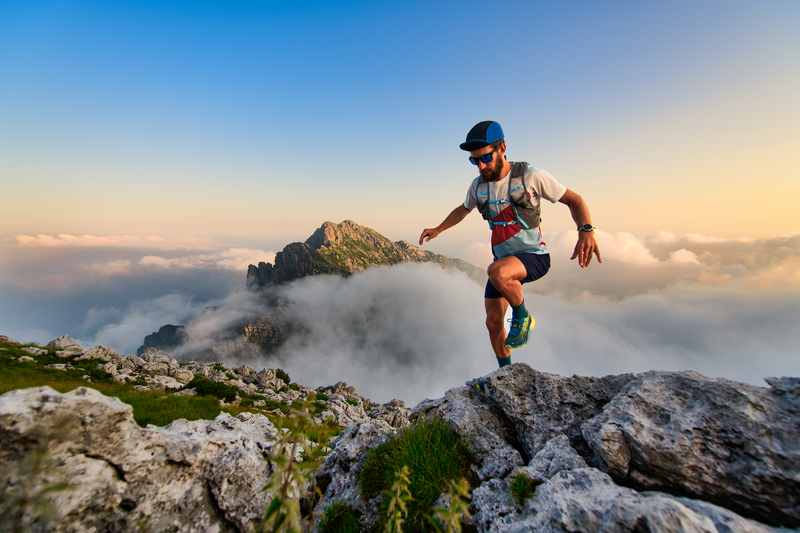
95% of researchers rate our articles as excellent or good
Learn more about the work of our research integrity team to safeguard the quality of each article we publish.
Find out more
ORIGINAL RESEARCH article
Front. Microbiol. , 25 September 2023
Sec. Microbiotechnology
Volume 14 - 2023 | https://doi.org/10.3389/fmicb.2023.1250308
This article is part of the Research Topic Microbial Hydrocarbon Degradation and Bioremediation: From Genes to Pathways View all 5 articles
Multi element compound-specific stable isotope analysis (ME-CSIA) is a tool to assess (bio)chemical reactions of molecules in the environment based on their isotopic fingerprints. To that effect, ME-CSIA concepts are initially developed with laboratory model experiments to determine the isotope fractionation factors specific for distinct (bio)chemical reactions. Here, we determined for the first time the carbon and hydrogen isotope fractionation factors for the monooxygenation of the short-chain alkanes ethane, propane, and butane. As model organism we used Thauera butanivorans strain Bu-B1211 which employs a non-haem iron monooxygenase (butane monooxygenase) to activate alkanes. Monooxygenation of alkanes was associated with strong carbon and hydrogen isotope effects: εbulkC = −2.95 ± 0.5 ‰ for ethane, −2.68 ± 0.1 ‰ for propane, −1.19 ± 0.18 ‰ for butane; εbulkH = −56.3 ± 15 ‰ for ethane, −40.5 ± 2.3 ‰ for propane, −14.6 ± 3.6 ‰ for butane. This resulted in lambda (Λ ≈ εHbulk/εCbulk) values of 16.2 ± 3.7 for ethane, 13.2 ± 0.7 for propane, and 11.4 ± 2.8 for butane. The results show that ME-CSIA can be used to track the occurrence and impact of monooxygenase-dependent aerobic processes converting short-chain alkanes in natural settings like marine and terrestrial seeps, gas reservoirs, and other geological formations impacted by natural gas.
Alkanes are the major fraction of non-degraded crude oils, accounting for over 50% by mass (Tissot and Welte 1984). Short-chain, gaseous alkanes are mainly released in the biosphere from deep-seated oil or gas reservoirs by natural seepage or during the anthropogenic exploration and exploitation of fossil fuels; global annual emissions have been estimated at 42–64 Tg/year for methane, about 10 Tg/year for ethane, propane and n-butane, respectively, and about 4 Tg/year for isobutane (Etiope and Ciccioli 2009; Pozzer et al., 2010; Musat et al., 2016).
Owing to their long-time presence in the biosphere, short-chain alkanes are used as growth substrates by a wide diversity of aerobic and anaerobic microorganisms which have evolved different alkane oxidation pathways. Under anoxic conditions, biodegradation of gaseous non-methane alkanes has been observed with sulfate and nitrate as terminal electron acceptors. Two major functionalization mechanisms and subsequent degradation pathways can be distinguished. Nitrate- and sulfate-reducing bacteria use glycyl radical enzymes which catalyze the hemolytic cleavage of a C-H-bond of the alkane, followed by carbon–carbon addition of the generated alkyl radical to fumarate (Savage et al., 2010; Musat 2015; Musat et al., 2016; Wu et al., 2022); the reaction can take place simultaneously at the terminal and subterminal carbon atoms (propane) or only at the subterminal carbon atom (butane) (Kniemeyer et al., 2007; Jaekel et al., 2014). The formed alkylsuccinates are subsequently channeled into the central metabolic pathways by ligation to coenzyme A, C-skeleton rearrangement, and beta-oxidation (Musat 2015; Chen et al., 2022). Distinct mechanisms of activation and downstream oxidation pathways have been recently documented for short-chain alkane oxidizing archaea forming syntrophic associations with partner sulfate-reducing bacteria. This pathway proceeds analogously to the anaerobic oxidation of methane and is initiated by enzymes bearing similarities to methyl-coenzyme M reductases (MCR), named alkyl-coenzyme M reductases, or ACR. This leads to formation of alkyl-coenzyme M as alkane activation products, which are further converted to acyl-CoA by yet unknown reactions, followed by beta oxidation and channeling of the generated acetyl-CoA into the C1 pathway, enetually leading to complete oxidation to carbon dioxide (Laso-Perez et al., 2016; Chen et al., 2019; Hahn et al., 2020; Zehnle et al., 2023).
