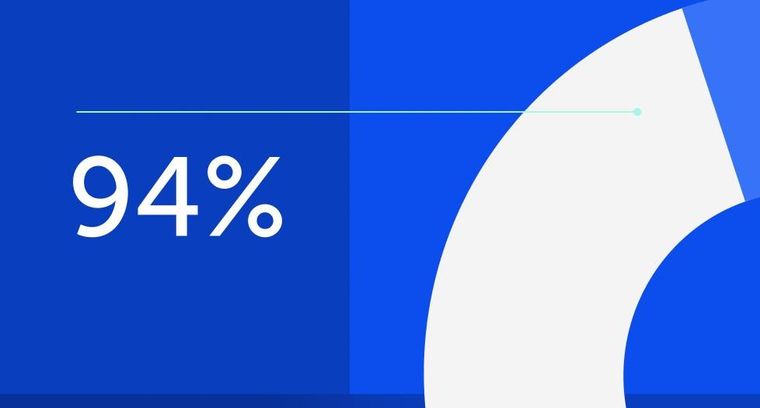
94% of researchers rate our articles as excellent or good
Learn more about the work of our research integrity team to safeguard the quality of each article we publish.
Find out more
REVIEW article
Front. Microbiol., 22 June 2023
Sec. Infectious Agents and Disease
Volume 14 - 2023 | https://doi.org/10.3389/fmicb.2023.1196774
This article is part of the Research TopicInsights in Infectious Agents and Disease: 2022View all 41 articles
Acinetobacter baumannii is increasingly associated with various epidemics, representing a serious concern due to the broad level of antimicrobial resistance and clinical manifestations. During the last decades, A. baumannii has emerged as a major pathogen in vulnerable and critically ill patients. Bacteremia, pneumonia, urinary tract, and skin and soft tissue infections are the most common presentations of A. baumannii, with attributable mortality rates approaching 35%. Carbapenems have been considered the first choice to treat A. baumannii infections. However, due to the widespread prevalence of carbapenem-resistant A. baumannii (CRAB), colistin represents the main therapeutic option, while the role of the new siderophore cephalosporin cefiderocol still needs to be ascertained. Furthermore, high clinical failure rates have been reported for colistin monotherapy when used to treat CRAB infections. Thus, the most effective antibiotic combination remains disputed. In addition to its ability to develop antibiotic resistance, A. baumannii is also known to form biofilm on medical devices, including central venous catheters or endotracheal tubes. Thus, the worrisome spread of biofilm-producing strains in multidrug-resistant populations of A. baumannii poses a significant treatment challenge. This review provides an updated account of antimicrobial resistance patterns and biofilm-mediated tolerance in A. baumannii infections with a special focus on fragile and critically ill patients.
Acinetobacter baumannii is an opportunistic pathogen causing severe nosocomial infections (Gonzalez-Villoria and Valverde-Garduno, 2016; Morris et al., 2019). The global estimated incidence rate of A. baumannii infections is approximately 1 million cases annually, with high crude mortality rates, particularly in critically ill patients (Peleg et al., 2008; Magill et al., 2014; Lob et al., 2016; Piperaki et al., 2019; Ma and McClean, 2021). Over the last 30 years, A. baumannii has emerged as one of the most troublesome pathogens for healthcare institutions, but it rarely causes disease outside of the healthcare setting (Wong et al., 2017). The clinical significance of A. baumannii has been raised due to its ability to acquire antibiotic resistance and tolerate desiccation. Indeed, multidrug-resistant (MDR), extensively drug-resistant (XDR), and A. baumannii isolates resistant to all clinically available antibiotics (pan-drug resistant—PDR) have been reported worldwide (Piperaki et al., 2019; Weinberg et al., 2020). The rates of MDR are approximately four times higher than those described for other major nosocomial pathogens (Hamidian and Nigro, 2019). Currently, 45% of all A. baumannii isolates are classified as MDR, with peaks of 70% in South America, Asia, and Europe (Giammanco et al., 2017; Hamidian and Nigro, 2019). These observations place A. baumannii among the most problematic nosocomial ESKAPE (Enterococcus faecium, Staphylococcus aureus, Klebsiella pneumoniae, Acinetobacter baumannii, Pseudomonas aeruginosa, and Enterobacter spp) pathogens, and a “high priority” by the World Health Organization (WHO) and Centers for Disease Control and Prevention [CDC; Tacconelli et al., 2018; Tiku, 2022].
