- 1Istituto Zooprofilattico Sperimentale dell’Abruzzo e del Molise “G. Caporale”, Teramo, Italy
- 2Central Veterinary Research Institute, Ministry of Fisheries and Livestock, Lusaka, Zambia
The contamination of ready to eat foods (RTE) products due to Listeria monocytogenes could compromise the products safety becoming a great risk for the consumers. The high presence of L. monocytogenes in RTE products has been described worldwide, but few data are available about these products from African countries. The aims of this study were to report the presence of L. monocytogenes in Zambian RTE products, providing genomic characterization and data on similarity with African circulating strains using whole genome sequencing (WGS). A total of 304 RTE products, produced by different Zambian manufacturers, were purchased at retail, from major supermarkets located in Lusaka, Zambia, comprising 130 dairy and 174 meat products. L. monocytogenes was detected only in 18 (10.3%) RTE meat products of the 174 samples tested. The MLST analysis grouped the 18 L. monocytogenes isolates in 7 clonal complexes (CCs): CC1 (n = 5), CC2 (n = 4), CC9 (n = 4), CC5 (n = 2), CC121 (n = 1), CC155 (n = 1), and CC3 (n = 1). According to the cgMLST results, several clusters were detected, in particular belonging to hyper-virulent clones CC1 and CC2. Regarding the virulence factors, a complete L. monocytogenes Pathogenicity Island 3 (LIPI-3) was present both in the CC1 and CC3, in addition to LIPI-1. Several resistance genes and mobile genetic elements were detected, including Stress Islands, the bcrABC cassette and Tn6188_qac transposon, plasmids and intact prophages. Despite being a first preliminary work with a limited number of samples and isolates, this study helped to increase existing knowledge on contaminated RTE products in Zambia, confirming the presence of hyper-virulent L. monocytogenes CCs, which could play an important role in human diseases, posing a public health concern for consumers.
1. Introduction
The consumption of convenience foods, such as sliced and prepackaged ready to eat (RTE) products, has increased worldwide. The RTE meat and dairy products require no post-processing heating or other additional antimicrobial treatments before consumption, hence the contamination of pathogenic microorganisms, such as Listeria monocytogenes, could compromise the safety of the products becoming a great risk for the final consumers (Calvo-Arrieta et al., 2021).
The presence of L. monocytogenes in RTE products has been worldwide described and confirmed, including European Union (European Food Safety Authority, 2022) and South Africa (SA) (Matle et al., 2019). The contamination of RTE products can occur along the entire food production chain as L. monocytogenes is widely distributed in primary production areas and in food production environments (FPE) of food producing plants (FPP), processing and packaging plants, storage facilities and retail shops (Gupta and Achyut, 2022). L. monocytogenes is ubiquitous and able to survive at low refrigeration temperatures, low pH and high salt concentrations, but also to develop biofilms, increasing the risks of food contamination (Lee et al., 2019; Osek and Wieczorek, 2022). L. monocytogenes contamination is associated with poor food processing practices and cross-contaminations in FPP, involving mainly RTE, such as vegetables, fish, dairy and meat products (Dufailu et al., 2021).
Listeria monocytogenes is a foodborne pathogen that can cause listeriosis, a potentially severe foodborne disease in human and animals (Maury et al., 2019). In healthy people, more frequently L. monocytogenes is a cause a febrile gastroenteritis, whereas in susceptible people, such as children, elderly, immunocompromised and pregnant women, it may lead to septicemia, meningitis, abortion and eventually death (Osek and Wieczorek, 2022). Even if rare, an occurrence of respiratory infection caused by L. monocytogens has been reported in Italy (Guidi et al., 2021a).
The European Food Safety Authority (European Food Safety Authority, 2022) reported 2,183 confirmed invasive human cases of L. monocytogenes in 2021. Moreover, the European case fatality rate is high (13.7%), similar to 2020 (European Food Safety Authority, 2022), confirming listeriosis as one of the most severe foodborne diseases. L. monocytogenes outbreaks reported were linked to different food matrices, such as ice cream (Chen et al., 2017b), cheese (Chen et al., 2017a), RTE fish products (Schjørring et al., 2017) and RTE meat products (Althaus et al., 2017; Duranti et al., 2018; Gelbíčová et al., 2018; Orsini et al., 2018).
Few data are available regarding the prevalence of L. monocytogenes in RTE food products from African countries (Garedew et al., 2015; Oyinloye, 2016; Matle et al., 2019), however, one of the biggest listeriosis outbreak, with 1,060 cases, was reported in SA between 2017 and 2018, due to polony, a RTE processed meat product (Smith et al., 2019; Thomas et al., 2020).
The aims of this study were to report the presence of L. monocytogenes in Zambian RTE products, provide data on the genomic characterization of isolated strains and evaluate their similarity with strains circulating in other African countries using whole genome sequencing (WGS).
2. Materials and methods
2.1. Sample collection and Listeria monocytogenes detection
Between October 2019 and February 2020, a total of 304 RTE Zambian products were collected; all the products were randomly selected and purchased at retail, from the major supermarkets located in Lusaka, Zambia. The RTE products considered in this study are usually consumed in the cities which has more wealthy people who prefer easy and fast made foods, hence the choice of selection site. The RTE product categories included 130 dairy products (milk drink n = 94, cheese n = 18, ice cream n = 16, butter n = 2) and 174 meat products (polony n = 84, ham n = 43, sausage n = 21, biltong n = 15, and salami n = 11). All products were produced in Zambia by local manufacturers, in particular: 16 different meat (M) and 23 different dairy (D) manufacturers. The sample identification, product type, RTE category, data of sampling and Zambian producer manufacturer were reported in Supplementary Table S1.
From the supermarkets, the packaged samples were placed in a cool box with ice, kept at 8°C and transported to the Central Veterinary Research Institute (CVRI) laboratory on the same day. The RTE products were processed immediately on arrival at the laboratory and tested for L. monocytogenes detection, according to ISO (International Organization for Standardization) (2017). Briefly, 25 g of each sample was cultured in 225 mL of Half Fraser enrichment broth (Oxoid, Hampshire, England) for 24 h ± 2 h at 30°C ± 1°C. After the incubation, 0.1 mL of the culture was poured into 10 mL of Fraser broth (Oxoid, Hampshire, England), incubated for 24 h ± 2 h at 37°C ± 1°C. A loopful of both culture suspensions were streaked on to two selective media plates: Agar Listeria according to Ottaviani and Agosti (ALOA) agar (Biolife, Milan, Italy) and Oxford agar (Oxoid, Hampshire, England) and both incubated at 37°C ± 1°C for 24–48 h. Suspect presumptive colonies of L. monocytogenes (small, convex bluish-green colonies with opaque white halo on ALOA plate and gray colonies with black zones and sunken center on Oxford plate) were selected in order to perform all confirmation tests reported in the ISO11290-1:2017 (i.e., hemolysis, sugar fermentation). All the L. monocytogenes isolates were stored in microbank tubes (Pro Lab Diagnostics, TX, USA) at −20°C and sent, with frozen transport, to the Italian National Reference Laboratory for L. monocytogenes (NRL-Lm) of Istituto Zooprofilattico Sperimentale dell’Abruzzo e del Molise (IZSAM) for further phenotypical and genomic characterization.
2.2. Antimicrobial susceptibility testing
All the L. monocytogenes isolates were tested for antimicrobial susceptibility (AST) by broth microdilution method with the Sensititre OptiRead Automated Fluorometric Plate Reading System (Thermo Scientific, Monza, Italy), in order to verify the susceptibility profile of L. monocytogenes strains. The minimum inhibitory concentration (MIC) was in vitro assessed using the Haemophilus spp. and Streptococcus pneumoniae Sensititre plate HPB1 (Thermo Scientific, Monza, Italy).
Listeria monocytogenes isolates, stored in microbank tubes (Pro Lab Diagnostics, TX, USA) in the NRL-Lm at −80°C, were cultured in Brain Heart Infusion (BHI) broth for 20–24 h at 37°C ± 1°C. A loop was streaked on blood agar plates (Liofilchem, Roseto degli Abruzzi, Teramo, Italy) and incubated at 37°C ± 1°C for 24 h ± 1 h. At the end of the incubation, the suspensions and Sensititre plates HPB1 were prepared according to the Sensititre plate guide booklet.1 The Sensititre plates HPB1 were incubated at 37°C ± 1°C for 20–24 h, and immediately after, the MIC values were manually read using the Sensititre Vizion Digital MIC Viewing System (Thermo Scientific, Monza, Italy). The interpretation of MIC results was carried out in accordance with EUCAST guidelines [EUCAST (European Committee on Antimicrobial Susceptibility Testing), 2021]. If no specific EUCAST breakpoints were available, results were interpreted considering as reference Enterococcus spp. and Streptococcus pneumoniae, according to Fischer et al. (2020) (Supplementary Table S2). The MIC value of cefixime was inferred based on cefuroxime susceptibility considering as reference Streptococcus pneumoniae. All the extended dilution range of tested antimicrobials are summarized in Supplementary Table S3.
