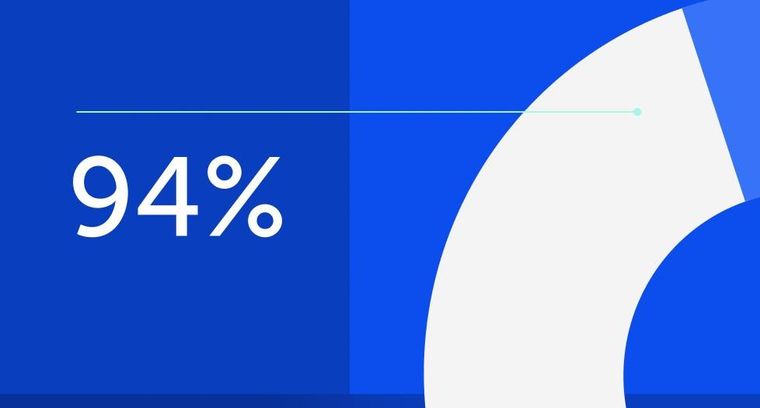
94% of researchers rate our articles as excellent or good
Learn more about the work of our research integrity team to safeguard the quality of each article we publish.
Find out more
ORIGINAL RESEARCH article
Front. Microbiol., 10 August 2023
Sec. Microbe and Virus Interactions with Plants
Volume 14 - 2023 | https://doi.org/10.3389/fmicb.2023.1216029
This article is part of the Research TopicEndophytic Fungi: Secondary Metabolites and Plant Biotic and Abiotic Stress ManagementView all 14 articles
Introduction: Alkanna tinctoria Tausch. is a medicinal plant well-known to produce important therapeutic compounds, such as alkannin/shikonin and their derivatives (A/Sd). It associates with arbuscular mycorrhizal fungi (AMF), which are known, amongst others beneficial effects, to modulate the plant secondary metabolites (SMs) biosynthesis. However, to the best of our knowledge, no study on the effects of AMF strains on the growth and production of A/Sd in A. tinctoria has been reported in the literature.
Methods: Here, three experiments were conducted. In Experiment 1, plants were associated with the GINCO strain Rhizophagus irregularis MUCL 41833 and, in Experiment 2, with two strains of GINCO (R. irregularis MUCL 41833 and Rhizophagus aggregatus MUCL 49408) and two native strains isolated from wild growing A. tinctoria (R. irregularis and Septoglomus viscosum) and were grown in a semi-hydroponic (S-H) cultivation system. Plants were harvested after 9 and 37 days in Experiment 1 and 9 days in Experiment 2. In Experiment 3, plants were associated with the two native AMF strains and with R. irregularis MUCL 41833 and were grown for 85 days in pots under greenhouse conditions. Quantification and identification of A/Sd were performed by HPLC-PDA and by HPLC-HRMS/MS, respectively. LePGT1, LePGT2, and GHQH genes involved in the A/Sd biosynthesis were analyzed through RT-qPCR.
Results: In Experiment 1, no significant differences were noticed in the production of A/Sd. Conversely, in Experiments 2 and 3, plants associated with the native AMF R. irregularis had the highest content of total A/Sd expressed as shikonin equivalent. In Experiment 1, a significantly higher relative expression of both LePGT1 and LePGT2 was observed in plants inoculated with R. irregularis MUCL 41833 compared with control plants after 37 days in the S-H cultivation system. Similarly, a significantly higher relative expression of LePGT2 in plants inoculated with R. irregularis MUCL 41833 was noticed after 9 versus 37 days in the S-H cultivation system. In Experiment 2, a significant lower relative expression of LePGT2 was observed in native AMF R. irregularis inoculated plants compared to the control.
Discussion: Overall, our study showed that the native R. irregularis strain increased A/Sd production in A. tinctoria regardless of the growing system used, further suggesting that the inoculation of native/best performing AMF is a promising method to improve the production of important SMs.
The hydroxynaphthoquinones (HNQs) of natural origin, and in particular the isohexenylnaphthazarins (IHNs), such as the chiral pair alkannin and shikonin (A/S), are lipophilic red pigments characterized by a wide spectrum of wound healing, antibacterial, anti-inflammatory, anticancer, and antithrombotic activities (Papageorgiou et al., 1999; Tappeiner et al., 2014). Monomeric A/S derivatives, mainly esters of the side chain hydroxyl group, have been found in the root periderm of several plants of the Boraginaceae family, including Alkanna tinctoria Tausch. This important medicinal plant, commonly known as alkanet or dyers’ bugloss/alkanet, is found across southern Europe, northern Africa, and southwestern Asia, with a central distribution in the Mediterranean region (Valdés, 2011). Preparations made with A. tinctoria roots are commonly used in traditional medicine to treat wounds, burns, and ulcers, while extracted A/S are employed as active ingredients in different marketed pharmaceutical formulations (e.g., Histoplastin Red® and HELIXDERM®) as strong wound healing medicines. Root extracts are also used as cosmetics, food additives or natural dyes for staining silk (Papageorgiou et al., 2008; Malik et al., 2016).
Today, nature remains the main source of commercial A/S and their derivatives (A/Sd), despite significant efforts to synthesize these compounds over the years (Papageorgiou et al., 2008). The only successful example of shikonin scaling up from cell cultures was with Lithospermum erythrorhizon Siebold & Zucc. (Boraginaceae) by Mitsui Petrochemical Industries Ltd. (now Mitsui Chemicals Inc., Tokyo, Japan) in 1984 (Yazaki, 2017). Few more attempts have been made with cell suspension cultures of Arnebia spp. (Boraginaceae) in stirred-tank (New Brunswick Scientific Company Inc., Edison, NJ, USA) and in air-lift bioreactors, but no further commercial successes have been reported (Gupta et al., 2014; Malik et al., 2016). For A. tinctoria, cell tissues and root cultures have been used, but the level of A/S production remained insufficient for commercial use (Urbanek et al., 1996; Gerardi et al., 1998). Yet, these important compounds remain mostly extracted from plants grown in the wild, with a risk of extinction as already documented for other Boraginaceae spp. (e.g., L. erythrorhizon in Japan, Alkanna sieheana Rech. Fil. and Alkanna orientalis L. Boiss. in Turkey). Indeed, A. tinctoria is considered as very rare in some European countries, such as Slovakia, Bulgaria, and Hungary (Yazaki, 2017; Yaman et al., 2019; Ahmad et al., 2021). Moreover, this plant is characterized by a very low seed germination rate and its cultivation under conventional agriculture practices is hampered by several constraints (e.g., time needed for A/S production, high costs of harvesting, and exposure to biotic/abiotic stresses) (Malik et al., 2016). For these reasons, in vitro and ex vitro cultivation protocols have been developed for the propagation and preservation of this plant (Cartabia et al., 2022). In addition, hydroponic cultivation of medicinal plants has attracted the attention of the scientific community and industrial sector, as it can effectively meet the nutrients needs of the plants under controlled/stable environmental conditions (Gontier et al., 2002; Sgherri et al., 2010). Interestingly, these cultivation systems are adapted to the growth of microorganisms such as arbuscular mycorrhizal fungi (AMF) (IJdo et al., 2011; Garcés-Ruiz et al., 2017). These obligate plant symbionts, belonging to the Glomeromycota phylum, provide their hosts with nutrients (especially N and P) in exchange for carbon and lipids (Smith and Read, 2008; Chen et al., 2018). A number of studies have reported their impact on the production of secondary metabolites (SMs) in leaves, roots or fruits/tubers of different crops used as food or for medicinal purposes (Zeng et al., 2013; Avio et al., 2018; Pandey et al., 2018; Kaur and Suseela, 2020; Zhao et al., 2022). For instance, artemisinin in leaves of Artemisia annua L. (Chaudhary et al., 2008), caffeic acid and rosmarinic acid in shoots of Ocimum basilicum L. (Toussaint et al., 2007), and triterpenes and phenolics in Dioscorea spp. (Lu et al., 2015) were increased in AMF-colonized plants. In addition, the AMF Rhizophagus irregularis MUCL 41833 was shown to modulate the primary and secondary metabolites (PMs and SMs, respectively) production of Anchusa officinalis L. (i.e., shoot and root tissues as well as exudates in the nutrient solution), another important Boraginaceae plant, under a semi-hydroponic (S-H) cultivation system (Cartabia et al., 2021). A similar study, conducted with A. officinalis associated with different AMF species (R. irregularis MUCL 41833, Rhizophagus intraradices MUCL 49410, Rhizophagus clarus MUCL 46238, and R. aggregatus MUCL 49408), demonstrated that specific symbiotic associations can affect the production of bioactive compounds differently in the same host (Tsiokanos et al., 2022).
Biosynthesis of A/Sd involves the phenylpropanoid and mevalonate pathways (Song et al., 2020). Several genes, responsible for encoding enzymes involved in the biosynthesis of shikonin, have been identified in Boraginaceae plant species (Wang et al., 2014; Wu et al., 2017; Takanashi et al., 2019). The p-hydroxybenzoate geranyltransferase (PGT) gene plays a key role in coding the enzyme catalyzing the coupling of p-hydroxybenzoic acid and geranyl diphosphate to produce m-geranyl-p-hydroxybenzoic acid, which is the first step in the formation of the basic carbon skeleton leading to A/Sd, and it has been cloned and characterized in cell cultures of L. erythrorhizon and Arnebia euchroma (Royle) Johnston (Yazaki et al., 2002; Singh et al., 2010). In addition, geranylhydroquinone 3″-hydroxylase (GHQH), an enzyme hydroxylating the isoprenoid side chain of geranylhydroquinone (GHQ), a well-known precursor of shikonin, has also been identified in L. erythrorhizon cell suspension cultures (Yamamoto et al., 2000). However, to our knowledge, no study has investigated the gene regulation of the biosynthesis of A/Sd in A. tinctoria, in particular in the presence of AMF.
In this study, the main objectives were to determine whether (1) AMF from international collection (i.e., GINCO) could enhance the production of A/Sd and whether this effect is more/less significant compared to AMF isolated from A. tinctoria grown in the wild; (2) AMF can modulate the expression of genes involved in the A/Sd biosynthetic pathway; (3) the production of A/Sd is affected similarly under semi-hydroponic and pot cultivation systems. To address these objectives, the quantification of A/Sd was performed through High-Performance Liquid Chromatography coupled with Photodiode Array (HPLC-PDA) detection and the related genes expression through Real-Time Quantitative Reverse Transcription PCR (RT-qPCR). Moreover, identification of the main A/Sd was performed using HPLC coupled with High-Resolution Mass Spectrometry detection (HPLC-HRMS/MS).
All used organic solvents [i.e., n-hexane 97%, methanol, trifluoroacetic acid (TFA), and acetonitrile (ACN) (VWR INTERNATIONAL, Leuven, Belgium)] were HPLC/LC-MS grade. Water was purified and demineralized with a Milli-Q system manufactured by Millipore (Bedford, MA, USA). Shikonin (purity >98%) was purchased from Cayman Chemical Company (Biomol GmbH, Hamburg, Germany) and used as internal standard.
For gene expression analysis (i.e., RNA extraction) all non-disposable materials (e.g., glass materials, mortars, and pestles) were first treated with RNase AWAY™ Surface Decontaminant (Thermo Fisher Scientific™, Belgium) and 0.1% diethylpyrocarbonate (DEPC)-treated sterile water. All the reagents were prepared with sterilized (121°C for 15 min) DEPC-treated water. Reagents used in RNA extraction were the following: extraction buffer [100 mM Tris–HCl (pH 8.0), 20 mM EDTA, 1.4 M NaCl, 2% CTAB (w/v) and 2% PVP (w/v)], 2% β-mercaptoethanol (v/v), 5 M NaCl, 1.2 M NaCl, 0.38 M trisodium citrate dihydrate, chloroform, isopropanol, and 70% ethanol (stored at −20°C).
