Corrigendum: Enhanced biodegradation of phenanthrene and anthracene using a microalgal-bacterial consortium
- 1Department of Bioengineering, King Fahd University of Petroleum and Minerals, Dhahran, Saudi Arabia
- 2Department of Chemistry, King Fahd University of Petroleum and Minerals, Dhahran, Saudi Arabia
- 3Interdisciplinary Research Center for Refining and Advanced Chemicals, King Fahd University of Petroleum and Minerals, Dhahran, Saudi Arabia
- 4Interdisciplinary Research Center for Membranes and Water Security, King Fahd University of Petroleum and Minerals, Dhahran, Saudi Arabia
- 5Environmental Research and Innovation (ERIN) Department, Luxembourg Institute of Science and Technology, Hautcharage, Luxembourg
- 6School of Biotechnology and Biomolecular Sciences (BABS), The University of New South Wales, Sydney, NSW, Australia
Polycyclic aromatic hydrocarbons (PAHs) are chemicals that are released into the environment during activities of the petroleum industry. The bioaccumulation, carcinogenic and mutagenic potential of PAHs necessitates the bioremediation of these contaminants. However, bioremediation of PAHs has a number of limitations including the inability of a single microbe to degrade all of the PAH fraction’s environmental constituents. Therefore, a different paradigm, employing microalgal-bacterial consortium (MBC), may be used to effectively remove PAHs contaminants. In this type of interaction, the microalgae and bacteria species in the consortium work together in a way that enhances the overall performance of the MBC. Bacterial species in the consortium provide essential nutrients or growth factors by degrading toxic substances and provide these to microalgae, while the microalgae species provide organic carbon for the bacterial species to grow. For the first time, the ability of Gonium pectorale (G. pectorale) microalgae to break down phenanthrene (PHE) and anthracene (ANT) was investigated. Phenanthrene was shown to be more effectively degraded by G. pectorale (98%) as compared to Bacillus licheniformis (B. licheniformis) 19%. Similarly, G. pectorale has effectively degrade anthracene (98%) as compared with B. licheniformis (45%). The consortia of G. pectorale and B. licheniformis has shown a slight increase in the degradation of PHE (96%) and ANT (99%). Our findings show that B. licheniformis did not inhibit the growth of G. pectorale and in the consortia has effectively eliminated the PAHs from the media. Therefore G. pectorale has a tremendous potential to remove PAHs from the polluted environment. Future research will be conducted to assess Gonium’s capacity to eliminate PAHs that exhibit high molar masses than that of PHE and ANT.
Introduction
Across recent decades, environmental pollution has been a global problem of great concern due to its detrimental effects on both human health and ecosystems. Anthropogenic activities have discharged a significant amount of pollutants into the environment, and the petroleum sector produces vast amounts of crude oil wastewater with significant pollution (Mojiri et al., 2019; Olajire, 2020; Alvarez-Ospina et al., 2021). Numerous investigations have shown that toxic substances, such as aliphatic or aromatic hydrocarbons, phenolic compounds, heavy metals, their derivatives, and chemicals used in the de-emulsification process, are always present in crude oil discharge (Trannum et al., 2011; Uzoekwe and Oghosanine, 2011). The repercussions for living things of the buildup of such substances in the associated water bodies are severe (Ngwoke et al., 2021).
Polycyclic aromatic hydrocarbons (PAHs), which have two or more fused aromatic rings arranged angularly, linearly, or in clusters, are regarded as a fundamental component of petroleum sewage. They are discovered in crude oil, which is created through the burning of fuel, the burning of waste, or as a byproduct of industrial activities like petroleum exploration (Suman et al., 2016; Lawal, 2017). Given their water solubility, toxicity, bioaccumulation potential, and persistent character, PAHs have been referred to as the main environmental pollutants that pose the greatest health risks (Dong et al., 2012; Adeniji et al., 2019; Patel et al., 2020). Various physicochemical, thermal, and photolytic remediation techniques are used; however, they have drawbacks such as the use of a high chemical dosage, energy demand, high investment, massive operating costs and cannot entirely rid the environment of toxins from the polluted site along with their negative effect on the environment (e.g., spent washing solution, air pollution, flora destruction). Hence, bioremediation with microorganisms is a sustainable, socially acceptable, and environmentally benign alternative to traditional remediation techniques that helps to restore the environment (Abdel-Shafy and Mansour, 2016; Gupte et al., 2016; Qutob et al., 2022). The sequence of decreasing susceptibility is followed by the biodegradability of petroleum hydrocarbons: linear alkanes > branched alkanes > monoaromatics > cyclic alkanes > polyaromatics > asphaltenes (Varjani and Upasani, 2017).
Bacteria often existed in a variety of habitats and were uniquely metabolically adaptable to break down PAHs in both aerobic and anaerobic environments. O2, which serves as both a co-substrate for the hydroxylation process involving the monooxygenase and dioxygenase enzymes and an absolute electron acceptor in aerobic conditions, breaks down an aromatic ring of a PAH molecule (Chen et al., 2016). While bacteria use an altogether different strategy to break down an aromatic ring in the absence of oxygen, subjecting it to reductases and other final electron acceptors. In ecosystems like phreatic zone, deep sea sediment, and water-flooded soil, anaerobic breakdown of PAHs is more successful (Ghosal et al., 2016; Dhar et al., 2020). PAHs are naturally broken down by bacteria to provide them with the carbon they need for energy. However, the cytotoxic effects of petroleum hydrocarbons, poor climatic circumstances, longer time, metabolic restrictions, hydrocarbon composition, and concentration inhibit its capacity to degrade (Xu et al., 2018).
The importance of microalgae, which are photosynthetic microorganisms present in terrestrial and marine ecosystems, has increased due to their widespread distribution, ease of cultivation, and exceptional capacity to degrade PAHs. The degradation of PAHs by microalgae has been documented in certain research at the laboratory or microcosm size, but field-scale PAH cleanup requires the development and optimization of advanced techniques. Several microalgae strains have been found to breakdown PAHs such as benzo[a]pyrene, phenanthrene, anthracene, and naphthalene (de Llasera et al., 2016, 2018).
For the biodegradation of resistant PAHs, researchers are currently concentrating on microalgae bacterium consortia. This co-culturing method, which combines bacteria and microalgae, is more advantageous than employing just one species and is thus more promising (Ahmad, 2021; García et al., 2021). The organisms coexist in a symbiotic relationship in a microalgae bacteria consortium, where microalgae provide oxygen during photosynthesis that may promote bacterial development. Furthermore, produced for the growth of microalgae are several vital vitamins, minerals, and trace elements, as well as crucial phytohormones, chelating agents, and other substances (Wang et al., 2016). Moreover, the bacteria may have a stable environment to grow on the microalgae cell surface, while pollutants present there may create a pollutant-augmented zone that will hasten the degradation of PAHs (Gutierrez et al., 2014). The greatest method to increase the degradation capability of these organisms in the removal of persistent PAHs from the contaminated ecosystems in the biosphere may be the symbiotic association between microalgae and bacteria (Kleindienst et al., 2015; Perera et al., 2018; Yao et al., 2018).
