- 1Department of Pharmacy, China-Japan Friendship Hospital, Beijing, China
- 2Department of Pharmacy, The Affiliated Taian City Central Hospital of Qingdao University, Taian, China
The World Health Organization has recently published a list of 12 drug-resistant bacteria that posed a significant threat to human health, and Pseudomonas aeruginosa (P. aeruginosa) was among them. In China, P. aeruginosa is a common pathogen in hospital acquired pneumonia, accounting for 16.9–22.0%. It is a ubiquitous opportunistic pathogen that can infect individuals with weakened immune systems, leading to hospital-acquired acute and systemic infections. The excessive use of antibiotics has led to the development of various mechanisms in P. aeruginosa to resist conventional drugs. Thus, there is an emergence of multidrug-resistant strains, posing a major challenge to conventional antibiotics and therapeutic approaches. Antimicrobial peptides are an integral component of host defense and have been found in many living organisms. Most antimicrobial peptides are characterized by negligible host toxicity and low resistance rates, making them become promising for use as antimicrobial products. This review particularly focuses on summarizing the inhibitory activity of natural antimicrobial peptides against P. aeruginosa planktonic cells and biofilms, as well as the drug interactions when these peptides used in combination with conventional antibiotics. Moreover, the underlying mechanism of these antimicrobial peptides against P. aeruginosa strains was mainly related to destroy the membrane structure through interacting with LPS or increasing ROS levels, or targeting cellular components, leaded to cell lysis. Hopefully, this analysis will provide valuable experimental data on developing novel compounds to combat P. aeruginosa.
1. Background
As a common opportunistic gram-negative pathogen, Pseudomonas aeruginosa (P. aeruginosa) is a significant cause of nosocomial infection, leading to a variety of infections such as pneumonia, bacteremia, and urinary tract infections (Terzi et al., 2014; Yaeger et al., 2021). Individuals with weakened immune systems, such as those with metabolic diseases, hematological diseases, and malignant tumors, are more susceptible to P. aeruginosa infection (Burrows, 2018; Yaeger et al., 2021). The drugs commonly used for treating P. aeruginosa infection in clinical settings include penicillin, cephalosporins, aztreonam, aminoglycosides, fluoroquinolones and carbapenems (Moore and Flaws, 2011; Jangra et al., 2022). However, due to the inappropriate use of antibiotics, the resistance of P. aeruginosa to carbapenems and many other antibacterial drugs has increased rapidly, making the number of drug-resistant strains continue to arise and bringing challenges to clinical treatment (Hancock and Speert, 2000; Jangra et al., 2022). Therefore, there is an urgent need to develop new strategies to combat resistant P. aeruginosa infections.
The natural immune system serves as a functional and physiological barrier against microbial infections. Within this system, there are important effectors called antimicrobial peptides (AMPs) or host defense peptides (HDPs). Studies have shown that AMPs not only play a role in the regulation of inflammatory response, immune system, and apoptosis pathway, but they also exhibit a broad spectrum of antimicrobial activities (Su et al., 2010; Cho et al., 2012; Hancock et al., 2012; Buda De Cesare et al., 2020). Currently, more than 3,000 natural AMPs derived from microorganisms, plants, mammals, amphibians, and insects have been registered in the Antimicrobial Peptide Database (unmc.edu) (Bin Hafeez et al., 2021).
Most AMPs are composed of 10–100 amino acids and have an amphiphilic conformation, which can bind to the negatively charged components of bacterial cell surface, and integrate with the lipid bilayer, or enter the cytoplasm through the cell membrane, thus exhibit great antimicrobial activity against pathogens (Huan et al., 2020; Lachowicz et al., 2020). Multiple studies have demonstrated that many AMPs possess good antibacterial properties on their own. For example, BCp12 had been found to possess obvious antimicrobial activity against Staphylococcus aureus, Listeria monocytogenes, Escherichia coli (E. coli) and Salmonella typhimurium strains, with minimum inhibitory concentrations (MICs) of 0.4–1.6 mg/mL. Moreover, BCp12 induced low hemolytic activity and cytotoxicity on mammalian cells (Zhao et al., 2020). Furthermore, the combination of AMPs and traditional antibiotics demonstrated synergistic antimicrobial effects, and the combination not only enhanced the effectiveness of the two, but also expanded the antimicrobial spectrum of antibiotics. Notably, L1G, L7A and L1GA5K possessed good bioactivity, mild cytotoxicity, and high stability, and could rapidly kill bacteria by membrane rupture and intracellular materials release, with a MIC of 4–32 μM. Moreover, when combined with rifampicin, polymyxin B, and gentamicin, they exhibited either synergistic or additive effects against gram-negative bacteria (Zhu et al., 2021). AMPs may exert their antibacterial effects through different mechanisms, either by directly interacting with the bacterial membrane or by targeting other cellular components. The membrane-mediated mechanisms of action can be described by models: (1) the toroidal pore model, AMPs can enter the membrane vertically, the lipids would be dragged and bended by AMPs when they form the toroidal pore; (2) the barrel-stave model, AMPs can insert into the membrane, forming barrels and opening stable and transmembrane pores, thereby destabilizing the membrane potential and promoting leakage of ions and biomolecules; (3) the carpet-stave model, AMPs can act as detergents by localizing on the horizontal plane of the plasma membrane, then causing alterations and destruction; (4) the aggregate channel model, AMPs can competitively displace lipopolysaccharides (LPS)-associated divalent cations, leading to nonstructural aggregation of AMPs and lipids that disrupts the outer and inner membranes (Lee et al., 2011; Huan et al., 2020; Roque-Borda et al., 2021). In the non-membrane-targeted mechanism of action of AMPs, they can enter cells by direct penetration or endocytosis, and achieve antimicrobial activity through several mechanisms: (1) affecting nucleic acid and protein synthesis; (2) inhibiting enzyme activity and energy metabolism; (3) destroying cellular organelles; (4) producing oxidative stress response (Nguyen et al., 2011; Lei et al., 2019; Saeed et al., 2022).
In this review, we have presented a comprehensive summary of the inhibitory effects of natural AMPs used alone, and in combination with other drugs against P. aeruginosa planktonic cells and biofilms in Tables 1, 2. Moreover, we also summarized the relevant antibacterial mechanisms of AMPs, although they still needed to be further investigated. We believe this review will provide valuable experimental data for developing novel anti-P. aeruginosa agents.
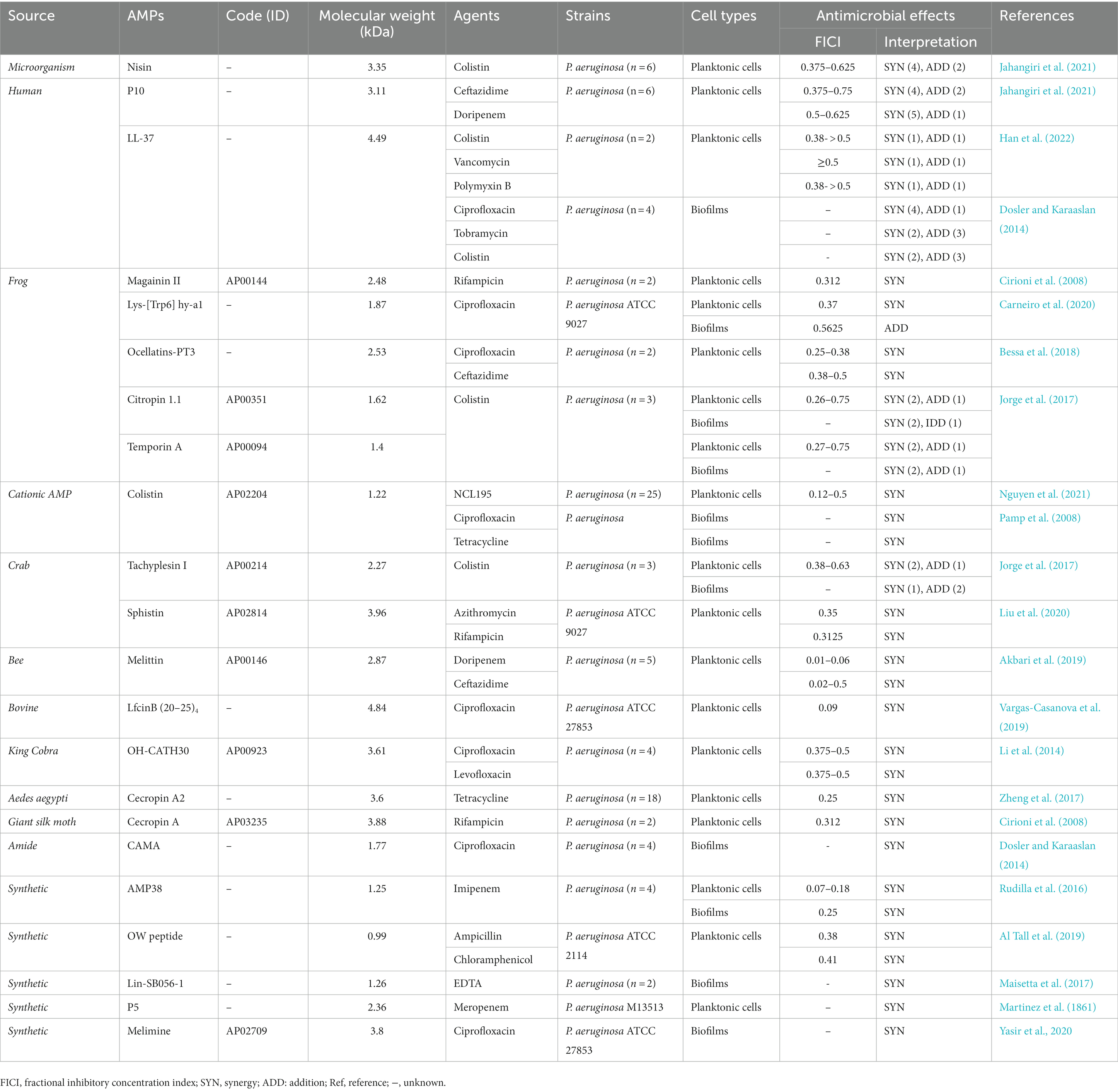
Table 2. The anti-Pseudomonas aeruginosa activity of antimicrobial peptides (AMPs) when used in combination with drugs.
2. Anti-Pseudomonas aeruginosa activity of AMPs
The AMP database has documented many AMPs that have been derived from various lives, including 335 bacteriocins from bacteria, 4 AMPs from archaea, 8 AMPs from protozoa, 13 AMPs from fungi, 342 AMPs from plants, 2,200 AMPs from animals, and some synthetic peptides (Zhu et al., 2017; Boparai and Sharma, 2020a; Perez-Rodriguez et al., 2022a). In this review, our focus is on AMPs that have anti-P. aeruginosa effects, and we have categorized them into six different groups according to their origins: microorganisms, plants, mammals, insects, amphibians, and a varied group of others. In Table 1, we summarized the inhibitory activity of natural AMPs against P. aeruginosa according to different sources, and ranked the antibacterial activity from strongest to weakest.
2.1. AMPs from microorganisms
Numerous researchers have found and isolated a variety of AMPs from bacteria and fungi, which have significant antibacterial activity against different types of pathogens (Andrä et al., 2001; Szekeres et al., 2005; Buda De Cesare et al., 2020; Boparai and Sharma, 2020b).
The rumen is a complex microbiome consisting of various microorganisms, including fungi, bacteria, and viruses (Morgavi et al., 2013; McCann et al., 2014; Oyama et al., 2017). Many researchers have regarded it as a potential resource for discovering new AMPs. For example, Oyama et al. and Mulkern et al. reported that Lynronne-1, 2, 3, and P15s (with 2.83–3.08 kDa in size) were identified from bovine rumen microbiome all possessed good antibacterial activity (Privé et al., 2015; Oyama et al., 2017; Mulkern et al., 2022). In these two studies, researchers found that the four peptides possessed significant in vitro activity against P. aeruginosa and E. coli, with MICs of 4–512 and 32–64 μg/mL, respectively. In vivo experiments, local administration of Lynronne-1 (10% w/v) significantly reduced the number of bacteria infected by methicillin-resistant Staphylococcus aureus (MRSA) in mice (Oyama et al., 2017). In addition, in Galleria mellonella (G. mellonella) infection model, treatment with Lynronne 1 and Lynronne 2 at 32 and 128 mg/kg resulted in a 100% survival rate of the larvae (Mulkern et al., 2022). Among them, Lynronne-2 had the highest safety, as it did not exhibit any cytotoxicity toward HUVEC and HepG2 cells at a concentration of 128 μg/mL. However, Lynronne-1 and Lynronne-3 showed some degree of toxicity, with their 50% lethal concentration (LC50) values of 98.1 and 128 μg/mL (Oyama et al., 2017). In addition to their ability to bind to bacterial membrane lipids and enhance membrane permeability, Lynronne-1 and 2 downregulated genes expression of arcA, arcB, and arcC, while P15s decreased arcD expression and indirectly affected arginine metabolism.
