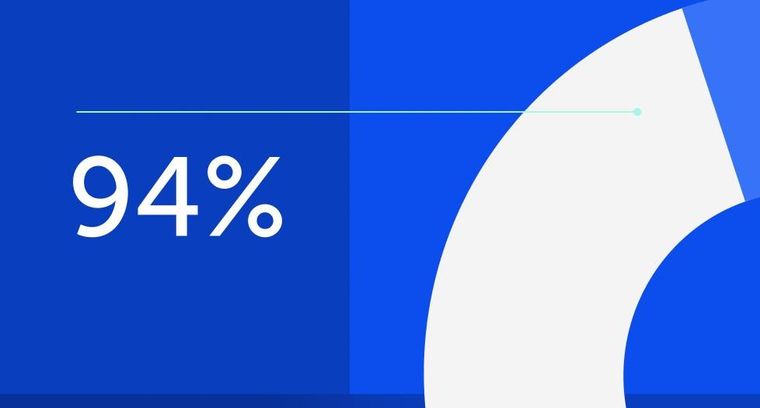
94% of researchers rate our articles as excellent or good
Learn more about the work of our research integrity team to safeguard the quality of each article we publish.
Find out more
ORIGINAL RESEARCH article
Front. Microbiol., 26 June 2023
Sec. Microbiotechnology
Volume 14 - 2023 | https://doi.org/10.3389/fmicb.2023.1223123
Propenylbenzenes, including isosafrole, anethole, isoeugenol, and their derivatives, are natural compounds found in essential oils from various plants. Compounds of this group are important and valuable, and are used in the flavour and fragrance industries as well as the pharmaceutical and cosmetic industries. The aim of this study was to develop an efficient process for synthesising oxygenated derivatives of these compounds and evaluate their potential biological activities. In this paper, we propose a two-step chemo-enzymatic method. The first step involves the synthesis of corresponding diols 1b–5b from propenylbenzenes 1a–5avia lipase catalysed epoxidation followed by epoxide hydrolysis. The second step involves the microbial oxidation of a diasteroisomeric mixture of diols 1b–5b to yield the corresponding hydroxy ketones 1c–4c, which in this study was performed on a preparative scale using Dietzia sp. DSM44016, Rhodococcus erythropolis DSM44534, R. erythropolis PCM2150, and Rhodococcus ruber PCM2166. Application of scaled-up processes allowed to obtain hydroxy ketones 1-4c with the following yield range 36–62.5%. The propenylbenzene derivatives thus obtained and the starting compounds were tested for various biological activities, including antimicrobial, antioxidant, haemolytic, and anticancer activities, and their impact on membrane fluidity. Fungistatic activity assay against selected strains of Candida albicans results in MIC50 value varied from 37 to 124 μg/mL for compounds 1a, 3a–c, 4a,b, and 5a,b. The highest antiradical activity was shown by propenylbenzenes 1-5a with a double bond in their structure with EC50 value ranged from 19 to 31 μg/mL. Haemolytic activity assay showed no cytotoxicity of the tested compounds on human RBCs whereas, compounds 2b–4b and 2c–4c affected the fluidity of the RBCs membrane. The tested compounds depending on their concentration showed different antiproliferative activity against HepG2, Caco-2, and MG63. The results indicate the potential utility of these compounds as fungistatics, antioxidants, and proliferation inhibitors of selected cell lines.
Propenylbenzenes, such as isosafrole, anethol, isoeugenol, and their derivatives, are widely found in essential oils from plants such as the aniseed tree, liquorice, and the cananga tree (Newberne et al., 1999; Thi Luu et al., 2009; Fahlbusch et al., 2003; Lummiss et al., 2012). Compounds of this group are economically important and widely used in the flavour and fragrance industries as well as the pharmaceutical and cosmetic industries, and as intermediates in the synthesis of more complex products (Atsumi et al., 2005; Cabral et al., 2014; Aprotosoaie et al., 2016). In recent years, various commercial processes have been developed to obtain these compounds through isomerization of safrole, estragole, and eugenol (Petersen et al., 2010; Rajagopalan et al., 2013; Hassam et al., 2015). Propenylbenzenes have broad biological activities, such as antioxidant, antimicrobial, anti-inflammatory, and antiproliferative actions. For example, essential oils containing isosafrole have shown antioxidant activity and antimicrobial effects on clinical isolates of Helicobacter pylori, Staphylococcus aureus, and Escherichia coli (Bruna et al., 2022). Additionally, safrole oil and its nanoemulgel had an antiproliferative effect on hepatocellular carcinoma cells Hep3B (Eid and Hawash, 2021). Anethole exerts an inhibitory effect on periodontitis by suppressing pro-inflammatory molecules (Moradi et al., 2014; Lal et al., 2022). Anethole-rich oil from Clausena heptaphylla leaf has anti-diabetic, tyrosinase-inhibiting, and anti-cholinesterase activities (Lal et al., 2022). Emulsion-encapsulated isoeugenol has antimicrobial effects against food pathogens and spoilage bacteria such as Listeria monocytogenes, Staphylococcus aureus, Pseudomonas fluorescens, and Leuconostoc mesenteroides as well as antioxidant and anti-inflammatory properties (Siva et al., 2019).
Given the different biological activities of propenylbenzenes and their widespread use in industry, it is worth looking for derivatives of these compounds. As the interest in compounds obtained via environmentally friendly methods is currently growing, the necessity for the development of new methods for producing propenylbenzene derivatives such as diols and hydroxy ketones has increased in recent years. Various methods for obtaining propenylbenzenes and their derivatives have been described in the literature (D’Accolti et al., 1993; Peng et al., 2005; Mecozzi et al., 2017; Nair, 2020; Tentori et al., 2021). In one chemo-enzymatic method, isosafrole is epoxidated and then hydrolysed to yield a stereoisomeric mixture of corresponding diols (Tentori et al., 2021). Peng et al. (2005) presented a method involving selective oxidation of sec-1,2-diols, using 2,3-dichloro-5,6-dicyano-1,4-benzoquinone and ultrasound waves to produce α-hydroxy ketones. The oxidation of vicinal diols to α-hydroxy ketones can also be accomplished using hydrogen peroxide (H2O2) and a manganese catalyst (Mecozzi et al., 2017), dimethyldioxirane or its trifluoromethyl analogue (D’Accolti et al., 1993), or 2-iodoxybenzoic acid (IBX) (Nair, 2020).
Due to the increasing attention paid to green chemistry and the advantages of biotechnological methods, it is important to replace chemical methods with those using biocatalysts in the form of whole cells or isolated enzymes. For instance, Kihumbu et al. (2002) described a method that allowed synthesising all four stereoisomers of 1-phenylpropane-1,2-diol as well as the corresponding hydroxy ketones with high yields starting from benzaldehyde and acetaldehyde using different lyase–alcohol dehydrogenase combinations. Oeggl et al. (2018) reported a method to synthesise hydroxy ketone isomers using lyase or decarboxylase and then 4-methoxyphenyl-1,2-propanediol using alcohol dehydrogenase, with good yields. Finally, the use of benzoylformate decarboxylase or benzaldehyde lyase from P. putida and P. fluorescens allows the synthesis of hydroxy ketone derivatives of propenylbenzenes (Kurlemann et al., 2009; Kulig et al., 2013; Pérez-Sánchez et al., 2013). However, methods that use whole cells of microorganisms for cost reduction are lacking.
In the presented work, we aimed to obtain oxygenated propenylbenzene derivatives, including diols 1b–5b and hydroxy ketones 1c–4c, starting from propenylbenzenes 1a–5a, using chemo-enzymatic synthesis followed by whole-cell transformation. In addition, biological activity tests were performed on the obtained compounds. This approach allowed us to assess the influence of various chemical groups attached to the propenyl chain and aromatic ring on the investigated biological activities. Based on our previous experience, we decided to test the following biological activities: antimicrobial, antioxidant, haemolytic, and anticancer actions, and the impact on membrane fluidity.
Bacillus subtilis PCM2238, B. subtilis PCM2850, Dietzia maris PCM2292, Gordonia bronchialis PCM2167, Gordonia rubripertincta PCM2144, Micrococcus luteus PCM525, Pseudomonas aeruginosa PCM2720, P. aeruginosa PCM3035, Rhodococcus coprophilus PCM2174, Rhodococcus erythropolis PCM2150, Rhodococcus rhodnii PCM2157, Rhodococcus rhodochrous PCM909, Rhodococcus ruber PCM2166, R. ruber PCM2171, R. ruber PCM2216, Serratia liquefaciens PCM2830, Serratia marcescens PCM549, Serratia plumuthica PCM550, Serratia sp. PCM1324, Streptomyces griseus subsp. griseus PCM2331 were obtained from the Polish Academy of Sciences (Wrocław, Poland). Dietzia sp. DSM44016 and Rhodococcus erythropolis DSM44534 were purchased from the German Collection of Microorganisms and Cell Cultures (Braunschweig, Germany). The biocatalysts were maintained at 4°C on PCM medium agar slants. For use in experiments, they were transferred into conical flasks containing PCM medium composed of sodium chlorine (6 g) (Chempur, Piekary Śląskie, Poland), glucose (20 g) (Chempur), casein (2 g) (Biocorp, Warszawa, Poland), bacteriological peptone (10 g) (Biocorp), and yeast extract (2 g) (Chempur) dissolved in distilled water (1 L) at 25°C, pH 5.5.