To the best of our knowledge, the symbiotic association between microalgae and bacteria has never been documented in the literature. The goals of this study were to (1) test the ability of green microalgae (G. pectorale) and bacteria (B. licheniformis) to grow together in the same media, and (2) determine the PAHs (phenanthrene and anthracene) degradation potential of microalgae and bacteria separately and in consortia settings.
Methods and materials
Culture media and microorganisms used in this study
G. pectorale obtained from the Microbial Culture Collection at the National Institute for Environmental Studies, Tsukuba, Japan; Available online: http://mcc.nies.go.jp/ was grown under continuous light (1,300 lux) in 50 mL of modified Bold’s 3 N medium adjusted to pH 6.8 (Guerriero et al., 2018). B. licheniformis isolated from petroleum contaminated soil in a previous study (Nzila et al., 2019) was used to test its compatibility with G. pectorale. For reviving bacterial cultures, a loop-full of bacteria from stock plates were transferred to nutrient broth and grown overnight at 37°C in a rotary shaker (Patel et al., 2015; Kahla et al., 2021).
G. pectorale was grown for a month in one-liter B3N in two 2-L flasks (total 2.0 L algae culture from both flasks combined). After 30 days, algae from both flasks were concentrated to 20 mL by centrifugation. This concentrated solution was then utilized in the main experiments. Bacteria were grown in 200 mL NB overnight whenever needed. Bacterial solution was always concentrated and adjusted to OD 1.0 for all experiments involving bacteria.
PAH preparation
The ability of the microbial strains to degrade phenanthrene (PHE) and anthracene (ANT) was tested in this experiment. Stock solutions of the PAHs were prepared by separately dissolving 0.1 g of each of the PAHs in 10 mL dimethyl sulfoxide (DMSO) to make a final concentration of 10,000 ppm. Next, working concentration of 5 ppm (5 mg/L) for the experiments were calculated using the formula C1V1 = C2V2 (Nzila et al., 2018).
Algae-bacteria growth compatibility experiments
For compatibility experiments, 200 mL of B3N medium adjusted to pH 6.8 was dispensed aseptically into each 500 milliliters Erlenmeyer and prepared as follows (Table 1).
Next, 1.0 mL suspension of isolates in a ratio of 1:1 for microalgae and bacteria was aseptically dispensed into the appropriate flasks. Culture temperature was maintained at 30°C in a rotary shaker at 170 rpm. All cultures received light from a white LED source (1,300 lux) at a light: dark ratio of 15:9 h/day. Experiments were conducted for 30 days.
To monitor growth of G. pectorale, 20.0 mL of culture media was removed weekly from algae cultures and filtered for dry weight measurement. Cultures were removed from flasks using sterile disposable 10 mL pipettes. Weight (W1) of dry (empty) filter papers were recorded prior to usage. After filtering, the filter papers with the G. pectorale were allowed to dry before measuring the weight again (W2). The weight of G. pectorale was then calculated (dry weight of algae = W2 – W1) as described by Ratha et al. (2016).
Bacterial growth was measured with plate count technique every 3 days. Bacterial cultures (1.0 mL) were removed from their respective flasks and serially diluted. Diluted cultures were then streaked onto nutrient agar plates and kept overnight at 37°C. The following day, colonies were counted on plates having 30–300 colonies per plate. Next, the CFU/ml was calculated for the original samples to generate growth curves (Nzila et al., 2021).
PAH degradability experiments
For degradability experiments, 50.0 mL of B3N medium adjusted to pH 6.8 was dispensed aseptically into each 250-ml Erlenmeyer flasks and prepared as reported previously with some modification (Nzila et al., 2021) (Table 2).
Process of dispensing PAHs in the flasks
Microalgae and bacteria were dispensed as described earlier. All culture conditions were also as mentioned above. Experiments were conducted for 30 days without any disturbance in-between. Residual PAHs in the media were measured at one time point only, i.e., after 30 days the residual PAHs were extracted and then analyzed by gas chromatography (GC).
Quantification of PAHs
After 30 days, the growth was stopped by adding 50 mL ethyl acetate to each flask followed by vigorous extraction. The organic layer containing the residual PAHs was carefully removed and transferred to a dry flask. The extraction was repeated twice with another 50 mL ethyl acetate for all flasks to ensure that all residual PAHs were extracted for GC analysis (Nzila et al., 2021). The combined organic layer was dried using sodium sulfate then concentrated under vacuum to evaporate ethyl acetate. The remaining residue was dissolved in chloroform and transferred to 2 mL GC vials to quantify the remaining PAHs. The following GC method was used. The column oven temperature was set at 130°C and with a hold time of 2 min. Next, a temperature ramps up to 250°C at 11°C/min with a final hold time of 50 min. Post run, a temperature increases up once again to 325°C and hold for 5 min before starting a new run. The total run time for one sample was 63 min approximately. Helium was used as the carrier gas at a flow rate of 1 mL/min. Sample injection volume was 1 μL. Standard samples of PHE and ANT dissolved in chloroform at 5 ppm concentrations were injected using the same GC method to determine their retention time.
Following the GC run, the peak areas obtained were used to calculate the percentage of PAHs removed from the samples by the individual organisms and consortia. The peak area from experimental controls were considered to be 100% remaining, i.e., the peak area from other samples were compared in relation to the control peak area (Patel et al., 2015; Kahla et al., 2021). Table 3 shows the current study compared with previously published work using microalgae-bacteria consortia.