In addition to rumen microbiome, bacteriocins produced by lactic acid bacteria (LAB) had been extensively studied due to their safety and utility. Acidocin 4356 (ACD) is a novel bacteriocin with a size of 8.3 kDa, which was isolated from Lactobacillus acidophilus ATCC 4356 (Modiri et al., 2020). ACD had antimicrobial activity against both E. coli and P. aeruginosa, causing 35 and 80% inhibition of their growth at the same concentration. As shown in Table 1, ACD had inhibitory effects against P. aeruginosa planktonic cells, with an MIC90 of 128.22 μg/mL. Additionally, ACD showed potent anti-P. aeruginosa biofilm activity, killing over 90% of biofilms at a concentration of 128.22 μg/mL and significantly reducing the pre-formed biofilms at a concentration of 256.44 μg/mL. In in vivo experiments, ACD was found to effectively suppress infection caused by P. aeruginosa in mice. Compared with the control group, the peptide-treated mouse lung slices exhibited the decreased recruitment of macrophages and lung cells, as well as a reduction in epithelial hyperplasia and structural degeneration. Mechanism of action experiments revealed that ACD not only enhanced bacterial membrane perturbation, but also reduced the production of P. aeruginosa virulence factors, such as pyoverdine siderophore, pyocyanin toxin, secretory protease, and elastase enzyme (Modiri et al., 2020). Moreover, it had a low hemolytic activity of less than 20% on human erythrocytes at a concentration of 400 μg/mL, indicating its potential as a promising therapeutic compound. Actifensin, a bacteriocin produced by Actinomyces ruminicola with a molecular weight of 4.1 kDa. It exhibited weak antibacterial activity against P. aeruginosa strains with MIC of 1448 μg/mL, whereas predominantly inhibited methicillin-resistant S. aureus and Candida albicans with MIC values of 23 μg/mL and 45 μg/mL. Its mechanism of anti-P. aeruginosa may be related to induce reactive oxygen species (ROS) generation, thereby destroyed cellular membrane permeability, but further molecular mechanisms were unclear (Sugrue et al., 2020; Gbala et al., 2022). More importantly, it has been found to have a high biosafety, and it causes less than 1.5% hemolysis on mouse erythrocytes in all tested concentrations.
2.2. AMPs from plants
Plant defensins are small cationic peptides with 5–7 kDa in size that contain multiple cysteine groups to safeguard plants against microbial invasion (Gbala et al., 2022). These peptides have a wide-ranging ability to inhibit the growth of various pathogens including filamentous fungi and bacteria (Perez-Rodriguez et al., 2022b), we summarized the data on the inhibitory activity of several plant-derived AMPs against P. aeruginosa in Table 1.
Many peptides found in spinach leaves exhibited antimicrobial activities. One such peptide is So-D1-7, which was effective to against gram-positive and gram-negative bacterial pathogens as well as fungi at concentrations below 20 μM (Segura et al., 1998). Another antibacterial component identified in spinach leaves was Defensin-d2, which had a molecular weight of 5.8 kDa. It had been found to be more active against P. aeruginosa than E. coli in vitro, with the MIC values of 7.5 and 30 μg/mL, respectively (Gbala et al., 2022). Defensin-d2 also had the ability to inhibit biofilm formation of P. aeruginosa in a concentration dependent manner. When treated with 3.75 μg/mL of defensin-d2 for 24 h, the mass percentage of biofilm was reduced by more than 70% (Gbala et al., 2022). The related mechanism was similar to that of actifensin, involving increased production of ROS production and disruption of cell membrane. The highest hemolysis rate of 2.89% was observed at the concentration of 985 μg/mL.
Medicago truncatula, a type of plant, contained various peptides that exhibited antimicrobial activities against human pathogens. These peptides interacted with the phospholipids of fungal cell membranes, causing an increase in membrane permeability and ultimately leading to fungi cell death (Sathoff et al., 2019). In a study conducted by Sathoff et al. it was found that core MtDef4 and MtDef5 (with the molecular weights of 1.98–2.04 kDa) derived from Medicago truncatula, were able to inhibit the growth of P. aeruginosa with IC50 = 1.7–4.2 and 8.5–14.6 μM, respectively (Sathoff et al., 2019, 2020. Moreover, Core MtDef4 could induce gene expression of the amino arabinose modification of LPS and surface polycation spermidine production operons, and it could damage outer membranes of P. aeruginosa, while MtDef5 appeared to interfere with DNA synthesis and transcription (Velivelli et al., 2018; Sathoff et al., 2020).
2.3. AMPs from mammals
AMPs derived from human play a crucial role in the human immune system, which can protect the body from pathogen invasion by reducing their virulence factors (Bosso et al., 2018). Therefore, the exploration of human peptides may be significant for developing antimicrobial drugs. As illustrated in Table 1, specific description of several AMPs derived from mammals with significant anti-P. aeruginosa activity are shown below.
Protegrins and their derivatives are a new class of AMP antibiotics derived from mammals, which have been proved to have a wide range of antimicrobial activities, including gram-positive and gram-negative bacteria as well as fungi (Bellm et al., 2000). Porcine protegrin-1 (PG-1), an AMP with a molecular weight of 2.59 kDa, had in vitro antimicrobial activities against gram-negative bacteria. It effectively inhibited the growth of E. coli and P. aeruginosa planktonic cells, with MICs of 0.2–0.5 and 0.3–0.8 μg/mL, respectively (Turner et al., 1998). The mechanistic studies revealed that PG-1 was capable of binding to the membrane, forming voltage-gated channels within the lipid bilayer and dissolving liposomes, ultimately leading to the disruption of the cell membrane and cell death (Bellm et al., 2000). The results of the cell cytotoxicity experiment showed that PG-1 had limited effects on mammalian cells, only reducing cell number at concentrations over 50 μg/mL (Morroni et al., 2019).
Apart from the physical skin barrier-stratum corneum, human skin also has an innate defense barrier. This barrier consists of AMPs and proteins, which help to control the growth of microorganisms on the body surface and reduce the risk of infection (Elias, 2005; Gallo and Hooper, 2012; Harder et al., 2013). Filaggrin-2 (FLG2), also known as ifapsoriasin, is expressed in human skin and serves to protect the skin from environmental damage (Wu et al., 2009). The radial diffusion assays provided evidence that FLG2-4, with a molecular mass ranging from 70 to 140 kDa, had powerful antimicrobial activities against P. aeruginosa and E. coli planktonic cells with MEC of 0.4 μM and 2.4 μM, respectively (Hansmann et al., 2015). Besides, the underlying anti-P. aeruginosa mechanism of FLG2-4 appears to involve inducing membrane blebbing, disrupting DNA polymerases activity, ultimately halting bacterial replication.
Cathelicidin LL-37 is a cationic AMP that is produced by humans, and has a molecular weight of 4.493 kDa. It is a broad-spectrum cathelicidin known for its strong chemotaxis and immunomodulatory properties. Besides, it plays a role in regulating the systems of immune, respiratory, gastrointestinal, and skin by participating in molecular pathways (Sørensen et al., 2001; Zanetti, 2005; Burton and Steel, 2009; Fabisiak et al., 2016).
LL-37 exhibited strong efficacy against most detected gram-negative bacteria. In broth microdilution assay, LL-37 demonstrated moderate antimicrobial activity against E. coli and P. aeruginosa with MIC values of 6–32 and 8–32 μg/mL, respectively (Turner et al., 1998). In addition, LL-37 displayed potent anti-biofilm activities, inhibiting 80% biofilm formation of P. aeruginosa at 16 μg/mL and reducing the thickness of pre-formed biofilm at 4 μg/mL (Overhage et al., 2008). The quorum sensing (QS) system was closely related to bacterial biofilms formation, the expression of virulence factors, and multiple drug resistance pathways (Al Akeel et al., 2019). There are two characteristic QS systems in P. aeruginosa: the Las system and the Rh1 system (Yu and Ma, 2017). LL-37 was found to inhibit P. aeruginosa biofilm formation through various mechanisms, including reducing bacterial cells attachment, stimulating motility twitch, suppressing the expression of key QS signaling molecules and significantly downregulating the expression of more than 50 biofilm formation-related genes, including lasI and rhlR genes (Overhage et al., 2008). However, it is important to note that LL-37 has high toxicity to mammalian cells. Therefore, further application of LL-37 may require structural modifications or combination with other drugs to mitigate this toxicity.
In a recent study, researchers identified two cathelicidins called BMAP-27 and 28 from bovine, they had the molecular masses of about 3.5 kDa. Both BMAP-27 and BMAP-28 had significant antibacterial properties against P. aeruginosa and E. coli, with MIC values of 1 μM and 0.25–2 μM (Skerlavaj et al., 1996). Another study found that these two AMPs have MIC90 values 16 and 32 μg/mL for 25 other strains of P. aeruginosa (Pompilio et al., 2012). Besides, BMAP-27 and 28 were not only effective in reducing the formation of P. aeruginosa biofilms, but also showed efficacy against biofilms that had already formed, with the concentration of 8–16 and 80–160 μg/mL, respectively (Pompilio et al., 2012). The antibacterial mechanism was related to the rapid induction of membrane permeabilization (Xie et al., 2020). However, these two cathelicidins have certain cytotoxicity to human red blood cells and neutrophils. One way to address this issue is by shortening the C-terminal of the cathelicidins, which greatly reduces their cytotoxicity without compromising their antibacterial activity.
Human beta-defensin 2 (HBD2) was one of the human β-defensins with a molecular mass of 4–5 kDa, it has been found to have multiple physiological functions (Yang et al., 1999). HBD2 showed significant antimicrobial activity against E. coli and P. aeruginosa with LD90 of 10 μg/mL (Schröder and Harder, 1999). At the concentration of 0.25–0.5 μM, HBD2 exhibited inhibitory effect against P. aeruginosa biofilm formation, although it did not inhibit metabolic activity (Parducho et al., 2020). The results also indicated that HBD2 could induce the change of the biofilm surface topology, then interfering with the transport of biofilm precursors into the extracellular space. Furthermore, HBD2 has been found to be biocompatible and safe, with no toxicity observed in hMSCs, osteoblasts, keratinocytes or HeLa cells at any concentration tested (Warnke et al., 2013).
2.4. AMPs from insects
Many AMPs were isolated from the plasma and leukocyte extracts of insects, and these have been shown to possess strong antibacterial effects (Van Moll et al., 2022). In recent years, many studies investigating the anti-P. aeruginosa activity of insect AMPs had been carried out, and we summarized some of the findings below.
Royal jelly (RJ) is a substance secreted by the hypopharyngeal and mandibular glands of worker bees, and it is enriched with protein, carbohydrates, vitamins, and minerals (Fujiwara et al., 1990; Fontana et al., 2004). A study has discovered novel AMPs (Jelleines) in RJ that have broad-spectrum antimicrobial activities, including gram-positive and gram-negative bacteria (Fontana et al., 2004). The molecular mass of Jelleines I-III is approximately 1 kDa. They displayed good in vitro antibacterial activity against E. coli and P. aeruginosa, with MIC values of 2.5–15 and 10–30 μg/mL, respectively (Fontana et al., 2004). Further investigations indicate that Jelleine-I could form aggregates that accumulated in the head group region of the membrane, leading to cell membrane disruption and leakage (Cabrera et al., 2014). Jelleine-I has been reported to have a hemolytic effect of 5–11.3% on rat erythrocytes and mouse erythrocytes, which possesses the potential for further study.
Two cecropins (Hill-Cec1 and Hill-Cec10) that were discovered in black soldier fly and are 4.79 and 5.28 kDa in size, had been found to effectively inhibit the growth of P. aeruginosa and E. coli planktonic cells, with the MIC values of 0.25 and 1 μM, respectively (Van Moll et al., 2022). The biofilm mass could be reduced by 50% when the concentration of Hill-Cec1 and Hill-Cec10 were 1.3 ± 0.57 μM and 7.5 ± 3.5 μM, respectively. However, they had no scavenging effect on the pre-formed biofilm. Furthermore, they exerted their antimicrobial effects by disrupting the cell membrane of P. aeruginosa and causing its depolarization. In addition, both cecropins showed a low hemolysis rate of less than 10% at a concentration of 64 μM, indicating a higher level of safety.
2.5. AMPs from amphibians
Previous studies had identified a variety of AMPs from amphibian skin and their antimicrobial activities had been demonstrated (Simmaco et al., 1994; Ponti et al., 1999; Luca et al., 2013). Here, we summarized the anti-P. aeruginosa activity of several AMPs.
Dermaseptins are a family of linear polycationic peptide consisting of 28 to 34 amino acids, which were originally isolated from the skin of Phyllomedusa sauvagei, a tree-dwelling South American frog (Mor et al., 1991). Numerous studies have confirmed that dermaseptins exhibited significant and extensive antimicrobial activities against various microorganisms, including gram-positive and gram-negative bacteria (Marynka et al., 2007; Zairi et al., 2007; Zaïri et al., 2013), fungi (Morton et al., 2007), viruses (Bergaoui et al., 2013), and protozoa (Dagan et al., 2002; Efron et al., 2002). A study demonstrated that dermaseptin K4S4 and K4K20S4, which have a molecular mass of about 2.8 kDa, displayed antibacterial effects against P. aeruginosa PA01 and E. coli MG1655, with MIC of 0.39–12.5 μg/mL and 0.19–0.39 μg/mL, respectively (Zaïri et al., 2014). In addition, when the concentration of dermaseptin derivatives were 2-fold of MIC, the pre-formed biofilm of P. aeruginosa wasn’t dissolved or disrupted, but the survival rate of biofilm cells was significantly reduced. The mechanisms of anti-P. aeruginosa biofilm may be similar to another dermaseptin derivative, S4(1–16) M4Ka, which inhibited biofilm formation by breaking down membrane lipids and dispersing bacteria (Quilès et al., 1858). Moreover, their CC50 (50% cytotoxic concentration) value was around 28 μg/mL. Although their toxicity is much lower than that of dermaseptin S4, further structural optimization is still urgent for their future study.
Wang G et al. had identified five AMPs (Temporin-LK1, gaegurin-LK1, gaegurin-LK2, rugosin-LK1, and rugosin-LK2) with molecular masses ranging from 1.95 to 3.52 kDa from skin secretions of Limnonectes kuhlii frogs (Wang et al., 2013). Experimental results demonstrated that they all possessed very effective antibacterial activities against P. aeruginosa and E. coli ML-35P strains, with MIC of 2.5–10 μg/mL and 10–50 μg/mL, respectively, indicating the potential to treat bacterial infections. These five AMPs also have slight hemolytic activities, causing the lysis of rabbit red blood cells in varying percentages. Temporin-LK1, gaegurin-LK1, gaegurin-LK2, rugosin-LK1, and rugosin-LK2 can induce the hemolysis of 10.2, 5.6, 6.2, 3.8, and 6.1%, respectively.