2.3. Whole genome sequencing and bioinformatics analysis
Listeria monocytogenes isolates, stored in microbank tubes (Pro Lab Diagnostics, Round Rock, TX, USA) in the NRL-Lm at −80°C, were firstly streaked on ALOA agar (Liofilchem, Roseto degli Abruzzi, Teramo, Italy), incubated at 37°C ± 1°C for 24–48 h. The DNA extraction was performed on each strain according to Portmann et al. (2018), with minor modifications, firstly adding lysozyme from chicken egg solution (20 mg/mL) (Sigma-Aldrich, Milan, Italy) and, then, using the QIAamp DNA Mini Kit (Qiagen Hilden, Germany), following the manufacturer’s protocol. The purity of the extracts was evaluated by Biospectrometer fluorescence (Eppendorf, Milan, Italy), measuring the absorbance (A), in particular A260/280 and A260/230 values.
Starting from 1 ng of input DNA, the Nextera XT DNA chemistry (Illumina, San Diego, CA) was used for library preparation, according to the manufacturer’s protocols. WGS was performed on the NextSeq 500 platform (Illumina, San Diego, CA, US) with the NextSeq 500/550 mid output reagent cartridge v2 (300 cycles, standard 150-bp paired-end reads).
For the WGS data analysis, an in-house pipeline (Cito et al., 2018) was used which included steps for trimming (Trimmomatic v0.36) (Bolger et al., 2014) and quality control check of the reads (FastQC v0.11.5) (Wingett and Andrews, 2018). Genome de novo assembly of paired-end reads was performed using SPAdes v3.11.1 (Bankevich et al., 2012) with default parameters for the Illumina platform 2 × 150 chemistry (−only-assembler –careful –k21, 33, 55, 77). Subsequently, the genome assembly quality check was performed with QUAST v.4.3 (Gurevich et al., 2013). The genomes quality was checked according to the parameters recommended by Timme et al. (2020).
The multilocus sequence typing (MLST) and core genome multilocus sequence typing (cgMLST) analysis were performed according to Pasteur’s reference schemes.2 The threshold of ≤7 allelic distance (AD) was considered for cgMLST cluster definition (Moura et al., 2016). The software GrapeTree (Zhou et al., 2018) was used to obtain the Minimum Spanning tree (MSTreeV2).
The L. monocytogenes genomes in this study were also characterized in silico using BIGSdb-Lm database tools (accessed on February 2022), querying for: “Virulence,” “Antibiotic resistance,” “Metal and disinfectant resistance” and “Stress Islands.” The identification of mobile genetic elements, such as prophages and plasmids, was performed using PHASTER (accessed on February 2022)3 and PlasmidFinder 2.1 (accessed on February 2022)4 platforms, respectively.
In addition, a genomic comparison based on AD was performed between L. monocytogenes genomes and genomic data available at the National Center for Biotechnology Information (NCBI), related to previous studies on food, environmental and clinical samples from African countries.
The genome assemblies were deposited at DDBJ/ENA/GenBank under the BioProject PRJNA965923.
3. Results
3.1. Listeria monocytogenes detection
Out of the 304 RTE food tested, L. monocytogenes was detected in 18 (10.3%) of the 174 RTE meat products samples and none from dairy products. Data in detail were reported in the Table 1.
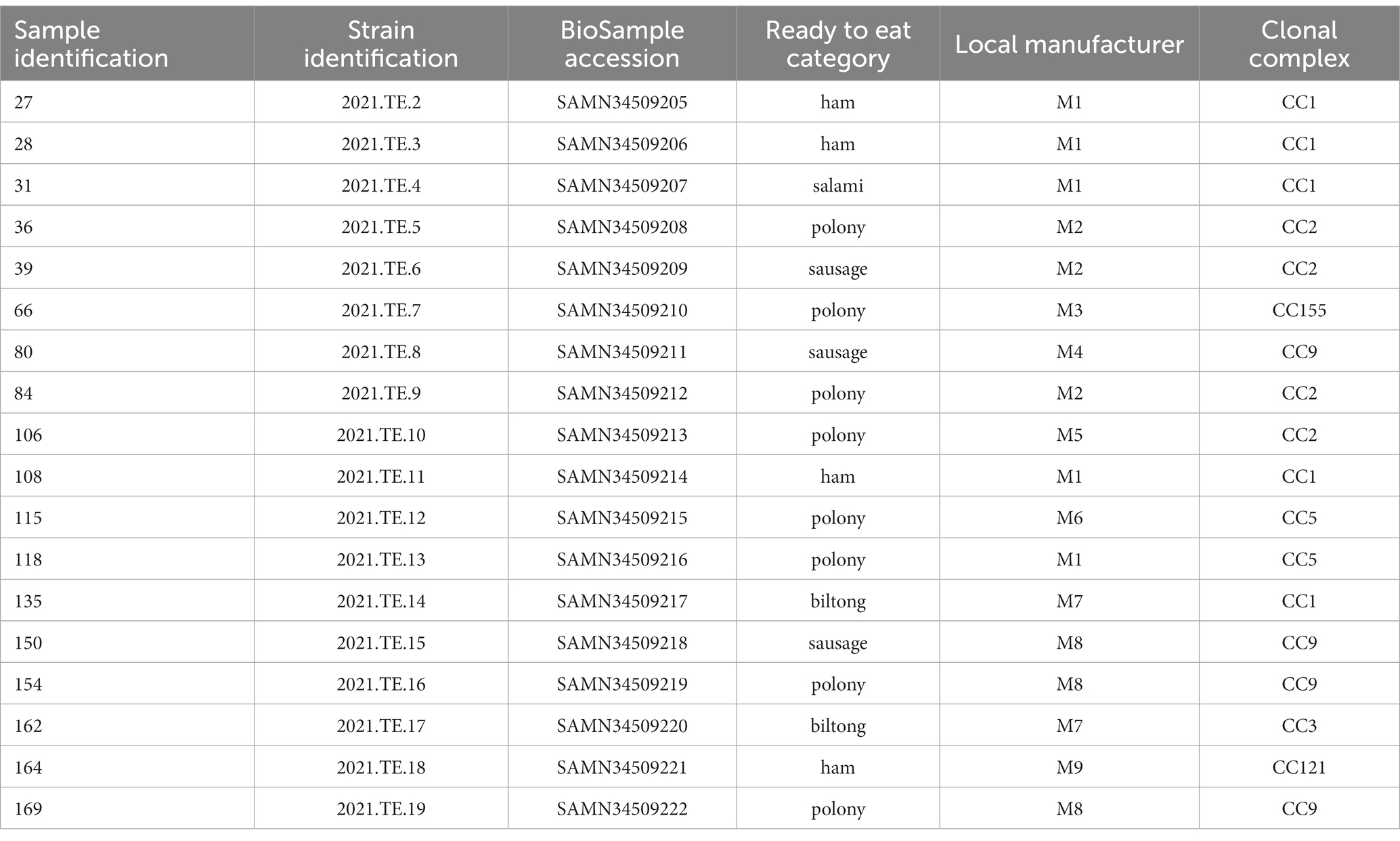
Table 1. Identification of 18 samples positive for L. monocytogenes and related ready to eat category, local producer manufacturer, BioSample accession number and clonal complex results of the related L. monocytogenes isolates.
The presence of L. monocytogenes in RTE meat products was observed in polony samples (n = 8), followed by ham (n = 4), sausage (n = 3), biltong (n = 2), and salami (n = 1). Among all the brands considered, more than one positive sample were detected in products of M1 (n = 5), M8 (n = 3), M2 (n = 3), and M7 (n = 2). For each positive sample, one L. monocytogenes colony was picked for the characterization, for a total of 18 isolates.
3.2. Antimicrobial susceptibility testing
The phenotypical AST results are reported in Figure 1 and Supplementary Table S4. All the 18 L. monocytogenes isolates showed a resistant phenotypic profile to cephalosporins (cefaclor, cefepime, cefixime, ceftriaxone, and cefuroxime) confirming their natural resistance to this molecule. In the case of penicillin, except one (2021.TE.16), all isolates were susceptible to ampicillin. Two strains were resistant to tetracycline (2021.TE.14 and 2021.TE.16), and finally one strain (2021.TE.11) was resistant to trimethoprim/sulfamethoxazole (miscellaneous agents).