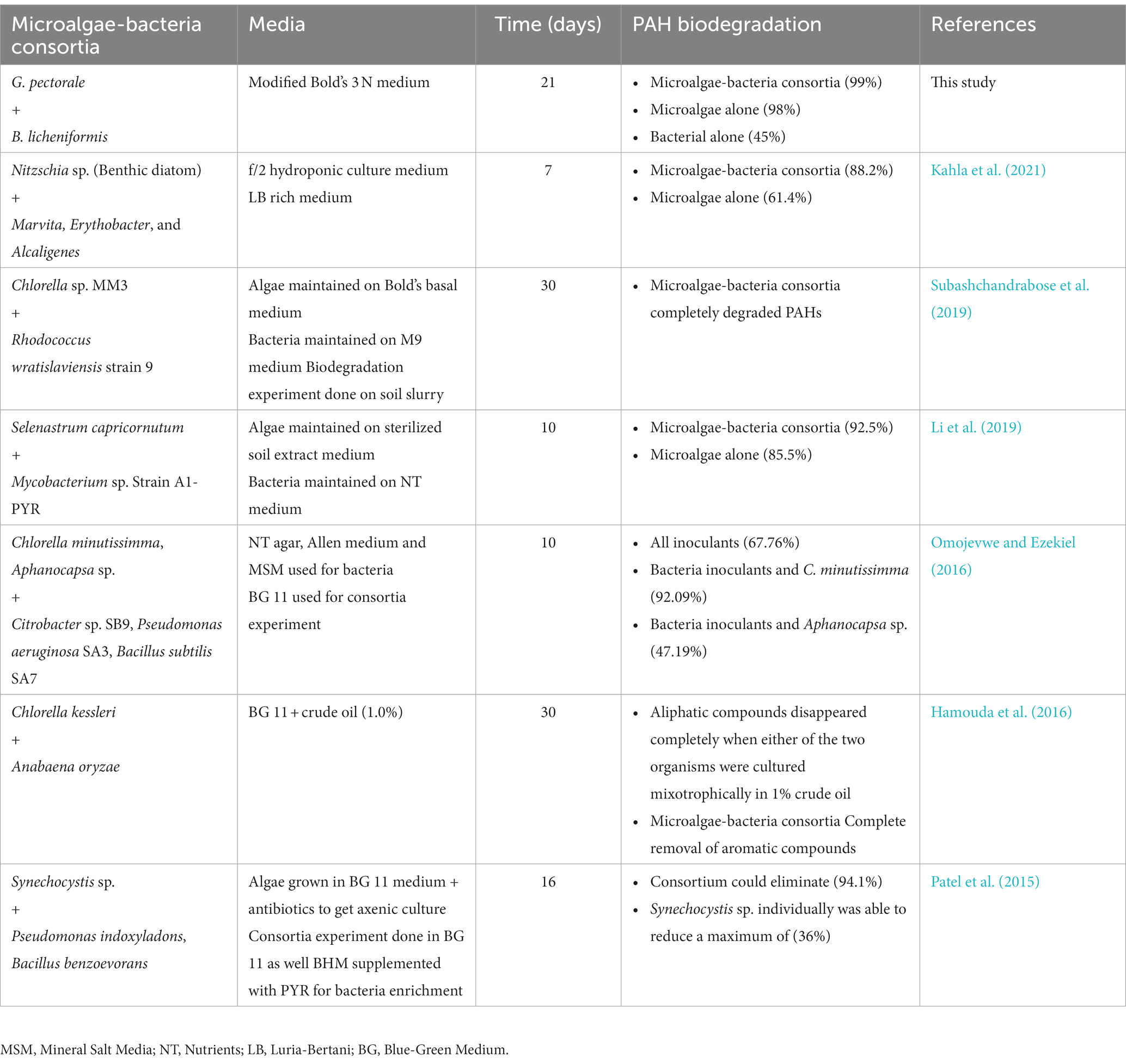
Table 3. Studies conducted to determine the potential of microalgae-bacteria consortia in degrading PAHs.
Analysis of intermediate metabolites during the PHE and ANT degradation
The gas chromatography (GC)- mass spectrometry (MS) analysis is conducted by using an Agilent 5975B MS coupled to 6,890 N GC. The GC separation was performed by using an HP-5 column (30 m length, 0.25 mm I.D., 0.25 μm film thickness) with Helium as the carrier gas. According to the protocol (Nzila et al., 2018) 1 μL Injections of the sample solutions were performed in a splitless mode. The GC oven program increased by 25°C per minute from 60°C to 150°C, then 10°C per minute to reach 260°C (this temperature lasts for 20 min), and afterwards it increased to 270°C (which also lasts for 20 min).
Results and discussion
Growth compatibility experiments
Overall, the growth of G. pectorale in the experiment performed well and was not hindered by the bacteria. The presence of PHE and ANT seemed to support its growth. G. pectorale alone and in consortia but without any PAH, showed a decrease in dry weight after 15 days. However, highest dry weight was observed in G. pectorale without any PAH (0.028 g). In the consortia experiment dry weight was slightly lower, i.e., 0.018 g. In both G-PHE and GB-PHE samples, the increase in dry weight of G. pectorale was slower (Figures 1A,B) respectively. The final dry weight was also lower than the final dry weights obtained in the presence of PHE and ANT which would have likely slowed down the growth rate of G. pectorale. Even visually, when PHE was added to the samples, it initially had a bleaching effect on G. pectorale. The green color in all G. pectorale samples with PHE was slightly cloudier than the rest of the G. pectorale samples. Perhaps, PHE being a three-ringed compound could have contributed to the growth patterns observed. In the consortia sample, the G. pectorale was seen to have an even slower growth rate than in the G-PHE sample when measured on day 7. Measured on the 15th day, the dry weights obtained for G. pectorale in the G-PHE and GB-PHE samples were 0.028 and 0.025 g/mL ± SD, respectively. In this case, again, why G. pectorale behaves in this manner can only be properly answered by further understanding of the interactions between algae and bacterial cells. To speculate, the lower rate of algal growth in the consortia may be due to a lack of CO2 which is to some extent contributed by bacteria. Support for this argument can be seen in Figure 1B where it is seen that the bacteria in the GB-PHE sample also decreased since the start of the experiment. Apart from B-PHE and GB-PHE, bacteria in all other samples showed an initial increase in cell count prior to its decrease. In the presence of PHE, the bacteria do not seem to thrive in the media and conditions provided in this study. Therefore, a continuous decrease of bacterial cells in the GB-PHE sample could be responsible for a decline in CO2 concentration in the sample which might have led to the slowest growth of G. pectorale in this sample. In further studies, it may be worth monitoring the CO2 concentrations in the samples to get a clearer picture of the growth kinetics and interactions of the organisms in the consortia.
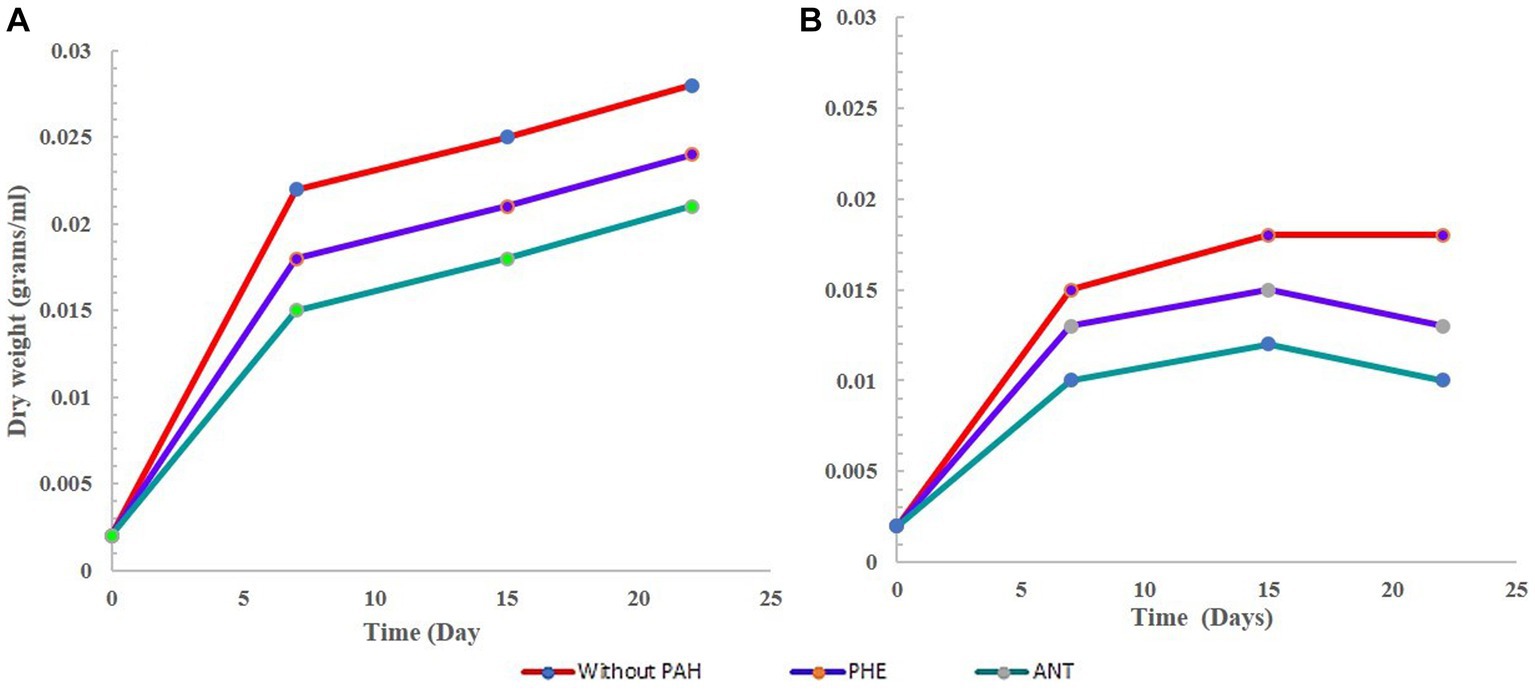
Figure 1. Growth curve of G. pectorale without any PHE and ANT. (A) Growth curve of G. pectorale alone. (B) Growth curve for G. pectorale in consortia.