Esculentin (1–21), which was isolated from amphibian skin and had a size of 2.61 kDa, demonstrated efficacy on planktonic cells of gram-negative bacterial pathogen P. aeruginosa and E. coli, with MIC of 3.2 μM and 0.65 μM (Islas-Rodrìguez et al., 2009). Another study found that Esc (1–21) also exhibited antibacterial effects against MDR clinical isolates of P. aeruginosa, with the MICs of 4–8 μM (Luca et al., 2013). Esc (1–21) also had potent anti-P. aeruginosa biofilm effects, the minimum biofilm eradication concentration (MBEC) and minimum bactericidal concentration (MBCb) were 6 μM and 12 μM, respectively. Furthermore, in in vivo experiments, it was observed that Esc (1–21) significantly increased the survival rate of mice with P. aeruginosa sepsis or pulmonary infection (Luca et al., 2013). The mechanism of action showed that it can anti-P. aeruginosa PAO1 biofilm by penetrating biofilm plasma membrane and causing the release of β-galactosidase (Luca et al., 2013). Esc (1–21) did not show any toxicity to human erythrocytes, lung epithelial cells or mouse macrophages in vitro at the concentration of MIC.
Lin et al. discovered three AMPs (brevin-1HL, temporin-HLa, and temperin-HLb) from the skin secretion of Hylarana latouchii, and they had a molecular weight of 1.5–2.5 kDa (Lin et al., 2021). Brevin-1HL exhibited a moderate effect against P. aeruginosa planktonic cells and biofilm, with MIC and MBIC of 256 and 512 μg/mL, respectively. Temporin-HLa and temperin-HLb only showed a weak inhibitory effect on P. aeruginosa, with MIC over 512 μg/mL. The study also revealed that these three peptides induced bacterial death by destroying cell membranes and binding to bacterial DNA. Among them, only Brevinin-1HL possesses the bactericidal effects against E. coli, with an MBC of 128 μg/mL. However, it should be noted that Brevinin-1HL exhibits strong hemolytic activity on horse red blood cells, causing 86.8% of hemolysis at 256 μg/mL (Lin et al., 2021).
2.6. AMPs from other groups
In addition to the AMPs mentioned above, we have also compiled a list of AMPs with great antimicrobial activities identified from other groups in Table 1, such as fishes, alligators, silkworm, and snails. One of these AMPs, called Gaduscidin-1 (Gad-1), was originated from Atlantic cod, and has been shown to have antibacterial effect against P. aeruginosa (Portelinha and Angeles-Boza, 2021). Gad-1 exhibited inhibitory effect against P. aeruginosa planktonic cells, with the MIC of 2 μg/mL and 16 μg/mL at PH 6.4 and 7.4, respectively. Furthermore, Gad-1 was also effective in removing 60–72% of the pre-formed biofilm at a concentration of 64 μM. It also could completely inhibit biofilm formation when the concentration exceeding 32 μM (Portelinha and Angeles-Boza, 2021). Moreover, biofilms contain extracellular DNA (eDNA), which not only helps in stabilizing the structure of biofilms but also promotes horizontal gene transfer, ultimately leading to increased biofilm resistance to antibiotics (Whitchurch et al., 2002; Wilton et al., 2016; Nolan et al., 2020). In addition to reducing the viability of P. aeruginosa in biofilms, Gad-1 can cleave eDNA, making it be a possible compound for the treatment of bacterial biofilms.
Piscidins were isolated from fishes and could kill a variety of microorganisms, including methicillin-resistant Staphylococcus aureus (Salger et al., 2016). Libardo et al. had tested the antimicrobial activities of piscidin 1 and piscidin 3 with the molecular mass of approximately 3 kDa, which showed excellent activity against E. coli and moderate anti-P. aeruginosa activities, with MICs of 2–8 μM and 16–32 μM, respectively (Salger et al., 2016). It is interesting to note that although these two AMPs are similar, they possess distinct antibacterial mechanisms of action. Piscidin 1 disrupts cell membrane, while piscidin-3 forms covalent bonds with Cu2+ through its N-terminal amino acid and exerts nuclease activity, leading to the disruption of P. aeruginosa eDNA and displaying anti-biofilm activity (Libardo et al., 2017). However, both piscidins have high hemolytic activity at a concentration of 25 μg/mL.
Crocodilian animals, which are a group of ancient creatures, have also been studied for their antimicrobial properties (Merchant et al., 2006; Darville et al., 2010). Researchers had discovered three AMPs derived from Alligator mississippiensis that have varying degrees of anti-P. aeruginosa activity and different mechanisms of action. Apo5 and Apo6, which have molecular masses of 3.13 and 2.79 kDa, respectively, displayed moderate anti-E. coli effects and stronger anti-P. aeruginosa activities, with EC50 of 3.85–19.7 and 0.0878–1.17 μg/mL. The underlying mechanism may be related to disrupt membrane (Barksdale et al., 2016). While A1P, with a molecular weight of 4.13 kDa, showed weaker inhibition against P. aeruginosa PAO1 with an EC50 of 38.6 μg/mL, and stronger inhibition against E. coli strains with an EC50 of 2.51–9.2 μg/mL. The main mechanism of action was not related to the disruption of cell membranes or DNA binding (Barksdale et al., 2016). These three AMPs have high safety profiles, with minimal hemolytic effects on erythrocytes at 300 μg/mL, and no significant cytotoxic to A549 cells at the concentration of 100 μg/mL. Bishop et al. discovered eight AMPs from Alligator mississippiensis plasma, and had evaluated their antimicrobial effects (Bishop et al., 2015). The data showed that five AMPs (APOC164-88, APOC167-88, FGG398-413, FGG401-413, A1P394–428), which have molecular masses ranging from 1.56 to 4.11 kDa, possessed antibacterial activities against E. coli and P. aeruginosa in vitro, with EC50 of 0.099–0.332 μM and 0.948–11.1 μM, respectively.
ABP-CM4, a peptide with a size of 3.79 kDa, was isolated from the hemolymph of the silkworm, Bombyx mori (Li et al., 2020). It showed promising activity against several microorganisms, in particular P. aeruginosa ATCC 27853 and E. coli K12D31, with MIC of 16 μM and 12 μM in vitro. The main mechanism of action of ABP-CM4 was the disruption of cell membrane and interaction with DNA. Importantly, ABP-CM4 was not cytotoxic to HEK-293 cells even at a concentration of 80 μM.
The mucus secreted by snails also exhibits antibacterial properties. For instance, Pitt et al. demonstrated that antimicrobial substance with 30–100 kDa in size in Helix aspersa mucus displayed inhibitory effects against two P. aeruginosa strains collected by laboratory. The antimicrobial disc diffusion assay showed obvious measurable zones of inhibition, with values of 11.12 mm and 11.63 mm (Pitt et al., 2015). Furthermore, researchers discovered that AMPs with molecular masses of 17.5–37.4 kDa, identified in Cornu aspersum mucus, could inhibited P. aeruginosa strains obtained from patients with cystic fibrosis. The mean zones of inhibition recorded were all between 9 and 13 mm (Pitt et al., 2019).
After summarizing the above antibacterial effects of AMPs, we found many AMPs from different sources exhibit activity against both P. aeruginosa and anti-E. coli. In general, higher concentrations of AMPs are required to kill P. aeruginosa compared to E. coli. The MIC values of AMPs were typically smaller than those of conventional antibiotics, indicating that the antibacterial potency of AMPs was generally stronger than conventional antibiotics. Notably, PG-1, FLG2-4, Jelleines and five AMPs derived from Limnonectes kuhlii skin secretions all possessed significant anti-planktonic cells effects in vitro, with MIC values ranged from 0.3 to 10 μg/mL. Furthermore, AMPs have a distinct antibacterial mechanism compared to traditional antibiotics, which makes it difficult for pathogenic bacteria to develop resistance to AMPs. The mechanism of action of AMPs involving disrupting cell membrane structure, as seen in protegrin-1, BMAP-27 and 28, Jelleines, Hill-Cec 1, and Dermaseptin K4S4. In addition, some AMPs exerted antibacterial effects by interacting with DNA of P. aeruginosa and impeding the substance metabolism, such as MtDef5, FLG2-4, Brevinin-1HL, Temporin-Hla, and ABP-CM4. Additionally, the hemolytic activity of these natural AMPs is quite low, except for LL-37 and piscidins. Thus, these results of these studies have shown that AMPs had the potential to be used as antibacterial candidate. However, further research is needed to determine their effectiveness in biofilm prevention, as well as their applicability in vivo and in clinical settings.
3. Interactions of AMPs with antibiotics
The above studies showed that AMPs primarily worked by damaging bacterial membrane. This was achieved by forming pores, which disrupted the osmotic pressure balance and finally leaded to cell lysis (Zhang et al., 2001; Hollmann et al., 2018; Luo and Song, 2021). The antibacterial mechanism of conventional antibiotics involves inhibiting of DNA replication, DNA transcription, cell wall synthesis, or targeting of topoisomerases and penicillin-binding proteins (PBPs) (Abushaheen et al., 2020). Unfortunately, MDR bacteria strains can prevent the entry of conventional antibiotics into bacterial cells, resulting in antimicrobial treatment failure (Abushaheen et al., 2020).
In theory, AMPs can increase the permeability of the cytoplasmic membrane, allowing antibiotics to enter the bacterial body and exert their antibacterial effects. Some studies have demonstrated that certain AMPs, when combined with conventional antibiotics, have a synergistic inhibitory effect against human pathogens. One example is the use of colistin in combination with azithromycin, erythromycin, and clarithromycin to treat MDR Klebsiella pneumoniae, P. aeruginosa and Acinetobacter baumannii. This combination increased membrane permeation and helped antibiotics enter the cells, thereby inhibiting the synthesis of ribosomal proteins (Ramchuran et al., 2018). Similarly, the combination of HBD3 and LL-37 with tigecycline, moxifloxacin, piperacillin / tazobactam and meropenem has been found to have synergistic effects against Clostridium difficile infections (Di Luca et al., 2014). However, not every combination of AMPs and conventional antibiotics have a synergistic antibacterial effect. Some combinations may exhibit indifference or even antagonistic effects. For instance, MDL-3 derived from housefly larvae demonstrated an antagonistic effect against Salmonella Typhimurium 50,013 when combined with penicillin or streptomycin. Consequently, more research is needed to understand the mechanisms of action for each AMP and antibiotic are different, as well as the complex relationship between the drug combination and bacteria.
One of the promising approaches for treating P. aeruginosa resistant infections is the combination therapy with two antibacterial drugs that have a synergistic effect (Giacometti et al., 1999; Mataraci Kara et al., 2020). Previous studies have shown that a considerable number of AMPs not only show anti-P. aeruginosa activities alone, but also display synergistic or additive effects in combination with drugs or compounds (Giacometti et al., 1999; Cirioni et al., 2006; Hollmann et al., 2018; Lei et al., 2019; Mataraci Kara et al., 2020). We have summarized these interactions of AMP-antibiotics combinations against P. aeruginosa in Table 2.
3.1. Microorganism-derived AMPs
Jahangiri et al. had evaluated the effects of nisin (with 3.35 kDa in size) in combination with colistin against six P. aeruginosa strains. They found that the MIC of nisin was reduced from 128 to 256 μg/mL to 16–32 μg/mL, the MIC of colistin was reduced from 0.5–8 μg/mL to 0.125–4 μg/mL, and the FICI was 0.375–0.625, displaying a synergistic antibacterial effect against four P. aeruginosa strains (Jahangiri et al., 2021). In a previous study, it was observed that nisin Z combined with antibiotics had synergistic effects on P. fluorescens isolates (n = 5). No obvious antibacterial effect was observed when nisin Z used alone, while the MIC was decreased to 0.125–25 μg/mL when combined with antibiotics. Also, the MIC of antibiotics was reduced to 0.015–125 μg/mL, and FICI values were 0.01–0.5 (Naghmouchi et al., 2012).
3.2. Human-derived AMPs
P10, a molecule with a size of 3.11 kDa, displayed antimicrobial activity against both Acinetobacter baumannii and P. aeruginosa strains with MICs of 8–32 and 8–16 μg/mL, respectively. When P10 was combined with antibiotics (ceftazidime and doripenem), it was found to have synergistic (n = 4–5) or additive (n = 1–2) activities against P. aeruginosa isolates. The MIC of P10 was reduced from 8 to 16 μg/mL to 2–8 μg/mL, the MIC of ceftazidime was reduced from 4 to 64 μg/mL to 1–32 μg/mL, the MIC of doripenem was reduced from 2 to 16 μg/mL to 0.5–4 μg/mL, respectively (Jahangiri et al., 2021). LL-37 also displayed synergistic or additive bactericidal effects on P. aeruginosa planktonic cells when used in combination with colistin, vancomycin, and polymyxin B, with FICI of ≥0.38 (Han et al., 2022). The research further explained that the LL-37-antibiotic combination worked synergistically by increasing permeability of bacterial cell membranes, allowing antibiotics to enter P. aeruginosa more easily and exerted the antibacterial effects. Another study demonstrated that LL-37-antibiotics combination also displayed synergism or addition against P. aeruginosa biofilms. The MBEC values for antibiotics and LL-37 were 80–5,120 and ≥ 640 mg/L, respectively. When combined with LL-37 at the concentration of 64 mg/L, the MBEC value of antibiotics could be significantly reduced by 8 times (Dosler and Karaaslan, 2014).
