- 1Environmental and Industrial Biotechnology Division, The Energy and Resources Institute, New Delhi, India
- 2ONGC Energy Centre, New Delhi, India
- 3Essar Oil and Gas Exploration and Production Limited, Durgapur, West Bengal, India
It is widely acknowledged that conventional mining and extraction techniques have left many parts of the world with depleting coal reserves. A sustainable method for improving the recovery of natural gas from coalbeds involves enhancing the production of biogenic methane in coal mines. By taking a culture-independent approach, the diversity of the microbial community present in the formation water of an Indian reservoir was examined using 16S rRNA gene amplification in order to study the potential of microbial-enhanced coal bed methane (CBM) production from the deep thermogenic wells at a depth of 800–1200 m. Physicochemical characterization of formation water and coal samples was performed with the aim of understanding the in situ reservoir conditions that are most favorable for microbial CBM production. Microbial community analysis of formation water showed that bacteria were more abundant than archaea. Proteobacteria, Firmicutes, and Bacteroidetes were found as the most prevalent phyla in all the samples. These phyla play a crucial role in providing substrate for the process of methanogenesis by performing fermentative, hydrolytic, and syntrophic functions. Considerable variation in the abundance of microbial genera was observed amongst the selected CBM wells, potentially due to variable local geochemical conditions within the reservoir. The results of our study provide insights into the impact of geochemical factors on microbial distribution within the reservoir. Further, the study demonstrates lab-scale enhancement in methane production through nutrient amendment. It also focuses on understanding the microbial diversity of the Raniganj coalbed methane block using amplicon sequencing and further recognizing the potential of biogenic methane enhancement through microbial stimulation. The findings of the study will help as a reference for better strategization and implementation of on-site microbial stimulation for enhanced biogenic methane production in the future.
1. Introduction
Coal bed methane (CBM) is oftentimes regarded as the fuel that bridges the gap between conventional fossil fuels and sustainable energy sources. Methane is known to have a lesser adverse effect on the environment and human health due to its cleaner combustion and higher calorific value than coal. Both thermogenic and biogenic pathways can produce methane in coalbeds (Stolper et al., 2014). High temperatures and pressures (157–221°C) during coalification lead to the formation of thermogenic methane, whereas biogenic methane is predominantly produced by methanogens via microbial degradation of coal at moderate temperatures (50°C) (Wei et al., 2014).
A sizeable fraction of the world’s natural gas reserves is made up of coalbed methane (CBM), and it has been speculated that up to 20% of all natural gas, which includes CBM, emanates from microbes (Rice and Claypool, 1981). Enhanced CBM, also known as microbially enhanced CBM (MECoM), is the technique of stimulating microbes to produce more methane from currently producing wells. It may be possible to facilitate the microbial communities involved in the dynamic process of methanogenesis that produces CBM to further increase the methane production from the biodegradation of coal over timescales that are relevant to the industry. The lifespans of deficient microbial CBM wells could be increased if microbial CBM production could be enhanced, and possibly new microbial methane could be produced in sites with no history of gas production. In addition to the commercial gain, enhancing microbial methane production could also lower the adverse environmental effects of CBM production as the existing infrastructure would be used instead of drilling new wells when the old wells are depleted. Similar techniques might be utilized to produce methane in gas deficient shales from coal waste or deep or thin, potentially unmineable coal seams (Ritter et al., 2015).
It is the current belief that the abundance and diversity of the microbial community present in the coal seams are crucial to the production of biogenic methane in the coal beds. Research in the past has repeatedly shown that the interaction of several microbe groups involved in bio-methanation, such as fermentative bacteria, syntrophs, and methanogens, is necessary for the microbial mineralization of organic materials to methane (Strąpoć et al., 2011; Wawrik et al., 2012; Park and Liang, 2016; Wang et al., 2016). Numerous studies have thus far investigated the microbial community of coalbeds from various geographical regions, including Japan (Shimizu et al., 2007), the United States (Barnhart et al., 2013), Vietnam (Hoang et al., 2021), Canada (Lawson et al., 2015), and China (Guo et al., 2012). In contrast, not much is known about the microbial population in coalbeds located in geologically differing regions of India.
Therefore, the principal objective of this study is to fill the knowledge gap by providing a thorough analysis of the microbial community and their potential functions in the formation water collected from the Raniganj CBM block located in Durgapur, West Bengal. Further, to understand the role of geochemical factors in shaping the microbial diversity within the reservoir, the relationship between the microbial communities and geochemical factors is evaluated. The study also illustrated significant enhancement in the production of biogenic methane at the lab scale. To the best knowledge of the authors, this study is a prime investigation elucidating the diversity of microbial communities present in this region by 16S rRNA-based metagenomic analyses. Understanding the role of the indigenous microbial population in methanogenesis would help highlight the potential of comparable high temperature CBM reservoirs for biogenic methane production via in situ stimulation in the future.
2. Materials and methods
2.1. Study site and geographical setting
Samples of formation water, gas, and coal were acquired from five CBM wells, namely Well-A, Well-B, Well-C, Well-D, and Well-E, present in ESSAR Oil’s Durgapur coal bed methane block, which is located in West Bengal, India. Figure 1 shows the coal block from which the coal wells were selected for this study. The Raniganj CBM (Coal Bed Methane) block is located in the Burdawan district of West Bengal, India. The block is spread over a 500 sq. km. area in the prolific Damodar Valley Basin with thick Permo-Carboniferous Gondwana coal seams. The majority of India’s coal reserves are found in the lower Gondwana stratigraphic sequence, which is of Permian age and encompasses the coal-rich Raniganj and Barakar measures. Figure 2 shows the generalized litho-stratigraphy of the basin, depicting that the effective thickness of the Raniganj formation with coal seams is at 95–698 m, which is seen to be related to high CBM productivity amongst all other assets of ESSAR Oil. The vast coalfields of Raniganj, Barakar, and Jharia hold enormous amounts of high-quality coal. On the basis of coal rank, coal maturity, available area, physicochemical properties of coal, CBM potential, depth of occurrence of coal, and geological age, the Indian coal basins are categorized into four kinds (Vedanti et al., 2020). The Raniganj coalfield is classified as Category I and has thick coal seams with high rank and maturity, as well as large reservoirs of methane gas and sorption capacity (Vishal et al., 2013). The field has been developed with over 340 production wells and an extensive network of infield pipelines; spread over 300 km, connecting the wells to four gas-gathering stations. Essar Oil & Gas Exploration and Production Limited (EOGEPL) is a significant natural gas supplier for the region and provides gas feed to many industries.
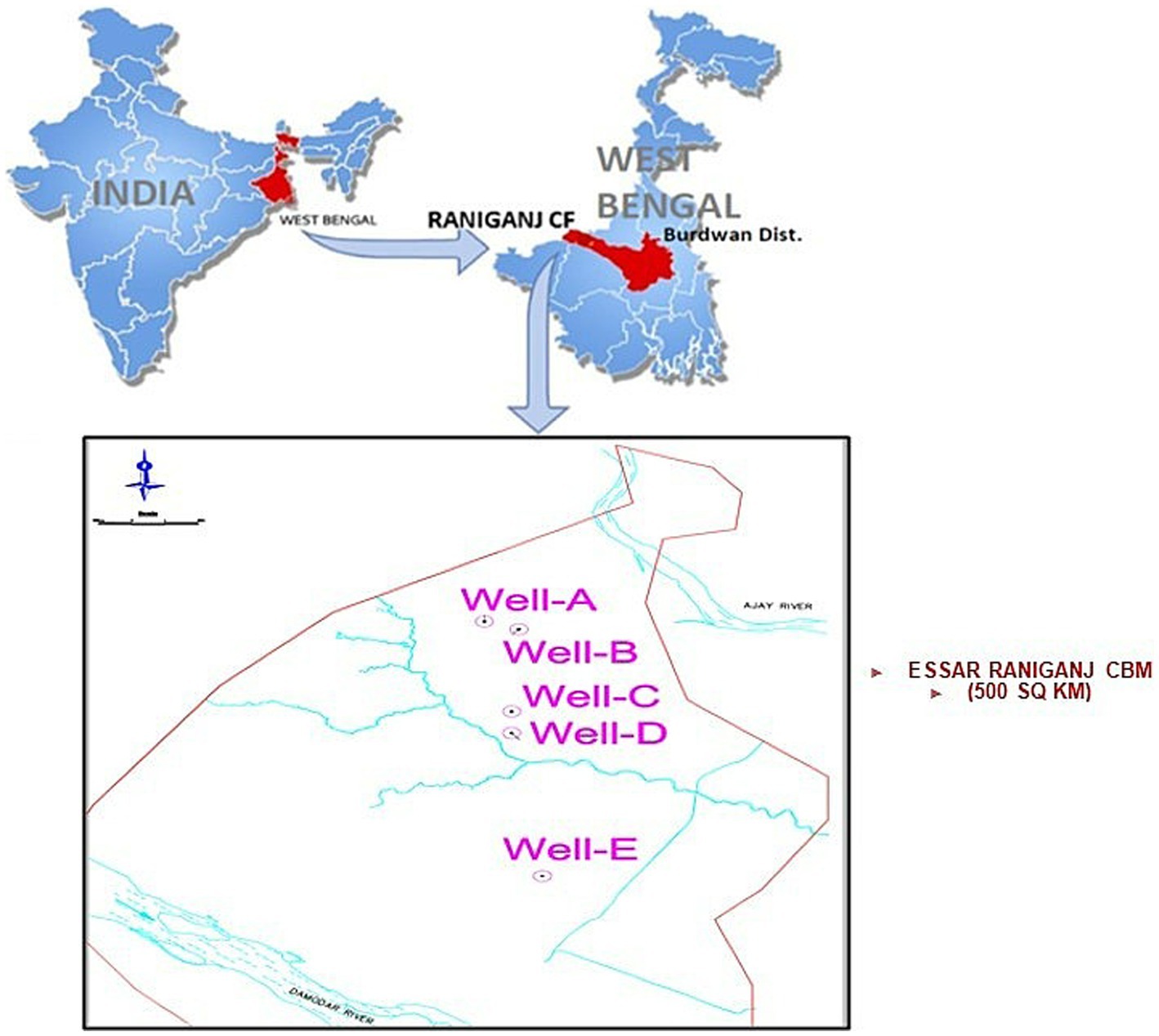
Figure 1. The Raniganj CBM (Coal Bed Methane) block located in the Burdawan district of West Bengal, India.
2.2. Site selection criteria
Understanding the in situ conditions and the history of the reservoir where the methane generation occurs is necessary, as these factors may affect the methane enhancement rates when the MECoM technology is applied in the reservoir. Producing wells with a gas and water production history were considered candidate CBM wells (Figure 3). The produced gas was studied for isotopic compositions to find evidence of biogenic, which could potentially be stimulated for enhanced methane production.