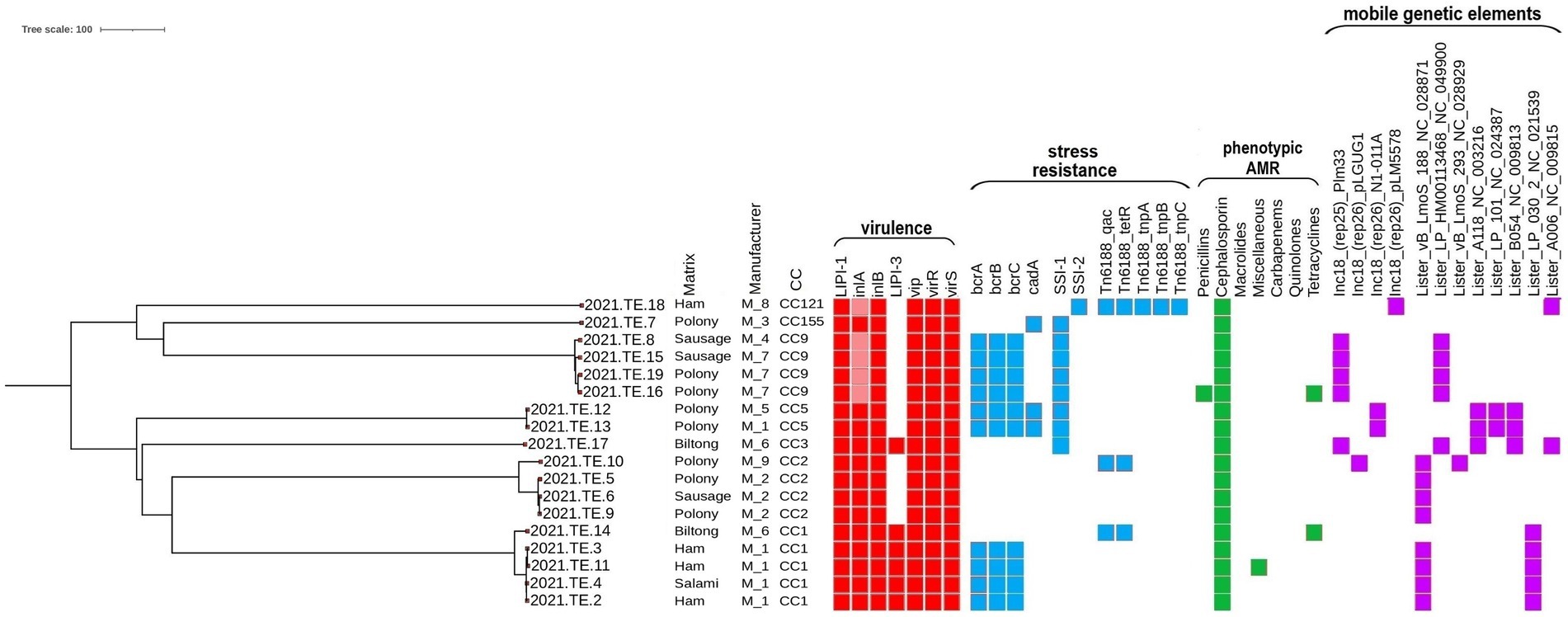
Figure 1. Core genome multilocus sequence typing (cgMLST) phylogenetic tree grouping of virulence, resistance, phenotypic antimicrobial resistance and mobile genetic elements across the 18 L. monocytogenes isolates. Virulence (red square), resistance (blue square), phenotypic antimicrobial resistance (green square), and mobile genetic elements (purple square) are shown in the heatmap. Colored square: presence of the gene or phenotypic resistance; light red: presence of premature stop codon and truncated inlA. The visualization of the genes profiles and genes presence/absence according to their cgMLST was visualized using the Interactive Tree of Life (iTOL) (https://itol.embl.de/).
For chloramphenicol, one isolate was susceptible; all the other strains showed a MIC value greater than the maximum value of concentration of this compound in the plate and makes results not interpretable. In the case of quinolones, for sparfloxacin, eight strains were susceptible, while all the other strains showed a MIC value greater than the maximum value of concentration of this compound in the plate making results not interpretable; whereas for levofloxacin, all the strains were susceptible. Finally, all strains were susceptible to ampicillin/sulbactam and amoxicillin/clavulanic acid, carbapenems (imipenem and meropenem) and macrolides (clarithromycin and erythromycin).
Additionally, the identification of antimicrobial resistance genes stated that in all the L. monocytogenes isolates tested intrinsic core genes were observed, in particular: fosX, mprF, norB, sul, conferring resistance to fosfomycin, quinolones and cationic peptides and sulfonamides, respectively.
3.3. WGS and bioinformatic analysis
The results of MLST, cgMLST, virulence, stress resistances and mobile genetic elements profiles were reported in Figure 1. The MLST analysis grouped the 18 L. monocytogenes isolates in 7 different Clonal Complexes (CCs): CC1 (n = 5), CC2 (n = 4), CC9 (n = 4), CC5 (n = 2), CC121 (n = 1), CC155 (n = 1), and CC3 (n = 1). Data in details are reported in Table 1.
According to the cgMLST results, several clusters were detected. For CC1, 4 of the 5 isolates (2021.TE.2; 2021.TE.3; 2021.TE.4; 2021.TE.11), from ham and salami of M1, showed only 1 AD from each other. One CC1 strain (2021.TE.14) from biltong was a singleton, showing 39 AD from CC1 cluster. Regarding the CC2, three strains (2021.TE.5, 2021.TE.6 and 2021.TE.9) isolated in different products from M2, showed ≤7 AD each other. One polony strain (2021.TE.10) from M5, showed 67 AD from CC2 cluster. For the CC9 strains, 7 AD was highlighted between two strains (2021.TE.16, 2021.TE.19) isolated from polonies of M7. The other CC9 strains (2021.TE.8 and 2021.TE.15), both isolated from sausages showed >7 AD from CC9 cluster. Two L. monocytogenes strains (2021.TE.12 and 2021.TE.13) were CC5 showing 3 AD from polonies of M1 and M6. Three strains (one CC121, one CC155 and one CC3) were singleton from 3 different M.
Regarding the virulence factors, all the CCs detected had a complete L. monocytogenes Pathogenicity Island 1 (LIPI-1), while a complete LIPI-3 was present both in the CC1 and CC3 isolates. All the CCs carried vip, virR, and virS genes and a full-length inlA for Internalin A and inlB for Internalin B. However, only in CC9 and CC121 isolates, a premature stop codon (PMSC) mutation was detected in the inlA.
The resistance to stresses, the Stress Survival Islet 1 (SSI-1) was carried by all the CC9, CC5, CC3 and CC155 isolates. Only the CC121 clone, indeed, highlighted the presence of SSI-2. The bcrABC cassette was present in 4 CC1 isolates, and in all the CC9 and CC5 L. monocytogenes isolates. Only the CC5 and CC155 revealed the presence of cadA gene. The Tn6188_qac transposon for Benzalkonium Chloride (BC) tolerance was observed in CC121, in one CC1 and CC2 L. monocytogenes, together with the Tn6188_tetR. The CC121 isolate carried out other transposons (Tn6188_tnpA, Tn6188_tnpB, Tn6188_tnpC).
Regarding mobile genetic elements, plasmids were identified in 9 isolates; the most frequent was plasmid Plm33 (n = 5), detected in all the CC9 and in CC3 isolates, followed by plasmid N1-011A (n = 2) in CC5 isolates. Plasmids pLM5578 and pLGUG1 were observed only in CC121 and in one CC2 isolates, respectively. Eight different intact prophages regions were found across 17 of the 18 L. monocytogenes isolates of this study. The prevalent one was the NC_028871, followed by NC_04990, NC_021539, NC_003216, NC_009813, NC_024387, NC_009815 and NC_028929, reported here as NCBI Reference Sequence.
The comparison between L. monocytogenes detected in Zambia and those selected from NCBI, available from SA, showed the presence of several relatedness’s. In regards to CC1 isolates (Figure 2), 3 AD was observed between one CC1 isolate from biltong (2021.TE.14) and one isolated in 2016 from a retail (processed meat-beef) in Gauteng (SA) (SAMN18679582). Similarly, 26 AD was observed between our strain (2021.TE.3) from ham sample and one L. monocytogenes isolated from a butchery (processed meat-beef) in Limpopo (SA) in 2016 (SAMN18679598).