Rhizophagus irregularis (Błaszk, Wubet, Renker and Buscot) C. Walker and A. Schüßler as [“irregulare”] MUCL 41833 and Rhizophagus aggregatus (N.C. Schenck & G.S. Sm.) C. Walker MUCL 49408 were supplied by the Glomeromycota in vitro collection (GINCO).1 Both AMF were mass-produced on Zea mays L. cv. ES Ballade (Euralis, Lescar, France) in 10 L plastic boxes containing sterilized (121°C for 15 min) lava (DCM, Grobbendonk, Belgium). Two other AMF strains (R. irregularis and Septoglomus viscosum) were isolated from A. tinctoria growing in a suburban pine forest, altitude 50 m of Northern Greece (Evaggelistria, Seih Sou, Thessaloniki, special collection permit obtained by the Institute of Plant Breeding and Genetic Resources, Hellenic Agricultural Organization Demeter – IPBGR, HAO Demeter). They were first trapped on Plantago lanceolata L. (Ecosem, Belgium) and Medicago truncatula Gaertn. (SARDI, Australia) to stimulate the production of numerous spores and then grown as monospores on P. lanceolata at the GINCO premises to obtain single species cultures (see section Isolation and mono-species culture of AMF in Supplementary material, Supplementary Figures 1–4, and Supplementary Table 1). They were finally mass-produced on Z. mays L. in 5 L plastic boxes containing the same sterilized lava substrate as above.
The four AMF strains were grown under greenhouse conditions at 25/18°C (day/night), a relative humidity (RH) of 38%, a photoperiod of 16 h day–1 and a photosynthetic photon flux (PPF) of 120 μmol m–2 s–1.
Alkanna tinctoria unrooted in vitro explants (International Plant Exchange Network – IPEN – accession number GR-1-BBGK-17,5975) were provide by IPBGR, HAO Demeter (Thessaloniki, Greece). The plants were proliferated on Murashige-Skoog (MS) basal medium enriched with plant growth regulators (PGRs) and rooted on Root Culture 1 (RC1) modified medium free of ammonium nitrate (Cartabia et al., 2022). The rooted A. tinctoria plants were further acclimatized following different steps resulting in an optimal plant survival rate (see section “Alkanna tinctoria acclimatization protocol” in Supplementary material and Supplementary Figure 5). The acclimatization protocol was applied for all the experiments in order to have plants of the same age and established under identical growth conditions (first acclimatization from 2nd November 2020 to 7th December 2020 and second from 1st February 2021 to 10th March 2021).
After the acclimatization phase, the plants were carefully removed from the substrate, gently washed under a stream of demineralized water, and transplanted into 1 L pots (11 × 11 × 12 cm) containing a sterilized (121°C for 15 min) substrate mixture (2 peatmoss/2 compost/1 perlite/1 quartz 0.4–0.8 mm/1 quartz 1–2 mm) (see Supplementary Figure 5). Total fresh weight (TFW) of the plants was evaluated before AMF inoculation.
In each experiment, plants were associated [i.e., the mycorrhizal (M) treatments] or not [i.e., the non-mycorrhizal (NM) treatments] with AMF. For the M plants, the substrate was half mixed with the AMF-inoculum substrate above, whereas for the NM treatments, the substrate was half mixed with the same AMF-inoculum substrate above but sterilized (121°C for 15 min). The plants were grown in the substrates for two months under greenhouse conditions set at 24/22°C (day/night), RH of 50%, photoperiod of 16 h day–1 and LED light (lumigrow) intensity of about 220 μmol m–2 s–1.
In Experiment 1, the objective was to evaluate the production of A/Sd and the expression of genes involved in the biosynthetic pathways of A/Sd in A. tinctoria roots colonized (later abbreviated for fluency as Mirr) or not (NM) with R. irregularis MUCL 41833, before (T0) and after 9 (T1) and 37 days (T2) of growth in the S-H cultivation system. These harvesting times were based on the study of Cartabia et al. (2021), conducted with Anchusa officinalis (another Boraginaceae). The authors made a kinetic study (before the transfer of the plants to the S-H cultivation system and after 9 and 30 days of growth in the S-H cultivation system) on the effect of R. irregularis MUCL 41833 on the metabolites profile of A. officinalis. Interesting results were obtained at these harvesting times, and therefore were considered in the present study.
Two-month-old Mirr and NM plants were gently removed from the pots above and their roots cleaned with demineralized water to eliminate substrate debris (see Supplementary Figure 5). Six plants belonging to the Mirr and NM treatments were randomly harvested, and AMF root colonization assessed before (T0) transfer to the S-H cultivation system (see section “2.7. Plants biomass and AMF root colonization”). Fourteen additional plants from the Mirr and NM treatments were transferred to the S-H cultivation system as detailed in Cartabia et al. (2021). The plants were placed in 500 ml plastic bottles (VWR INTERNATIONAL, Leuven, Belgium), cut at the base and covered with a 100 μm size pore nylon mesh (Prosep B.V.B.A., Zaventem, Belgium) glued on the top. The bottles (called containers thereafter) were used bottom-up, filled with 32 g of perlite (KNAUF GMBH, Dortmund, Germany), covered with a superficial layer of black lava rock (1–3 mm) and wrapped in aluminum foil to avoid algae development and inhibition of shikonin production (as shikonin is sensitive to light) (Yazaki, 2017). The containers were transferred randomly in holes made in flex foam supports and were maintained in the greenhouse set at the same conditions as described above. A 90% P-impoverished modified Hoagland solution (see Garcés-Ruiz et al., 2017; and Supplementary Table 2) lacking ammonium nitrate (NH4NO3) [as NH4+ is an inhibitor of shikonin production (Yazaki, 2017)] was used at two different concentrations as detailed in Cartabia et al. (2021). After 7 days of acclimatization, the circulatory system was started. Each container was connected to a 1 L glass bottle covered with aluminum foil containing Hoaglanddil100X solution. A 4.6 mm diameter black supply pipe (GARDENA®, Micro-Drip System, Ulm, Germany) connected a dropper cap fixed on the bottom of the plant container with the glass bottle, and another black pipe of the same diameter connected the glass bottle to the upper part of the plant container via a multichannel peristaltic pump (Gilson’s Minipuls Evolution, France). Once the circulation started, the nutrient solution was pumped from the glass bottle into the plant container and the liquid flowed back by gravity into the bottle. Before starting with the circulation, initial flushing was performed as described in Cartabia et al. (2021). Then, four successive circulations were performed at a velocity of 7.5 ml min–1 for different durations: 42 h at day 9 (T1), 8 h at day 17, 24, and 31, and finally 42 h at day 37 (T2).
In Experiment 2, the objective was to evaluate the production of A/Sd and the expression of genes involved in the biosynthetic pathways of A/Sd in A. tinctoria roots colonized (later abbreviated for fluency as Mirr, Maggreg, MRhiz, and MSept) or not (NM) with R. irregularis MUCL 41833, R. aggregatus MUCL 49408, the native R. irregularis and S. viscosum and grown for 9 days in the S-H cultivation system. This harvesting time was based on the study of Tsiokanos et al. (2022) where different AMF strains were associated to A. officinalis, and plants were harvested after 9 days of growth in the S-H cultivation system. Interesting results were obtained following this harvesting time and therefore considered in the present study. The same procedure as in Experiment 1 was applied. Briefly, after cleaning the roots system, 2-month-old plants (six per treatment) were transferred in the S-H cultivation system, and the containers were randomly placed in the holes made in the flex foam supports. Then, after the acclimatization period of 7 days, a regular circulation was initiated and maintained at 7.5 ml min–1 for 42 h.
In Experiment 3, the objective was to evaluate the production of A/Sd and the expression of genes involved in the biosynthetic pathways of A/Sd in A. tinctoria roots colonized (Mirr, MRhiz, and MSept) or not (NM) with R. irregularis MUCL 41833, the native R. irregularis and S. viscosum and growing for 85 days in pots under greenhouse conditions. In this Experiment, R. aggregatus MUCL 49408 was not considered due to inoculum limitation. Six plants per treatment were gently removed from the 1 L pots above and transplanted in 3 L pots (3 × 18.5 × 17 cm) containing a sterilized (2 × 121°C for 15 min) substrate mixture (2 peatmoss/2 compost/1 perlite/1 quartz 0.4–0.8 mm/1 quartz 1–2 mm). They received 200 ml Hoaglanddil200X solution and were randomly moved every week until harvest. The plants were grown under the same conditions as for the S-H cultivation system in Experiments 1 and 2.
For the three experiments, plants were harvested and biomass as well as AMF root colonization evaluated. In Experiment 1, plants were harvested before transfer to the S-H cultivation system (T0) and after 9 (T1) and 37 days (T2) corresponding to 57 and 94 days after AMF inoculation of the plants, respectively. In Experiment 2, they were harvested after 9 days (i.e., 79 days after AMF inoculation of the plants), and in Experiment 3, 85 days after AMF inoculation of the plants.
Whatever the experiment, shoots were separated from roots (cleaned under demineralized water and gently dried). Shoot fresh weigh (SFW) and roots fresh weight (RFW) were then measured. Root colonization was further evaluated by McGonigle et al. (1990). Roots (an approximate of 2 g fresh material) were cut into circa 1 cm length pieces and placed in 50-ml Falcon tubes. Twenty-five milliliter of KOH 10% was added to the roots before incubation at 70°C for 40 min. The KOH solution was then removed and replaced with H2O2 3.5% before incubation at 70°C for 5 min. The roots were then rinsed with demineralized water and HCl 1% was added for 1 min at room temperature. After discarding the solution, the roots were stained with ink 2% (Parker Blue Ink, United States) in HCl 1% (Walker, 2005) by placing the tubes at 70°C for 30 min. The roots were finally rinsed with demineralized water and kept in lactoglycerol (lactic acid/glycerol/distilled water, 1:1:1, v/v) until observation. For colonization assessment, the root fragments were placed on microscope slides and covered with 40 × 22 mm coverslips before observation under a bright field light microscope (Olympus BH2-RFCA, Japan) at ×10 magnification. Around 200 root intersections were observed for each plant in Experiments 1 and 2 and around 300 intersections for the plants in Experiment 3. The total colonization percentage (TC%) of roots (e.g., hyphae, arbuscules, and vesicles/spores), and arbuscules colonization percentage (AC%) was further calculated (McGonigle et al., 1990).
The remaining root system of each replicate was divided in two parts of around 5 g each for metabolites and gene expression analysis. Regarding the analysis of metabolites, roots were freeze-dried during 72 h and kept at −80°C before proceeding with A/Sd extraction (see section “2.8. Quantitative and qualitative analysis of A/Sd in A. tinctoria roots”). For analysis of gene expression, roots were transferred within 5–10 min after sampling into liquid nitrogen and kept at −80°C before proceeding with the RNA extraction (see section “2.9. Analysis of A/Sd target genes expression in A. tinctoria roots”).