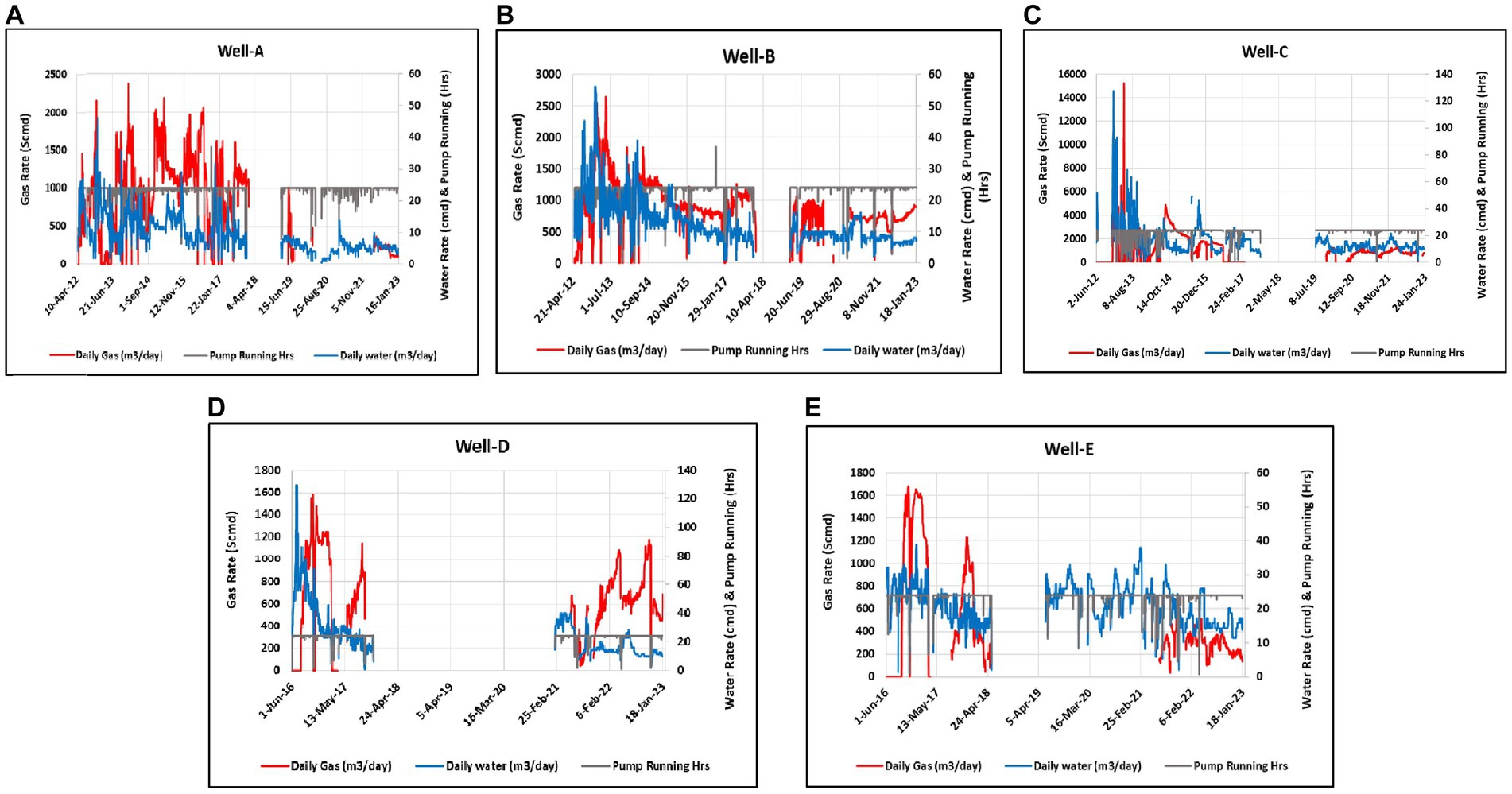
Figure 3. Daily production data of selected wells. (A) Complete production history of Well-A. (B) Complete production history of Well-B. (C) Complete production history of Well-C. (D) Complete production history of Well-D. (E) Complete production history of Well-E.
The majority of the coal-degrading microbial communities involved in methane production resides in the fractures (cleats) in coal seams and are typically 3–10 μm broad or at the interface of coal and surrounding rocks. Thus, further selection of the wells was made on the basis of feasibility tests performed on the field, which included testing the porosity and virgin permeability, as well as the post-fracture permeability and the half-fracture length. Essential criteria such as the bottom-hole temperature of the well and the pH and salinity of the formation water were also taken into account. The five selected wells had bottom-hole temperatures of less than 65°C, and the pH of formation water from all wells was less than 8, with salinity being less than 5%. The number of producing coal seams and the drilling depth of the wells were also considered important parameters in the selection process. The drilling depth of the selected wells is in the range of 901–1435 m, with the producing coal seams in the range of 5–8 having varying thicknesses between 0.3 and 5.82 TVD m.
2.3. Sampling
The collection of samples was conducted using a polyvinyl chloride pipe that was 1 m long with a diameter of 10 cm, sealed at the bottom, and closed with an open steel mesh at the top. At the field site, a bleach solution was used to sterilize the pipe and mesh enclosure prior to lowering them to the bottom of the well on a wireline. Sterilized 1000 mL anaerobic serum bottles sparged with nitrogen were used to collect the sample upto the brim and then sealed with airtight rubber septa and aluminum crimps. Samples were also collected for on-site testing of pH and conductivity. The sample bottles were then brought to the laboratory for further analysis. Conforming to the method outlined by Rathi et al. (2015), formation water samples were also collected from the well-heads of the selected candidate wells, based on selection studies, and transferred into sterile 100 mL anaerobic serum bottles containing 1 mL of 2% Na2S. All the samples were brought to the laboratory in under 48 h, kept at 4°C, and then promptly processed for microbial analysis. Additionally, produced gas samples collected from the well heads were processed for compositional and isotopic analysis. A representative coal sample was drilled from the CBM block of Essar Oil, located in Durgapur, West Bengal, and was used as representative coal for all CBM wells involved in the study.
2.4. Physicochemical analysis
2.4.1. Formation water
Each well’s collected formation water samples underwent physicochemical analysis for pH and Electrical conductivity at room temperature per the American Petroleum Institute (API) standards. The presence of heavy metals such as arsenic, cadmium, chromium, copper, and Fluoride was also estimated as per the standard methods (IS3025 PT 37:1988, APHA3100(B), APHA3500(B), APHA3111(B), APHA4500 (F-D)) respectively. The presence of cation [calcium APHA 3500 (B)] and anion [chloride: APHA 4500, nitrate: IS 3025, phosphate: APHA 4500 (D), and sulfate APHA 4500 (E)] was also estimated in the formation water.
2.4.2. Coal
The detailed analysis of coal in terms of ash, moisture, volatile matter, and fixed carbon, along with the specific carbon, hydrogen, nitrogen, sulfur, and oxygen (CHNSO) profile, was determined as per the guideline of ASTM standards.
2.4.3. Carbon stable isotopes of collected gas
A critical tool for understanding the origin of CBM gas is the carbon isotope composition study. The molecular and isotopic compositions of the gases collected from the Raniganj field were studied by the Keshava Deva Malaviya Insititute of Petroleum Exploration, Oil and Natural Gas Corporation Ltd., according to the method stated by Wang et al. (2019).
2.5. Genomic analysis of formation water from the Raniganj block
Formation water samples were processed for genomic DNA extraction in order to evaluate the capacity of the thermogenic CBM reservoir to produce biogenic methane. 500 mL of each formation water sample was run through a filter membrane (0.22 μm) to extract genomic DNA. Coal particles were suspended in the formation water. All the microbes adhering to the coal particles that had remained on the filter were processed using the DNeasy Power Water kit for DNA extraction. The protocol provided by the manufacturer was followed for DNA extraction; quantification and analysis of DNA were performed using a NanoDrop spectrophotometer. The extracted DNA samples that exhibited high quality with A260/280 ratio between 1.8 and 2.0 and concentrations greater than 50 ng/μl were taken up further for sequencing.
To understand the overall diversity of the microbial population, extracted DNA of all five well samples was processed for PCR amplification using universal bacterial primers (27F and 1492R) as described by Rathi et al. (2019), V3-V4 amplicon sequencing was performed on the Illumina MiSeq 2500 platform by Medgenome Pvt. Ltd. The 16S rRNA gene sequences were checked for purity with the Check-Chimera program, and OTU picking at 97% sequence similarity thresholds (Open reference method) were performed. Data rarefaction, cumulative sum scaling (CSS), low variance, and low count filtering were carried out before downstream analysis. These pre-processing steps enabled to avoid the sequencing depth bias for better comparative analysis (Rajeev et al., 2021).
The microbial analysis was done in the software MicrobiomeAnalyst (Dhariwal et al., 2017). The prediction of the functional profiles of bacterial taxa based on 16S rRNA sequencing data was performed with the Metagenassist software using the amplicon sequencing data according to the Silva-132 database (Arndt et al., 2012). This tool was used to predict functional community profiles based on 16S rRNA data to outline microbial metabolism in the studied samples in the Raniganj coal bed formation water. Canonical correspondence analysis (CCA) and correlation analysis were used to analyze the relationship between the geochemical factors and taxonomic groups at the class level using software PAST 4.03 (Arndt et al., 2012).
The metagenome sequence reads of the samples from the candidate wells, Well-A, Well-B, Well-C, Well-D, and Well-E, were submitted to the NCBI archive under the BioProject accession number PRJNA965902.
2.6. Enrichment of a methane-producing microbial consortium from the formation water
Methane-producing microbes were selectively screened from the collected formation water samples (10% (v/v)) in the laboratory by enriching them in methanogen-specific nutrient media containing 1% (w/v) coal as the carbon source (Rathi et al., 2015). The composition (per liter) included K2HPO4; 0.4 g, KH2PO4; 0.2 g, MgCl2.6H2O; 0.1 g, NaCl; 0.5 g, NH4Cl; 0.2 g, NaHCO3; 0.2 g, and Yeast extract; 0.5 g. The pH was then adjusted to 7.0 ± 0.2. 100 μL of Resazurin was added to the media as an oxygen indicator. The media was boiled for 10 min and allowed to cool down while being purged with nitrogen to remove the dissolved oxygen. The remaining dissolved oxygen was then subsequently removed by adding 0.5 g L-cysteine hydrochloride, making the environment of the media completely oxygen-free. A volume of 30 mL media was dispensed into 60 mL anaerobic serum bottles and flushed again with N2. The bottles were sealed with butyl rubber stoppers and crimped with aluminum caps. The sealed and pressurized bottles were autoclaved before inoculation for 15 min at 121°C. The bottles that were not inoculated were considered as control.
