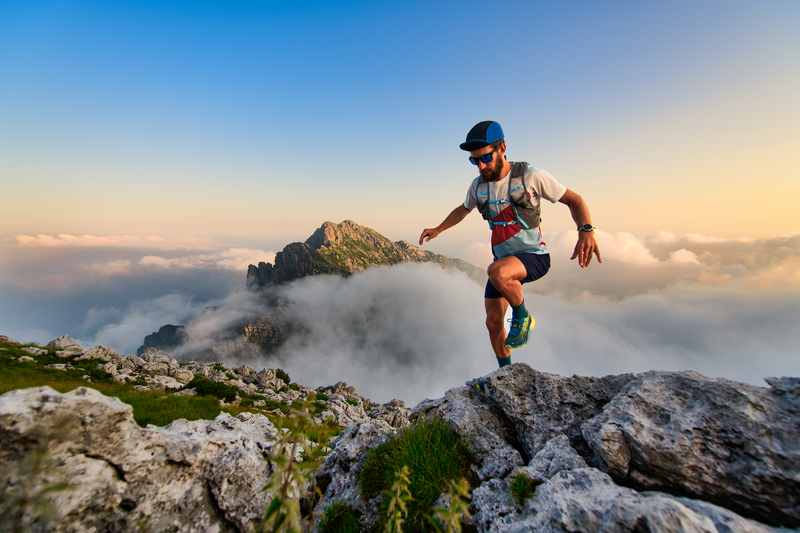
94% of researchers rate our articles as excellent or good
Learn more about the work of our research integrity team to safeguard the quality of each article we publish.
Find out more
PERSPECTIVE article
Front. Microbiol. , 02 August 2023
Sec. Biology of Archaea
Volume 14 - 2023 | https://doi.org/10.3389/fmicb.2023.1233221
This article is part of the Research Topic The Methane Moment – Cross-Boundary Significance of Methanogens View all 20 articles
Methanogenic archaea stand out as multipurpose biocatalysts for different applications in wide-ranging industrial sectors due to their crucial role in the methane (CH4) cycle and ubiquity in natural environments. The increasing demand for raw materials required by the manufacturing sector (i.e., metals-, concrete-, chemicals-, plastic- and lubricants-based industries) represents a milestone for the global economy and one of the main sources of CO2 emissions. Recovery of critical raw materials (CRMs) from byproducts generated along their supply chain, rather than massive mining operations for mineral extraction and metal smelting, represents a sustainable choice. Demand for lithium (Li), included among CRMs in 2023, grew by 17.1% in the last decades, mostly due to its application in rechargeable lithium-ion batteries. In addition to mineral deposits, the natural resources of Li comprise water, ranging from low Li concentrations (seawater and freshwater) to higher ones (salt lakes and artificial brines). Brines from water desalination can be high in Li content which can be recovered. However, biological brine treatment is not a popular methodology. The methanogenic community has already demonstrated its ability to recover several CRMs which are not essential to their metabolism. Here, we attempt to interconnect the well-established biomethanation process with Li recovery from brines, by analyzing the methanogenic species which may be suitable to grow in brine-like environments and the corresponding mechanism of recovery. Moreover, key factors which should be considered to establish the techno-economic feasibility of this process are here discussed.
The wide diversity and distribution of methanogens, unicellular obligate anaerobes from the Archaea domain, make them suitable for multiple biotechnological applications beyond high-energy fuel production, i.e., methane CH4 (Pfeifer et al., 2021; Bellini et al., 2022; Carr and Buan, 2022; Contreras et al., 2022; Lyu et al., 2022). Biomining and biohydrometallurgy exploit microorganisms for metal extraction and recovery from different resources such as mineral rocks, mine waste, electric and electronic waste (e-waste), new- and old-scrap metals generated during the device manufacturing and at end-of-life, respectively (Kaksonen et al., 2020; Magoda and Mekuto, 2022; Abdel Azim et al., 2023). Methanogens, in the form of consortia, have already demonstrated the ability to recover platinum group metals (PGMs) such as platinum (Pt) and palladium (Pd) (Pat-Espadas et al., 2016; Simon-Pascual et al., 2018). As a single culture, the hydrogenotrophic methanogen Methanobacterium bryatii BKYH was found to be able of chelating copper (Cu2+) from Cu-rich mineral deposits (Kim et al., 1995), while Methanothermobacter thermoautotrophicus could recover vanadium (V4+), chromium (Cr3+) and cobalt (Co2+) via bioreduction, an immobilization process which changes the oxidation state of dissolved metals by donating electrons (Zhang et al., 2014; Singh et al., 2015a,b). In this context, biobased processes are of considerable interest, being economically convenient and environmentally sustainable compared to the common techniques (Baniasadi et al., 2019). Indeed, material production typically relies on energy-consuming practices like mineral mining, processing, and refining (Intergovernmental Panel on Climate Change (IPCC), 2023). The ever-growing need for raw materials in the manufacturing industry is driving the exploration and development of alternative sources and technologies. However, the replacement of fossil-based technologies by renewable energy sources (RES) and the drive for electrification implies the exploitation of more raw materials (Zhang et al., 2023). Among raw materials, several are listed as critical (CRMs) due to their increasing demand but limited availability (Mosley, 2022; U.S. Geological Survey, 2022). Lithium (Li+), the demand of which is projected to grow by 32% within 2030 (Andreas et al., 2022), has been recently included among CRMs and in the strategic raw material (SRMs) list (European Commission, 2023). Li is intensively employed in single-discharge- and rechargeable-batteries construction (74%), (U.S. Geological Survey, 2022), used in electronic devices, electric (EVs) and hybrid vehicles, and smart grid factories. The demand for LIBs led the global Li production to grow from 82,500 tons in 2020 to almost 100,000 tons in 2021 along with a significant price increase of Li (as Li2CO3). However, beyond batteries, there are other well-settled applications of lithium such as ceramics and glass manufacturing, aluminum alloys for aerospace applications, as fuel in nuclear reactors (U.S. Geological Survey, 2022). Overall, the extraction procedure represents the main shortcoming in the Li supply chain in terms of energy and time demands, in addition to the use of strong reagents which makes this methodology poorly sustainable (Gruber et al., 2011; Meng et al., 2021). Total lithium resources globally account for 89 million tons (U.S. Geological Survey, 2022). Lithium only exists as salts or minerals (i.e., lithium carbonate, lithium chloride, spodumene, lepidolite, and petalite) due to its high reactivity. Hence, it can be found in hard rock ores and sedimentary rocks or water resources, including seawater and brines (Flexer et al., 2018; Baudino et al., 2022; Khalil et al., 2022; Barbosa et al., 2023). Natural