The standard stock solution of shikonin was serially diluted in HPLC-grade methanol to obtain a range of concentrations from 0.05 to 0.8 mg ml–1 (i.e., stock solution of 1 mg ml–1 of shikonin was prepared and diluted in HPLC-grade methanol to obtain the calibration solutions of 0.8, 0.6, 0.4, 0.2, 0.1, and 0.05 mg ml–1). The calibration curve was made by plotting the average peak areas of three independent experiments versus the concentration of each analyte. The method was validated with three independent series of experiments based on total error and tolerance intervals (Hubert et al., 2003). Specificity was evaluated by analysis of an extract sample by HPLC-HRMS and comparison of MS1 signals of blank, shikonin standard, and A. tinctoria extract at 0.8 μg ml–1, respectively (see section “2.8.4. MS data treatment, organization, and dereplication”). Linear regression equation, response function, linearity (R2), precision, trueness, accuracy, limit of detection (LOD), limit of quantification (LOQ), and stability were provided (see section “HPLC quantification: methodology validation” in Supplementary material, Supplementary Figures 6, 7, and Supplementary Table 3).
For the three experiments, primary and secondary roots of A. tinctoria plants were freeze-dried separately and reduced into powder using liquid nitrogen, mortar and pestle. Fifty milligram of each root type was then mixed in glass test tube (labbox 10 ml neutral glass, TU04-160-100) closed with a plastic cap. Then, the total 100 mg of root material was subjected to a 20-min ultrasound-assisted extraction (two cycles), using n-hexane 97% (2 ml) at room temperature. The samples were finally centrifuged at 1,500 rpm for 10 min at 4°C and the supernatants of each cycle were combined and evaporated at room temperature. The extraction protocol was adapted from Bossard et al. (2022).
Prior to analysis, the plant extracts and nutrient solution residues were weighed and solubilized in methanol and were filtered through a 45-μm PTFE membrane (Whatman™, Maidstone, UK). Each sample was adjusted to the final concentration of 2 mg ml–1, using methanol LCMS-grade.
For the three experiments, the quantification of HNQ enantiomers (A/Sd) in their free form (not linked to sugars) was performed following the protocol of Bossard et al. (2022). For HPLC-PDA analysis, we used an Accela HPLC system (Thermo Fischer Scientific™, Bremen, Germany) coupled with a photodiode array (PDA) detector, an autosampler equipped with a conventional sample tray compartment with its cooler (set at 4°C), an injection system with a sample loop of 20 μl, and a quaternary pump, all piloted by ChromQuest software. The column used was an Alltech ALLTIME C8 250 × 4.6 mm, packed with 5 μm particles. Twenty microliter of sample was injected in full loop injection mode by the autosampler. The column was eluted at constant flow rate of 1 ml min–1 using a binary solvent system: solvent A, Milli-Q water 0.1% TFA and solvent B, ACN, isocratic mode (25% A: 75% B). Quantification analyses were conducted at a wavelength of 510 nm corresponding to the maximum absorption of A/Sd and their content was calculated according to the shikonin standard curve. The total shikonin equivalent production was calculated as the sum of peaks corresponding to A/Sd free forms (i.e., by integration of the different peaks identified as A/Sd-type compounds by their UV spectra) contained in the root powder, reported as mg g–1 root powder using the same shikonin standard curve (so not corresponding to an absolute quantification of each compound). Peaks were identified by comparing their retention times (RT) and UV spectra with the standard chromatogram of shikonin. The chromatographic profiles were in agreement with the chemical profile reported by Bossard et al. (2022), for A/Sd obtained from A. tinctoria roots. In total, 18 M and 18 NM root samples (6 NM and 6 Mirr plants for each time harvest-T0, T1, and T2) were considered in Experiment 1, 30 root samples (6 Mirr, 6 Maggreg, 6 MRhiz, 6 MSept, and 6 NM) in Experiment 2, and 24 root samples (6 Mirr, 6 MRhiz, 6 MSept, and 6 NM) in Experiment 3. All samples were analyzed in duplicates.
The HPLC-HRMS/MS analysis was performed at the end of Experiments 2 and 3 on an HPLC-PDA-HRMS system consisting of an Accela pump and PDA detector (Thermo Fisher Scientific™, Bremen, Germany) connected with a LTQ orbitrap XL mass spectrometer (Thermo Fisher Scientific™, Bremen, Germany). The instrument was controlled using a Thermo Fisher Scientific™ Xcalibur X software. The LC separation was done as reported above except for the solvent A: Milli-Q water 0.1% formic acid (FA). The chromatograms were recorded between 200 and 600 nm. HRMS analyses were realized in ESI positive and negative modes with the following inlet conditions for the positive mode: capillary temperature 250°C; sheath gas flow 10 a.u.; auxiliary gas flow 5 a.u. and sweep gas flow 5 a.u; ionization spray voltage 3 kV; capillary voltage of 15 V; tube lens voltage of 90 V. For negative mode, the only differences from the positive mode were the capillary voltage (−10 V) and tube lens voltage (−125 V). The data-dependent MS/MS events were performed on the three most intense ions detected in full scans MS. Peaks were tentatively identified by comparing their HRMS and MS/MS spectra with the literature data of A/Sd.
All HRMS run data (.RAW) files were treated using MZmine software suite version 2.5.3 (Pluskal et al., 2010). For mass detection at MS1 level, the noise level was set to 1.5 × 104 for positive mode and to 8.5 × 103 for negative mode. For MS2 detection, the noise level was set to 1. The ADAP chromatogram builder was used and set to a minimum group size of scans of 4, a minimum group intensity of 1.0 × 104, a minimum highest intensity of 1.0 × 104, and m/z tolerance of 8 ppm. The ADAP algorithm (wavelets) was used for chromatogram deconvolution. The intensity window signal to noise (S/N) was used as a S/N estimator with S/N ratio set at 10, a minimum feature height of 1.0 × 104, a coefficient area threshold of 25, a peak duration ranging from 0.02 to 0.8 min, and a RT wavelet range from 0.02 to 0.2 min. Isotopes were detected using the isotope peak grouper with a m/z tolerance of 8 ppm, a RT tolerance of 0.02 min (absolute), a maximum charge set at 1, and the representative isotope used was the most intense. Then, the aligned list peak was gap-filled with RT range of 0.05 min and m/z tolerance of 8 ppm. The resulting list was filtered using the peak list rows filter option to remove all the duplicates and all the features without MS2 spectrum associated.
A molecular network was constructed from the .mgf file exported from MZmine, using the online workflow on the GNPS website (Wang et al., 2016). The precursor ion mass tolerance was set to 0.02 Da with a MS/MS fragment ion tolerance of 0.02 Da. A network was then created where edges were filtered to have a cosine of 0.7 and more than 3 matched peaks. The spectra in the network were then searched against GNPS’s spectral libraries filtered under the same conditions as before. Putative identification was carried out comparing available MS/MS fragmentation patterns from the literature. Data visualization was achieved using Cytoscape 3.8.0 (Shannon et al., 2003). Peak area data from the .csv file obtained from MZmine was added to the network. Size nodes were set proportionally to the total area of each peak detected in both analyzed extracts.
Frozen roots of each replicate were ground in liquid nitrogen (−196°C) with a pestle and mortar. Total RNA extraction was done on 0.2 g root material using the protocol from Xu et al. (2010) slightly modified. Main differences were in the extraction buffer composition and in the last steps of the protocol, where the pellet was washed with 70% ethanol and the total RNA was dissolved in 55 μl DEPC-treated water. Further, the total RNA was treated with TURBO DNA-free™ Kit (Thermo Fisher Scientific™, Belgium), according to the manufacturer protocol. The RNA of all samples was loaded on 1.5% agarose gel, electrophoresed to separate RNA, stained with GelRed® (Biotium, United States), and visualized under UV light to assess the integrity of ribosomal bands. Moreover, concentration of each RNA sample was measured using NanoDrop-ND 1000 UV-vis Spectrophotometer (NanoDrop® Technologies, United States) and RNA purity estimated from the A260/A280 and A260/A230 absorbance ratios. Finally, a 1 μg aliquot of total RNA was used for the first-strand cDNA synthesis according to the protocol of the Transcriptor High Fidelity cDNA Synthesis Kit (Roche, Montreal, QC, Canada). For each RNA sample, a reaction without Transcriptor High Fidelity Reverse Transcriptase (Hifi RT) enzyme was performed as a control for contamination by genomic DNA.
Five biological replicates (i.e., means of relative genes expression) for M and NM treatments at each time harvest (T0, T1, and T2) were considered in Experiment 1, six biological replicates for treatments Mirr, Maggreg, MRhiz, MSept, and NM in Experiment 2, and six biological replicates for treatments Mirr, MRhiz, and NM and five for MSept in Experiment 3.
For all the experiments, tissue samples for A/Sd and molecular determinations were simultaneously collected at the same period of the day, between 9:00 and 11:00 a.m.
For RT-qPCR, the expression of three target genes: LePGT1, LePGT2, and GHQH involved in the shikonin (A/Sd) biosynthesis was analyzed (see section “Analysis of A/Sd target genes expression in A. tinctoria roots” in Supplementary material and Supplementary Table 4). Since no published genomic resources are available for A. tinctoria, a previously published primer for amplification of LePGT1 in Onosma paniculatum Bur. et Franch was used, while for PGT2 and GHQH new primer pairs were designed. Sequences of each gene (LePGT2 or GHQH) from two phylogenetically distant Boraginaceae species (L. erythrorhizon and A. euchroma) were obtained from NCBI, aligned, and primers generated from conserved regions (see section “Alignments used to design primers” in Supplementary material). The glyceraldehyde-3-phosphate dehydrogenase gene (GAPDH) was used as internal reference control. RT-qPCR was performed using a LightCycler® FastStart Essential DNA Green Master (Roche) in 10 μl volume of reaction formed as follows: 5 μl Master mix (or Mix SYBR 2x), 0.5 μl of each primer from the pair (10 μM), and 4 μl cDNA (dil 5x). The reaction was carried out in a Roche LightCycler® 96 System using the following parameters: 10 min at 95°C, followed by 40 (for housekeeping gene) and 50 (for target genes) cycles of denaturation (95°C, 10 s)/annealing (60°C housekeeping gene/56°C target genes, 15 s)/extension (72°C, 10 s), and finalized by a standard melting curve analysis (95°C). Reactions were performed in three replicates. Normalization was achieved for each experiment separately using the reference gene (i.e., GAPDH) and the “Pfaffl” method (Pfaffl, 2001).
For all the experiments, a one-way ANOVA followed by HSD Tukey post-hoc test (p < 0.05) was applied to discriminate between means for plant growth parameters (i.e., SFW, RFW, and TFW), AMF colonization (i.e., TC% and AC%), A/Sd content, and relative genes expression at different time points (Experiment 1) or different AMF treatments (Experiments 2 and 3). Moreover, in Experiment 1, differences between M and NM treatments were highlighted by pairwise comparison with Bonferroni correction (p < 0.05) at each harvesting time (T0, T1, and T2). For all parameters, normal distribution of residuals variance and normality was checked before analyses. Non-normal data were normalized by log10 transformation before analysis. Data analyses were performed by IBM SPSS Statistics for Windows, version 28 (IBM Corp., Armonk, N.Y., USA).
In Experiment 1, no significant differences between harvesting times (before plants transfer to the containers-T0, and after 9-T1 and 37-T2 days of growth in the S-H cultivation system) were noticed for SFW, RFW, and TFW of plants in the Mirr treatment and for SFW of plants in the NM treatment. Conversely, a significantly greater RFW was noticed at T1 and T2 as compared to T0, and greater TFW at T2 compared to T0 for plants in the NM treatment. Irrespective of the harvest time, no significant difference was reported between the SFW of plants in the Mirr and NM treatments, while at T2, the RFW and TFW of the plants in the NM treatment was significantly greater than that in the Mirr treatment. In the Mirr treatment, a significant decrease in TC% was observed at T2 compared to T0 and T1 and in AC% at T1 and T2 compared to T0 (Table 1). No root colonization was observed in plants of the NM treatment.