Methane generation in incubated culture bottles was monitored during the enrichment process by collecting 0.5 mL of headspace gas samples from the serum bottles via a gas-tight syringe and quantifying the anticipated headspace gases, such as methane, carbon dioxide, nitrogen, and hydrogen, using gas chromatography. All experiments were run in a triplicate set. The data points are the average of triplicate±standard deviation (<5% of average).
3. Results
3.1. Understanding the role of limiting factors for biological methane production via physio-chemical analysis
Physio-chemical analysis of formation water collected for this study is shown in Table 1. The pH was seen to be in the range of 7.0–8.3, suggesting suitable conditions in the reservoir for biogenic methane production. It is notable that similar recent studies have reported the formation water samples in their studies to be pH range up to 8.3 (Hoang et al., 2021). The concentration of heavy metals, including Arsenic (As5+), Cadmium (Cd2+), Chromium (Cr2+), Copper (Cu2+), Zinc (Zn2+), Silver (Ag2+), and Nickel (Ni2+), was found to be below detectable range, signifying a non-toxic environment for the indigenous microbial community to flourish and produce methane. The presence of Sulfate (SO4 2−), Fluoride (F−), and Iron (Fe2+) was found and is shown in Table 1. In this study, the concentration of sulfate ions in the formation water also corroborates what was seen in past investigations in Indian reservoirs (Rathi et al., 2019).
The proximate analysis of coal of the seams from all selected CBM wells was carried out (Table 2). The coals are of low moisture (1.71–4.69%) and medium ash (11.38–19.25%) with an average of 33% volatile matter and approximately 50% fixed carbon. These results indicate that the ASTM rank of the coal is high volatile ‘A’ bituminous (HVAB). A laboratory feasibility study was conducted by Fallgren et al. (2013), focusing on the comparison of coal ranks for biogenic methane production via the enrichment of microbial consortia. The study indicated that the rate of methane production was higher in the case of bituminous coal as compared to lower-rank coals. Ultimate analysis results indicated low sulfur content (0.32–0.47%) with up to 67% carbon and up to 4.14% hydrogen.
3.2. Molecular and isotopic compositions of studied gases
Analyzing the carbon isotopic fingerprints (δ13 C-CH4) produced by biogenic and thermogenic CH4 allows one to determine the source of the gas (Schoell, 1980). Biogenic CH4 can have δ13 C values between-110% and-40%, but the values of thermogenic CH4 carbon stable isotopes are between −50% and −20% (Whiticar, 1999). The molecular and isotopic compositions of the studied gases from the Raniganj field indicate their mixed origin. The gases are also dry in nature (C1/(C2 + C3) >50) (Bernard et al., 1976). The significant difference in stable carbon isotope ratios of methane and ethane (~20%), and the presence of isotopically heavier carbon dioxide (6.6–15.5%) indicate its association with biogenic processes (Kinnon et al., 2010), particularly H2 utilizing hydrogenotrophic methanogens. The results of the molecular and isotopic compositions are provided in detail in Table 3.
3.3. 16S rRNA amplicon sequencing depicting the relative abundance of microbial diversity
In this investigation, five coal bed methane wells were chosen for sampling as they are representative examples of relatively high temperature coal bed methane reservoirs with a bottom-hole temperature of about 50°C. The 16S rRNA gene clone library was created from the formation water samples of the CBM wells in order to study the microbial population of these high temperature thermogenic CBM wells and their relationship in connection to the potential for biogenic methane generation. The samples had a total of 153,403 effective read counts of the 16S rRNA gene amplicons. The rarefaction curve plotted between species richness and sequence sample size has been shown in Supplementary Figure 1.
The amplicon sequencing reveals the relative abundance of the microbes present in the studied wells, showing both archaeal and bacterial diversity (Figure 4). Proteobacteria, Firmicutes, Bacteroidetes, and Actinobacteria were the most prevalent phyla in all the samples. The formation water from the thermogenic high temperature CBM reservoir in this study demonstrated that the abundance of Proteobacteria was primarily composed of Gammaproteobacteria and Alphaproteobacteria. Other than the ubiquitously dominant phyla in the reservoir, Well-A shows a relatively high abundance consisting of Spirochaetes, Acetothermia, Synergistetes, Chloroflexi, and Caldiserica. Well-B shows a relatively high abundance of Nitrospirae and Chloroflexi. A significant proportion of uncultured bacteria is found in the Well-C, followed by Spirochaetes, Acetothermia, and Chloroflexi, among other relatively less dominant phyla. Well-D is also found to be abundant with Acetothermia, Spirochaetes, Caldiserica, Chloroflexi, and Synergistetes, along with Hydrothermae, Coprothermobacteraeota, Acidobacteria, and Thermotoga. In the case of Well-E, the presence of Euryarchaeota, Nitrospirae, and Synergistetes is seen among other dominant phyla.
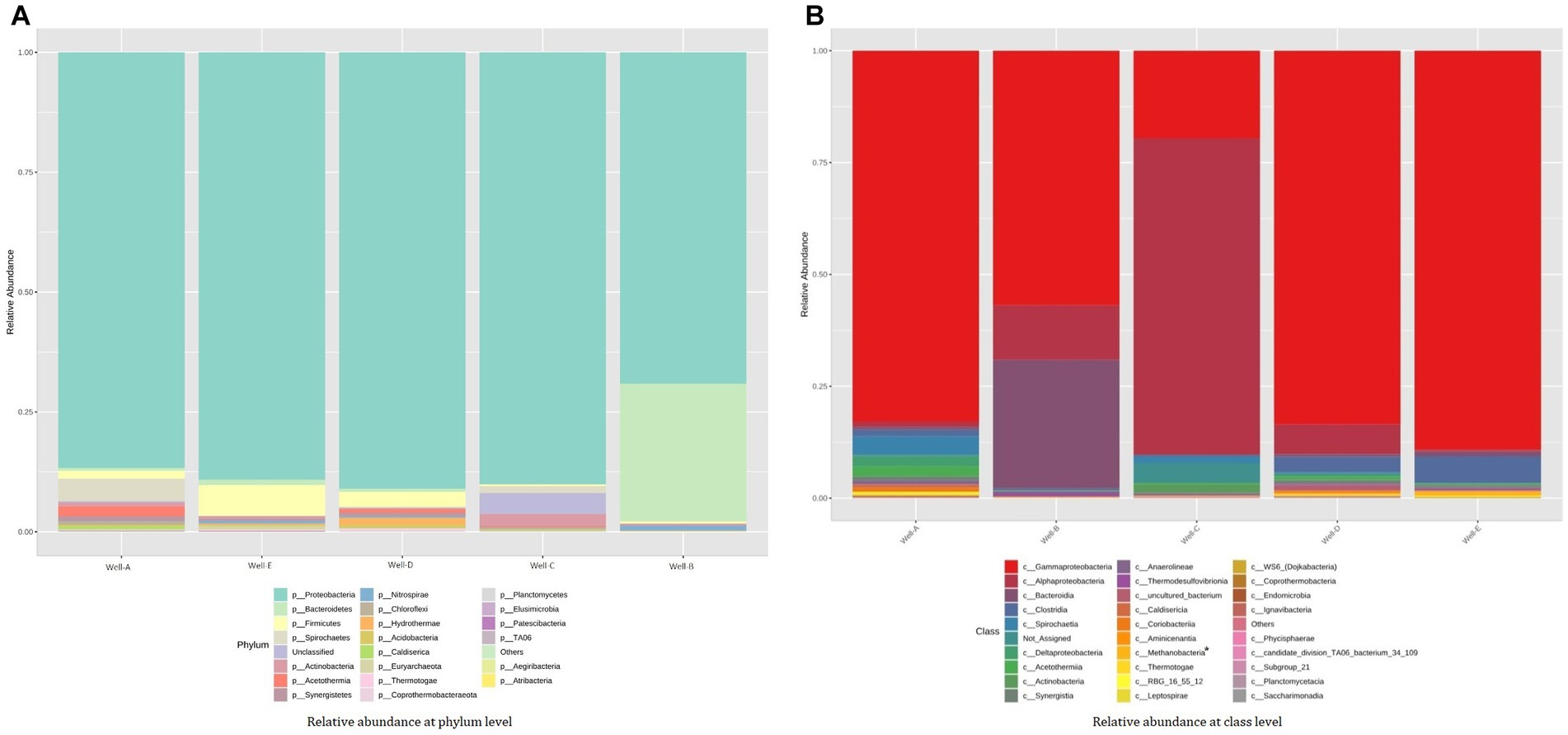
Figure 4. Stacked bar plot representing the relative abundance assessed by 16S rRNA-gene amplicon sequencing at (A) phylum-level and (B) class level (* denotes archaeal group).
The taxonomic analysis at the class level shows the relative abundance of class Gammaproteobacteria, Alphaproteobacteria, and Deltaproteobacteria. The relative abundance pattern also showed the presence of class Bacteroidia and Clostridia in all the samples. Class Methanobacteria was the methanogenic group present in the samples.
At the genus level (as shown in Figure 5), the microbial community found in well number Well-A has a relative abundance of Smithella, Aquabacterium, Desulfonatronum, Syntrophobacter, Caulobacter, Proteiniphium, and Desulfitibacter, among others. The relative abundance, as seen in Well-B, majorly includes Thermodesulfovibrio, Thermanaerothrix, Hydrogenophaga, Phenylobacterium, and Caulobacter. Well-C also shows a dominant abundance of Caulobacter, along with Methylobacillus, Brevundimonas, Novosphingobium, Parvibaculum, Bosea, Microbacterium, Sphingopyxis, Treponema, Bradyrhizobium, Rhodococcus, and Mahella. In the case of Well-D, the most dominant community consists of Methylomicrobium, Sphingomonas, Coprothermobacter, Thauera, Spirochaeta, Azoacrus, Pannonibacter, Stappia, and Acetomicrobium. The microbial community found in well number Well-E majorly consists of Methanobacterium, Methanothermobacter, Pseudomonas, Thermoanaerobacter, Acinetobacter, Methylobacterium, Thermovirga, Desulfobacca, and Desulfotomaculum. Methanobacterium and Methanothermobacter, two well-known hydrogenotrophic methanogens, were the most prevalent archaeal groups found in Well-E.