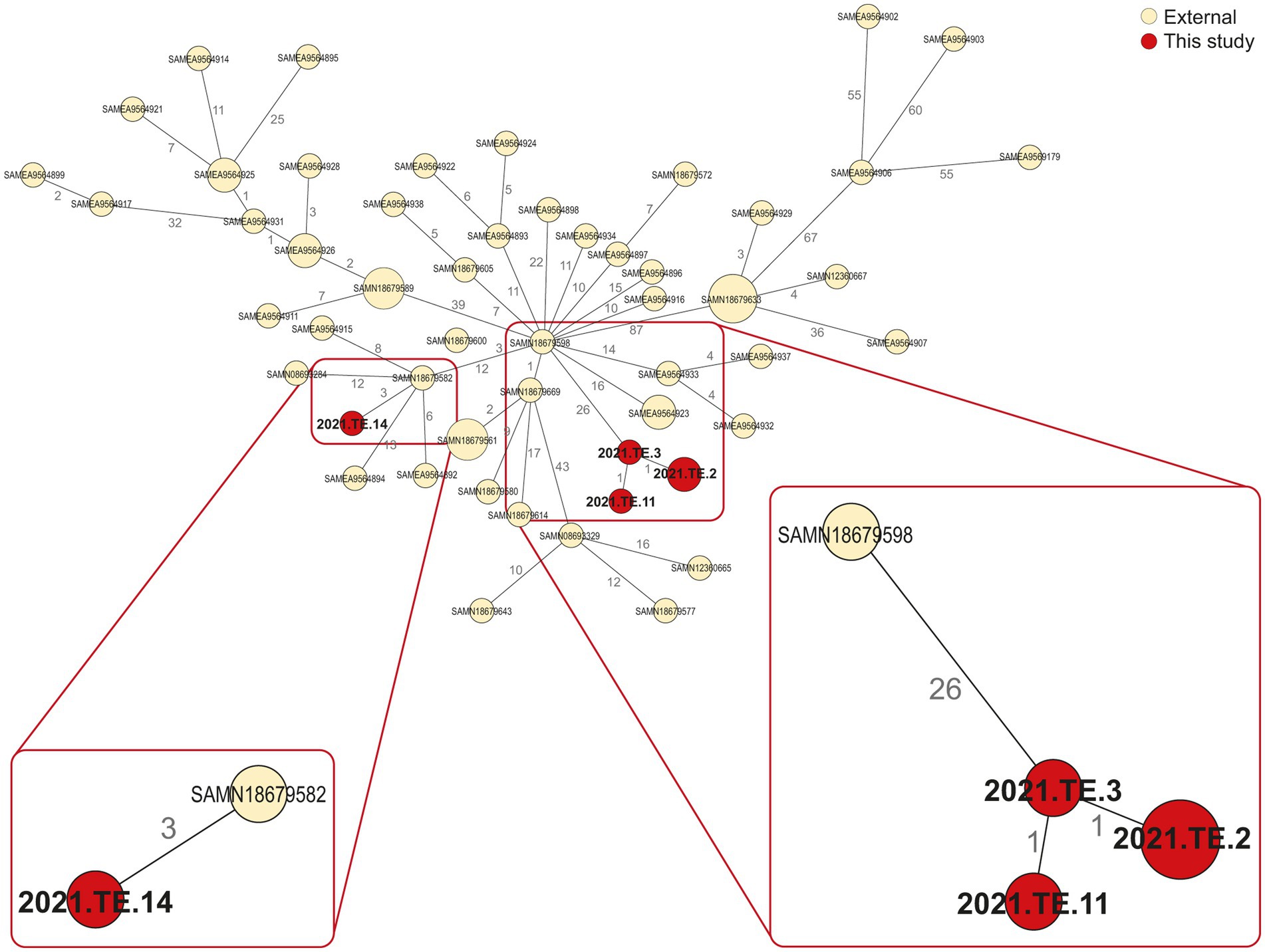
Figure 2. Minimum spanning tree (MST) based on the cgMLST profiles of L. monocytogenes CC1. In red were reported the strains from this study, in light yellow were reported the public available genomic data (external) African strains from NCBI. The MST was visualized using GrapeTree (https://github.com/achtman-lab/GrapeTree) and graphically elaborated by Adobe Illustrator to highlight the genomic relatedness (red square).
Regarding the CC2 (Figure 3A), 17 AD was observed between our isolate (2021.TE.10) and one isolated from a retailer in SA, in 2018 (SAMN25275984). For the CC9 (Figure 3B), one isolate (2021.TE.16) from polony showed 29 AD from an isolate detected in 2014, in North West (SA) from retail (raw poultry) (SAMN18679544). Moreover, the same isolate from this study, showed 42 AD from SAMN18679541, isolated in 2015 from Coldstore (Raw-Beef) in Eastern Cape (SA). Another CC9 isolate from this study (2021.TE.19) isolated from polony, showed 36 AD from an isolate SAMN25275560 detected in 2018 in a retailer located in SA. Lastly, for CC5 (Figure 3C), <30 AD was observed between our isolates (2021.TE.12 and 2021. TE.13), both from polony samples, and a clinical isolate from SA (SAMN08693280).
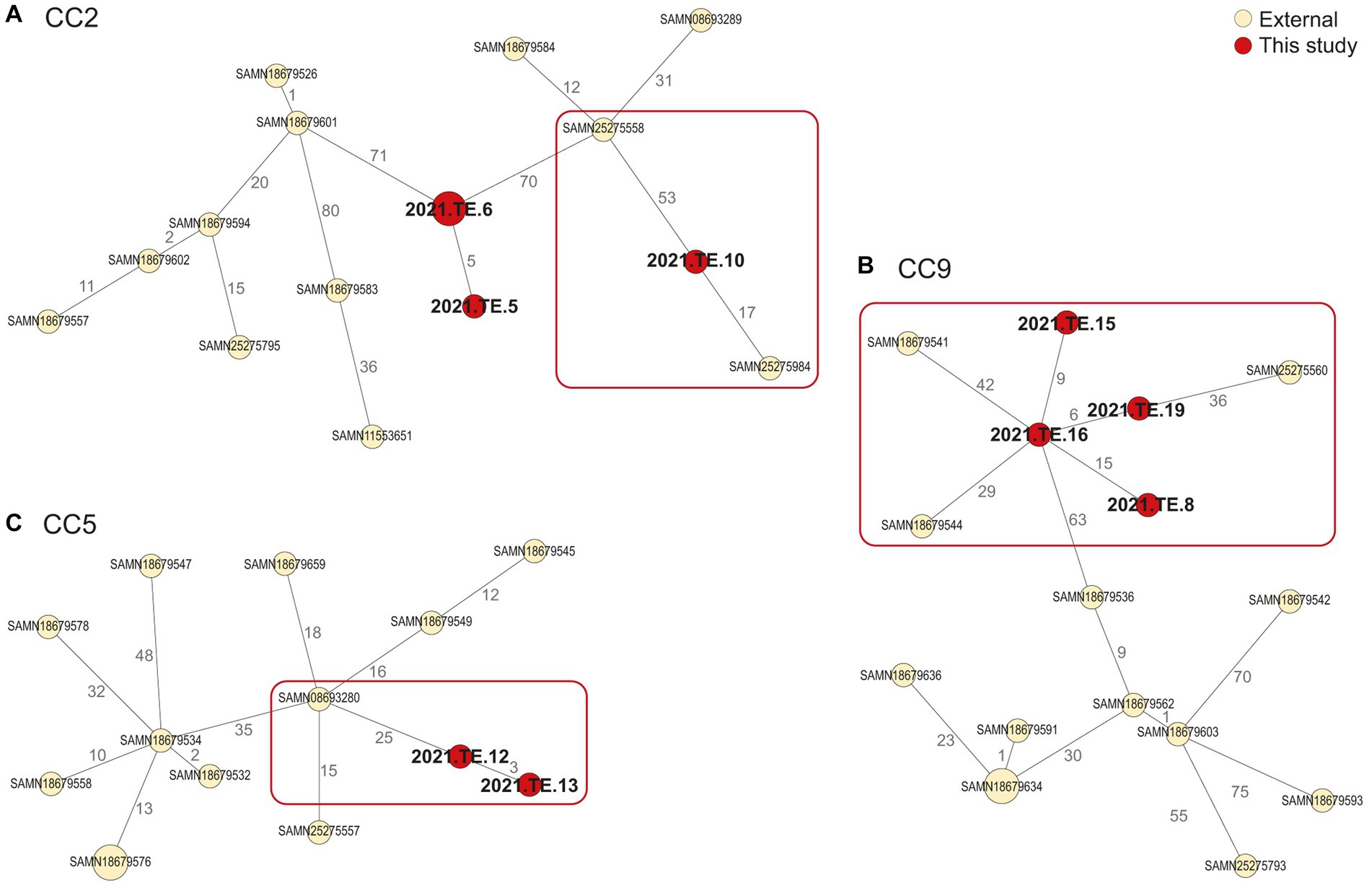
Figure 3. Minimum spanning tree (MST) based on the cgMLST profiles of L. monocytogenes CC2 (A), CC9 (B), and CC5 (C). In red were reported the strains from this study, in light yellow were reported the public available genomic data (external) African strains from NCBI. The MST was visualized using GrapeTree (https://github.com/achtman-lab/GrapeTree) and graphically elaborated by Adobe Illustrator to highlight the genomic relatedness (red square).