Table 1. Shoot, root and total fresh weights (SFW, RFW, and TFW, respectively), total colonization percentage (TC%), arbuscules colonization percentage (AC%), and total A/Sd expressed as shikonin equivalent content of A. tinctoria plants inoculated (Mirr) or not (NM) with R. irregularis MUCL 41833 before (T0) and after 9 (T1) and 37 (T2) days in the S-H cultivation system (Experiment 1), and of A. tinctoria plants inoculated (Mirr, Maggreg, MRhiz, MSept) or not (NM) with different AMF strains (two from GINCO – R. irregularis MUCL 41833 and R. aggregatus MUCL 49408, and two isolated from wild A. tinctoria – R. irregularis and S. viscosum) after 9 days in the S-H cultivation system (Experiment 2).
In Experiment 2, no significant differences were observed in SFW, RFW and TFW and in TC% between the different treatments (Mirr, Maggreg, MRhiz, MSept, and NM) after 9 days of growth of the plants in the S-H cultivation system. Conversely, a significant higher AC% was observed for the plants in the MRhiz treatment compared to those in the Maggreg treatment, while intermediate values were noticed in the plants of the MSept and Mirr treatments (Table 1). No root colonization was observed in plants of the NM treatment.
In Experiment 3, no significant differences were observed in SFW and RFW of plants between the different treatments (Mirr, MRhiz, MSept, and NM) after 85 days of growth in pots. Similarly, no significant differences were observed for TC% between the different treatments, while a significantly higher AC% was observed for the plants in the MSept treatment compared to those of the Mirr treatment. Plants in the MRhiz treatment had intermediate value (Table 2). No root colonization was observed in plants of the NM treatment.
Table 2. Shoot and root fresh weights (SFW and RFW, respectively), total colonization percentage (TC%), arbuscules colonization percentage (AC%), shikonin, and total A/Sd expressed as shikonin equivalent of A. tinctoria inoculated (Mirr, MRhiz, and MSept) or not (NM) with different AMF strains (one from GINCO – R. irregularis MUCL 41833, and two isolated from wild A. tinctoria – R. irregularis and S. viscosum) after 85 days in pots under greenhouse conditions (Experiment 3).
In Experiment 1, whatever the harvesting time (T0, T1, or T2) or treatment (Mirr or NM) no significant differences in content of A/Sd were noticed (Table 1).
In Experiment 2, a significant higher content of total A/Sd expressed as shikonin equivalent was observed in the plants of the MRhiz treatment compared to those in the Mirr, Magreeg, and NM treatments, while an intermediate value was observed for the plants in the MSept treatment (Table 1).
In Experiment 3, a significantly higher content of shikonin, whose values were nevertheless below the LOQ (Supplementary Table 2), and in total A/Sd expressed as shikonin equivalent, was reported in the roots of the plants in the MRhiz treatment compared to those in the Mirr and NM treatments, while an intermediate value was observed for the plants in the MSept treatment (Table 2).
In Experiment 1, whatever the time of evaluation (T0, T1, or T2) or treatment (Mirr or NM) no significant differences were noticed in gene expression of GHQH. Similarly, no significant differences between harvesting times were observed in expression of LePGT1 gene of the plants in the Mirr treatment and expression of LePGT1 and LePGT2 genes of the plants in the NM treatment. Conversely, a significantly higher expression of LePGT2 gene was observed at T2 compared to T1 for the plants in the Mirr treatment, while an intermediate gene expression was noticed at T0. A significant higher relative expression of both LePGT1 and LePGT2 genes was observed in the plants of the Mirr treatment compared to those in the NM treatment at T2 (Figure 1A).
Figure 1. (A) Graphical representation of RT-qPCR relative genes expression analysis of GHQH, LePGT1, and LePGT2 in A. tinctoria roots inoculated (Mirr) or not (NM) with R. irregularis MUCL 41833 before (T0) and after 9 (T1) and 37 (T2) days in the S-H cultivation system (Experiment 1); (B) graphical representation of RT-qPCR relative genes expression analysis of GHQH, LePGT1, and LePGT2 in A. tinctoria roots inoculated (Mirr, Maggreg, MRhiz, and MSept) or not (NM) with different AMF strains (two from GINCO – R. irregularis MUCL 41833 and R. aggregatus MUCL 49408, and two isolated from wild A. tinctoria – R. irregularis and S. viscosum) after 9 days in the S-H cultivation system (Experiment 2). Means followed by different lowercase letters within the same column are significantly different according to HSD Tukey post-hoc test (p < 0.05). Means followed by asterisk within the same column are significantly different according to pairwise comparison with Bonferroni correction (p < 0.05).
In Experiment 2, whatever the treatment (Mirr, Maggreg, MRhiz, MSept, and NM), no significant differences were observed in expression of GHQH and LePGT1 genes. A significant lower relative expression of LePGT2 was observed in the plants of the MRhiz treatment compared with the other treatments that did not differ among them (Figure 1B).
In Experiment 3, LePGT2 target gene was not considered due to contamination by the primer dimer. Whatever the treatment (Mirr, MRhiz, MSept, and NM), no significant differences were observed in expression of GHQH and LePGT1 genes (data not presented).
To better recognize the metabolites produced in A. tinctoria roots, the main chemical compounds were tentatively identified in Experiments 2 and 3 by performing a dereplication strategy based on HPLC-HRMS/MS analysis (Figure 2 and Tables 3, 4) and molecular network representation (Figures 3–5). These analyses were not performed in Experiment 1, since no significant differences were reported in the production of A/Sd.
Figure 2. Chromatographic profile of A. tinctoria root samples associated with Septoglomus viscosum detected under (A) HPLC-MS (BP+) and (B) HPLC-PDA (510 nm). Each peak defined by a number referred to a tentative identified compound, which has been described in Table 4.
Table 3. Putative identification of major chemical constituents in A. tinctoria extracts from Experiment 2 (positive mode ESI).
Table 4. Putative identification of major chemical constituents in A. tinctoria extracts (Experiment 3, negative mode, ESI).
Figure 3. Molecular network of A. tinctoria root extracts obtained in Experiment 2 in positive mode. (A) HNQ naphthoquinone’s enantiomers (A/Sd); (B) lipid amides. Clusters were built with a cosine of 0.7 with a minimum of 3 common ions. Size nodes are proportional to corresponding peak area.
Figure 4. Comparative molecular networking of shikonin derivatives cluster (different nodes) between A. tinctoria roots inoculated or not (control) with different AMF strains (two from GINCO – R. irregularis MUCL 41833 and R. aggregatus MUCL 49408, and two isolated from wild A. tinctoria – R. irregularis and S. viscosum) and growing for 9 days in the S-H cultivation system (Experiment 2).
Figure 5. Molecular network in A. tinctoria root extracts obtained in Experiment 3 in negative mode. Size nodes are proportional to corresponding peak area.
In Experiment 2, the HPLC-HRMS/MS analysis was performed in positive and negative modes. However, shikonin was not detected in any root sample analyzed. Dereplication analyses of shikonin derivatives were done on positive mode to compare our results with published data available in the literature (Bossard et al., 2022). The major detected compounds were organized in two distinctive clusters: A/Sd naphthoquinones (cluster A, Figures 3A and Table 3) and lipid amides derivatives (cluster B, Figure 3B and Table 3). In cluster A, the first eluted derivative was putatively identified as a methylshikonin isomer (1) which gave a protonated molecular ion [M + H]+ at m/z 303 and fragmented to m/z 285, 243, and 233, corresponding to the loss of a H2O molecule, a C2H4O2 and a C5H10 fragment from the alkyl chain. Similar fragmentation pattern was observed for compound 4 with a protonated dehydrated molecular ion [M + H-H2O]+, putatively identified as a second methylshikonin derivative. Similar loss was observed for compounds 3 and 6, putatively corresponding to methyl-1′-deoxyshikonin and anhydroalkannin at m/z of 287 and 271, respectively. Data also indicated that the major shikonin derivative produced by the plants under these conditions was anhydroalkannin (6) (Kyogoku et al., 1973; Bai and Jin, 1994). Moreover, a group of lipid amides were also putatively detected from the A. tinctoria roots samples and represented in cluster B. These amides derivatives were tentatively identified as palmitoleamide (7), linoleamide (8), oleamide (9), and stearamide (10) with protonated molecular ions [M + H]+ at m/z 254, 280, 282, and 284, presenting a loss of a fragment of m/z 17, corresponding to the loss of an ammoniac molecule (Divito et al., 2012). Other isomeric compounds were also detected by HPLC-HRMS/MS, but in the absence of their fragmentation patterns, putative identities were not proposed. No significant differences were noticed in the metabolites’ production profile of A. tinctoria roots associated or not with different AMF strains (Figure 4).
In Experiment 3, shikonin was detected only in negative mode and this result was corroborated by co-injection with shikonin standard solution (Table 4). Due to the important differences in the fragmentation spectra in positive mode of A/Sd, clusters of these compounds were not formed (Figure 5). In addition, the abovementioned shikonin derivatives isomers (1 and 6) were also observed as deprotonated molecular ion [M − H]– at m/z 301 with fragmentation signals at m/z 286 and 232 corresponding to the loss of a CH3 and C5H9 groups, for 1, and at m/z 269 with fragment loss at m/z 251 and 241, corresponding to the loss of a H2O and CO molecules, for 6. The deprotonated molecular ion [M − H]– of shikonin (11) was observed at m/z 287 with fragmentation signals at m/z 218 and 190, corresponding to the loss of C5H9 and the subsequent loss of a CO group (Liao et al., 2015). Moreover, an additional shikonin derivative was observed with a deprotonated molecular ion [M − H]– at m/z 329, putatively identified as acetylshikonin (13), with fragmentation loss at m/z 269, 251, and 241 corresponding to a McLafferty rearrangement (i.e., neutral loss of acetic acid), and the subsequent loss of a H2O and CO molecules (Liao et al., 2015). Three others unidentified compounds (12, 14, and 15) were detected under negative ionization mode with deprotonated molecular ion [M-H]– at m/z 797, 1111, and 1147. However, no clusters were observed between compounds 1, 6, 11, and 13 (shikonin derivatives) and for 12, 14, and 15 (unknown compounds) because they show a low degree of similarities in their fragmentation spectra (in positive ionization mode).
Slight differences in metabolites production were observed between Experiments 2 and 3, when samples were analyzed in the same ionization mode. Nevertheless, the most important difference between the two experiments was the detection of shikonin only in Experiment 3. Analyses performed in negative mode for root samples of Experiment 2 did not show the presence of shikonin. Similarly, in Experiment 3, no significant differences were observed in the metabolites’ production profile of A. tinctoria roots associated or not with different AMF strains, thus producing similar chromatographic profiles.