3.3. Frog and crab-derived AMPs
Magainins are a kind of AMPs that originate from the skin of African clawed frog Xenopus laevis (Zasloff, 1987; Chopra, 1993). Numerous studies have demonstrated that magainin II has inhibitory activities on gram-negative and gram-positive bacteria, fungi, and protozoa (Jacob and Zasloff, 1994; Zairi et al., 2009). Magainin II has a molecular mass of 2.48 kDa and exhibits antimicrobial activity when used alone. Results of microbroth dilution assay showed that the MIC of magainin II against E. coli D31 and P. aeruginosa was 5 and 4 μg/mL, while rifampicin had no significant antibacterial effect when used alone. Furthermore, Cirioni and his colleagues demonstrated that magainin II in combination with rifampicin exhibited synergistic effects against P. aeruginosa strains both in vitro and in vivo, with a FICI of 0.312 (Cirioni et al., 2008). It is worth mentioning that in vivo experiments showed that the combination intervention resulted in good outcomes, including reduced mortality rates, cytokines levels, and improved treatment of bacteremia.
Lys-[Trp6]hy-a1 (lys-al) was an AMP isolated from the skin secretion of the frog Hypsiboas albopunctatus, with a molecular weight of 1.87 kDa (Castro et al., 2009; da Silva et al., 2013). Lys-al had the ability to inhibit P. aeruginosa ATCC 9027 planktonic strains, with the MIC and MBC of 125 μg/mL (Carneiro et al., 2020). Moreover, when Lys-al was used in combination with ciprofloxacin, it showed synergistic effects against P. aeruginosa isolates with a FICI of 0.37, the MIC of ciprofloxacin and lys-a1 was reduced by 4–8 times. Besides, the combination of ciprofloxacin/lys-a1 also displayed an additive inhibitory effect on pre-formed biofilm of P. aeruginosa. Furthermore, it was observed that the shape of the cells had changed and the surface had become uneven after treatment with ciprofloxacin/lys-a1. Researchers speculated that cell membrane damage could lead to cytoplasm leakage or increase the possibility of antibiotic entry, ultimately resulting in synergistic antimicrobial effects.
Bessa et al. demonstrated that ocellatinPT3 (with a molecular weight of 2.53 kDa), an AMP isolated from the skin secretion of the frog Leptodactylus pustulatus, possessed inhibitory activities against planktonic cells and biofilms of P. aeruginosa (Oliveira et al., 2016; Bessa et al., 2018). When ocellain-PT3 combined with ciprofloxacin and ceftazidime, it showed synergistic effects on MDR strains, the MIC of them could be reduced 4–8 times, and the FICI was between 0.25 and 0.5. Furthermore, ocellain PT3 with a concentration over 256 μg/mL could inhibit the biofilm formation of P. aeruginosa. Ocellatin-PT3 exerted its antimicrobial effects primarily by acting on the LPS of P. aeruginosa.
Colistin is the last line of AMP antibiotic in clinical practice, and it is generally used in combination with other antibiotics to treat MDR bacterial infection (Petrosillo et al., 2008; Park et al., 2016; Almutairi, 2022; Xie et al., 2022). AMPs called Citropin 1.1 and temporin A with molecular weights of 1.62 and 1.4 kDa were secreted by the dorsal gland and submental gland of Litoria citropa and the skin of European red frog Rana temporaria, respectively (Simonetti et al., 2008; Ghiselli et al., 2011). Previous research by Jorge et al. demonstrated that colistin-citropin 1.1 combination and colistin-temporin A combination both had synergistic and additive inhibitory effects against P. aeruginosa planktonic cells, with the FICI range from 0.26 to 0.75 (Jorge et al., 2017). Similar synergistic and additive effects were also observed against P. aeruginosa biofilms. In addition, Pamp SJ et al. found that colistin was effective in killing cells with low metabolic activity in P. aeruginosa biofilms (Pamp et al., 2008). Ciprofloxacin and tetracycline could kill metabolically active biofilm cells, therefore, when colistin combined with these two antibiotics, a significant synergistic effect was observed, resulting in the complete eradication of almost all biofilm cells of P. aeruginosa (Pamp et al., 2008). NCL195 is a new antibiotic with little cytotoxicity, which belongs to the analog of robenidine (Ogunniyi et al., 2017). It exerted obvious antibacterial activity against Streptococcus pneumoniae, Staphylococcus aureus, A. baumannii, and K. pneumoniae strains by disturbing their cell membrane potential, with the MIC ranged from 0.25 to 8 μg/mL (Ogunniyi et al., 2017). Recently, Nguyen and his colleagues found that the combination of NCL195 and colistin exhibited significant synergism against P. aeruginosa (n = 18) planktonic cells with a time- and concentration-dependent manner, the MIC of NCL95 was reduced from >256 to 0.5–4 μg/mL, the MIC of colistin was reduced from 0.25–2 to 0.03–1 μg/mL, and the FICI index was 0.12–0.5 (Nguyen et al., 2021). In addition, the researchers also observed that the combination of NCL195-colistin caused more cell membrane damage to the cell membrane compared to colistin alone, which was considered as the synergistic antibacterial mechanism.
Tachyplesin I was originated from the blood cells of Tachypleus tridentatus, it had a molecular weight of 2.27 kDa and possessed extensive bactericidal abilities (Xie et al., 2016). It is found that tachyplesin I in combination with colistin had the synergistic and additive inhibitory activities against P. aeruginosa planktonic cells, the MICs of them were reduced by 4–8 times and 2–8 times, respectively. Similarly, they also exhibited synergism and addition against biofilms (Jorge et al., 2017). Another AMP called sphistin, with the molecular mass of 3.96 kDa, was derived from the mud crab Scylla paramamosain. It showed potent synergistic activity when used in combination with azithromycin and rifampicin against P. aeruginosa planktonic cells, the MICs of antibiotics were reduced from 180 to 18 and 2.5 to 0.625 μg/mL, respectively (Chen et al., 2015; Liu et al., 2020). The MIC of AMP was decreased from 24 to 1.5–6 μmol/L, and the FICI was below 0.35 (Liu et al., 2020). When sphistin combined with the two antibiotics, the cell membrane permeability of P. aeruginosa was increased, which facilitated the uptake of antibiotics and allowed them to display antibacterial effects.
3.4. Others
AMP melittin (with 2.87 kDa in size) is the main constituent of bee (Apis mellifera) venom and has broad-spectrum antibacterial activities (Li et al., 2017). The MIC values of melittin against P. aeruginosa were ranged from 1 to 8 μg/mL, while the MIC values against Acinetobacter baumannii were even lower at 0.25–0.5 μg/mL. Many reports demonstrated that it also possessed synergistic effect in combination with some conventional antibiotics (such as vancomycin, oxacillin, and amikacin) against MDR strains (Al-Ani et al., 2015). Akbari et al. reported that melittin exerted synergism against MDR P. aeruginosa strains when combined with doripenem and ceftazidime with the FICI of 0.01–0.5 (Akbari et al., 2019). The MIC of antibiotics was reduced from 8 to 64 μg/mL to 0.12–1 μg/mL and 4–64 μg/mL to 0.5–8 μg/mL, the MIC of AMP was reduced from 1 to 8 μg/mL to 0.03–0.5 μg/mL, as well as cytotoxicity was reduced by 100-fold.
LfcinB is a 25-amino acid peptide derived from bovine lactoferricin, which possessed a broad spectrum of phycological activity (Román et al., 2019). The AMP LfcinB (20–25)4 was a short peptide with 4.84 kDa in size derived from LfcinB, which has been demonstrated to have antibacterial activity against P. aeruginosa and E. coli, with MICs of 11 μM and 5–22 μM, respectively. When the concentration of LfcinB (20–25)4 was 11 μM, it exhibited a 14% hemolytic activity. When LfcinB (20–25)4 combined with ciprofloxacin, it showed synergistic effects against P. aeruginosa (Vargas-Casanova et al., 2019). The MIC of AMP was decreased from 100 to 3.1 μg/mL, the MICs of ciprofloxacin were reduced from 0.4 to 0.02 μg/mL, and FICI was 0.09.
OH-CATH30, a peptide isolated from the king cobra with a size of 3.61 kDa, exhibited antibacterial activity with MIC values of 3.125–25 μg/mL against P. aeruginosa and MIC values of 1.56–12.5 ug/mL against E. coli (Zhao et al., 2008; Li et al., 2012). OH-CATH30 had a low hemolytic activity against human red blood cells, even at a high concentration of 400 μg/mL. It also exhibited low cytotoxicity against HaCaT cell lines, with an LD50 (half-lethal dose) above 200 μg/mL (Li et al., 2012). Moreover, studies of the interaction between the peptide and ciprofloxacin/levofloxacin demonstrated synergistic effects against four P. aeruginosa isolates, with the FICI value of 0.375–0.5 (Li et al., 2014).
Cecropin A2 is a 36-residue α-helical cationic AMP with 3.6 kDa in size derived from mosquito Aedes aegypti, and it possessed antibacterial activities (Zheng et al., 2017). The AMP exhibited little hemolytic activity and toxicity toward mammalian cells. The MICs against clinical P. aeruginosa isolates were found to be 32–64 μg/mL, while the MICs against other gram-negative bacteria ranged from 2 to 32 μg/mL. The combination of cecropin A2 and tetracycline exerted synergistic activities against the planktonic cells of eighteen P. aeruginosa strains, and the MIC of AMP and antibiotic was reduced by 8-fold, with a FICI value of 0.25. Furthermore, the intervention of Cecropin A2-tetracycline combination also showed synergistic protection in G. mellonella models in vivo experiments. The researchers found that Cecropin A2 could bind to the LPS of P. aeruginosa, causing membrane permeation and interacting with bacterial DNA, thereby facilitating the transfer of tetracycline to the cytoplasm. In addition, an earlier study proved that the combination of cecropin A (with the molecular mass of 3.88 kDa) and rifampicin also had synergistic inhibitory activity against P. aeruginosa strains, with the FICI index of 0.312 < 0.5 (Cirioni et al., 2008).
A study found that CAMA, a cecropin (1–7)-melittin A (2–9) amide with the molecular weight of 1.77 kDa, exerted a strong synergism when used in combination with ciprofloxacin against preformed biofilms of P. aeruginosa (n = 4) (Dosler and Karaaslan, 2014). After treatment with this combination, MBEC values of antibiotic and CAMA were reduced 8 times and 10 times, respectively.
The existing AMPs can be designed in a more thoughtful way to enhance chemical and physical properties, leading to improved effectiveness. Studies have shown that certain synthetic or semi-synthetic AMPs exhibited more significant synergistic activities against P. aeruginosa planktonic cells and biofilms when used in combination with traditional antibiotics (Martinez et al., 1861; Rudilla et al., 2016; Maisetta et al., 2017; Al Tall et al., 2019; Yasir et al., 2020). For example, the combination of AMP 38 (a novel synthetic cyclodipeptide analog of polymyxin) with imipenem had synergistic activities against imipenem-resistant P. aeruginosa in vitro, with a FICI of 0.07–0.18 (Rudilla et al., 2016). The AMP-antibiotic combination also exhibited a synergistic effect against biofilms, the MBEC of them were reduced from ≥500 to 62.5 μg/mL, and the FICI was 0.25. Cytotoxicity tests showed that mice administered 100 and 200 mg/kg AMP 38 survived without signs of toxicity, and it had an LD50 value of 283 mg/kg. The synergistic antibacterial mechanism was similar to the above-mentioned mechanisms, AMP 38 destroyed cell membrane, and facilitated the entry of imipenem, which subsequently exerted its antibacterial effect at low concentrations. The synthesis of AMPs provides new insights for fighting against MDR strains, since an unlimited number of new compounds with antibacterial effects can be designed.
Based on the above studies, we found that most of the AMPs showed significant synergistic anti-P. aeruginosa effects in vitro when used in combination with antibacterial drugs. The combination therapy reduced the effective concentration of antibiotics, expanded the range of action of antibiotics, and improved the efficacy of antibiotics, suggesting the feasibility of drug combination strategy. Studies have shown that the synergistic antimicrobial mechanisms of AMP in combination with conventional antibiotics mainly include the following four mechanisms: (1) AMP could inhibit membrane ion channels, prevented the pumping out of antibiotics, or inhibited antibiotic-degrading enzymes’ activities (Dey et al., 2021); (2) AMP could load antibiotics and promote the uptake of antibiotics by bacteria to broaden the antibiotic’s antimicrobial spectrum; (3) AMP could interact with microbial membranes to increase the permeability, which in turn allowed more antibiotics access to the interior of the bacteria; (4) AMP could enhance the anti-biofilm activity of antibiotics by interfering with signaling pathways involved in biofilm formation and maintenance (de la Fuente-Núñez et al., 2014, 2015). In this article, we have summarized the synergistic anti-P. aeruginosa mechanisms of LL-37, lys-al, Ocellatin-PT3, NCL195, sphistin, Cecropin A2 and AMP 38, they could increase the cell membrane permeability by disrupting the cell membrane or binding to LPS, which increased the opportunity of antibiotics into bacterial interior. However, most of the current studies on AMPs-antibiotic synergistic antimicrobials did not have enough sufficient clinical data, therefore, in-depth studies on AMPs combined with antimicrobials against P. aeruginosa need to be carried out in the future.
4. Clinical studies on some AMPs
With the increase in research, some AMPs had entered clinical trials, while most required more detailed and validated studies to learn about their antimicrobial activity and mechanism of action. To date, at least 20 AMPs had entered clinical trials (Mercer and O’Neil, 2013).
For example, Pexiganan, which possessed potent antibacterial activities in vitro, has passed two phase III clinical trials, demonstrated its efficacy, safety, and effectiveness against infected diabetic foot ulcers (Lipsky et al., 2008). In addition, Omiganan exhibited rapid and potent antibacterial activity by disrupting the bacterial plasma membrane. Currently, a 1% gel product of Omiganan (Omigard) was in a phase III trial to demonstrate its efficacy in the prevention of catheter-related infections. Besides, Omiganan had also been used in the treatment of rosacea and has successfully completed a phase II trial (Fritsche et al., 2008; Kang et al., 2014). Brilacidin had potent bactericidal activity against both resistant gram-positive and gram-negative bacteria. In 2014, a phase II trial enrolled 215 patients with acute bacterial skin and skin structure infections caused by MRSA and found that the therapeutic efficacy of Brilacidin administered intravenously was comparable to daptomycin (Méndez-Samperio, 2014). POL7080, a mimic of the antimicrobial peptide Protegrin-1, had anti-MDR P. aeruginosa effects both in vitro and in vivo. Currently, POL7080 had successfully completed Phase I clinical trial for the treatment of P. aeruginosa infections, demonstrating the safety and good tolerability (Tillotson and Theriault, 2013; Romano et al., 2019). There are also many AMPs that have passed Phase I/II clinical trials, but failed to obtain satisfactory results in Phase III trials, such as Iseganan (Giles et al., 2004; Trotti et al., 2004; Kollef et al., 2006; Elad et al., 2012; Mercer and O’Neil, 2013). Moreover, several AMPs, such as Plectasin NZ2114 and MU1140, had exhibited remarkable therapeutic efficacy in preclinical researches, and they held great promise for further clinical development (Marr et al., 2006; Ghobrial et al., 2009; Breidenstein et al., 2015).