In oxic settings, short-chain alkanes are oxidized by a high diversity of aerobic microorganisms (Shennan 2006) which could exert a tremendous impact on geochemical cycles in environments impacted by massive emissions of hydrocarbons (Valentine et al., 2010). Despite their phylogenetic diversity, aerobic microorganisms use common mechanistic principles to activate the alkane molecules. This is done by hydroxylation of the terminal (primary) carbon atom of the alkane, yielding primary alcohols; in addition, propane and butane are activated to a lesser extent at their subterminal (secondary) carbon atoms, yielding secondary alcohols. Both reactions are catalyzed by molecular oxygen-dependent alkane hydroxylases, also named monooxygenases. Various structurally different types of alkane hydroxylases are currently known, including methane monooxygenases, alkane hydroxylases related to an integral-membrane non-haem diiron monooxygenase (AlkB), or alkane hydroxylases related to cytochrome P450 (Rojo 2009). A well-studied strain assimilating gaseous alkanes is Thauera butanivorans (formerly Pseudomonas butanovora, Dubbels et al., 2009). It activates gaseous alkanes primarily by monooxygenation of the primary carbon atom using a butane monooxygenase, a non-haem iron monooxygenase related to soluble methane monooxygenases (Rojo 2009).
In the last two decades, compound-specific stable isotope analysis (CSIA) has been established as state-of-the-art technique for source appointment and for monitoring of (bio)degradation reactions at contaminated sites (Elsner 2010; Fischer et al., 2016). The principle of CSIA is that isotopologues react in slightly different rates upon rate-limiting steps of (bio)chemical reactions; in most cases the lighter isotopologues react faster, leading to residual substrate pools enriched in heavier isotopes, and to reaction products pools enriched in lighter isotopes. This is termed normal kinetic isotope fractionation. The kinetic isotope fractionation effect is dependent on the rate limitation of bond change in the irreversible bond cleavage reaction (commitment to catalysis) and is related to the mode of bond change. The extent of observed isotope fractionation can be affected by rate limitation prior to the bond change reaction which is typically lowering the observed isotope fractionation – this is termed masking of the kinetic isotope effect (KIE). The magnitude of isotope fractionation, defined by the enrichment factor ε, depends on various parameters, like the type of (bio)chemical reaction (see above), the mass of the respective isotopes, or bioavailability restrictions and other factors such as uptake of reactant into the cell, transport of reactant to the enzyme, or binding to the enzyme (Vogt et al., 2016). The latter leads to masking of isotope fractionation which can be circumvented by analyzing stable isotopes of two or more elements within the molecule (Multi element-CSIA, ME-CSIA) which may cancel out the rate limitation prior the commitment of catalysis. The fractionation of stable isotopes of two (or more) elements usually correlates over a wide concentration range, so that the resulting correlation factor (termed lambda, Λ) can be used to specify a distinct (bio)chemical reaction. Hence, in case of hydrocarbons, analysis of isotope fractionation of both stable carbon and hydrogen isotopes allows characterizing distinct (bio)chemical hydrocarbon activation mechanisms. Whereas Λ values and isotope fractionation factors of various aerobic and anaerobic activation mechanisms for aromatic hydrocarbons have been determined (for an overview see Vogt et al., 2016), less data is available for similar bond cleavage reactions initiating a degradation pathway of alkanes. Jaekel et al. (2014) characterized the reaction of propane and butane activation by addition to fumarate by ME-CSIA using sulfate-reducing enriched and pure cultures. Due to the gaseous nature of the short-chain alkanes, carbon and hydrogen stable isotope fractionation can be significantly impacted by limited alkane diffusion toward the cells in systems with gas and liquid phases. It has been shown that mass transfer limitations caused by insufficient mixing, low substrate bioavailability, or high cell densities as those occurring in aggregates and biofilms, lead to substantial decrease of observable isotope fractionation (Templeton et al., 2006; Staal et al., 2007; Kampara et al., 2008, 2009; Jaekel et al., 2014), emphasizing the importance of ME-CSIA to characterize the reaction patterns. In addition, the anaerobic oxidation of propane via the addition to fumarate mechanism was characterized by intramolecular, position-specific carbon isotope fractionation, which allowed to detect and quantify anaerobic oxidation processes in natural gas reservoirs (Gilbert et al., 2019). Although C and H isotope fractionation associated with the aerobic oxidation of short-chain alkanes have been reported for sediment incubations (Kinnaman et al., 2007; Bouchard et al., 2008), a characterization of the stable isotope fractionation of aerobic monooxygenation using defined cultures is currently missing. In this study, we determined for the first-time bulk and reactive position-specific enrichment factors for carbon (εC) and hydrogen (εH) stable isotopes and bulk Λ values for the monooxygenation of ethane, propane and butane, using as model organism Thauera butanivorans Bu-B1211, a strain which contains a single alkane monooxygenase dubbed butane monooxygenase. We further compare the isotope fractionation factors of monooxygenation with fractionation factors obtained for anaerobic degradation pathways. The data will lead to a better understanding of isotope effects linked to the biodegradation of gaseous alkanes in the environment.
Ethane, propane, and butane (purity ≥ 99.95 mol %) were purchased from Air Liquide (Düsseldorf, Germany).