In the present study, two native AMF strains isolated from A. tinctoria roots and two strains from GINCO were tested and compared on the production of A/Sd and on the expression of genes involved in A/Sd biosynthesis in A. tinctoria plants grown either in a semi-hydroponic or in a pot cultivation system. In Experiment 1, conducted only with the GINCO strain R. irregularis MUCL 41833, no effect was observed on the production of A/Sd, while a significantly higher relative expression of LePGT2 was observed after 37 days compared to 9 days of growth in the S-H cultivation system. Furthermore, the relative expression of LePGT1 and LePGT2 genes were significantly higher in the plants colonized with this AMF compared to the non-mycorrhized ones. In Experiment 2, four AMF strains (two from GINCO: R. irregularis MUCL 41833 and R. aggregatus MUCL 49408 and two isolated from A. tinctoria: R. irregularis and S. viscosum) were associated to A. tinctoria in the S-H cultivation system. The native strain R. irregularis significantly increased the production of A/Sd in the roots of A. tinctoria, although this was not accompanied by an increased expression of genes involved in the A/Sd biosynthesis pathway. In Experiment 3, the two native strains (R. irregularis and S. viscosum) and the GINCO one (R. irregularis MUCL 41833) were associated to A. tinctoria in pots. The native strain R. irregularis was confirmed to significantly enhance the content of shikonin and total A/Sd in A. tinctoria roots. However, no significant increase in relative genes expression was observed.
Whatever the growth system (S-H or pot), an increase in biomass of A. tinctoria was observed in the presence as well as absence of AMF. No significant difference was observed in SFW between the plants in the M and NM treatments after 9 days in the S-H cultivation system (Experiments 1 and 2) or after 85 days in the pots cultivation system (Experiment 3). However, a significantly lower RFW and TFW was reported for the plants in the M treatment compared to those in the NM treatment after 37 days in the S-H cultivation system (Experiment 1), unlike the results obtained with A. officinalis colonized by R. irregularis MUCL 41833 in the same S-H cultivation system (Cartabia et al., 2021). These results are not surprising as it has often been reported that plant growth response to AMF inoculation can vary among AMF species and that the direction (e.g., increase or decrease in plant biomass) and magnitude of the response strongly depends on the combination of plant and AMF taxa (Klironomos, 2003).
Root colonization was observed in each AMF-inoculated plant. However, colonization measured at transfer and after 9 and 37 days in the S-H cultivation system (Experiment 1), decreased steadily, probably because of damages caused to the extraradical mycelium at transfer to the S-H cultivation system with no full recovery and development within the roots throughout the experiment (Cartabia et al., 2021). It is not excluded that this is also a reason for the lower plant biomass reported at the end of Experiment 1 compared to the control, due to an imbalance between the carbon resources transported from plant to the fungus in exchange for nutrients transported from fungus to plant. Interestingly, in both Experiments 2 and 3, the total root colonization was almost similar among the AMF strains, while the percentages of arbuscules were higher in presence of the native R. irregularis and S. viscosum strains, suggesting that they were better adapted to A. tinctoria than the GINCO strains. This has often been reported by comparing commercial strains with local strains; the latter being more prone to rapid and effective colonization (Chenchouni et al., 2020; Wu et al., 2021). Unfortunately, this was not translated into higher plant biomass, probably because of the same justification explained above.
The production of A/Sd was not impacted by the GINCO strain R. irregularis MUCL 41833 (Experiment 1), whereas a significant increase in production was observed with the native R. irregularis strain (Experiments 2 and 3) and an intermediate production between this strain and the GINCO strains with the other native strain S. viscosum (Experiment 2). Similarly, no significant effect was observed with the GINCO strains R. irregularis MUCL 41833 and R. aggregatus MUCL 49408 (Experiments 2 and 3). It cannot be ruled out that these results are related to the fungal genotypes, with the native ones being more prone to stimulate the production of A/Sd than the GINCO ones. The difference between the two native strains further suggests some degree of functional specialization. This is supported by recent studies revealing that various species/strains of AMF can induce different changes in the production of metabolites in the same plant species (Kapoor et al., 2002; Larose et al., 2002; Copetta et al., 2006; Khaosaad et al., 2006; Toussaint et al., 2007; Zubek et al., 2010). For example, Toussaint et al. (2007) showed that Funneliformis caledonium2 increased rosmarinic acid and caffeic acid production in O. basilicum, whereas Funneliformis mosseae only increased caffeic acid production. In another study, Jurkiewicz et al. (2010) showed that total phenolic acids accumulation was significantly higher in the roots of Arnica montana L. associated with AMF collected from particular plant’s natural stands (in Kurpie or in Karkonosze), while intermediate increase was observed with AMF isolated from other regions (i.e., R. intraradices UNIJAG PL24-1 and R. intraradices BEG 140 or a mixture composed of the above and of Funneliformis geosporum UNIJAG PL 12-2, Septoglomus constrictum 265-5 Walker and F. mosseae BEG 12). Finally, Frew (2020) reported that the inoculation with a mixture of four commercial species of AMF (Claroideoglomus etunicatum, Funneliformis coronatum, F. mosseae, and R. irregularis) had a greater effect on phenolic concentrations in Hordeum vulgare L. cv. “Hindmarsh” as compared to a single commercial inoculant (R. irregularis). Interestingly, the author also demonstrated that the effects of the commercial mixture was not different from the results obtained with a native multi-species AMF inoculant extracted from field soil. This suggested that a commercial AMF mixture provided little to no additional benefits (Frew, 2020). Conversely, several specialized compounds produced by plants may be synthesized as a chemical defense against the presence of AMF in the roots (Copetta et al., 2006). However, this defense mechanism would be likely to occur with AMF strains that have never been associated to the plant. Our native strains isolated from the wild A. tinctoria in their natural habitat are more adapted to the plant and are likely to have evolved as good associates. Natives R. irregularis and S. viscosum could be better adapted to the presence of A/Sd, which are characterized by different biological activities and by various effects on soil microorganisms (e.g., antifungal activities), and thus better interact with their host plants to regulate and enhance the production of these important therapeutic metabolites (Yan et al., 2019). As reported above, a significant higher AC% was observed with MRhiz and MSept treatments in Experiments 2 and 3, respectively. An increase in nutrients uptake via the arbuscules can lead to an enhanced production of precursor compounds, such as NADPH, ATP, acetyl-CoA (mevalonic acid pathway), and pyruvate glyceraldehyde and phosphate (methylerythritol-4-phosphate pathway) that are required for the biosynthesis of various SMs (e.g., terpenoids, phenolic, and alkaloids) (Kapoor et al., 2017). In our study, a higher AC% could have led to an increase in the precursor compounds essential to produce A/Sd. However, this was not confirmed in Experiment 3, where the higher AC% in MSept treatment was not accompanied by a higher production of A/Sd. If we parallel this to plant biomass, it is well known that the response of plants to inoculation with an AMF may vary depending on the AMF species as previously reported (Klironomos, 2003). It is not excluded that similar effect is observed on metabolites production, although this needs to be demonstrated.
At the end of Experiment 1, a significantly higher relative expression of LePGT1 and LePGT2 genes was observed in A. tinctoria associated with R. irregularis MUCL 41833 compared to the NM treatment. In addition, a significantly higher relative expression of LePGT2 was observed in the AMF-colonized plants at day 37 compared to day 9. However, these increases were not accompanied by an enhanced production of A/Sd. Conversely, in Experiment 2, a significant lower relative expression of LePGT2 was observed in plants associated with the native R. irregularis strain, compared with the other AMF and NM treatments, whereas a significantly higher production of A/Sd was observed with this strain. In both Experiments, no differences were reported for GHQH. In Experiment 3, no significant differences between the treatments were noted for all the genes tested (data not presented).
Although these results may seem surprising, similar outcomes were reported in the literature. For example, Wang et al. (2014) demonstrated that the application of methyl jasmonate (MeJA) on shikonin-deficient A. euchroma cell lines rapidly led to the overexpression of several genes involved in the biosynthesis of A/Sd, including PGT, but was not accompanied by a significant higher production of A/Sd. In another study, Ahmad et al. (2022b) showed a significantly higher expression of LePGT1, LePGT2, and LeGHQH1 in Lithospermum officinale L. roots treated with MeJA, but no higher accumulation of total A/Sd, after eight weeks. Conversely, the same authors (Ahmad et al., 2022a) demonstrated that the bacterium Chitinophaga sp. strain R-73072 significantly upregulated LePGT1 and LePGT2, and a cytochrome P450-LeCYP76B101 genes, resulting in a significant enhanced production of total A/Sd in L. erythrorhizon roots, after 2-3 weeks.
In our study, a few hypotheses can be advanced to explain why upregulation of PGTs was not followed by higher production of A/Sd and, conversely, why downregulation was followed by higher production of A/Sd. Firstly, it is not excluded that the duration of Experiment 1 (37 days) was too short to result in a significant increased production of A/Sd, or that the timing of plant harvesting in our experiments was not optimal (i.e., gene expression behavioral oscillations due to the plant circadian cycle) (Merrow et al., 2005). The PGTs regulate the first biosynthetic steps forming the basic carbon skeleton that leads to A/Sd, and therefore, it is possible that the resulting modulation of A/Sd production took longer after the upregulation of the relative expression of target PGT genes, as supported by Ahmad et al. (2022b), or that the genes oscillations were not timely targeted during our harvest times, as suggested by Wang et al. (2014) (Figure 6). Secondly, in a study by Andrade et al. (2013), a mismatch between alkaloid levels, another important SM group, and gene expression was reported in different Catharanthus roseus (L.) G. Don tissues. In this study, the AMF C. etunicatum had a greater influence (i.e., alkaloids production) in roots than in shoots, and a higher gene expression was reported in the older leaves of M plants as well as in the youngest leaves of NM plants (leaves were harvested at the same time) (Andrade et al., 2013). These results suggest a very precise phenological and spatial regulation process during alkaloid biosynthesis (Mahroug et al., 2007). Moreover, the reported influence of AMF on idioblast and laticifer density in C. roseus plants might have enhanced the expression of enzymes specifically located in these cells (St-Pierre et al., 1999). An increase in glandular trichome density upon mycorrhization was also linked with an enhanced concentration of another group of SMs, the terpenoids (Zhao et al., 2022). This is an interesting aspect that needs to be further verified. In fact, A/Sd compounds are sequestered as granules in the phospholipid layer and are accumulated in the apoplastic spaces, and they can be found in the cork layer of mature roots (Brigham et al., 1999; Singh et al., 2010; Tatsumi et al., 2016). A difference in A/Sd accumulation might have occurred (i.e., higher content in the primary roots as compared to the secondary roots) upon AMF colonization and might have not been fully displayed across A. tinctoria root samples used for our RT-qPCR analysis (i.e., no homogenous samples analyzed with mostly secondary roots utilized). Thirdly, the regulation and accumulation of SMs in plants is usually controlled by a complex network characterized by transcription factors (TFs), which promote or inhibit the expression of multiple genes involved in one or more biosynthetic pathways (Yang et al., 2012; Wu et al., 2021). Transcription factors can act alone or in combination with other TFs to modulate the expression of target genes, and also one TF can regulate the expression of multiple genes participating in one or more biosynthetic pathways (Pinson et al., 2009; Goossens et al., 2017; Hassani et al., 2020). In our study, different TFs might have regulated the target genes (PGTs and GHQH), yet the full landscape of the A/Sd biosynthesis pathway is not entirely understood, with co-expressed genes still to be identified (Suttiyut et al., 2022). Finally, even after a gene has been transcribed, its expression could still be regulated at various stages. Post transcriptional modifications changes might occur to a newly transcribed primary RNA transcript after transcription has occurred and prior to its translation into a protein.
