- Department of Pediatrics, Xijing Hospital, The Fourth Military Medical University, Xi’an, Shaanxi, China
Introduction: Previous studies have found that unique patterns of gut microbial colonization in infancy associated with the development of allergic diseases. However, there is no research on the gut microbiota characteristics of AR children in Chinese Mainland.
Objective: To investigate the changes of gut microbial of AR children in Chinese Mainland and evaluate the correlation between gut microbial and clinical indexes.
Methods: In this clinical study, fecal samples from 24 AR children and 25 healthy control children (HCs) were comparative via next generation sequencing of the V3-V4 regions of the 16S rRNA gene. Analyzed the relationship between clinical features and gut microbial using Spearman correlation.
Results: Compared to HCs, AR children showed significant decreases in Shannon index and significant increases in Simpson index at both the family and genera levels (all p < 0.05). In terms of bacterial composition, at the phylum level, AR children had higher abundance of Bacteroidetes than that in the HCs group (p < 0.05) and were significantly positively correlated with TNSS (p < 0.05). At the family level, AR children had higher abundance of Prevotellaceae and Enterobacteriaceae higher than that in the HCs group (all p < 0.05) and had a significantly positive correlation with TNSS, eosinophils (EOS) and total immunoglobulin E (tIgE) (all p < 0.05). At the genus level, reduced abundance of Agathobacter, Parasutterella, Roseburia and Subdoligranulum were also observed in the AR cohorts compared to HCs (all p < 0.05) and significantly negatively associated with TNSS, EOS, tIgE, QOL, and FeNO (all p < 0.05).
Conclusion: AR children in Chinese Mainland were characterized by reduced microbial diversity and distinguished microbial characteristics in comparison with HCs. The observations of this study offer proof that distinctive gut microbiota profiles were present in AR children and necessitate further investigation in the form of mechanistic studies.
1. Introduction
Allergic rhinitis (AR) is a common allergic disease characterized by paroxysmal sneezing, nasal itching, nasal congestion, and runny nose caused by IgE-mediated immune responses to inhaled allergens (Ciprandi et al., 2015). The immune response involves inflammation of the mucosa, which is driven by the activation of type 2 helper T (Th2) cells (Bousquet J. et al., 2008). AR affects 10 to 40% of the world’s population, with a higher prevalence as high as 50% in some countries (Bousquet P. J. et al., 2008; Brożek et al., 2017). The prevalence of AR among Chinese children has been reported to be 15.79%, and it continues to increase (Hu et al., 2017). AR contributes to inefficiency at school, sleep problems, and reduces children’s chances of participating in outdoor activities (Devillier et al., 2016). Regretfully, the exact etiology of AR and its underlying biological mechanism are still unclear (Emeryk et al., 2019). A growing number of studies in recent years have suggested that imbalances in microecology are related to the occurrence of allergic disease (Zimmermann et al., 2019; Barcik et al., 2020; Shah and Bunyavanich, 2021). Microecology dysbiosis, especially in genetically susceptible hosts, may be a cofactor in the development of allergic diseases due to its role in undermining immune balance, including the proportion of effector T cells and regulatory T (Treg) cells (Salem et al., 2018).
In the 1980s, Strachan proposed the “hygiene hypothesis”, which suggests that children living in rural areas, having close contact with animals and drinking unpasteurized cow’s milk had a lower incidence of developing allergic diseases in the future (Huang et al., 2017). In 2015, the “microbiome hypothesis” was proposed, which suggested that the disruption of gut microbiome-mediated immune tolerance due to antibiotic use, infection, and other factors could eventually promote the development of the immune system towards allergy. The symbiosis between host and microbiome plays an essential role in maintaining immune homeostasis and human health, thus, changes in the gut microbiota are believed to be an important factor in the development of allergic disease (Mccoy and Köller, 2015).
Gut microbiota is in dynamic equilibrium and can regulate itself according to the change of environment. Children’s gut microbiota is less resistant and stable than adults and is sensitive to external factors such as the use of antibiotic and glucocorticoids (Fang et al., 2021). Glucocorticoids have remarkable local anti-inflammatory and anti-allergic effects and are the most effective drugs to treat allergic diseases in children, it could directly or indirectly affect gut microbial (Zhang et al., 2021; Liu et al., 2022). At the same time, antibiotics are widely used clinically, they could upset the balance of gut microbiota, leading to decline in microbial diversity and the overgrowth of bacteria such as Clostridium difficile (Ianiro et al., 2020).
With the development of the amplification and sequencing of the 16S rRNA gene, we can obtain the distribution map of microbial species presented in different allergic diseases (Su et al., 2021; Yuan et al., 2022). Currently, clinical studies have demonstrated alterations in the composition and function of the gut microbiome in adults and children with AR (Watts et al., 2021; Zhou et al., 2021). However, there is no study on the gut microbiome of children with AR in Chinese Mainland.
Therefore, in order to investigate the alters of children with AR in Chinese Mainland and evaluate the relationship between the gut microbiota and clinical indicators such as Total Nasal Symptom Score (TNSS), Visual Analog Scale (VAS), Quality of Life Score (QOL), Fractional exhaled nitric oxide (FeNO) value etc. We conducted this study, which will contribute to a better understanding of the underlying biological mechanisms of AR, and help to develop more effective prevention and treatment strategies for AR in children.
2. Materials and methods
2.1. Study design
This prospective and cross-sectional observational study was conducted with ethics approval from the Ethics Committee of Xijing Hospital at the Fourth Military Medical University in Xi’an, China (KY20222169-F-1), and was registered with the Chinese Clinical Trial Registry (ChiCTR2200065166). The study enrolled subjects from the Department of Pediatrics at Xijing Hospital during the period of July to December 2022. All parents or guardians of the participants provided written consent, and the study was conducted in accordance with the ethical principles outlined in the Declaration of Helsinki.
2.2. Study population
This is a description of the diagnostic and inclusion/exclusion criteria used in a clinical study of participants. AR diagnosis relied on the ARIA guidelines, which includes both seasonal and perennial AR (Cheng et al., 2018). After 1 month of treatment, a follow-up assessment was conducted to confirm the diagnosis of AR.
The inclusion criteria of AR group required children to have had a history of allergies in the past year, the presence of allergen-specific IgE antibody (sIgE) test ≥0.35 IU/mL and/or positive skin prick tests (SPT), aged 3-14 years, stools that appeared snake-shaped or banana-shaped. The inclusion criteria of HCs group required children without allergic history, aged 3–14 years, and stools that appeared snake-shaped or banana-shaped. The exclusion criteria of both groups were numerous and included as follows: using immunomodulatory medications in the past 12 months; using probiotics, prebiotics and synbiotics in the past 2 months; using antibiotics, local or systemic corticosteroids, antihistamines in the past 2 weeks; using laxatives or antidiarrheals in the past 1 week, having constipation, diarrhea or respiratory tract infection in the past week; having congenital heart disease, tuberculosis or tuberculosis contact history, mental illness history, autoimmune disease, gastrointestinal disease, and allergic asthma (Zhu et al., 2020; Zhou et al., 2021). The study participants were selected from the same geographic region and had similar dietary habits.
2.3. Stool sample collection
Before the start of the treatment, the parents of the study participants were provided with a fecal sample collection kit and dry ice, and were instructed on how to collect the stool sample. The collection instructions emphasized the importance of avoiding contamination of the samples with urine or water, and the samples were frozen immediately, and carefully transported to our laboratory using dry ice within 24 h. The samples were stored at a temperature of −80°C until they were processed (Chiu et al., 2019).
2.4. Total nasal symptom score
Four nasal symptoms (sneezing, nasal itching, rhinorrhea, nasal obstruction) were assessed using a four-point scale. The scale ranged from 0 to 3, where 0 indicated the absence of symptoms, 1 indicated mild symptoms, 2 indicated moderate symptoms, and 3 indicated severe symptomss (Gevaert et al., 2022).
2.5. Visual analog scale
At the initial consultation, the children were requested to indicate the intensity of their allergic rhinitis symptoms using a scale ranging from 0 to 10. A score of 0 meant that the child was asymptomatic, while a score of 10 indicated that the symptoms were most severe (Eifan et al., 2010).
2.6. Quality of life score
Quality of life scores of AR children are shown in Supplementary Table S1. During the initial consultation, the child or their guardian was required to mark the applicable space in the table that reflected the impact of the disease on the child’s life in the past 1–2 weeks. The quality of life scores in the supplementary table ranged from low to high, with higher scores indicating a worse quality of life (Okubo et al., 2020).