Fungistatic activity was determined using Candida albicans ATCC 90028 from the American Type Culture Collection (ATCC, Manassas, VA, United States), and clinical isolates, C. albicans 636/20, C. albicans 595/20, and C. albicans 38 obtained from Wrocław Medical University, Wrocław, Poland.
Propenylbenzenes: isosafrole (1a), prop-1-en-1-yl benzene (2a), anethole (3a), 1,2-dimethoxy-4-prop-1-en-1-yl benzene (4a), and isoeugenol (5a) were purchased from Sigma-Aldrich Chemical Co. (St. Louis, MO, United States). All chemicals and solvents were purchased from Zentek s.r.l. (Milan, Italy) and used without further purification.
Vicinal diols 1-(1,3-benzodioxol-5-yl)propane-1,2-diol (1b), 1-phenylpropane-1,2-diol (2b), 1-(4-methoxyphenyl)propane-1,2-diol (3b), 1-(3,4-dimethoxyphenyl)propane-1,2-diol (4b), and 1-(4-hydroxy-3-methoxyphenyl)propane-1,2-diol (5b) were obtained as mixtures of two racemic diastereoisomers by chemo-enzymatic synthesis according to the procedure herein exemplified (Tentori et al., 2021).
Propenylbenzenes 1a–5a (2.96 mmol) were dissolved in EtOAc (15 mL). A 35% w/w aqueous solution of H2O2 (382 μL, 4.44 mmol) and Novozym 435 (10 mg) was added to the solution, which was incubated in a thermoshaker at 30°C for 18 h. Then, the enzyme was filtered out and the reaction was quenched first with Na2SO3, then with a saturated NaHCO3 solution. Extraction with EtOAc afforded an organic phase that was dried (Na2SO4) and concentrated under reduced pressure. The resulting residue was dissolved in MeOH (15 mL), and an excess of KOH (250 mg, 1.5 equiv.) was added to the solution. The reaction was left under magnetic stirring at room temperature for 24 h. The solution volume was reduced to one-third under vacuum, poured into diluted H2SO4 solution, and extracted with EtOAc. The organic phase was dried (Na2SO4) and concentrated under vacuum. Trituration of the solid residue with hexane/EtOAc (8,2) afforded mixtures of (R*,S*)- and (R*,R*)-diols 1b–5b. The structure of the final compounds and the diastereoisomeric ratio, in which they were obtained, were established by GC and NMR analyses.
In order to obtain reference compounds for the identification of products after biotransformation, the following oxidation reaction was implemented. A mixture of corresponding diol 1-4b (4.9 mmol), TEMPO (8.0 mg, 0.049 mmol) and NaCl (3 mg, 0.049 mmol) in toluene (45 mL) was stirred at 100°C for 4–5 h. The reaction mixture was poured into water and extracted with ethyl acetate. The organic phase was dried and concentrated under reduced pressure to give the corresponding diketone and hydroxy ketone derivatives (approximately 1.8 g from a mixture of each diol). The extract obtained in this way was then purified and allowed to obtain GC/MS chromatograms, 1H and 13C NMR spectra of corresponding diketones and hydroxy ketones.
Forty millilitres of PCM medium was added into 100-mL tapered flasks and sterilized at 121°C under a pressure of 1 atm. The medium was inoculated with 0.5 mL of pre-cultured bacteria at OD600 = 0.3–0.5. The bacterial cultures were incubated at 22°C under shaking at 150 rpm for 3 days. Then, 0.001 g of diols 1b–5b dissolved in 0.5 mL of dimethyl sulfoxide (DMSO) was added into the flasks. For simple extraction, ethyl acetate (3 mL) was added to the samples (5 mL) in Falcon tubes and shaken at 200 rpm for 5 min. The organic phase was transferred to a vial and dehydrated with anhydrous MgSO4. Then, it was filtered through a filter paper into a GC vial. Biotransformation was controlled after 3, 7, and 11 days by GC.
Five hundred millilitres of PCM medium was added into a 2,000-mL Erlenmeyer flask and sterilized at 121°C for 15 min. The medium was inoculated with 5 mL of pre-prepared bacterial cultures at OD600 = 0.3–0.5. The cultures were incubated at 22°C under shaking at 150 rpm for 3 days. Then, 0.2 g of diols 1b–5b dissolved in 5 mL of DMSO was added into the cultures. The samples were extracted after 3, 7, 11 days and evaluated by GC to estimate the progress of the biotransformation.
All compounds were tested for biological activity against C. albicans ATTC 90028, C. albicans 636/20, C. albicans 595/20, and C. albicans 38 using the broth microdilution method. YPD medium (20 g glucose, 20 g Bacto Peptone, 10 g yeast extract, and 1 L of distilled water, pH 6.5) was used for the tests. The compound solutions were prepared in DMSO and diluted in YPD to obtain final concentrations in the range of 10–250 μg/mL. One hundred microlitres of each solution was pipetted into the wells of a 96-well microtiter plate. The inoculum was standardized to 0.5 McFarland standard and then diluted to obtain a final suspension with a cell density of 0.5–2.5 × 103 CFU/mL. The inoculum size was 100 μL. The positive control comprised DMSO added in the same concentration as the tested compounds in the inoculum, whereas the negative control consisted of DMSO diluted in the broth without the addition of inoculum. The microtiter plates were incubated in a Biosan PST-60 HL Plate Shaker-Thermostat (Riga, Latvia) at 35°C under shaking at 1,000 rpm for 24 h. The fungistatic activity of the compounds was assessed by measuring the absorbance at a wavelength of 595 nm (Epoch; BioTek, Winooski, VT, United States) to determine the MIC50 value (i.e., the concentration of a compound required to inhibit the growth of 50% of the microorganisms).
The antiradical activity of all compounds was tested using the 2,2-diphenyl-1-picrylhydrazyl (DPPH) test according to a previously described method (Herald et al., 2012; Dudek et al., 2022). One litre of a fresh solution of 190 μM DPPH in pure methanol was prepared in a dark glass bottle. The solution was kept protected from light and used within a week. The tested compounds and ascorbic acid as a positive control were prepared at concentrations in the range of 5–100 μg/mL. Two hundred microlitres of the test compound dilutions was added to each well of a 96-well microtiter plate. Then, 800 μL of a 190 μM solution of DPPH in methanol was added. DPPH was also added to the negative control, in which 200 μL of methanol was added instead of the test compounds. All samples were tested at least in triplicate. The microtiter plates were incubated in the Biosan PST-60 HL thermoshaker at 25°C under shaking at 1,000 rpm for 1 h. The antiradical activity of the compounds was assessed by measuring the absorbance at 517 nm (Epoch) to determine the EC50 value (i.e., the concentration of a compound that gives 50% maximal response). EC50 values were calculated using Quest Graph™ EC50 Calculator (AAT Bioquest, Inc.).
Haemolytic activity was tested using the method described by Włoch et al. (2020) with minor modifications. This test is based on comparison of the cytotoxicity of all tested compounds in human red blood cells (RBCs) with that in a control group. RBCs for this method were obtained from a blood center from examined and healthy donors. The compounds were tested at 10, 20, 40, 60, 80, and 100 μM concentrations. The control sample contained only ethanol in the same volume as the test samples. The final haematocrit in the test samples was 1.2%. The samples were incubated at 37°C for 1 h. Then, the absorbance at 540 nm was measured using a UV–Vis spectrophotometer (Specord 40, Analytik Jena, Jena, Germany). The haemolytic activity of the compounds was determined as the ratio of the absorbance of haemoglobin in the test samples to that in completely haemolyzed cells multiplied by 100%.
Using the DPH probe, the anisotropy values of cell membranes of RBCs modified with the test compounds were measured. RBCs for this analysis were prepared according to the method described in subsection 2.5.3. Then, the DPH probe was added to RBCs with a haematocrit of 0.2%. The final concentration of the probe in the sample was 1.3 μM. The mixture was incubated protected from light at 37°C for 30 min. Then, the compounds dissolved in ethanol were added. The compounds were tested at 20, 60, and 100 μM concentrations. Samples with compounds and control samples with alcohol of the appropriate concentration were incubated at 37°C for 1 h. Measurements in triplicate were made in quartz cuvettes using a CARY Eclipse fluorimeter (Varian, San Diego, CA, United States) at 37°C. The excitation wavelength for the DPH probe is λexc = 360 nm and the emission wavelength is λem = 426 nm. Based on the changes in DPH on the intensity under polarized light, the anisotropy value was determined according to the formula used in our previous publication (Pruchnik et al., 2018).
The human HepG2 and Caco-2 cell lines were obtained from the American Type Culture Collection (HB-8065™ and HTB-37™) and the MG-63 cell line was obtained from the European Collection of Authenticated Cell Cultures (Merck, Poznań, Poland). HepG2 cells were cultured in Dulbecco’s modified Eagle’s Medium - low glucose (Merck) supplemented with 10% foetal bovine serum (FBS) (Merck). Caco-2 cells were cultured in Dulbecco’s modified Eagle’s medium - high glucose (Merck) supplemented with 10% FBS, 2% HEPES buffer (Thermo Fisher Scientific, Warszawa, Poland), 1% Penicillin–Streptomycin-Amphotericin B Solution (100×, Merck), 1% MEM solution (100×, Thermo Fisher Scientific), and 1% Gentamycin Solution (10 mg/mL, Merck). MG-63 cells were cultured in Minimum Essential Medium (Merck) supplemented with 10% FBS. All cells were cultured in an atmosphere of 5% CO2 at 37°C.