Figure 6. An abridged scheme of the shikonin derivatives biosynthesis pathway. The genes that were investigated in this study are reported in bold font. Single arrows represent one step reaction, while double arrows represent multiple step reactions. Dashed arrows signify undefined steps or the enzymes have not been verified yet. HMGS, 3-hydroxy-3-methylglutaryl-CoA synthase; HMGR, 3-hydroxy-3-methylglutaryl-CoA reductase; MVD, mevalonate diphosphate decarboxylase; GDPS, geranyl diphosphate synthase; PAL, phenylalanine ammonia-lyase; C4H, cinnamic acid 4-hydroxylase; 4CL, 4-coumaroyl-CoA ligase; PGT, p-hydroxybenzoate geranyltransferase; GHQH, geranylhydroquinone 3″-hydroxylase.
A similar chemical composition was reported in A. tinctoria roots associated with the different AMF and non-colonized control plants in Experiments 2 (S-H cultivation system) and 3 (pot cultivation system) (Figures 4, 5) except for the detection of shikonin in Experiment 3 (Tables 3, 4).
The major compound detected in both experiments was putatively identified as anhydroalkannin (6). This compound is also a main product of shikonin biotransformation by several human intestinal bacteria in aerobic conditions and it was found to be less cytotoxic against a series of human tumor cell lines in vitro, in comparison with shikonin (Meselhy et al., 1994; Min et al., 2000). Methyl A/Sd isomers, as detected in our samples, were also identified from Lithospermum, Alkanna, and Onosma species. 1′-methyl shikonin was isolated and characterized from roots of L. erythrorhizon, exerting moderate antioxidant activity (Han et al., 2008). To the best of our knowledge, 5- or 8- O-methoxyshikonin derivatives have not yet been identified from natural sources. To better clarify the chemical composition of our samples, putative methylshikonin derivatives (1 and 4) must be purified and the methoxyl position elucidated in the structure of these isomers. In addition, in both experiments, four main lipid amides were tentatively identified as palmitoleamide (7), linoleamide (8), oleamide (9), and stearamide (10). Fatty acids amides are a group of nitrogen-containing, lipid-soluble fatty acid derivatives, which act against a variety of diseases such as cancer, bacterial infections, parasitic infection, inflammations, diabetes, and obesity (Kim et al., 2010; Tanvir et al., 2018). Further, it has been reported that fatty acid amides as oleamide from plant root exudates can participate in strong plant-microbe interactions, stimulating nitrogen metabolism in rhizospheric bacteria (Sun et al., 2016).
The data of chemical composition obtained from A. tinctoria growing in our cultivation systems cannot be accurately compared with data from literature (i.e., from nature, commercial samples, and/or from cell plant suspension). Indeed, the proportion and quantity of A/Sd varies depending on the level of stress and microorganisms present in the rhizosphere (Brigham et al., 1999). The influence of the cultivation systems on the production of these methoxy and anhydro A/Sd must be deeply studied to better understand their significance. Secondary metabolites’ production and compositional changes have a strong correlation and association with the environment, and thus synthesized only under specific growth conditions (Peñuelas and Llusià, 1997). Variations in an environmental factor, such as light, temperature, soil water, soil fertility, and salinity, may alter the plant metabolites content (Yang et al., 2018). Indeed, many chemical and physical factors have been found to inhibit shikonin production, such as NH4+, 2,4-dichlorophenoxyacetic acid (a synthetic auxin), low pH, temperature higher than 28°C, and light, especially blue light (Tatsumi et al., 2016). Skoneczny et al. (2017), using a metabolomic approach profiling hydroxynaphthoquinones (HNQs) and pyrrolizidine alkaloids (PAs), demonstrated the influence of high temperatures and water withholding on the accumulation of A/Sd in Echium plantagineum L. Abundance of HNQs, especially deoxyshikonin, shikonin, and dimethylacrylshikonin, rapidly increased in roots exposed to elevated temperatures. Water withholding initially increased NQ abundance, but prolonged drought resulted in reduced total PAs and HNQs (Skoneczny et al., 2017). In our study, A. tinctoria growing in the S-H cultivation system were kept in perlite, while a mixture of peatmoss, perlite, and quartz was used in the pots experiment. Perlite is a growing medium frequently used in hydroponic cultivation system since it has a high-water retention and provide the plants’ roots with strong anchor points for stability and strength. Moreover, in this system, plants received minerals by the circulating nutrient solution flowing directly through the plants’ container, while in the pots it was added at constant intervals and left to be completely absorbed by the plants. Therefore, it is possible that the temperature and the water/nutrient solution retention was higher in the conventional pots than in the plant containers of the S-H cultivation system. Moreover, shikonin and A/Sd might have better accumulated in the pots, while leached out in the S-H system. Finally, plant developmental stage qualitatively and quantitatively influences primary and secondary metabolism. A recent study by Csorba et al. (2022) reported this aspect as the most important driver influencing A. tinctoria metabolites content, revealing a peak content of A/Sd at the fruiting stage. In our study, plants were harvested during the vegetative growth (around five months old), and thus, might have played a role in the chemical profile reported.
For the first time, to the best of our knowledge, AMF isolated from wild-growing A. tinctoria were identified and applied under the cultivation systems described in this study. Native R. irregularis significantly increased A/Sd production in A. tinctoria roots, whatever the system used (S-H or pots), thus opening new perspectives toward the application of AMF in the production of these valuable therapeutic compounds in medicinal plants. A better adaptation of this indigenous strain toward its host was demonstrated, with higher arbuscules formation and production of A/Sd reported in A. tinctoria roots. This result suggests that the selection of the most effective AMF species (native or not; single or combinations of different AMF strains) remains a key point in studying the modulation/increase of SMs. However, the mechanisms behind AM symbiosis and their impact on the A/Sd biosynthetic pathways still need to be further clarified. Additionally, the conditions characterizing the conventional pots system seemed to be the optimal one in term of shikonin detection. Therefore, the application of the best growing conditions should be further investigated as well. Since shikonin was reported only in the pot system, the recovery of SMs can yet be conducted only in a destructive way (i.e., using the roots of A. tinctoria). For this reason, further studies applying S-H cultivation systems/innovative systems are required, especially for testing non-destructive ways of trapping the metabolites exudates by the roots in the circulating nutrient solution.
The datasets presented in this study can be found in online repositories. The names of the repository/repositories and accession number(s) can be found in the article/Supplementary material.
YZ: isolation and identification of AMF from A. tinctoria plants, experimental set up, data collection, analysis and interpretation, drafting the work, commentaries, corrections, final approval, and agreement with all aspects of the work. AC: A. tinctoria in vitro and ex vitro production, experimental setup, data collection, development of protocols for the analyses and interpretation, drafting the work, commentaries, corrections, final approval, and agreement with all aspects of the work. SO: data analysis and interpretation, draft corrections, final approval, and agreement with all aspects of the work. MG-R: contribution on strain identification and assembly of AMF phylogenetic tree. M-FH: data analysis and interpretation, final approval, and agreement with all aspects of the work. JQ-L and SD: substantial contributions to the conception and design of the experiments, interpretation of the data, draft corrections, final approval, and agreement with all aspects of the work. IL: contribution to the development of the experiment, data analysis and interpretation, draft correction, and final approval and agreement with all aspects of the work. All authors contributed to the article and approved the submitted version.
YZ and AC were financially supported by the Special Research Funds (FSR) of the Université Catholique de Louvain (Belgium).
We would like to thank the Université Catholique de Louvain (Belgium) for financial support via the Special Research Funds (FSR). We would also like to thank Dr. Muhammad Ahmad and Dr. rer. nat. Eva Maria Molin from the Center for Health and Bioresources, AIT Austrian Institute of Technology GmbH (Tulln, Austria), for the design of the primers applied in the RT-qPCR analysis, the Institute of Plant Breeding and Genetic Resources, Hellenic Agricultural Organization Demeter (IPBGR, HAO Demeter), in particular Dr. Virginia Sarropoulou and Dr. Katerina Grigoriadou, for the supply of the A. tinctoria in vitro explants, and Dr. Nikos Krigas for the collection and botanical identification of A. tinctoria plants. Moreover, we thank Stéphanie Huret and Céline Bivort (Technician at UCLouvain Earth and Life Institute – Applied Microbiology – BCCM/MUCL) for the help in the RNA extraction and genes expression analysis and molecular identification of AMF, respectively, and Catherine Rasse (Statistical Methodology and Computing Service/Louvain Institute of Data Analysis and Modeling in Economics and Statistics, SMCS/LIDAM, UCLouvain) for the support in the statistical analysis. Finally, MS data were obtained at the MASSMET platform of UCLouvain.
The authors declare that the research was conducted in the absence of any commercial or financial relationships that could be construed as a potential conflict of interest.
All claims expressed in this article are solely those of the authors and do not necessarily represent those of their affiliated organizations, or those of the publisher, the editors and the reviewers. Any product that may be evaluated in this article, or claim that may be made by its manufacturer, is not guaranteed or endorsed by the publisher.
The Supplementary Material for this article can be found online at: https://www.frontiersin.org/articles/10.3389/fmicb.2023.1216029/full#supplementary-material
Ahmad, M., Leroy, T., Krigas, N., Temsch, E. M., Weiss-Schneeweiss, H., Lexer, C., et al. (2021). Spatial and ecological drivers of genetic structure in Greek populations of Alkanna tinctoria (Boraginaceae), a polyploid medicinal herb. Front. Plant Sci. 12:706574. doi: 10.3389/fpls.2021.706574
Ahmad, M., Varela, A. A., Koletti, A. E., Rodić, N., Reichelt, M., Rödel, P., et al. (2022b). Dynamics of alkannin/shikonin biosynthesis in response to jasmonate and salicylic acid in Lithospermum officinale. Sci Rep. 12:17093. doi: 10.1038/s41598-022-21322-0
Ahmad, M., Varela, A. A., Koletti, A. E., Assimopoulou, A. N., Declerck, S., Schneider, C., et al. (2022a). Transcriptional dynamics of Chitinophaga sp. strain R-73072-mediated alkannin/shikonin biosynthesis in Lithospermum officinale. Front. Microbiol. 13:978021. doi: 10.3389/fmicb.2022.978021
Andrade, S. A. L., Malik, S., Sawaya, A. C. H. F., Bottcher, A., and Mazzafera, P. (2013). Association with arbuscular mycorrhizal fungi influences alkaloid synthesis and accumulation in Catharanthus roseus and Nicotiana tabacum plants. Acta Physiol Plant. 35, 867–880. doi: 10.1007/s11738-012-1130-8
Avio, L., Turrini, A., Giovannetti, M., and Sbrana, C. (2018). Designing the ideotype mycorrhizal symbionts for the production of healthy food. Front. Plant Sci. 9:1089. doi: 10.3389/fpls.2018.01089
Bai, G., and Jin, X. J. (1994). Chemical constituents of Lithospermum erythrorhizon. Chem. Res. Chin. Univ. 10, 263–265.
