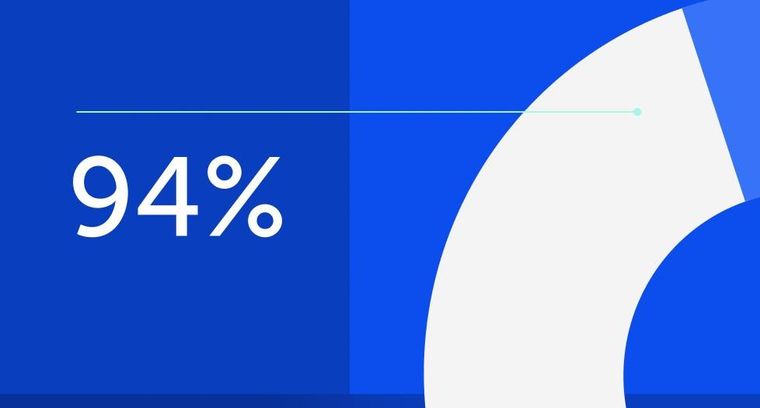
94% of researchers rate our articles as excellent or good
Learn more about the work of our research integrity team to safeguard the quality of each article we publish.
Find out more
ORIGINAL RESEARCH article
Front. Microbiol., 13 December 2023
Sec. Extreme Microbiology
Volume 14 - 2023 | https://doi.org/10.3389/fmicb.2023.1253436
This article is part of the Research TopicThe Impact of the Space Environment on Microbial Growth and BehaviorView all 13 articles
Planetary protection is a guiding principle aiming to prevent microbial contamination of the solar system by spacecraft (forward contamination) and extraterrestrial contamination of the Earth (backward contamination). Bioburden reduction on spacecraft, including cruise and landing systems, is required to prevent microbial contamination from Earth during space exploration missions. Several sterilization methods are available; however, selecting appropriate methods is essential to eliminate a broad spectrum of microorganisms without damaging spacecraft components during manufacturing and assembly. Here, we compared the effects of different bioburden reduction techniques, including dry heat, UV light, isopropyl alcohol (IPA), hydrogen peroxide (H2O2), vaporized hydrogen peroxide (VHP), and oxygen and argon plasma on microorganisms with different resistance capacities. These microorganisms included Bacillus atrophaeus spores and Aspergillus niger spores, Deinococcus radiodurans, and Brevundimonas diminuta, all important microorganisms for considering planetary protection. Bacillus atrophaeus spores showed the highest resistance to dry heat but could be reliably sterilized (i.e., under detection limit) through extended time or increased temperature. Aspergillus niger spores and D. radiodurans were highly resistant to UV light. Seventy percent of IPA and 7.5% of H2O2 treatments effectively sterilized D. radiodurans and B. diminuta but showed no immediate bactericidal effect against B. atrophaeus spores. IPA immediately sterilized A. niger spores, but H2O2 did not. During VHP treatment under reduced pressure, viable B. atrophaeus spores and A. niger spores were quickly reduced by approximately two log orders. Oxygen plasma sterilized D. radiodurans but did not eliminate B. atrophaeus spores. In contrast, argon plasma sterilized B. atrophaeus but not D. radiodurans. Therefore, dry heat could be used for heat-resistant component bioburden reduction, and VHP or plasma for non-heat-resistant components in bulk bioburden reduction. Furthermore, IPA, H2O2, or UV could be used for additional surface bioburden reduction during assembly and testing. The systemic comparison of sterilization efficiencies under identical experimental conditions in this study provides basic criteria for determining which sterilization techniques should be selected during bioburden reduction for forward planetary protection.
Space exploration missions are promoted to comply with the Planetary Protection Policy and Requirements established by the Committee on Space Research (COSPAR) to ensure that future scientific investigations related to the chemical evolution of the solar-system bodies and the origin and distribution of life are not compromised (DeVincenzi et al., 1998; Nicholson et al., 2009; Kminek et al., 2019; COSPAR, 2021; Coustenis et al., 2023). The microbial contamination (bioburden) of flight systems, including landers or rovers, must be below the acceptable limit, especially for missions to Mars, Europa, Enceladus, and other solar-system bodies where the existence of extant life cannot be excluded (National Research Council, 2006; Grotzinger et al., 2012; European Cooperation for Space Standardization, 2019; Kminek et al., 2019; Rettberg et al., 2019; NASA, 2021, 2022).
Several sterilization methods are available for bioburden reduction, each with different mechanisms of effect, advantages, and disadvantages. Thus, a comparative study on sterilization methods against different microorganisms is essential to selecting appropriate techniques for bioburden reduction. Dry heat has been the standard method for sterilizing spacecraft since the Viking lander (Barengoltz and Stabekis, 1983; Grotzinger et al., 2012; European Cooperation for Space Standardization, 2013a; Shirey et al., 2017). However, this method is not applicable to non-heat-resistant materials such as electronics (European Cooperation for Space Standardization, 2013a): Dry heat also negatively affects metals, such as accelerated aging and possible softening (European Cooperation for Space Standardization, 2008a). UV irradiation is also widely used as a disinfection method. However, UV light can cause component surface degradation and cannot reach shaded areas. Alcohol disinfection is commonly used for bioburden reduction during spacecraft assembly, such as for the Mars Science Laboratory or Mars 2020 (Grotzinger et al., 2012; Farley et al., 2020); however, alcohol wets the object and only disinfects the surfaces. Isopropyl alcohol (IPA) is considered to be preferable over ethanol because some bacteria may use ethanol as a carbon source (Mogul et al., 2018). Treatment with aqueous solution and vapor of hydrogen peroxide (H2O2) is a non-residual method because it degrades into water and oxygen (Rohatgi et al., 2002; Kempf et al., 2005; Chung et al., 2006, 2008; European Cooperation for Space Standardization, 2013b; Rummel and Pugel, 2019). However, this method requires a high chemical concentration and may alter the material, including surface oxidation, strength loss, or volume change (European Cooperation for Space Standardization, 2008a). Plasma sterilization has the advantages of no residual chemicals and minimal adverse effects on components (Moisan et al., 2001, 2002; Hayashi et al., 2018; Choudhury et al., 2023); however, quantitative knowledge is still limited, and plasma sterilization has not been tested for spacecraft bioburden reduction.
We selected four microorganisms that are considered to be important in planetary protection based on previous studies (Patel et al., 2017; Craven et al., 2021; NASA, 2022): Bacillus atrophaeus is a gram-positive and spore forming bacterium, and its endogenous spores are commonly used for testing the resistance to dry heat, ethylene oxide, steam, and radiation (Setlow, 2006; Craven et al., 2021; NASA, 2022). In planetary protection, bioburden reduction aims to minimize the presence of entire microorganisms on spacecraft, with a specific focus on reducing the number of microbial spores that can survive a wet heat shock at 80°C for 15 min. This focus is considered crucial because spores have the capability to endure extremely harsh conditions for long periods (e.g., over 10,000 years), and in fact, for the mission of potentially habitable planet, the number of spores per spacecraft is documented in the Planetary Protection Policy (European Cooperation for Space Standardization, 2008b; Benardini et al., 2014a,b; Planetary Protection Independent Review Board, 2019). Deinococcus radiodurans is a gram-positive, non-spore forming bacterium known for their ability to survive exposure to strong radiation and desiccation in space (Anderson, 1956; Makarova et al., 2001; Cox and Battista, 2005; Kawaguchi et al., 2020). A gram-negative bacterium, Brevundimonus diminuta is a standard organism for validating the quality of sterilizing-grade membrane filters because its small cell size and monodispersed with a narrow size distribution (Sandle, 2013; Ryan and Pembroke, 2018; Roy, 2019). Aspergillus niger is a filamentous fungus whose spores are highly pigmented, resistant to UV-C radiation, and easily dispersed in the air (Schuster et al., 2002; Cortesão et al., 2020).