HepG2, Caco-2, and MG63 cells were seeded in 96-well plates at 20,000 cells per well, and MG-63 cells at 10,000 cells per well, as described previously (Marycz et al., 2012; Kornicka et al., 2017). The tested compounds were dissolved in ethanol at 10 mg/mL. The concentrations were then adjusted to 1, 50, and 200 μg/mL in culture medium. Four controls were prepared: no ethanol added, and 0.01, 0.5, and 2% EtOH. The cells were treated with the compounds for 24 h. Then, cell viability was evaluated using the resazurin-based assay kit (TOX8), as described previously (Grzesiak et al., 2011; Marycz et al., 2018). The culture medium was replaced with medium containing 10% resazurin dye. The cells were incubated at 37°C in an atmosphere of 5% CO2 for 2 h. Absorbance levels were measured spectrophotometrically (Epoch) at a wavelengths of 600 nm for resazurin and 690 nm as a reference.
Thin-layer chromatography was conducted using aluminium foil plates coated with silica gel. Compounds were detected by spraying the plates with 1% Ce(SO4)2 and 2% H3[P(Mo3O10)4] in 10% H2SO4. Gas chromatography (GC; flame ionisation detection, carrier gas H2) was carried out on a 7,890 N GC system (Agilent Technologies, Santa Clara, CA, United States) equipped with an HP-5 column (30 m × 0.32 mm × 0.25 μm, Agilent Technologies) according to the following temperature program: 70°C, 300°C (30°C/min) (1 min). Samples (2 μL) were injected with split 9:1; the carrier gas flow was 1 mL/min. The total run time was 9.8 min. Retention times (tR) were established as follow: tR = 5.62 min for (1R*,2S*)-1b and 5.65 min for (1R*,2R*)-1b, tR = 5.5 min for 1c; tR = 4.16 min for (1R*,2S*)-2b and 4.20 min for (1R*,2R*)-2b, tR = 3.92 min for 2c; tR = 5.22 min for (1R*,2S*)-3b and 5.26 min for (1R*,2R*)-3b, tR = 5.17 min for 3c; tR = 5.91 min for (1R*,2S*)-4b and 5.94 min for (1R*,2R*)-4b, tR = 5.89 min for 4c, and tR = 5.05 min for (1R*,2S*)-5b and (1R*,2R*)-5b. The structures of the compounds were confirmed using 1H nuclear magnetic resonance (NMR) and 13C NMR spectra of CDCl3 solutions recorded on Avance DRX 600 (600 MHz) and Avance II (400 MHz) spectrometers (Bruker, Billerica, MA, United States). GC–MS analyses were conducted using a HP-5MS column (30 m × 0.25 mm × 0.25 μm) (Agilent Technologies Italia S.p.A., Cernusco sul Naviglio, Italy) with the following temperature program: 60°C (1 min), 150°C (6°C/min) (1 min), 280°C (12°C/min) (5 min).
The NMR spectra of obtained products are as follows:
1-(1,3-benzodioxol-5-yl)propane-1,2-diol as a 3:1 diastereoisomeric mixture of (1R*,2S*) and (1R*,2R*)-1b.
(1R*,2S*)-1b 1H NMR (CDCl3, 400 MHz): δ = 6.86–6.84 (m, 1H, Ar–H), 6.81–6.76 (m, 2H, Ar–H), 5.96 (s, 2H, CH2), 4.29 (d, 1H, J = 7.4 Hz, CHOH), 3.81 (m, 1H, CHOH), 2.56 (s, 1H, OH), 2.42 (s, 1H; OH), 1.06 (d, 3H, J = 6.3 Hz, CH3); 13C NMR (CDCl3, 100 MHz): δ = 147.91, 147.5, 135.1, 120.5, 108.3, 107.2, 101.1, 79.4, 72.3, 18.9; GC/MS (EI) tr = 21.23 min: m/z (%) = 196 (M+, 16), 178 (8), 162 (8), 151 (100), 135 (25), 123 (25).
(1R*,2R*)-1b 1H NMR (CDCl3, 400 MHz): δ = 6.90–6.89 (m, 1H, Ar–H), 6.80–6.78 (m, 2H, Ar–H), 5.95 (s, 2H, CH2), 4.57 (d, 1H, J = 4.6 Hz, CHOH), 3.96 (m,1H, CHOH), 2.28 (s, 1H, OH), 1.83 (s, 1H; OH), 1.11 (d, 3H, J = 6.4 Hz, CH3); 13C NMR (CDCl3, 100 MHz): δ = 147.87, 147.3, 134.4, 120.2, 108.2, 107.1, 101.2, 77.5, 71.3, 17.6; GC/MS (EI) tr = 21.31 min: m/z (%) = 196 (M+, 16), 178 (8), 162 (8), 151 (100), 135 (25), 123 (25).
1-(1,3-benzodioxol-5-yl)-2-hydroxypropan-1-one 1c 1H NMR (CDCl3, 600 MHz): δ = 7.52–7.50 (m, 1H, Ar–H), 7.40–7.42 (m, 1H, Ar–H), 6.86–6.90 (m, 1H, Ar–H), 6.06 (s, 2H, CH2), 5.05 (q, 1H, J = 7.0 Hz, CHOH), 1.43 (d, 3H, J = 7.0 Hz, CH3); 13C NMR (CDCl3, 100 MHz): δ = 200.4, 152.6, 148.5, 127.9, 125.2, 108.4, 108.3, 102.2, 69.1, 22.8.; GC/MS (EI) tr = 20.82 min: m/z (%) = 194 (M+, 11), 149 (100), 121 (21), 91 (4), 65 (15).
1-(1,3-benzodioxol-5-yl)-1-hydroxypropan-2-one GC/MS (EI) tr = 19.95 min: m/z (%) = 194 (M+, 13), 178 (3), 151 (100), 135 (5), 123 (13), 93 (95), 65 (52).
1-(1,3-benzodioxol-5-yl)propane-1,2-dione 1H NMR (CDCl3, 400 MHz): δ = 7.64–7.62 (m, 1H, Ar–H), 7.49–7.49 (m, 1H, Ar–H), 6.89–6.87 (m, 1H, Ar–H), 6.07 (s, 2H, CH2), 2.49 (s, 3H, CH3);
13C NMR (CDCl3, 100 MHz): δ = 200.6, 189.4, 153.0, 148.2, 127.8, 126.2, 108.8, 108.1, 102.0, 26.3.; GC/MS (EI) tr = 19.21 min: m/z (%) = 192 (M+, 6), 149 (100), 121 (31), 91 (6), 65 (19).
1-phenylpropane-1,2-diol as a 1.25:1 diastereoisomeric mixture of (1R*,2S*) and (1R*,2R*)-2b.
(1R*,2S*)-2b 1H NMR (CDCl3, 400 MHz): δ = 7.39–7.27 (m, 5H, Ar–H), 4.38 (dd, 1H, J1 = 7.3 Hz, J2 = 2.7 Hz, CHOH), 3.91–3.82 (m, 1H, CHOH), 2.63–2.62 (m, 1H, OH), 2.47–2.46 (m, 1H, OH), 1.07 (d, 3H, J = 6.3 Hz, CH3); 13C NMR (CDCl3, 100 MHz): δ = 128.4, 128.0, 126.6, 125.6, 59.5, 59.0, 17.9.; GC/MS (EI) tr = 14.40 min: m/z (%) = 134 (M+ − 18, 3), 134 (3), 117 (7), 108 (100), 103 (2), 91 (17), 79 (93).
(1R*,2R*)-2b 1H NMR (CDCl3, 400 MHz): δ = 7.38–7.33 (m, 5H, Ar–H), 4.69–4.67 (m, 1H, CHOH), 4.06–3.97 (m, 1H, CHOH), 2.38–2.37 (m, 1H,OH), 1.88–1.87 (m, 1H, OH), 1.09 (d, 3H, J = 6.4 Hz); 13C NMR (CDCl3, 100 MHz): δ = 128.4, 128.0, 126.6, 125.6, 59.5, 59.0, 17.9.; GC/MS (EI) tr = 14.60 min: m/z (%) = 134 (M+ − 18, 3), 134 (3), 117 (13), 108 (100), 103 (3), 91 (18), 79 (93).
2-hydroxy-1-phenylpropan-1-one 2c 1H NMR (CDCl3, 600 MHz): δ = 7.93–7.91 (m, 2H, Ar–H), 7.93–7.91 (m, 1H, Ar–H), 7.50–7.47 (m, 2H, Ar–H), 5.19–5.14 (q, 1H, J = 7.0 Hz, CHOH), 1.24 (s, 1H, OH), 1.21 (d, 3H, J = 9.5 Hz, CH3); 13C NMR (CDCl3, 100 MHz): δ = 202.5, 134.1, 133.7, 130.3, 128.9, 128.8, 128.6, 69.4, 13.3. GC/MS (EI) tr = 13.07 min: m/z (%) = 135 (M+ − 15, 1), 135 (1), 105 (100), 77 (43), 51 (12).
1-hydroxy-1-phenylpropan-2-one GC/MS (EI) tr = 12.34 min: m/z (%) = 150 (M+, 2), 107 (100), 89 (2), 79 (85).