Bossard, E., Tsafantakis, N., Aligiannis, N., and Fokialakis, N. (2022). A development strategy of tailor-made natural deep eutectic solvents for the enhanced extraction of hydroxynaphthoquinones from Alkanna tinctoria roots. Planta Med. 88, 826–837. doi: 10.1055/a-1738-5648
Brigham, L. A., Michaels, P. J., and Flores, H. E. (1999). Cell-specific production and antimicrobial activity of naphthoquinones in roots of Lithospermum erythrorhizon. Plant Physiol. 119, 417–428. doi: 10.1104/pp.119.2.417
Cartabia, A., Sarropoulou, V., Grigoriadou, K., Maloupa, E., and Declerck, S. (2022). In vitro propagation of Alkanna tinctoria Tausch.: A medicinal plant of the Boraginaceae family with high pharmaceutical value. Ind. Crops Prod. 182:114860. doi: 10.1016/j.indcrop.2022.114860
Cartabia, A., Tsiokanos, E., Tsafantakis, N., Lalaymia, I., Termentzi, A., Miguel, M., et al. (2021). The arbuscular mycorrhizal fungus Rhizophagus irregularis MUCL 41833 modulates metabolites production of Anchusa officinalis L. under semi-hydroponic cultivation. Front. Plant Sci. 12:724352. doi: 10.3389/fpls.2021.724352
Chaudhary, V., Kapoor, R., and Bhatnagar, A. K. (2008). Effectiveness of two arbuscular mycorrhizal fungi on concentrations of essential oil and artemisinin in three accessions of Artemisia annua L. Appl. Soil Ecol. 40, 174–181. doi: 10.1016/j.apsoil.2008.04.003
Chen, M., Arato, M., Borghi, L., Nouri, E., and Reinhardt, D. (2018). Beneficial services of arbuscular mycorrhizal fungi – From ecology to application. Front. Plant Sci. 9:1270. doi: 10.3389/fpls.2018.01270
Chenchouni, H., Mekahlia, M. N., and Beddiar, A. (2020). Effect of inoculation with native and commercial arbuscular mycorrhizal fungi on growth and mycorrhizal colonization of olive (Olea europaea L.). Sci. Hortic. Vol. 261:108969. doi: 10.1016/j.scienta.2019.108969
Copetta, A., Lingua, G., and Berta, G. (2006). Effects of three AM fungi on growth, distribution of glandular hairs, and essential oil production in Ocimum basilicum L. var. Genovese. Mycorrhiza 16, 485–494. doi: 10.1007/s00572-006-0065-6
Csorba, C., Rodić, N., Zhao, Y., Antonielli, L., Brader, G., Vlachou, A., et al. (2022). Metabolite production in Alkanna tinctoria links plant development with the recruitment of individual members of microbiome thriving at the root-soil interface. mSystems 7, e451–e422. doi: 10.1128/msystems.00451-22
Divito, E. B., Davic, A. P., Johnson, M. E., and Cascio, M. (2012). Electrospray ionization and collision induced dissociation mass spectrometry of primary fatty acid amides. Anal. Chem. 84, 2388–2394. doi: 10.1021/ac203158u
Frew, A. (2020). Contrasting effects of commercial and native arbuscular mycorrhizal fungal inoculants on plant biomass allocation, nutrients and phenolics. Plants People Planet 3, 536–540. doi: 10.1002/ppp3.10128
Garcés-Ruiz, M., Calonne-Salmon, M., Plouznikoff, K., Misson, C., Navarrete-Mier, M., Cranenbrouck, S., et al. (2017). Dynamics of short-term phosphorus uptake by intact mycorrhizal and non-mycorrhizal maize plants grown in a circulatory semi-hydroponic cultivation system. Front. Plant Sci. 8:1471. doi: 10.3389/fpls.2017.01471
Gerardi, C., Mita, G., Grillo, E., Giovinazzo, G., Miceli, A., and De Leo, P. (1998). “Alkanna tinctoria T. (Alkanets): In vitro culture and the production of alkannin and other secondary metabolites,” in Medicinal and aromatic plants X, biotechnology in agriculture and forestry, ed. Y. P. S. Bajaj (Berlin: Springer Berlin Heidelberg), doi: 10.1007/978-3-642-58833-4_2
Gontier, E., Clément, A., Tran, T. L. M., Gravot, A., Lièvre, K., Guckert, A., et al. (2002). Hydroponic combined with natural or forced root permeabilization: A promising technique for plant secondary metabolite production. Plant Sci. 163, 723–732. doi: 10.1016/S0168-9452(02)00171-1
Goossens, J., Mertens, J., and Goossens, A. (2017). Role and functioning of bHLH transcription factors in jasmonate signalling. J. Exp. Bot. 68, 1333–1347. doi: 10.1093/jxb/erw440
Gupta, K., Garg, S., Singh, J., and Kumar, M. (2014). Enhanced production of napthoquinone metabolite (shikonin) from cell suspension culture of Arnebia sp. and its up-scaling through bioreactor. 3 Biotech 4, 263–273. doi: 10.1007/s13205-013-0149-x
Han, J., Weng, X. C., and Bi, K. (2008). Antioxidants from a Chinese medicinal herb – Lithospermum erythrorhizon. Food Chemistry 106, 2–10. doi: 10.1016/j.foodchem.2007.01.031
Hassani, D., Fu, X., Shen, Q., Khalid, M., Rose, J. K. C., and Tang, K. (2020). Parallel transcriptional regulation of artemisinin and flavonoid biosynthesis. Trends Plant Sci. 25, 466–476. doi: 10.1016/j.tplants.2020.01.001
Hubert, P., Nguyen-Huu, J. J., Boulanger, B., Chapuzet, E., Chiap, P., Cohen, N., et al. (2003). Validation des procédures analytiques quantitatives, Harmonisation des démarches. S.T.P. Pharma Pratiq. 13, 101–138.
IJdo, M., Cranenbrouck, S., and Declerck, S. (2011). Methods for large-scale production of AM fungi: Past, present, and future. Mycorrhiza 21, 1–16. doi: 10.1007/s00572-010-0337-z
Jurkiewicz, A., Ryszka, P., Anielska, T., Waligórski, P., Białońska, D., Góralska, K., et al. (2010). Optimization of culture conditions of Arnica montana L.: Effects of mycorrhizal fungi and competing plants. Mycorrhiza 20, 293–306. doi: 10.1007/s00572-009-0280-z
Kapoor, R., Anand, G., Gupta, P., and Mandal, S. (2017). Insight into the mechanisms of enhanced production of valuable terpenoids by arbuscular mycorrhiza. Phytochem Rev. 16, 677–692. doi: 10.1007/s11101-016-9486-9
Kapoor, R., Giri, B., and Mukerji, K. G. (2002). Glomus macrocarpum: A potential bioinoculant to improve essential oil quality and concentration in Dill (Anethum graveolens L.) and Carum (Trachyspermum ammi (Linn.) Sprague). World J. Microbiol. Biotechnol. 18, 459–463. doi: 10.1023/A:1015522100497
Kaur, S., and Suseela, V. (2020). Unraveling arbuscular mycorrhiza-induced changes in plant primary and secondary metabolome. Metabolites 10:335. doi: 10.3390/metabo10080335
Khaosaad, T., Vierheilig, H., Nell, M., Zitterl-Eglseer, K., and Novak, J. (2006). Arbuscular mycorrhiza alter the concentration of essential oils in oregano (Origanum sp., Lamiaceae). Mycorrhiza 16, 443–446. doi: 10.1007/s00572-006-0062-9
Kim, K. H., Choi, S. U., Son, M. W., and Lee, K. R. (2010). Two new phenolic amides from the seeds of pharbitis nil. Chem. Pharm Bull. 58, 1532–1535. doi: 10.1248/cpb.58.1532
Klironomos, J. (2003). Variation in plant response to native and exotic arbuscular mycorrhizal fungi. Ecology. 84, 2292–2301. doi: 10.1890/02-0413
Kyogoku, K., Terayama, H., Tachi, Y., Suzuki, T., and Komatsu, M. (1973). Studies on the constituents of “shikon”. I. Structure of three new shikonin derivatives and isolation of anhydroalkanin. Shoyakugaku Zasshi. 27, 31–36.
Larose, G., Chênevert, R., Moutoglis, P., Gagné, S., Piché, Y., and Vierheilig, H. (2002). Flavonoid levels in roots of Medicago sativa are modulated by the developmental stage of the symbiosis and the root colonizing arbuscular mycorrhizal fungus. J. Plant Physiol. 159, 1329–1339. doi: 10.1078/0176-1617-00896
Liao, M., Li, A., Chen, C., Ouyang, H., Zhang, Y., Xu, Y., et al. (2015). Systematic identification of shikonins and shikonofurans in medicinal Zicao species using ultra-high performance liquid chromatography quadrupole time of flight tandem mass spectrometry combined with a data mining strategy. J. Chromatogr. A. 1425, 158–172. doi: 10.1016/j.chroma.2015.11.028
Lu, F. C., Lee, C. Y., and Wang, C. L. (2015). The influence of arbuscular mycorrhizal fungi inoculation on yam (Dioscorea spp.) tuber weights and secondary metabolite content. PeerJ 3, e1266. doi: 10.7717/peerj.1266
Mahroug, S., Burlat, V., and St-Pierre, B. (2007). Cellular and sub-cellular organisation of the monoterpenoid indole alkaloid pathway in Catharanthus roseus. Phytochem. Rev. 6, 363–381. doi: 10.1007/s11101-006-9017-1
Malik, S., Bhushan, S., Sharma, M., and Ahuja, P. S. (2016). Biotechnological approaches to the production of shikonins: A critical review with recent updates. Crit. Rev. Biotechnol. 36, 327–340. doi: 10.3109/07388551.2014.961003
McGonigle, T. P., Miller, M. H., Evans, D. G., Fairchild, G. L., and Swan, J. A. (1990). A new method which gives an objective measure of colonization of roots by vesicular-arbuscular mycorrhizal fungi. New Phytol. 115, 495–501. doi: 10.1111/j.1469-8137.1990.tb00476.x
Merrow, M., Spoelstra, K., and Roenneberg, T. (2005). The circadian cycle: Daily rhythms from behaviour to genes: First in the cycles review series. EMBO Rep. 6, 930–935. doi: 10.1038/sj.embor.7400541
Meselhy, R. M., Shigetoshi, K., Koji, T., Masao, H., and Tsuneo, N. (1994). Biotransformation of shikonin by human intestinal bacteria. Tetrahedron 50, 3081–3098. doi: 10.1016/S0040-4020(01)81108-X
Min, B. S., Hattori, M., Kim, H. M., and Kim, Y. H. (2000). Cytotoxicity of shikonin metabolites with biotransformation of human intestinal bacteria. J. Microbial. Biotechnol. 10, 514–517.
Pandey, D. K., Kaur, P., and Dey, A. (2018). “Arbuscular mycorrhizal fungi: Effects on secondary metabolite production in medicinal plants,” in Fungi and their role in sustainable development: Current perspectives, eds P. Gehlot and J. Singh (Singapore: Springer Singapore), doi: 10.1007/978-981-13-0393-7_28
Papageorgiou, V., Assimopoulou, A., and Ballis, A. (2008). Alkannins and shikonins: A new class of wound healing agents. CMC 15, 3248–3267. doi: 10.2174/092986708786848532
Papageorgiou, V. P., Assimopoulou, A. N., Couladouros, E. A., Hepworth, D., and Nicolaou, K. C. (1999). The chemistry and biology of alkannin, shikonin, and related naphthazarin natural products. Angew Chem. Int. Ed. Engl. 38, 270–301.
