium perfringens with the use of an animal model of necrotic enteritis. Avian Dis. 52, 14–22. doi: 10.1637/7997-041707-reg
Parvizi, P., Andrzejewski, K., Read, L. R., Behboudi, S., and Sharif, S. (2010). Expression profiling of genes associated with regulatory functions of T-cell subsets in Marek’s disease virus-infected chickens. Avian Pathol. 39, 367–373. doi: 10.1080/03079457.2010.508776
Reeves, A. E., Theriot, C. M., Bergin, I. L., Huffnagle, G. B., Schloss, P. D., and Young, V. B. (2011). The interplay between microbiome dynamics and pathogen dynamics in a murine model of Clostridium difficile infection. Gut Microbes 2, 145–158. doi: 10.4161/gmic.2.3.16333
Rescigno, M. (2011). The intestinal epithelial barrier in the control of homeostasis and immunity. Trends Immunol. 32, 256–264. doi: 10.1016/j.it.2011.04.003
Round, J. L., and Mazmanian, S. K. (2010). Inducible Foxp3+ regulatory T-cell development by a commensal bacterium of the intestinal microbiota. Proc. Natl. Acad. Sci. U. S. A. 107, 12204–12209. doi: 10.1073/pnas.0909122107
Shojadoost, B., Alizadeh, M., Boodhoo, N., Astill, J., Karimi, S. H., Shoja Doost, J., et al. (2022). Effects of treatment with lactobacilli on necrotic enteritis in broiler chickens. Probiotics Antimicrob. Proteins. 14, 1110–1129. doi: 10.1007/s12602-021-09901-5
Shojadoost, B., Vince, A. R., and Prescott, J. F. (2012). The successful experimental induction of necrotic enteritis in chickens by Clostridium perfringens: a critical review. Vet. Res. 43:74. doi: 10.1186/1297-9716-43-74
Song, A. A. L., In, L. L. A., Lim, S. H. E., and Rahim, R. A. (2017). A review on Lactococcus lactis: from food to factory. Microb. Cell Factories 16:55. doi: 10.1186/s12934-017-0669-x
St Paul, M., Barjesteh, N., Paolucci, S., Pei, Y., and Sharif, S. (2012a). Toll-like receptor ligands induce the expression of interferon-gamma and interleukin-17 in chicken CD4+ T cells. BMC. Res. Notes 5:616. doi: 10.1186/1756-0500-5-616
St Paul, M., Paolucci, S., Barjesteh, N., Wood, R. D., Schat, K. A., and Sharif, S. (2012b). Characterization of chicken thrombocyte responses to toll-like receptor ligands. PLoS One 7:e43381. doi: 10.1371/journal.pone.0043381
Stahl, M., Ries, J., Vermeulen, J., Yang, H., Sham, H. P., Crowley, S. M., et al. (2014). A novel mouse model of Campylobacter jejuni gastroenteritis reveals key pro-inflammatory and tissue protective roles for toll-like receptor signaling during infection. PLoS Pathog. 10:e1004264. doi: 10.1371/journal.ppat.1004264
Stanley, D., Keyburn, A. L., Denman, S. E., and Moore, R. J. (2012). Changes in the caecal microflora of chickens following Clostridium perfringens challenge to induce necrotic enteritis. Vet. Microbiol. 159, 155–162. doi: 10.1016/j.vetmic.2012.03.032
Tap, J., Mondot, S., Levenez, F., Pelletier, E., Caron, C., Furet, J. P., et al. (2009). Towards the human intestinal microbiota phylogenetic core. Environ. Microbiol. 11, 2574–2584. doi: 10.1111/j.1462-2920.2009.01982.x
Uzal, F. A., Freedman, J. C., Shrestha, A., Theoret, J. R., Garcia, J., Awad, M. M., et al. (2014). Towards an understanding of the role of Clostridium perfringens toxins in human and animal disease. Future Microbiol. 9, 361–377. doi: 10.2217/fmb.13.168
van Immerseel, F., de Buck, J., Pasmans, F., Huyghebaert, G., Haesebrouck, F., and Ducatelle, R. (2004). Clostridium perfringens in poultry: an emerging threat for animal and public health. Avian Pathol. 33, 537–549. doi: 10.1080/03079450400013162
Wang, H., Latorre, J. D., Bansal, M., Abraha, M., Al-Rubaye, B., Tellez-Isaias, G., et al. (2019). Microbial metabolite deoxycholic acid controls Clostridium perfringens-induced chicken necrotic enteritis through attenuating inflammatory cyclooxygenase signaling. Sci. Rep. 9:14541. doi: 10.1038/s41598-019-51104-0
Wise, M. G., and Siragusa, G. R. (2005). Quantitative detection of Clostridium perfringens in the broiler fowl gastrointestinal tract by real-time PCR. Appl. Environ. Microbiol. 71, 3911–3916. doi: 10.1128/AEM.71.7.3911-3916.2005
Keywords: intestinal immunity, Clostridium Perfringens, Lactococcus Lactis, mucosal response, chickens, T cells, macrophages
Citation: Boodhoo N, Shojadoost B, Alizadeh M, Astill J, Behboudi S and Sharif S (2023) Effect of treatment with Lactococcus lactis NZ9000 on intestinal microbiota and mucosal immune responses against Clostridium perfringens in broiler chickens. Front. Microbiol. 14:1257819. doi: 10.3389/fmicb.2023.1257819
Edited by:
Debabrata Biswas, University of Maryland, United StatesReviewed by:
Muhammad Suleman, University of Veterinary and Animal Sciences, PakistanDinesh Kumar Dahiya, Roswell Park Comprehensive Cancer Center, United States
Copyright © 2023 Boodhoo, Shojadoost, Alizadeh, Astill, Behboudi and Sharif. This is an open-access article distributed under the terms of the Creative Commons Attribution License (CC BY). The use, distribution or reproduction in other forums is permitted, provided the original author(s) and the copyright owner(s) are credited and that the original publication in this journal is cited, in accordance with accepted academic practice. No use, distribution or reproduction is permitted which does not comply with these terms.
*Correspondence: Shayan Sharif, Shayan@uoguelph.ca