In addition to their different physiological properties, the four microorganisms have actually been detected in spacecraft related cleanrooms or labs. While a wide variety of microorganisms have been detected in spacecraft assembly and other related facilities by various methods, including incubation, DNA-based detection, and MALDI-TOF mass spectrometry, Bacillus spp. are often reported as the predominant species (Puleo et al., 1977; Venkateswaran et al., 2001; La Duc et al., 2004; Moissl-Eichinger et al., 2015; Koskinen et al., 2017; Hendrickson et al., 2021; Wood et al., 2021; Schubert et al., 2023). Of the bacteria detected on the six Mars spacecrafts, approximately 30% of those belonged to the genus Bacillus, 1–2% to the genus Brevundimonas, and 1–2% to the genus Deinococcus (Schwendner et al., 2020). Kminek et al. reported that the genera Bacillus and Brevundimonas accounted for 9 and 4% of the microorganisms in the cleanroom, respectively (Kminek et al., 2019). The genus Deinococcus was also often detected, and reported that they were in all NASA’s cleanrooms at the Jet Propulsion Laboratory, Kennedy Space Center, and Johnson Space Center (Moissl et al., 2007; Wood et al., 2021). Cultivable fungi were also detected in the cleanroom in spacecraft assembly facility of NASA (Hendrickson et al., 2017). Regberg et al. (2018) reported that between 83 and 97% of the microorganisms were fungi in the meteorite lab cleanrooms at NASA.
To select suitable sterilization techniques for bioburden reduction for forward planetary protection, we compared the effects of dry heat, UV light, IPA, H2O2, VHP, and oxygen and argon plasma on four different microorganisms in this study. A colony counting method, gold standard for sterilization tests, was used to evaluate living organisms after microbial reduction treatment (European Cooperation for Space Standardization, 2008b; Patel et al., 2017; Chen et al., 2023). Bacillus atrophaeus and A. niger were used in the spore state as represented the bacterial and fungal spores. Deinococcus radiodurans and B. diminuta, which do not form spores, were used as dried vegetative cells. Based on comprehensive and systematic information, we proposed the optimal bioburden reduction process of spacecraft.
A list of the microorganisms used in this study is shown in Supplementary Table S1. Bacillus atrophaeus NBRC 13721, D. radiodurans NBRC 15346, and B. diminuta NBRC 14213 were purchased from NITE Biological Resource Center (NBRC; Chiba, Japan). Aspergillus niger ATCC 16888 was purchased from Microbiologics (St. Cloud, MN, United States). Deinococcus radiodurans and B. diminuta were cultivated in NBRC No. 702 liquid medium [10 g Hipolypepton (390-02116, Fujifilm Wako, Japan), 2 g yeast extract, and 1 g MgSO4·7H2O per liter; pH 7.0] at 30°C, with shaking at 120 rpm (WB-205QMC, WakenBtech, Japan). Bacillus atrophaeus spores were harvested from the agar plates after 10 days of incubation at 37°C for spore formation [8 g nutrient broth, 4 g yeast extract, 0.0157 g MnCl2·4H2O, and 15 g agar/L] by gently scraping with sterilized saline solution (0.9% NaCl) using a cell spreader. The spores were washed thrice with saline solution, incubated at 80°C for 15 min, washed twice with ethanol, and rewashed with saline solution. Aspergillus niger spores were harvested after 10 days of incubation at 25°C from NBRC No. 5 agar plates [20 g malt extract, 20 g glucose, 1 g peptone, and 20 g agar/L; pH 6.0] by gently stroking with a droplet of 0.05% TritonX-100 solution using a Pasteur pipette. The spores were suspended in a sterilized saline solution (0.9% NaCl). Spore formation was determined using phase contrast microscopy (IX73, Olympus, Japan) and the spore content was approximately 90%.
The vegetative cell suspensions (10 or 50 μL) of mid-log phase cultures with an optical density of 0.4 (CO7500, Funakoshi, Japan) of D. radiodurans and B. diminuta and the 10 μL of B. atrophaeus spore solutions and 50 μL of A. niger spore solutions were dropped onto glass slides and aluminum plates with holes (φ18 mm and a depth of 2.8 mm) and air-dried for over 1 h in biological safety cabinets (AC2-4 N7, Esco, Singapore). The glass slide coupons were used for bioburden reduction experiments using dry heat, UV, vaporized hydrogen peroxide (VHP), and oxygen and argon plasma (Figure 1). The aluminum plate coupons were used for the bioburden reduction experiments using 70% IPA and 7.5% H2O2. Approximately 6–7 log orders of bacterial cells (including spores) and 4–5 log orders of fungal spores per 1 cm in diameter were dried on each plate. Coupons used as controls were prepared at the same time as the samples, stored in desiccators at a relative humidity of ≤30% and a temperature of 22.8°C, and incubated for the same time as the samples. Drying, microbial reduction treatment, collection, and evaluation of live cells were performed within 30 days for the plasma sterilization test and 7 days for the other tests. After drying, survival during inoculated coupons storage was examined by placing them in the desiccators. The thicknesses of surface-attached microbial cells or spores were measured by calculating the average height over 100 μm horizontally (n ≥ 8) using a laser microscope (VK-X 100, Keyence, Japan).
Dry heat treatment was performed in a dry oven (MOV-212S, Sanyo, Japan). The treatment time was measured from when the samples were placed in the preheated oven (100, 112, 120, 140, 160, and 180°C) until removal. Inoculated coupons were covered in aluminum foil throughout the treatment. After the treatment, inoculated coupons were removed from the oven and cooled at 22.8°C. The surface temperatures on the inoculated coupons were measured using a data logger (GL240, Graph Tech, Japan).
UV irradiation was performed using a UV lamp with a 253.7 nm wavelength (GL 15, Toshiba, Japan) mounted on a clean bench (MCV-131BNS, Sanyo, Japan). The UV intensity was measured using a data logger (YK-37UVSD, Mothertool, Japan), and the UV dose was adjusted to 1.26 J/cm2/h. The relative humidity was <60%. Throughout the treatment, inoculated coupons were not exposed to intense light sources other than the UV lamp to prevent the photoactivation of the cellular DNA repair systems mediated by photolyase.
Alcohol and H2O2 treatment were performed by adding 250 μL of 70% IPA (2-propanol; 32435-2B, Kanto Chemical, Japan) and 7.5% H2O2 (20779-65, Nacalai Tesque, Japan) to the samples, respectively. The concentrations followed the methods of the Mars Organic Molecule Analyzer for bioburden-controlled clean rooms (Lalime and Berlin, 2016). After the treatments, each sample was diluted with sterilized saline solution (0.9% NaCl) to >200-fold for IPA and > 20,000-fold for H2O2 to avoid cell death by residual IPA or H2O2. We have confirmed that microbicidal activities did not continue under each diluted condition.
Vaporized hydrogen peroxide treatments were performed using a vacuum chamber (diameter: 210 mm; length: 495 mm; volume: 17.1 L). The chamber was evacuated, and 35% of liquid H2O2 was supplied from the top of the reactor through continuous evacuation using a vacuum pump and was gasified under a pressure of 400 ± 20 Pa. The temperature inside the vacuum chamber was maintained at 22.8°C. Hence, the relative humidity and concentration of VHP in the treatment were calculated as <11.6% RH and 1.07 ± 0.06 mg/L, respectively. The total moles of mixed gas comprising H2O and H2O2 derived from 35% H2O2 (by weight) were calculated as 2.44 mmol using the ideal gas law as the pressure in the evacuated chamber was 50 Pa without 35% H2O2. Subsequently, the moles of VHP molecules in the chamber were determined as 0.54 mmol (18.4 mg) according to Dalton’s law. The concentration-time (CT) values of VHP treatment were calculated as the product of VHP concentration and exposure time.