2.7. Microbiome analysis
To analyze the diversity and composition of the microbial community, DNA was extracted from fecal samples, the concentration and purity were measured using the NanoDrop One (Thermo Fisher Scientific, MA, United States). 16S rRNA genes of V3-V4 regions were amplified used specific primer 338F (5’-ACTCCTACGGGAGGCA GCAG-3′) and 806R (5’-GGACTACHVGGGTWTCTAAT-3′) with 12 bp barcode. Primers were synthesized by Invitrogen (Invitrogen, Carlsbad, CA, United States). Sequencing libraries were generated using NEBNext® Ultra™ II DNA Library Prep Kit for Illumina® (New England Biolabs, MA, United States) following manufacturer’s recommendations and index codes were added. The library quality was assessed on the Qubit@ 2.0 Fluorometer (Thermo Fisher Scientific, MA, United States). At last, the library was sequenced on an Illumina Nova6000 platform and 250 bp paired-end reads were generated (Guangdong Magigene Biotechnology Co., Ltd. Guangzhou, China).
Fastp (version 0.14.1, https://github.com/OpenGene/fastp) was used to control the quality of the Raw Data by sliding window, the primers were removed by using cutadapt software (https://github.com/marcelm/cutadapt/) according to the primer information at the beginning and end of the sequence to obtain the paired-end Clean Reads. Paired-end clean reads were merged using usearch-fastq_mergepairs (V10, http://www.drive5.com/usearch/), Fastp (version 0.14.1, https://github.com/OpenGene/fastp) was used to control the quality of the Raw Data by sliding window(-W 4 -M 20) to obtain the paired-end Clean Tags.
Operational taxonomic units (OTUs) were generated using the UPARSE default clustering approach. Taxonomic information was annotated using the Silva database and the usearch -sintax tool. Alpha diversity was analyzed using three indicators: chao1 index, Shannon index, and Simpson index. All this indices in our samples were calculated with usearch-alpha_div (V10, http://www.drive5.com/usearch/). The differences between groups were analyzed by alpha diversity index using R software. If there were only two groups, student’s t-test or Wilcox rank sum test was selected. Beta diversity was analyzed using the Bray-Curtis algorithm. PCoA was displayed by vegan package in R software. To identify biomarkers for each group, linear discriminant analysis (LDA) and linear discriminant analysis effect size (LEfSe) analysis were performed, setting LDA score ≥ 3.5 and obtained the biomarkers in different groups. All analyses were completed on the Magichand Cloud platform.
2.8. Statistical analyses
SPSS software (v.26.0) was used for the analysis of the baseline characteristics data. Continuous variables were reported as Mean ± SD, student t-test or Wilcoxon signed-rank test method was used for inter-group comparison; count data were reported as n (%), χ2 test or Fisher’s exact probabilities test was used for inter-group comparison. Correlation analysis between the clinical index and gut bacteria using Spearman’s correlation coefficient. p value < 0.05 was considered statistically significant.
3. Results
3.1. Characteristics of all participants
A total of 49 participants were enrolled in this study, including 24 children with AR and 25 HCs. Table 1 presents the clinical demographics of the study. Basic natural characteristics such as age, gender, and BMI (Body Mass Index), birth history including term birth and cesarean delivery, feeding history including breastfeeding for at least 3 months, and living environment such as the presence of siblings, exposure to passive smoking, and exposure to pets did not show significant differences between the two groups (all p > 0.05). However, the number of maternal allergy was significantly higher in the AR group compared to the HCs (p < 0.05). All AR children had positive sIgE results, 83.3% of the AR children had seasonal allergies, and 87.5% are in acute attack.
3.2. Gut microbiota of AR children versus HCs
The 16S rRNA gene sequencing was performed on the Illumina Nova 6,000 platform. A total of 4,573,805 high-quality sequences were acquired from 49 samples, after quality control filtering and minimum sample OTU normalization, averaging 61,446 effective sequences per sample (Supplementary Table S2). The Venn diagram showed that the number of OTUs in the AR group was 3,134, while that in the HCs group was 3,450. There were 2,260 OTUs shared by both groups, 874 OTUs unique to the AR group, and 1,190 OTUs unique to the HCs group. The total number of observed OTUs in the AR group was lower than that in the HCs group (Figure 1).
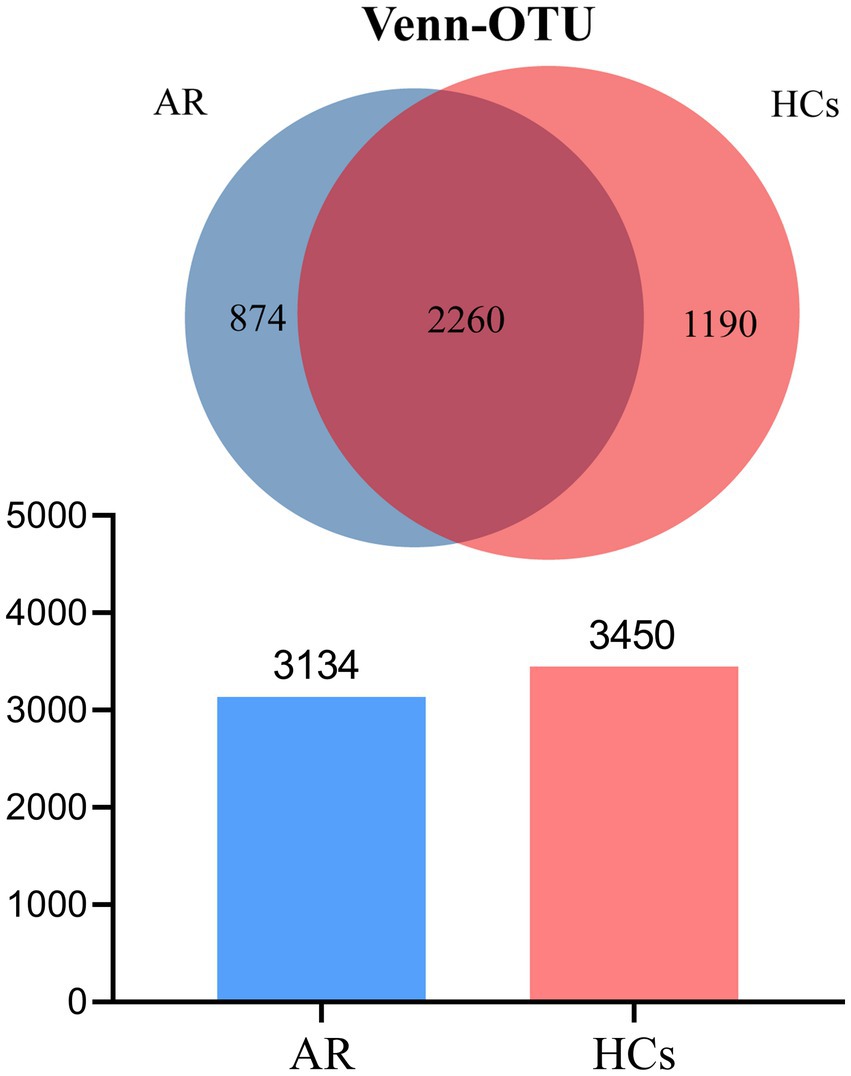
Figure 1. Venn plot. The total number of OTUs observed in the AR group was lower than that in the HCs group. AR, allergic rhinitis group; HCs, healthy control group.
3.2.1. Alpha-diversity
At the phylum level, there was no statistically significant difference between the two groups for both Chao1 index and Shannon index (all p > 0.05, Figures 2A,B), but there was a statistically significant difference for the Simpson index (p = 0.058, Figure 2C). At the family level, there was no statistically significant difference between the two groups for the Chao1 index (p > 0.05, Figure 2D), but there was a statistically significant difference for both the Shannon and Simpson indices (all p < 0.05, Figures 2E,F). At the genus level, there was no statistically significant difference between the two groups for the Chao1 index (p > 0.05, Figure 2G), but there was a statistically significant difference for both the Shannon and Simpson indices (all p < 0.05, Figures 2H,I). The outcomes mentioned above suggest that there is no variation in species richness between the two categories at all levels. However, a significant divergence in evenness exists, and it becomes more explicit as the taxonomic hierarchy becomes more detailed (Supplementary Table S3).