Thauera butanivorans strain Bu-B1211 (DSM 2080) was obtained from the Leibnitz Institute DSMZ German Collection of Microorganisms and Cell Cultures, Braunschweig, Germany. Strain Bu-B1211 was cultivated in aerobic, bicarbonate-buffered mineral salt medium provided with gaseous alkanes as sole source of carbon and energy. The medium contained 0.5 g KH2PO4, 0.3 g NH4C1, 0.4 g MgSO4·7H2O, 0.1 g CaCl2·2H2O, and 1.0 g NaCl in 1 L of distilled water. After autoclaving and cooling under air in bottles sealed with butyl rubber stoppers, the following substances were added (according to Widdel and Bak 1992): CO2 (10%, v/v), 30 mL NaHCO3 solution (84 g/L, autoclaved under CO2), vitamins, EDTA-chelated mixture of trace elements, and selenite and tungstate solution. The pH of the medium was adjusted to 7.0–7.4.
Thauera butanivorans was cultured in 120 mL serum bottles containing 30 mL medium under a headspace of air (21% v/v O2). Ethane, propane, and butane were added to the headspace at 1 bar partial pressure (final pressure: 2 bar). The bottles were closed with butyl rubber stoppers. Subcultures were prepared by inoculating fresh culture medium with 3% v/v of an active culture. The cultures were incubated on a horizontal shaker (100 rotations per minute, rpm) at 28°C in a horizontal position in order to increase the surface-to-volume ratio and thus the rate of mass transfer of alkanes into the liquid medium, due to their low solubility in water.
Isotope fractionation experiments with ethane, propane and butane were done in 120 mL serum bottles containing 30 mL medium and defined amounts of pure ethane (5 mL), propane (3.6 mL) or butane (2.8 mL). In order to stimulate the biodegradation of ethane, the medium was supplemented with FeSO4·7H2O and yeast extract, in final concentrations of 0.1 g/L, respectively. The bottles were subsequently inoculated with 1 mL of culture previously grown on the same gaseous alkane. For each alkane, 15 parallel bottles were prepared, of which 3 bottles served as sterile controls without added cells. The microcosms were incubated at 28°C on a rotary shaker (100 rpm) and monitored with respect to alkane degradation and growth, the latter measured as changes in optical density (OD 600 nm). After approximately 0, 10, 20, 50, 60, 80, 90, and 95% of alkane had been consumed, individual cultures were inactivated by the addition of 4 M NaOH (1 mL), adjusting the pH to 12, and subsequent heating in a water bath at 80°C for 15 min. Inactivated cultures were stored at room temperature until analysis. The sterile, control bottles were treated by the same procedure at the times when the biodegradation had reached 0, 50 and 95% in inoculated cultures, respectively.
Alkane concentrations were quantified using a gas chromatograph (Chrompack CP-3800, Varian) equipped with a flame ionization detector. The alkanes were resolved using a GS-Q PLOT column (30 m × 0.53 mm, 30 μm film thickness; Agilent Technologies), with N2 as carrier gas at a flow rate of 3 mL min−1. The oven was maintained at 140°C, with the injection and detection temperatures maintained at 220°C and 350°C, respectively. Ethane, propane, and butane concentrations were calculated based on external calibration curves using the pure gases as standards and reported as the mean values of duplicate measurements (technical replicates). Headspace samples (500 μL) were taken from the culture bottles using gastight glass syringes and injected with a split ratio of 1:50. For each culture and time point duplicate headspace samples were analyzed to account for possible instrumental deviations.
Carbon isotope analyses were determined by gas chromatography isotope-ratio mass spectrometry (GC-IRMS) using a GC (6,890 N Gas chromatograph, Agilent Technology) equipped with a PoraBOND Q column (50 m × 0.32 mm × 5 μm, Agilent Technology). The GC was linked via a GCCIII combustion unit (Thermo Fisher Scientific) and a ConFlo IV interface (Thermo Fisher Scientific) to an isotope ratio mass spectrometer (MAT 253, Thermo Fisher Scientific). For hydrogen isotope analyses a GC (7890A Gas chromatograph, Agilent technology) equipped with the same column as described for carbon isotope analyses was used. The GC was linked via a GC-Isolink (Thermo Fisher Scientific) and a ConFlo IV interface (ThermoFisher Scientific) to an isotope ratio mass spectrometer (MAT 253, Thermo Fisher Scientific). For both analyses He was used as a carrier gas, and the GC oven was maintained at 100°C for ethane, 140°C for propane and 180°C for butane, respectively. Samples were taken from the head space in volumes ranging from 50 to 1,000 μL and injected in split mode with a split ratio ranging from 1:20 to 1:3 into the split/splitless injector, maintained at 250°C. Each sample was measured in triplicate. The total analytical uncertainty with respect to both accuracy and reproducibility, estimated from the triplicate measurements, was always better than ±0.5‰ for δ13C and ± 10‰ for δ2H, respectively. The obtained isotope ratios were expressed in the δ-notation (δ13C and δ2H) relative to international isotope standards of the international atomic energy agency (Coplen 2011): Vienna Pee Dee Belemnite (VPDB) for stable carbon isotopes and Vienna standard Mean Ocean Water (VSMOW) for hydrogen isotopes. Pure ethane, propane, and butane gases were used as standards for the isotopic ratios before biodegradation.
Stable isotope fractionation of alkane hydroxylation was described by calculating bulk isotope enrichment factors (εbulk) using the logarithmic form of the Rayleigh equation (Equation 1).