Plasma treatments were performed using a capacitively coupled radio-frequency discharge reactor (Hayashi et al., 2006, 2018). The discharge electrode was a serpentine folding-shaped stainless-steel wire (diameter: 4 mm; total length: 1,800 mm) placed approximately 20 mm beneath the upper wall of the reactor. Pure oxygen or argon gas was supplied from the top of the reactor with a pressure of 60 Pa. A radio-frequency voltage of 13.56 MHz was applied to the discharge electrode. The discharge power was maintained at 80 W. The temperature inside the vacuum chamber was maintained at <65°C. The samples were placed 90 mm below the top of the discharge electrode.
After each microbicidal treatment, the microbial cells attached to inoculated coupons were collected by pipetting in triplicate using a total of 100 μL NBRC No. 702 medium. After dilution with NBRC No. 702 media, B. atrophaeus, B. diminuta, and D. radiodurans cells were inoculated on NBRC No. 802 agar plates [pH 7.0, composition: 10 g hipolypepton (390-02116, Fujifilm Wako, Japan), 2 g yeast extract, and 1 g MgSO4·7H2O, and 15 g agar/L]. Aspergillus niger spores were inoculated on potato dextrose agar plates (Solabia, France). The agar plates were incubated at 30°C for bacteria and 25°C for fungi in an incubator (IC602, Yamato, Japan): 24–48 h for B. atrophaeus cells and 72–96 h for the other microorganisms. The resultant colonies were counted (Scan 300, Interscience, France) to calculate the survival rate. All experiments were performed simultaneously on two dry microbial pellets, and the values were averaged. All experiments were repeated at least once for confirmation of repeatability. Cells collection was confirmed by colony counting and the repeatability was approximately 80%.
During dry or moist heat treatment, the thermal death of microbial cells generally follows the first-order of kinetics (Pflug et al., 2001) as described by Equation (1)
where N and t are the viable cell number and time (min or h), respectively, and kT is the death rate constant (min−1 or h−1) at a constant heating temperature T (°C). When taking the integral of both sides of Equation (1), Equation (2) is obtained using N0 as the initial viable cell number.
The D-value (DT, min or h) is the time required to reduce a microbial population by 10-fold at heating temperature T and is calculated from the slope of the line obtained from the logarithmic plot of survival rate, log (N/N0) against t. Therefore, Equation (3) can be described by Stoforos (2015) as:
The z-value (°C) is defined as the change in temperature that will increase the D-value by a factor of 10 and is also calculated from the slope of the line obtained from the plot of log DT against T.
The F-value (min or h) is the heating time equivalent at any heating temperature T for a specified z-value and reference temperature TR (Moldenhauer, 2013). Generally, the F-value is obtained through Equation (4),
where LT is the lethal rate defined as the constant death rate at temperature T divided by that of reference temperature TR. When T is a constant value, Equation (5) is obtained.
During heating and cooling periods, thermal processes were performed under time-varying temperatures T(t), and the F-value is obtained by Equation (6), where FP is the F-value of the process, which also approximates Equation (7).
In Equation (7), is the average temperature of the process at the time interval . Additionally, using Equation (5), the FP-values between two thermal processes with different processing temperatures T1 and T2 can be described as Equation (8).
Generally, when the F-value of the dry heat sterilization is calculated, a z-value of 20°C is used as that temperature is indicated as the z-value of the bacterial spores (Gould, 2004).
The FP-value of each dry heat treatment was obtained using Equation (7). The total processing time, t, was first divided into n pieces of segments, each with an interval of 20 s. Subsequently, L-values at the average temperature of each time segment were calculated and added (Supplementary Table S2; Supplementary Figures S1, S2). In this calculation, the processing temperature of each dry heat treatment was considered as each reference temperature TR, and a z-value of 20°C was used. When the processing time value, t, was markedly shorter than the obtained FP, survival rate data at that time were not used to estimate the D-values.
No substantial changes in the colony-forming units of dried B. atrophaeus and D. radiodurans cells were observed after a storage period of over 30 days (Supplementary Figure S3). The change in dried A. niger after 30 days of storage was less than one log reduction. In contrast, dried B. diminuta cells showed a one log reduction after 7 days of storage and an approximately three log reduction after 1 month. Supplementary Table S3 shows the thickness of dried cells (approximately 1 cm in diameter) on the glass slide used in the bioburden reduction tests. The dried D. radiodurans and B. diminuta cells tended to become thicker than those of B. atrophaeus and A. niger. The cells thicknesses of B. atrophaeus and A. niger locally exceeded 10 μm due to clumped cells.
While each dry heat treatment was set at 100, 112, 120, 140, 160, and 180°C, the actual temperatures were 100.8 ± 0.2, 113.4 ± 0.2, 122.0 ± 0.3, 141.9 ± 0.5, 161.3 ± 0.6, and 181.8 ± 0.4°C, respectively. The results for dry heat treatment set at 100-, 112-, and 120°C for B. atrophaeus are shown in Figure 2A. The reduction curves obtained from these temperatures were linear for two or three log orders. Subsequent microbial reduction showed tailing. The D-values for 100-, 112-, and 120°C-heat treatments were calculated as 604, 163, and 65.8 min, respectively. The dry heat treatment of B. atrophaeus exposed to 140-, 160-, and 180°C are shown in Figure 2B. Over six log orders of spores could be inactivated at 140°C for 5 h, 160°C for 45 min, and 180°C for 6 min. However, the D-values could not be determined for these treatments because the survival rate values dropped before the temperature of the inoculated coupons reached a steady state (Supplementary Table S2; Supplementary Figures S1, S2). Deinococcus radiodurans, B. diminuta, and A. niger dry heat treatment results are shown in Figure 2C. The three microorganisms were sterilized (i.e., under detection limit) more rapidly than B. atrophaeus (Table 1). All strains tested in this study were sterilized using a dry heat set at 120°C and above.
Figure 2. Microbial reduction after dry heat treatment. Reduction curves of Bacillus atrophaeus spores heated using a 100-, 112-, and 120°C-oven (A) and a 140-, 160-, and 180°C-oven (B). Reduction curves of Deinococcus radiodurans, Brevundimonas diminuta, and Aspergillus niger spores (C).
Table 1. Treatment time required to sterilize the surficial bioburden using dry heat, UV irradiation, and treatment with antimicrobial agents, including vaporized hydrogen peroxide (VHP) and oxygen- and argon plasma.
The reduction curve of UV treatment is shown in Figure 3. The irradiation intensity measured by the UV lamp at 32.7 cm was 0.35 ± 0.1 mW/cm2, equal to 1 J/cm2 for 47.62 min of irradiation. An approximately five log reduction was observed in B. atrophaeus and B. diminuta after a UV irradiation of 2.5 J/cm2 (2 h irradiation). In contrast, UV light irradiation resistance was observed for D. radiodurans and A. niger. For both strains, a tailing of the survival curve was observed after the 1.5–2 log reduction in viable cells at a UV dose of 1.26 J/cm2 (1 h irradiation). Deinococcus radiodurans required a UV dose of 37.8 J/cm2 (irradiate for 30 h) for sterilization, whereas A. niger remained at a 2–3 log reduction in viable cells after the same treatment. These results suggested that the UV sterilization was effective for B. diminuta and B. atrophaeus but relatively ineffective for D. radiodurans and A. niger (Table 1), differing from the dry heat treatment results.
Figure 3. Microbial reduction after UV light irradiation of dry Bacillus atrophaeus spores, Deinococcus radiodurans, Brevundimonas diminuta, and Aspergillus niger spores.