brines, classified as geothermal, oilfield, and continental, are typically characterized by high salinity values with a mineral salt concentration range of 2.9–5.6 M (Flexer et al., 2018). Besides chloride Cl−, anions in brine include carbonates CO32−, sulfates SO22− and borates BO33− (Talens Peiró et al., 2013). The cationic fraction is mostly represented by sodium Na+, potassium K+, magnesium Mg2+, and calcium Ca2+ in addition to less abundant elements like Li+ (Flexer et al., 2018), rubidium (Rb+) and gallium (Ga3+) (del Villar et al., 2023). Li content in many brines is several hundred mgL-1 and few brines contain more than 1 gL−1 of Li (Kamienski et al., 2004). Dry lakes and salt aquifers (i.e., continental brines) hold the highest concentration of Li+, ranging between 20 and 1,500 mgL−1 (Barbosa et al., 2023). The concentration of Li in marine basins such as the Atlantic Ocean and the Dead Sea is 220 μgL−1 and 21 mgL−1, respectively (Barbosa et al., 2023). The Lithium Triangle in the Andean region among Chile, Bolivia, and Argentina accounts for up to 80% of the global lithium brine resources: among valuable commercial brines the Atacama salar in Chile has the highest lithium content besides bohrium and potassium (An et al., 2012; Ogawa et al., 2014). The current production capacity in the above-mentioned area is detained by two societies corresponding to 48,000 Li2CO3/6,000 LiCl and 27,000 Li2CO3/4500 LiCl tons per year, respectively (Flexer et al., 2018). Concentrated brines (NaCl >0.8 M), intended as the by-product of the water desalination process to produce clean water, also present massive concentrations of valuable minerals (five times the input seawater) in comparison to other brine sources (Khalil et al., 2022; Prasad, 2023). The number of elements in rejected brines varies based on the origin of the processed water: Li concentration in the Mediterranean Sea is higher than in the Atlantic Ocean but still lower than in underground brackish sources, i.e., formation waters or deep saline aquifers (del Villar et al., 2023). Hence, depending on the treated rejected water, the corresponding economic potential varies with the elemental composition (del Villar et al., 2023). The current brine production is 141.5 million m3 day−1 worldwide, 70.3 % of which is concentrated in the Middle East (Saudi Arabia, United Arab Emirates, and Kuwait) and North Africa regions. The brine is disposed directly into the ocean taking advantage of the proximity of the desalination plants to the coast, despite the related environmental risks and the volume of generated brine exceeding the volume of produced desalinated water by up to 50% (Jones et al., 2019). Although the cost estimation of Li extraction from rocks is nearly twice that of Li from brines, mineral mining is still the prevalent technology due to the limited offer of brines (Flexer et al., 2018; Meng et al., 2021). Among the existing studies on the recovery of Li as well as of other critical metals from secondary sources, bacteria (Işıldar et al., 2019; Naseri et al., 2019; Moazzam et al., 2021) and fungi (Amiri et al., 2012; Horeh et al., 2016; Bahaloo-Horeh and Mousavi, 2017) are the most represented microorganisms, while almost no data are available on the application of methanogens. Brines valorization is quite unpopular among the studies involving biological processes, though biosorption technologies are already widely applied for the treatment of other industrial wastewaters contaminated by heavy metals. Fungi, Algae and Bacteria have been broadly exploited as biosorbents (Dayana et al., 2013; Kanamarlapudi et al., 2018; Elgarahy et al., 2021; Kurniawan et al., 2023; Paper et al., 2023; Tripathi et al., 2023) compared to the Archaea whose utilization is less common (Calderón et al., 2013; Vítězová et al., 2020). Among the few studies reporting biobased treatment of brines, that of Mainka et al. investigated the use of halophilic bacteria for the degradation of organic compounds in waste brines with the goal to obtain a high-quality brine to be used as raw material (Mainka et al., 2022). The work by McAdam and Judd (2008) on the application of biological removal of anionic pollutants from concentrated waste brine on ion-exchange membranes for clean water generation should also be mentioned. In this panorama, the authors aim to open a discussion on the application of ad hoc methanogenic consortia for Li-brines treatment as a complementary or alternative strategy to other methodologies for industrial brine valorization. Possible mechanisms of Li recovery carried out by methanogens and the possibility to pair them with biomethanation is herein examined.
Microbial diversity is very high in hypersaline environments, with the salinity gradient being an important factor for microbial community composition and species diversity (McGenity and Sorokin, 2019). Redox potential and stable anaerobic conditions are key enablers for methanogenesis occurrence. Moreover, methanogens and sulfate-reducing bacteria (SRB; Barton and Fauque, 2022) are historically in competition for common electron donors such as H2, formate, and acetate, in the sulfate-methane transition zone (SMTZ), hence methanogenesis also depends on sulfate concentration. SRB becomes predominant when the level of sulfate is sufficiently high to be the final electron acceptor of the above-mentioned substrates. Conversely, methanogenesis is an important process in marine and hypersaline environments, like in deeper sediments poor in sulfates (Wilms et al., 2007) in highly hydrogen-productive areas (Hoehler et al., 2001 Buckley et al., 2008). Apart from Halobacteria class, methanogenic archaea living at concentration of NaCl >0.2 M have been identified as halophiles (Supplementary Table S1). Most of them belong to the Methanosarcinaceae family including Methanosarcina, Methanohalophilus, Methanohalobium, and Methanosalsum genera.