In the bacterial sample (B-only), the results are not surprising regarding the growth pattern. The curve in Figure 2A shows a dramatic decrease in cell count measured on day 5 followed by a further decrease. This is expected since the B3N media has no carbon source, and no PAH was added. In its G-only counterpart, we see an increase in dry weight as the cells divide and grow during photosynthesis. The bacteria in the GB-only sample (Figure 2B) shows that within the first 8 days, bacterial cell counts increased due to in the presence of G. pectorale in the consortia. When cultured individually without any PAH, it showed a decrease in cell count from the initial 3.0 × 107 CFU/mL to 1 × 107 CFU/mL on the 12th day until finally no growth was observed on the plates (Figure 2A). In the consortia without any PAH, the B. licheniformis cell count kept increasing even after 5 days with the highest count reaching up to 3.8 × 107 measured on 5th day until no colonies were observed on the plates. In contrast, the B. licheniformis when co-cultured with G. pectorale in the presence of PHE (GB-PHE), exhibited a slower rate of decrease with a final count of 2 × 107 CFU/mL on day 12 from 1.6 × 108 CFU/mL on day 8. In the B-ANT and GB-ANT samples, the situation is different with regards to cell count. There was no increase in cell count observed in either sample after initial inoculation. The final cell counts recorded on day 12 were 1 × 107 CFU/mL (Figure 2B). Importantly, G. pectorale in samples without any PAH (G-only and GB-only) exhibited an increase in dry weight until 22 days. In the consortia the presence of PHE and ANT in the media did not inhibit the growth of G. pectorale. The B. licheniformis strain used in this study was previously established to be able to degrade these PAHs (Nzila et al., 2019).
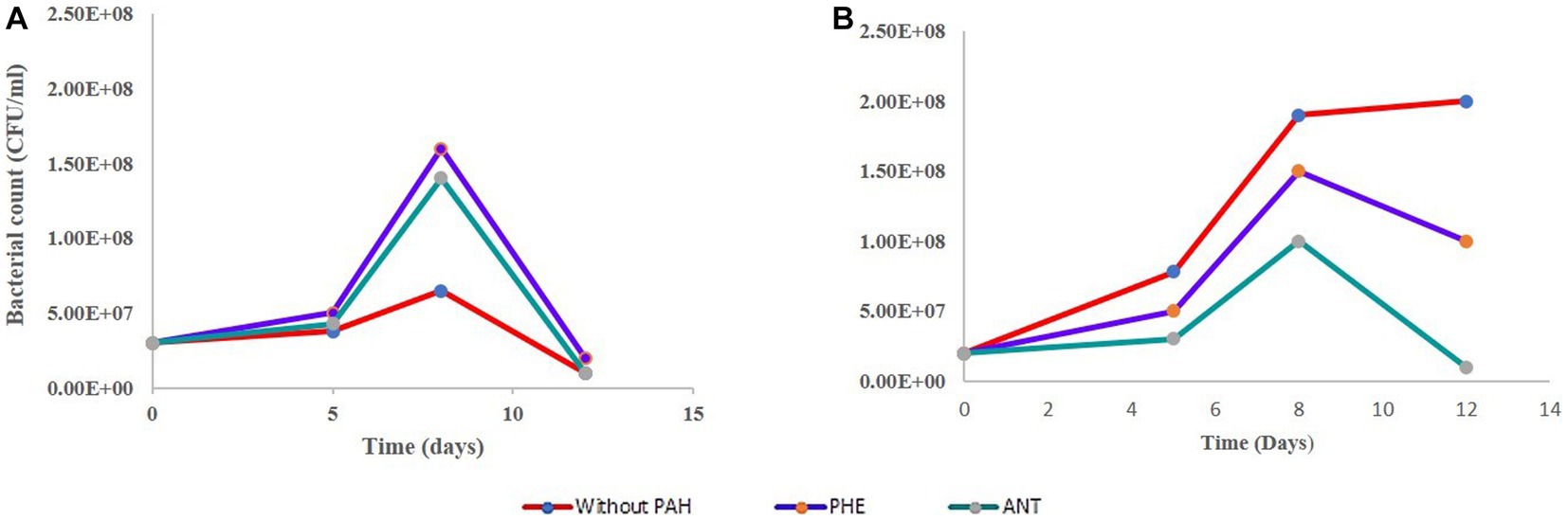
Figure 2. Growth curve of B. licheniformis without any PHE and ANT. (A) Growth curve of B. licheniformis alone. (B) Growth curve for B. licheniformis in consortia.
PAH degradation experiments
To assess the potential of microalgae-bacteria consortia to degrade PAH, experiments were conducted using PAHs with lower molecular weights. Since there are no reports on the ability of G. pectorale to degrade PAHs, it was ideal to begin with PHE and ANT. Samples were extracted after 22 days of incubation and subjected to GC-FID. The retention times obtained from GC-FID analysis for pure samples of PHE and ANT at 5 ppm concentration were 13.69 and 15.4 min, respectively. Peak areas obtained for the experimental samples and controls were converted to PAH percentage removed using the formula mentioned earlier. The highest removal of PHE by G. pectorale and B. licheniformis was 99 and 19%, respectively. The consortia of both strains degraded PHE (96%). Similarly, G. pectorale and B. licheniformis have removed ANT 99 and 45%, respectively. The consortia of both strains degraded ANT (99%) (Figure 3).