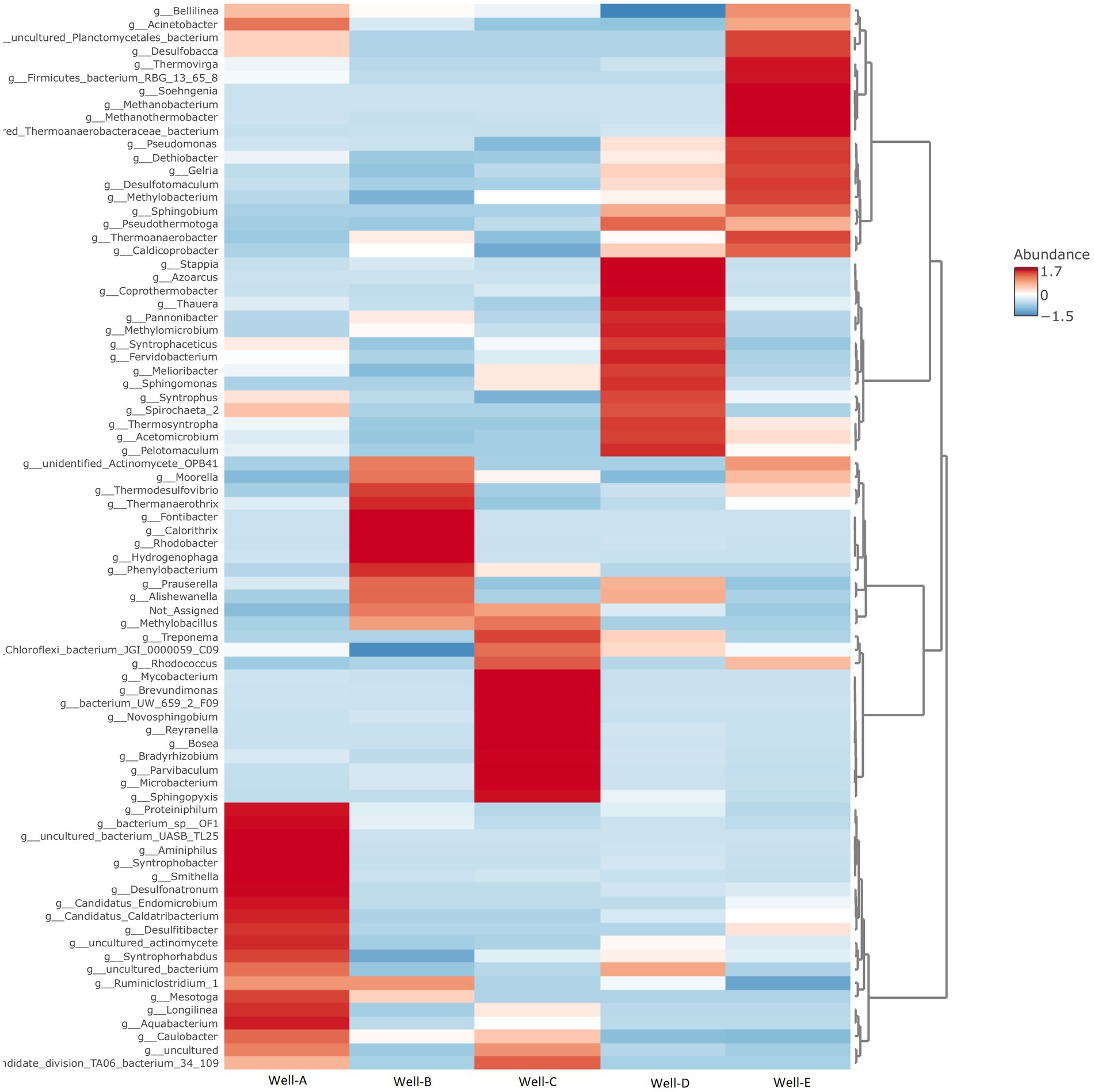
Figure 5. Heat map representing the relative abundance of microbial communities at the genus level, as seen in the formation water samples of selected CBM wells (Darker colors indicate a greater percentage of abundance than lighter colors).
3.4. Predictive functional analysis
The heatmap of predictive functional analysis shows the presence of methanogenic functions in all the wells suggesting the potential for enhancement in CBM (Figure 6). Among all the samples, the highest methanogenic activity is present in Well E, which is also supported by microbial diversity analysis. There is a high abundance of aromatic degradation in wells D, E, and A. The analysis also showed the existence of sulfate-reducing metabolism in the CBM wells. The abundance of the sulfate reducers was found to be lower than the methanogens in wells A, B, and C. It also reveals the presence of chitin and xylan degraders.
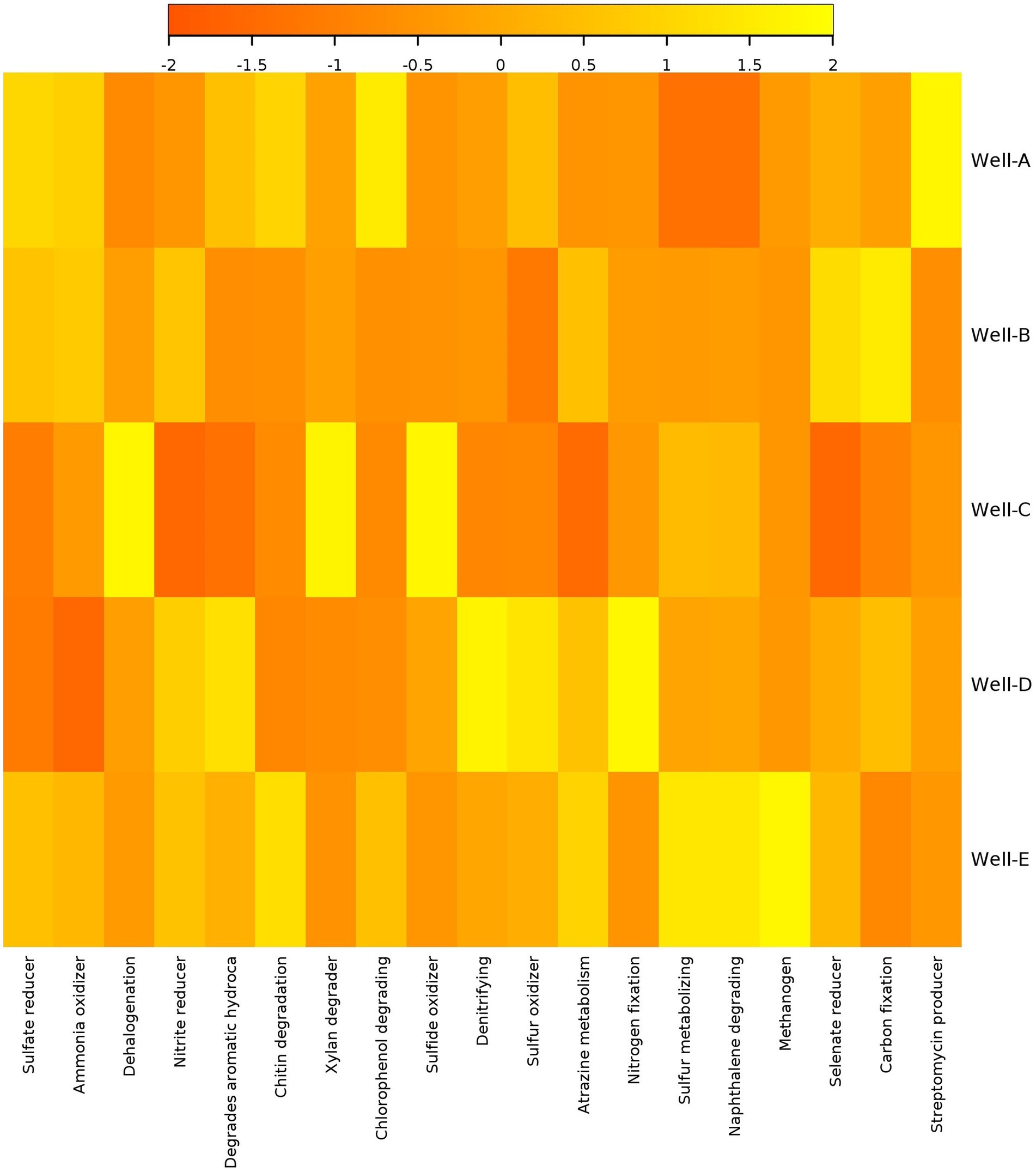
Figure 6. Heatmap showing relative function abundance predictions of the microbial communities based on metagenassist analysis.
3.5. Understanding the effects of physicochemical parameters of formation water on microbial community
In the present study, Canonical correspondence analysis (CCA) was used to analyze whether correlation in microbial diversity is related to geochemical factors. According to the CCA analysis (Figure 7A), class Clostridia, Gammaproteobacteria, Aminicenantia, Coprothermobacteria, Planctomycetacia, Methanobacteria, and Thermodesulfovibriona were found to be related. This is further verified by the correlation analysis (Figure 7B), which showed a significant correlation between Iron, Electrical conductivity, and TDS with classes Aminicenantia, Coprothermobacteria, Planctomycetacia, Thermodesulfovibriona, and Ignavibacteria. The results show how geochemical factors impact the diversity and distribution of microbes in the environment.
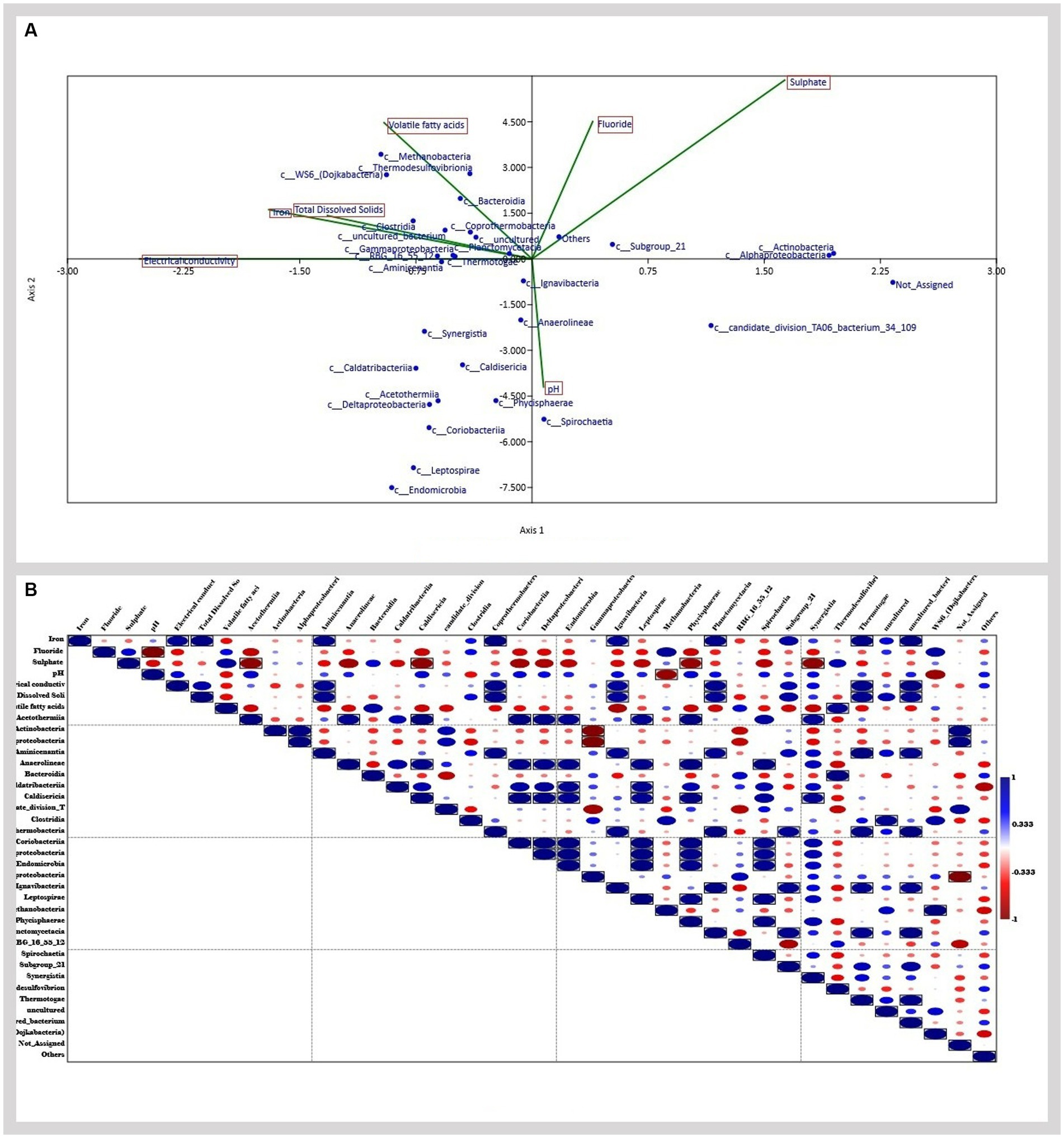
Figure 7. (A) Canonical correspondence analysis (CCA) analyzing the relationship between geochemical factors and microbial diversity (B) correlation analysis.