Additionally, Methanolobus oregonensis, an alkaliphilic, methylotrophic methanogen, is classified as halotolerant rather than halophilic (Liu et al., 1990) due to its optimal growth with salinity <0.2 M (Didari et al., 2020). Methylotrophic methanogens cannot grow on hydrogen (H2) and carbon dioxide (CO2) or acetate, rather they use non-competitive molecules such as methanol, methylated amines and methylated sulfide as electron acceptors and formate or H2 as electron donors (Sorokin et al., 2018) to produce methane and gain energy (Oren, 1999). Methanosalis sp. SBSPR1A, a closely related taxon in the Methanolobus and Methanomethylovorans genera, is a methylotrophic methanogen tolerating up to 3.6 M of salinity and performing methanogenesis only from dimethylamine and trimethylamine (Bueno de Mesquita et al., 2021). Methylated amines, particularly trimethylamines, originate from glycine betaine fermentation (Welsh, 2000). Quaternary amines like glycine betaine and choline can be directly used as substrates in methanogenesis by some marine strains (i.e., genus Methanococcoides) without the need for syntrophic metabolism. However, only partial degradation of glycine betaine to dimethylglycine (DMG) has been reported in hypersaline environments (Watkins et al., 2014). A possible explanation is that these molecules also act as compatible solutes, i.e., substances fitting with microbial metabolism that accumulates in the cytoplasm to balance external osmotic pressure (McGenity and Sorokin, 2019). Two main strategies to achieve microbial osmoregulation and survival in hypersaline environments have been recognized: the salt-in and the salt-out mechanism. The former is typically used by Haloarchaea and involves the rise of salt concentrations in the cytoplasm, usually with potassium chloride (KCl; Bueno de Mesquita et al., 2021). The latter, typically used by bacteria, involves the production of compatible solutes thus avoiding salt secretion in the cytoplasm, as described in Halobacteriales. Other methyl-reducing methanogens have been identified as Methanonatronarchaeum thermophilum and Candidatus Methanohalarchaeum thermophilum, formerly related to the Halobacteria from neutral salt lakes and highly alkaline soda lakes (Sorokin et al., 2017, 2018). In saline environments, a hybrid methanogenic pathway, which uses C1-methylated compounds as electron acceptors and H2 as an electron donor (i.e., methyl-reduction route) can be predominant (Borrel et al., 2014). This is the case of the Methanomassiliicoccus genus typically found in insect/animal digestive tracts and performing a methyl-dependent hydrogenotrophic methanogenesis (Cozannet et al., 2021); this was detected in a smooth hypersaline microbial mat from Shark Bay (Wong et al., 2017; García-Maldonado et al., 2018). Although methanogenesis in hypersaline environments is typically ascribed to methylotrophic methanogens, recent studies have reported evidence of putative hydrogenotrophic methanogens presence (i.e., methanogens reducing CO2 to CH4 using H2 or formate) in hypersaline microbial mats and endoevaporite (García-Maldonado et al., 2015, 2018; Wong et al., 2017). Methanogens from Methanobacteriales, Methanococcales, Methanopyrales orders were identified in samples from these environments. Among hydrogenotrophic methanogens, Methanocalculus genera are representative of halophiles living in highly alkaline environments. Methanocalculus halotolerans was instead isolated from a hypersaline oil reservoir, with the ability to grow at up to 2 M of salinity (Ollivier et al., 1998). Representatives of the genus Methanothermobacter was enriched in the formation waters of a gas field, showing tolerance to salinity up to 1.5 M (Gray et al., 2009). Besides Methanosarcina, other abundant methanogenic communities found in anaerobic treatment plants of diary wastewaters characterized by elevated salt concentrations (Vítězová et al., 2020) correspond to the hydrogenotrophic Methanocorpusculum, Methanobrevibacter, Methanobacterium and Methanoculleus genera (Zeb et al., 2019).
Taxa belonging to the archaeal kingdom are characterized by a heterogenic organization and composition of the cell membrane, although they all have in common the lack of peptidoglycan (König et al., 2014) and a lipid belayer consisting of C5-isoprenoid units linked to glycerol via ether bonds (Klingl, 2014). Almost all archaea own a protein surface layer known as the S-layer with different lattice structures. Among halophilic archaea, methanogens share the same S-layer configuration (i.e., hexagonal lattice type). Besides allowing access to nutrients, the S-layer has a cell-protective and stabilizing role in environments with extreme salinity, temperature, and pH (Rodrigues-Oliveira et al., 2017). Studies conducted on a modelled S-layer structure belonging to Methanosarcina acetivorans, demonstrated the role of the S-layer as a charge and size barrier preventing the access of specific molecules (Arbing et al., 2012). Selectivity for specific-target metals is a desirable quality in metal-rich waste separation and recovery technologies (Echavarri-Bravo et al., 2022). Among the methanogens, the hyperthermophilic strain Methanocaldococcus jannaschii has been reported to selectively adsorb dissolved Fe3+, Ca2+, Zn2+, Cu2+, and Pb2+ metal cations (Orange et al., 2011) due to the presence of negatively charged functional groups on the cell membrane.
In a metal-rich environment, extracellular polymeric substance (EPS) production is part of a stress-response mechanism to support the cell in reducing the metal ions availability, by chelation and sequestration as an ion exchange matrix. The EPS matrix behaves like a gel-like grid that keeps microbial cells together, supporting biofilms' adhesion on surfaces and protecting the cells from extreme environments (van Wolferen et al., 2018; Li et al., 2022; Wang et al., 2022). Carboxyl, hydroxyl, sulfate, phosphoryl, and amino groups of protein in EPS are responsible for metals biosorption (Torres, 2020). For instance, dark deposits of Pb2+ ions found around Methanocaldococcus jannaschii cells suggested a mechanism of particle fixation by the EPS (Orange et al., 2011). The study by Kurniawan and Yamamoto gave us fundamental information about the biosorption power of a natural biofilm matrix isolated from a Japanese lake: Li+ biosorption is a physicochemical process mainly driven by the electrostatic interaction between ion species and the negatively charged sites of the proteins in the biofilm (Kurniawan and Yamamoto, 2015). Moreover, the adsorption of Li+ corresponded to the parallel desorption of other cations (i.e., Na+, Mg2+, Ca2+ and K+) via an ion exchange mechanism. The biosorption process observed in this study was fast (1 min) and more performing (85 μmol g−1 of dry biofilm) than strong and weak cation exchange resins (18 and 33 μmol g−1, respectively). Concerning the use of active biomass, both bacteria and fungi showed a superior uptake capacity in the magnitude of tens and hundreds mg g−1 of dissolved metals (Srinath et al., 2002; Iram et al., 2015). As an example of industrial application, Artola and colleagues designed and operated a biosorption pilot plant for Cu2+ removal from municipal water treatment plant using anaerobic sludge as biosorbent (Artola et al., 2001). The highest uptake capacity was 75 mg of metal g−1 of total solids in the sludge. Pagliaccia and coworkers investigated the efficiency of EPS in native biomass from annamox granular sludge as biosorbent of heavy metals in synthetic wastewaters (Pagliaccia et al., 2022). A recent study on a methanogenic consortium revealed the relationship of EPS with the release of soluble biogenic products and with metal solubility in the presence of elevated cobalt (Co2+) and nickel (Ni2+) concentrations, as in waste streams of metallurgical and LIBs industry (Hasani Zadeh et al., 2022). Hydroxyl and carboxyl terminals of proteins in EPS are the main ones responsible for the metal-cations biosorption mechanism (Fomina and Gadd, 2014; Kurniawan and Yamamoto, 2015; Liu et al., 2015) because cationic species are predominant among metals in aqueous solutions. This means that pH around 7-8 is the most suitable range for ensuring a negative charge on the protein terminals that bind dissolved metals (Torres, 2020). The concentration of dispersed metals is dependent on EPS protein content whose variation is caused by metal-induced stress, e.g., concentrations of essential or non-essential metals that exceed the cells requirement. For instance, the activity of a methanogenic consortium in an anaerobic granular sludge was compromised by both Ni and Co as reported by Hasani Zadeh and colleagues. Moreover, the presence of a Ni-protein complex proved the selective metal-binding based on the ligand affinity in metalloproteins (Hasani Zadeh et al., 2022).