The isotope enrichment factors of the reactive position (εrp), apparent kinetic isotope effects (AKIEs) and Λ-values were calculated as reported elsewhere (Jaekel et al., 2014).
In brief, bulk enrichment factors, εbulk (corresponding to ε in Equation 1) were converted to reactive position enrichment factors (εrp) using Equation 2.
Due to the hydroxylation at the C1 position of alkanes by butane monooxygenase, we used the following numbers for n (number of atoms in the molecule) and x (number of atoms in reactive positions, indicated in the following in boldface): ethane, n = 2 and x = 2 for carbon (H3C-CH3) and n = 6 and x = 6 for hydrogen (H3C-CH3); propane, n = 3 and x = 2 for carbon (H3C-CH2-CH3) and n = 8 and x = 6 for hydrogen (H3C-CH2-CH3); butane, n = 4 and x = 2 for carbon (H3C-CH2-CH2-CH3) and n = 10 and x = 6 for hydrogen (H3C-CH2-CH2-CH3). The errors of the obtained bulk and reactive position specific enrichment factors were given as a 95% confidence interval (CI) and were calculated by regression analysis as described elsewhere (Elsner et al., 2007).
AKIEs for carbon and hydrogen were calculated according to Equation 3.
Where z is the number of atoms in identical positions. We set z = 2 for C and 6 for H for ethane, propane, and butane.
Lambda (Λ) values were calculated according to Equation 4.
Under the cultivation conditions employed, T. butanivorans consumed over 80% of the added ethane, propane, and butane (initial concentrations of ~2.3, 1.6 and 1.3 mM, respectively) within around 40 h of incubation (Supplementary Figure S1). Alkane consumption was coupled to cell growth (Supplementary Figure S1; Takahashi et al., 1980). Within 60 h of incubation, over 95% of the initial amounts of propane and butane were consumed. Overall, the oxidation rates decreased when over 80% of the initially added alkane was consumed (Supplementary Figure S1), most likely a consequence of mass transfer limitations under low alkane concentrations in the gas phase. Notably, within the same incubation time, only 85% of the initially added ethane was consumed, suggesting that the monooxygenase initiating alkane oxidation has lower affinity for ethane than for the other alkanes.
Natural abundance stable isotope fractionation during consumption of a certain substrate is typically governed by the enzyme initiating the oxidation pathway (Elsner 2010; Vogt et al., 2016). Degradation of ethane, propane, and butane in T. butanivorans is initiated by the same enzyme, butane monooxygenase (sBMO), a soluble three-component diiron monooxygenase system with a broad substrate spectrum (Sluis et al., 2002; Dubbels et al., 2007). Experiments with purified sBMO showed that ethane is converted to ethanol, while, propane and butane are converted mainly to their corresponding primary alcohols (1-propanol and 1-butanol, respectively), which account for over 80% of the enzyme product (Dubbels et al., 2007); the rest of the enzyme products (about 20%) are the corresponding secondary alcohols, 2-propanol and 2-butanol. The pathway of 1-butanol assimilation was elucidated by Arp (1999): 1-butanol is further oxidized via butyraldehyde to butyrate, which is metabolized to acetyl-CoA by beta-oxidation. Mechanistically, like other soluble diiron monooxygenases, the catalytic cycle of sBMO is initiated by activation of molecular oxygen to a bound peroxide state, which homolytically cleaves the covalent C-H bond of the alkane. This generates an enzyme-bound alkyl radical and an iron-coordinated hydroxyl radical which recombine (rebound mechanism) to generate the hydroxylated alkane (an alcohol) (Widdel and Musat 2019). The oxidation of ethane, propane, and butane by T. butanivorans was associated with normal carbon and hydrogen isotope fractionation, hence 13C and 2H became enriched in the residual substrates during biodegradation (Figures 1A–F). This indicates that the cleavage of the C-H-bond during monooxygenation by sBMO is a rate-determining step of the enzymatic reaction. The correlation between substrate concentration and isotope ratios (R2) was always higher than 0.89 (Figures 1A–F), showing that the process could be described by the Rayleigh equation. Removal of alkanes was solely biotic, since no losses of ethane, propane or butane or changes in carbon and hydrogen isotope ratios were observed in abiotic controls (data not shown). Bulk and reactive-position-specific values for εC and εH as well as values for AKIEC and AKIEH are listed in Table 1. For both carbon and hydrogen isotopes, εbulk values decreased with increasing chain length: εbulkC values of ethane, propane and butane were − 2.95 ‰, −2.68 ‰, and − 1.19 ‰, respectively, whereas εbulkH values were − 56.3 ‰, −40.5 ‰, and − 14.6 ‰, respectively. Such trend is thought to be caused by dilution of isotope fractionation by additional non-reacting carbon and hydrogen atoms in longer alkanes. The same trend – decreasing enrichment factors at increasing chain length - was also observed for εrpH values, which could be explained as a contribution of the additional enzymatic activation of propane and butane at the secondary C atoms (and thus the bounded hydrogen atoms) as described by Dubbels et al. (2007) for the butane monooxygenase of T. butanivorans, leading to a dilution of the isotope effect at the reactive position. In contrast, εrpC values were in a similar range (Table 1). Since hydrogen isotope fractionation was around 10 times higher than carbon isotope fractionation, as expected for kinetic isotope effects associated with the cleavage of a C-H-bond, the dilution effect was probably below the detection limit for reactive carbon positions. AKIE values for C and H were 1.005–1.008 and 1.17–1.51, respectively. Lambda (Λ) values were rather similar for these three alkanes, with ethane showing the highest value (16.2 ± 3.7) and butane the lowest (11.4 ± 2.8) (Figure 2; Table 1). In summary, the isotope fractionation data are consistent with the hypothesis that the reaction mechanism of the butane monooxygenase of T. butanivorans is similar for all substrates.