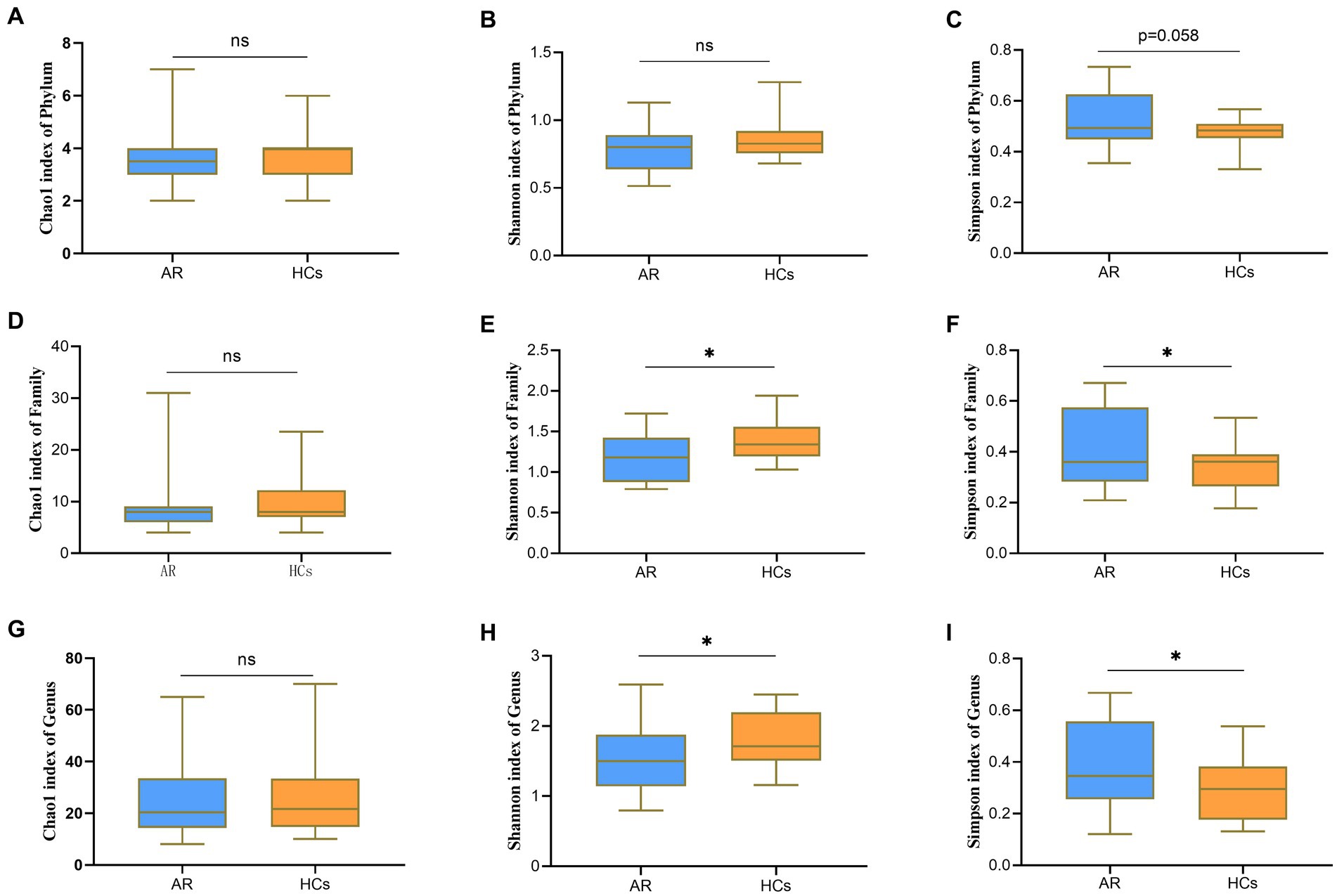
Figure 2. Alpha-Diversity analysis chart. The Alpha-Diversity indexes comparing including Chao1, Shannon and Simpson. (A) Chao1 index at the phylum level; (B) Shannon index at the phylum level; (C) Simpson index at the phylum level; (D) Chao1 index at the family level; (E) Shannon index at the family level; (F) Simpson index at the family level; (G) Chao1 index at the genus level; (H) Shannon index at the genus level; and (I) Simpson index at the genus level. AR = allergic rhinitis group; HCs, healthy control group. ns, no statistical difference between the two groups; *there is a statistical difference between the two groups, p < 0.05.
3.2.2. Beta-diversity
Figure 3A shows the PCoA based on Bray–Curtis dissimilarity analysis. The PCoA1 from Adonis analysis showed that R2 = 0.052, p = 0.003, indicating a significant difference between the two groups (Figure 3B).
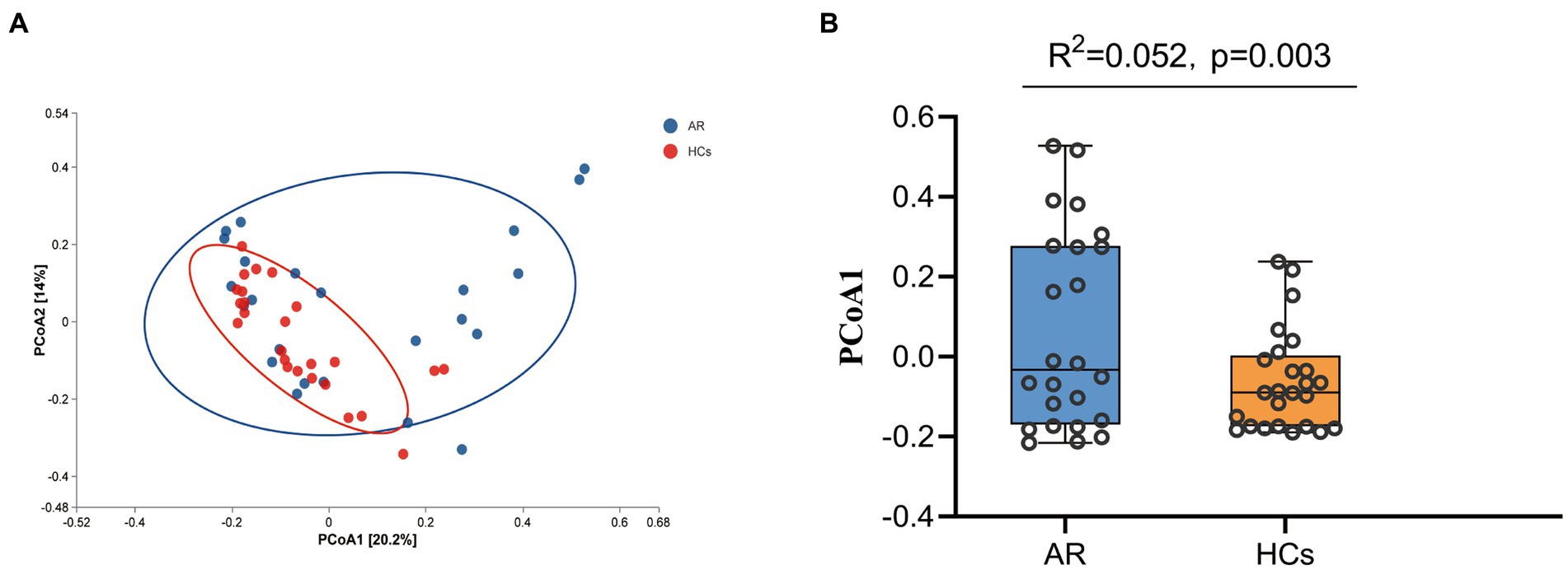
Figure 3. Beta-Diversity Analysis Chart. The Beta-Diversity including PcoA and Adonis analysis. (A) PCoA analysis results based on Bray curtis and (B) PCoA1 difference based on Adonis analysis. AR, allergic rhinitis group; HCs, healthy control group.
3.2.3. Microbial composition classification
The sequence information of the three taxonomic levels of phylum, family, and genus in the OTU table was counted to calculate the relative abundance of microbial communities at each taxonomic level. The taxa with relative abundance greater than 1% were selected to draw a relative abundance diagram. Student’s t-test or Wilcoxon rank sum test were used to compare the differences in microbial communities (Supplementary Table S4).
At the phylum level, Bacteroidetes, Firmicutes, Proteobacteria, Verrucomicrobia, and Actinobacteria were the dominant phyla in the two groups (Figure 4A). The relative abundance of Bacteroidetes in the AR group was significantly higher than that in the HCs group (p < 0.05, Figure 4B), while the relative abundance of Firmicutes in the AR group was lower than in the HCs group (p = 0.08, Figure 4C). In addition, the ratio of Firmicutes to Bacteroidetes also differed between the two groups (p = 0.057, Figure 4D).