3.6. Quantifying the methane gas generated in the laboratory by the consortium via coal bioconversion
The amount of methane gas generated in the headspace of the experimental bottles containing the modified MPB nutrient media was quantified using gas chromatography. 3063.35 μmol CH4/g coal, 3190.64 μmol CH4/g coal, 1202.15 μmol CH4/g coal, 1004.28 μmol CH4/g coal, and 697.09 μmol CH4/g coal was observed to be produced by the thermophilic methanogenic consortium inoculated with formation water from well-A, well-B, well-C, well-D, and well-E, respectively, after 30 days of incubation (Figure 8). The experimental control media bottles containing 0.3 g coal under similar conditions showed no methane production at 55°C after 30 days.
4. Discussion
Numerous studies have examined the microbiological communities of coal/formation water for comprehending the methanogenic potential of coal beds in the USA, Canada, Japan, and Australia (Mochimaru et al., 2007; Shimizu et al., 2007; Green et al., 2008; Klein et al., 2008; Li et al., 2008; Strąpoć et al., 2008; Midgley et al., 2010; Penner et al., 2010). These microbial communities detected in coal beds have undergone culture-independent investigations that have revealed the identities of many bacterial species that are key players in the biodegradation of coal as well as archaea that produce methane via hydrogenotrophic or acetoclastic pathways. In this investigation, the 16S rRNA Amplicon sequencing revealed the relative abundance of the microbes present in the wells, showing that the most abundant phyla included Proteobacteria, Firmicutes, Bacteriodetes, and Actinobacteria. These phyla have been reported to be involved in the biotransformation of coal into methane in earlier studies (Strąpoć et al., 2011; Singh et al., 2012). The presence of Gammaproteobacteria and Alphaproteobacteria in the wells indicated different hydrocarbon-degrading pathways. It is widely recognized that bacteria from the phylum Alphaproteobacteria can break down hydrophobic compounds such as polycyclic aromatic hydrocarbons (Meslé et al., 2013). According to certain reports, Gammaproteobacteria can break down hydrocarbons when nitrate serves as the electron acceptor (Alain et al., 2012). In the past, Bacteroidetes have also been mentioned as pentachlorophenol degraders (Singh et al., 2012). The relative abundance shows the dominance of Euryarchaeota in Well-E. Euryarchaeota majorly consists of acetoclastic and hydrogenotrophic methanogens. Evidence suggesting that syntrophic acetate oxidation is coupled with hydrogenotrophic methanogenesis in the high temperature petroleum reservoirs, such as the Yabase oil field (Japan), has also been documented (Mayumi et al., 2011). Similar to the present study, a dominance of Spirochaetes has also been found by Singh et al. in the Jharia coal reservoir and in the coal bed of the Illinois basin (Strąpoć et al., 2008). As seen in the study by Green et al. (2008), the methanogenic consortium in the Powder River Basin included Spirochaetes in addition to Firmicutes, which predominated the bacterial community in coal bed methane wells there.
The relative abundance of class Gammaproteobacteria, Alphaproteobacteria, and Deltaproteobacteria in the taxonomic analysis of our samples at the class level is of significance, as members of Gammaproteobacteria and Alphaproteobacteria have been studied to be involved in the degradation of various organic compounds, including petroleum hydrocarbons (Zhou et al., 2020; Sudiana et al., 2022). Members of Deltaproteobacteria can anaerobically metabolize a wide variety of normal, iso-, and cyclic alkanes, as well as mono- and polycyclic aromatic hydrocarbons (Davidova et al., 2018). The hydrocarbonoclastic Deltaproteobacteria can take part in syntrophic consortia that ultimately transmit reducing equivalents to methanogenic Archaea without a respiratory electron acceptor. This shows their role in methane generation (Davidova et al., 2018). The presence of class Bacteroidia and Clostridia in all the samples is noteworthy, as previous studies have shown significant enhancement in methane production in consortia rich in Bacteroides and Clostridium (Tukanghan et al., 2021). Also, class Clostridium contains many hydrogen-producing bacteria, and since hydrogen is the limiting factor in the hydrogenotrophic mode of methanation, their presence is highly significant for enhanced CBM production (Palacios et al., 2021; Wang and Yin, 2021). The presence of Methanobacteria in the samples suggests hydrogenotrophic methanogenesis as a significant pathway of methane generation in our study.
There is a noteworthy variation in the genus level abundance within the reservoir. We speculate that this variation is due to the age of the coalbed, the depth of sampling, geological conditions, and the availability of substrates in the coalbed and formation water (Barnhart et al., 2016). Well-A is rich in Smithella and Syntrophobacter, which are syntrophic bacteria. These bacteria have been associated with coal degradation and with providing a substrate for methanogens to produce methane from mesophilic coal reservoirs in earlier research (Strąpoć et al., 2011; Singh et al., 2012). Well-B shows the presence of Hydrogenophaga, a typical hydrogen-utilizing bacteria which is also able to utilize biphenyl. In the study by Wei et al. (2014), the most dominant genus identified in abandoned coal mines was Hydrogenophaga. Another study highlighted that Hydrogenophaga, which was found in an oil-degrading consortium, is capable of metabolizing 4-aminobenzenesulfonate (Beckmann et al., 2011). It has been found in coal samples from the Liulin District (Guo et al., 2012) and in methanogenic enrichment modified with coal (Beckmann et al., 2011). The presence of Phenylobacterium was also abundant, and it also has been detected in the biogenic coal bed methane reserves of the Southern Qinshui Basin, China (Guo et al., 2014). The presence of sulfate-reducing bacteria such as Thermodesulfovibrio, Desulfotomaculum, and Desulfitibacter, which are known to be capable of degrading volatile organic matter (i.e., hydrocarbons and organic acids) in the presence of sulfate as the terminal electron acceptor, is attributed to the presence of sulfate ions and their concentration in the formation water. The prevalence of Methylobacillus, a methanotrophic bacterium, in the formation water of Well-C correlates with the findings of the study by Zhang et al. (2018) that methanogenic and methanotrophic pathways are present in the formation water of the coal reservoir, responsible for the carbon metabolism.
Thauera is amongst the most abundant in Well-D and is known to be a nitrate-reducing, hydrocarbon-degrading bacterium. It has been found in several other basins, including the Jharia basin (Singh et al., 2012), the Surat basin in Australia (Li et al., 2008), as well as the coal beds of Western Canada (Penner et al., 2010). The presence of Coprothermobacter is also notable in this study. Coprothermobacter sp. of the Thermodesulfobiaceae was previously identified as a thermophilic, chemo-organotrophic proteolytic acetogen in methanogenic enrichment culture from a thermophilic digester (55°C) (Ollivier et al., 1985). These proteolytic bacteria can convert yeast extract protein into acetate, hydroxyl, and carbon dioxide, as well as other acids in lower amounts, like isobutyric, isovaleric, and propionic acids. A recent study by Kobayashi et al. (2012) also claimed that Coprothermobacter works as a possible syntrophic acetate oxidizer in high-temperature methanogenic enrichment cultures. Moreover, Singh et al. noted that Coprothermobacter was dominant in their methanogenic enrichment culture.
The prevalence of Methanobacterium and Methanothermobacter in Well-E indicates that hydrogenotrophic pathways constitute the primary means of methane production. The presence of Methanothermobacter, which is also a thermophilic CO2-reducing methanogen, was found in similar studies, including the coal reservoir of Ruhr Basin, Germany, a biogenic methane-producing source, and the Powder River Basin, USA (Flores et al., 2008). The predominance of the genera Pseudomonas has been reported for hydrocarbon degradation and has been found in the coal seams of Western Canada (Penner et al., 2010). Similarly, in the present study, the presence of Pseudomonas was observed. According to various reports, Pseudomonas is involved in the breakdown of complex organic substances such as alkanes, alkenes, and polycyclic aromatic hydrocarbons into fatty acids like formate, lactate, acetate, ethanol, and CO2. Furthermore, the production of biosurfactants by the Pseudomonas genus can promote the breakdown of polycyclic aromatic hydrocarbons because these substances enable a decrease in surface and interfacial tensions between coal molecules to enhance solubility (Singh and Tripathi, 2013; Gründger et al., 2015; Colosimo et al., 2016; Mehboob et al., 2016; Davis and Gerlach, 2018). Desulfotomaculum, a methylotrophic sulfate-reducing bacteria found in our samples, has been previously reported in the formation water of the Jharia basin (Singh et al., 2012).
The results of the predictive functional analysis support the microbial diversity analysis, showing the presence of methanogenic functions in the reservoir. This indicates toward the potential for CBM enhancement in the studied wells. The highest methanogenic activity is observed in Well-E. The degradation of hydrocarbon compounds is an important step in the process of methanogenesis (Liu et al., 2022). A high abundance of aromatic degradation in Well-D, Well-E, and Well-A shows the potential of indigenous microbes to convert complex organic compounds into substrates used in the process of methane generation. The analysis reveals the existence of sulfate-reducing metabolism in the reservoir. Studies have also shown the coexistence of methanogens with sulfate-reducing bacteria in various environments (Sela-Adler et al., 2017). The presence of chitin and xylan degraders also represents the abundance of organic compounds degrading microbes, thus reflecting good potential of CBM enhancement in the wells.