1-phenylpropane-1,2-dione 1H NMR (CDCl3, 400 MHz): δ = 8.02–8.00 (m, 2H, Ar–H), 7.66–7.60 (m, 1H, Ar–H), 7.52–7.48 (m, 2H, Ar–H), 2.53 (s, 3H, CH3); 13C NMR (CDCl3, 100 MHz): δ = 200.5, 191.4, 134.6, 131.8, 130.3, 128.9, 26.4.; GC/MS (EI) tr = 11.23 min: m/z (%) = 148 (M+, 4), 105 (100), 77 (71), 51 (20).
1-(4-methoxyphenyl)propane-1,2-diol as a 3:1 diastereoisomeric mixture of (1R*,2S*) and (1R*,2R*)-3b.
(1R*,2S*)-3b 1H NMR (CDCl3, 400 MHz) δ = 7.30–7.25 (m, 2H, Ar-H), 6.91–6.87 (m, 2H, Ar-H), 4.33 (dd, 1H, J1 = 7.7 Hz, J2 = 2.9 Hz, CHOH), 3.89–3.82 (m, 1H, CHOH), 3.81 (s, 3H, OCH3), 2.47–2.42 (m, 2H, OH), 1.05 (d, 3H, J = 7.4 Hz, CH3); 13C NMR (CDCl3, 100 MHz): δ = 159.5, 146.6, 133.2, 128.0, 114.0, 79.2, 72.3, 18.8.; GC/MS (EI) tr = 19.75 min: m/z (%): 182 (M+, 4), 164 (6), 137 (100), 121 (35), 109 (20), 94 (17).
(1R*,2R*)-3b 1H NMR (CDCl3, 400 MHz) δ = 7.30–7.25 (m, 2H, Ar-H), 6.91–6.87 (m, 2H, Ar-H), 4.61–4.59 (m, 1H, CHOH), 4.01–3.97 (m, 1H, CHOH), 3.81 (s, 3H, OCH3), 2.21–2.20 (m, 1H, OH), 1.79–1.78 (m, 1H, OH), 1.11 (d, 3H, J = 6.4 Hz, CH3); 13C NMR (CDCl3, 100 MHz): δ = 159.5, 146.6, 133.2, 128.0, 114.0, 79.2, 72.3, 18.8.; GC/MS (EI) tr = 19.83: m/z (%): 182 (M+, 3), 164 (6), 137 (100), 121 (37), 109 (19), 94 (17).
2-hydroxy-1-(4-methoxyphenyl)propan-1-one 3c 1H NMR (CDCl3, 600 MHz): δ = 7.51–7.49 (m, 2H, Ar–H), 6.92–6.88 (m, 2H, Ar–H), 5.16–5.06 (m, 1H, CHOH), 3.93 (s, 3H, OCH3), 1.43 (d, 3H, J = 7.0 Hz, CH3); 13C NMR (CDCl3, 100 MHz): δ = 201.2, 164.1, 132.9, 132.4, 131.1, 114.2, 113.8, 69.0, 55.6, 22.8; GC/MS (EI) tr = 19.96: m/z (%): 180 (M+, 3), 135 (100), 107 (9), 92 (10), 77 (14).
1-hydroxy-1-(4-methoxyphenyl)propan-2-one GC/MS (EI) tr = 18.79: m/z (%): 180 (M+, 3), 137 (100), 109 (25), 94 (25), 77 (23).
1-(4-methoxyphenyl)propane-1,2-dione 1H NMR (CDCl3, 400 MHz): δ = 8.03–8.00 (m, 2H, Ar–H), 6.98–6.94 (m, 2H, Ar-H), 3.89 (s, 3H, OCH3), 2.50 (s, 3H, CH3); 13C NMR (CDCl3, 100 MHz): δ = 201.1, 190.0, 164.8, 132.8, 124.7, 114.2, 55.6, 26.5.; GC/MS (EI) tr = 17.63 min: m/z (%) = 178 (M+, 2), 135 (100), 107 (11), 92 (16), 77 (22).
1-(3,4-dimethoxyphenyl)propane-1,2-diol as a 3:1 diastereoisomeric mixture of (1R*,2S*) and (1R*,2R*)-4b.
(1R*,2S*)-4b 1H NMR (CDCl3, 400 MHz) δ = 6.94–6.84 (m, 3H, Ar-H), 4.32–4.30 (d, 1H, CHOH), 3.89 (s, 3H, OCH3), 3.87 (s, 3H, OCH3), 3.85–3.81 (m, 1H, CHOH), 2.69 (s, 1H, OH), 2.54 (s, 1H, OH), 1.06 (d, 3H, J = 6.3 Hz, CH3); 13C NMR (CDCl3, 100 MHz): δ = 149.1, 148.9, 133.7, 119.3, 119.1, 111.1, 109.8, 79.3, 72.2, 55.9, 18.8.; GC/MS (EI) tr = 22.22 min: m/z (%) = 212 (M+, 2), 194 (24), 178 (9), 167 (12), 151 (100), 139 (8).
(1R*,2R*)-4b 1H NMR (CDCl3, 400 MHz) δ = 6.84–6.82 (m, 3H, Ar-H), 4.59–4.58 (m, 1H, CHOH), 3.89 (s, 3H, OCH3), 4.01–3.95 (m, 1H, CHOH), 3.87 (s, 3H, OCH3), 2.41 (s, 1H, OH), 1.91 (s, 1H, OH), 1.12 (d, 3H, J = 6.1 Hz, CH3); 13C NMR (CDCl3, 100 MHz): δ = 149.0, 148.9, 133.1, 119.1, 111.0, 109.8, 77.5, 71.4, 55.9, 17.5; GC/MS (EI) tr = 22.22 min: m/z (%) = 212 (M+, 2), 194 (24), 178 (9), 167 (12), 151 (100), 139 (8).
1-(3,4-dimethoxyphenyl)-2-hydroxypropan-1-one 4c 1H NMR (CDCl3, 600 MHz): δ = 7.52–7.49 (m, 2H, Ar–H), 6.91–6.89 (m, 1H, Ar–H), 5.14–5.08 (m, 1H, CHOH), 3.94 (s, 3H, OCH3), 3.93 (s, 3H, OCH3), 1.43 (d, 3H, J = 7.1 Hz, CH3); 13C NMR (CDCl3, 100 MHz): δ = 200.9, 154.1, 149.4, 126.2, 123.5, 110.8, 110.2, 68.9, 56.2, 56.1, 23.0.; GC/MS (EI) tr = 22.20 min: m/z (%) = 210 (M+, 9), 192 (2), 165 (100), 151 (5), 137 (9), 122 (6).
1-(3,4-dimethoxyphenyl)-1-hydroxypropan-2-one GC/MS (EI) tr = 21.20 min: m/z (%) = 210 (M+, 6), 192 (7), 167 (100), 151 (22), 139 (61), 124 (23).
1-(3,4-dimethoxyphenyl)propane-1,2-dione 1H NMR (CDCl3, 400 MHz): δ = 7.67–7.65 (m, 1H, Ar–H), δ = 7.58–7.76 (m, 1H, Ar–H), 6.92–6.90 (m, 1H, Ar–H), 3.97 (s, 3H, OCH3), 3.94 (s, 3H, OCH3), 2.51 (s, 3H, CH3); 13C NMR (CDCl3, 100 MHz): δ = 200.9, 190.0, 154.7, 149.3, 126.6, 124.6, 111.0, 110.2, 56.0, 55.9, 26.5.; GC/MS (EI) tr = 21.00 min: m/z (%) = 208 (M+, 3), 165 (100), 137 (9), 122 (8).
1-(4-hydroxy-3-methoxyphenyl)propane-1,2-diol as a 3:1 diastereoisomeric mixture of (1R*,2S*) and (1R*,2R*)-5b.
(1R*,2S*)-5b 1H NMR (CDCl3, 400 MHz) δ = 6.94–6.87 (m, 3H, Ar-H), 5.62 (s, 1H, Ar-OH), 4.31 (d, 1H, J = 7.5 Hz, CHOH), 3.90 (s, 3H, OCH3), 3.87–3.81 (m, 1H, CHOH), 1.06 (d, 3H, J = 6.3 Hz, CH3); 13C NMR (CDCl3, 100 MHz): δ = 146.7, 145.6, 133.0, 120.0, 114.3, 109.0, 79.4, 72.3, 56.0, 18.9.; GC/MS (EI) tr = 21.71 min: m/z (%) = 198 (M+, 12), 180 (9), 153 (100), 137 (40), 125 (19).
(1R*,2R*)-5b 1H NMR (CDCl3, 400 MHz) δ = 6.84–6.80 (m, 3H, Ar-H), 5.62 (s, 1H, Ar-OH), 4.57 (d, 1H, J = 4.8 Hz, CHOH), 3.99–3.96 (m, 1H, CHOH), 3.90 (s, 3H, OCH3), 1.12 (d, 3H, J = 6.4 Hz, CH3); 13C NMR (CDCl3, 100 MHz): δ = 146.0, 145.4, 132.4, 119.9, 114.2, 109.2, 77.6, 71.4, 56.0, 17.6.; GC/MS (EI) tr = 21.71 min: m/z (%) = 198 (M+,12), 180 (9), 153 (100), 137 (40), 125 (19).
The biotransformation and biological activity experiments were performed in triplicate, and the data are presented in tables and figures as means and standard deviations. Student’s t-test was used to compare the means. Differences with p < 0.05 were considered significant. Statistical analyses were performed using Past 4.02 (Oyvind Hammer).