So far there are no reports on the ability of G. pectorale to degrade PAHs and the only bioremediation-related data found for Gonium sp. was where it was shown to remove various dyes from solution for application in wastewater treatment. In one study it was shown to remove Remazol Brilliant Blue R with the highest rate of 56% among other synthetic dyes tested (Reactive Black 5, Reactive Orange 14 and Reactive Red 120) (Kılıç et al., 2011). Another study reported Gonium sp. to be able to remove Reactive Blue 220 with a maximum yield of 54.2% at pH 8 (Boduroğlu et al., 2014). PAH degradation data of G. pectorale from this study can be compared to its closest relative Chlamydomonas as both of them belong to the order Chlamydomonadales. Literature exists for Chlamydomonas and other algae’s ability to degrade various PAHs. For instance, in a previous study, Scenedesmus obliquus ES-55 was shown to have the highest PHE degradation of 42% in Bold’s Basal Medium after 56 days incubation (Safonova et al., 2005). In contrast, the Gonium sp. in this study removed 71% PHE within 30 days. In the study involving Scenedesmus obliquus ES-55, the authors also mention dihydroxy-dihydro-phenanthrene as a byproduct of the degradation process. Therefore, it is crucial that in any future studies with G. pectorale, the degradation products are identified, and a degradation pathway established. This is important before any large-scale bioremediation process is designed because the degradation products must also be environmentally safe.
GC–MS analysis of phenanthrene metabolites revealed that G. pectorale produced a metabolite (9,10-Phenanthrenequinone) during PHE degradation (Figure 4). Mass spectrum of 9,10-phenanthrenequinone with a retention time of 20.50 min and contained a molecular ion (M+) at m/z 208 and fragment ions 180, 152, 126. Even though the data from NST98 library matches this metabolite spectrum to 9,10-anthracenedione, this is most likely due the similarity in both PHE and ANT chemical structures and thus undergo same fragmentation. Moreover, in a biodegradation study, 9,10-Phenanthrenequinone was identified in Polyporus sp. S133 and Agmenellum quadruplicatum PR-6 (Narro et al., 1992; Hadibarata and Tachibana, 2010).
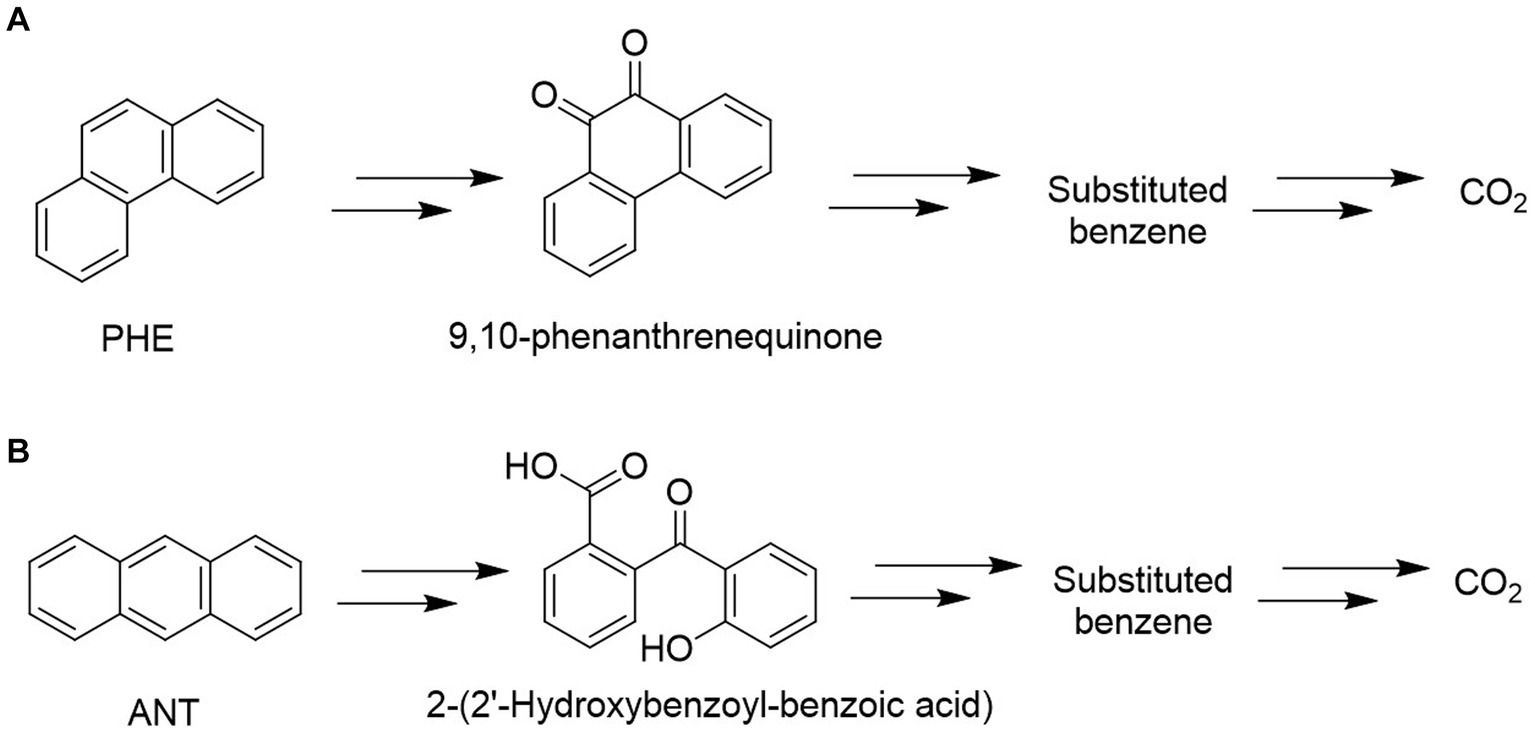
Figure 4. Proposed metabolic pathways for degradation of PHE (A) and ANT (B) using Gonium pectorale.
Mass spectral characteristics of 2-(2′-hdroxybenzoyl-benzoic acid) was obtained in this study with a retention time: 21.011 (min), m/z 224(M+), and base peaks (196,168, and 139). The identity of this metabolite is determined due to similar peaks that were also found for anthracene degradation metabolite produced by Fungi (Jové et al., 2016). The detected metabolites in the degradation of PHE and ANT using G. pectorale indicate an inner ring cleavage in both as shown in Figure 4.
Identification of putative genes in PhycoCosm database
Degradation of PAHs was reported in Chlamydomonas reinhardtii and this activity was associated with an increased expression of genes coding for homogentisate 1,2-dioxygenase (HGD) and carboxymethylenebutenolidase, besides ribulose 1,5-bisphosphate carboxylase/oxygenase (RubisCO) and ubiquinol oxidase (Luo et al., 2020). An approach coupling blast and keyword searches into the PhycoCosm database1 led to the identification of six putative carboxymethylenebutenolidases and one homogentisatee 1,2-dioxygenase were identified (Supplementary material) in G. pectorale.
The promoter analysis of the 6 carboxymethylenebutenolidases (ca. 2000 nucleotides upstream the ATG start codon) using PlantPAN 3.0 (Chow et al., 2019) revealed the presence of AP2 superfamily transcription factors binding sites (Figure 5). In this respect, it should be noted that in the green microalga Auxenochlorella protothecoides, transcription factors belonging to the AP2 superfamily were associated with T stress and lipid biosynthesis (Xing et al., 2021). Interestingly, in Chlorella sorokiniana, pyrene at 230 ppm caused no significant decrease in biomass, but induced lipid biosynthesis by ca. 24% (Jaiswal et al., 2021). Hence, it will be worthwhile investigating in the future whether the addition of PAHs to G. pectorale growth medium is accompanied by an increased lipid biosynthesis and a concomitant induction of genes encoding transcription factors of the AP2 superfamily and carboxymethylenebutenolidases.