5. Discussion
In this review, we summarized the anti-P. aeruginosa activities of AMPs used alone and interactions with some conventional antibiotics. In vitro studies have shown that certain AMPs display obvious inhibitory effects against P. aeruginosa planktonic cells with MICs ≤10 μg/mL, AMPs exerted their antimicrobial effects mainly by disrupting bacterial cell membranes or interacting with DNA. Moreover, a considerable number of AMPs-antibiotics combination have significant synergistic inhibitory effects against P. aeruginosa planktonic cells, as well as the biofilm formation. In vivo studies, some combinations have also increased the survival rate and reduced the extent of infection among infected animal models. The AMPs-antibiotics synergistic combination not only helped to reduce the individual concentration and broaden the antibacterial spectrum, but also decreased drug resistance, toxicity, and other side effects. Most of the synergistic mechanisms of action were that AMPs could increase cell membrane permeability, making it easier for antibiotics to enter bacterial interior, and then exerted their antimicrobial effects. Although the synergistic antibacterial effects of AMP in combination with antibiotics had been demonstrated in vitro, the validation of their clinical synergistic activity has been rarely studied due to its limitations and different pharmacological properties from antibiotics. In addition, it is worth noting that some AMPs have been found to exert their antimicrobial effects through a combination of membrane-targeted and non-membrane-targeted modalities. For instance, arenicin-3 is a novel AMP that not only binds to the outer and cellular membranes of bacteria and disrupts their integrity, but also transfers to the cytoplasm to inhibit protein synthesis, ultimately exerting significant anti-gram-negative effects (Ciumac et al., 2019). Therefore, the cell membrane is no longer the only target of AMPs against pathogens. AMPs can act on the cell wall or intracellular nucleic acids, proteins, enzymes, or organelles to inhibit crucial intracellular processes, then leading to metabolic inhibition of the pathogens and causing bacterial death. Further research on the intracellular target of AMPs is of great significance in preventing the development of resistance in pathogens.
Although AMPs have potential therapeutic benefits compared with traditional antibiotics, they also have certain limitations, which hinder the clinical development and application. AMPs extracted naturally have low purity and quantity, as well as poor absorption, distribution, metabolism, excretion characteristics, low permeability and solubility (Di, 2015). A great number of researchers found that some properties of AMPs can be improved by changing peptide composition, making post-translational modification, recombining AMPs, and using computer-assisted discovery and design, which were meaningful to translate AMPs into useful clinical candidates for development (Irazazabal et al., 1858; Matsuzaki, 2009; Deo et al., 2022). Researchers usually use a variety of methods to design efficient, low-toxicity, and stable AMPs. These methods include: (1) replacement of amino acids in the peptide sequence with non-natural α-amino acid derivatives, such as D-amino acids, hexafluoroleucine, and α-amino-guanidinopropionic acid; (2) N-terminal acetylation or C-terminal amidation; (3) dimerization and disulfide bond cyclization; (4) optimization of physicochemical properties. Focusing on these studies will provide valuable insights into the application of AMPs in complex disease and lay a solid foundation for innovative drug development.
6. Conclusion
Natural AMPs have anti-P. aeruginosa and E. coli activity with low MIC values when used alone, and they act primarily by interacting with cell membranes or targeting organelles within the cell. Membrane-targeted antimicrobial mechanisms include four models. In this review, the antimicrobial mechanisms of Ocellatin-PT3 and Cecropin A2 are associated with LPS binding; the antimicrobial mechanisms of ACD and defensin-d2 are associated with an increase in the level of ROS. And the profound antimicrobial mechanisms of many other AMPs still need to be further discussed. In addition, based on the study of the antibacterial effect of natural AMPs alone or in combination with antibiotics against P. aeruginosa in vitro, it is still necessary to explore the synergistic antibacterial mechanism further or continue to develop AMPs structural analogs. Besides, it is imperative to further ensure the efficacy and safety of combined treatment in vivo animal models, which will be of great significance against clinical P. aeruginosa infection.
Author contributions
XC collected and analyzed the data of the review, and wrote the whole review and created Tables 1, 2. SS, YY, and LY helped with it. LH contributed to the writing to this article. All authors have reviewed and approved the manuscript. All authors contributed to the article and approved the submitted version.
Acknowledgments
Thanks for the department of pharmacy, China Japan Friendship Hospital.
Conflict of interest
The authors declare that the research was conducted in the absence of any commercial or financial relationships that could be construed as a potential conflict of interest.
Publisher’s note
All claims expressed in this article are solely those of the authors and do not necessarily represent those of their affiliated organizations, or those of the publisher, the editors and the reviewers. Any product that may be evaluated in this article, or claim that may be made by its manufacturer, is not guaranteed or endorsed by the publisher.
References
Abushaheen, M. A., Muzaheed, A. J., Fatani, M., Alosaimi, W., Mansy, M., George, S., et al. (2020). Antimicrobial resistance, mechanisms and its clinical significance. Disease-a-Month 66:100971. doi: 10.1016/j.disamonth.2020.100971.
Akbari, R., Hakemi-Vala, M., Pashaie, F., Bevalian, P., Hashemi, A., and Pooshang Bagheri, K. (2019). Highly synergistic effects of melittin with conventional antibiotics against multidrug-resistant isolates of Acinetobacter baumannii and Pseudomonas aeruginosa. Microb. Drug Resist. 25, 193–202. doi: 10.1089/mdr.2018.0016
Al Akeel, R., Mateen, A., and Syed, R. (2019). An alanine-rich peptide attenuates quorum sensing-regulated virulence and biofilm formation in Staphylococcus aureus. J. AOAC Int. 102, 1228–1234. doi: 10.5740/jaoacint.18-0251
Al Tall, Y., Abualhaijaa, A., Qaoud, M. T., Alsaggar, M., Masadeh, M., and Alzoubi, K. H. (2019). The ultrashort peptide OW: a new antibiotic adjuvant. Curr. Pharm. Biotechnol. 20, 745–754. doi: 10.2174/1389201020666190618111252
Al-Ani, I., Zimmermann, S., Reichling, J., and Wink, M. (2015). Pharmacological synergism of bee venom and melittin with antibiotics and plant secondary metabolites against multi-drug resistant microbial pathogens. Phytomed. Int. J. Phytother. Phytopharmacol. 22, 245–255. doi: 10.1016/j.phymed.2014.11.019
Almutairi, M. M. (2022). Synergistic activities of colistin combined with other antimicrobial agents against colistin-resistant Acinetobacter baumannii clinical isolates. PLoS One 17:e0270908. doi: 10.1371/journal.pone.0270908
Andrä, J., Berninghausen, O., and Leippe, M. (2001). Cecropins, antibacterial peptides from insects and mammals, are potently fungicidal against Candida albicans. Med. Microbiol. Immunol. 189, 169–173. doi: 10.1007/s430-001-8025-x
Barksdale, S. M., Hrifko, E. J., Chung, E. M., and van Hoek, M. L. (2016). Peptides from American alligator plasma are antimicrobial against multi-drug resistant bacterial pathogens including Acinetobacter baumannii. BMC Microbiol. 16:189.
Bellm, L., Lehrer, R. I., and Ganz, T. (2000). Protegrins: new antibiotics of mammalian origin. Expert Opin. Investig. Drugs 9, 1731–1742.
Bergaoui, I., Zairi, A., Tangy, F., Aouni, M., Selmi, B., and Hani, K. (2013). In vitro antiviral activity of dermaseptin S(4) and derivatives from amphibian skin against herpes simplex virus type 2. J. Med. Virol. 85, 272–281. doi: 10.1002/jmv.23450
Bessa, L. J., Eaton, P., Dematei, A., Plácido, A., Vale, N., Gomes, P., et al. (2018). Synergistic and antibiofilm properties of ocellatin peptides against multidrug-resistant Pseudomonas aeruginosa. Future Microbiol. 13, 151–163. doi: 10.2217/fmb-2017-0175
Bin Hafeez, A., Jiang, X., Bergen, P. J., and Zhu, Y. (2021). Antimicrobial peptides: an update on classifications and databases. Int. J. Mol. Sci. 22. doi: 10.3390/ijms222111691
Bishop, B. M., Juba, M. L., Devine, M. C., Barksdale, S. M., Rodriguez, C. A., Chung, M. C., et al. (2015). Bioprospecting the American alligator (Alligator mississippiensis) host defense peptidome. PLoS One 10:e0117394. doi: 10.1371/journal.pone.0117394
Boparai, J. K., and Sharma, P. K. (2020a). Mini review on antimicrobial peptides, sources, mechanism and recent applications. Protein Pept. Lett. 27, 4–16. doi: 10.2174/18755305MTAwENDE80
Boparai, J. K., and Sharma, P. K. (2020b). Mini review on antimicrobial peptides, sources, mechanism and recent applications. Protein Pept. Lett. 27, 4–16. doi: 10.2174/18755305MTAwENDE80
Bosso, M., Ständker, L., Kirchhoff, F., and Münch, J. (2018). Exploiting the human peptidome for novel antimicrobial and anticancer agents. Bioorg. Med. Chem. 26, 2719–2726. doi: 10.1016/j.bmc.2017.10.038
Breidenstein, E. B., Courvalin, P., and Meziane-Cherif, D. (2015). Antimicrobial activity of Plectasin NZ2114 in combination with Cell Wall targeting antibiotics against VanA-type Enterococcus faecalis. Microb. Drug Resist. 21, 373–379. doi: 10.1089/mdr.2014.0221
Buda De Cesare, G., Cristy, S. A., Garsin, D. A., and Lorenz, M. C. (2020). Antimicrobial peptides: a new frontier in antifungal therapy. MBio 11. doi: 10.1128/mBio.02123-20
Burrows, L. L. (2018). The therapeutic pipeline for Pseudomonas aeruginosa infections. ACS Infect. Dis. 4, 1041–1047. doi: 10.1021/acsinfecdis.8b00112
Burton, M. F., and Steel, P. G. (2009). The chemistry and biology of LL-37. Nat. Prod. Rep. 26, 1572–1584. doi: 10.1039/b912533g
Cabrera, M. P., Baldissera, G., Silva-Gonçalves Lda, C., Souza, B. M., Riske, K. A., Palma, M. S., et al. (2014). Combining experimental evidence and molecular dynamic simulations to understand the mechanism of action of the antimicrobial octapeptide jelleine-I. Biochemistry 53, 4857–4868. doi: 10.1021/bi5003585
Carneiro, V. A., de Oliveira, S. T., Silva, R. L., de Sousa Duarte, H., Silva, M. L., Matos, M. N. C., et al. (2020). Antimicrobial and antibiofilm activity of Lys-[Trp6]hy-a1 combined with ciprofloxacin against gram-negative Bacteria. Protein Pept. Lett. 27, 1124–1131. doi: 10.2174/0929866527666200416145549
Castro, M. S., Ferreira, T. C., Cilli, E. M., Crusca, E., Mendes-Giannini, M. J., Sebben, A., et al. (2009). Hylin a1, the first cytolytic peptide isolated from the arboreal south American frog Hypsiboas albopunctatus (“spotted treefrog”). Peptides 30, 291–296. doi: 10.1016/j.peptides.2008.11.003
Chen, B., Fan, D. Q., Zhu, K. X., Shan, Z. G., Chen, F. Y., Hou, L., et al. (2015). Mechanism study on a new antimicrobial peptide Sphistin derived from the N-terminus of crab histone H2A identified in haemolymphs of Scylla paramamosain. Fish Shellfish Immunol. 47, 833–846. doi: 10.1016/j.fsi.2015.10.010
Cho, J., Hwang, I. S., Choi, H., Hwang, J. H., Hwang, J. S., and Lee, D. G. (2012). The novel biological action of antimicrobial peptides via apoptosis induction. J. Microbiol. Biotechnol. 22, 1457–1466. doi: 10.4014/jmb.1205.05041
Chopra, I. (1993). The magainins: antimicrobial peptides with potential for topical application. J. Antimicrob. Chemother. 32, 351–353. doi: 10.1093/jac/32.3.351
Cirioni, O., Giacometti, A., Ghiselli, R., Kamysz, W., Orlando, F., Mocchegiani, F., et al. (2006). Citropin 1.1-treated central venous catheters improve the efficacy of hydrophobic antibiotics in the treatment of experimental staphylococcal catheter-related infection. Peptides 27, 1210–1216. doi: 10.1016/j.peptides.2005.10.007
Cirioni, O., Silvestri, C., Ghiselli, R., Orlando, F., Riva, A., Mocchegiani, F., et al. (2008). Protective effects of the combination of alpha-helical antimicrobial peptides and rifampicin in three rat models of Pseudomonas aeruginosa infection. J. Antimicrob. Chemother. 62, 1332–1338. doi: 10.1093/jac/dkn393
Ciumac, D., Gong, H., Hu, X., and Lu, J. R. (2019). Membrane targeting cationic antimicrobial peptides. J. Colloid Interface Sci. 537, 163–185. doi: 10.1016/j.jcis.2018.10.103
da Silva, B. R., de Freitas, V. A., Carneiro, V. A., Arruda, F. V., Lorenzón, E. N., de Aguiar, A. S., et al. (2013). Antimicrobial activity of the synthetic peptide Lys-a1 against oral streptococci. Peptides 42, 78–83. doi: 10.1016/j.peptides.2012.12.001