EPS can also host biotransformation process as it is for Methanococcus maripaludis OS7 producing an extracellular Ni-Fe hydrogenase that oxidizes iron of carbon steel oil and gas pipelines. The hydrogenase has the function of producing hydrogen and triggering the microbially influenced corrosion phenomenon (Lahme et al., 2021). Although archaeal EPS do not have a relevant role at the industrial level yet, its importance is progressively growing. EPS production in archaea (i.e., Halobacterium mediterranei) is currently estimated to be at TRL 2, based on (Pfeifer et al., 2021). There are different strategies to improve the microbial recovery mechanisms with the purpose of transferring this technology to the industrial scale such as surface-culture immobilization (fixation, entrapment, and chemicals cross-linking) and optimal conditions for process implementation (e.g., temperature, pH, initial dissolved metals, biosorbent concentration, i.e., biomass or EPS concentrations, biosorbent/metal contact time; Fomina and Gadd, 2014). As a successful example is worth to mention the study by Manasi and colleagues using a halophilic bacterium Halomonas BVR 1 in combination with reduced graphene oxide to remove Cd, Zn and Pb from real effluent from electronic manufacturing sector: metals removal efficiency achieved 98% (Manasi et al., 2018).
In addition to the biosorption mechanism, which is not necessarily dependent on living microorganisms, metals recovery can also occur via bioaccumulation or else through metals uptake via passive or active transport trough the cell membrane, and biotransformation and bioprecipitation, which instead involve active cells (Gavrilescu, 2022; Liapun and Motola, 2023).
Brine disposal is an emerging environmental and economic issue not only for the drinking water supply chain, considering that 41% of the global population still does not have access to it, but also for the primary sector (e.g., agroforestry, zootechnic and mining) and secondary sector (e.g., metallurgy) where water is an essential resource. Therefore, desalination is expected to expand rapidly, and so brine production is associated with it. The ecological effect of direct brine discharging in surface water bodies is currently under discussion due to the related-potential physiochemical alteration and the associated threat to marine ecosystem and life. Hence, valorization of rejected brines rather than direct disposal represents the core of the future water-resources management. Extraction and recovery strategies of valuable metals from secondary sources must be expanded and implemented. Among the valuable metals lithium is particularly attractive because its demand is expected to increase enormously by 2030. Emerging technologies relying on biological approaches are very promising in terms of low cost and sustainability but require further investigation to enable their application on a large scale. Based on what is currently known, we suggest that natural-adapted consortia of methanogens could be exploited as a flexible platform for the selective recovery of Li and other critical metals from brines in a CO2-upcycling process (Figure 1). Thus, primary and/or secondary sectors emitting CO2 as a waste effluent represent a valuable source of carbon that supports the growth and productivity of methanogens. From an industrial point of view, examples of pilot and demonstration scale biomethanation plants are available in the literature with the technology being widely investigated (TRL >5). Even though biomethanation technologies appear mature and deployable, integration of Li recovery from brines would require the evaluation of some additional factors concerning resources and process operating conditions.
Figure 1. Li removal from rejected brine through biomethanation process. Sources, input, and output with possible destination are shown. The bioreactor design here is generic: biosorption at the industrial level has been investigated in stirred tank bioreactors, air lift bioreactors, fluidized bed bioreactors, and fixed bed bioreactors (Kanamarlapudi et al., 2018). Zoom in on the mechanisms enabling the recovery of metals in brine. RES, renewable energy sources; HMC, hydrogenotrophic methanogens consortia. Mn and Mn−1: charge of target metals.
In particular, future research should investigate the ability of methanogenic consortia to adapt and grow on brines as substrates while carrying out methanogenesis. It is indeed important to test the resistance to salinity stress and elements which are not essential to their metabolism (e.g., Li, Sr, F). In order to maintain stable biomethane generation provided levels of salinity and thus Li should be kept under the threshold of inhibition, thus dilution of brine might be required along with culture preadaptation steps. Moreover, the optimal conditions to favor selectivity toward specific metals should be explored with the view of scaling-up the bio-recovery process. When using mixed microbial communities defining the organisms actively contributing to metal recovery and their affinity towards the removal of different brine components should be considered to eventually develop a functional synthetic consortium. In this regard, the location of origin and the type of water resource should be considered as important factors affecting the bioprocess and its profitability due to the different elemental composition. Considerable attention should be paid also to the recovery mechanism (biosorption, bioaccumulation, and biotransformation) carried out by the involved methanogenic species (Figure 1) in order to define the best implementation strategies for microbial recovery optimization (e.g., cell-immobilization). This aspect is also crucial to evaluate and deploy technically and economically feasible downstream procedures for Li desorption from cells and possibly the regeneration of the biosorbent, i.e., active methanogens, which is required by the biomethanation process. Given the lack of knowledge on the biological recovery of CRMs from rejected brine even at the laboratory scale, a techno-economic assessment of the research and development target must be still explored in order to reveal the potential benefit of this process. However, the use of renewable sources, the CO2 mitigation and utilization, and eventually, the heat and water generated along with the production of CH4 and reused within the process itself, should be an added value contributing to the process feasibility.
The original contributions presented in the study are included in the article/Supplementary material, further inquiries can be directed to the corresponding author.
AA: conceptualization and writing—original draft preparation. AA, AV, RB, and IB: investigation and visualization. BM: supervision. FP: funding acquisition. AA, AV, RB, IB, LB, FP, FV, AL, and BM: writing—review and editing. All authors contributed to the article and approved the submitted version.
All figures presented in this work have been created or modified with BioRender.com.
The authors declare that the research was conducted in the absence of any commercial or financial relationships that could be construed as a potential conflict of interest.
All claims expressed in this article are solely those of the authors and do not necessarily represent those of their affiliated organizations, or those of the publisher, the editors and the reviewers. Any product that may be evaluated in this article, or claim that may be made by its manufacturer, is not guaranteed or endorsed by the publisher.