Figure 1. Double logarithmic plots of the isotopic composition versus the residual concentration of ethane (A,B), propane (C,D), and butane (E,F) during monooxygenation by T. butanivorans; the lines correspond to a linear regression model, resulting in carbon (A,C,E) and hydrogen (B,D,F) enrichment factors (see Table 1, εbulkC and εbulkH values).
Table 1. Enrichment factors (εbulkC, εbulkH), lambda (Λ) values and apparent kinetic isotope effects (AKIEC, AKIEH) for n-alkane activation by aerobic and anaerobic microbial strains or environmental samples.
Figure 2. Plots of Δδ2H vs. Δδ13C for ethane (A), propane (B), and butane (C) as substrates. The slopes of the regression curve give the ΛH/C values.
Generally, previous isotope fractionation data for aerobic ethane, propane and butane degradation have been determined in complex microbial communities (Table 1) where the alkanes might have been simultaneously degraded by different organisms using different enzymes and pathways, hence leading to a mixed isotope fractionation factor representing the average sum of different isotope fractionation factors. In contrast, the isotope fractionation values presented here are the first ones generated for aerobic oxidation of alkanes by a pure culture containing a single enzyme for alkane oxidation and may serve as a standard for future investigations using other model strains harboring different alkane-activating enzymes.
The εbulkH value for ethane monooxygenation (−56.3 ‰) is similar to those reported for aerobic ethane biodegradation by undefined microbial communities of marine sediments, whereas the εbulkC ethane observed here for T. butanivorans (−2.95 ‰) is significantly smaller than that observed for the same marine microbial community (Table 1; Kinnaman et al., 2007). This resulted in significantly different Λ values of aerobic ethane biodegradation (16 of T. butanivorans vs. 8 of the marine community). As pointed out above, the fractionation factors determined for the marine community may be caused by the simultaneous operation of different ethane degradation pathways and cannot be directly compared. The Λ window for aerobic methane degradation, a reaction catalyzed by methane monooxygenase and characterized by strong carbon and hydrogen isotope fractionation, is considerably smaller and lies between 7 and 11, determined using several pure cultures representing distinct methane monooxygenases (Feisthauer et al., 2011). No enrichment factors have been determined yet for ethane biodegradation under anoxic conditions, thus it remains to be shown whether aerobic and anaerobic ethane biodegradation can be differentiated by ME-CSIA, and if anaerobic or microaerophilic oxidation may have contributed to the C and H fractionation during ethane oxidation in marine sediments.
Similar to aerobic ethane degradation, the isotope fractionation pattern of propane monooxygenation by T. butanivorans was different from values described before for an aerobic propane-degrading microbial community from marine sediments (Table 1; Kinnaman et al., 2007). Particularly, εbulkH values of the marine community were considerably lower than those observed for T. butanivorans, resulting in different Λ values (Λ = 13 for T. butanivorans vs. Λ = 3 for the marine community, Table 1). As discussed above, the previously determined fractionation factors for this complex marine community cannot be directly linked to a single enzymatic reaction as for T. butanivorans, preventing a direct comparison of these factors. Notably, the obtained values for T. butanivorans are in the same range determined for anaerobic activation of propane by a microbial community from marine sediment, marine enrichment cultures and a marine pure strain (Table 1; Mastalerz et al., 2009; Jaekel et al., 2014), the latter two activating propane by fumarate addition. This picture is general complicated by the fact that isotope fractionation has been shown to be significantly affected by mass-transfer limitations in biodegradation experiments, since continuously mixed cultures showed higher isotope fractionation than non-mixed, mass-transfer limited cultures (Jaekel et al., 2014; Table 1).
Nevertheless, the current data indicates that propane biodegradation is generally trackable by ME-CSIA at various environmental conditions due to strong carbon and hydrogen isotope fractionation, but degradation under oxic and anoxic conditions cannot be easily differentiated. A similar picture was observed for analysis of methane biodegradation by ME-CSIA (Feisthauer et al., 2011).
The εCbulk values for aerobic butane biodegradation by soil and marine microbial communities are higher than the value determined here for T. butanivorans (Table 1); however, to date no εHbulk have been determined for aerobic butane degradation, hence no Λ values can be compared. Bulk carbon and hydrogen fractionations of butane monooxygenation by T. butanivorans are in the same range or significantly lower as values determined for anaerobic butane activation by fumarate addition by marine enrichment cultures and pure strains (Table 1; Jaekel et al., 2014). However, the Λ value (11.4 ± 2.8) for T. butanivorans is at the upper range observed for anaerobic butane degradation (Λ values =4.9 ± 1.2 to 8.7 ± 0.4) (Table 1). This range overlap challenges the distinctions of anaerobic and aerobic butane degradation based on isotope values.