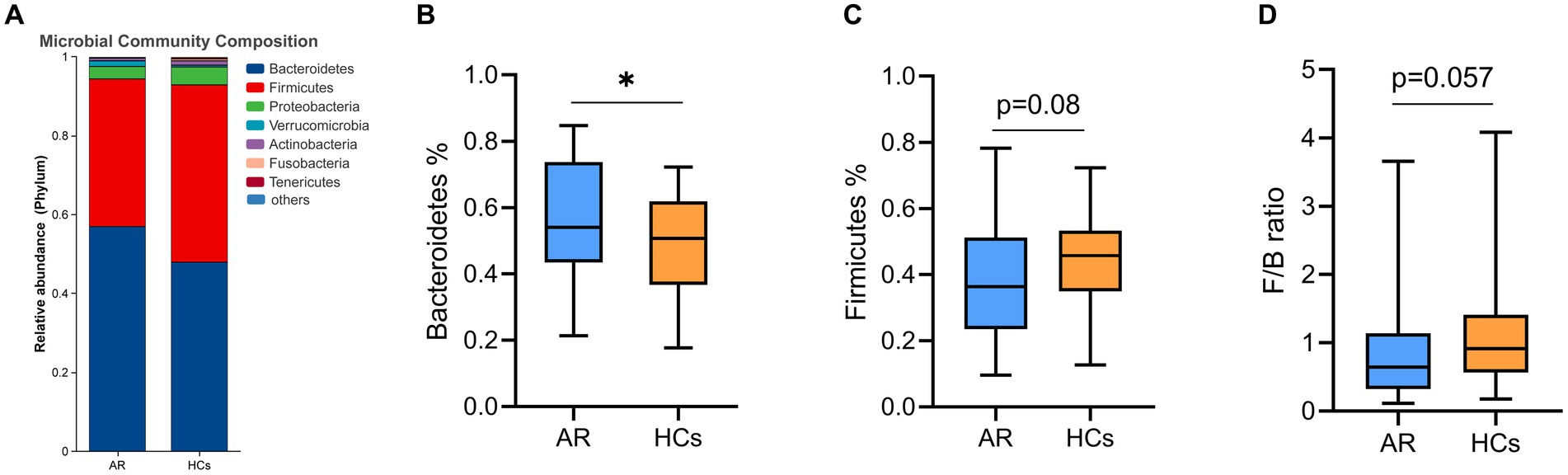
Figure 4. The difference of gut microbiota at phylum level. (A) Community composition at the phylum level; (B) Inter-group differences of Bacteroidetes in phylum; (C) Inter-group differences of Firmicutes in phylum; and (D) Inter-group differences in the ratio of Firmicutes to Bacteroidetes. AR, allergic rhinitis group; HCs, healthy control group. *there is a statistical difference between the two groups, p < 0.05.
At the family level, Bacteroidaceae, Lachnospiraceae, Ruminococcaceae, and Prevotellaceae were the dominant families in the two groups (Figure 5A). The relative abundance of Prevotellaceae and Enterobacteriaceae in the AR group was significantly higher than that in the HCs group (all p < 0.05, Figures 5B,D). While the relative abundance of Burkholderiaceae in the AR group was significantly lower than HCs (p < 0.05, Figure 5C).
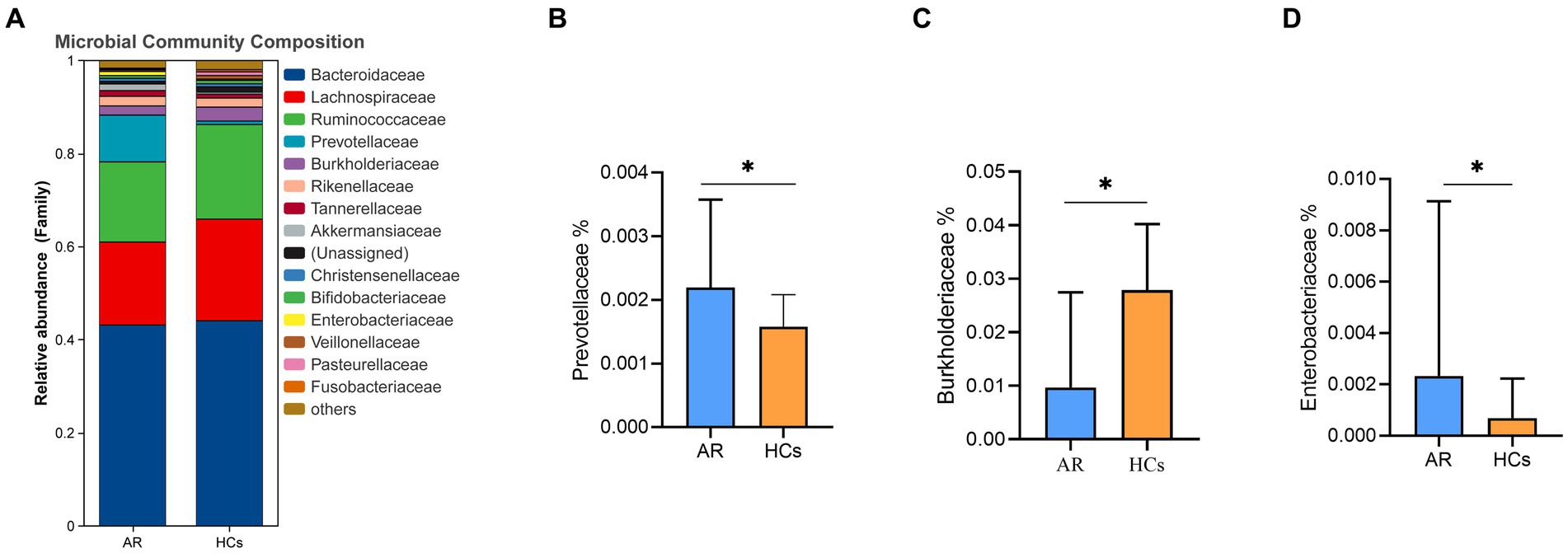
Figure 5. The difference of gut microbiota at family level. (A) Community composition at the family level; (B) Inter-group differences of Prevotellaceae in family; (C) Inter-group differences of Burkholderiaceae in family; (D) Inter-group differences of Enterobacteriaceae in family. AR, allergic rhinitis group; HCs, healthy control group. *there is a statistical difference between the two groups, p < 0.05.
At the genus level, Bacteroides, Faecalibacterium, Agathobacter, Prevotella_9, Alistipes, Parasutterella, Prevotella_2, Blautia, Roseburia and Subdoligranulum were the dominant genus in the two groups (Figure 6A). Among them, the relative abundance of Agathobacter, Parasutterella, Roseburia and Subdoligranulum in the AR group was lower than that in the HCs group (all p < 0.05, Figures 6B–E).
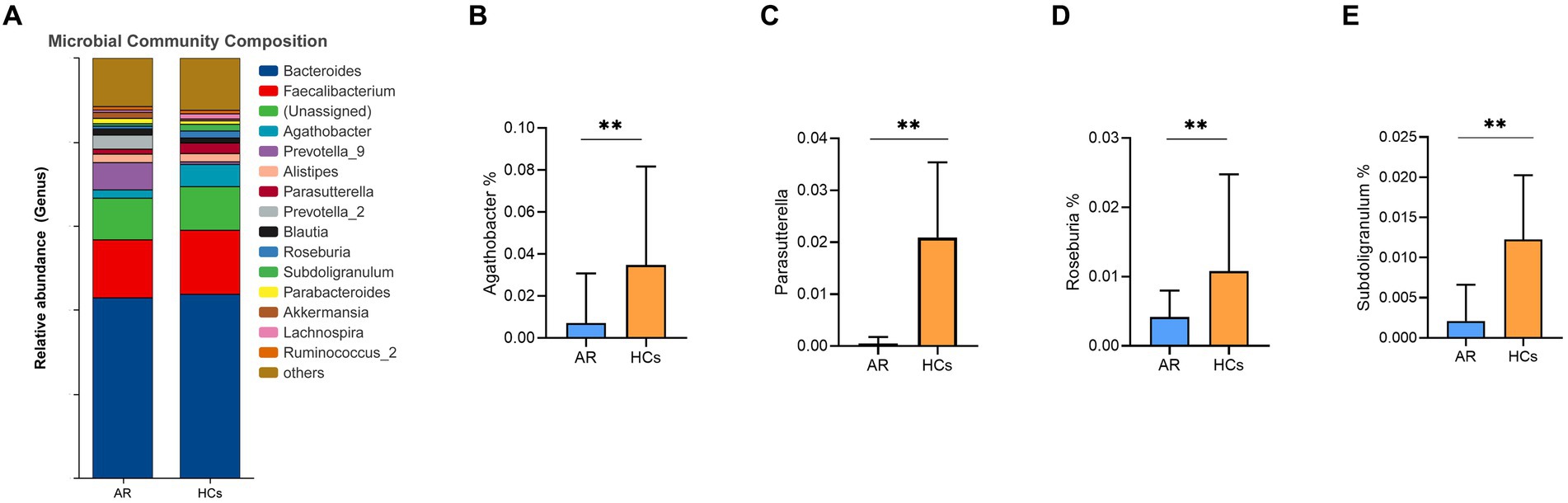
Figure 6. The difference of gut microbiota at genus level. (A) Community composition at the genus level; (B) Inter-group differences of Agathobacter in genus; (C) Inter-group differences of Parasutterella in genus; (D) Inter-group differences of Roseburia in genus; (E) Inter-group differences of Subdoligranulum in genus. AR, allergic rhinitis group; HCs, healthy control group. **there is a statistical difference between the two groups, p < 0.01.