Dagan, A., Efron, L., Gaidukov, L., Mor, A., and Ginsburg, H. (2002). In vitro antiplasmodium effects of dermaseptin S4 derivatives. Antimicrob. Agents Chemother. 46, 1059–1066. doi: 10.1128/AAC.46.4.1059-1066.2002
Darville, L. N., Merchant, M. E., Hasan, A., and Murray, K. K. (2010). Proteome analysis of the leukocytes from the American alligator (Alligator mississippiensis) using mass spectrometry. Comp. Biochem. Physiol. Part D Genomics Proteomics 5, 308–316. doi: 10.1016/j.cbd.2010.09.001
de la Fuente-Núñez, C., Reffuveille, F., Haney, E. F., Straus, S. K., and Hancock, R. E. (2014). Broad-spectrum anti-biofilm peptide that targets a cellular stress response. PLoS Pathog. 10:e1004152. doi: 10.1371/journal.ppat.1004152
de La Fuente-Núñez, C., Reffuveille, F., Mansour, S. C., Reckseidler-Zenteno, S. L., Hernández, D., Brackman, G., et al. (2015). D-enantiomeric peptides that eradicate wild-type and multidrug-resistant biofilms and protect against lethal Pseudomonas aeruginosa infections. Chem. Biol. 22, 196–205. doi: 10.1016/j.chembiol.2015.01.002
Deo, S., Turton, K. L., Kainth, T., Kumar, A., and Wieden, H. J. (2022). Strategies for improving antimicrobial peptide production. Biotechnol. Adv. 59:107968. doi: 10.1016/j.biotechadv.2022.107968
Dey, R., Mukherjee, S., Barman, S., and Haldar, J. (2021). Macromolecular nanotherapeutics and antibiotic adjuvants to tackle bacterial and fungal infections. Macromol. Biosci. 21:e2100182. doi: 10.1002/mabi.202100182
Di, L. (2015). Strategic approaches to optimizing peptide ADME properties. AAPS J. 17, 134–143. doi: 10.1208/s12248-014-9687-3
Di Luca, M., Maccari, G., and Nifosì, R. (2014). Treatment of microbial biofilms in the post-antibiotic era: prophylactic and therapeutic use of antimicrobial peptides and their design by bioinformatics tools. Pathog. Dis. 70, 257–270. doi: 10.1111/2049-632X.12151
Dosler, S., and Karaaslan, E. (2014). Inhibition and destruction of Pseudomonas aeruginosa biofilms by antibiotics and antimicrobial peptides. Peptides 62, 32–37. doi: 10.1016/j.peptides.2014.09.021
Efron, L., Dagan, A., Gaidukov, L., Ginsburg, H., and Mor, A. (2002). Direct interaction of dermaseptin S4 aminoheptanoyl derivative with intraerythrocytic malaria parasite leading to increased specific antiparasitic activity in culture. J. Biol. Chem. 277, 24067–24072. doi: 10.1074/jbc.M202089200
Elad, S., Epstein, J. B., Raber-Durlacher, J., Donnelly, P., and Strahilevitz, J. (2012). The antimicrobial effect of Iseganan HCl oral solution in patients receiving stomatotoxic chemotherapy: analysis from a multicenter, double-blind, placebo-controlled, randomized, phase III clinical trial. J. Oral Pathol. Med. 41, 229–234. doi: 10.1111/j.1600-0714.2011.01094.x
Elias, P. M. (2005). Stratum corneum defensive functions: an integrated view. J. Invest. Dermatol. 125, 183–200. doi: 10.1111/j.0022-202X.2005.23668.x
Fabisiak, A., Murawska, N., and Fichna, J. (2016). LL-37: cathelicidin-related antimicrobial peptide with pleiotropic activity. Pharmacol. Rep. 68, 802–808. doi: 10.1016/j.pharep.2016.03.015
Fontana, R., Mendes, M. A., de Souza, B. M., Konno, K., César, L. M., Malaspina, O., et al. (2004). Jelleines: a family of antimicrobial peptides from the Royal Jelly of honeybees (Apis mellifera). Peptides 25, 919–928. doi: 10.1016/j.peptides.2004.03.016
Fritsche, T. R., Rhomberg, P. R., Sader, H. S., and Jones, R. N. (2008). Antimicrobial activity of omiganan pentahydrochloride tested against contemporary bacterial pathogens commonly responsible for catheter-associated infections. J. Antimicrob. Chemother. 61, 1092–1098. doi: 10.1093/jac/dkn074
Fujiwara, S., Imai, J., Fujiwara, M., Yaeshima, T., Kawashima, T., and Kobayashi, K. (1990). A potent antibacterial protein in royal jelly. Purification and determination of the primary structure of royalisin. J. Biol. Chem. 265, 11333–11337. doi: 10.1016/S0021-9258(19)38596-5
Gallo, R. L., and Hooper, L. V. (2012). Epithelial antimicrobial defence of the skin and intestine. Nat. Rev. Immunol. 12, 503–516. doi: 10.1038/nri3228
Gbala, I. D., Macharia, R. W., Bargul, J. L., and Magoma, G. (2022). Membrane permeabilization and antimicrobial activity of recombinant defensin-d2 and Actifensin against multidrug-resistant Pseudomonas aeruginosa and Candida albicans. Molecules 27:4325. doi: 10.3390/molecules27144325
Ghiselli, R., Silvestri, C., Cirioni, O., Kamysz, W., Orlando, F., Calcinari, A., et al. (2011). Protective effect of citropin 1.1 and tazobactam-piperacillin against oxidative damage and lethality in mice models of gram-negative sepsis. J. Surg. Res. 171, 726–733. doi: 10.1016/j.jss.2010.03.055
Ghobrial, O. G., Derendorf, H., and Hillman, J. D. (2009). Pharmacodynamic activity of the lantibiotic MU1140. Int. J. Antimicrob. Agents 33, 70–74. doi: 10.1016/j.ijantimicag.2008.07.028
Giacometti, A., Cirioni, O., Barchiesi, F., Fortuna, M., and Scalise, G. (1999). In-vitro activity of cationic peptides alone and in combination with clinically used antimicrobial agents against Pseudomonas aeruginosa. J. Antimicrob. Chemother. 44, 641–645. doi: 10.1093/jac/44.5.641
Giles, F. J., Rodriguez, R., Weisdorf, D., Wingard, J. R., Martin, P. J., Fleming, T. R., et al. (2004). A phase III, randomized, double-blind, placebo-controlled, study of iseganan for the reduction of stomatitis in patients receiving stomatotoxic chemotherapy. Leuk. Res. 28, 559–565. doi: 10.1016/j.leukres.2003.10.021
Han, W., Wei, Z., and Camesano, T. A. (2022). New antimicrobial peptide-antibiotic combination strategy for Pseudomonas aeruginosa inactivation. Biointerphases 17:041002. doi: 10.1116/6.0001981
Hancock, R. E., Nijnik, A., and Philpott, D. J. (2012). Modulating immunity as a therapy for bacterial infections. Nat. Rev. Microbiol. 10, 243–254. doi: 10.1038/nrmicro2745
Hancock, R. E., and Speert, D. P. (2000). Antibiotic resistance in Pseudomonas aeruginosa: mechanisms and impact on treatment. Drug Resist. Updat. 3, 247–255. doi: 10.1054/drup.2000.0152
Hansmann, B., Schröder, J. M., and Gerstel, U. (2015). Skin-derived C-terminal Filaggrin-2 fragments are Pseudomonas aeruginosa-directed antimicrobials targeting bacterial replication. PLoS Pathog. 11:e1005159. doi: 10.1371/journal.ppat.1005159
Harder, J., Schröder, J. M., and Gläser, R. (2013). The skin surface as antimicrobial barrier: present concepts and future outlooks. Exp. Dermatol. 22, 1–5. doi: 10.1111/exd.12046
Hollmann, A., Martinez, M., Maturana, P., Semorile, L. C., and Maffia, P. C. (2018). Antimicrobial peptides: interaction with model and biological membranes and synergism with chemical antibiotics. Front. Chem. 6:204.
Huan, Y., Kong, Q., Mou, H., and Yi, H. (2020). Antimicrobial peptides: classification, design, application and research Progress in multiple fields. Front. Microbiol. 11:582779. doi: 10.3389/fmicb.2020.582779
Irazazabal, L. N., Porto, W. F., Ribeiro, S. M., Casale, S., Humblot, V., Ladram, A., et al. (1858). Selective amino acid substitution reduces cytotoxicity of the antimicrobial peptide mastoparan. Biochim. Biophys. Acta 2016, 2699–2708.
Islas-Rodrìguez, A. E., Marcellini, L., Orioni, B., Barra, D., Stella, L., and Mangoni, M. L. (2009). Esculentin 1-21: a linear antimicrobial peptide from frog skin with inhibitory effect on bovine mastitis-causing bacteria. J. Peptid. Sci. Off. Publ. Euro. Pept. Soc. 15, 607–614. doi: 10.1002/psc.1148
Jacob, L., and Zasloff, M. (1994). Potential therapeutic applications of magainins and other antimicrobial agents of animal origin. Ciba Found. Symp. 186, 197–216.
Jahangiri, A., Neshani, A., Mirhosseini, S. A., Ghazvini, K., Zare, H., and Sedighian, H. (2021). Synergistic effect of two antimicrobial peptides, nisin and P10 with conventional antibiotics against extensively drug-resistant Acinetobacter baumannii and colistin-resistant Pseudomonas aeruginosa isolates. Microb. Pathog. 150:104700. doi: 10.1016/j.micpath.2020.104700
Jangra, V., Sharma, N., and Chhillar, A. K. (2022). Therapeutic approaches for combating Pseudomonas aeruginosa infections. Microbes Infect. 24:104950. doi: 10.1016/j.micinf.2022.104950
Jorge, P., Grzywacz, D., Kamysz, W., Lourenço, A., and Pereira, M. O. (2017). Searching for new strategies against biofilm infections: colistin-AMP combinations against Pseudomonas aeruginosa and Staphylococcus aureus single- and double-species biofilms. PLoS One 12:e0174654. doi: 10.1371/journal.pone.0174654
Kang, S. J., Park, S. J., Mishig-Ochir, T., and Lee, B. J. (2014). Antimicrobial peptides: therapeutic potentials. Expert Rev. Anti-Infect. Ther. 12, 1477–1486. doi: 10.1586/14787210.2014.976613
Kollef, M., Pittet, D., Sánchez García, M., Chastre, J., Fagon, J. Y., Bonten, M., et al. (2006). A randomized double-blind trial of iseganan in prevention of ventilator-associated pneumonia. Am. J. Respir. Crit. Care Med. 173, 91–97. doi: 10.1164/rccm.200504-656OC
Lachowicz, J. I., Szczepski, K., Scano, A., Casu, C., Fais, S., Orrù, G., et al. (2020). The best peptidomimetic strategies to undercover antibacterial peptides. Int. J. Mol. Sci. 21. doi: 10.3390/ijms21197349
Lee, C. C., Sun, Y., Qian, S., and Huang, H. W. (2011). Transmembrane pores formed by human antimicrobial peptide LL-37. Biophys. J. 100, 1688–1696. doi: 10.1016/j.bpj.2011.02.018
Lei, J., Sun, L., Huang, S., Zhu, C., Li, P., He, J., et al. (2019). The antimicrobial peptides and their potential clinical applications. Am. J. Transl. Res. 11, 3919–3931.
Li, J., Koh, J. J., Liu, S., Lakshminarayanan, R., Verma, C. S., and Beuerman, R. W. (2017). Membrane active antimicrobial peptides: translating mechanistic insights to design. Front. Neurosci. 11:73. doi: 10.3389/fnins.2017.00073
Li, S. A., Lee, W. H., and Zhang, Y. (2012). Efficacy of OH-CATH30 and its analogs against drug-resistant bacteria in vitro and in mouse models. Antimicrob. Agents Chemother. 56, 3309–3317. doi: 10.1128/AAC.06304-11
Li, S. A., Liu, J., Xiang, Y., Wang, Y. J., Lee, W. H., and Zhang, Y. (2014). Therapeutic potential of the antimicrobial peptide OH-CATH30 for antibiotic-resistant Pseudomonas aeruginosa keratitis. Antimicrob. Agents Chemother. 58, 3144–3150. doi: 10.1128/AAC.00095-14
Li, J. F., Zhang, J. X., Li, G., Xu, Y. Y., Lu, K., Wang, Z. G., et al. (2020). Antimicrobial activity and mechanism of peptide CM4 against Pseudomonas aeruginosa. Food Funct. 11, 7245–7254. doi: 10.1039/D0FO01031F
Libardo, M. D. J., Bahar, A. A., Ma, B., Fu, R., McCormick, L. E., Zhao, J., et al. (2017). Nuclease activity gives an edge to host-defense peptide piscidin 3 over piscidin 1, rendering it more effective against persisters and biofilms. FEBS J. 284, 3662–3683. doi: 10.1111/febs.14263
Lin, Y., Lin, T., Cheng, N., Wu, S., Huang, J., Chen, X., et al. (2021). Evaluation of antimicrobial and anticancer activities of three peptides identified from the skin secretion of Hylarana latouchii. Acta Biochim. Biophys. Sin. 53, 1469–1483. doi: 10.1093/abbs/gmab126
Lipsky, B. A., Holroyd, K. J., and Zasloff, M. (2008). Topical versus systemic antimicrobial therapy for treating mildly infected diabetic foot ulcers: a randomized, controlled, double-blinded, multicenter trial of pexiganan cream. Clin. Infect. Dis. 47, 1537–1545. doi: 10.1086/593185
Liu, J., Chen, F., Wang, X., Peng, H., Zhang, H., and Wang, K. J. (2020). The synergistic effect of Mud crab antimicrobial peptides Sphistin and Sph(12-38) with antibiotics azithromycin and rifampicin enhances bactericidal activity against Pseudomonas Aeruginosa. Front. Cell. Infect. Microbiol. 10:572849. doi: 10.3389/fcimb.2020.572849
Luca, V., Stringaro, A., Colone, M., Pini, A., and Mangoni, M. L. (2013). Esculentin(1-21), an amphibian skin membrane-active peptide with potent activity on both planktonic and biofilm cells of the bacterial pathogen Pseudomonas aeruginosa. Cell. Mol. Life Sci. 70, 2773–2786. doi: 10.1007/s00018-013-1291-7
Luo, Y., and Song, Y. (2021). Mechanism of antimicrobial peptides: antimicrobial, anti-inflammatory and antibiofilm activities. Int. J. Mol. Sci. 22. doi: 10.3390/ijms222111401
Maisetta, G., Grassi, L., Esin, S., Serra, I., Scorciapino, M. A., Rinaldi, A. C., et al. (2017). The semi-synthetic peptide Lin-SB056-1 in combination with EDTA exerts strong antimicrobial and antibiofilm activity against Pseudomonas aeruginosa in conditions mimicking cystic fibrosis sputum. Int. J. Mol. Sci. 18. doi: 10.3390/ijms18091994
Marr, A. K., Gooderham, W. J., and Hancock, R. E. (2006). Antibacterial peptides for therapeutic use: obstacles and realistic outlook. Curr. Opin. Pharmacol. 6, 468–472. doi: 10.1016/j.coph.2006.04.006
Martinez, M., Gonçalves, S., Felício, M. R., Maturana, P., Santos, N. C., Semorile, L., et al. (1861). Synergistic and antibiofilm activity of the antimicrobial peptide P5 against carbapenem-resistant Pseudomonas aeruginosa. Biochim. Biophys. Acta Biomembr. 2019, 1329–1337.