In this research, we focused on propenylbenzenes 1a–5a (Figure 1), as these compounds have been reported to possess various biological activities, such as antimicrobial, antioxidant, and antiproliferative activities (Moradi et al., 2014; Siva et al., 2019; Bruna et al., 2022; Lal et al., 2022). Taking this into consideration, several derivatives of these compounds were obtained in order to study the influence of their structure on biological activity.
The two-step biocatalytic synthesis (Figure 2) of oxygenated propenylbenzene derivatives involved (1) chemo-enzymatic epoxidation followed by hydrolysis of the starting compounds 1a–5a to the corresponding diols 1b–5b and (2) microbial oxidation of the diols 1b–5b into hydroxy ketones 1c–4c. The thus-obtained propenylbenzenes derivatives were tested for their antimicrobial, antioxidant, haemolytic, and anticancer activities and their impact on membrane fluidity.
Figure 2. Two-step synthesis of propenylbenzene derivatives: diols 1b–5b and hydroxy ketones 1c–4c. 1. aq. H2O2, Novozym 435, EtOAc, 30°C, 18 h, KOH, MeOH; 2. PCM medium, 23°C, 3–11 days.
In the first step, compounds 1a–5a were converted into the corresponding diols accordingly to the method recently described by Tentori et al. (2021) for isosafrole (1a). This method is based on the Prilezhaev reaction of isosafrole with a peroxycarboxylic acid obtained in situ by lipase-catalysed perhydrolysis of the corresponding carboxylic acid in the presence of H2O2. We used aqueous H2O2 and commercial immobilized lipase B from Candida antarctica (Novozym 435) based on a report by Björkling et al. (1990). We modified this method, using ethyl acetate, which undergoes lipase-mediated perhydrolysis, resulting in the formation of ethanol and peroxyacetic acid. This avoided the addition of octanoic acid and other carboxylic acids to promote the reaction. However, after applying this method to compound 1a, we noticed that diol 1b was formed only in small amounts, whereas the dominant products were epoxide and monoacetate derivatives. Therefore, methanolic KOH was added to the compound mixture, which allowed for complete oxirane ring cleavage and promoted monoacetate hydrolysis. As a result of this reaction, epoxide and the monoacetate derivatives were readily converted into vicinal diol 1b, with a high yield (69%). Considering these results, we decided to apply this method also to the other structurally similar propenylbenzenes 2a–5a, which allowed to obtain corresponding diols 2b–5b for use as substrates in biotransformation processes. As a result of implementing the aforementioned method, a diasteroisomeric mixture of (R*,S*)- and (R*,R*)-diols 1b–5b was obtained, with high isolation yields in the range of 65–80%. Compared to other methods described in the literature, the method we proposeis highly efficient, using an immobilized biocatalyst that can be easily recovered from the reaction by filtration, and employing cheap H2O2 as an oxidant. Other enzymatic methods described so far (Kihumbu et al., 2002; Oeggl et al., 2018) were aimed at obtaining pure enantiomers of selected propenylbenzenes, whereas in our method we focused on obtaining diols as racemic diastereoisomers to be submitted to further microbial transformations.
Screening-scale whole-cell transformations of diols 1b–5b were conducted using 22 bacterial strains belonging to the genera Bacillus, Dietzia, Gordonia, Micrococcus, Pseudomonas, Rhodococcus, Serratia, and Streptomyces. In biotransformations with diols 1b–4b, corresponding hydroxy ketones 1c–4c were formed as the main products. In addition, small amounts of diketones and isomeric forms of hydroxy ketones were detected in the reaction mixture (data are shown in the Supplementary Tables S1–S4). The most efficient biocatalysts (Dietzia sp. DSM44016, R. erythropolis PCM2150, R. erythropolis DSM44534, and R. ruber PCM2166) in the screening-scale experiments are listed in Table 1. In all biotransformations, the amount of hydroxy ketones 1c–4c (determined by GC) clearly increased over time up to day 11. The highest percentage of product 1c (85%) was observed in the biotransformation with Dietzia sp. DSM44016. In contrast, hydroxy ketones 2c and 3c were the most efficiently produced in the biotransformation with R. ruber PCM2166, and a high amount of product 4c (77–79%) was obtained in the biotransformations conducted by R. erythropolis DSM44534 and R. ruber PCM2166. All strains mentioned effectively produced hydroxy ketones, except for the biotransformation of compound 3b with strain R. erythropolis PCM2150, after which only 38% hydroxy ketone 3c was detected. Due to the small differences in biotransformations with these bacteria, all strains were selected for preparative scale biotransformations, which allowed to isolate products and determine their structures by NMR analysis. Biotransformation of diol 5b did not yield hydroxy ketones or other products; therefore, this substrate was not studied on a preparative scale.
When planning the scale-up process, we decided to add substrates 1b–4b in an amount of 0.2 g each and to conduct the biotransformation process for 11 days (Table 2). During preparative-scale biotransformation of substrate 1b, the highest amount of product 1c (0.125 g, yield = 62.5%) was obtained using Dietzia sp. DSM44016. In the course of biotransformation of diol 2b, only R. ruber PCM2166 catalysed the reaction, affording 0.081 g of 2c (yield = 40.5%). The high concentration of substrate 2b had an inhibitory effect on all other strains, and only 10–24% of hydroxy ketone 2c and unreacted substrate were detected in the samples. The highest amount of hydroxy ketone 3c (0.115 g, yield = 57.5%) was obtained with R. erythropolis DSM44534. Finally, R. erythropolis PCM2150 yielded 0.119 g (59.5%) of product 4c.
Table 2. Summary of the biotransformations of propenylbenzenes 1a–4a performed on a preparative scale after 11 days.
During the preparative-scale biotransformations, we noticed that an increase in the substrate concentration significantly affected the course of the process with selected bacterial strains. In the case of Dietzia sp. DSM44016, the highest yields were achieved in the biotransformation of substrate 1b, which has a dioxolane group in its structure, whereas hydroxy ketones 3c and 4c (with one and two methoxy groups, respectively) were obtained with lower yields. However, diol 2b (without additional substituent) was toxic to this strain and only small amount of product 2c was detected by GC. These findings indicated that the dioxolane, methoxy, and dimethoxy groups reduce the toxicity of diols derived from propenylbenzenes on Dietzia sp. DSM44016. Biotransformation with R. ruber PCM2166 yielded a significantly smaller amount of compound 2c, and with substrates 1b and 3b–4b, no products were formed. This indicates that R. ruber PCM2166 accepts only propenylbenzene diol derivatives without aromatic ring substituents. Biotransformations with R. erythropolis DSM44534 and R. erythropolis PCM2150 were characterized by a similar course of bio-oxidation, which is not surprising considering that they belong to the same species. These microorganisms effectively catalysed the oxidation of diols 1b and 3b–4b to corresponding hydroxy ketones 1c and 3c–4c. The high concentration of substrate 2b had an inhibitory effect on these strains, as observed with Dietzia sp. DSM44016.
Several methods for the synthesis of hydroxy ketones 1c–4c have been reported (Kihumbu et al., 2002; Peng et al., 2005; Kurlemann et al., 2009). In one method using 2,3-dichloro-5,6-dicyano-1,4-benzoquinone and ultrasound waves, compound 1c was obtained with a 72% yield (Peng et al., 2005). Enzymatic reaction with benzaldehyde lyase or benzoylformate decarboxylase afforded hydroxy ketone 2c with a yield of 95% (Kihumbu et al., 2002). Hydroxy ketone 3c (yield = 81–84%) was obtained in a two-step process involving oxidation of trans-anethol using Trametes hirsuta lyophilisate followed by ligation of para-anisaldehyde with acetaldehyde using benzaldehyde lyase or benzoylformate decarboxylase (Kurlemann et al., 2009). The biocatalytic method presented in this paper is an attractive alternative to the methods described in the literature. It does not require expensive reagents and enzymes and allows to work starting from propenylbenzenes, most of which can be recovered from renewable feedstocks (natural essential oils and plants extracts).
The fungistatic activities of propenylbenzenes 1a–5a have been described in the literature (Moradi et al., 2014; Siva et al., 2019; Bruna et al., 2022; Lal et al., 2022). The MIC values of these compounds range from 100 to 400 μg/mL, with the lowest value reported for isosafrole (1a) and the highest for isoeugenol (5a) and its derivative 4a (Kubo et al., 1993). These studies were conducted using C. albicans ATTC18804, and it should be noted that MIC50 values were not calculated. Additionally, studies using the disc diffusion method for C. albicans ATCC 66027 and the microdilution method (with a different cell density) for C. albicans ATCC 22019 showed that anethole (3a) and essential oil containing isosafrole (1a) have antifungal activity (Moradi et al., 2014; Bruna et al., 2022; Lal et al., 2022). The antimicrobial activities of isosafrole (1a), anethol (3a), and isoeugenol (5a) have been reported; however, antimicrobial activities of their derivatives, such as diols and hydroxy ketones, have not been reported. Therefore, we assessed how the structures of these compounds affect their biological activity (Moradi et al., 2014; Siva et al., 2019; Bruna et al., 2022; Lal et al., 2022). In our previous studies on fungistatic activity, we used yeasts of the genus Candida (Gach et al., 2021; Krężel et al., 2022) therefore, we used C. albicans strains as the test model in the current study.