3.2.4. LEfSe analyze
LEfSe analysis is one of the tools used for identifying and interpreting biological markers in high-dimensional data. It can also be applied to compare groups to identify species (or biomarkers) with significant differences in abundance between those groups. Figures 7A,B shows the differential bacterial taxa with LDA scores above 3.5. The relative abundance of Firmicutes, Lachnospiraceae, Agathobacter, Parasutterella, Roseburia, and Subdoligranulum was higher in the HCs group than that in the AR group. However, the relative abundance of Bacteroidetes, Prevotellaceae, and Enterobacteriaceae was higher in the AR group compared to the HCs.
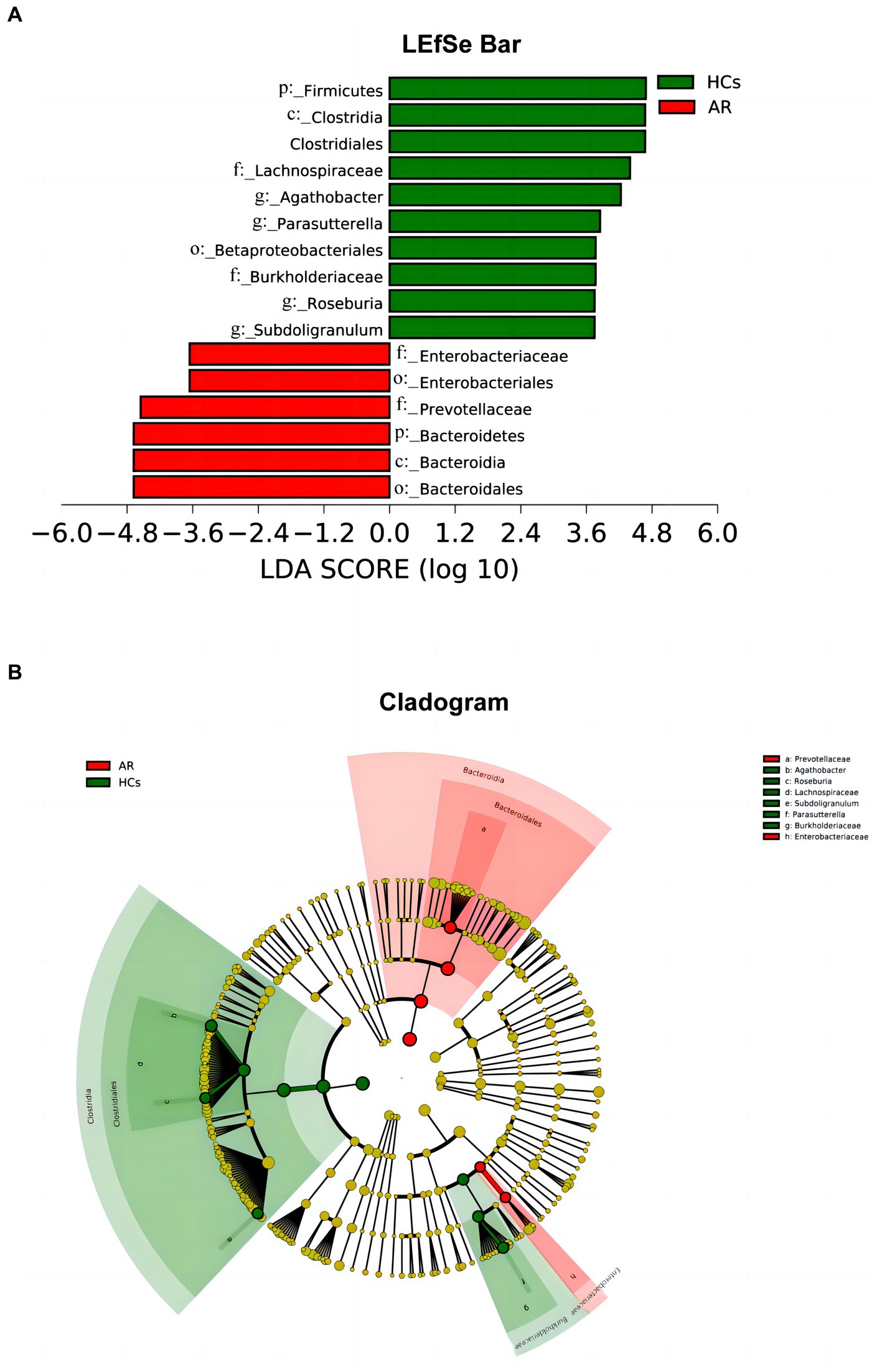
Figure 7. LEfSe chart. LEfSe analysis showed differential taxa with LDA scores greater than 3.5 between two groups (A) linear discriminant analysis (LDA) distribution histogram (LDA > 3.5, p < 0.05) and (B) Cladogram. AR, allergic rhinitis group; HCs, healthy control group.
3.3. Association of gut microbiota related to clinical indicators
Spearman correlation analysis was performed to investigate the correlation between different bacterial taxa and clinical indicators at the different levels. At the phylum level, Bacteroidetes was positively correlated with TNSS (p < 0.05), while Synergistetes and Acidobacteria were negatively correlated with EOS, tIgE, TNSS, and FeNO (all p < 0.05) (Figure 8A). At the family level, Enterobacteriaceae was positively correlated with EOS, tIgE, TNSS, and FeNO; Prevotellaceae was positively correlated with EOS, tIgE, TNSS, and QOL (all p < 0.05), while Burkholderiaceae was negatively correlated with EOS, tIgE and TNSS (all p < 0.05) (Figure 8B). At the genus level, Parasutterella, Roseburia, Agathobacter, and Subdoligranulum were negatively correlated with EOS, tIgE, TNSS, QOL, and FeNO (all p < 0.05) (Figure 8C).
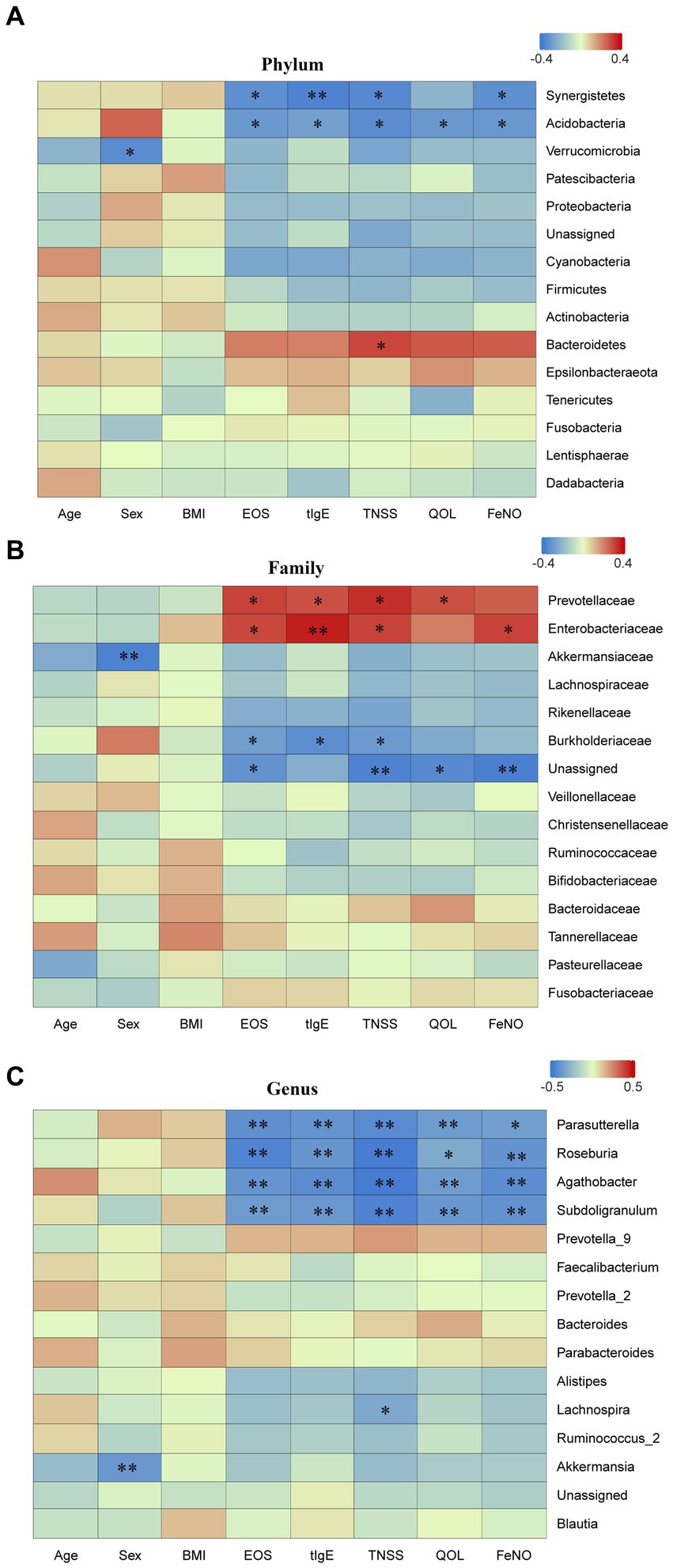
Figure 8. Spearman correlation analysis. Spearman correlation analysis was performed to examine the correlation between different taxa at various levels and clinical indicators. (A) phylum level; (B) family level; and (C) genus level. EOS, eosinophils; tIgE, total immunoglobulin E; TNSS, total nasal symptom score; QOL, quality of life score; FeNO, fractional exhaled nitric oxide. Red represents positive correlation, while blue represents negative correlation. AR, allergic rhinitis group; HCs, healthy control group. *there is a statistical difference between the two groups, p < 0.05. **there is a statistical difference between the two groups, p < 0.01.