Marynka, K., Rotem, S., Portnaya, I., Cogan, U., and Mor, A. (2007). In vitro discriminative antipseudomonal properties resulting from acyl substitution of N-terminal sequence of dermaseptin s4 derivatives. Chem. Biol. 14, 75–85. doi: 10.1016/j.chembiol.2006.11.009
Mataraci Kara, E., Yilmaz, M., İstanbullu Tosun, A., and Özbek Çelik, B. (2020). Synergistic activities of ceftazidime-avibactam in combination with different antibiotics against colistin-nonsusceptible clinical strains of Pseudomonas aeruginosa. Infect. Dis. 52, 616–624.
Matsuzaki, K. (2009). Control of cell selectivity of antimicrobial peptides. Biochim. Biophys. Acta 1788, 1687–1692. doi: 10.1016/j.bbamem.2008.09.013
McCann, J. C., Wickersham, T. A., and Loor, J. J. (2014). High-throughput methods redefine the rumen microbiome and its relationship with nutrition and metabolism. Bioinform. Biol. Insig. 8, 109–125. doi: 10.4137/BBI.S15389
Méndez-Samperio, P. (2014). Peptidomimetics as a new generation of antimicrobial agents: current progress. Infect. Drug Resist. 7, 229–237. doi: 10.2147/IDR.S49229
Mercer, D. K., and O’Neil, D. A. (2013). Peptides as the next generation of anti-infectives. Future Med. Chem. 5, 315–337. doi: 10.4155/fmc.12.213
Merchant, M. E., Leger, N., Jerkins, E., Mills, K., Pallansch, M. B., Paulman, R. L., et al. (2006). Broad spectrum antimicrobial activity of leukocyte extracts from the American alligator (Alligator mississippiensis). Vet. Immunol. Immunopathol. 110, 221–228. doi: 10.1016/j.vetimm.2005.10.001
Modiri, S., Kasra Kermanshahi, R., Soudi, M. R., Arab, S. S., Khammari, A., Cousineau, B., et al. (2020). Multifunctional Acidocin 4356 combats Pseudomonas aeruginosa through membrane perturbation and virulence attenuation: experimental results confirm molecular dynamics simulation. Appl. Environ. Microbiol. 86. doi: 10.1128/AEM.00367-20
Moore, N. M., and Flaws, M. L. (2011). Treatment strategies and recommendations for Pseudomonas aeruginosa infections. Clin. Lab. Sci. J. Am. Soc. Med. Technol. 24, 52–56. doi: 10.29074/ascls.24.1.52
Mor, A., Nguyen, V. H., Delfour, A., Migliore-Samour, D., and Nicolas, P. (1991). Isolation, amino acid sequence, and synthesis of dermaseptin, a novel antimicrobial peptide of amphibian skin. Biochemistry 30, 8824–8830. doi: 10.1021/bi00100a014
Morgavi, D. P., Kelly, W. J., Janssen, P. H., and Attwood, G. T. (2013). Rumen microbial (meta)genomics and its application to ruminant production. Anim. Int. J. Anim. Biosci. 7, 184–201.
Morroni, G., Simonetti, O., Brenciani, A., Brescini, L., Kamysz, W., Kamysz, E., et al. (2019). In vitro activity of Protegrin-1, alone and in combination with clinically useful antibiotics, against Acinetobacter baumannii strains isolated from surgical wounds. Med. Microbiol. Immunol. 208, 877–883. doi: 10.1007/s00430-019-00624-7
Morton, C. O., Dos Santos, S. C., and Coote, P. (2007). An amphibian-derived, cationic, alpha-helical antimicrobial peptide kills yeast by caspase-independent but AIF-dependent programmed cell death. Mol. Microbiol. 65, 494–507. doi: 10.1111/j.1365-2958.2007.05801.x
Mulkern, A. J., Oyama, L. B., Cookson, A. R., Creevey, C. J., Wilkinson, T. J., Olleik, H., et al. (2022). Microbiome-derived antimicrobial peptides offer therapeutic solutions for the treatment of Pseudomonas aeruginosa infections. NPJ Biofil. Microb. 8:70. doi: 10.1038/s41522-022-00332-w
Naghmouchi, K., Le Lay, C., Baah, J., and Drider, D. (2012). Antibiotic and antimicrobial peptide combinations: synergistic inhibition of Pseudomonas fluorescens and antibiotic-resistant variants. Res. Microbiol. 163, 101–108. doi: 10.1016/j.resmic.2011.11.002
Nguyen, L. T., Haney, E. F., and Vogel, H. J. (2011). The expanding scope of antimicrobial peptide structures and their modes of action. Trends Biotechnol. 29, 464–472. doi: 10.1016/j.tibtech.2011.05.001
Nguyen, H. T., Venter, H., Veltman, T., Williams, R., O’Donovan, L. A., Russell, C. C., et al. (2021). In vitro synergistic activity of NCL195 in combination with colistin against gram-negative bacterial pathogens. Int. J. Antimicrob. Agents 57:106323. doi: 10.1016/j.ijantimicag.2021.106323
Nolan, L. M., Turnbull, L., Katrib, M., Osvath, S. R., Losa, D., Lazenby, J. J., et al. (2020). Pseudomonas aeruginosa is capable of natural transformation in biofilms. Microbiology 166, 995–1003. doi: 10.1099/mic.0.000956
Ogunniyi, A. D., Khazandi, M., Stevens, A. J., Sims, S. K., Page, S. W., Garg, S., et al. (2017). Evaluation of robenidine analog NCL195 as a novel broad-spectrum antibacterial agent. PLoS One 12:e0183457. doi: 10.1371/journal.pone.0183457
Oliveira, M., Gomes-Alves, A. G., Sousa, C., Mirta Marani, M., Plácido, A., Vale, N., et al. (2016). Ocellatin-PT antimicrobial peptides: high-resolution microscopy studies in antileishmania models and interactions with mimetic membrane systems. Biopolymers 105, 873–886. doi: 10.1002/bip.22925
Overhage, J., Campisano, A., Bains, M., Torfs, E. C., Rehm, B. H., and Hancock, R. E. (2008). Human host defense peptide LL-37 prevents bacterial biofilm formation. Infect. Immun. 76, 4176–4182. doi: 10.1128/IAI.00318-08
Oyama, L. B., Girdwood, S. E., Cookson, A. R., Fernandez-Fuentes, N., Privé, F., Vallin, H. E., et al. (2017). The rumen microbiome: an underexplored resource for novel antimicrobial discovery. NPJ Biofil. Microb. 3:33.
Pamp, S. J., Gjermansen, M., Johansen, H. K., and Tolker-Nielsen, T. (2008). Tolerance to the antimicrobial peptide colistin in Pseudomonas aeruginosa biofilms is linked to metabolically active cells, and depends on the pmr and mexAB-oprM genes. Mol. Microbiol. 68, 223–240. doi: 10.1111/j.1365-2958.2008.06152.x
Parducho, K. R., Beadell, B., Ybarra, T. K., Bush, M., Escalera, E., Trejos, A. T., et al. (2020). The antimicrobial peptide human Beta-defensin 2 inhibits biofilm production of Pseudomonas aeruginosa without compromising metabolic activity. Front. Immunol. 11:805. doi: 10.3389/fimmu.2020.00805
Park, G. C., Choi, J. A., Jang, S. J., Jeong, S. H., Kim, C. M., Choi, I. S., et al. (2016). In vitro interactions of antibiotic combinations of colistin, tigecycline, and Doripenem against extensively drug-resistant and multidrug-resistant Acinetobacter baumannii. Ann. Lab. Med. 36, 124–130. doi: 10.3343/alm.2016.36.2.124
Perez-Rodriguez, A., Eraso, E., Quindós, G., and Mateo, E. (2022a). Antimicrobial peptides with anti-Candida activity. Int. J. Mol. Sci. 23:9264. doi: 10.3390/ijms23169264
Perez-Rodriguez, A., Eraso, E., Quindós, G., and Mateo, E. (2022b). Antimicrobial peptides with anti-Candida activity. Int. J. Mol. Sci. 23. doi: 10.3390/ijms23169264
Petrosillo, N., Ioannidou, E., and Falagas, M. E. (2008). Colistin monotherapy vs. combination therapy: evidence from microbiological, animal and clinical studies. Clin. Microbiol. Infect. 14, 816–827. doi: 10.1111/j.1469-0691.2008.02061.x
Pitt, S. J., Graham, M. A., Dedi, C. G., Taylor-Harris, P. M., and Gunn, A. (2015). Antimicrobial properties of mucus from the brown garden snail Helix aspersa. Br. J. Biomed. Sci. 72, 174–181; quiz 208. doi: 10.1080/09674845.2015.11665749
Pitt, S. J., Hawthorne, J. A., Garcia-Maya, M., Alexandrovich, A., Symonds, R. C., and Gunn, A. (2019). Identification and characterisation of anti - Pseudomonas aeruginosa proteins in mucus of the brown garden snail, Cornu aspersum. Br. J. Biomed. Sci. 76, 129–136. doi: 10.1080/09674845.2019.1603794
Pompilio, A., Crocetta, V., Scocchi, M., Pomponio, S., Di Vincenzo, V., Mardirossian, M., et al. (2012). Potential novel therapeutic strategies in cystic fibrosis: antimicrobial and anti-biofilm activity of natural and designed α-helical peptides against Staphylococcus aureus, Pseudomonas aeruginosa, and Stenotrophomonas maltophilia. BMC Microbiol. 12:145. doi: 10.1186/1471-2180-12-145
Ponti, D., Mignogna, G., Mangoni, M. L., De Biase, D., Simmaco, M., and Barra, D. (1999). Expression and activity of cyclic and linear analogues of esculentin-1, an anti-microbial peptide from amphibian skin. Eur. J. Biochem. 263, 921–927. doi: 10.1046/j.1432-1327.1999.00597.x
Portelinha, J., and Angeles-Boza, A. M. (2021). The antimicrobial peptide Gad-1 clears Pseudomonas aeruginosa biofilms under cystic fibrosis conditions. Chembiochem 22, 1646–1655. doi: 10.1002/cbic.202000816
Privé, F., Newbold, C. J., Kaderbhai, N. N., Girdwood, S. G., Golyshina, O. V., Golyshin, P. N., et al. (2015). Isolation and characterization of novel lipases/esterases from a bovine rumen metagenome. Appl. Microbiol. Biotechnol. 99, 5475–5485. doi: 10.1007/s00253-014-6355-6
Quilès, F., Saadi, S., Francius, G., Bacharouche, J., and Humbert, F. (1858). In situ and real time investigation of the evolution of a Pseudomonas fluorescens nascent biofilm in the presence of an antimicrobial peptide. Biochim. Biophys. Acta 2016, 75–84.
Ramchuran, E. J., Somboro, A. M., Abdel Monaim, S. A. H., Amoako, D. G., Parboosing, R., Kumalo, H. M., et al. In Vitro antibacterial activity of Teixobactin derivatives on clinically relevant bacterial isolates Front Microbiol. 9 (2018) 1535.