Our data show that monooxygenation of the short-chain, volatile alkanes ethane, propane and butane is associated with strong carbon and hydrogen stable isotope fractionation, demonstrating the general applicability of ME-CSIA for assessing biodegradation of such alkanes in the environment. Identifying this reaction solely by its compound-specific Λ values is however difficult due to the similarity of Λ values observed for monooxygenation (oxic conditions) and addition to fumarate (anoxic conditions). Similar observations were made for methane oxidation under oxic and anoxic conditions (Feisthauer et al., 2011). The current landscape of C and H fractionation associated with volatile alkane oxidation can be completed by acquiring additional ME-CSIA data of enzyme-specific alkane hydroxylation reactions. While the sBMO of T. butanivorans is a non-haem diiron enzyme related to soluble methane monooxygenases, other enzymes initiating the oxidation of volatile alkanes belong to the AlkB family of alkane hydroxylases, an enzyme family with a high sequence diversity (Rojo 2009). Diverse enzymes may cause slightly different reaction mechanisms and may result in different εbulkC, εbulkH and Λ values as observed here (Table 1). Such effects have been formerly described for other highly diverse classes of enzymes. For example, benzylsuccinate synthases catalyzing the anaerobic activation of toluene show a high sequence diversity, and display a broad range of εbulkC, εbulkH and Λ values essentially for the same enzymatic reaction (Vogt et al., 2008; Kümmel et al., 2013). The recent discovery of archaea oxidizing volatile alkanes like ethane and butane via alkyl-coenzyme M reductases, or ACR (Laso-Perez et al., 2016; Chen et al., 2019; Seitz et al., 2019; Hahn et al., 2020, 2021) adds to the complexity of distinguishing between aerobic and anaerobic processes in environmental samples. Stable isotope fractionation patterns for ACR-dependent pathways have not been reported so far. Judging from ME-CSIA values determined for the analogous pathway of anaerobic oxidation of methane by methyl-coenzyme M reductases (Holler et al., 2009), one can anticipate that ACR-dependent reactions are also associated with strong carbon and hydrogen effects.
The raw data supporting the conclusions of this article will be made available by the authors, without undue reservation.
FM, CV, and H-HR designed the experiments. ZS performed the experiments. ZS, FM, and CV analyzed the data. CV drafted the manuscript. FM and H-HR revised the manuscript. All authors contributed to the article and approved the submitted version.
This study was funded by the Helmholtz Centre for Environmental Research – UFZ. Additional funding was provided by the China Scholarship Council (grant to ZS), and by the Novo Nordisk Foundation through an NNF Young Investigator Award, Grant NNF22OC0071609 ReFuel (grant to FM).
We are grateful for the analytical support of Steffen Kümmel and Matthias Gehre in the Stable Isotope Lab of the Department of Isotope Biogeochemistry at the UFZ.
The authors declare that the research was conducted in the absence of any commercial or financial relationships that could be construed as a potential conflict of interest.
All claims expressed in this article are solely those of the authors and do not necessarily represent those of their affiliated organizations, or those of the publisher, the editors and the reviewers. Any product that may be evaluated in this article, or claim that may be made by its manufacturer, is not guaranteed or endorsed by the publisher.
The Supplementary material for this article can be found online at: https://www.frontiersin.org/articles/10.3389/fmicb.2023.1250308/full#supplementary-material
Arp, D. J. (1999). Butane metabolism by butane-grown ‘Pseudomonas butanovora’. Microbiology 145, 1173–1180. doi: 10.1099/13500872-145-5-1173
Bouchard, D., Hunkeler, D., and Höhener, P. (2008). Carbon isotope fractionation during aerobic biodegradation of n-alkanes and aromatic compounds in unsaturated sand. Org. Geochem. 39, 23–33. doi: 10.1016/j.orggeochem.2007.10.002
Chen, S. C., Musat, N., Lechtenfeld, O. J., Paschke, H., Schmidt, M., Said, N., et al. (2019). Anaerobic oxidation of ethane by archaea from a marine hydrocarbon seep. Nature 568:108. doi: 10.1038/s41586-019-1063-0
Chen, S.C., Ji, J., Popp, D., Jaekel, U., Richnow, H.H., Sievert, S.M., et al. (2022) Genome and proteome analyses show the gaseous alkane degrader Desulfosarcina sp. strain BuS5 as an extreme metabolic specialist. Environ. Microbiol. 24: 1964–11976. doi: 10.1111/1462-2920.15956
Coplen, T. B. (2011). Guidelines and recommended terms for expression of stableisotope-ratio and gas-ratio measurement results. Rapid Commun. Mass Spectrom. 25, 2538–2560. doi: 10.1002/rcm.5129
Dubbels, B. L., Sayavedra-Soto, L. A., and Arp, D. A. (2007). Butane monooxygenase of ‘Pseudomonas butanovora’: purification and biochemical characterization of a terminal-alkane hydroxylating diiron monooxygenase. Microbiology 153, 1808–1816. doi: 10.1099/mic.0.2006/004960-0
Dubbels, B. L., Sayavedra-Soto, L. A., Bottomley, P. J., and Arp, D. J. (2009). Thauera butanivorans sp. nov., a C2–C9 alkane-oxidizing bacterium previously referred to as ‘Pseudomonas butanovora’. Int. J. Syst. Evol. Microbiol. 59, 1576–1578. doi: 10.1099/ijs.0.000638-0
Elsner, M., McKelvie, J., Lacrampe Couloume, G., and Sherwood Lollar, B. (2007). Insight into methyl tert-butyl ether (MTBE) stable isotope fractionation from abiotic reference experiments. Environ. Sci. Technol. 41, 5693–5700. doi: 10.1021/es070531o
Elsner, M. (2010). Stable isotope fractionation to investigate natural transformation mechanisms of organic contaminants: principles, prospects and limitations. J. Environ. Monit. 12, 2005–2031. doi: 10.1039/c0em00277a
Etiope, G., and Ciccioli, P. (2009). Earth’s degassing: a missing ethane and propane source. Science 323:478. doi: 10.1126/science.1165904
Feisthauer, S., Vogt, C., Modrzynski, J., Szlenkier, M., Krüger, M., Siegert, M., et al. (2011). Different types of methane monooxygenases produce similar carbon and hydrogen isotope fractionation patterns during methane oxidation. Geochim. Cosmochim. Acta 75, 1173–1184. doi: 10.1016/j.gca.2010.12.006
Fischer, A., Manefield, M., and Bombach, P. (2016). Application of stable isotope tools for evaluating natural and stimulated biodegradation of organic pollutants in field studies. Cur. Op. Biotechnol. 41, 99–107. doi: 10.1016/j.copbio.2016.04.026
Gilbert, A., Sherwood Lollar, B., Musat, F., Giunta, T., Chen, S., Kajimoto, Y., et al. (2019). Intramolecular isotopic evidence for bacterial oxidation of propane in subsurface natural gas reservoirs. Proc. Natl. Acad. Sci. U. S. A. 116, 6653–6658. doi: 10.1073/pnas.1817784116
Hahn, C. J., Laso-Pérez, R., Vulcano, F., Vaziourakis, K. M., Stokke, R., Steen, I. H., et al. (2020). Candidatus Ethanoperedens, a thermophilic genus of Archaea mediating the anaerobic oxidation of ethane. mBio 11, e00600–20.