4. Discussion
An increasing amount of research suggests that gut microbiota dysbiosis was closely linked to the incidence and development of allergic diseases (Arrieta et al., 2015; Fujimura et al., 2016; Khadka et al., 2021). However, only one past study investigated the link between gut microbiota and childhood AR, but this study is limited to the Taiwan region (Chiu et al., 2019). Considering that different regional environments may have an impact on microecology. The aims of this study was to investigate the alters of children with AR in Chinese Mainland and evaluate the relationship between the gut microbiota and clinical indicators.
In this research, we observed that the alpha diversity in the gut microbiota was decreased, beta diversity and gut microbiota structure were significantly changed in AR children compared to HCs. The conclusions of our study showed a more significant statistical difference compared to the previous ones (Chiu et al., 2019). This study mainly found that the occurrence of AR in children is associated with changes in gut microbiota diversity. As compared to HCs, the Shannon index in AR children was significantly reduced, particularly at the family and genus levels. Similar trends were observed with the Simpson index and OTU count. Reduced gut microbiota diversity weakens their ability to resist external interferences, and it is believed to be an important indicator of whether the gut microbiota is stable or not (Bäckhed et al., 2012). Other studies also shown that people with allergic diseases have lower gut microbiota diversity than healthy ones. Hua et al. analyzed 16S rRNA gene data obtained from US public database and found that the increased incidence of allergic diseases in adults was positively correlated with the reduction in gut microbiota diversity (Melli et al., 2016). Meanwhile, Bisgaard et al. reported that the decrease in gut microbiota diversity at 1 month and 12 months after birth increased the risk of AR in children in the first 6 years of life (Bisgaard et al., 2011). All of the above studies suggest that a decrease of gut microbiota diversity may influence the development of allergic diseases.
This study also found that the gut microbiota composition of children with AR significantly differs from HCs at various levels. At the phylum level, there is a certain difference in the proportion of Bacteroidetes and Firmicutes between the two groups, with a higher relative abundance of Bacteroidetes in AR children. Similar findings of Bacteroidetes abundance in fecal samples of allergy sufferers have been reported in other studies. Data analysis from the American Gut Project revealed that the phylum Bacteroidetes was more abundant in adults with nut and pollen allergies than in healthy adults (Melli et al., 2016). A study from Japan also showed that infants with high abundance of Bacteroidetes were more prone to developing allergic diseases after the age of 2 (Songjinda et al., 2007). Bacteroidetes and Firmicutes are the most common phyla in fecal microbiota, and they play important roles in gut health and immune regulation through the production of short-chain fatty acids (SCFAs) (Brody, 2020). In general, the presence of Bacteroidetes was often linked to a higher production of acetate and propionate, whereas the presence of Firmicutes was often linked to a higher production of butyrate (den Besten et al., 2013). Of all the SCFAs, butyrate is a key component in keeping the colon healthy by serving as a source of energy for colonic epithelial cells and regulating tight junction expression to preserve the integrity of the gut barrier (Rauf et al., 2022). Lack of butyrate can cause transfer of inflammatory molecules and antigens to the submucosa and the circulatory system, leading to localized and systemic inflammatory reactions. The prevalence of Bacteroidetes over Firmicutes may decrease the production of butyrate, thereby impacting the integrity of the gut barrier (Rauf et al., 2022) and the gut permeability of allergic disease patients is significantly higher than that of healthy controls (Benard et al., 1996; Majamaa and Isolauri, 1996). This implies that alterations in the abundance of Bacteroidetes and Firmicutes have an impact on the incidence and progression of allergic diseases, prompting the need for more research to clarify the connection between the level of butyrate-producing bacteria and intestinal permeability in patients with atopic diseases.
At the family level, there was a significant increase in the relative abundance of Enterobacteriaceae in the AR group. Enterobacteriaceae are Gram-negative facultative anaerobes, including intestinal bacteria such as Escherichia coli, Shigella, and Salmonella. Some strains of Enterobacteriaceae can adhere to the intestinal mucosa and stimulate immune reactions (Kim et al., 2018). Studies have found that in patients with allergies, the quantity of Enterobacteriaceae increases significantly. This phenomenon could be associated with the disturbance of the gut microbiota, leading to potential impacts on host immune and allergic responses (Zimmermann et al., 2019). Because E. coli and Shigella have the same 16S rRNA gene sequence, it is difficult to identify the specific adherent Enterobacteriaceae species only by 16S sequencing. Therefore, we will perform metagenomic sequencing in the future to determine the exact types of adherent Enterobacteriaceae.
In the AR group, the relative abundance of Subdoligranulum, Agathobacter, and Roseburia at the genus level was significantly lower when compared to healthy controls. Subdoligranulum, a member of the Ruminococcaceae family, can activate the neonatal Treg cells’ MyD88/ROR-γt pathway, thus effectively preventing food allergies (Duncan et al., 2004). The newly discovered Agathobacter belongs to the Lachnospiraceae family (Ahn et al., 2016) and Roseburia has become a potential health marker for gut microbiota due to its anti-inflammatory properties (Tamanai-Shacoori et al., 2017). Subdoligranulum, Agathobacter, and Roseburia can all produce butyrate, promoting gut health by providing energy to host cells and maintaining the integrity of the gut barrier (Ahn et al., 2016; Deaver et al., 2018). The decrease in relative abundance of Subdoligranulum, Agathobacter, and Roseburia in children with AR may indicate a decrease in butyrate levels and gut barrier disruption.
The cellular mechanism behind AR primarily involves the differentiation of CD4+ T cells into Th2 cells. This results in an imbalance of the Th1/Th2 ratio, thus promoting B cell activation and class switching to IgE (Kulkarni et al., 2011). Additionally, it causes the differentiation of B cells into IgE-producing plasma cells, as well as mast cell and eosinophil activation to release inflammatory mediators (Wang et al., 1992; Iinuma et al., 2018). Previous studies have demonstrated that an imbalanced gut microbiota can activate innate and adaptive immune cells, which may contribute to the onset of atopic diseases in children (Fujimura et al., 2016). In addition, SCFAs, which are metabolic products of gut microbiota, serve as mediators of communication between the immune system and gut microbiota (Milligan et al., 2017). SCFAs facilitate the differentiation of T cells into both regulatory and effector T cells, maintaining the balance between anti-inflammatory/pro-inflammatory responses, and promoting the establishment of immune tolerance (Park et al., 2015). Our study discovered alterations of gut microbiota in AR children, particularly, a reduction in the prevalence of butyrate-producing bacteria. This finding possibly supports the conclusion that SCFAs can prevent allergic respiratory diseases through their effects on T cells (Cait et al., 2018).
This study has some limitations. First, the sample size is relatively small, as most of the children had already used corticosteroids or antihistamines before their visit to the hospital. Second, the classification level in this study is relatively high, mainly at the phylum, family, and genus levels. Therefore, further research is needed to explore the changes in gut microbiota at the species level in children with AR to provide more precise microbiota-based treatments in the future. Lastly, as the role of gut microbiota metabolites in diseases becomes increasingly important, future studies will also perform metabolomics to further understand the changes in gut microbiota metabolites in children with AR.
5. Conclusion
In summary, we observed a close relationship between childhood AR and gut microbiota, confirming the changes in gut microbiota composition and diversity in children with AR. We believe that gut microbiota has the potential for diagnosis and treatment of childhood AR, and also provides some ideas for mechanism of AR.
Data availability statement
The datasets presented in this study can be found in online repositories. The names of the repository/repositories and accession number(s) can be found at: https://figshare.com/s/8b5ae4eaa3489e55578c.
Ethics statement
The experimental procedures were approved by the Ethics Committee of Xijing Hospital at the Fourth Military Medical University in Xi’an, China (approval number: KY20222169-F-1). Written informed consent to participate in this study was provided by the participants’ legal guardian/next of kin.