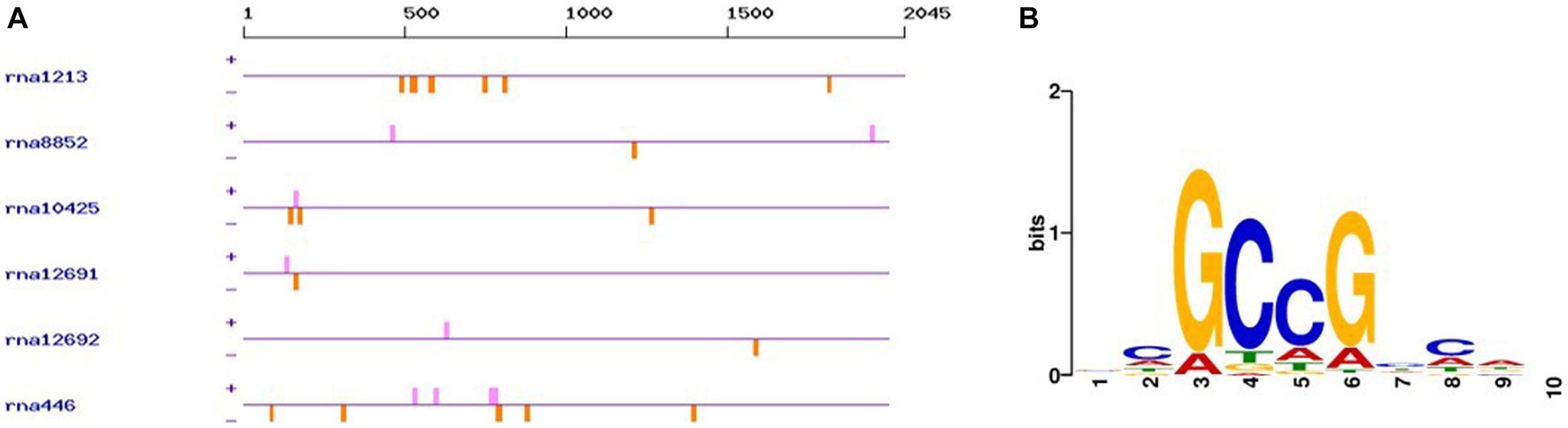
Figure 5. AP2 family transcription factor binding sites in the promoter sequences of the G. pectorale carboxymethylenebutenolidases. (A) Occurrence of the transcription factor binding sites (orange: negative strand, pink: positive strand); (B) Consensus transcription factor binding sequence found.
Conclusion
In this study G. pectorale and B. licheniformis have been screened for their potential against PAHs (PHE and ANT) bioremediation. G. pectorale degraded PHE and ANT (98%) more efficiently as compared to B. licheniformis (PHE 19%, ANT 45%). Our findings show that B. licheniformis did not inhibit the growth of G. pectorale and their combined consortia have shown a slight increase in the degradation of PHE (96%) and ANT (99%). GC–MS analysis has confirmed the metabolites 9,10-phenanthrenequinone and 2-(2′-hydroxybenzoyl)-benzoic acid. Based on our findings, we suggest the bioremediation of PAHs using a consortium approach that seems to be a very attractive alternative to the conventional mechanical and/or other physicochemical methods because it may be the best sustainable and ecofriendly option.
Data availability statement
The original contributions presented in the study are included in the article/Supplementary material, further inquiries can be directed to the corresponding author.
Author contributions
MH, AA, SS, DA, AN, and IA designed the study. MH, AA, SS, and MM performed the experiment. AA, GG, KS, MM, and IA analyzed the data. MH, AA, SS, DA, and IA wrote the manuscript. All authors contributed to the article and approved the submitted version.
Funding
This study was supported by project number INMW2301 by Interdisciplinary Research Center for Membranes and Water Security (IRC-MWS), King Fahd University of Petroleum and Minerals (KFUPM) Dhahran, Saudi Arabia.
Acknowledgments
We acknowledge the King Fahd University of Petroleum and Minerals (KFUPM), Dhahran, 31261, Saudi Arabia for providing the facilities for this work. Authors would like to acknowledge the funding provided by Deanship of Research Oversight and Coordination (DROC), King Fahd University of Petroleum and Minerals (KFUPM) Dhahran, Saudi Arabia for article processing charges (APC).
Conflict of interest
The authors declare that the research was conducted in the absence of any commercial or financial relationships that could be construed as a potential conflict of interest.
Publisher’s note
All claims expressed in this article are solely those of the authors and do not necessarily represent those of their affiliated organizations, or those of the publisher, the editors and the reviewers. Any product that may be evaluated in this article, or claim that may be made by its manufacturer, is not guaranteed or endorsed by the publisher.