The predictive functional analysis is also supported by the flowchart diagram showing the pathway of degradation of coal into methane (Figure 9). The diagram showed various microbes present in the formation water that are known to be involved in various steps of coal degradation. The analysis revealed the abundance of hydrolytic, fermentative, and syntrophic microbes in the wells. These are the major key players in the process of methanogenesis (Nazaries et al., 2013). The analysis also revealed the presence of Methanobacterium and Methanothermobacter as the main methanogens in the wells; thus, hydrogenotrophic is the main route in the process of methane generation. Acinetobacterium is a well-known bacterial genus involved in the degradation of complex organic compounds (Zhang et al., 2021). The high abundance of Acinetobacter in the wells shows the capability of coal degradation in the wells. Genus Fervidobacterium, Gelria, and Pseudomonas are the hydrolytic and fermenting bacteria involved in methane generation (Harirchi et al., 2022). Genus Rhodobacter and Rhodococcus are involved in the degradation of various complex organic and inorganic compounds (Do et al., 2003; Margesin et al., 2005). Species of Aquabacterium are known to be involved in the biodegradation of oils; thus, they have the capability of degrading complex hydrocarbons (Wang et al., 2019). Genus Thermoanaerobacter and Treponema present in the wells are known to be involved in acetogenesis (Harirchi et al., 2022). Various syntrophic bacteria were found in the wells essential for methane generation (Figure 6).
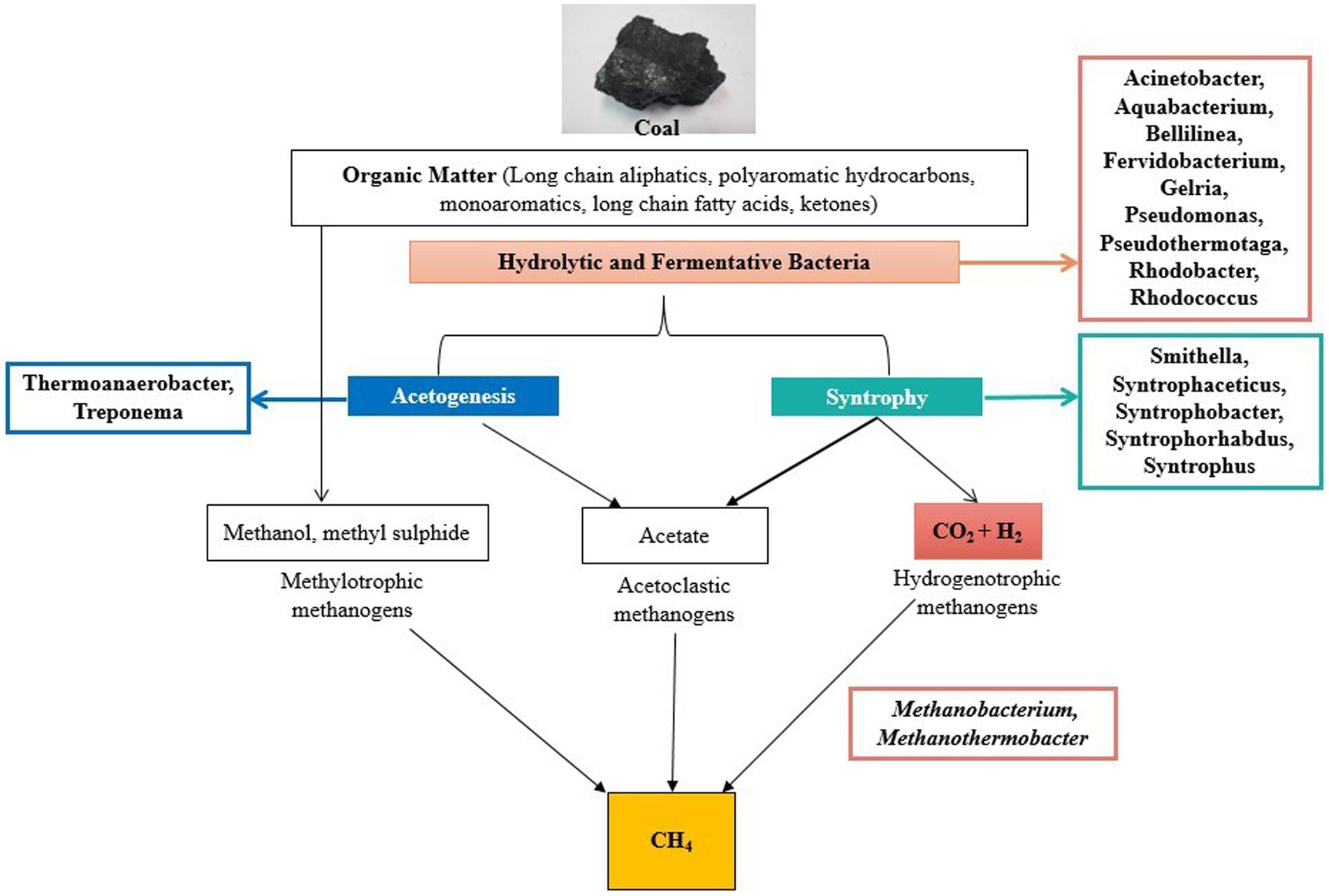
Figure 9. Pathway showing metabolism and microbes present in the formation water involved in coal degradation and generation of biogenic methane.
Several biotic and abiotic factors play huge roles in the shaping of microbial communities (Wang et al., 2020; Rajeev et al., 2021). In the case of CBM wells, the physicochemical parameters of the formation water significantly impact the distribution and diversity of microbial communities in the sample (Davis and Gerlach, 2018). Previous studies have shown the impact of geochemical factors like salinity, temperature, and pH significantly impacting the diversity of methanogens in their natural environments (Hoehler et al., 2010; Wen et al., 2017). In the present study, the results of the canonical corresponding analysis identify the effect of geochemical factors on the microbial diversity of the reservoir. The analysis shows a relation between various classes found in the samples. A correlation analysis further corroborates this relation, indicating a significant correlation between Iron, Electrical Conductivity, and TDS with classes Aminicenantia, Coprothermobacteria, Planctomycetacia, Thermodesulfovibriona, and Ignavibacteria. Members of these classes play an important role in the initial step of methanogenesis. They are involved in hydrocarbon degradation, fermentative, and hydrogen-producing metabolism (Liu et al., 2012; Kadnikov et al., 2019; Pavan et al., 2019; Storesund et al., 2020). A study has shown the relation between high bulk conductivities and TDS with enhanced mineral weathering, interlinked with the activities of hydrocarbon-degrading microbes in aquifers contaminated with hydrocarbons (Atekwana et al., 2004). Another study has reported that Fe(III) can be used as an electron acceptor in microbial hydrocarbon degradation, zero-valent iron can donate electrons for improved methanogenesis, and conductive iron oxides may help electron transfers in methanogenic processes. These pieces of evidence show how the geochemical parameters can impact the metabolisms and distribution of hydrocarbon degraders in the ecosystem (Castro et al., 2022). These findings validate the results of the correlation analysis done in the present study.
To further emphasize the role and functions of the indigenous archaea and bacteria present in the CBM reservoir of the Raniganj block, indigenous microbes from the formation water were enriched in methanogen-specific nutrient media, and methane gas produced by the consortium via coal conversion was quantified. The results clearly indicate the real potential of the indigenous microbes present in the formation water for the bioconversion of coal into methane. They also confirm the capability of the nutrient media to support the growth of a selective methanogenic consortium that can utilize bituminous coal present in thermogenic CBM reservoirs as a carbon source and lead to in situ methane enhancement.
5. Conclusion
As per the authors’ knowledge, this is the first study that provides the microbial analysis of the thermogenic gas-producing CBM reservoir in the Raniganj block and its biogenic methane potential. The microbial diversity found in the formation water samples in this investigation comprised abundant syntrophic bacteria (e.g., Coprothermobacter), methylotrophic bacteria (e.g., Methylobacterium, Methylobacillus), fermentative bacteria (e.g., Microbacterium, Pseudomonas, Acinetobacter) and methanogenic archaea. Proteobacteria, Bacteroidetes, and Firmicutes were the three bacterial phyla that were consistent in all formation water samples, with Proteobacteria being the most prevalent of them. The archaeal domain at the genus level showed the presence of methanogenic archaea, including Methanobacterium and Methanothermobacter, which are two well-known hydrogenotrophic methanogens, indicating that the primary pathway of biogenic methane production in the Raniganj CBM block is hydrogenotrophic. This analysis lays the groundwork for greater comprehension of the functional pathways that predominate and the underlying geomicrobiological diversity, enabling us to examine how it affects methane generation on a lab scale. A deeper understanding of the processes at play could be beneficial in the development of strategies for enhanced methane production.
Data availability statement
The datasets presented in this study can be found in online repositories. The names of the repository/repositories and accession number(s) can be found in the article/Supplementary material.
Author contributions
ML conceptualized the complete project and designed the experiments. NSi and ML provided the resources to set up and perform the experiments. MC conducted all the experiments, recorded and analyzed the data, and wrote the original draft. NSa conceptualized the idea of metagenomic analysis. MC, NSa, and ML reviewed and edited the manuscript. SS and KS participated in sampling. AM, MI, and SK provided the geological data. All authors contributed to the article and approved the submitted version.
Acknowledgments
We are sincerely thankful to Director General, TERI, for providing the infrastructure required for us to carry out the present study. We also express our appreciation toward Essar Oil and Gas Exploration and Production Limited (EOGEPL) for providing us the financial support for the study.
Conflict of interest
AM, MI, and SK were employed by Essar Oil and Gas Exploration and Production Limited. SS and KS were employed by ONCG Energy Centre.
The authors declare that this study received financial support from Essar Oil and Gas Exploration and Production Limited (EOGEPL). The funder had the following involvement with the study: EOGEPL provided technical support in the manuscript, including well production history, and geographical and geological content of the studied CBM wells; Figures 1 and 2 in the manuscript were also provided by EOGEPL.
Publisher’s note
All claims expressed in this article are solely those of the authors and do not necessarily represent those of their affiliated organizations, or those of the publisher, the editors and the reviewers. Any product that may be evaluated in this article, or claim that may be made by its manufacturer, is not guaranteed or endorsed by the publisher.