The reduction curve after alcohol treatment is shown in Figure 4A. Seventy percent IPA solution was dropped onto the dried cells, and no suspension or wiping treatment was performed. Brevundimonas diminuta, D. radiodurans, and A. niger were sterilized quickly within a few minutes. However, no B. atrophaeus sporicidal activity was observed even when IPA infiltration was continued for 120 min.
Figure 4. Microbial reduction after 70% of isopropyl alcohol (IPA; A) and 7.5% hydrogen peroxide (H2O2) treatments (B) of dry Bacillus atrophaeus spores, Deinococcus radiodurans, Brevundimonas diminuta, and Aspergillus niger spores. *Due to the dilution associated with processing steps, the dynamic range is <5 log order.
The reduction value of viable cells after H2O2 treatment is shown in Figure 4B. H2O2 treatment was performed similarly to the IPA treatment. Brevundimonas diminuta and D. radiodurans were also sterilized quickly. In contrast, B. atrophaeus and A. niger microbicidal activities were scarcely observed after 10 min of treatment. Continuous H2O2 treatments inactivated more than four log orders of A. niger spores after 30 min and over six log orders of B. atrophaeus spores in almost all tests after 60 min (data not shown).
Vaporized hydrogen peroxide treatment was performed under low pressure (400 ± 20 Pa) at 22.8°C with a relative humidity of ≤11.6%. VHP was continuously supplied and exhausted at 0.93 ± 0.06 mg/L/min throughout the treatment (Supplementary Figure S4). The VHP concentration was maintained at 1.07 ± 0.06 mg/L in the chamber. The CT value of the treatment increased by 64.2 mg·min/L every hour.
The VHP treatment results are shown in Figure 5. Brevundimonas diminuta and D. radiodurans were immediately sterilized; however, A. niger and B. atrophaeus were inactivated by one to two log orders after 10 min of exposure, corresponding to the CT value of 10.7 mg∙min/L, and further effect was not observed. No substantial reduction was observed in vacuum-only treatment for all microorganisms in this study.
Figure 5. Microbial reduction after vaporized hydrogen peroxide (VHP) treatments of dry Bacillus atrophaeus spores, Deinococcus radiodurans, Brevundimonas diminuta, and Aspergillus niger spores.
Temperatures in the vacuum chamber during oxygen- and argon plasma treatment were increased during the first 20 min and reached constant values of 64 and 59°C, respectively. The reduction curves of test strains after oxygen plasma treatment are shown in Figure 6A. Aspergillus niger was sterilized after 1.3 h of treatment. Deinococcus radiodurans showed a reduction curve with a shoulder and was sterilized after 8 h. Bacillus atrophaeus showed a reduction curve with a tailing, and approximately two and four log orders of reduction in survival spores were observed at 1.3 and 8 h, respectively. The reduction curve after argon plasma treatment is shown in Figure 6B. Approximately 1.5 log orders of D. radiodurans cells were killed after 8 h of treatment; however, sterilization was incomplete under these conditions. In contrast, B. atrophaeus and A. niger were sufficiently sterilized within 4 h. Plasma treatment can reduce B. atrophaeus spores by three or more log order; however, the survival of D. radiodurans must be investigated. Brevundimonas diminuta sterilization using oxygen- and argon plasma treatment has been confirmed with at least two log reductions (data not shown); however, sufficient initial cell numbers were not prepared because B. diminuta died during dry storage (Supplementary Figure S3).
Figure 6. Microbial reduction after oxygen (A) and argon plasma treatments (B) of dry Bacillus atrophaeus spores, Deinococcus radiodurans, and Aspergillus niger spores.
A spacecraft is composed of several essential components, including an engine, power subsystem, steering system, communications system, and scientific instruments. Typically, it operates in space for several years. Consequently, planetary protection engineers must carefully choose suitable sterilization methods to ensure to reduce microbial cells without causing any damage to the spacecraft components. This study is valuable for this purpose, as it meticulously compares representative sterilization methods and provides sterilization efficiencies in D- or z-values wherever feasible.
Bacillus atrophaeus had the highest resistance to dry heat, 70% IPA, 7.5% H2O2, and VHP compared to the other strains (Table 1). In contrast, B. atrophaeus did not show resistance to UV light irradiation and argon plasma. Deinococcus radiodurans showed higher resistance to UV light and argon plasma than B. atrophaeus but did not show resistance to other treatments, except for oxygen plasma treatment. Aspergillus niger was resistant to UV light, similar to D. radiodurans, and also showed resistance to 7.5% H2O2 and VHP, like B. atrophaeus. Brevundimonas diminuta did not show resistance to any bioburden reduction method used in this study. Brevundimonas diminuta are sterilized when treated under conditions that would inactivate B. atrophaeus, D. radiodurans, and A. niger.
Logarithmic microbial cell death is theoretically observed when cellular damage from the sterilization treatment is homogeneous within the microbial cell population, which is often seen when cells are in a liquid solution. Inoculated coupons used in this study had a mass of air-dried vegetative or spored cells attached to the surface of the material; thus, the cellular damage in the microbial population tended to be heterogeneous since the damage caused by sterilization can differ depending on the location of the cells.
Heat sterilization, either dry or wet, is known to be effective. Air-dried D. radiodurans and B. diminuta cells and B. subtilis spores are reported to be easily killed, presumably by protein denaturation and DNA damage (Setlow, 2006). The tailing portion of the reduction curve of B. atrophaeus treated with dry heat were observed (Figure 2A) It is possible that there is variation in heat sensitivity within the inoculated B. atrophaeus population, resulting in a decrease in more sensitive cells during the earlier stages of the bioburden reduction process. Compared to the dry heat treatment conducted by Kempf et al. (2008) and Schubert and Beaudet (2011) who used an oil bath or stainless-steel vessel cylinders, our dry heat treatment (oven heating) was closer situation to the dry heat chamber of spacecraft component for bioburden reduction. However, in this study, the velocity during the heat-up or cool-down processes was slower than that described by Kempf et al. (2008) and Schubert and Beaudet (2011). The coupon temperature (processing temperature) took approximately 20 min to reach a steady-state temperature condition at any oven temperature during dry heat treatment (Supplementary Figures S1, S2). The accuracy of the processing time was confirmed using the FP-value (Supplementary Table S2). The D-values were calculated when the heating time during the non-steady state (heating and cooling) could be ignored compared to the total processing time of dry heat treatments. The reduction curve for B. atrophaeus ATCC9372 (synonym for NBRC13721) spores obtained in this study agrees with that reported by Kempf et al. (2008) and Schubert and Beaudet (2011), with an estimated z-value of 22.0°C, similar to the z-value of general bacterial spores during dry heating (Supplementary Figure S5).
UV light is known to cause DNA damage, leading to microbial inactivation (Horneck et al., 1984, 2006, 2010; Horneck, 1993). Deinococcus radiodurans and A. niger are reported to have strong radioresistance due to their superior DNA repair mechanism (Cox and Battista, 2005; Cortesão et al., 2020). Our results also showed that the UV light resistance was higher in these species than the other microorganisms (Figure 3). The inoculated A. niger coupons used in our bioburden reduction experiments were approximately 4 μm thick (Supplementary Table S3) and > 10 μm thick locally. The tailing off in the reduction curve of the UV treatment may be caused by the fact that only the cells at the outermost layer of the multi-layered structure were killed by the UV light and the UV light could not reach cells located within the inner position of the layer (Kawaguchi et al., 2020; Cortesão et al., 2021; Schuerger and Headrick, 2023). The B. atrophaeus and A. niger were suspended in the saline solution and D. radiodurans and B. diminuta were suspended in media before dropped on the coupon, so the ingredients of the incubation medium might shield against UV treatment in the test of D. radiodurans and B. diminuta and the UV radiation to sample coupon without media can be more effective.