4. Discussion
To date, very few data are available on L. monocytogenes in RTE foods from Zambia and in Zambian products. Our findings highlighted the presence of L. monocytogenes mainly in ham and polony; the latter was the same type of product involved in the SA outbreak (Smith et al., 2019). Previous studies conducted in Zambia reported the prevalence of L. monocytogenes in 74% of poultry dressed carcasses (Mpundu et al., 2022a) and 26.3% in cattle carcasses (Mpundu et al., 2022b).
Data are available from African countries, reporting the presence of L. monocytogenes in different matrices, such as foods, animal and environmental samples, in Nigeria, SA, Egypt, Ethiopia, and Botswana (Dufailu et al., 2021). In particular data related to L. monocytogenes in East Africa, Ethiopia, reported a prevalence of 6.25% in RTE food of animal origin (Garedew et al., 2015), lower than our findings. In Nigeria, a prevalence of 7% was detected in raw meat samples from Rivers State (Odu and Okonko, 2017), while 91.8% in chicken flocks and meat in Oyo (Ishola et al., 2016). In Lafia (Nigeria), the 64.4% of isolates from beef and chevon were confirmed to be L. monocytogenes (Chuku et al., 2020). Additionally, in locally made soft cheeses the 12.4% of isolates were L. monocytogenes (Oyinloye, 2016). In a study, Matle et al. (2019), conducted in meat samples from SA over several years, reported L. monocytogenes in 13.5% of RTE foods collected, higher than the one obtained in our study. The presence of L. monocytogenes in the RTE meat placed on markets could be mainly due to inadequate hygiene management systems (Matle et al., 2019), which is a problem in many African countries.
It emerged in this study that several FPP considered presented more than one RTE meat category contaminated by L. monocytogenes. The subsequent placing on the market of these contaminated products and their availability for the consumers, poses a high risk especially for vulnerable people. The risk associated with RTE products depends mainly on the effectiveness of control measures applied by food business operators (FBOs), including good hygiene and good manufacturing practices, both at processing and retail (European Food Safety Authority, 2022). In smaller retail store and local manufacturers, inadequate equipment for the preparation of RTE foods may also increase the contamination risk of the final products. In addition, inadequate procedures applied to clean and sanitize FPE (i.e., cutting boards) may increase the cross-contamination of other food products and the persistence of L. monocytogenes. Moreover, the presence of L. monocytogenes is reported in animals (Heredia and García, 2018), then the contamination of processed foods could be also related to inadequate management of animals, agriculture and farming practices, not only to the post-processing phases.
In literature is well known that listeriosis cases are predominantly associated with RTE foods, including RTE meat products (European Food Safety Authority, 2022). As reported, in the USA, the main listeriosis cases were caused by meat products sliced at retail (Forauer et al., 2021). Food products sliced in retail shops presented a higher bacterial contamination than products prepared in FPP (Forauer et al., 2021), hence RTE food preparation at retail shops could increase the risk of cross-contamination of products from different FBOs (Kurpas et al., 2018).
The WGS approach provided crucial information regarding the CCs distribution and the genomic characteristics of L. monocytogenes isolated from Zambian RTE meat foods, especially related to CC1 and CC2 clones, the main CCs detected in the RTE products tested. CC1 and CC2 are both well known to be hyper-virulent clones, often associated with clinical infections (Maury et al., 2016), their presence in RTE foods is a crucial concern for consumer safety. In literature, CC1 clone was often isolated in the pork-meat production sector and it is frequently associated with dairy sector (Félix et al., 2018). The presence of hyper-virulent clones in foods has been reported worldwide, including African countries, suggesting the L. monocytogenes adaptation to food products (Matle et al., 2020; Mafuna et al., 2021). The clustering conducted in this study with other public available African L. monocytogenes genomes, showed that one CC1 isolate from biltong sample, seems to be closely related to CC1 strain previously detected in 2016 from a SA processed meat.
According to our results, both CC1 and CC2, carried a full-length internalins, inlA and inlB, which are considered one of the most influent factors for L. monocytogenes invasiveness (Su et al., 2019). Increased hyper-virulence and bacterial colonization has been reported in isolates with a full-length inlA and a complete LIPI-1 and LIPI-3 (Maury et al., 2016; Yin et al., 2019), suggesting that some isolates have increased virulence potential (Gray et al., 2021). In addition to a complete LIPI-1, CC1 isolates also harbored a complete LIPI-3, encoding the production of Listeriolysin S, a hemolytic and cytotoxic factor also observed to be released in acid stress (Tavares et al., 2020).
Previous studies reported that CC9 and CC121 were often associated with meat products, including RTE, and FPP worldwide, also in African countries (Matle et al., 2020; Mafuna et al., 2021). As known, L. monocytogenes is able to resist under stress conditions, supporting its spread in many types of foods (Kurpas et al., 2018). In particular, CC9 and CC121 are well known to be better adapted to meat processing environments (Maury et al., 2019; Centorotola et al., 2021; Guidi et al., 2021b). Specific factors are reported to be crucial for L. monocytogenes resistance to the environmental stress. In particular, the presence of SSI-1 ensures resistances to low pH, high osmolarity, bile and nisin, while SSI-2 confers resistances to alkaline and oxidative stresses (Maury et al., 2019). According to our results, SSI-1 was observed in L. monocytogenes strains belonging to different clones, whereas the SSI-2 genes were mainly found in CC121 isolates (Harter et al., 2017). The presence of these genes increases the survival, resistance and persistence capacity of L. monocytogenes in the FPP, increasing the risk for consumers. In addition, the presence of Tn6188_qac transposon confers tolerance to BC disinfectant, mainly used for sanitation in food processing plants. In this study, in fact, CC9 was one of the main clones observed in the RTE meat products tested, and this finding indicates a need for improvement in the FPP sanitation. However, a PMSC mutation was detected in the inlA gene, confirming CC9 and CC121 clones as hypo-virulent (Maury et al., 2019; Guidi et al., 2021b). Moreover, L. monocytogenes strains showing PMSC in inlA were reported in literature, isolated both from clinical case (Magagna et al., 2023) and animal (Sévellec et al., 2020). Our results showed the CC5 as one of the main clones in RTE meat products, as reported also in China (Wang et al., 2015). In the study of Maury et al. (2016), CC5 was not classified as a hyper-virulent clone, but further studies are needed. This clone caused several outbreak cases, such as the cantaloupe case in the USA in 2011 (Lomonaco et al., 2013) and in a previous study by Zhang et al. (2019), the CC5 was reported as the second common clone in clinical L. monocytogenes strains in Beijing (China).
Regarding the minor CCs detected in this study, CC155 strains were mostly found in farm and environmental samples, but also this clone was detected worldwide both in food samples and in clinical cases (Matle et al., 2020). L. monocytogenes CC3 was reported as prevalent in a study conducted on cooked food tested in China and also in clinical samples (Wang et al., 2018). Similarly to previous data (Chen et al., 2019), and like CC1, the CC3 isolate reported in this study harbored a complete LIPI-3 that could be responsible for the increasing of virulence (Vilchis-Rangel et al., 2019). CC3 strains were also reported in poultry RTE foods during a previous outbreak in SA (Matle et al., 2020).
The comparison between L. monocytogenes detected in Zambian RTE and those selected from NCBI, available from SA, showed the presence of several relatedness’s, in particular for hyper-virulent CCs such as CC1 and CC2. This findings was crucial, highlighting how is important to continue to detect and characterize African L. monocytogenes strains in order to improve knowledge on strains circulation, surveillance and the monitoring of any possible clusters or new outbreak. About antimicrobial resistance L. monocytogenes strains of this study showed to be widely susceptible to clinically relevant classes of antibiotics (penicillin, carbapenems and quinolones) similar to previous reports (Wiśniewski et al., 2022). Our results also confirmed the intrinsic resistance to broad-spectrum cephalosporin antibiotics, commonly used in therapy of bacterial infections (Krawczyk-Balska and Markiewicz, 2016). WGS identification of antimicrobial resistance genes revealed that in all the L. monocytogenes tested, intrinsic core genes were observed, as expected, according to what is found in literature (Kurpas et al., 2020). Two strains showed to be phenotypically resistant to tetracycline, despite the absence of tet genes. This could be driven by adaptive mechanism such as the antimicrobial resistance determinants exchange with other bacteria through horizontal gene transfer or plasmid mobilization (Wiśniewski et al., 2022). Just one strains showed a resistant phenotype against trimethoprim-sulfamethoxazole concordant with the presence of sul gene. However, all the strains resulted susceptible to the antimicrobials used as first choice for the therapy of human listeriosis (Baquero et al., 2020). Listeria spp. isolated from different parts of Africa are generally susceptible to ciprofloxacin, but resistant to penicillin (Dufailu et al., 2021). Previous studies reported the increase in resistance of L. monocytogenes strains to antibiotics of different classes (Godreuil et al., 2003; Swetha et al., 2021). Keet and Rip (2021) described the presence of isolates susceptible to all antibiotics tested in RTE food, at the same time some isolates were resistant to erythromycin and tetracycline and some strains from polony were multi-drug resistant.