Author contributions
PZ, XZ, HT, FJ, ZJ, HW, YZ, JL, JZ, and XS contributed to the study conception and design. PZ, XZ, and HT had the idea for the article. HW, YZ, and JL performed the data collection. The data analysis was performed by PZ and XZ. The first draft of the manuscript was written by PZ. XS and JZ critically revised the work. FJ and ZJ commented on several drafts. All authors contributed to the article and approved the submitted version.
Funding
This work was supported by the National Natural Science Foundation of China (82170026); Technological Innovation Guidance Special Project of Shaanxi Province (2022QFY01-09); Natural Science Basic Research Plan in Shaanxi Province (2022JQ-764) and Discipline Promotion Project of Xijing Hospital (XJZT18MJ23 and XJZT21L11); and Clinical Research Project of Air Force Military Medical University (2022LC2210).
Conflict of interest
The authors declare that the research was conducted in the absence of any commercial or financial relationships that could be construed as a potential conflict of interest.
Publisher’s note
All claims expressed in this article are solely those of the authors and do not necessarily represent those of their affiliated organizations, or those of the publisher, the editors and the reviewers. Any product that may be evaluated in this article, or claim that may be made by its manufacturer, is not guaranteed or endorsed by the publisher.
Supplementary material
The Supplementary material for this article can be found online at: https://www.frontiersin.org/articles/10.3389/fmicb.2023.1208816/full#supplementary-material
References
Ahn, S., Jin, T. E., Chang, D. H., Rhee, M. S., Kim, H. J., Lee, S. J., et al. Agathobaculum butyriciproducens gen. nov. sp. nov., a strict anaerobic, butyrate-producing gut bacterium isolated from human faeces and reclassification of Eubacterium desmolans as Agathobaculum desmolans comb. nov. Int. J. Syst. Evol. Microbiol. (2016). 66, (9): 3656–3661. doi: 10.1099/ijsem.0.001195
Arrieta, M. C., Stiemsma, L. T., Dimitriu, P. A., Thorson, L., Russell, S., Yurist-Doutsch, S., et al. (2015). Early infancy microbial and metabolic alterations affect risk of childhood asthma. Sci. Transl. Med. 7:307ra152. doi: 10.1126/scitranslmed.aab2271
Bäckhed, F., Fraser, C. M., Ringel, Y., Sanders, M. E., Sartor, R. B., Sherman, P. M., et al. (2012). Defining a healthy human gut microbiome: current concepts, future directions, and clinical applications. Cell Host Microbe 12, 611–622. doi: 10.1016/j.chom.2012.10.012
Barcik, W., Boutin, R. C. T., Sokolowska, M., and Finlay, B. B. (2020). The role of lung and gut microbiota in the pathology of asthma. Immunity 52, 241–255. doi: 10.1016/j.immuni.2020.01.007
Benard, A., Desreumeaux, P., Huglo, D., Hoorelbeke, A., Tonnel, A. B., and Wallaert, B. (1996). Increased intestinal permeability in bronchial asthma. J. Allergy Clin. Immunol. 97, 1173–1178. doi: 10.1016/S0091-6749(96)70181-1
Bisgaard, H., Li, N., Bonnelykke, K., Chawes, B. L., Skov, T., Paludan-Müller, G., et al. (2011). Reduced diversity of the intestinal microbiota during infancy is associated with increased risk of allergic disease at school age. J. Allergy Clin. Immunol. 128, 646–652.e5. doi: 10.1016/j.jaci.2011.04.060
Bousquet, J., Khaltaev, N., Cruz, A. A., Denburg, J., Fokkens, W. J., Togias, A., et al. (2008). Allergic rhinitis and its impact on asthma (ARIA) 2008 update (in collaboration with the World Health Organization, GA(2)LEN and AllerGen). Allergy 63, 8–160. doi: 10.1111/j.1398-9995.2007.01620.x
Bousquet, P. J., Leynaert, B., Neukirch, F., Sunyer, J., Janson, C. M., Anto, J., et al. (2008). Geographical distribution of atopic rhinitis in the European Community respiratory health survey I. Allergy 63, 1301–1309. doi: 10.1111/j.1398-9995.2008.01824.x
Brożek, J. L., Bousquet, J., Agache, I., Agarwal, A., Bachert, C., Bosnic-Anticevich, S., et al. (2017). Allergic rhinitis and its impact on asthma (ARIA) guidelines—2016 revision. J. Allergy Clin. Immunol. 140, 950–958. doi: 10.1016/j.jaci.2017.03.050
Cait, A., Hughes, M. R., Antignano, F., Cait, J., Dimitriu, P. A., Maas, K. R., et al. (2018). Microbiome-driven allergic lung inflammation is ameliorated by short-chain fatty acids. Mucosal Immunol. 11, 785–795. doi: 10.1038/mi.2017.75
Cheng, L., Chen, J., Fu, Q., He, S., Li, H., Liu, Z., et al. (2018). Chinese Society of Allergy Guidelines for diagnosis and treatment of allergic rhinitis. Allergy, Asthma Immunol. Res. 10, 300–353. doi: 10.4168/aair.2018.10.4.300
Chiu, C. Y., Chan, Y. L., Tsai, M. H., Wang, C. J., Chiang, M. H., and Chiu, C. C. (2019). Gut microbial dysbiosis is associated with allergen-specific IgE responses in young children with airway allergies. World Allergy Organ. J. 12:100021. doi: 10.1016/j.waojou.2019.100021
Ciprandi, G., Marseglia, G. L., Castagnoli, R., Valsecchi, C., Tagliacarne, C., Caimmi, S., et al. (2015). From IgE to clinical trials of allergic rhinitis. Expert. Rev. Clin. Immunol. 11, 1321–1333. doi: 10.1586/1744666X.2015.1086645
Deaver, J. A., Eum, S. Y., and Toborek, M. (2018). Circadian disruption changes gut microbiome taxa and functional gene composition. Front. Microbiol. 9:737. doi: 10.3389/fmicb.2018.00737
den Besten, G., van Eunen, K., Groen, A. K., Venema, K., Reijngoud, D. J., and Bakker, B. M. (2013). The role of short-chain fatty acids in the interplay between diet, gut microbiota, and host energy metabolism. J. Lipid Res. 54, 2325–2340. doi: 10.1194/jlr.R036012
Devillier, P., Bousquet, J., Salvator, H., Naline, E., Grassin-Delyle, S., and de Beaumont, O. (2016). In allergic rhinitis, work, classroom and activity impairments are weakly related to other outcome measures. Clin. Exp. Allergy 46, 1456–1464. doi: 10.1111/cea.12801
Duncan, S. H., Louis, P., and Flint, H. J. (2004). Lactate-utilizing bacteria, isolated from human feces, that produce butyrate as a major fermentation product. Appl. Environ. Microbiol. 70, 5810–5817. doi: 10.1128/aem.70.10.5810-5817.2004
Eifan, A. O., Akkoc, T., Yildiz, A., Keles, S., Ozdemir, C., Bahceciler, N. N., et al. (2010). Clinical efficacy and immunological mechanisms of sublingual and subcutaneous immunotherapy in asthmatic/rhinitis children sensitized to house dust mite: an open randomized controlled trial. Clin. Exp. Allergy 40, 922–932. doi: 10.1111/j.1365-2222.2009.03448.x
Emeryk, A., Emeryk-Maksymiuk, J., and Janeczek, K. (2019). New guidelines for the treatment of seasonal allergic rhinitis. Postepy. Dermatol. Alergol. 36, 255–260. doi: 10.5114/ada.2018.75749
Fang, Z., Li, L., Zhang, H., Zhao, J., Lu, W., and Chen, W. (2021). Gut microbiota, probiotics, and their interactions in prevention and treatment of atopic dermatitis: a review. Front. Immunol. 12:720393. doi: 10.3389/fimmu.2021.720393
Fujimura, K. E., Sitarik, A. R., Havstad, S., Lin, D. L., Levan, S., Fadrosh, D., et al. (2016). Neonatal gut microbiota associates with childhood multisensitized atopy and T cell differentiation. Nat. Med. 22, 1187–1191. doi: 10.1038/nm.4176
Gevaert, P., Saenz, R., Corren, J., Han, J. K., Mullol, J., Lee, S. E., et al. (2022). Long-term efficacy and safety of Omalizumab for nasal polyposis in an open-label extension study. J. Allergy Clin. Immunol. 149, 957–65.e3. doi: 10.1016/j.jaci.2021.07.045
Hu, S. J., Wei, P., Kou, W., Wu, X. F., Liu, M. Y., Chen, C., et al. (2017). Prevalence and risk factors of allergic rhinitis: a meta-analysis. Lin Chung Er Bi Yan Hou Tou Jing Wai Ke Za Zhi 31, 1485–1491. doi: 10.13201/j.issn.1001-1781.2017.19.006.