Hahn, C. J., Lemaire, O. N., Kahnt, J., Engilberge, S., Wegener, G., and Wagner, T. (2021). Crystal structure of a key enzyme for anaerobic ethane activation. Science 373, 118–121. doi: 10.1126/science.abg1765
Holler, T., Wegener, G., Knittel, K., Boetius, A., Brunner, B., Kuypers, M. M. M., et al. (2009). Substantial 13C/12C and D/H fractionation during anaerobic oxidation of methane by marine consortia enriched in vitro. Environ. Microbiol. Rep. 1, 370–376. doi: 10.1111/j.1758-2229.2009.00074.x
Jaekel, U., Vogt, C., Fischer, A., Richnow, H. H., and Musat, F. (2014). Carbon and hydrogen stable isotope fractionation associated with the anaerobic degradation of propane and butane by marine sulfate-reducing bacteria. Environ. Microbiol. 16, 130–140. doi: 10.1111/1462-2920.12251
Kampara, M., Thullner, M., Richnow, H. H., Harms, H., and Wick, L. Y. (2008). Impact of bioavailability restrictions on microbially induced stable isotope fractionation: 2. Experimental evidence. Environ. Sci. Technol. 42, 6552–6558. doi: 10.1021/es702781x
Kampara, M., Thullner, M., Harms, H., and Wick, L. Y. (2009). Impact of cell density on microbially induced stable isotope fractionation. Appl. Microbiol. Biotechnol. 81, 977–985. doi: 10.1007/s00253-008-1755-0
Kinnaman, F. S., Valentine, D. L., and Tyler, S. C. (2007). Carbon and hydrogen isotope fractionation associated with the aerobic microbial oxidation of methane, ethane, propane and butane. Geochim. Cosmochim. Acta. 71, 271–283. doi: 10.1016/j.gca.2006.09.007
Kniemeyer, O., Musat, F., Sievert, S. M., Knittel, K., Wilkes, H., Blumenberg, M., et al. (2007). Anaerobic oxidation of short-chain hydrocarbons by marine sulphate-reducing bacteria. Nature 449, 898–901. doi: 10.1038/nature06200
Kümmel, S., Kuntze, K., Vogt, C., Boll, M., Heider, J., and Richnow, H. H. (2013). Evidence for benzylsuccinate synthase subtypes by stable isotope tools. J. Bacteriol. 195, 4660–4667. doi: 10.1128/JB.00477-13
Laso-Perez, R., Wegener, G., Knittel, K., Widdel, F., Harding, K. J., Krukenberg, V., et al. (2016). Thermophilic archaea activate butane via alkyl-coenzyme M formation. Nature 539, 396–401. doi: 10.1038/nature20152
Mastalerz, V., de Lange, G. J., and Dählmann, A. (2009). Differential aerobic and anaerobic oxidation of hydrocarbon gases discharged at mud volcanoes in the Nile deep-sea fan. Geochim. Cosmochim. Acta 73, 3849–3863. doi: 10.1016/j.gca.2008.12.030
Musat, F. (2015). The anaerobic degradation of gaseous, nonmethane alkanes - from in situ processes to microorganisms. Comp. Struct. Biotechnol. J. 13, 222–228. doi: 10.1016/j.csbj.2015.03.002
Musat, F., Vogt, C., and Richnow, H. H. (2016). Carbon and hydrogen stable isotope fractionation associated with the aerobic and anaerobic degradation of saturated and alkylated aromatic hydrocarbons. J. Mol. Microbiol. Biotechnol. 26, 211–226. doi: 10.1159/000442161
Pozzer, A., Pollmann, J., Taraborrelli, D., Jöckel, P., Helmig, D., Tans, P., et al. (2010). Observed and simulated global distribution and budget of atmospheric C 2 -C 5 alkanes. Atmos. Chem. Phys. 10, 4403–4422. doi: 10.5194/acp-10-4403-2010
Rojo, F. (2009). Degradation of alkanes by bacteria. Environ. Microbiol. 11, 2477–2490. doi: 10.1111/j.1462-2920.2009.01948.x
Savage, K. N., Krumholz, L. R., Gieg, L. M., Parisi, V. A., Suflita, J. M., Allen, J., et al. (2010). Biodegradation of low-molecular-weight alkanes under mesophilic, sulfate-reducing conditions: metabolic intermediates and community patterns. FEMS Microbiol. Ecol. 72, 485–495. doi: 10.1111/j.1574-6941.2010.00866.x
Seitz, K. W., Dombrowski, N., Eme, L., Spang, A., Lombard, J., Sieber, J. R., et al. (2019). Asgard archaea capable of anaerobic hydrocarbon cycling. Nat. Commun. 10:1822. doi: 10.1038/s41467-019-09364-x
Shennan, J. L. (2006). Utilisation of C2–C4 gaseous hydrocarbons and isoprene by microorganisms. J. Chem. Technol. Biotechnol. 81, 237–256. doi: 10.1002/jctb.1388
Sluis, M. K., Sayavedra-Soto, L. A., and Arp, D. J. (2002). Molecular analysis of the soluble butane monooxygenase from ‘Pseudomonas butanovora’. Microbiol 148, 3617–3629. doi: 10.1099/00221287-148-11-3617