Supplementary material
The Supplementary material for this article can be found online at: https://www.frontiersin.org/articles/10.3389/fmicb.2023.1227210/full#supplementary-material
Footnotes
References
Abdel-Shafy, H. I., and Mansour, M. S. (2016). A review on polycyclic aromatic hydrocarbons: source, environmental impact, effect on human health and remediation. Egypt. J. Pet. 25, 107–123. doi: 10.1016/j.ejpe.2015.03.011
Adeniji, A., Okoh, O., and Okoh, A. (2019). Levels of polycyclic aromatic hydrocarbons in the water and sediment of Buffalo River estuary, South Africa and their health risk assessment. Arch. Environ. Con. Tox. 76, 657–669. doi: 10.1007/s00244-019-00617-w
Ahmad, I. (2021). Microalgae–Bacteria consortia: a review on the degradation of polycyclic aromatic hydrocarbons (PAHs). Arab. J. Sci. Eng. 47, 19–43. doi: 10.1007/s13369-021-06236-9
Alvarez-Ospina, H., Giordano, S., Ladino, L. A., Raga, G. B., Muñoz-Salazar, J. I., Leyte-Lugo, M., et al. (2021). Particle-bound polycyclic aromatic hydrocarbons (pPAHs) in Merida, Mexico. Aerosol Air Qual. Res. 21:200245. doi: 10.4209/aaqr.200245
Boduroğlu, G., Kılıç, N. K., and Dönmez, G. (2014). Bioremoval of reactive blue 220 by Gonium sp. biomass. Environ. Technol. 35, 2410–2415. doi: 10.1080/09593330.2014.908240
Chen, B., Huang, J., Yuan, K., Lin, L., Wang, X., Yang, L., et al. (2016). Direct evidences on bacterial growth pattern regulating pyrene degradation pathway and genotypic dioxygenase expression. Mar. Pollut. Bull. 105, 73–80. doi: 10.1016/j.marpolbul.2016.02.054
Chow, C. N., Lee, T. Y., Hung, Y. C., Li, G. Z., Tseng, K. C., Liu, Y. H., et al. (2019). PlantPAN3.0: a new and updated resource for reconstructing transcriptional regulatory networks from ChIP-seq experiments in plants. Nucleic Acids Res. 47, D1155–D1163. doi: 10.1093/nar/gky1081
de Llasera, M. P. G., de Olmos-Espejel, J. J., Díaz-Flores, G., and Montaño-Montiel, A. (2016). Biodegradation of benzo(a)pyrene by two freshwater microalgae Selenastrum capricornutum and Scenedesmus acutus: a comparative study useful for bioremediation. Environ. Sci. Pollut. Res. 23, 3365–3375. doi: 10.1007/s11356-015-5576-2
de Llasera, M. P. G., Santiago, M. L., Flores, E. J. L., Toris, D. N. B., and Herrera, M. R. C. (2018). Mini-bioreactors with immobilized microalgae for the removal of benzo (a) anthracene and benzo (a) pyrene from water. Ecol. Eng. 121, 89–98. doi: 10.1016/j.ecoleng.2017.06.059
Dhar, K., Subashchandrabose, S. R., Venkateswarlu, K., Krishnan, K., and Megharaj, M. (2020). Anaerobic microbial degradation of polycyclic aromatic hydrocarbons: a comprehensive review. Rev. Environ. Contam. Toxiol. 251, 25–108. doi: 10.1007/398_2019_29
Dong, Y., Yan, Z., Wu, H., Zhang, H., and Yang, M. (2012). Polycyclic aromatic hydrocarbons in sediments from typical algae, macrophyte Lake Bay and adjoining river of Taihu Lake, China: distribution, sources, and risk assessment. Water 13:470. doi: 10.3390/w13040470
García, M. M., and de Llasera, M. P. G. (2021). A review on the enzymes and metabolites identified by mass spectrometry from bacteria and microalgae involved in the degradation of high molecular weight PAHs. Sci. Total Environ. 797:149035. doi: 10.1016/j.scitotenv.2021.149035
Ghosal, D., Ghosh, S., Dutta, T. K., and Ahn, Y. (2016). Current state of knowledge in microbial degradation of polycyclic aromatic hydrocarbons (PAHs): a review. Front. Microbiol. 7:1369. doi: 10.3389/fmicb.2016.01369
Guerriero, G., Sergeant, K., Legay, S., Hausman, J.-F., Cauchie, H.-M., Ahmad, I., et al. (2018). Novel insights from comparative in silico analysis of green microalgal cellulases. Int. J. Mol. Sci. 19:1782. doi: 10.3390/ijms19061782
Gupte, A., Tripathi, A., Patel, H., Rudakiya, D., and Gupte, S. (2016). Bioremediation of polycyclic aromatic hydrocarbon (PAHs): a perspective. Open Biotechnol. J. 10, 363–378. doi: 10.2174/1874070701610010363
Gutierrez, T., Rhodes, G., Mishamandani, S., Berry, D., Whitman, W. B., Nichols, P. D., et al. (2014). Polycyclic aromatic hydrocarbon degradation of phytoplankton-associated Arenibacter spp. and description of Arenibacter algicola sp. nov., an aromatic hydrocarbon-degrading bacterium. Appl. Environ. Microbiol. 80, 618–628. doi: 10.1128/AEM.03104-13
Hadibarata, T., and Tachibana, S. (2010). Characterization of phenanthrene degradation by strain polyporus sp. S133. J. Environ. Sci. 22, 142–149. doi: 10.1016/s1001-0742(09)60085-1
Hamouda, R. A. E. F., Sorour, N. M., and Yeheia, D. S. (2016). Biodegradation of crude oil by Anabaena oryzae, Chlorella kessleri and its consortium under mixotrophic conditions. Internat. Biodeterior. Biodegradation. 112, 128–134. doi: 10.1016/j.ibiod.2016.05.001
Jaiswal, K. K., Kumar, V., Vlaskin, M. S., and Nanda, M. (2021). Impact of pyrene (polycyclic aromatic hydrocarbons) pollutant on metabolites and lipid induction in microalgae Chlorella sorokiniana (UUIND6) to produce renewable biodiesel. Chemosphere 285:131482. doi: 10.1016/j.chemosphere.2021.131482
Jové, P., Olivella, M. À., Camarero, S., Caixach, J., Planas, C., Cano, L., et al. (2016). Fungal biodegradation of anthracene-polluted cork: a comparative study. J. Environ. Sci. Health A 51, 70–77. doi: 10.1080/10934529.2015.1079114
Kahla, O., Garali, M. B., Karray, S., Abdallah, F. B., Kallel, M., Mhiri, N., et al. (2021). Efficiency of benthic diatom-associated bacteria in the removal of benzo(a) pyrene and fluoranthene. Sci. Total Environ. 751:141399. doi: 10.1016/j.scitotenv.2020.141399
Kılıç, N. K., Karatay, S. E., Duygu, E., and Dönmez, G. (2011). Potential of Gonium spp. in synthetic reactive dye removal, possible role of laccases and stimulation by Triacontanol hormone. Water Air Soil Pollut. 222, 297–303. doi: 10.1007/s11270-011-0824-7
Kleindienst, S., Paul, J. H., and Joye, S. B. (2015). Impacts on the composition and activity of microbial communities. Nat. Rev. Microbiol. 13, 388–396. doi: 10.1038/nrmicro3452
Lawal, A. T. (2017). Polycyclic aromatic hydrocarbons. A review. Cogent. Environ. Sci. 3:1339841. doi: 10.1080/23311843.2017.1339841
Li, X., Cai, F., Luan, T., Lin, L., and Chen, B. (2019). Pyrene metabolites by bacterium enhancing cell division of green alga Selenastrum capricornutum. Sci. Total Environ. 689, 287–294. doi: 10.1016/j.scitotenv.2019.06.162
Luo, J., Deng, J., Cui, L., Chang, P., Dai, X., Yang, C., et al. (2020). The potential assessment of green alga Chlamydomonas reinhardtii CC-503 in the biodegradation of benz(a)anthracene and the related mechanism analysis. Chemosphere 249:126097. doi: 10.1016/j.chemosphere.2020.126097