Huang, Y. J., Marsland, B. J., Bunyavanich, S., O’Mahony, L., Leung, D. Y. M., Muraro, A., et al. (2017). The microbiome in allergic disease: current understanding and future opportunities—2017 PRACTALL document of the American Academy of Allergy, Asthma & Immunology and the European academy of allergy and clinical immunology. J. Allergy Clin. Immunol. 139, 1099–1110. doi: 10.1016/j.jaci.2017.02.007
Ianiro, G., Mullish, B. H., Kelly, C. R., Kassam, Z., Kuijper, E. J., Ng, S. C., et al. (2020). Reorganisation of faecal microbiota transplant services during the COVID-19 pandemic. Gut 69, 1555–1563. doi: 10.1136/gutjnl-2020-321829
Iinuma, T., Okamoto, Y., Morimoto, Y., Arai, T., Sakurai, T., Yonekura, S., et al. (2018). Pathogenicity of memory Th2 cells is linked to stage of allergic rhinitis. Allergy 73, 479–489. doi: 10.1111/all.13295
Khadka, V. D., Key, F. M., Romo-González, C., Martínez-Gayosso, A., Campos-Cabrera, B. L., Gerónimo-Gallegos, A., et al. (2021). The skin microbiome of patients with atopic dermatitis normalizes gradually during treatment. Front. Cell. Infect. Microbiol. 11:720674. doi: 10.3389/fcimb.2021.720674
Kim, M., Galan, C., Hill, A. A., Wu, W. J., Fehlner-Peach, H., Song, H. W., et al. (2018). Critical role for the microbiota in cx(3)Cr1(+) intestinal mononuclear phagocyte regulation of intestinal T cell responses. Immunity 49, 151–163.e5. doi: 10.1016/j.immuni.2018.05.009
Kulkarni, R., Behboudi, S., and Sharif, S. (2011). Insights into the role of toll-like receptors in modulation of T cell responses. Cell Tissue Res. 343, 141–152. doi: 10.1007/s00441-010-1017-1
Liu, H., Zhang, B., Li, F., Liu, L., and Li, F. (2022). Shifts in the intestinal microflora of meat rabbits in response to glucocorticoids. J. Sci. Food Agric. 102, 5422–5428. doi: 10.1002/jsfa.11895
Majamaa, H., and Isolauri, E. (1996). Evaluation of the gut mucosal barrier: evidence for increased antigen transfer in children with atopic eczema+. J. Allergy Clin. Immunol. 97, 985–990. doi: 10.1016/S0091-6749(96)80074-1
Mccoy, K. D., and Köller, Y. (2015). New developments providing mechanistic insight into the impact of the microbiota on allergic disease. Clin. Immunol. 159, 170–176. doi: 10.1016/j.clim.2015.05.007
Melli, L. C., do Carmo-Rodrigues, M. S., Araújo-Filho, H. B., Solé, D., and de Morais, M. B. (2016). Intestinal microbiota and allergic diseases: a systematic review. Allergol Immunopathol (Madr) 44, 177–188. doi: 10.1016/j.aller.2015.01.013
Milligan, G., Shimpukade, B., Ulven, T., and Hudson, B. D. (2017). Complex pharmacology of free fatty acid receptors. Chem. Rev. 117, 67–110. doi: 10.1021/acs.chemrev.6b00056
Okubo, K., Kurono, Y., Ichimura, K., Enomoto, T., Okamoto, Y., Kawauchi, H., et al. (2020). Japanese guidelines for allergic rhinitis 2020. Allergol. Int. 69, 331–345. doi: 10.1016/j.alit.2020.04.001
Park, J., Kim, M., Kang, S. G., Jannasch, A. H., Cooper, B., Patterson, J., et al. (2015). Short-chain fatty acids induce both effector and regulatory T cells by suppression of histone deacetylases and regulation of the Mtor-S6k pathway. Mucosal Immunol. 8, 80–93. doi: 10.1038/mi.2014.44
Rauf, A., Khalil, A. A., Rahman, U. U., Khalid, A., Naz, S., Shariati, M. A., et al. (2022). Recent advances in the therapeutic application of short-chain fatty acids (SCFAs): an updated review. Crit. Rev. Food Sci. Nutr. 62, 6034–6054. doi: 10.1080/10408398.2021.1895064
Salem, I., Ramser, A., Isham, N., and Ghannoum, M. A. (2018). The gut microbiome as a major regulator of the gut-skin Axis. Front. Microbiol. 9:1459. doi: 10.3389/fmicb.2018.01459
Shah, R., and Bunyavanich, S. (2021). The airway microbiome and pediatric asthma. Curr. Opin. Pediatr. 33, 639–647. doi: 10.1097/MOP.0000000000001054
Songjinda, P., Nakayama, J., Tateyama, A., Tanaka, S., Tsubouchi, M., Kiyohara, C., et al. (2007). Differences in developing intestinal microbiota between allergic and non-allergic infants: a pilot study in Japan. Biosci. Biotechnol. Biochem. 71, 2338–2342. doi: 10.1271/bbb.70154
Su, Y. J., Luo, S. D., Hsu, C. Y., and Kuo, H. C. (2021). Differences in gut microbiota between allergic rhinitis, atopic dermatitis, and skin urticaria. Medicine (Baltimore) 100:E25091. doi: 10.1097/MD.0000000000025091
Tamanai-Shacoori, Z., Smida, I., Bousarghin, L., Loreal, O., Meuric, V., Fong, S. B., et al. (2017). Roseburiaspp.: a marker of health? Future Microbiol. 12, 157–170. doi: 10.2217/fmb-2016-0130
Wang, B., Rieger, A., Kilgus, O., Ochiai, K., Maurer, D., Födinger, D., et al. (1992). Epidermal Langerhans cells from normal human skin bind monomeric IgE via fc epsilon RI. J. Exp. Med. 175, 1353–1365. doi: 10.1084/jem.175.5.1353
Watts, A. M., West, N. P., Zhang, P., Smith, P. K., Cripps, A. W., and Cox, A. J. (2021). The gut microbiome of adults with allergic rhinitis is characterised by reduced diversity and an altered abundance of Key microbial taxa compared to controls. Int. Arch. Allergy Immunol. 182, 94–105. doi: 10.1159/000510536
Yuan, Y., Wang, C., Wang, G., Guo, X., Jiang, S., Zuo, X., et al. (2022). Airway microbiome and serum metabolomics analysis identify differential candidate biomarkers in allergic rhinitis. Front. Immunol. 12:771136. doi: 10.3389/fimmu.2021.771136
Zhang, J., Feng, D., Law, H. K., Wu, Y., Zhu, G. H., Huang, W. Y., et al. (2021). Integrative analysis of gut microbiota and fecal metabolites in rats after prednisone treatment. Microbiol. Spectr. 9:E0065021. doi: 10.1128/Spectrum.00650-21
Zhou, M. S., Zhang, B., Gao, Z. L., Zheng, R. P., Marcellin, D. F. H. M., Saro, A., et al. (2021). Altered diversity and composition of gut microbiota in patients with allergic rhinitis. Microb. Pathog. 161, (Pt A):105272. doi: 10.1016/j.micpath.2021.105272
Zhu, L., Xu, F., Wan, W., Yu, B., Tang, L., Yang, Y., et al. (2020). Gut microbial characteristics of adult patients with allergy rhinitis. Microb. Cell Factories 19:171. doi: 10.1186/s12934-020-01430-0
Keywords: allergic rhinitis, children, 16S rRNA gene sequencing, gut, microbiota
Citation: Zhang P, Zhou X, Tan H, Jian F, Jing Z, Wu H, Zhang Y, Luo J, Zhang J and Sun X (2023) Microbial signature of intestine in children with allergic rhinitis. Front. Microbiol. 14:1208816. doi: 10.3389/fmicb.2023.1208816
Edited by:
Mingsong Kang, Canadian Food Inspection Agency (CFIA), CanadaReviewed by:
Nar Singh Chauhan, Maharshi Dayanand University, IndiaZhengzhong Zou, Oregon Health and Science University, United States
Copyright © 2023 Zhang, Zhou, Tan, Jian, Jing, Wu, Zhang, Luo, Zhang and Sun. This is an open-access article distributed under the terms of the Creative Commons Attribution License (CC BY). The use, distribution or reproduction in other forums is permitted, provided the original author(s) and the copyright owner(s) are credited and that the original publication in this journal is cited, in accordance with accepted academic practice. No use, distribution or reproduction is permitted which does not comply with these terms.
*Correspondence: Juan Zhang, ODA1MTI0MjU3QHFxLmNvbQ==; Xin Sun, c3VueGluNkBmbW11LmVkdS5jbg==
†These authors have contributed equally to this work and share first authorship