Treatment with three different chemical agents, IPA, H2O2, and VHP, also exhibited varying sterilization profiles among the four microorganisms. Alcohol is thought to be a nonspecific antimicrobial agent because of the diverse toxicity mechanisms (Ali et al., 2001) and treatment with H2O2, which is mediated by the hydroxyl radical (•OH) generated by the Fenton reaction with intracellular iron, also presumably kill any types of microorganisms (Repine et al., 1981; Clifford and Repine, 1982). However, only slight reduction was observed when B. atrophaeus were treated with 70% IPA for 120 min or 7.5% H2O2 for 10 min and when A. niger was treated with 7.5% H2O2 for 10 min (Figure 4). VHP treatment is more effective to the spores of B. atrophaeus and A. niger but they could not be completely sterilized with VHP as well in this study (Figure 5). All these indicated that chemical treatment may not be quite effective to the fungal and bacterial spores due to the lower envelope permeabilities of spored cells although the mechanism of action of VHP may differ from that of liquid H2O2 (Linley et al., 2012). Meanwhile, D. radiodurans and B. diminuta were quickly sterilized using VHP (Figure 5), as with IPA and liquid H2O2.
The mechanism of oxygen-plasma sterilization is considered an etching effect of the cell surface by oxygen plasma and cell membrane disruption by hydroxyl radicals and atomic oxygen. The heat generated as a side effect may also have bioburden reduction effects. The mechanism of argon plasma bioburden reduction is also an etching of the cell surface and the UV light emitted from de-excitation particles in the plasma (Moisan et al., 2001, 2002). Interestingly, oxygen and argon plasma showed different sterilization spectra from any other sterilization techniques used in this study (Figure 6). Oxygen and argon plasma inactivated A. niger. Argon plasma sterilized B. atrophaeus, whereas D. radiodurans was not sterilized, differing from the oxygen plasma results but similar to its response to UV light. However, although A. niger was UV light-resistant, it was quickly sterilized; therefore, other mechanisms could contribute to D. radiodurans being resistant.
Decontamination processes of spacecraft components can be divided into initial bioburden reduction prior to delivery to assembly facilities and additional bioburden reduction during assembly and testing (Chen et al., 2023). Encapsulated, enclosed, or mated bioburdens are challenging to reduce through additional bioburden reduction (Kminek et al., 2019); however, if they are sufficiently sterilized, re-contamination risk is small. In contrast, the exposed surfaces on the spacecraft components could be easily re-contaminated but re-disinfection is also easy with additional treatment.
For the initial overall bioburden reduction of spacecraft components before assembly, the dry heat method must be suitable if the material is heat resistant. Dry heat has the capability to inactivate various microbial species, as shown in Figure 2, and it reproduces results consistently and can penetrate both surface and deep layers within the components. Using Equation (8), the processing times of dry heat for various processing temperatures obtained in this study can be converted to the dry heat treatment processing times for an optimal reference temperature using FP-values. Reduction curves were estimated for dry heat treatments at 111.7 and 125.0°C as typically described in the COSPAR guidelines (Supplementary Figure S6; European Cooperation for Space Standardization, 2013a; Kminek et al., 2019; NASA, 2022). Tailings in the curves and data variability were observed after four logs order of reduction. Less than four log spores of initial bioburden would be desirable for accurate bioburden reduction using dry heat.
Techniques other than dry heat should be carefully used for non-heat-resistant components in initial and additional bioburden reduction. IPA sterilized D. radiodurans, B. diminuta, and A. niger immediately, whereas H2O2 (solution and vapor) sterilized D. radiodurans and B. diminuta immediately. Although IPA treatment is a convenient technique, it was ineffective concerning B. atrophaeus (Figure 4A), and H2O2 was not effective immediately for B. atrophaeus and A. niger (Figure 4B). Chemical treatments must be more effective when applied with wiping than when used alone for bioburden reduction. UV light is a valuable technique for surface sterilization as B. atrophaeus can be sterilized relatively easily (Figure 3). In contrast, when UV light is used to reduce the bioburden, being aware of microorganisms that show higher resistance than B. atrophaeus, such as D. radiodurans and A. niger, is essential. VHP treatment did not sterilize B. atrophaeus or A. niger, but it achieved two log orders of bioburden reduction in 10 min, even at room temperature (Figure 5). If used with a cleaning process in advance, VHP could be an effective sterilization technique. Plasma treatment produces UV light and heat as secondary factors in addition to the respective sterilization factors (Moisan et al., 2002). Although quantifying each effect is challenging, the bioburden reduction of several microbial species could be achieved (Figure 6), and continuously introducing different types of gases may be helpful for the sterilization. Plasma sterilization can be effective because it can be performed at relatively low temperatures, does not use chemicals, and has no residual properties. In addition to the low-pressure plasma tested in this study, portable atmospheric-pressure plasma may be effective in additional bioburden reduction.
For fitting to the individual systems or situations, the required time for the sterilization can be reduced by optimizing the conditions, such as increasing the voltage for UV light and plasma treatments, increasing the number of lamps and adjusting the distance to the light source for UV treatment, changing the pressure and agent concentration for VHP, changing the agent concentration for H2O2, and the temperature and humidity for all bioburden reduction techniques, or using a combination of each sterilization technique. For example, VHP batch treatment could sterilize Geobacillus stearothermophilus spores to approximately five log orders when treated with heat (Chung et al., 2006), unlike this study that performed treatments at room temperature. Furthermore, less exposure to an aerosolized hypochlorous solution is required to obtain similar sporicidal activity levels at 100%RH than at 70%RH (Ishikawa et al., 2019). Considering that the effectiveness of sterilization treatments varies among different microorganism species, bioburden reduction methods should be evaluated using the most tolerant microorganisms for each technique as model organisms. For instance, A. niger should be employed to assess the UV treatment, and D. radiodurans should be used for argon plasma treatments. Furthermore, it should be noted that solid surface bioburden may result in less uniform microbial reduction. Hence, sterilization methods should be designed under conditions where the initial bioburden is sufficiently low. For this purpose, it could be valuable to reduce contamination through pre-cleaning before the bioburden reduction process.
The anticipated process of the spacecraft components from the introduction into the facility to assembly and launch is shown in Figure 7. Dry heat treatment is appropriate for initial bioburden reduction if the components are heat-resistant. UV light, VHP, and plasma treatment are options for non-heat-resistant components. IPA is the primary method for additional surficial bioburden reduction in assembly cleanrooms. However, H2O2, UV light, or portable plasma treatment could be alternatives if IPA is insufficient to reduce the bioburden. These sterilization techniques, combined with storing components in a high-efficiency particulate air-filtered space and reducing the bioburden in liquids using autoclaves and filter filtration, would provide a system for launching a sterile spacecraft. Bioburden monitoring at each of these stages is essential. Bioburden reduction confirmation through incubation is the standard method; however, this technique is time-consuming, and detection is limited to microorganisms that form colonies on solid media. Therefore, using a rapid, culture-independent detection method is preferred.
This study quantitatively compared the effectiveness of bioburden reduction techniques on typical resistant microorganisms that can attach to spacecraft (Table 1). The results of this study are useful for selecting the appropriate sterilization methods for planetary protection, and this study will also help planetary protection engineers to choose the most tolerant model organism when they assess each bioburden reduction method. However, other more resistant microorganisms may be present in the natural system. Future studies should expand our knowledge of bioburden reduction methods to improve the techniques, including using different detection methods, expanding the range of microorganisms and developing new sterilization methods. These efforts should aim to reduce the risk of contaminating other planets.