Peñuelas, J., and Llusià, J. (1997). Effects of carbon dioxide, water supply, and seasonally on terpene content and emission by Rosmarinus officinalis. J. Chem. Ecol. 23, 979–993. doi: 10.1023/B:JOEC.0000006383.29650.d7
Pfaffl, M. W. (2001). A new mathematical model for relative quantification in real-time RT-PCR. Nucleic Acids Res. 29, 45e–445e. doi: 10.1093/nar/29.9.e45
Pinson, B., Vaur, S., Sagot, I., Coulpier, F., Lemoine, S., and Daignan-Fornier, B. (2009). Metabolic intermediates selectively stimulate transcription factor interaction and modulate phosphate and purine pathways. Genes Dev. 23, 1399–1407. doi: 10.1101/gad.521809
Pluskal, T., Castillo, S., Villar-Briones, A., and Oresic, M. (2010). MZmine 2: Modular framework for processing, visualizing, and analyzing mass spectrometry-based molecular profile data. BMC Bioinform. 11:395. doi: 10.1186/1471-2105-11-395
Sgherri, C., Cecconami, S., Pinzino, C., Navari-Izzo, F., and Izzo, R. (2010). Levels of antioxidants and nutraceuticals in basil grown in hydroponics and soil. Food Chem. 123, 416–422. doi: 10.1016/j.foodchem.2010.04.058
Shannon, P., Markiel, A., Ozier, O., Baliga, N. S., Wang, J. T., Ramage, D., et al. (2003). Cytoscape: A software environment for integrated models of biomolecular interaction networks. Genome Res. 13, 2498–2504. doi: 10.1101/gr.1239303
Singh, R. S., Gara, R. K., Bhardwaj, P. K., Kaachra, A., Malik, S., Kumar, R., et al. (2010). Expression of 3-hydroxy-3-methylglutaryl-CoA reductase, p-hydroxybenzoate-m-geranyltransferase and genes of phenylpropanoid pathway exhibits positive correlation with shikonins content in arnebia [Arnebia euchroma (Royle) Johnston]. BMC Mol. Biol. 11:88. doi: 10.1186/1471-2199-11-88
Skoneczny, D., Weston, P. A., Zhu, X., Gurr, G. M., Callaway, R. M., Barrow, R. A., et al. (2017). Metabolic profiling and identification of shikonins in root periderm of two invasive Echium spp. weeds in Australia. Molecules 22:330. doi: 10.3390/molecules22020330
Smith, S. E., and Read, D. (2008). Mineral nutrition, toxic element accumulation and water relations of arbuscular mycorrhizal plants. Amsterdam: Elsevier, doi: 10.1016/B978-012370526-6.50007-6
Song, W., Zhuang, Y., and Liu, T. (2020). Potential role of two cytochrome P450s obtained from Lithospermum erythrorhizon in catalyzing the oxidation of geranylhydroquinone during Shikonin biosynthesis. Phytochemistry 175:112375. doi: 10.1016/j.phytochem.2020.112375
St-Pierre, B., Vazquez-Flota, F. A., and De Luca, V. (1999). Multicellular compartmentation of Catharanthus roseus alkaloid biosynthesis predicts intercellular translocation of a pathway intermediate. Plant Cell 11, 887–900. doi: 10.1105/tpc.11.5.887
Sun, L., Lu, Y., Kronzucker, H. J., and Shi, W. (2016). Quantification and enzyme targets of fatty acid amides from duckweed root exudates involved in the stimulation of denitrification. J. Plant Physiol. 198, 81–88. doi: 10.1016/j.jplph.2016.04.010
Suttiyut, T., Auber, R. P., Ghaste, M., Kane, C. N., McAdam, S. A. M., Wisecaver, J. H., et al. (2022). Integrative analysis of the shikonin metabolic network identifies new gene connections and reveals evolutionary insight into shikonin biosynthesis. Hortic. Res. 9:uhab087. doi: 10.1093/hr/uhab087
Takanashi, K., Nakagawa, Y., Aburaya, S., Kaminade, K., Aoki, W., Saida-Munakata, Y., et al. (2019). Comparative proteomic analysis of Lithospermum erythrorhizon reveals regulation of a variety of metabolic enzymes leading to comprehensive understanding of the shikonin biosynthetic Pathway. Plant Cell Physiol. 60, 19–28. doi: 10.1093/pcp/pcy183
Tanvir, R., Javeed, A., and Rehman, Y. (2018). Fatty acids and their amide derivatives from endophytes: New therapeutic possibilities from a hidden source. FEMS Microbiol. Lett. 365:fny114. doi: 10.1093/femsle/fny114
Tappeiner, J., Vasiliou, A., Ganzera, M., Fessas, D., Stuppner, H., Papageorgiou, V. P., et al. (2014). Quantitative determination of alkannins and shikonins in endemic Mediterranean Alkanna species: Quantitative determination of alkannins and shikonins. Biomed. Chromatogr. 28, 923–933. doi: 10.1002/bmc.3096
Tatsumi, K., Yano, M., Kaminade, K., Sugiyama, A., Sato, M., Toyooka, K., et al. (2016). Characterization of shikonin derivative secretion in Lithospermum erythrorhizon hairy roots as a model of lipid-soluble metabolite secretion from plants. Front. Plant Sci. 7:1066. doi: 10.3389/fpls.2016.01066
Toussaint, J.-P., Smith, F. A., and Smith, S. E. (2007). Arbuscular mycorrhizal fungi can induce the production of phytochemicals in sweet basil irrespective of phosphorus nutrition. Mycorrhiza 17, 291–297. doi: 10.1007/s00572-006-0104-3
Tsiokanos, E., Cartabia, A., Tsafantakis, N., Lalaymia, I., Termentzi, A., Miguel, M., et al. (2022). The metabolic profile of Anchusa officinalis L. differs according to its associated arbuscular mycorrhizal fungi. Metabolites 12:573. doi: 10.3390/metabo12070573
Urbanek, H., Bergier, K., Saniewski, M., and Patykowski, J. (1996). Effect of jasmonates and exogenous polysaccharides on production of alkannin pigments in suspension cultures of Alkanna tinctoria. Plant Cell Rep. 15, 637–641. doi: 10.1007/BF00232468
Valdés, B. (2011). Boraginaceae in Euro+Med plantbase: The information resource for Euro-Mediterranean plant diversity. Poltava: EuroPlus.
Walker, C. (2005). A simple blue staining technique for arbuscular mycorrhizal and other root-inhabiting fungi. Inoculum 56, 68–69.
Wang, M., Carver, J. J., Phelan, V. V., Sanchez, L. M., Garg, N., Peng, Y., et al. (2016). Sharing and community curation of mass spectrometry data with global natural products social molecular networking. Nat. Biotechnol. 34, 828–837. doi: 10.1038/nbt.3597
Wang, S., Guo, L. P., Xie, T., Yang, J., Tang, J. F., Li, X., et al. (2014). Different secondary metabolic responses to MeJA treatment in shikonin-proficient and shikonin-deficient cell lines from Arnebia euchroma (Royle) Johnst. Plant Cell Tiss. Organ. Cult. 119, 587–598. doi: 10.1007/s11240-014-0558-5
Wu, F. Y., Tang, C. Y., Guo, Y. M., Bian, Z. W., Fu, J. Y., Lu, G. H., et al. (2017). Transcriptome analysis explores genes related to shikonin biosynthesis in Lithospermeae plants and provides insights into boraginales’ evolutionary history. Sci. Rep. 7:4477. doi: 10.1038/s41598-017-04750-1
Wu, Y. H., Wang, H., Liu, M., Li, B., Chen, X., Ma, Y. T., et al. (2021). Effects of native arbuscular mycorrhizae isolated on root biomass and secondary metabolites of Salvia miltiorrhiza Bge. Front. Plant Sci. 12:617892. doi: 10.3389/fpls.2021.617892
Xu, J., Aileni, M., Abbagani, S., and Zhang, P. (2010). A reliable and efficient method for total RNA isolation from various members of spurge family (Euphorbiaceae). Phytochem. Anal. 21, 395–398. doi: 10.1002/pca.1205
Yamamoto, H., Inoue, K., Li, S. M., and Heide, L. (2000). Geranylhydroquinone 3”-hydroxylase, a cytochrome P-450 monooxygenase from Lithospermum erythrorhizon cell suspension cultures. Planta 210, 312–317. doi: 10.1007/PL00008139
Yaman, C., Uranbey, S., Ahmed, H. A., Ozcan, S., Tugay, O., and Basalma, D. (2019). Callus induction and regeneration of Alkanna orientalis var. orientalis and A. sieheana. Bangladesh J. Bot. 48, 633–640. doi: 10.3329/bjb.v48i3.47941
Yan, Y., Tan, F., Miao, H., Wang, H., and Cao, Y. (2019). Effect of shikonin against Candida albicans biofilms. Front Microbiol. 10:1085. doi: 10.3389/fmicb.2019.01085
Yang, C. Q., Fang, X., Wu, X. M., Mao, Y. B., Wang, L. J., and Chen, X. Y. (2012). Transcriptional regulation of plant secondary metabolism. F. J. Integr. Plant Biol. 54, 703–712. doi: 10.1111/j.1744-7909.2012.01161.x
Yang, L., Wen, K. S., Ruan, X., Zhao, Y. X., Wei, F., and Wang, Q. (2018). Response of plant secondary metabolites to environmental factors. Molecules 23, 762. doi: 10.3390/molecules23040762
Yazaki, K. (2017). Lithospermum erythrorhizon cell cultures: Present and future aspects. Plant Biotechnol. 34, 131–142. doi: 10.5511/plantbiotechnology.17.0823a
Yazaki, K., Kunihisa, M., Fujisaki, T., and Sato, F. (2002). Geranyl diphosphate:4-Hydroxybenzoate geranyltransferase from Lithospermum erythrorhizon. J. Biol. Chem. 277, 6240–6246. doi: 10.1074/jbc.M106387200
Zeng, Y., Guo, L.-P., Chen, B.-D., Hao, Z.-P., Wang, J.-Y., Huang, L.-Q., et al. (2013). Arbuscular mycorrhizal symbiosis and active ingredients of medicinal plants: Current research status and prospectives. Mycorrhiza 23, 253–265. doi: 10.1007/s00572-013-0484-0
Zhao, Y., Cartabia, A., Lalaymia, I., and Declerck, S. (2022). Arbuscular mycorrhizal fungi and production of secondary metabolites in medicinal plants. Mycorrhiza 32, 221–256. doi: 10.1007/s00572-022-01079-0
Keywords: arbuscular mycorrhizal fungi, Alkanna tinctoria, semi-hydroponic cultivation system, alkannin/shikonin derivatives, native strains
Citation: Zhao Y, Cartabia A, Garcés-Ruiz M, Herent M-F, Quetin-Leclercq J, Ortiz S, Declerck S and Lalaymia I (2023) Arbuscular mycorrhizal fungi impact the production of alkannin/shikonin and their derivatives in Alkanna tinctoria Tausch. grown in semi-hydroponic and pot cultivation systems. Front. Microbiol. 14:1216029. doi: 10.3389/fmicb.2023.1216029
Received: 03 May 2023; Accepted: 24 July 2023;
Published: 10 August 2023.
Edited by:
Mina Salehi, Tarbiat Modares University, IranReviewed by:
Siamak Farhadi, Tarbiat Modares University, IranCopyright © 2023 Zhao, Cartabia, Garcés-Ruiz, Herent, Quetin-Leclercq, Ortiz, Declerck and Lalaymia. This is an open-access article distributed under the terms of the Creative Commons Attribution License (CC BY). The use, distribution or reproduction in other forums is permitted, provided the original author(s) and the copyright owner(s) are credited and that the original publication in this journal is cited, in accordance with accepted academic practice. No use, distribution or reproduction is permitted which does not comply with these terms.
*Correspondence: Stéphane Declerck, c3RlcGhhbi5kZWNsZXJja0B1Y2xvdXZhaW4uYmU=
†These authors have contributed equally to this work and share first authorship
Disclaimer: All claims expressed in this article are solely those of the authors and do not necessarily represent those of their affiliated organizations, or those of the publisher, the editors and the reviewers. Any product that may be evaluated in this article or claim that may be made by its manufacturer is not guaranteed or endorsed by the publisher.
Research integrity at Frontiers
Learn more about the work of our research integrity team to safeguard the quality of each article we publish.