Mojiri, A., Zhou, J. L., Ohashi, A., Ozaki, N., and Kindaichi, T. (2019). Comprehensive review of polycyclic aromatic hydrocarbons in water sources, their effects and treatments. Sci. Total Environ. 696:133971. doi: 10.1016/j.scitotenv.133971
Narro, M. L., Cerniglia, C. E., Van Baalen, C., and Gibson, D. T. (1992). Metabolism of phenanthrene by the marine cyanobacterium Agmenellum quadruplicatum PR-6. Appl. Environ. Microbiol. 58, 1351–1359. doi: 10.1128/aem.58.4.1351-1359.1992
Ngwoke, M., Igwe, O., and Ozioko, O. (2021). Acute toxicity assessment of produced water effluent stream on selected local organisms in Delta state, Nigeria. Environ. Monit. Assess. 193:254. doi: 10.1007/s10661-021-09032-y
Nzila, A., Musa, M. M., Sankara, S., Al-Momani, M., Xiang, L., and Li, Q. X. (2021). Degradation of benzo[a]pyrene by halophilic bacterial strain Staphylococcus haemoliticus strain 10SBZ1A. PLoS One 16:e0247723. doi: 10.1371/journal.pone.0247723
Nzila, A., Sankara, S., Al-Momani, M., and Musa, M. M. (2018). Isolation and characterisation of bacteria degrading polycyclic aromatic hydrocarbons: Phenanthrene and anthracene. Arch. Environ. Prot. 44, 43–54. doi: 10.24425/119693
Nzila, A., Thukair, A., Sankara, S., Chanbasha, B., and Musa, M. M. (2019). Biodegradation of highly complex polyaromatic-hydrocarbon pyrene by Bacillus lenichiformis and Ralstonia sp. from the coastal region of Saudi Arabia. New Biotechnol. 33, S140–S141. doi: 10.1016/j.nbt.2016.06.1208
Olajire, A. A. (2020). Recent advances on the treatment technology of oil and gas produced water for sustainable energy industry mechanistic aspects and process chemistry perspectives. Chem. Eng. J. Adv. 4:100049. doi: 10.1016/j.ceja.100049
Omojevwe, E. G., and Ezekiel, F. O. (2016). Microalgal-bacterial consortium in Polyaromatic hydrocarbon degradation of petroleum–based effluent. J. Bioremed. Biodegr. 7:359. doi: 10.4172/2155-6199.1000359
Patel, J. G., Nirmal Kumar, J. I., Kumar, R. N., and Khan, S. R. (2015). Enhancement of pyrene degradation efficacy of Synechocystis sp., by construction of an artificial microalgal-bacterial consortium. Cogent. Chem. 1:1. doi: 10.1080/23312009.2015.1064193
Patel, A. B., Shaikh, S., Jain, K. R., Desai, C., and Madamwar, D. (2020). Polycyclic aromatic hydrocarbons: sources, toxicity, and remediation approaches. Front. Microbiol. 11:562813. doi: 10.3389/fmicb.2020.562813
Perera, I., Subashchandrabose, S. R., Venkateswarlu, K., Naidu, R., and Megharaj, M. (2018). Consortia of cyanobacteria/microalgae and bacteria in desert soils: an underexplored microbiota. Appl. Microbiol. Biotechnol. 102, 7351–7363. doi: 10.1007/s00253-018-9192-1
Qutob, M., Rafatullah, M., Muhammad, S. A., Alosaimi, A. M., Alorfi, H. S., and Hussein, M. A. (2022). A review of pyrene bioremediation using Mycobacterium strains in a different matrix. Fermentation 8:260. doi: 10.3390/fermentation8060260
Ratha, S. K., Rao, P. H., Govindaswamy, K., Jaswin, R. S., Lakshmidevi, R., Bhaskae, S., et al. (2016). A rapid and reliable method for estimating microalgal biomass using a moisture analyser. J. Appl. Phycol. 28, 1725–1734. doi: 10.1007/s10811-015-0731-1
Safonova, E., Kvitko, K., Kuschk, P., Möder, M., and Reisser, W. (2005). Biodegradation of Phenanthrene by the green Alga Scenedesmus obliquus ES-55. Eng. Life Sci. 5, 234–239. doi: 10.1002/ELSC.200520077
Subashchandrabose, S. R., Venkateswarlu, K., Venkidusamy, K., Palanisami, T., Naidu, R., and Megharaj, M. (2019). Bioremediation of soil long-term contaminated with PAHs by algal-bacterial synergy of Chlorella sp. MM3 and Rhodococcus wratislaviensis strain 9 in slurry phase. Sci. Total Environ. 659, 724–731. doi: 10.1016/j.scitotenv.2018.12.453
Suman, S., Sinha, A., and Tarafdar, A. (2016). Polycyclic aromatic hydrocarbons (PAHs) concentration levels, pattern, source identification and soil toxicity assessment in urban traffic soil of Dhanbad. India. Sci. Total Environ. 545–546, 353–360. doi: 10.1016/j.scitotenv.2015.12.061
Trannum, H. C., Setvik, Å., Norling, K., and Nilsson, H. C. (2011). Rapid macrofaunal colonization of water-based drill cuttings on different sediments. Mar. Pollut. Bull. 62, 2145–2156. doi: 10.1016/j.marpolbul.07.007
Uzoekwe, S. A., and Oghosanine, F. A. (2011). The effect of refinery and petrochemical effluent on water quality of Ubeji Creek Warri, southern Nigeria. Ethiop. J. Environ. Stud. Manag. 4, 107–116. doi: 10.4314/ejesm.v4i2.12
Varjani, S. J., and Upasani, V. N. (2017). A new look on factors affecting microbial degradation of petroleum hydrocarbon pollutants. Int. Biodeterior. Biodegrad. 120, 71–83. doi: 10.1016/J.IBIOD.2017.02.006
Wang, H., Hill, R. T., Zheng, T., Hu, X., and Wang, B. (2016). Effects of bacterial communities on biofuel-producing microalgae: stimulation, inhibition and harvesting. Crit. Rev. Biotechnol. 36, 341–352. doi: 10.3109/07388551.2014.961402
Xing, G., Li, J., Li, W., Lam, S. M., Yuan, H., Shui, G., et al. (2021). AP2/ERF and R2R3-MYB family transcription factors: potential associations between temperature stress and lipid metabolism in Auxenochlorella protothecoides. Biotechnol. Biofuels 14:22. doi: 10.1186/s13068-021-01881-6
Xu, X., Liu, W., Tian, S., Wang, W., Qi, Q., Jiang, P., et al. (2018). Petroleum hydrocarbon-degrading bacteria for the remediation of oil pollution under aerobic conditions: a perspective analysis. Front. Microbiol. 9:2885. doi: 10.3389/fmicb.2018.02885
Keywords: microalgal-bacterial consortium, polycyclic aromatic hydrocarbons, phenanthrene and anthracene, biodegradation genes, petrogenic pollutants, water contaminants, Gonium pectorale
Citation: Hoque MZ, Alqahtani A, Sankaran S, Anand D, Musa MM, Nzila A, Guerriero G, Siddiqui KS and Ahmad I (2023) Enhanced biodegradation of phenanthrene and anthracene using a microalgal-bacterial consortium. Front. Microbiol. 14:1227210. doi: 10.3389/fmicb.2023.1227210
Edited by:
Kian Mau Goh, University of Technology Malaysia, MalaysiaReviewed by:
Mohd Rafatullah, University of Science Malaysia (USM), MalaysiaGaurav Saxena, Shoolini University, India
Copyright © 2023 Hoque, Alqahtani, Sankaran, Anand, Musa, Nzila, Guerriero, Siddiqui and Ahmad. This is an open-access article distributed under the terms of the Creative Commons Attribution License (CC BY). The use, distribution or reproduction in other forums is permitted, provided the original author(s) and the copyright owner(s) are credited and that the original publication in this journal is cited, in accordance with accepted academic practice. No use, distribution or reproduction is permitted which does not comply with these terms.
*Correspondence: Irshad Ahmad, aXJzaGFkQGtmdXBtLmVkdS5zYQ==