Systemically comparing the various sterilization techniques under the same experimental conditions revealed the different sensitivities among microorganisms. Bacillus atrophaeus showed the highest resistance to dry heat, alcohol, 7.5% H2O2, and VHP. Deinococcus radiodurans and A. niger were more resistant to UV than B. atrophaeus, and D. radiodurans showed the highest resistance to argon plasma treatment. Notably, IPA, widely used for bioburden reduction in planetary protection, had no inactivation effect on B. atrophaeus spores. These experimental results provide an accurate estimation of the effectiveness of the sterilization techniques during spacecraft assembly. The method used for bioburden reduction depends on the compatibility of the spacecraft components to withstand the particular technique. Evaluating the damage caused to the spacecraft components by sterilization and selecting and using components that can withstand the entire sterilization process would be effective for future space exploration missions compatible with planetary protection policies and requirements.
The raw data supporting the conclusions of this article will be made available by the authors, without undue reservation.
SK: Conceptualization, Data curation, Funding acquisition, Investigation, Methodology, Validation, Writing – original draft, Writing – review & editing, Formal analysis, Project administration, Resources. SI: Formal analysis, Methodology, Validation, Writing – original draft, Writing – review & editing. NH: Data curation, Methodology, Writing – review & editing. KF: Investigation, Writing – review & editing. YI: Funding acquisition, Investigation, Writing – review & editing, Project administration, Resources. SS: Conceptualization, Funding acquisition, Investigation, Methodology, Supervision, Validation, Writing – original draft, Writing – review & editing, Project administration, Resources.
The author(s) declare financial support was received for the research, authorship, and/or publication of this article. This research was supported by the Space Exploration Innovation Hub Center (TansaX) and Front-loading on Technology for Space and Astronautical Science of the Japan Aerospace Exploration Agency (JAXA). This research was partly funded by the Astrobiology Center (ABC) Program of the National Institutes of Natural Sciences (NINS) nos. AB041015 and AB0514, JST ACT-X no. JPMJAX2015 to SK, and JSPS KAKENHI no. 20H04620 to SS.
The authors greatly appreciate Yoshinobu Matsumura (Department of Life Science and Biotechnology, Kansai University) for the valuable discussions, Chiharu Tanaka (Center for Fungal Consultation, incorporated nonprofit organization, Japan) for providing information on the experimental spore methods, Tetsuya Matsunaga (ISAS, JAXA) for helping with the observation using a 3D laser scanning microscope, and Shoko Mamiya and Chieko Kawanokuchi (TansaX, JAXA) for the technical assistance. We would like to thank Editage (www.editage.com) for English language editing.
SI was employed by Kajima Corporation.
The remaining authors declare that the research was conducted in the absence of any commercial or financial relationships that could be construed as a potential conflict of interest.
All claims expressed in this article are solely those of the authors and do not necessarily represent those of their affiliated organizations, or those of the publisher, the editors and the reviewers. Any product that may be evaluated in this article, or claim that may be made by its manufacturer, is not guaranteed or endorsed by the publisher.
The Supplementary material for this article can be found online at: https://www.frontiersin.org/articles/10.3389/fmicb.2023.1253436/full#supplementary-material
ATCC, American type culture collection; COSPAR, Committee on Space Research; CT value, Concentration-time value; IPA, Isopropyl alcohol; NBRC, NITE Biological Resource Center; NITE, National Institute of Technology and Evaluation; UV light, Ultraviolet light; VHP, Vaporized hydrogen peroxide.
Ali, Y., Dolan, M. J., Fendler, E. J., and Larson, E. L. (2001). “Alcohols” in Disinfection, Sterilization and Preservation. ed. S. S. Block. 5th ed (Philadelphia, USA: Lippincott Williams & Wilkins), 229–253.
Anderson, A. W. (1956). Studies on a radio-resistant micrococcus. I. Isolation, morphology, cultural characteristics, and resistance to gamma radiation. Food Technol. 10, 575–578.
Barengoltz, J., and Stabekis, P. D. (1983). U.S. planetary protection program: implementation highlights. Adv. Space Res. 3, 5–12. doi: 10.1016/0273-1177(83)90166-7
Benardini, J. N. III, La Duc, M. T., Ballou, D., and Koukol, R. (2014b). Implementing planetary protection on the atlas V fairing and ground systems used to launch the Mars science laboratory. Astrobiology 14, 33–41. doi: 10.1089/ast.2013.1011
Benardini, J. N. III, La Duc, M. T., Beaudet, R. A., and Koukol, R. (2014a). Implementing planetary protection measures on the Mars science laboratory. Astrobiology 14, 27–32. doi: 10.1089/ast.2013.0989
Chen, F., Ly, C., Mikellides, I., Bernard, D., and Cooper, M. (2023). Mars 2020 Mission biological return sample contamination control approach and verification. Astrobiology 23, 862–879. doi: 10.1089/ast.2022.0048
Choudhury, B., Revazishvili, T., Lozada, M., Roy, S., Mastro, E. N., Portugal, S., et al. (2023). Distributed compact plasma reactor decontamination for planetary protection in space missions. Sci. Rep. 13:1928. doi: 10.1038/s41598-023-29049-2
Chung, S., Barengoltz, J., Kern, R., Koukol, R., and Cash, H. (2006). The Validation of Vapor Phase Hydrogen Peroxide Microbial Reduction for Planetary Protection and a Proposed Vacuum Process Specification. Pasadena, California: JPL Publication, 06.
Chung, S., Kern, R., Koukol, R., Barengoltz, J., and Cash, H. (2008). Vapor hydrogen peroxide as alternative to dry heat microbial reduction. Adv. Space Res. 42, 1150–1160. doi: 10.1016/j.asr.2008.01.005
Clifford, D. P., and Repine, J. E. (1982). Hydrogen peroxide mediated killing of bacteria. Mol. Cell. Biochem. 49, 143–149. doi: 10.1007/BF00231175
Cortesão, M., De Haas, A., Unterbusch, R., Fujimori, A., Schütze, T., Meyer, V., et al. (2020). Aspergillus Niger spores are highly resistant to space radiation. Front. Microbiol. 11:560. doi: 10.3389/fmicb.2020.00560
Cortesão, M., Siems, K., Koch, S., Beblo-Vranesevic, K., Rabbow, E., Berger, T., et al. (2021). MARSBOx: fungal and bacterial endurance from a balloon-flown analog mission in the stratosphere. Front. Microbiol. 12:601713. doi: 10.3389/fmicb.2021.601713
COSPAR (2021). COSPAR policy on planetary protection. Available at: https://cosparhq.cnes.fr/assets/uploads/2021/07/PPPolicy_2021_3-June.pdf (Accessed July 5, 2023).
Coustenis, A., Hedman, N., Doran, P. T., Al Shehhi, O., Ammannito, E., Fujimoto, M., et al. (2023). Planetary protection: an international concern and responsibility. Front. Astron. Space Sci. 10:156. doi: 10.3389/fspas.2023.1172546
Cox, M. M., and Battista, J. R. (2005). Deinococcus radiodurans: the consummate survivor. Nat. Rev. Microbiol. 3, 882–892. doi: 10.1038/nrmicro1264
Craven, E., Winters, M., Smith, A. L., Lalime, E., Mancinelli, R., Shirey, B., et al. (2021). Biological safety in the context of backward planetary protection and Mars sample return: conclusions from the sterilization working group. Int. J. Astrobiol. 20, 1–28. doi: 10.1017/S1473550420000397
DeVincenzi, D. L., Race, M. S., and Klein, H. P. (1998). Planetary protection, sample return missions and Mars exploration: history, status, and future needs. J. Geophys. Res. Planets 103, 28577–28585. doi: 10.1029/98JE01600
European Cooperation for Space Standardization (2008a). Materials and hardware compatibility tests for sterilization processes. ECSS-Q-ST-70-53C.