Supplementary material
The Supplementary material for this article can be found online at: https://www.frontiersin.org/articles/10.3389/fmicb.2023.1233605/full#supplementary-material
References
Alain, K., Harder, J., Widdel, F., and Zengler, K. (2012). Anaerobic utilization of toluene by marine alpha- and gammaproteobacteria reducing nitrate. Microbiology 158, 2946–2957. doi: 10.1099/mic.0.061598-0
Arndt, D., Xia, J., Liu, Y., Zhou, Y., Guo, A. C., Cruz, J. A., et al. (2012). METAGENassist: a comprehensive web server for comparative metagenomics. Nucleic Acids Res. 40, W88–W95. doi: 10.1093/nar/gks497
Atekwana, E. A., Atekwana, E. A., Rowe, R. S., Werkema, D. D., and Legall, F. D. (2004). The relationship of total dissolved solids measurements to bulk electrical conductivity in an aquifer contaminated with hydrocarbon. J. Appl. Geophys. 56, 281–294. doi: 10.1016/j.jappgeo.2004.08.003
Barnhart, E. P., De León, K. B., Ramsay, B. D., Cunningham, A. B., and Fields, M. W. (2013). Investigation of coal-associated bacterial and archaeal populations from a diffusive microbial sampler (DMS). Int. J. Coal Geol. 115, 64–70. doi: 10.1016/j.coal.2013.03.006
Barnhart, E. P., Weeks, E. P., Jones, E. J., Ritter, D. J., McIntosh, J. C., Clark, A. C., et al. (2016). Hydrogeochemistry and coal-associated bacterial populations from a methanogenic coal bed. International Journal of Coal Geology, 162, 14–26.
Beckmann, S., Lueders, T., Krüger, M., von Netzer, F., Engelen, B., and Cypionka, H. (2011). Acetogens and acetoclastic Methanosarcinales govern methane formation in abandoned coal mines. Appl. Environ. Microbiol. 77, 3749–3756. doi: 10.1128/AEM.02818-10
Bernard, B. B., Brooks, J. M., and Sackett, W. M. (1976). Natural gas seepage in the Gulf of Mexico. Earth Planet. Sci. Lett. 31, 48–54. doi: 10.1016/0012-821X(76)90095-9
Castro, A. R., Martins, G., Salvador, A. F., and Cavaleiro, A. J. (2022). Iron compounds in anaerobic degradation of petroleum hydrocarbons: a review. Microorganisms 10:2142. doi: 10.3390/microorganisms10112142
Colosimo, F., Thomas, R., Lloyd, J. R., Taylor, K. G., Boothman, C., Smith, A. D., et al. (2016). Biogenic methane in shale gas and coal bed methane: a review of current knowledge and gaps. Int. J. Coal Geol. 165, 106–120. doi: 10.1016/j.coal.2016.08.011
Davidova, I. A., Marks, C. R., and Suflita, J. M. (2018). “Anaerobic hydrocarbon-degrading deltaproteobacteria” in Taxonomy, genomics and ecophysiology of hydrocarbon-degrading microbes. ed. T. J. McGenity (Cham: Springer International Publishing), 1–38.
Davis, K. J., and Gerlach, R. (2018). Transition of biogenic coal-to-methane conversion from the laboratory to the field: a review of important parameters and studies. Int. J. Coal Geol. 185, 33–43. doi: 10.1016/j.coal.2017.11.006
Dhariwal, A., Chong, J., Habib, S., King, I. L., Agellon, L. B., and Xia, J. (2017). Microbiome analyst: a web-based tool for comprehensive statistical, visual and meta-analysis of microbiome data. Nucleic Acids Res. 45, W180–W188. doi: 10.1093/nar/gkx295
Do, Y. S., Schmidt, T. M., Zahn, J. A., Boyd, E. S., Mora, A. de la, and DiSpirito, A. A. (2003). Role of Rhodobacter sp. strain PS9, a purple non-sulfur photosynthetic bacterium isolated from an anaerobic swine waste lagoon, in odor remediation. Appl. Environ. Microbiol. 69, 1710–1720. doi: 10.1128/AEM.69.3.1710-1720.2003
Fallgren, P. H., Jin, S., Zeng, C., Ren, Z., Lu, A., and Colberg, P. J. S. (2013). Comparison of coal rank for enhanced biogenic natural gas production. Int. J. Coal Geol. 115, 92–96. doi: 10.1016/j.coal.2013.01.014
Flores, R. M., Rice, C. A., Stricker, G. D., Warden, A., and Ellis, M. S. (2008). Methanogenic pathways of coal-bed gas in the Powder River Basin, United States: the geologic factor. Int. J. Coal Geol. 76, 52–75. doi: 10.1016/j.coal.2008.02.005
Green, M. S., Flanegan, K. C., and Gilcrease, P. C. (2008). Characterization of a methanogenic consortium enriched from a coalbed methane well in the Powder River Basin, U.S.A. Int. J. Coal Geol. 76, 34–45. doi: 10.1016/j.coal.2008.05.001
Gründger, F., Jiménez, N., Thielemann, T., Straaten, N., Lüders, T., Richnow, H.-H., et al. (2015). Microbial methane formation in deep aquifers of a coal-bearing sedimentary basin, Germany. Front. Microbiol. 6:200. doi: 10.3389/fmicb.2015.00200
Guo, H., Liu, R., Yu, Z., Zhang, H., Yun, J., Li, Y., et al. (2012). Pyrosequencing reveals the dominance of methylotrophic methanogenesis in a coal bed methane reservoir associated with Eastern Ordos Basin in China. Int. J. Coal Geol. 93, 56–61. doi: 10.1016/j.coal.2012.01.014
Guo, H., Yu, Z., Thompson, I. P., and Zhang, H. (2014). A contribution of hydrogenotrophic methanogenesis to the biogenic coal bed methane reserves of Southern Qinshui Basin, China. Appl. Microbiol. Biotechnol. 98, 9083–9093. doi: 10.1007/s00253-014-5908-z
Harirchi, S., Wainaina, S., Sar, T., Nojoumi, S. A., Parchami, M., Parchami, M., et al. (2022). Microbiological insights into anaerobic digestion for biogas, hydrogen or volatile fatty acids (VFAs): a review. Bioengineered 13, 6521–6557. doi: 10.1080/21655979.2022.2035986
Hoang, L., Phung, T. T., Urynowicz, M., To, K. A., Le, Q. H., Huang, Z., et al. (2021). First investigation of microbial diversity and biogenic methane potential in coal mines located in the Red River Basin, Vietnam. Int. J. Coal Geol. 234:103674. doi: 10.1016/j.coal.2020.103674
Hoehler, T., Gunsalus, R. P., and McInerney, M. J. (2010). Environmental constraints that limit methanogenesis. In Handbook of hydrocarbon and lipid microbiology. Eds T. McGenity, J. R. Meervan der, and V Lorenzode. (pp. 635–654). Springer: Berlin.
Kadnikov, V. V., Mardanov, A. V., Beletsky, A. V., Karnachuk, O. V., and Ravin, N. V. (2019). Genome of the candidate phylum aminicenantes bacterium from a deep subsurface thermal aquifer revealed its fermentative saccharolytic lifestyle. Extremophiles 23, 189–200. doi: 10.1007/s00792-018-01073-5
Kinnon, E. C. P., Golding, S. D., Boreham, C. J., Baublys, K. A., and Esterle, J. S. (2010). Stable isotope and water quality analysis of coal bed methane production waters and gases from the Bowen Basin, Australia. Int. J. Coal Geol. 82, 219–231. doi: 10.1016/j.coal.2009.10.014
Klein, D. A., Flores, R. M., Venot, C., Gabbert, K., Schmidt, R., Stricker, G. D., et al. (2008). Molecular sequences derived from paleocene fort union formation coals vs. associated produced waters: implications for CBM regeneration. Int. J. Coal Geol. 76, 3–13. doi: 10.1016/j.coal.2008.05.023
Kobayashi, H., Kawaguchi, H., Endo, K., Mayumi, D., Sakata, S., Ikarashi, M., et al. (2012). Analysis of methane production by microorganisms indigenous to a depleted oil reservoir for application in microbial enhanced oil recovery. J. Biosci. Bioeng. 113, 84–87. doi: 10.1016/j.jbiosc.2011.09.003
Lawson, C. E., Strachan, C. R., Williams, D. D., Koziel, S., Hallam, S. J., and Budwill, K. (2015). Patterns of endemism and habitat selection in coalbed microbial communities. Appl. Environ. Microbiol. 81, 7924–7937. doi: 10.1128/AEM.01737-15
Li, D., Hendry, P., and Faiz, M. (2008). A survey of the microbial populations in some Australian coalbed methane reservoirs. Int. J. Coal Geol. 76, 14–24. doi: 10.1016/j.coal.2008.04.007
Liu, B., Wang, Y., Li, Y., Yang, Y., Chen, J., and Zhang, T. (2022). Improved formation of biogenic methane by cultivable bacteria in highly volatile bituminous coals. J. Clean. Prod. 366:132900. doi: 10.1016/j.jclepro.2022.132900
Liu, Z., Frigaard, N.-U., Vogl, K., Iino, T., Ohkuma, M., Overmann, J., et al. (2012). Complete genome of Ignavibacterium album, a metabolically versatile, flagellated, facultative anaerobe from the phylum chlorobi. Front. Microbiol. 3:185. doi: 10.3389/fmicb.2012.00185
Margesin, R., Fonteyne, P.-A., and Redl, B. (2005). Low-temperature biodegradation of high amounts of phenol by Rhodococcus spp. and Basidiomycetous yeasts. Res. Microbiol. 156, 68–75. doi: 10.1016/j.resmic.2004.08.002
Mayumi, D., Mochimaru, H., Yoshioka, H., Sakata, S., Maeda, H., Miyagawa, Y., et al. (2011). Evidence for syntrophic acetate oxidation coupled to hydrogenotrophic methanogenesis in the high-temperature petroleum reservoir of Yabase oil field (Japan). Environ. Microbiol. 13, 1995–2006. doi: 10.1111/j.1462-2920.2010.02338.x
Mehboob, F., Oosterkamp, M. J., Koehorst, J. J., Farrakh, S., Veuskens, T., Plugge, C. M., et al. (2016). Genome and proteome analysis of P seudomonas chloritidismutans AW-1 T that grows on n-decane with chlorate or oxygen as electron acceptor. Environ. Microbiol. 18, 3247–3257. doi: 10.1111/1462-2920.12880
Meslé, M., Dromart, G., and Oger, P. (2013). Microbial methanogenesis in subsurface oil and coal. Res. Microbiol. 164, 959–972. doi: 10.1016/j.resmic.2013.07.004
Midgley, D. J., Hendry, P., Pinetown, K. L., Fuentes, D., Gong, S., Mitchell, D. L., et al. (2010). Characterisation of a microbial community associated with a deep, coal seam methane reservoir in the Gippsland Basin, Australia. Int. J. Coal Geol. 82, 232–239. doi: 10.1016/j.coal.2010.01.009
Mochimaru, H., Yoshioka, H., Tamaki, H., Nakamura, K., Kaneko, N., Sakata, S., et al. (2007). Microbial diversity and methanogenic potential in a high temperature natural gas field in Japan. Extremophiles 11, 453–461. doi: 10.1007/s00792-006-0056-8