Isosafrole (1a) efficiently inhibited the growth of C. albicans strains 636/20, 595/20, 38, and ATTC90028, with MIC50 values below 100 μg/mL (Figure 3A). The highest inhibitory activity (MIC50 = 65 μg/mL) of this compound was noted for C. albicans 595/20. The fungistatic activity of dihydroxy derivative 1b was decreased compared to that of the starting compound 1a. The MIC50 values of diol 1b for C. albicans 595/20 and 636/20 were 107 and 177 μg/mL, respectively. Compared to those of 1a and 1b, the inhibitory activity of hydroxy ketone 1c against C. albicans strains 595/20, 38, and ATTC90028 was substantially decreased (MIC50 = 178–200 μg/mL). Compound 1c showed no inhibitory activity against C. albicans 636/20, even at a concentration of 250 μg/mL. The presence of hydroxy and carbonyl groups in compounds 1b–c suppressed their fungistatic activity against all tested strains, whereas the presence of two hydroxy groups in compounds 2a–c increased their fungistatic activity against all tested strains (Figure 3B). Compared to 2a and 2c, diol 2b showed the highest inhibitory activity against all tested strains, with the lowest noted for C. albicans 636/20 (MIC50 = 121 μg/mL) and ATTC90028 (MIC50 = 125 μg/mL). The growth of C. albicans 38 and ATTC90028 was not affected by compounds 2a and 2c, even at concentrations above 250 μg/mL. Anethole (3a) exhibited significant inhibitory activity against all tested strains, with MIC50 values of 62–100 μg/mL and the highest activity noted for C. albicans 595/20 (MIC50 = 62 μg/mL) (Figure 3C). Diol 3b showed increased fungistatic activity against C. albicans 595/20, 636/20, and 38 (MIC50 = 47–76 μg/mL), with the lowest MIC50 of 47 μg/mL for C. albicans 595/20. Hydroxy ketone 3c showed increased fungistatic activity against C. albicans 38 (MIC50 = 61 μg/mL). Compounds 3a–c showed variable fungistatic activity against the tested strains; therefore, the impacts of their structures on fungistatic activity were difficult to determine. Among compounds 4a–c, 4a (MIC50 = 39–67 μg/mL) and 4b (MIC50 = 37–63 μg/mL) showed the highest fungistatic activity against all strains, with diol 4b showing higher activity against C. albicans 636/20, 595/20, and ATTC90028 (Figure 3D). The presence of a carboxyl group in compound 4c substantial suppressed its fungistatic activity. Isoeugenol (5a) showed substantially higher fungistatic activity than its diol derivative 5b against all four strains (MIC50 = 52–88 μg/mL) (Figure 3E). By comparing the various compounds and their derivatives, we noticed that the presence of additional substituents in the aromatic ring of the starting propenylbenzenes 1a, 3a, 4a, and 5a had a positive effect on their fungistatic activity when compared to that of 2a. The presence of hydroxy groups in diols 2b–4b had a positive effect on their inhibitory activity against most strains tested when compared with that of the starting compounds 2a–4a. All hydroxy diols, except 3c, tended to be less active than the starting compounds and diols.
Figure 3. MIC50 values [μg/mL] for all tested compounds against C. albicans strains. The results are shown as mean values ± standard deviations.
The antiradical activities of some propenylbenzenes or essential oils containing them have been reported in the literature. Eid and Hawash (2021) reported that safrole (the isomer of compound 1a) exhibits antioxidant activity, with an IC50 value of 50.28 μg/mL. A study on the antiradical activity of isosafrole (1a) in essential oils containing a certain amount of this compound (19.5%) revealed that such mixtures of different compounds exhibit IC50 values >1,000 μg/mL (Bruna et al., 2022). For anethol (3a), an EC50 value of 8.69 has been reported (Lal et al., 2022). According to Siva et al. (2019), the antioxidant activity of isoeugenol (5a) was 86%.
The antioxidant activities of all compounds were compared with that of the standard, ascorbic acid, to estimate their free radical-scavenging power. The lowest EC50 value was noted for ascorbic acid (15.21 μg/mL), which was used as a positive control (Figure 4). Among the tested compounds, those with a double bond in the propenyl group had the lowest EC50 values. Among these, anethole (3a) showed the highest antiradical activity (EC50 = 19.13 μg/mL). Significantly lower antioxidant activity was observed for diols 1b–5b and hydroxy ketones 1c–4c, with EC50 values ranging from 36.34 to 72.08 μg/mL. Among the diols, 3b showed the highest antiradical activity. All hydroxy ketones showed lower activity than the starting compounds and diols.
Figure 4. EC50 values [μg/mL] for all tested compounds in comparison to ascorbic acid. The results are shown as mean values ± standard deviations.
The degree of cytotoxicity of the compounds was evaluated using a haemolytic activity assay, in which the haemolytic activity of 13 compounds at 10–100 μM on human RBCs was evaluated. According to toxicity classification, compounds are highly toxic if the haemolysis rate is 90–100% and nontoxic if the haemolysis rate is 0–9% (Pagano and Faggio, 2015). Data shown in the Supplementary Table S5 show the percentage of haemolysis after a 1 h incubation with the compounds at various concentrations at 37°C. The percentage of haemolysis was similar to that of the control for all compounds and did not exceed 3%; therefore, oxygenated derivatives of propenylbenzenes do not cause haemolysis of RBCs. Our results indicate that the compounds in the range of used concentrations do not have a toxic effect on human red blood cells.
The results obtained for compounds 1a and 5a are in agreement with the results of other authors, in which it was shown that derivatives of safrole or eugenol present negligible hemolytic capacity (Hidalgo and De la Rosa, 2009; Madrid et al., 2014). However, all derivatives of safrole exhibited haemolytic activity (minor than 10%), and for the derivatives of eugenol activity lower than 1% was detected, which indicate their non-toxicity. Other authors have also shown that isoeugenol has low cytotoxic activity (Bhatia et al., 2011). Due to the fact that compounds 1-5b and 1-4c were described for the first time, the results obtained for these compounds are novel.
The effect of each compound on the membrane fluidity of RBCs was examined using the fluorescent marker DPH. The DPH probe binds to the hydrophobic region of the membrane. Based on the change in anisotropy, one can infer the degree of change in the membrane fluidity of RBCs (Lakowicz, 2006). An increase in the anisotropy value indicates an increase in membrane stiffness, whereas a decrease suggests liquefaction of the membrane (complete data are shown in the Supplementary Table S6). In general, diols, particularly 2b and 4b, were found to cause an increase in anisotropy (Figure 5). Interestingly, compound 3b caused an increase in anisotropy at lower concentrations and a decrease at higher concentrations. In contrast, hydroxy ketones 2c, 3c, and, to a lesser extent, 4c caused a decrease in anisotropy, indicating an increase in membrane fluidity. The biological activity of the studied compounds likely is also related to their different effects on the cell membrane; depending on their structure, they cause an increase in membrane stiffness or membrane liquefaction. Determining the exact localization of the compounds in the membrane requires further research.
Figure 5. Fluorescence anisotropy values of the DPH probe in membranes of RBCs treated with the compounds at 20 μM concentration. The results are shown as mean values ± standard deviations.
The proliferative activities of HepG2, Caco-2, and MG63 cells were differentially affected by compounds (1a–5b), in a dose-dependent manner (complete data are shown in the Supplementary Figures S27−S29). Compound 3b significantly increased the proliferative potential of HepG-2 cells, whereas compound 2b showed the highest inhibitory effect on HepG2 cell viability (Figure 6). A substantially different cellular response to the compounds was observed in the Caco-2 cell line. Caco-2 cells exhibited the lowest proliferative activity when treated with all tested compounds among all others tested cell lines. The strongest inhibition of Caco-2 cells was observed when the cells were treated with 1b, 2b, 3b, and 4b. Interestingly, 1a and 2a more strongly significantly suppressed the viability of HepG-2 cells. Compound 5b at 1 μg/mL induced the proliferative activity of all cell lines to a similar level as compound 3c did at 50 μg/mL. The proliferation of MG63 cells was differentially modulated by the compounds, depending on the dosage. Compounds 1c, 3b, and 5b suppressed the proliferation of MG63 cells. However, at 50 μg/mL, 5b significantly increased cell viability. Interestingly, compounds 1a, 2a, 2c, 3a, 3c, 4a, 4c, and 5a promoted MGC63 proliferation.
Figure 6. Absorbance values of HepG2, Caco-2 and MG63 cell lines treated with the compounds at concentrations from 0 to 200 mg/mL, where 1. 0 mg/mL (0% EtOH); 2. 1 mg/mL (0.01% EtOH); 3. 50 mg/mL (0.5% EtOH); 4. 200 mg/mL (2% EtOH). The results are shown as mean values ± standard deviations.
The results obtained by us for starting propenylbenzenes 1-5a are in agreement with the available literature. Anethol (3a), safrole and eugenol (isomers of compounds 1a and 5a) inhibited the growth of human hepatocellular carcinoma HepG2 cells (Yoo et al., 2005; Song et al., 2014, 2020). Whereas, isosafrole (1a) and eugenol induced apoptosis in human colon carcinoma Caco-2 cell line (Lea et al., 2016; Padhy et al., 2022). In human osteosarcoma MG63 cell line, trans-anethole (3a) caused abrogation in proliferation and induces apoptosis through the mitochondrial mediated pathway (Pandit et al., 2020). The effect of compounds 1-5b and 1-4c on the cell lines we studied has not been described in the available literature so far.