According to literature, the presence of genetic mobile elements, such as plasmids and prophages, increases the L. monocytogenes resistance. Interestingly, several studies indicated that some plasmids might confer increasing tolerance to stresses, heavy metals, quaternary ammonium compounds, salt, oxidative, heat, and cold stress (Mafuna et al., 2021; Schmitz-Esser et al., 2021). According to previous studies, among the hyper-virulent clones, few strains carried plasmids; on the contrary, the hypo-virulent clones normally show a higher number of plasmids (Schmitz-Esser et al., 2021). With the additional presence of disinfectant resistances, such as the bcrABC cassette and the Tn6188_qac for BC tolerance, various prophages and plasmids involved in stress response, the hyper-virulent clones could resist several stresses and probably persist in the environment for years. Data from literature highlighted the distribution of the hyper-virulent clones and their ability to contaminate, during the years and for long time, food products, food processing plants in Africa, increasing risk for human (Mafuna et al., 2021).
5. Conclusion
Although there has been listeriosis cases reported worldwide, L. monocytogenes data from Africa remains limited. Despite being a first preliminary work with a limitation in the sampling plan and a limited number of samples and isolates, this study reported our findings providing justification for a more complete surveys in future work, and helping to increase existing knowledge on contaminated RTE products circulating in Africa countries. Furthermore, this study highlighted interesting results about L. monocytogenes in Zambian RTE meat foods, confirming the presence of hyper-virulent CCs, which could play an important role in human diseases, posing a public health concern for consumers. Moreover, the presence of stress resistance factors could help these hyper-virulent clones to adapt, survive and persist over the years, as described by relatedness found in this study with other L. monocytogenes isolated in SA, during the years, from different matrices.
This preliminary study was conducted in order to test local Zambian foods available in local supermarkets, but also to allow generalizable findings. Further studies should be conducted on RTE products from Zambia and other African country, in order to deeply investigate the worldwide diffusion and the genomic characteristics of L. monocytogenes strains with particular attention on hyper-virulent clones aiming to improve worldwide surveillance and food safety.
In the future it will become crucial to improve the sampling plan, test more RTE foods and food environmental samples, but also to collect and characterize by WGS more L. monocytogenes isolates, especially from each positive sample, improving data and knowledge on L. monocytogenes in food products from Africa.
Due to the global increasing request, trade and consumption of foreign foods, it would be necessary to improve hygiene conditions and standardize procedures for hygiene and sanitation in food processing plants in Africa countries. Moreover, it could be useful to apply a better practices related to animal, agriculture and farming management in order to reduce L. monocytogenes in preharvest animals and consequentially in RTE meat products. According to One Health approach, it will be important compare available L. monocytogenes WGS data with clinical strains, in order to identify any clusters, including any potential connections to listeriosis cases and outbreaks.
Data availability statement
The datasets presented in this study can be found in online repositories. The names of the repository/repositories and accession number(s) can be found in the article/Supplementary material.
Author contributions
MZ, GC, GM, and FP conceptualized the study. MZ, BB, SM, and PM carried out sample collection, identification and isolation experiments. GC, ACo, ACh, and CC carried out the WGS experiment. ACh and GC carried out the bioinformatics analysis. GC, MZ, ACo, and ACh analyzed the data, organized the draft, and wrote the manuscript. FP, MT, and GM supervised the entire work. ND’A and MS acquired the funding. GC, MZ, ACo, ACh, MT, FG, CC, BB, SM, PM, ND’A, MS, FP, and GM contributed to editing the manuscript and reviewed the final version. All authors have read and approved the manuscript.
Funding
This work was supported financially by “Enhancing Research For Africa Network” (ERFAN) project, Italy, in collaboration with the Ministry of Fisheries and Livestock, Zambia.
Acknowledgments
We thank the National Reference Laboratory for Listeria monocytogenes and the National Reference Centre for Whole Genome Sequencing of microbial pathogens: database and bioinformatic analysis of Istituto Zooprofilattico Sperimentale dell’Abruzzo e del Molise “G. Caporale” (Teramo, Italy), Laura De Antoniis and Chiara Sensoli as ERFAN secretariat team. We also would like to thank Paola Di Giuseppe from the Library Services and Communication unit of Istituto Zoopofilattico Sperimentale dell’Abruzzo e del Molise “G. Caporale” (Teramo, Italy) for her graphic support.
Conflict of interest
The authors declare that the research was conducted in the absence of any commercial or financial relationships that could be construed as a potential conflict of interest.
Publisher’s note
All claims expressed in this article are solely those of the authors and do not necessarily represent those of their affiliated organizations, or those of the publisher, the editors and the reviewers. Any product that may be evaluated in this article, or claim that may be made by its manufacturer, is not guaranteed or endorsed by the publisher.
Supplementary material
The Supplementary material for this article can be found online at: https://www.frontiersin.org/articles/10.3389/fmicb.2023.1228726/full#supplementary-material
Footnotes
1. ^https://assets.thermofisher.com/TFS-Assets/MBD/brochures/Sensititre-Plate-Guide-Booklet-EN.pdf
References
Althaus, D., Jermini, M., Giannini, P., Martinetti, G., Reinholz, D., Nüesch-Inderbinen, M., et al. (2017). Local outbreak of Listeria monocytogenes serotype 4b sequence type 6 due to contaminated meat pâté. Foodborne Pathog. Dis. 14, 219–222. doi: 10.1089/fpd.2016.2232
Bankevich, A., Nurk, S., Antipov, D., Gurevich, A. A., Dvorkin, M., Kulikov, A. S., et al. (2012). SPAdes: a new genome assembly algorithm and its applications to single-cell sequencing. J. Comput. Biol. 19, 455–477. doi: 10.1089/cmb.2012.0021
Baquero, F., Lanza, V. F., Duval, M., and Coque, T. M. (2020). Ecogenetics of antibiotic resistance in Listeria monocytogenes. Mol. Microbiol. 113, 570–579. doi: 10.1111/mmi.14454
Bolger, A. M., Lohse, M., and Usadel, B. (2014). Trimmomatic: a flexible trimmer for Illumina sequence data. Bioinformatics 30, 2114–2120. doi: 10.1093/bioinformatics/btu170
Calvo-Arrieta, K., Matamoros-Montoya, K., Arias-Echandi, M. L., Huete-Soto, A., and Redondo-Solano, M. (2021). Presence of Listeria monocytogenes in ready-to-eat meat products sold at retail stores in Costa Rica and analysis of contributing factors. J. Food Prot. 84, 1729–1740. doi: 10.4315/JFP-21-020
Centorotola, G., Guidi, F., D'Aurizio, G., Salini, R., Di Domenico, M., Ottaviani, D., et al. (2021). Intensive environmental surveillance plan for Listeria monocytogenes in food producing plants and retail Stores of Central Italy: prevalence and genetic diversity. Foods 10:1944. doi: 10.3390/foods10081944
Chen, M., Cheng, J., Zhang, J., Chen, Y., Zeng, H., Xue, L., et al. (2019). Isolation, potential virulence, and population diversity of Listeria monocytogenes from meat and meat products in China. Front. Microbiol. 10:946. doi: 10.3389/fmicb.2019.00946
Chen, Y., Luo, Y., Carleton, H., Timme, R., Melka, D., Muruvanda, T., et al. (2017a). Whole genome and core genome multilocus sequence typing and single nucleotide polymorphism analyses of Listeria monocytogenes isolates associated with an outbreak linked to cheese, United States, 2013. Appl. Environ. Microbiol. 83, e00633–e00617. doi: 10.1128/AEM.00633-17
Chen, Y., Luo, Y., Curry, P., Timme, R., Melka, D., Doyle, M., et al. (2017b). Assessing the genome level diversity of Listeria monocytogenes from contaminated ice cream and environmental samples linked to a listeriosis outbreak in the United States. PLoS One 12:e0171389. doi: 10.1371/journal.pone.0171389
Chuku, A., Obande, G. A., and Eya, S. B. (2020). Listerial contamination of raw beef and chevon in north-Central Nigeria. IMC J Med Sci. 13, 1–8. doi: 10.3329/imcjms.v13i2.45274
Cito, F., Di Pasquale, A., Cammà, C., and Cito, P. (2018). The Italian information system for the collection and analysis of complete genome sequence of pathogens isolated from animal, food and environment. Int. J. Infect. Dis. 73, 296–297. doi: 10.1016/j.ijid.2018.04.4090
Dufailu, O. A., Yaqub, M. O., Owusu-Kwarteng, J., and Addy, F. (2021). Prevalence and characteristics of Listeria species from selected African countries. Trop Dis Travel Med Vaccines. 7:26. doi: 10.1186/s40794-021-00151-5
Duranti, A., Sabbatucci, M., Blasi, G., Acciari, V. A., Ancora, M., Bella, A., et al. (2018). A severe outbreak of listeriosis in Central Italy with a rare pulsotype associated with processed pork products. J. Med. Microbiol. 67, 1351–1360. doi: 10.1099/jmm.0.000785
EUCAST (European Committee on Antimicrobial Susceptibility Testing). (2021). The European Committee on antimicrobial susceptibility testing. Breakpoint tables for interpretation of MICs and zone diameters, version 11.0.