European Cooperation for Space Standardization (2008b). Microbial examination of flight hardware and cleanrooms. ECSS-Q-ST-70-55C.
European Cooperation for Space Standardization (2013a). Space product assurance: Dry heat bioburden reduction for flight hardware, ECSS-Q-ST-70-57C.
European Cooperation for Space Standardization (2013b). Space product assurance: Vapour phase bioburden reduction for flight hardware, ECSS-Q-ST-70-56C.
European Cooperation for Space Standardization (2019). Space sustainability: Planetary protection, ECSS-U-ST-20C.
Farley, K. A., Williford, K. H., Stack, K. M., Bhartia, R., Chen, A., de la Torre, M., et al. (2020). Mars 2020 mission overview. Space Sci. 216, 1–41. doi: 10.1007/s11214-020-00762-y
Gould, G. W. (2004). “Heat sterilization” in Russell, Hugo and Ayliffe’s Principles and Practice of Disinfection, Preservation and Sterilization. eds. A. P. Fraise, P. A. Lambert, and J.-Y. Maillaed. 4th ed (Oxford, UK: Blackwell Publishing Ltd), 361–383.
Grotzinger, J. P., Crisp, J., Vasavada, A. R., Anderson, R. C., Baker, C. J., Barry, R., et al. (2012). Mars science laboratory mission and science investigation. Space Sci. 170, 5–56. doi: 10.1007/s11214-012-9892-2
Hayashi, N., Goto, M., Itarashiki, T., Yonesu, A., and Sakudo, A. (2018). Current plasma sterilization and disinfection studies. J. Photopolym. Sci. Technol. 31, 389–398. doi: 10.2494/photopolymer.31.389
Hayashi, N., Guan, W., Tsutsui, S., Tomari, T., and Hanada, Y. (2006). Sterilization of medical equipment using radicals produced by oxygen/water vapor RF plasma. JJAP. 45, 8358–8363. doi: 10.1143/JJAP.45.8358
Hendrickson, R., Lundgren, P., Mohan, G. B. M., Urbaniak, C., Benardini, J., and Venkateswaran, K. (2017). “Comprehensive measurement of microbial burden in nutrient-deprived cleanrooms” in 47th International Conference on Environmental Systems. ICES-2017-177.
Hendrickson, R., Urbaniak, C., Minich, J. J., Aronson, H. S., Martino, C., Stepanauskas, R., et al. (2021). Clean room microbiome complexity impacts planetary protection bioburden. Microbiome 9, 238–217. doi: 10.1186/s40168-021-01159-x
Horneck, G. (1993). Responses of Bacillus subtilis spores to space environment: results from experiments in space. Orig. Life Evol. Biosph. 23, 37–52. doi: 10.1007/BF01581989
Horneck, G., Bücker, H., Reitz, G., Requardt, H., Dose, K., Martens, K. D., et al. (1984). Microorganisms in the space environment. Science 225, 226–228. doi: 10.1126/science.225.4658.226
Horneck, G., Klaus, D. M., and Mancinelli, R. L. (2010). Space microbiology. MMBR 74, 121–156. doi: 10.1128/MMBR.00016-09
Horneck, G., Rettberg, P., Facius, R., and Scherer, K. (2006). “Quantification of biological effectiveness of UV radiation” in Environmental UV Radiation: Impact on Ecosystems and Human Health and Predictive Models: Proceedings of the NATO Advanced Study Institute on Environmental UV Radiation: Impact on Ecosystems and Human Health and Predictive Models. Pisa, Italy June 2001. Springer Netherlands, 51–69.
Ishikawa, S., Ueno, S., Mitsui, M., Matsumura, Y., and Hatsuoka, T. (2019). Construction of its evaluation system in originally designed test chamber system and sporicidal activity of aerosolized hypochlorite solution to Bacillus subtilis spores. Biocontrol Sci. 24, 57–65. doi: 10.4265/bio.24.57
Kawaguchi, Y., Shibuya, M., Kinoshita, I., Yatabe, J., Narumi, I., Shibata, H., et al. (2020). DNA damage and survival time course of deinococcal cell pellets during 3 years of exposure to outer space. Front. Microbiol. 11:2050. doi: 10.3389/fmicb.2020.02050
Kempf, M. J., Chen, F., Kern, R., and Venkateswaran, K. (2005). Recurrent isolation of hydrogen peroxide-resistant spores of Bacillus pumilus from a spacecraft assembly facility. Astrobiology 5, 391–405. doi: 10.1089/ast.2005.5.391
Kempf, M. J., Schubert, W. W., and Beaudet, R. A. (2008). Determination of lethality rate constants and D-values for Bacillus atrophaeus (ATCC 9372) spores exposed to dry heat from 115°C to 170°C. Astrobiology 8, 1169–1182. doi: 10.1089/ast.2007.0208
Kminek, G., Fellous, J. L., Rettberg, P., Moissl-Eichinger, C., Sephton, M. A., Royle, S. H., et al. (2019). The International Planetary Protection Handbook. An Online-Only Supplement to Space Research Today, vol. 205. Available at: https://www.sciencedirect.com/journal/space-research-today.
Koskinen, K., Rettberg, P., Pukall, R., Auerbach, A., Wink, L., Barczyk, S., et al. (2017). Microbial biodiversity assessment of the European Space Agency’s ExoMars 2016 mission. Microbiome 5, 143–116. doi: 10.1186/s40168-017-0358-3
La Duc, M. T., Kern, R., and Venkateswaran, K. (2004). Microbial monitoring of spacecraft and associated environments. Microb. Ecol. 47, 150–158. doi: 10.1007/s00248-003-1012-0
Lalime, E. N., and Berlin, D. (2016). Establishing and monitoring an aseptic workspace for building the MOMA mass spectrometer. Syst. Contaminat. Predict. Control Perform. 2016, 149–159. doi: 10.1117/12.2238226
Linley, E., Denyer, S. P., McDonnell, G., Simons, C., and Maillard, J. Y. (2012). Use of hydrogen peroxide as a biocide: new consideration of its mechanisms of biocidal action. J. Antimicrob. Chemother. 67, 1589–1596. doi: 10.1093/jac/dks129
Makarova, K. S., Aravind, L., Wolf, Y. I., Tatusov, R. L., Minton, K. W., Koonin, E. V. J., et al. (2001). Genome of the extremely radiation-resistant bacterium Deinococcus radiodurans viewed from the perspective of comparative genomics. MMBR 65, 44–79. doi: 10.1128/mmbr.65.1.44-79.2001
Mogul, R., Barding, G. A. Jr., Lalla, S., Lee, S., Madrid, S., Baki, R., et al. (2018). Metabolism and biodegradation of spacecraft cleaning reagents by strains of spacecraft-associated Acinetobacter. Astrobiology 18, 1517–1527. doi: 10.1089/ast.2017.1814
Moisan, M., Barbeau, J., Crevier, M. C., Pelletier, J., Philip, N., and Saoudi, B. (2002). Plasma sterilization. Methods and mechanisms. Pure Appl. Chem. 74, 349–358. doi: 10.1351/pac200274030349
Moisan, M., Barbeau, J., Moreau, S., Pelletier, J., Tabrizian, M., and L'H, Y. (2001). Low-temperature sterilization using gas plasmas: a review of the experiments and an analysis of the inactivation mechanisms. Int. J. Pharm. 226, 1–21. doi: 10.1016/S0378-5173(01)00752-9
Moissl, C., Osman, S., La Duc, M. T., Dekas, A., Brodie, E., DeSantis, T., et al. (2007). Molecular bacterial community analysis of clean rooms where spacecraft are assembled. FEMS Microbiol. Ecol. 61, 509–521. doi: 10.1111/j.1574-6941.2007.00360.x
Moissl-Eichinger, C., Auerbach, A. K., Probst, A. J., Mahnert, A., Tom, L., Piceno, Y., et al. (2015). Quo vadis? Microbial profiling revealed strong effects of cleanroom maintenance and routes of contamination in indoor environments. Sci. Rep. 5:9156. doi: 10.1038/srep09156
Moldenhauer, J. (2013). “Validation of moist and dry heat sterilization” in Sterile Product Development: Formulation, Process, Quality and Regulatory Considerations. eds. P. Kolhe, M. Shah, and N. Rathore (Berlin/Heidelberg, Germany: Springer Science+Business Media), 535–574.