Román, J. T., Fuenmayor, C. A., Zuluaga Dominguez, C. M., Clavijo-Grimaldo, D., Acosta, M., García-Castañeda, J. E., et al. (2019). Pullulan nanofibers containing the antimicrobial palindromic peptide LfcinB (21-25)(pal) obtained via electrospinning. RSC Adv. 9, 20432–20438. doi: 10.1039/C9RA03643A
Romano, K. P., Warrier, T., Poulsen, B. E., Nguyen, P. H., Loftis, A. R., Saebi, A., et al. (2019). Mutations in pmrB confer cross-resistance between the LptD inhibitor POL7080 and colistin in Pseudomonas aeruginosa. Antimicrob. Agents Chemother. 63. doi: 10.1128/AAC.00511-19
Roque-Borda, C. A., da Silva, P. B., Rodrigues, M. C., Azevedo, R. B., Di Filippo, L., Duarte, J. L., et al. (2021). Challenge in the discovery of new drugs: antimicrobial peptides against WHO-list of critical and high-priority Bacteria. Pharmaceutics 13. doi: 10.3390/pharmaceutics13060773
Rudilla, H., Fusté, E., Cajal, Y., Rabanal, F., Vinuesa, T., and Viñas, M., Synergistic antipseudomonal effects of synthetic peptide AMP38 and carbapenems. Molecules (Basel, Switzerland) 21 (2016). doi: 10.3390/molecules21091223
Saeed, S. I., Mergani, A., Aklilu, E., and Kamaruzzman, N. F. (2022). Antimicrobial peptides: bringing solution to the rising threats of antimicrobial resistance in livestock. Front. Vet. Sci. 9:851052. doi: 10.3389/fvets.2022.851052
Salger, S. A., Cassady, K. R., Reading, B. J., and Noga, E. J. (2016). A diverse family of host-Defense peptides (Piscidins) exhibit specialized anti-bacterial and anti-protozoal activities in fishes. PLoS One 11:e0159423. doi: 10.1371/journal.pone.0159423
Sathoff, A. E., Lewenza, S., and Samac, D. A. (2020). Plant defensin antibacterial mode of action against Pseudomonas species. BMC Microbiol. 20:173.
Sathoff, A. E., Velivelli, S., Shah, D. M., and Samac, D. A. (2019). Plant defensin peptides have antifungal and antibacterial activity against human and plant pathogens. Phytopathology 109, 402–408. doi: 10.1094/PHYTO-09-18-0331-R
Schröder, J.-M., and Harder, J. (1999). Human beta-defensin-2. Int. J. Biochem. Cell Biol. 31, 645–651. doi: 10.1016/S1357-2725(99)00013-8
Segura, A., Moreno, M., Molina, A., and García-Olmedo, F. (1998). Novel defensin subfamily from spinach (Spinacia oleracea). FEBS Lett. 435, 159–162. doi: 10.1016/S0014-5793(98)01060-6
Simmaco, M., Mignogna, G., Barra, D., and Bossa, F. (1994). Antimicrobial peptides from skin secretions of Rana esculenta. Molecular cloning of cDNAs encoding esculentin and brevinins and isolation of new active peptides. J. Biol. Chem. 269, 11956–11961. doi: 10.1016/S0021-9258(17)32666-2
Simonetti, O., Cirioni, O., Goteri, G., Ghiselli, R., Kamysz, W., Kamysz, E., et al. (2008). Temporin a is effective in MRSA-infected wounds through bactericidal activity and acceleration of wound repair in a murine model. Peptides 29, 520–528. doi: 10.1016/j.peptides.2007.12.011
Skerlavaj, B., Gennaro, R., Bagella, L., Merluzzi, L., Risso, A., and Zanetti, M. (1996). Biological characterization of two novel cathelicidin-derived peptides and identification of structural requirements for their antimicrobial and cell lytic activities. J. Biol. Chem. 271, 28375–28381. doi: 10.1074/jbc.271.45.28375
Sørensen, O. E., Follin, P., Johnsen, A. H., Calafat, J., Tjabringa, G. S., Hiemstra, P. S., et al. (2001). Human cathelicidin, hCAP-18, is processed to the antimicrobial peptide LL-37 by extracellular cleavage with proteinase 3. Blood 97, 3951–3959. doi: 10.1182/blood.V97.12.3951
Su, Y., Zhang, K., and Schluesener, H. J. (2010). Antimicrobial peptides in the brain. Arch. Immunol. Ther. Exp. 58, 365–377. doi: 10.1007/s00005-010-0089-7
Sugrue, I., O’Connor, P. M., Hill, C., Stanton, C., and Ross, R. P. (2020). Actinomyces produces defensin-like bacteriocins (Actifensins) with a highly degenerate structure and broad antimicrobial activity. J. Bacteriol. 202. doi: 10.1128/JB.00529-19
Szekeres, A., Leitgeb, B., Kredics, L., Antal, Z., Hatvani, L., Manczinger, L., et al. (2005). Peptaibols and related peptaibiotics of Trichoderma. A review. Acta Microbiol. Immunol. Hung. 52, 137–168. doi: 10.1556/AMicr.52.2005.2.2
Terzi, H. A., Kulah, C., and Ciftci, I. H. (2014). The effects of active efflux pumps on antibiotic resistance in Pseudomonas aeruginosa. World J. Microbiol. Biotechnol. 30, 2681–2687. doi: 10.1007/s11274-014-1692-2
Tillotson, G. S., and Theriault, N. (2013). New and alternative approaches to tackling antibiotic resistance. F1000prime Rep. 5:51. doi: 10.12703/P5-51
Trotti, A., Garden, A., Warde, P., Symonds, P., Langer, C., Redman, R., et al. (2004). A multinational, randomized phase III trial of iseganan HCl oral solution for reducing the severity of oral mucositis in patients receiving radiotherapy for head-and-neck malignancy. Int. J. Radiat. Oncol. Biol. Phys. 58, 674–681. doi: 10.1016/S0360-3016(03)01627-4
Turner, J., Cho, Y., Dinh, N. N., Waring, A. J., and Lehrer, R. I. (1998). Activities of LL-37, a cathelin-associated antimicrobial peptide of human neutrophils. Antimicrob. Agents Chemother. 42, 2206–2214. doi: 10.1128/AAC.42.9.2206
Van Moll, L., De Smet, J., Paas, A., Tegtmeier, D., Vilcinskas, A., Cos, P., et al. (2022). In vitro evaluation of antimicrobial peptides from the black soldier Fly (Hermetia Illucens) against a selection of human pathogens. Microbiol. Spectr. 10:e0166421. doi: 10.1128/spectrum.01664-21
Vargas-Casanova, Y., Rodríguez-Mayor, A. V., Cardenas, K. J., Leal-Castro, A. L., Muñoz-Molina, L. C., Fierro-Medina, R., et al. (2019). Synergistic bactericide and antibiotic effects of dimeric, tetrameric, or palindromic peptides containing the RWQWR motif against gram-positive and gram-negative strains. RSC Adv. 9, 7239–7245. doi: 10.1039/C9RA00708C
Velivelli, S. L. S., Islam, K. T., Hobson, E., and Shah, D. M. (2018). Modes of action of a bi-domain plant defensin MtDef5 against a bacterial pathogen Xanthomonas campestris. Front. Microbiol. 9:934. doi: 10.3389/fmicb.2018.00934
Wang, G., Wang, Y., Ma, D., Liu, H., Li, J., Zhang, K., et al. (2013). Five novel antimicrobial peptides from the Kuhl’s wart frog skin secretions, Limnonectes kuhlii. Mol. Biol. Rep. 40, 1097–1102. doi: 10.1007/s11033-012-2152-4
Warnke, P. H., Voss, E., Russo, P. A., Stephens, S., Kleine, M., Terheyden, H., et al. (2013). Antimicrobial peptide coating of dental implants: biocompatibility assessment of recombinant human beta defensin-2 for human cells. Int. J. Oral Max. Impl. 28. doi: 10.11607/jomi.2594
Whitchurch, C. B., Tolker-Nielsen, T., Ragas, P. C., and Mattick, J. S. (2002). Extracellular DNA required for bacterial biofilm formation. Science 295:1487. doi: 10.1126/science.295.5559.1487
Wilton, M., Charron-Mazenod, L., Moore, R., and Lewenza, S. (2016). Extracellular DNA acidifies biofilms and induces aminoglycoside resistance in Pseudomonas aeruginosa. Antimicrob. Agents Chemother. 60, 544–553. doi: 10.1128/AAC.01650-15
Wu, Z., Hansmann, B., Meyer-Hoffert, U., Gläser, R., and Schröder, J. M. (2009). Molecular identification and expression analysis of filaggrin-2, a member of the S100 fused-type protein family. PLoS One 4:e5227. doi: 10.1371/journal.pone.0005227
Xie, M., Chen, K., Chan, E. W., and Chen, S. (2022). Synergistic antimicrobial effect of colistin in combination with econazole against multidrug-resistant Acinetobacter baumannii and its Persisters. Microbiology Spectrum 10:e0093722. doi: 10.1128/spectrum.00937-22
Xie, H., Wei, J., and Qin, Q. (2016). Antiviral function of Tachyplesin I against iridovirus and nodavirus. Fish Shellfish Immunol. 58, 96–102. doi: 10.1016/j.fsi.2016.09.015
Xie, F., Zan, Y., Zhang, X., Zhang, H., Jin, M., Zhang, W., et al. (2020). Differential abilities of mammalian Cathelicidins to inhibit bacterial biofilm formation and promote multifaceted immune functions of neutrophils. Int. J. Mol. Sci. 21. doi: 10.3390/ijms21051871
Yaeger, L. N., Coles, V. E., Chan, D. C. K., and Burrows, L. L. (2021). How to kill Pseudomonas-emerging therapies for a challenging pathogen. Ann. N. Y. Acad. Sci. 1496, 59–81. doi: 10.1111/nyas.14596
Yang, D., Chertov, O., Bykovskaia, S. N., Chen, Q., Buffo, M. J., Shogan, J., et al. (1999). Beta-defensins: Linking innate and adaptive immunity through dendritic and T cell CCR6. Science 286, 525–528. doi: 10.1126/science.286.5439.52
Yasir, M., Dutta, D., and Willcox, M. D. P. (2020). Activity of antimicrobial peptides and ciprofloxacin against Pseudomonas aeruginosa biofilms. Molecules 25. doi: 10.3390/molecules25173843
Yu, S., and Ma, L. (2017). [Iron uptake and biofilm formation in Pseudomonas aeruginosa]. Sheng wu gong cheng xue bao =. Chin. J. Biotechnol. 33, 1489–1512. doi: 10.13345/j.cjb.170140
Zaïri, A., Ferrières, L., Latour-Lambert, P., Beloin, C., Tangy, F., Ghigo, J. M., et al. (2014). In vitro activities of dermaseptins K4S4 and K4K20S4 against Escherichia coli, Staphylococcus aureus, and Pseudomonas aeruginosa planktonic growth and biofilm formation. Antimicrob. Agents Chemother. 58, 2221–2228. doi: 10.1128/AAC.02142-13
Zairi, A., Tangy, F., Bouassida, K., and Hani, K. (2009). Dermaseptins and magainins: antimicrobial peptides from frogs’ skin-new sources for a promising spermicides microbicides-a mini review. J. Biomed. Biotechnol. 2009:452567. doi: 10.1155/2009/452567
Zairi, A., Tangy, F., Ducos-Galand, M., Alonso, J. M., and Hani, K. (2007). Susceptibility of Neisseria gonorrhoeae to antimicrobial peptides from amphibian skin, dermaseptin, and derivatives. Diagn. Microbiol. Infect. Dis. 57, 319–324. doi: 10.1016/j.diagmicrobio.2006.11.006
Zaïri, A., Tangy, F., and Hani, K. (2013). Dermaseptin S4 derivative K4K20S4: a potential candidate for development of a new microbicide contraceptive agent--an in vitro study. Euro. J. Contracept. Reprod. Health Caren 18, 79–87. doi: 10.3109/13625187.2013.769950
Zanetti, M. (2005). The role of cathelicidins in the innate host defenses of mammals. Curr. Issues Mol. Biol. 7, 179–196.
Zasloff, M. (1987). Magainins, a class of antimicrobial peptides from Xenopus skin: isolation, characterization of two active forms, and partial cDNA sequence of a precursor. Proc. Natl. Acad. Sci. U. S. A. 84, 5449–5453. doi: 10.1073/pnas.84.15.5449
Zhang, L., Rozek, A., and Hancock, R. E. (2001). Interaction of cationic antimicrobial peptides with model membranes. J. Biol. Chem. 276, 35714–35722. doi: 10.1074/jbc.M104925200
Zhao, H., Gan, T. X., Liu, X. D., Jin, Y., Lee, W. H., Shen, J. H., et al. (2008). Identification and characterization of novel reptile cathelicidins from elapid snakes. Peptides 29, 1685–1691. doi: 10.1016/j.peptides.2008.06.008
Zhao, Q., Shi, Y., Wang, X., and Huang, A. (2020). Characterization of a novel antimicrobial peptide from buffalo casein hydrolysate based on live bacteria adsorption. J. Dairy Sci. 103, 11116–11128. doi: 10.3168/jds.2020-18577
Zheng, Z., Tharmalingam, N., Liu, Q., Jayamani, E., Kim, W., Fuchs, B. B., et al. (2017). Synergistic efficacy of Aedes aegypti antimicrobial peptide cecropin A2 and tetracycline against Pseudomonas aeruginosa. Antimicrob. Agents Chemother. 61. doi: 10.1128/AAC.00686-17
Zhu, M., Liu, P., and Niu, Z.-W. (2017). A perspective on general direction and challenges facing antimicrobial peptides. Chin. Chem. Lett. 28, 703–708. doi: 10.1016/j.cclet.2016.10.001
Keywords: antimicrobial peptides, Pseudomonas aeruginosa, Escherichia coli, drug resistance, biofilms, interaction
Citation: Chen X, Su S, Yan Y, Yin L and Liu L (2023) Anti-Pseudomonas aeruginosa activity of natural antimicrobial peptides when used alone or in combination with antibiotics. Front. Microbiol. 14:1239540. doi: 10.3389/fmicb.2023.1239540
Edited by:
Rustam Aminov, University of Aberdeen, United KingdomReviewed by:
Jianhua Wang, Chinese Academy of Agricultural Sciences (CAAS), ChinaEugene A. Rogozhin, Institute of Bioorganic Chemistry (RAS), Russia
Copyright © 2023 Chen, Su, Yan, Yin and Liu. This is an open-access article distributed under the terms of the Creative Commons Attribution License (CC BY). The use, distribution or reproduction in other forums is permitted, provided the original author(s) and the copyright owner(s) are credited and that the original publication in this journal is cited, in accordance with accepted academic practice. No use, distribution or reproduction is permitted which does not comply with these terms.
*Correspondence: Lihong Liu, hongllh@126.com