The Supplementary material for this article can be found online at: https://www.frontiersin.org/articles/10.3389/fmicb.2023.1233221/full#supplementary-material
Abdel Azim, A., Bellini, R., Vizzarro, A., Bassani, I., Pirri, C. F., and Menin, B. (2023). Highlighting the role of archaea in urban mine waste exploitation and valorisation. Recycling 8:20. doi: 10.3390/recycling8010020
Amiri, F., Mousavi, S. M., Yaghmaei, S., and Barati, M. (2012). Bioleaching kinetics of a spent refinery catalyst using Aspergillus Niger at optimal conditions. Biochem Eng J 67, 208–217. doi: 10.1016/j.bej.2012.06.011
An, J. W., Kang, D. J., Tran, K. T., Kim, M. J., Lim, T., and Tran, T. (2012). Recovery of lithium from Uyuni salar brine. Hydrometallurgy 117–118, 64–70. doi: 10.1016/j.hydromet.2012.02.008
Andreas, Breiter, Horetsky, Evan, Linder, Martin, and Rettig, Raphael (2022). Power Spike: How Battery Makers Can Respond to Surging Demand from EVs. Available at: https://www.mckinsey.com/capabilities/operations/our-insights/power-spike-how-battery-makers-can-respond-to-surging-demand-from-evs (Accessed January 13, 2023).
Arbing, M. A., Chan, S., Shin, A., Phan, T., Ahn, C. J., Rohlin, L., et al. (2012). Structure of the surface layer of the methanogenic archaean Methanosarcina acetivorans. Proc Natl Acad Sci U S A 109, 11812–11817. doi: 10.1073/pnas.1120595109
Artola, A., Martin, M. J., Balaguer, M., and Rigola, M. (2001). Pilot plant biosorption in an integrated contact-settling system: Application to Cu(II) removal by anaerobically digested sludge. J. Chem. Technol. Biotechnol. 76, 1141–1146. doi: 10.1002/jctb.496
Bahaloo-Horeh, N., and Mousavi, S. M. (2017). Enhanced recovery of valuable metals from spent lithium-ion batteries through optimization of organic acids produced by Aspergillus Niger. Waste Management 60, 666–679. doi: 10.1016/j.wasman.2016.10.034
Baniasadi, M., Vakilchap, F., Bahaloo-Horeh, N., Mousavi, S. M., and Farnaud, S. (2019). Advances in bioleaching as a sustainable method for metal recovery from e-waste: a review. J. Ind. Eng. Chem. 76, 75–90. doi: 10.1016/j.jiec.2019.03.047
Barbosa, H., Soares, A. M. V. M., Pereira, E., and Freitas, R. (2023). Lithium: a review on concentrations and impacts in marine and coastal systems. Sci. Total Environ. 857:159374. doi: 10.1016/j.scitotenv.2022.159374
Barton, L. L., and Fauque, G. D. (2022). Sulfate-Reducing Bacteria and Archaea. Cham: Springer International Publishing.
Baudino, L., Santos, C., Pirri, C. F., la Mantia, F., and Lamberti, A. (2022). Recent advances in the lithium recovery from water resources: from passive to electrochemical methods. Adv. Sci. 9:2201380. doi: 10.1002/advs.202201380
Bellini, R., Bassani, I., Vizzarro, A., Azim, A. A., Vasile, N. S., Pirri, C. F., et al. (2022). Biological aspects, advancements and techno-economical evaluation of biological methanation for the recycling and valorization of CO2. Energies (Basel) 15:4064. doi: 10.3390/en15114064
Borrel, G., Parisot, N., Harris, H. M., Peyretaillade, E., Gaci, N., Tottey, W., et al. (2014). Comparative genomics highlights the unique biology of Methanomassiliicoccales, a Thermoplasmatales-related seventh order of methanogenic archaea that encodes pyrrolysine. BMC Genomics 15:679. doi: 10.1186/1471-2164-15-679
Bueno de Mesquita, C. P., Zhou, J., Theroux, S. M., and Tringe, S. G. (2021). Methanogenesis and salt tolerance genes of a novel halophilic methanosarcinaceae metagenome-assembled genome from a former solar saltern. Genes (Basel) 12:1609. doi: 10.3390/genes12101609
Buckley, D. H., Baumgartner, L. K., and Visscher, P. T. (2008). Vertical distribution of methane metabolism in microbial mats of the Great Sippewissett Salt Marsh. Environ Microbiol. 10, 967–977. doi: 10.1111/j.1462-2920.2007.01517.x
Calderón, K., González-Martínez, A., Gómez-Silván, C., Osorio, F., Rodelas, B., and González-López, J. (2013). Archaeal diversity in biofilm technologies applied to treat urban and industrial wastewater: recent advances and future prospects. Int J Mol Sci 14, 18572–18598. doi: 10.3390/ijms140918572
Carr, S., and Buan, N. R. (2022). Insights into the biotechnology potential of methanosarcina. Front Microbiol 13:1034674. doi: 10.3389/fmicb.2022.1034674
Contreras, G., Thomsen, J., Pfitzer, M., Hafenbradl, D., Kostner, D., Holtmann, D., et al. (2022). New perspectives for biotechnological applications of methanogens. Curr Res Biotechnol 4, 468–474. doi: 10.1016/j.crbiot.2022.10.001
Cozannet, M., Borrel, G., Roussel, E., Moalic, Y., Allioux, M., Sanvoisin, A., et al. (2021). New insights into the ecology and physiology of methanomassiliicoccales from terrestrial and aquatic environments. Microorganisms 9, 1–31. doi: 10.3390/microorganisms9010030
Dayana, K., Sowjanya, C. V., and Ramachandramurthy, C. V. (2013). Eco-friendly remediation of industrial effluents via biosorption technology – an overview. Int. J. Eng. Res. Technol. 2, 1275–1284. doi: 10.17577/IJERTV2IS110328
del Villar, A., Melgarejo, J., García-López, M., Fernández-Aracil, P., and Montano, B. (2023). The economic value of the extracted elements from brine concentrates of Spanish desalination plants. Desalination 560:116678. doi: 10.1016/j.desal.2023.116678
Didari, M., Bagheri, M., Amoozegar, M. A., Bouzari, S., Babavalian, H., Tebyanian, H., et al. (2020). Diversity of halophilic and halotolerant bacteria in the largest seasonal hypersaline lake (Aran-Bidgol-Iran). J Environ Health Sci Eng 18, 961–971. doi: 10.1007/s40201-020-00519-3
Echavarri-Bravo, V., Amari, H., Hartley, J., Maddalena, G., Kirk, C., Tuijtel, M. W., et al. (2022). Selective bacterial separation of critical metals: towards a sustainable method for recycling lithium ion batteries. Green Chemistry 24, 8512–8522. doi: 10.1039/D2GC02450K