A two-step chemo-enzymatic method for obtaining oxygenated derivatives of commercially available propenylbenzenes was developed. The process involves chemo-enzymatic epoxidation followed by epoxide hydrolysis of starting compounds 1a–5a to corresponding diols 1b–5b, followed by microbial oxidation of thus obtained diols 1b–5b into hydroxy ketones 1c–4c. Among bacteria from different genera, Dietzia sp. DSM44016, R. erythropolis DSM44534, R. erythropolis PCM2150, and R. ruber PCM2166 effectively oxidized diols 1b–4b to corresponding hydroxy ketones 1c–4c, indicating high alcohol dehydrogenase activity. Bio-oxidation performed on preparative scale afforded hydroxy ketones 1c–4c with good isolation yields. The obtained compounds and starting propenylbenzenes were tested for antimicrobial, antioxidant, haemolytic, and anticancer activities, and their impact on membrane fluidity. Compounds 1a, 3a–c, 4a,b, and 5a,b showed high fungistatic activity against selected strains of C. albicans. The type of substituent can significantly affect the MIC50 value. The starting propenylbenzenes 1a–5a with a double bond in their structure showed the highest antiradical activity among the tested compounds. The compounds showed no cytotoxicity against human RBCs. However, compounds 2b–4b and 2c–4c affected the fluidity of the RBCs membrane; diols generally increased membrane stiffness, whereas hydroxy ketones increased membrane fluidity. Several compounds inhibited or promoted HepG2, Caco-2, and MG63 cell proliferation, depending on the concentration they were used at. Obtained data shed promising light on the application of tested compounds as hepatoprotective and promoting bone remodeling.
The original contributions presented in the study are included in the article/Supplementary material, further inquiries can be directed to the corresponding authors.
DH, FB, and EB: conceptualization. DH, AW, HP, KM, and FB: formal analysis. DH: funding acquisition. DH, ES, MG, AW, HP, and MM: investigation. DH, AW, HP, KM, TO, EB, and FB: methodology. DH and FB: resources. FB and EB: supervision. DH, AW, HP, and KM: visualization. DH, AW, HP, KM, and FB: writing – original draft. ES, TO, and EB: writing – review and editing. All authors contributed to the article and approved the submitted version.
This research was funded by the project “UPWR 2.0: international and interdisciplinary program of development of Wrocław University of Environmental and Life Sciences,” co-financed by the European Social Fund under the Operational Program Knowledge Education Development, under contract No. POWR.03.05.00-00-Z062/18 of 4 June 2019. The APC is financed by Wroclaw University of Environmental and Life Science.
The authors would like to thank Editage for providing the editing service.
The authors declare that the research was conducted in the absence of any commercial or financial relationships that could be construed as a potential conflict of interest.
All claims expressed in this article are solely those of the authors and do not necessarily represent those of their affiliated organizations, or those of the publisher, the editors and the reviewers. Any product that may be evaluated in this article, or claim that may be made by its manufacturer, is not guaranteed or endorsed by the publisher.
The Supplementary material for this article can be found online at: https://www.frontiersin.org/articles/10.3389/fmicb.2023.1223123/full#supplementary-material
Aprotosoaie, A. C., Costache, I., and Miron, A. (2016). Anethole and its role in chronic diseases. Adv Exp. Med. Biol. 929, 247–267. doi: 10.1007/978-3-319-41342-6_11
Atsumi, A., Fujisawa, S., and Tonosaki, K. (2005). A comparative study of the antioxidant/prooxidant activities of eugenol and isoeugenol with various concentrations and axidation conditions. Toxicol. In Vitro 19, 1025–1033. doi: 10.1016/j.tiv.2005.04.012
Bhatia, R., Shreaz, S., Khan, N., Muralidhar, S., Basir, S. F., Manzoor, N., et al. (2011). Proton pumping ATPase mediated fungicidal activity of two essential oil components. J. Basic Microbiol. 51, 504–512. doi: 10.1002/jobm.201100272
Björkling, F., Godtfredsen, S. E., and Kik, O. (1990). Lipase-mediated formation of peroxycarboxylic acids used in catalytic epoxidation of alkenes. J. Chem. Soc. Chem. Commun. 19, 1301–1303. doi: 10.1039/C39900001301
Bruna, F., Fernandez, K., Urrejola, F., Touma, J., Navarro, M., Sepulveda, B., et al. (2022). Chemical composition, antioxidant, antimicrobial and antiproliferative activity of Laureliopsis philippiana essential oil of Chile, study in vitro and in silico. Arab. J. Chem. 15:104271. doi: 10.1016/j.arabjc.2022.104271
Cabral, P. H. B., Campos, R., Fonteles, M. C., Santos, C. F., Cardoso, J. H. L., and Falcao do Nascimento, N. R. F. (2014). Effects of the essential oil of Croton zehntneri and its major components, anethole and estragole, on the rat corpora cavernosa. Life Sci. 112, 74–81. doi: 10.1016/j.lfs.2014.07.022
D’Accolti, L., Detomaso, A., Fusco, C., Rosa, A., and Curci, R. (1993). Selective oxidation of optically active sec,sec-1,2-diols by dioxiranes. A practical method for the synthesis of homochiral α-hydroxy ketones in high optical purity. J. Org. Chem. 58, 3600–3601. doi: 10.1021/jo00066a002
Dudek, A., Spiegel, M., Strugała-Danak, P., and Gabrielska, J. (2022). Analitical and theoretical studies of antioxidant properties of chosen anthocyanins; a structure-dependent relationship. Int. J. Mol. Sci. 23:5432. doi: 10.3390/ijms23105432
Eid, A. M., and Hawash, M. (2021). Biological evaluation of safrole oil and safrole oil nanoemulgel as antioxidant, antibacterial, antifungal and anticancer. BMC Complement. Med. Ther. 21:159. doi: 10.1186/s12906-021-03324-z
Fahlbusch, K. G., Hammerschmidt, F. J., Panten, J., Pickenhagen, W., Schatkowski, D., Bauer, K., et al. (2003). “Flavors and fragrances,” in Ullmann’s Encyclopedia of Industrial Chemistry, (Weinheim: Wiley-VCH Verlag GmbH), 341–358. doi: 10.1002/14356007.a11_141
Gach, J., Olejniczak, T., Krężel, P., and Boratyński, F. (2021). Microbial synthesis and evaluation of fungistatic activity of 3-butyl-3-hydroxyphthalide, the mammalian metabolite of 3-n-butylidenephthalide. Int. J. Mol. Sci. 22:7600. doi: 10.3390/ijms22147600
Grzesiak, J., Marycz, K., Czogala, J., Wrzeszcz, K., and Nicpoń, J. (2011). Comparison of behavior, morphology and morphometry of equine and canine adipose derived mesenchymal stem cells in culture. Int. J. Morphol. 29, 1012–1017. doi: 10.4067/S0717-95022011000300059
Hassam, M., Taher, A., Arnott, G. E., Green, I. R., and van Otterlo, W. A. L. (2015). Isomerization of allylbenzenes. Chem. Rev. 115, 5462–5569. doi: 10.1021/acs.chemrev.5b00052
Herald, T. J., Gadgil, P., and Tilley, M. (2012). High-throughput micro plate assays for screening flavonoid content and DPPH-scavenging activity in sorghum bran and flour. J. Sci. Food Agric. 92, 2326–2331. doi: 10.1002/jsfa.5633
Hidalgo, M. E., and De la Rosa, C. (2009). Antioxidant capacity of eugenol derivatives. Quim Nova 6, 1467–1470. doi: 10.1590/S0100-40422009000600020
Kihumbu, D., Stillger, T., Hummel, W., and Liese, A. (2002). Enzymatic synthesis of all stereoisomers of 1-phenylpropane-1,2-diol. Tetrahedron Asymmetry 13, 1069–1072. doi: 10.1016/S0957-4166(02)00247-1
Kornicka, K., Kocherova, I., and Marycz, K. (2017). The effect of chosen plant extracts and compounds on mesenchymal stem cells-a bridge between molecular nutrition and regenerative medicine-concise review. Phytother. Res. 31, 947–958. doi: 10.1002/ptr.5812
Krężel, P., Olejniczak, T., Tołoczko, A., Gach, J., Weselski, M., and Bronisz, R. (2022). Synergic effect of phthalide lactones and fluconazole and its new analogues as a factor limiting the use of azole drugs against candidiasis. Antibiotics 11:1500. doi: 10.3390/antibiotics11111500
Kubo, I., Muroi, H., and Himejima, M. (1993). Combination effects of antifungal nagilactones against Candida albicans and two other fungi with phenylpropanoids. J. Nat. Prod. 56, 220–226. doi: 10.1021/np50092a006
Kulig, J., Frese, A., Kroutil, W., Pohl, M., and Rother, D. (2013). Biochemical characterization of an alcohol dehydrogenase from Ralstonia sp. Biotechnol. Bioeng. 110, 1838–1848. doi: 10.1002/bit.24857
Kurlemann, N., Lara, M., Pohl, M., Kroutil, W., and Liese, A. (2009). Asymmetric synthesis of chiral 2-hydroxy ketones by coupled biocatalytic alkene oxidation and C-C bond formation. J. Mol. Catal. B Enzym. 61, 111–116. doi: 10.1016/j.molcatb.2008.08.009
Lakowicz, J. R. (2006). “Fluorescence anisotropy” in Principles of fluorescence spectroscopy. ed. J. R. Lakowicz (New York: Plenum Press), 353–382.