European Food Safety Authority (2022). The European Union one health 2021 Zoonoses report. EFSA J. 20:7666. doi: 10.2903/j.efsa.2022.7666
Félix, B., Feurer, C., Maillet, A., Guillier, L., Boscher, E., Kerouanton, A., et al. (2018). Population genetic structure of Listeria monocytogenes strains isolated from the pig and pork production chain in France. Front. Microbiol. 9:684. doi: 10.3389/fmicb.2018.00684
Fischer, M. A., Wamp, S., Fruth, A., Allerberger, F., Flieger, A., and Halbedel, S. (2020). Population structure-guided profiling of antibiotic resistance patterns in clinical Listeria monocytogenes isolates from Germany identifies pbpB3 alleles associated with low levels of cephalosporin resistance. Emerg. Microbes Infect. 9, 1804–1813. doi: 10.1080/22221751.2020.1799722
Forauer, E., Wu, S. T., and Etter, A. J. (2021). Listeria monocytogenes in the retail deli environment: a review. Food Control 119:107443. doi: 10.1016/j.foodcont.2020.107443
Garedew, L., Taddese, A., Biru, T., Nigatu, S., Kebede, E., Ejo, M., et al. (2015). Prevalence and antimicrobial susceptibility profile of listeria species from ready-to-eat foods of animal origin in Gondar Town, Ethiopia. BMC Microbiol. 15:100. doi: 10.1186/s12866-015-0434-4
Gelbíčová, T., Zobaníková, M., Tomáštíková, Z., Van Walle, I., Ruppitsch, W., and Karpíšková, R. (2018). An outbreak of listeriosis linked to Turkey meat products in the Czech Republic, 2012-2016. Epidemiol. Infect. 146, 1407–1412. doi: 10.1017/S0950268818001565
Godreuil, S., Galimand, M., Gerbaud, G., Jacquet, C., and Courvalin, P. (2003). Efflux pump Lde is associated with fluoroquinolone resistance in Listeria monocytogenes. Antimicrob. Agents Chemother. 47, 704–708. doi: 10.1128/AAC.47.2.704-708.2003
Gray, J. A., Chandry, P. S., Kaur, M., Kocharunchitt, C., Bowman, J. P., and Fox, E. M. (2021). Characterisation of Listeria monocytogenes food-associated isolates to assess environmental fitness and virulence potential. Int. J. Food Microbiol. 350:109247. doi: 10.1016/j.ijfoodmicro.2021.109247
Guidi, F., Chiaverini, A., Repetto, A., Lorenzetti, C., Centorotola, G., Bazzucchi, V., et al. (2021a). Hyper-virulent Listeria monocytogenes strains associated with respiratory infections in Central Italy. Front. Cell. Infect. Microbiol. 11:765540. doi: 10.3389/fcimb.2021.765540
Guidi, F., Orsini, M., Chiaverini, A., Torresi, M., Centorame, P., Acciari, V. A., et al. (2021b). Hypo- and hyper-virulent Listeria monocytogenes clones persisting in two different food processing plants of Central Italy. Microorganisms 9:376. doi: 10.3390/microorganisms9020376
Gupta, P., and Achyut, A. (2022). Novel approaches to environmental monitoring and control of Listeria monocytogenes in food production facilities. Foods 12:1760. doi: 10.3390/foods11121760
Gurevich, A., Saveliev, V., Vyahhi, N., and Tesler, G. (2013). QUAST: quality assessment tool for genome assemblies. Bioinformatics 29, 1072–1075. doi: 10.1093/bioinformatics/btt086
Harter, E., Wagner, E. M., Zaiser, A., Halecker, S., Wagner, M., and Rychli, K. (2017). Stress survival islet 2, predominantly present in Listeria monocytogenes strains of sequence type 121, is involved in the alkaline and oxidative stress responses. Appl. Environ. Microbiol. 83, e00827–e00817. doi: 10.1128/AEM.00827-17
Heredia, N., and García, S. (2018). Animals as sources of food-borne pathogens: a review. Anim. Nutr. 4, 250–255. doi: 10.1016/j.aninu.2018.04.006
ISO (International Organization for Standardization) (2017). Microbiology of the food chain - horizontal method for the detection and enumeration of Listeria monocytogenes and of Listeria spp. Part 1: detection method, international standard; ISO 11290-1, 2017. International Organization for Standardization: Geneva.
Ishola, O. O., Mosugu, J. I., and Adesokan, H. K. (2016). Prevalence and antibiotic susceptibility profiles of Listeria monocytogenes contamination of chicken flocks and meat in Oyo state, South-Western Nigeria. J. Prev. Med. Hyg. 57, 157–163.
Keet, R., and Rip, D. (2021). Listeria monocytogenes isolates from Western cape, South Africa exhibit resistance to multiple antibiotics and contradicts certain global resistance patterns. AIMS Microbiol. 7, 40–58. doi: 10.3934/microbiol.2021004
Krawczyk-Balska, A., and Markiewicz, Z. (2016). The intrinsic cephalosporin resistome of Listeria monocytogenes in the context of stress response, gene regulation, pathogenesis and therapeutics. J. Appl. Microbiol. 120, 251–265. doi: 10.1111/jam.12989
Kurpas, M., Osek, J., Moura, A., Leclercq, A., and Lecuit, M., and Wieczorek, K. (2020). Genomic characterization of listeria monocytogenes isolated from ready-to-eat meat and meat processing environments in poland. Front Microbiol. 11:1412. doi: 10.3389/fmicb.2020.01412
Kurpas, M., Wieczorek, K., and Osek, J. (2018). Ready-to-eat meat products as a source of Listeria monocytogenes. J Vet Res. 62, 49–55. doi: 10.2478/jvetres-2018-0007
Lee, B. H., Cole, S., Badel-Berchoux, S., Guillier, L., Felix, B., Krezdorn, N., et al. (2019). Biofilm formation of Listeria monocytogenes strains under food processing environments and pan-genome-wide association study. Front. Microbiol. 10, 1–18. doi: 10.3389/fmicb.2019.02698
Lomonaco, S., Verghese, B., Gerner-Smidt, P., Tarr, C., Gladney, L., Joseph, L., et al. (2013). Novel epidemic clones of Listeria monocytogenes, United States, 2011. Emerg. Infect. Dis. 19, 147–150. doi: 10.3201/eid1901.121167
Mafuna, T., Matle, I., Magwedere, K., Pierneef, R. E., and Reva, O. N. (2021). Whole genome-based characterization of Listeria monocytogenes isolates recovered from the food chain in South Africa. Front. Microbiol. 12:669287. doi: 10.3389/fmicb.2021.669287
Magagna, G., Gori, M., Russini, V., De Angelis, V., Spinelli, E., Filipello, V., et al. (2023). Evaluation of the virulence potential of Listeria monocytogenes through the characterization of the truncated forms of Internalin a. Int. J. Mol. Sci. 24:10141. doi: 10.3390/ijms241210141
Matle, I., Mafuna, T., Madoroba, E., Mbatha, K. R., Magwedere, K., and Pierneef, R. (2020). Population structure of non-ST6 Listeria monocytogenes isolated in the red meat and poultry value chain in South Africa. Microorganisms 8, 1–18. doi: 10.3390/microorganisms8081152
Matle, I., Mbatha, K. R., Lentsoane, O., Magwedere, K., Morey, L., and Madoroba, E. (2019). Occurrence, serotypes, and characteristics of Listeria monocytogenes in meat and meat products in South Africa between 2014 and 2016. J. Food Saf. 39, 1–14. doi: 10.1111/jfs.12629
Maury, M. M., Bracq-Dieye, H., Huang, L., Vales, G., Lavina, M., Thouvenot, P., et al. (2019). Hypervirulent Listeria monocytogenes clones’ adaption to mammalian gut accounts for their association with dairy products. Nat. Commun. 10:2488. doi: 10.1038/s41467-019-10380-0
Maury, M., Tsai, Y. H., Charlier, C., Touchon, M., Chenal-Francisque, V., and Leclercq, A. (2016). Uncovering Listeria monocytogenes hypervirulence by harnessing its biodiversity. Nat. Genet. 48, 308–313. doi: 10.1038/ng.3501
Moura, A., Criscuolo, A., Pouseele, H., Maury, M. M., Leclercq, A., Tarr, C., et al. (2016). Whole genome-based population biology and epidemiological surveillance of Listeria monocytogenes. Nat. Microbiol. 2:16185. doi: 10.1038/nmicrobiol.2016.185