Elgarahy, A. M., Elwakeel, K. Z., Mohammad, S. H., and Elshoubaky, G. A. (2021). A critical review of biosorption of dyes, heavy metals and metalloids from wastewater as an efficient and green process. Clean Eng Technol 4:100209. doi: 10.1016/j.clet.2021.100209
Flexer, V., Baspineiro, C. F., and Galli, C. I. (2018). Lithium recovery from brines: a vital raw material for green energies with a potential environmental impact in its mining and processing. Sci. Total Environ. 639, 1188–1204. doi: 10.1016/j.scitotenv.2018.05.223
Fomina, M., and Gadd, G. M. (2014). Biosorption: current perspectives on concept, definition and application. Bioresour Technol 160, 3–14. doi: 10.1016/j.biortech.2013.12.102
García-Maldonado, J. Q., Bebout, B. M., Everroad, R. C., and López-Cortés, A. (2015). Evidence of novel phylogenetic lineages of methanogenic archaea from hypersaline microbial mats. Microb Ecol 69, 106–117. doi: 10.1007/s00248-014-0473-7
García-Maldonado, J. Q., Escobar-Zepeda, A., Raggi, L., Bebout, B. M., Sanchez-Flores, A., and López-Cortés, A. (2018). Bacterial and archaeal profiling of hypersaline microbial mats and endoevaporites, under natural conditions and methanogenic microcosm experiments. Extremophiles 22, 903–916. doi: 10.1007/s00792-018-1047-2
Gavrilescu, M. (2022). Microbial recovery of critical metals from secondary sources. Bioresour Technol 344:126208. doi: 10.1016/j.biortech.2021.126208
Gray, N. D., Sherry, A., Larter, S. R., Erdmann, M., Leyris, J., Liengen, T., et al. (2009). Biogenic methane production in formation waters from a large gas field in the North Sea. Extremophiles 13, 511–519. doi: 10.1007/s00792-009-0237-3
Gruber, P. W., Medina, P. A., Keoleian, G. A., Kesler, S. E., Everson, M. P., and Wallington, T. J. (2011). Global lithium availability: a constraint for electric vehicles? J Ind Ecol 15, 760–775. doi: 10.1111/j.1530-9290.2011.00359.x
Hasani Zadeh, P., Serrano, A., Collins, G., and Fermoso, F. G. (2022). Interrelating EPS, soluble microbial products and metal solubility in a methanogenic consortium stressed by nickel and cobalt. Ecotoxicol Environ Saf 238:113579. doi: 10.1016/j.ecoenv.2022.113579
Hoehler, T. M., Alperin, M. J., Albert, D. B., and Martens, C. S. (2001). Apparent minimum free energy requirements for methanogenic Archaea and sulfate-reducing bacteria in an anoxic 412 marine sediment. FEMS Microbiol Ecol 38, 33–41. doi: 10.1111/j.1574-6941.2001.tb00879.x
Horeh, N. B., Mousavi, S. M., and Shojaosadati, S. A. (2016). Bioleaching of valuable metals from spent lithium-ion mobile phone batteries using Aspergillus Niger. J Power Sources 320, 257–266. doi: 10.1016/j.jpowsour.2016.04.104
Intergovernmental Panel on Climate Change (IPCC) (2023). Climate Change 2022 – Impacts, Adaptation and Vulnerability. 2022nd Cambridge, UK and New York, NY, USA: Cambridge University Press.
Iram, S., Shabbir, R., Zafar, H., and Javaid, M. (2015). Biosorption and bioaccumulation of copper and lead by heavy metal-resistant fungal isolates. Arab J Sci Eng 40, 1867–1873. doi: 10.1007/s13369-015-1702-1
Işıldar, A., van Hullebusch, E. D., Lenz, M., Du Laing, G., Marra, A., Cesaro, A., et al. (2019). Biotechnological strategies for the recovery of valuable and critical raw materials from waste electrical and electronic equipment (WEEE) – a review. J Hazard Mater 362, 467–481. doi: 10.1016/j.jhazmat.2018.08.050
Jones, E., Qadir, M., van Vliet, M. T. H., Smakhtin, V., and Kang, S. (2019). The state of desalination and brine production: a global outlook. Sci. Total Environ. 657, 1343–1356. doi: 10.1016/j.scitotenv.2018.12.076
Kaksonen, A. H., Deng, X., Bohu, T., Zea, L., Khaleque, H. N., Gumulya, Y., et al. (2020). Prospective directions for biohydrometallurgy. Hydrometallurgy 195:105376. doi: 10.1016/j.hydromet.2020.105376
Kamienski, C. W., McDonald, D. P., Stark, M. W., and Papcun, J. R. (2004). “Lithium and lithium compounds” in Kirk-Othmer Encyclopedia of Chemical Technology (Wiley)15, 1–40. doi: 10.1002/0471238961.1209200811011309.a01.pub2
Kanamarlapudi, S. L. R. K., Chintalpudi, V. K., and Muddada, S. (2018). “Application of biosorption for removal of heavy metals from wastewater” in Biosorption. eds. J. Derco and B. Vrana. (London: InTech) doi: 10.5772/intechopen.77315
Khalil, A., Mohammed, S., Hashaikeh, R., and Hilal, N. (2022). Lithium recovery from brine: Recent developments and challenges. Desalination 528:115611. doi: 10.1016/j.desal.2022.115611
Kim, B. K., Pihl, T. D., Reeve, J. N., and Daniels, L. (1995). Purification of the copper response extracellular proteins secreted by the copper-resistant methanogen Methanobacterium bryantii BKYH and cloning, sequencing, and transcription of the gene encoding these proteins. J Bacteriol 177, 7178–7185. doi: 10.1128/jb.177.24.7178-7185.1995
Klingl, A. (2014). S-layer and cytoplasmic membrane - exceptions from the typical archaeal cell wall with a focus on double membranes. Front Microbiol 5, 1–6. doi: 10.3389/fmicb.2014.00624
König, H., Rachel, R., and Claus, H. (2014). “Proteinaceous surface layers of archaea: ultrastructure and biochemistry” in Archaea ed. C. Ricardo (Washington, DC, USA: ASM Press), 315–340. doi: 10.1128/9781555815516.ch14
Kurniawan, T. A., Lo, W. H., Liang, X., Goh, H. H., Othman, M. H. D., Chong, K. K., et al. (2023). Heavy metal removal from aqueous solutions using biomaterials and/or functional composites: recent advances and the way forward in wastewater treatment using digitalization. J. Composites Sci. 7, 84–98. doi: 10.3390/jcs7020084
Kurniawan, A., and Yamamoto, T. (2015). Biosorption of lithium using biofilm matrix of natural microbial consortium. Microbiol Indones 9, 106–112. doi: 10.5454/mi.9.3.2