Staal, M., Thar, R., Kuhl, M., van Loordsrecht, M. C. M., Wolf, G., de Brouwer, J. F. C., et al. (2007). Different carbon isotope fractionation pattern during the development of phototrophic freshwater and marine biofilms. Biogeosciences. 4, 613–626. doi: 10.5194/bg-4-613-2007
Takahashi, J., Ichikawa, Y., Sagae, H., Komura, I., Kanou, H., and Yamada, K. (1980). Isolation and identification of n-butane-assimilating bacterium. Agric. Biol. Chem. 44, 1835–1840. doi: 10.1271/bbb1961.44.1835
Templeton, A. S., Chu, K.-H., Alvarez-Cohen, L., and Conrad, M. E. (2006). Variable carbon isotope fractionation expressed by aerobic CH4-oxidizing bacteria. Geochim. Cosmochim. Acta. 70, 1739–1752. doi: 10.1016/j.gca.2005.12.002
Tissot, B.P., and Welte, D.H. (1984) Petroleum formation and occurrence (2nd Edn.). Berlin, Springer.
Valentine, D. L., Kessler, J. D., Redmond, M. C., Mendes, S. D., Heintz, M. B., Farwell, C., et al. (2010). Propane respiration jump-starts microbial response to a deep oil spill. Science 330, 208–211. doi: 10.1126/science.1196830
Vogt, C., Cyrus, E., Herklotz, I., Herrmann, S., Bahr, A., Richnow, H. H., et al. (2008). Evaluation of aerobic and anaerobic toluene degradation pathways by two dimensional stable isotope fractionation. Environ. Sci. Technol. 42, 7793–7800. doi: 10.1021/es8003415
Vogt, C., Dorer, C., Musat, F., and Richnow, H. (2016). Multi-element isotope fractionation concepts to characterize the biodegradation of hydrocarbons – from enzymes to the environment. Curr. Op. Biotechnol. 41, 90–98. doi: 10.1016/j.copbio.2016.04.027
Widdel, F., and Bak, F. (1992) in Gram-negative mesophilic sulfate-reducing bacteria, in the prokaryotes. eds. A. Balows, H. G. Trüper, M. Dworkin, W. Harder, and K.-H. Schleifer. 2nd ed (Berlin; New York: Springer-Verlag), 3352–3378.
Widdel, F., and Musat, F. (2019). “Diversity and common principles in enzymatic activation of hydrocarbons: an introduction” in Aerobic utilization of hydrocarbons, oils, and lipids. ed. F. Rojo (Cham: Springer International Publishing), 3–32.
Wu, M., Li, J., Leu, A. O., Erler, D. V., Stark, T., Tyson, G. W., et al. (2022). Anaerobic oxidation of propane coupled to nitrate reduction by a lineage within the class Symbiobacteriia. Nat. Commun. 13:6115. doi: 10.1038/s41467-022-33872-y
Keywords: alkanes, CSIA, monooxygenase, Thauera butanivorans, stable isotopes, isotope fractionation
Citation: Vogt C, Song Z, Richnow H-H and Musat F (2023) Carbon and hydrogen stable isotope fractionation due to monooxygenation of short-chain alkanes by butane monooxygenase of Thauera butanivorans Bu-B1211. Front. Microbiol. 14:1250308. doi: 10.3389/fmicb.2023.1250308
Received: 29 June 2023; Accepted: 07 September 2023;
Published: 25 September 2023.
Edited by:
Xuwang Zhang, Dalian University of Technology, ChinaReviewed by:
Lei Cheng, Chinese Academy of Agricultural Sciences, ChinaCopyright © 2023 Vogt, Song, Richnow and Musat. This is an open-access article distributed under the terms of the Creative Commons Attribution License (CC BY). The use, distribution or reproduction in other forums is permitted, provided the original author(s) and the copyright owner(s) are credited and that the original publication in this journal is cited, in accordance with accepted academic practice. No use, distribution or reproduction is permitted which does not comply with these terms.
*Correspondence: Carsten Vogt, Y2Fyc3Rlbi52b2d0QHVmei5kZQ==; Florin Musat, ZmxvcmluLm11c2F0QGJpby5hdS5kaw==
Disclaimer: All claims expressed in this article are solely those of the authors and do not necessarily represent those of their affiliated organizations, or those of the publisher, the editors and the reviewers. Any product that may be evaluated in this article or claim that may be made by its manufacturer is not guaranteed or endorsed by the publisher.
Research integrity at Frontiers
Learn more about the work of our research integrity team to safeguard the quality of each article we publish.