National Research Council (2006). Preventing the Forward Contamination of Mars. Washington, DC: The National Academies Press
Nicholson, W. L., Schuerger, A. C., and Race, M. S. (2009). Migrating microbes and planetary protection. Trends Microbiol. 17, 389–392. doi: 10.1016/j.tim.2009.07.001
Patel, M., Gow, J., Paton, S., and Truscott, P. (2017). SterLim: Sterilisation limits for sample return planetary protection measures. ESA contract no. 4000112742/14/NL/HB.
Pflug, I. J., Holcomb, R. G., and Gómez, M. M. (2001). “Principles of the thermal destruction of microorganisms” in Disinfection, Sterilization and Preservation. ed. S. S. Block. 5th ed (Philadelphia, USA: Lippincott Williams & Wilkins), 79–129.
Planetary Protection Independent Review Board (2019). NASA planetary protection independent review board (PPIRB).
Puleo, J. R., Fields, N. D., Bergstrom, S. L., Oxborrow, G. S., Stabekis, P. D., and Koukol, R. (1977). Microbiological profiles of the Viking spacecraft. Appl. Environ. Microbiol. 33, 379–384. doi: 10.1128/aem.33.2.379-384.1977
Regberg, A. B., Burton, A. S., Castro, C. L., Stahl, S. E., Wallace, S. L., and McCubbin, F. M. (2018). “Microbial ecology of the Johnson Space Center meteorite curation lab and associated infrastructure” in 49th Annual Lunar and Planetary Science Conference (No. 2083, p. 2056).
Repine, J. E., Fox, R. B., and Berger, E. M. (1981). Hydrogen peroxide kills Staphylococcus aureus by reacting with staphylococcal iron to form hydroxyl radical. J. Biol. Chem. 256, 7094–7096. doi: 10.1016/S0021-9258(19)68927-1
Rettberg, P., Antunes, A., Brucato, J., Cabezas, P., Collins, G., Haddaji, A., et al. (2019). Biological contamination prevention for outer solar system moons of astrobiological interest: what do we need to know? Astrobiology 19, 951–974. doi: 10.1089/ast.2018.1996
Rohatgi, N., Schubert, W., Koukol, R., Foster, T. L., and Stabekis, P. D. (2002). Certification of vapor phase hydrogen peroxide sterilization process for spacecraft application. SAE Technical Paper 2002-01-2471.
Roy, S. (2019). An insight into membrane filter validation. ASMI 2, 29–31. doi: 10.31080/ASMI.2019.02.0425
Rummel, J. D., and Pugel, D. B. (2019). Planetary protection technologies for planetary science instruments, spacecraft, and missions: report of the NASA planetary protection technology definition team (PPTDT). Life Sci. Space Res. 23, 60–68. doi: 10.1016/j.lssr.2019.06.003
Ryan, M. P., and Pembroke, J. T. (2018). Brevundimonas spp: emerging global opportunistic pathogens. Virulence 9, 480–493. doi: 10.1080/21505594.2017.1419116
Sandle, T. (2013). “Sterilisation by filtration” in Sterility, Sterilisation and Sterility Assurance for Pharmaceuticals Technology, Validation and Current Regulations (Woodhead Publishing Series in Biomedicine), 143–155.
Schubert, W. W., and Beaudet, R. A. (2011). Determination of lethality rate constants and D-values for heat-resistant Bacillus spores ATCC 29669 exposed to dry heat from 125°C to 200°C. Astrobiology 11, 213–223. doi: 10.1089/ast.2010.0502
Schubert, W. W., Seto, E. P., Hinzer, A. A., and Guan, L. (2023). Identification and archive of Mars 2020 spacecraft microbial isolates. Astrobiology 23, 835–845. doi: 10.1089/ast.2022.0052
Schuerger, A. C., and Headrick, E. L. (2023). Microbial protocols for spacecraft: 3. Spore monolayer preparation methods for ultraviolet irradiation exposures. Astrobiology 23, 908–920. doi: 10.1089/ast.2022.0072
Schuster, E., Dunn-Coleman, N., Frisvad, J., and van Dijck, P. (2002). On the safety of Aspergillus niger: a review. Appl. Microbiol. Biotechnol. 59, 426–435. doi: 10.1007/s00253-002-1032-6
Schwendner, P., Jobson, M. E., and Schuerger, A. C. (2020). Addition of anaerobic electron acceptors to solid media did not enhance growth of 125 spacecraft bacteria under simulated low-pressure Martian conditions. Sci. Rep. 10, 18290–18211. doi: 10.1038/s41598-020-75222-2
Setlow, P. (2006). Spores of Bacillus subtilis: their resistance to and killing by radiation, heat and chemicals. J. Appl. Microbiol. 101, 514–525. doi: 10.1111/j.1365-2672.2005.02736.x
Shirey, T. B., Schubert, W., and Benardini, J. (2017). “An overview of surface heat microbial reduction as a viable microbial reduction modality for spacecraft surfaces” in 47th International Conference on Environmental Systems, ICES-2017-201.
Stoforos, N. G. (2015). “Thermal processing” in Handbook of Food Processing: Food Preservation. eds. T. Varzakas and C. Tzia (Florida, USA: CRC Press), 27–56.
Venkateswaran, K., Satomi, M., Chung, S., Kern, R., Koukol, R., Basic, C., et al. (2001). Molecular microbial diversity of a spacecraft assembly facility. Syst. Appl. Microbiol. 24, 311–320. doi: 10.1078/0723-2020-00018
Keywords: sterilization, bioburden reduction, planetary protection, forward contamination, spacecraft, microbial contamination
Citation: Kimura S, Ishikawa S, Hayashi N, Fujita K, Inatomi Y and Suzuki S (2023) Bacterial and fungal bioburden reduction on material surfaces using various sterilization techniques suitable for spacecraft decontamination. Front. Microbiol. 14:1253436. doi: 10.3389/fmicb.2023.1253436
Received: 05 July 2023; Accepted: 28 November 2023;
Published: 13 December 2023.
Edited by:
Rob Van Houdt, Belgian Nuclear Research Center (SCK CEN), BelgiumReviewed by:
Teresa Rinaldi, Sapienza University of Rome, ItalyCopyright © 2023 Kimura, Ishikawa, Hayashi, Fujita, Inatomi and Suzuki. This is an open-access article distributed under the terms of the Creative Commons Attribution License (CC BY). The use, distribution or reproduction in other forums is permitted, provided the original author(s) and the copyright owner(s) are credited and that the original publication in this journal is cited, in accordance with accepted academic practice. No use, distribution or reproduction is permitted which does not comply with these terms.
*Correspondence: Shunta Kimura, a2ltdXJhLnNodW50YUBqYXhhLmpw; Shino Suzuki, c3V6dWtpLnNoaW5vMkBqYXhhLmpw
Disclaimer: All claims expressed in this article are solely those of the authors and do not necessarily represent those of their affiliated organizations, or those of the publisher, the editors and the reviewers. Any product that may be evaluated in this article or claim that may be made by its manufacturer is not guaranteed or endorsed by the publisher.
Research integrity at Frontiers
Learn more about the work of our research integrity team to safeguard the quality of each article we publish.