Lahme, S., Mand, J., Longwell, J., Smith, R., and Enning, D. (2021). Severe Corrosion of Carbon Steel in Oil Field Produced Water Can Be Linked to Methanogenic Archaea Containing a Special Type of [NiFe] Hydrogenase. Appl. Environ. Microbiol 87. doi: 10.1128/AEM.01819-20
Li, H., Chang, F., Li, Z., and Cui, F. (2022). The role of extracellular polymeric substances in the toxicity response of anaerobic granule sludge to different metal oxide nanoparticles. Int J Environ Res Public Health 19:95371. doi: 10.3390/ijerph19095371
Liapun, V., and Motola, M. (2023). Current overview and future perspective in fungal biorecovery of metals from secondary sources. J Environ Manage 332:117345. doi: 10.1016/j.jenvman.2023.117345
Liu, Y., Boone, D. R., and Choy, C. (1990). Methanohalophilus oregonense sp. nov., a Methylotrophic Methanogen from an Alkaline, Saline Aquifer. Int J Syst Bacteriol 40, 111–116. doi: 10.1099/00207713-40-2-111
Liu, W., Zhang, J., Jin, Y., Zhao, X., and Cai, Z. (2015). Adsorption of Pb(II), Cd(II) and Zn(II) by extracellular polymeric substances extracted from aerobic granular sludge: Efficiency of protein. J Environ Chem Eng 3, 1223–1232. doi: 10.1016/j.jece.2015.04.009
Lyu, Z., Rotaru, A.-E., Pimentel, M., Zhang, C.-J., and Rittmann, S. K.-M. R. (2022). Editorial: The methane moment - Cross-boundary significance of methanogens: Preface. Front Microbiol 13:1055494. doi: 10.3389/fmicb.2022.1055494
Magoda, K., and Mekuto, L. (2022). Biohydrometallurgical recovery of metals from waste electronic equipment: current status and proposed process. Recycling 7:67. doi: 10.3390/recycling7050067
Mainka, T., Herwig, C., and Pflügl, S. (2022). Optimized operating conditions for a biological treatment process of industrial residual process brine using a halophilic mixed culture. Fermentation 8:246. doi: 10.3390/fermentation8060246
Manasi,, Rajesh, V., and Rajesh, N. (2018). Biosorption study of cadmium, lead and zinc ions onto halophilic bacteria and reduced graphene oxide. J Environ Chem Eng 6, 5053–5060. doi: 10.1016/j.jece.2018.07.042
McAdam, E. J., and Judd, S. J. (2008). Biological treatment of ion-exchange brine regenerant for re-use: A review. Sep Purif Technol 62, 264–272. doi: 10.1016/j.seppur.2008.01.007
McGenity, T. J., and Sorokin, D. Y. (2019). “Methanogens and methanogenesis in hypersaline environments” in Biogenesis of Hydrocarbons eds. A. J. M. Stams and D. Z. Sousa (Cham: Springer International Publishing), 283–309.
Meng, F., McNeice, J., Zadeh, S. S., and Ghahreman, A. (2021). Review of lithium production and recovery from minerals, brines, and lithium-ion batteries. Mineral Process Extractive Metallurgy Rev. 42, 123–141. doi: 10.1080/08827508.2019.1668387
Moazzam, P., Boroumand, Y., Rabiei, P., Baghbaderani, S. S., Mokarian, P., Mohagheghian, F., et al. (2021). Lithium bioleaching: An emerging approach for the recovery of Li from spent lithium ion batteries. Chemosphere 277:130196. doi: 10.1016/j.chemosphere.2021.130196
Mosley, J (2022). 2022 Final List of Critical Minerals. Available at: https://www.usgs.gov/news/national-news-release/us-geological-survey-releases-2022-list-critical-minerals (Accessed February 02, 2023).
Naseri, T., Bahaloo-Horeh, N., and Mousavi, S. M. (2019). Bacterial leaching as a green approach for typical metals recovery from end-of-life coin cells batteries. J Clean Prod 220, 483–492. doi: 10.1016/j.jclepro.2019.02.177
Ogawa, Y., Koibuchi, H., Suto, K., and Inoue, C. (2014). Effects of the chemical compositions of Salars de Uyuni and Atacama brines on lithium concentration during evaporation. Resource Geology 64, 91–101. doi: 10.1111/rge.12030
Ollivier, B., Fardeau, M.-L., Cayol, J.-L., Magot, M., Patel, B. K. C., Prensier, G., et al. (1998). Methanocalculus halotolerans gen. nov., sp. nov., isolated from an oil-producing well. Int J Syst Bacteriol 48, 821–828. doi: 10.1099/00207713-48-3-821
Orange, F., Disnar, J. R., Westall, F., Prieur, D., and Baillif, P. (2011). Metal cation binding by the hyperthermophilic microorganism, archaea methanocaldococcus jannaschii, and its effects on silicification. Palaeontology 54, 953–964. doi: 10.1111/j.1475-4983.2011.01066.x
Pagliaccia, B., Carretti, E., Severi, M., Berti, D., Lubello, C., and Lotti, T. (2022). Heavy metal biosorption by extracellular polymeric substances (EPS) recovered from anammox granular sludge. J Hazard Mater 424:126661. doi: 10.1016/j.jhazmat.2021.126661
Paper, M., Koch, M., Jung, P., Lakatos, M., Nilges, T., and Brück, T. B. (2023). Rare earths stick to rare cyanobacteria: future potential for bioremediation and recovery of rare earth elements. Front Bioeng Biotechnol 11:1130939. doi: 10.3389/fbioe.2023.1130939
Pat-Espadas, A. M., Field, J. A., Otero-Gonzalez, L., Razo-Flores, E., Cervantes, F. J., and Sierra-Alvarez, R. (2016). Recovery of palladium(II) by methanogenic granular sludge. Chemosphere 144, 745–753. doi: 10.1016/j.chemosphere.2015.09.035
Pfeifer, K., Ergal, İ., Koller, M., Basen, M., Schuster, B., and Rittmann, S. K. M. R. (2021). Archaea biotechnology. Biotechnol Adv 47:107668. doi: 10.1016/j.biotechadv.2020.107668
Prasad, T. L. (2023). ‘Brine management through brine mining of trace metals’ for developing secondary sources of nuclear fuel. Nucl. Eng. Technol. 55, 674–680. doi: 10.1016/j.net.2022.10.026
Rodrigues-Oliveira, T., Belmok, A., Vasconcellos, D., Schuster, B., and Kyaw, C. M. (2017). Archaeal S-layers: overview and current state of the art. Front Microbiol 8:2597. doi: 10.3389/fmicb.2017.02597
Simon-Pascual, A., Sierra-Alvarez, R., Ramos-Ruiz, A., and Field, J. A. (2018). Reduction of platinum (IV) ions to elemental platinum nanoparticles by anaerobic sludge. J. Chem. Technol. Biotechnol. 93, 1611–1617. doi: 10.1002/jctb.5530