Mpundu, P., Muma, J. B., Mukubesa, A. N., Kainga, H., Mudenda, S., Bumbangi, F. N., et al. (2022a). Antibiotic resistance patterns of Listeria species isolated from broiler abattoirs in Lusaka, Zambia. Antibiotics. 11:591. doi: 10.3390/antibiotics11050591
Mpundu, P., Muma, J. B., Mukumbuta, N., Mukubesa, A. N., Muleya, W., Kapila, P., et al. (2022b). Isolation, discrimination, and molecular detection of Listeria species from slaughtered cattle in Namwala District, Zambia. BMC Microbiol. 22:160. doi: 10.1186/s12866-022-02570-6
Odu, N. N., and Okonko, I. O. (2017). Prevalence and antibiotic susceptibility of Listeria monocytogenes in retailed meats in Port Harcourt Metropolis, Nigeria. Public Heal Res. 7, 91–99. doi: 10.5923/j.phr.20170704.02
Orsini, M., Torresi, M., Patavino, C., Centorame, P., Rinaldi, A., Acciari, V. A., et al. (2018). Whole-genome sequence of a reemerging Listeria monocytogenes serovar 1/2a strain in Central Italy. Microbiol Resour Announc. 7, e01069–e01018. doi: 10.1128/MRA.01069-18
Osek, J., and Wieczorek, K. (2022). Listeria monocytogenes-how this pathogen uses its virulence mechanisms to infect the hosts. Pathogens. 11:1491. doi: 10.3390/pathogens11121491
Oyinloye, J. M. A. (2016). Detection and molecular characterization of Listeria species in ‘Wara’, a west African local cheese sold in Ekiti state. Int. J. Curr. Microbiol. App. Sci. 5, 941–948. doi: 10.20546/ijcmas.2016.506.101
Portmann, A. C., Fournier, C., Gimonet, J., Ngom-Bru, C., Barretto, C., and Baert, L. (2018). A validation approach of an end-to-end whole genome sequencing workflow for source tracking of Listeria monocytogenes and Salmonella enterica. Front. Microbiol. 9:446. doi: 10.3389/fmicb.2018.00446
Schjørring, S., Gillesberg Lassen, S., Jensen, T., Moura, A., Kjeldgaard, J. S., Müller, L., et al. (2017). Cross-border outbreak of listeriosis caused by cold-smoked salmon, revealed by integrated surveillance and whole genome sequencing (WGS), Denmark and France, 2015 to 2017. Euro Surveill. 22, 17–00762. doi: 10.2807/1560-7917.ES.2017.22.50.17-00762
Schmitz-Esser, S., Anast, J. M., and Cortes, B. W. (2021). A large-scale sequencing-based survey of plasmids in Listeria monocytogenes reveals global dissemination of plasmids. Front. Microbiol. 12:653155. doi: 10.3389/fmicb.2021.653155
Sévellec, Y., Torresi, M., Félix, B., Palma, F., Centorotola, G., Bilei, S., et al. (2020). First report on the finding of Listeria mnocytogenes ST121 strain in a dolphin brain. Pathogens (Basel, Switzerland) 9:802. doi: 10.3390/pathogens9100802
Smith, A. M., Tau, N. P., Smouse, S. L., Allam, M., Ismail, A., Ramalwa, N. R., et al. (2019). Outbreak of Listeria monocytogenes in South Africa, 2017-2018: laboratory activities and experiences associated with whole-genome sequencing analysis of isolates. Foodborne Pathog. Dis. 16, 524–530. doi: 10.1089/fpd.2018.2586
Su, X., Cao, G., Zhang, J., Pan, H., Zhang, D., Kuang, D., et al. (2019). Characterization of internalin genes in Listeria monocytogenes from food and humans, and their association with the invasion of Caco-2 cells. Gut Pathog. 11, 1–10. doi: 10.1186/s13099-019-0307-8
Swetha, C. S., Porteen, K., Elango, A., Ronald, B. S. M., Senthil Kumar, T. M. A., Milton, A. P., et al. (2021). Genetic diversity, virulence and distribution of antimicrobial resistance among Listeria monocytogenes isolated from milk, beef, and bovine farm environment. Iran. J. Vet. Res 22, 1–8. doi: 10.22099/ijvr.2020.37618.5472
Tavares, R. M., Silva, D. A. L. D., Camargo, A. C., Yamatogi, R. S., and Nero, L. A. (2020). Interference of the acid stress on the expression of llsX by Listeria monocytogenes pathogenic island 3 (LIPI-3) variants. Food Res. Int. 132:109063. doi: 10.1016/j.foodres.2020.109063
Thomas, J., Govender, N., McCarthy, K. M., Erasmus, L. K., Doyle, T. J., Allam, M., et al. (2020). Outbreak of listeriosis in South Africa associated with processed meat. N. Engl. J. Med. 382, 632–643. doi: 10.1056/NEJMoa1907462
Timme, R. E., Wolfgang, W. J., Balkey, M., Venkata, S. L. G., Randolph, R., Allard, M., et al. (2020). Optimizing open data to support one health: best practices to ensure interoperability of genomic data from bacterial pathogens. One Health Outlook 2:20. doi: 10.1186/s42522-020-00026-3
Vilchis-Rangel, R. E., Espinoza-Mellado, M. D. R., Salinas-Jaramillo, I. J., Martinez-Peña, M. D., and Rodas-Suárez, O. R. (2019). Association of Listeria monocytogenes LIPI-1 and LIPI-3 marker llsX with invasiveness. Curr. Microbiol. 76, 637–643. doi: 10.1007/s00284-019-01671-2
Wang, H., Luo, L., Zhang, Z., Deng, J., Wang, Y., Miao, Y., et al. (2018). Prevalence and molecular characteristics of Listeria monocytogenes in cooked products and its comparison with isolates from listeriosis cases. Front. Med. 12, 104–112. doi: 10.1007/s11684-017-0593-9
Wang, G., Qian, W., Zhang, X., Wang, H., Ye, K., Bai, Y., et al. (2015). Prevalence, genetic diversity and antimicrobial resistance of Listeria monocytogenes isolated from ready-to-eat meat products in Nanjing, China. Food Control 50, 202–208. doi: 10.1016/j.foodcont.2014.07.057
Wingett, S. W., and Andrews, S. (2018). FastQ screen: a tool for multi-genome mapping and quality control. F1000Res 7:1338. doi: 10.12688/f1000research.15931.1
Wiśniewski, P., Zakrzewski, A. J., Zadernowska, A., and Chajęcka-Wierzchowska, W. (2022). Antimicrobial resistance and virulence characterization of Listeria monocytogenes strains isolated from food and food processing environments. Pathogens 11:1099. doi: 10.3390/pathogens11101099
Yin, Y., Yao, H., Doijad, S., Kong, S., Shen, Y., Cai, X., et al. (2019). A hybrid sub-lineage of Listeria monocytogenes comprising hypervirulent isolates. Nat. Commun. 10:4283. doi: 10.1038/s41467-019-12072-1
Zhang, Y., Dong, S., Chen, H., Chen, J., Zhang, J., Zhang, Z., et al. (2019). Prevalence, genotypic characteristics and antibiotic resistance of Listeria monocytogenes from retail foods in bulk in Zhejiang Province, China. Front. Microbiol. 10, 1–14. doi: 10.3389/fmicb.2019.01710
Keywords: ready to eat, meat products, African countries, Listeria monocytogenes , whole genome sequencing
Citation: Centorotola G, Ziba MW, Cornacchia A, Chiaverini A, Torresi M, Guidi F, Cammà C, Bowa B, Mtonga S, Magambwa P, D’Alterio N, Scacchia M, Pomilio F and Muuka G (2023) Listeria monocytogenes in ready to eat meat products from Zambia: phenotypical and genomic characterization of isolates. Front. Microbiol. 14:1228726. doi: 10.3389/fmicb.2023.1228726
Edited by:
Arun K. Bhunia, Purdue University, United StatesReviewed by:
Matthew J. Stasiewicz, University of Illinois at Urbana-Champaign, United StatesBeatrix Stessl, University of Veterinary Medicine Vienna, Austria
Copyright © 2023 Centorotola, Ziba, Cornacchia, Chiaverini, Torresi, Guidi, Cammà, Bowa, Mtonga, Magambwa, D’Alterio, Scacchia, Pomilio and Muuka. This is an open-access article distributed under the terms of the Creative Commons Attribution License (CC BY). The use, distribution or reproduction in other forums is permitted, provided the original author(s) and the copyright owner(s) are credited and that the original publication in this journal is cited, in accordance with accepted academic practice. No use, distribution or reproduction is permitted which does not comply with these terms.
*Correspondence: Alessandra Cornacchia, YS5jb3JuYWNjaGlhQGl6cy5pdA==
†These authors have contributed equally to this work