Lal, M., Begum, T., Gogoi, R., Sarma, N., Munda, S., Pandey, S. K., et al. (2022). Anethole rich Clausena heptaphylla (ROXB.) Wight & Arn., essential oil pharmacology and genotoxic efficiencies. Sci. Rep. 12:9978. doi: 10.1038/s41598-022-13511-8
Lea, M. A., Guzman, Y., and Desbordes, C. (2016). Inhibition of growth by combined treatment with inhibitors of lactate dehydrogenase and either phenformin or inhibitors of 6-phosphofructo-2-kinase/fructose-2,6-bisphosphatase 3. Anticancer Res. 36, 1479–1488.
Lummiss, J. A. M., Oliveira, K. C., Pranckevicius, A. M. T., Santos, A. G., dos Santos, E. N., and Fogg, D. E. (2012). Chemical plants: high-value molecules from essential oils. J. Am. Chem. Soc. 134, 18889–18891. doi: 10.1021/ja310054d
Madrid, A., Espinoza, L., Pavéz, C., Carrasco, H., and Hidalgo, M. E. (2014). Antioxidant and toxicity activity in vitro of twelve safrole derivatives. J. Chil. Chem. Soc. 59, 2598–2601. doi: 10.4067/S0717-97072014000300015
Marycz, K., Grzesiak, J., Wrzeszcz, K., and Golonka, P. (2012). Adipose stem cell combined with plasma-based implant bone tissue differentiation in vitro and in a horse with a phalanx digitalis distalis fracture: a case report. Vet. Med. 57, 610–617. doi: 10.17221/6469-VETMED
Marycz, K., Kornicka, K., and Röcken, M. (2018). Static magnetic field (SMF) as a regulator of stem cell fate – new perspectives in regerative medicine arising from an underestimated tool. Stem Cells Rev Rep. 14, 185–792. doi: 10.1007/s12015-018-9847-4
Mecozzi, F., Dong, J. J., Saisaha, P., and Browne, W. R. (2017). Oxidation of vicinal diols to α-hydroxy ketones with H2O2 and a simple manganese catalyst. Eur. J. Org. Chem. 2017, 6919–6925. doi: 10.1002/ejoc.201701314
Moradi, J., Abbasipour, F., Zaringhalam, J., Maleki, B., Ziaee, N., Khodadoustan, A., et al. (2014). Anethole, a medicinal plant compound, decreases the production of pro-inflammatory TNF-α and IL-1β in a rat model of LPS-induced periodontitis. Iran. J. Pharm. Res. 13, 1319–1325.
Nair, V. A. (2020). 2-lodoxybenzoic acid: an oxidant for functional group transformations: (A-review). Orient. J. Chem. 36, 792–803. doi: 10.13005/ojc/360501
Newberne, P., Smith, R. L., Doull, J., Goodman, J. I., Munro, I. C., Portoghese, P. S., et al. (1999). The FEMA GRAS assessment of trans-anethole used as a flavouring substance. Food Chem. Toxicol. 7, 789–811. doi: 10.1016/S0278-6915(99)00037-X
Oeggl, R., Mabmann, T., Jupke, A., and Rother, D. (2018). Four atom efficient enzyme cascades for all 4-methoxyphenyl-1,2-propanediol isomers including product crystallization targeting high product concentrations and excellent E-factors. Sust. Chem. Eng. 6, 11819–11826. doi: 10.1021/acssuschemeng.8b02107
Padhy, I., Paul, P., Sharma, T., Banerjee, S., and Mondal, A. (2022). Molecular mechanisms of action of eugenol in cancer: recent trends and advancement. Life 12:1795. doi: 10.3390/life12111795
Pagano, M., and Faggio, C. (2015). The use of erythrocyte fragility to assess xenobiotic cytotoxicity. Cell Biochem. Funct. 33, 351–355. doi: 10.1002/cbf.3135
Pandit, K., Kaur, S., Kumar, A., Bhardwaj, R., and Kaur, S. (2020). Trans-anethole abrogates cell proliferation and induces apoptosis through the mitochondrial-mediated pathway in human osteosarcoma cells. Nutr. Cancer 73, 1727–1745. doi: 10.1080/01635581.2020.1803927
Peng, K., Chen, F., She, X., Yang, C., Cui, Y., and Pan, X. (2005). Selective oxidation of benzylic or allylic hydroxyl group of sec-1,1-diols. Tetrahedron Lett. 46, 1217–1220. doi: 10.1016/j.tetlet.2004.12.073
Pérez-Sánchez, M., Müller, C. R., and de Maria, P. D. (2013). Multistep oxidase-lyase reactions: synthesis of optically active 2-hydroxyketones by using biobased aliphatic alcohols. ChemCatChem 5, 2512–2516. doi: 10.1002/cctc.201300093
Petersen, M., Hans, J., and Matern, U. (2010). “Biosynthesis of Phenylpropanoids and related compounds” in Annual Plant Reviews Volume 40: Biochemistry of Plant Secondary Metabolism. ed. M. Wink (Oxford: Wiley-Blackwell), 182–257.
Pruchnik, H., Włoch, A., Bonarska-Kujawa, D., and Kleszczyńska, H. (2018). An In Vitro Study of the Effect of Cytotoxic Triorganotin Dimethylaminophenylazobenzoate Complexes on Red Blood Cells. J. Membr. Biol. 251, 735–745. doi: 10.1007/s00232-018-0051-x
Rajagopalan, A., Lara, M., and Kroutil, W. (2013). Oxidative alkene cleavage by chemical and enzymatic methods. Adv. Synth. Catal. 355, 3321–3335. doi: 10.1002/adsc.201300882
Siva, S., Li, C., Cui, H., and Lin, L. (2019). Encompassment of isoeugenol in 2-hydroxypropyl-β-cyclodextrin using ultrasonication: characterization, antioxidant and antibacterial activities. J. Mol. Liq. 296:111777. doi: 10.1016/j.molliq.2019.111777
Song, A., Park, Y., Kim, B., and Lee, S. G. (2020). Modulation of lipid metabolism by trans-anethole in hepatocytes. Molecules 25:4946. doi: 10.3390/molecules25214946
Song, X., Yin, Z., Ye, K., Wei, Q., Jia, R., Zhou, L., et al. (2014). Anti-hepatoma effect of safrole from Cinnamommum longepaniculatum leaf essential oil in vitro. Int. J. Clin. Exp. Pathol. 7, 2265–2272.
Tentori, F., Brenna, E., Ferrari, C., Gatti, F. G., Ghezzi, M. C., and Parmeggiani, F. (2021). Chemo-enzymatic oxidative cleavage of isosafrole for the synthesis of piperonal. React. Chem. Eng. 6:1591. doi: 10.1039/D1RE00173F
Thi Luu, T. X., To Lam, T, Ngoc Le, T., and Duus, F. (2009). Fast and green microwave-assisted conversion of essential oil allylbenzenes into the corresponding aldehydes via alkene isomerization and subsequent potassium permanganate promoted oxidative alkene group cleavage. Molecules 14, 3411–3424. doi: 10.3390/molecules14093411
Włoch, A., Stygar, D., Bahri, F., Bażanów, B., Kuropka, P., Chełmecka, E., et al. (2020). Antiproliferative, antimicrobial and antiviral activity of β-aryl-δ-iodo-γ-lactones, their effect on cellular oxidative stress markers and biological membranes. Biomol. Ther. 10, 1–21. doi: 10.3390/biom10121594
Keywords: biotransformation, fragrances, propenylbenzenes, oxidation, fungistatic activity, antioxidant activity, haemolytic activity, proliferative activity
Citation: Hernik D, Szczepańska E, Ghezzi MC, Brenna E, Włoch A, Pruchnik H, Mularczyk M, Marycz K, Olejniczak T and Boratyński F (2023) Chemo-enzymatic synthesis and biological activity evaluation of propenylbenzene derivatives. Front. Microbiol. 14:1223123. doi: 10.3389/fmicb.2023.1223123
Received: 15 May 2023; Accepted: 12 June 2023;
Published: 26 June 2023.
Edited by:
Deniz Yildirim, Çukurova University, TürkiyeReviewed by:
Ahmet Tülek, Iğdır Üniversitesi, TürkiyeCopyright © 2023 Hernik, Szczepańska, Ghezzi, Brenna, Włoch, Pruchnik, Mularczyk, Marycz, Olejniczak and Boratyński. This is an open-access article distributed under the terms of the Creative Commons Attribution License (CC BY). The use, distribution or reproduction in other forums is permitted, provided the original author(s) and the copyright owner(s) are credited and that the original publication in this journal is cited, in accordance with accepted academic practice. No use, distribution or reproduction is permitted which does not comply with these terms.
*Correspondence: Dawid Hernik, ZGF3aWQuaGVybmlrQHVwd3IuZWR1LnBs; Filip Boratyński, ZmlsaXAuYm9yYXR5bnNraUB1cHdyLmVkdS5wbA==
Disclaimer: All claims expressed in this article are solely those of the authors and do not necessarily represent those of their affiliated organizations, or those of the publisher, the editors and the reviewers. Any product that may be evaluated in this article or claim that may be made by its manufacturer is not guaranteed or endorsed by the publisher.
Research integrity at Frontiers
Learn more about the work of our research integrity team to safeguard the quality of each article we publish.