Singh, R., Dong, H., Liu, D., Marts, A. R., Tierney, D. L., and Almquist, C. B. (2015a). [Cobalt(III)-EDTA]- reduction by thermophilic methanogen Methanothermobacter thermautotrophicus. Chem Geol 411, 49–56. doi: 10.1016/j.chemgeo.2015.06.025
Singh, R., Dong, H., Liu, D., Marts, A. R., Tierney, D. L., and Almquist, C. B. (2015b). Reduction of hexavalent chromium by the thermophilic methanogen Methanothermobacter thermautotrophicus. Chem Geol 411, 49–56. doi: 10.1016/j.chemgeo.2015.06.025
Sorokin, D. Y., Makarova, K. S., Abbas, B., Ferrer, M., Golyshin, P. N., Galinski, E. A., et al. (2017). Discovery of extremely halophilic, methyl-reducing euryarchaea provides insights into the evolutionary origin of methanogenesis. Nat Microbiol 2:17081. doi: 10.1038/nmicrobiol.2017.81
Sorokin, D. Y., Merkel, A. Y., Abbas, B., Makarova, K. S., Rijpstra, W. I. C., Koenen, M., et al. (2018). Methanonatronarchaeum thermophilum gen. nov., sp. nov. and “candidatus methanohalarchaeum thermophilum”, extremely halo(natrono)philic methyl-reducing methanogens from hypersaline lakes comprising a new euryarchaeal class Methanonatronarchaeia classis nov. Int J Syst Evol Microbiol 68, 2199–2208. doi: 10.1099/ijsem.0.002810
Srinath, T., Verma, T., Ramteke, P. W., and Garg, S. K. (2002). Chromium (VI) biosorption and bioaccumulation by chromate resistant bacteria. Chemosphere 48, 427–435. doi: 10.1016/S0045-6535(02)00089-9
Talens Peiró, L., Villalba Méndez, G., and Ayres, R. U. (2013). Lithium: sources, production, uses, and recovery outlook. JOM 65, 986–996. doi: 10.1007/s11837-013-0666-4
Torres, E. (2020). Biosorption: a review of the latest advances. Processes 8, 1–23. doi: 10.3390/pr8121584
Tripathi, M., Singh, P., Singh, R., Bala, S., Pathak, N., Singh, S., et al. (2023). Microbial biosorbent for remediation of dyes and heavy metals pollution: a green strategy for sustainable environment. Front Microbiol 14:1168954. doi: 10.3389/fmicb.2023.1168954
van Wolferen, M., Orell, A., and Albers, S.-V. (2018). Archaeal biofilm formation. Nat Rev Microbiol 16, 699–713. doi: 10.1038/s41579-018-0058-4
Vítězová, M., Kohoutová, A., Vítěz, T., Hanišáková, N., and Kushkevych, I. (2020). Methanogenic microorganisms in industrial wastewater anaerobic treatment. Processes 8:1546. doi: 10.3390/pr8121546
Wang, Y., Zhang, R., Duan, J., Shi, X., Zhang, Y., Guan, F., et al. (2022). Extracellular polymeric substances and biocorrosion/biofouling: recent advances and future perspectives. Int J Mol Sci 23:5566. doi: 10.3390/ijms23105566
Watkins, A. J., Roussel, E. G., Parkes, R. J., and Sass, H. (2014). Glycine betaine as a direct substrate for methanogens (Methanococcoides spp.). Appl Environ Microbiol 80, 289–293. doi: 10.1128/AEM.03076-13
Welsh, D. T. (2000). Ecological significance of compatible solute accumulation by micro-organisms: from single cells to global climate. FEMS Microbiol Rev 24, 263–290. doi: 10.1111/j.1574-6976.2000.tb00542.x
Wilms, R., Sass, H., Köpke, B., Cypionka, H., and Engelen, B. (2007). Methane and sulfate profiles within the subsurface of a tidal flat are reflected by the distribution of sulfate-reducing bacteria and methanogenic archaea. FEMS Microbiol Ecol 59, 611–621. doi: 10.1111/j.1574-6941.2006.00225.x
Wong, H. L., Visscher, P. T., White, R. A., Smith, D. L., Patterson, M. M., and Burns, B. P. (2017). Dynamics of archaea at fine spatial scales in Shark Bay mat microbiomes. Sci Rep 7:46160. doi: 10.1038/srep46160
Zeb, I., Ma, J., Mehboob, F., Kafle, G. K., Amin, B. A. Z., Nazir, R., et al. (2019). Kinetic and microbial analysis of methane production from dairy wastewater anaerobic digester under ammonia and salinity stresses. J Clean Prod 219, 797–808. doi: 10.1016/j.jclepro.2019.01.295
Zhang, J., Dong, H., Zhao, L., McCarrick, R., and Agrawal, A. (2014). Microbial reduction and precipitation of vanadium by mesophilic and thermophilic methanogens. Chem Geol 370, 29–39. doi: 10.1016/j.chemgeo.2014.01.014
Keywords: water desalination, lithium recovery, biomethanation, critical raw material, biosorption, salinity, methanogens, brine mining
Citation: Abdel Azim A, Vizzarro A, Bellini R, Bassani I, Baudino L, Pirri CF, Verga F, Lamberti A and Menin B (2023) Perspective on the use of methanogens in lithium recovery from brines. Front. Microbiol. 14:1233221. doi: 10.3389/fmicb.2023.1233221
Received: 01 June 2023; Accepted: 19 July 2023;
Published: 02 August 2023.
Edited by:
Amelia-Elena Rotaru, University of Southern Denmark, DenmarkReviewed by:
Julia Kurth, University of Marburg, GermanyCopyright © 2023 Abdel Azim, Vizzarro, Bellini, Bassani, Baudino, Pirri, Verga, Lamberti and Menin. This is an open-access article distributed under the terms of the Creative Commons Attribution License (CC BY). The use, distribution or reproduction in other forums is permitted, provided the original author(s) and the copyright owner(s) are credited and that the original publication in this journal is cited, in accordance with accepted academic practice. No use, distribution or reproduction is permitted which does not comply with these terms.
*Correspondence: Annalisa Abdel Azim, YW5uYWxpc2EuYWJkZWxhemltQGlpdC5pdA==
Disclaimer: All claims expressed in this article are solely those of the authors and do not necessarily represent those of their affiliated organizations, or those of the publisher, the editors and the reviewers. Any product that may be evaluated in this article or claim that may be made by its manufacturer is not guaranteed or endorsed by the publisher.
Research integrity at Frontiers
Learn more about the work of our research integrity team to safeguard the quality of each article we publish.