Nazaries, L., Murrell, J. C., Millard, P., Baggs, L., and Singh, B. K. (2013). Methane, microbes and models: fundamental understanding of the soil methane cycle for future predictions. Environ. Microbiol. 15, 2395–2417. doi: 10.1111/1462-2920.12149
Ollivier, B., Smiti, N., and Garcia, J. L. (1985). Thermophilic methanogenesis from sugar beet pulp by a defined mixed bacterial culture. Biotechnol. Lett. 7, 847–852. doi: 10.1007/BF01025568
Palacios, P. A., Francis, W. R., and Rotaru, A.-E. (2021). A win-loss interaction on Fe(0) between methanogens and Acetogens from a climate Lake. Front. Microbiol. 12:638282. doi: 10.3389/fmicb.2021.638282
Park, S. Y., and Liang, Y. (2016). Biogenic methane production from coal: a review on recent research and development on microbially enhanced coalbed methane (MECBM). Fuel 166, 258–267. doi: 10.1016/j.fuel.2015.10.121
Pavan, M. E., Pavan, E. E., Kämpfer, P., Pettinari, M. J., and López, N. I. (2019). “Coprothermobacteria” in Bergey’s manual of systematics of archaea and bacteria. ed. W. B. Whitman (Chichester: Wiley)
Penner, T. J., Foght, J. M., and Budwill, K. (2010). Microbial diversity of western Canadian subsurface coal beds and methanogenic coal enrichment cultures. Int. J. Coal Geol. 82, 81–93. doi: 10.1016/j.coal.2010.02.002
Rajeev, A. C., Sahu, N., Arvind, K., Deori, M., Grace, T., Dev, S. A., et al. (2021). Exploring prevalence of potential pathogens and fecal indicators in geographically distinct river systems through comparative metagenomics. Environ. Pollut. 282:117003. doi: 10.1016/j.envpol.2021.117003
Rathi, R., Lavania, M., Sawale, M., Kukreti, V., Kumar, S., and Lal, B. (2015). Stimulation of an indigenous thermophillic anaerobic bacterial consortium for enhanced oil recovery. RSC Adv. 5, 88115–88124. doi: 10.1039/C5RA10489K
Rathi, R., Lavania, M., Singh, N., Sarma, P. M., Kishore, P., Hajra, P., et al. (2019). Evaluating indigenous diversity and its potential for microbial methane generation from thermogenic coal bed methane reservoir. Fuel 250, 362–372. doi: 10.1016/j.fuel.2019.03.125
Rice, D. D., and Claypool, G. E. (1981). Generation, accumulation, and resource potential of biogenic gas. AAPG Bull. 65, 5–25. doi: 10.1306/2F919765-16CE-11D7-8645000102C1865D
Ritter, D., Vinson, D., Barnhart, E., Akob, D. M., Fields, M. W., Cunningham, A. B., et al. (2015). Enhanced microbial coalbed methane generation: a review of research, commercial activity, and remaining challenges. Int. J. Coal Geol. 146, 28–41. doi: 10.1016/j.coal.2015.04.013
Schoell, M. (1980). The hydrogen and carbon isotopic composition of methane from natural gases of various origins. Geochim. Cosmochim. Acta 44, 649–661. doi: 10.1016/0016-7037(80)90155-6
Sela-Adler, M., Ronen, Z., Herut, B., Antler, G., Vigderovich, H., Eckert, W., et al. (2017). Co-existence of methanogenesis and sulfate reduction with common substrates in sulfate-rich estuarine sediments. Front. Microbiol. 8:766. doi: 10.3389/fmicb.2017.00766
Shimizu, S., Akiyama, M., Naganuma, T., Fujioka, M., Nako, M., and Ishijima, Y. (2007). Molecular characterization of microbial communities in deep coal seam groundwater of northern Japan. Geobiology 5, 423–433. doi: 10.1111/j.1472-4669.2007.00123.x
Singh, D. N., Kumar, A., Sarbhai, M. P., and Tripathi, A. K. (2012). Cultivation-independent analysis of archaeal and bacterial communities of the formation water in an Indian coal bed to enhance biotransformation of coal into methane. Appl. Microbiol. Biotechnol. 93, 1337–1350. doi: 10.1007/s00253-011-3778-1
Singh, D. N., and Tripathi, A. K. (2013). Coal induced production of a rhamnolipid biosurfactant by Pseudomonas stutzeri, isolated from the formation water of Jharia coalbed. Bioresour. Technol. 128, 215–221. doi: 10.1016/j.biortech.2012.10.127
Stolper, D. A., Lawson, M., Davis, C. L., Ferreira, A. A., Neto, E. V. S., Ellis, G. S., et al. (2014). Formation temperatures of thermogenic and biogenic methane. Science 344, 1500–1503. doi: 10.1126/science.1254509
Storesund, J. E., Lanzèn, A., Nordmann, E.-L., Armo, H. R., Lage, O. M., and Øvreås, L. (2020). Planctomycetes as a vital constituent of the microbial communities inhabiting different layers of the meromictic Lake Sælenvannet (Norway). Microorganisms 8:1150. doi: 10.3390/microorganisms8081150
Strąpoć, D., Mastalerz, M., Dawson, K., Macalady, J., Callaghan, A. V., Wawrik, B., et al. (2011). Biogeochemistry of microbial coal-bed methane. Annu. Rev. Earth Planet. Sci. 39, 617–656. doi: 10.1146/annurev-earth-040610-133343
Strąpoć, D., Picardal, F. W., Turich, C., Schaperdoth, I., Macalady, J. L., Lipp, J. S., et al. (2008). Methane-producing microbial community in a coal bed of the illinois basin. Appl. Environ. Microbiol. 74, 2424–2432. doi: 10.1128/AEM.02341-07
Sudiana, I. M., Idris, I., Napitupulu, T. P., Ikhwani, A. Z. N., Sumerta, I. N., Sugiharto, A., et al. (2022). Diversity of hydrocarbon-degrading bacteria in Pulau Pari and their potential roles for bioremediation. IOP Conf. Ser. Earth Environ. Sci. 950:012013. doi: 10.1088/1755-1315/950/1/012013
Tukanghan, W., Hupfauf, S., Gómez-Brandón, M., Insam, H., Salvenmoser, W., Prasertsan, P., et al. (2021). Symbiotic bacteroides and clostridium-rich methanogenic consortium enhanced biogas production of high-solid anaerobic digestion systems. Bioresource Technol. Rep. 14:100685. doi: 10.1016/j.biteb.2021.100685
Vedanti, N., Vadapalli, U., and Sain, K. (2020). A brief overview of CBM development in India. Proc. Indian Natl. Sci. Acad. 86, 623–629. doi: 10.16943/ptinsa/2020/49799
Vishal, V., Singh, L., Pradhan, S. P., Singh, T. N., and Ranjith, P. G. (2013). Numerical modeling of Gondwana coal seams in India as coalbed methane reservoirs substituted for carbon dioxide sequestration. Energy 49, 384–394. doi: 10.1016/j.energy.2012.09.045
Wang, C., Michalet, R., Liu, Z., Jiang, X., Wang, X., Zhang, G., et al. (2020). Disentangling large- and small-scale abiotic and biotic factors shaping soil microbial communities in an alpine cushion plant system. Front. Microbiol. 11:925. doi: 10.3389/fmicb.2020.00925
Wang, H., Lin, H., Rosewarne, C. P., Li, D., Gong, S., Hendry, P., et al. (2016). Enhancing biogenic methane generation from a brown coal by combining different microbial communities. Int. J. Coal Geol. 154-155, 107–110. doi: 10.1016/j.coal.2015.12.006
Wang, J., and Yin, Y. (2021). Clostridium species for fermentative hydrogen production: an overview. Int. J. Hydrog. Energy 46, 34599–34625. doi: 10.1016/j.ijhydene.2021.08.052
Wang, Q., Guo, H., Wang, H., Urynowicz, M. A., Hu, A., Yu, C.-P., et al. (2019). Enhanced production of secondary biogenic coalbed natural gas from a subbituminous coal treated by hydrogen peroxide and its geochemical and microbiological analyses. Fuel 236, 1345–1355. doi: 10.1016/j.fuel.2018.09.114
Wawrik, B., Mendivelso, M., Parisi, V. A., Suflita, J. M., Davidova, I. A., Marks, C. R., et al. (2012). Field and laboratory studies on the bioconversion of coal to methane in the San Juan Basin. FEMS Microbiol. Ecol. 81, 26–42. doi: 10.1111/j.1574-6941.2011.01272.x
Wei, M., Yu, Z., Jiang, Z., and Zhang, H. (2014). Microbial diversity and biogenic methane potential of a thermogenic-gas coal mine. Int. J. Coal Geol. 134-135, 96–107. doi: 10.1016/j.coal.2014.09.008
Wen, X., Yang, S., Horn, F., Winkel, M., Wagner, D., and Liebner, S. (2017). Global biogeographic analysis of methanogenic Archaea identifies community-shaping environmental factors of natural environments. Front. Microbiol. 8:1339. doi: 10.3389/fmicb.2017.01339
Whiticar, M. J. (1999). Carbon and hydrogen isotope systematics of bacterial formation and oxidation of methane. Chem. Geol. 161, 291–314. doi: 10.1016/S0009-2541(99)00092-3
Zhang, J., Anderson, K., Britt, D., and Liang, Y. (2018). Sustaining biogenic methane release from Illinois coal in a fermentor for one year. Fuel 227, 27–34. doi: 10.1016/j.fuel.2018.04.061
Zhang, X., Kong, D., Liu, X., Xie, H., Lou, X., and Zeng, C. (2021). Combined microbial degradation of crude oil under alkaline conditions by Acinetobacter baumannii and Talaromyces sp. Chemosphere 273:129666. doi: 10.1016/j.chemosphere.2021.129666
Keywords: coalbed methane (CBM), methanogenesis, microbial community, biogenic CBM enhancement, Raniganj coal block
Citation: Chawla M, Lavania M, Sahu N, Shekhar S, Singh N, More A, Iyer M, Kumar S, Singh K and Lal B (2023) Culture-independent assessment of the indigenous microbial diversity of Raniganj coal bed methane block, Durgapur. Front. Microbiol. 14:1233605. doi: 10.3389/fmicb.2023.1233605
Edited by:
Amelia-Elena Rotaru, University of Southern Denmark, DenmarkReviewed by:
Renxing Liang, Princeton University, United StatesSabrina Beckmann, Oklahoma State University, United States
Copyright © 2023 Chawla, Lavania, Sahu, Shekhar, Singh, More, Iyer, Kumar, Singh and Lal. This is an open-access article distributed under the terms of the Creative Commons Attribution License (CC BY). The use, distribution or reproduction in other forums is permitted, provided the original author(s) and the copyright owner(s) are credited and that the original publication in this journal is cited, in accordance with accepted academic practice. No use, distribution or reproduction is permitted which does not comply with these terms.
*Correspondence: Meeta Lavania, bWVldGFsQHRlcmkucmVzLmlu