The ability to acquire antibiotic resistance, the environmental persistence, along with the absence of identified toxins in its genome suggest that the virulence potential of A. baumannii resides in the ability to survive for prolonged periods throughout a hospital environment (Whiteway et al., 2022). Indeed, adhering to plastics allows A. baumannii to colonize endotracheal tubes or central venous catheters, thus increasing its persistence and transmission in hospitalized patients (Peleg et al., 2008; Roca et al., 2012). In particular, A. baumannii has been demonstrated to grow as a biofilm on different materials, including health-care-associated equipment, porcelain, stainless steel, rubber, endotracheal tubes, polycarbonate plastic, and polypropylene plastic (Greene et al., 2016a,b). Biofilm formation contributes significantly to establishing medical-device-associated infections conferring a high desiccation resistance and survival of A. baumannii isolates (Pour et al., 2011; Greene et al., 2016a,b). Recent reports also suggested that biofilm-producing A. baumannii strains are commonly isolated from intensive care units and in oncological patients (Zeighami et al., 2019; Asaad et al., 2021; Di Domenico et al., 2021; Roy et al., 2022). MDR A. baumannii (MDRAB) forms robust biofilms, both in the wound and on occlusive dressings in the skin and soft-tissue infections (Thompson et al., 2014). Notably, A. baumannii exhibits several adhesive and protective elements that significantly contribute to the formation and maintenance of biofilms, thus increasing tolerance to environmental stressors (Greene et al., 2016a,b). Biofilm is also important to the virulence of A. baumannii because it facilitates horizontal gene transfer (HGT) of antibiotic-resistance mobile elements while physically protecting bacteria from the immune system (Eze et al., 2018; Harding et al., 2018).
Infections caused by MDRAB in immunocompromised individuals result from complex relationships between several factors, including A. baumannii pathogenicity, the fitness costs of resistance, the site-specific microflora composition of the human host, and the selective forces following clinical interventions such as antibiotic therapy. Therefore, understanding the consequences of mutations driving antibiotic resistance and the worrisome convergence of virulent traits, including biofilm production, has important implications for controlling the spread of A. baumannii and developing novel treatment strategies in critically ill patients.
This review provides an updated analysis of antimicrobial resistance mechanisms and biofilm-mediated tolerance in A. baumannii. Moreover, we discuss current therapeutic options for carbapenem-resistant A. baumannii (CRAB) infections, with a special focus on fragile and critically ill patients.
Various studies have revealed that A. baumannii owns more human virulence potential than other Acinetobacter spp. In particular, A. baumannii resists macrophage uptake and grows better at 37°C than other species (Tayabali et al., 2012). Some elements, such as the outer membrane proteins (OMP), secretion systems, immunity interaction, or adhesion to the host cells, are highly characterized by virulence and pathogenicity in A. baumannii (Morris et al., 2019; Tiku, 2022).
Outer membrane proteins (OMPs) are a class of integral membrane proteins anchored in the outer membrane with a β-barrel structure. OmpA is one of the most abundant porins in the outer membrane of A. baumannii (Park et al., 2011; Uppalapati et al., 2020). OmpA is connected to the diaminopimelic acid of the peptidoglycan by two conserved residues (Asp271 and Arg286) in its periplasmic C-terminal domain (Park et al., 2012.). These characteristics give OmpA high stability in the membrane and the capability to fight against harsh environments (Moon et al., 2012). Indeed, being exposed to the outside of the bacterial cell OmpA provides the first line of contact between the bacterium and its surroundings. Given its central position, OmpA acts as an adhesion factor in virulence, channels for the uptake of nutrients, siderophore receptors, and enzymes such as proteases and lipases. Three OMPs were identified as fibronectin-binding proteins, such as OmpA, TonB-dependent copper receptor, and 34 kDa Omp (Smani et al., 2012). OmpA forms a non-selective channel in bacterial outer membranes that permits the passage of ions and other solutes (Sugawara and Nikaido, 2012; Confer and Ayalew, 2013). Furthermore, OmpA contributes to the antimicrobial resistance of A. baumannii (Sugawara and Nikaido, 2012; Smani et al., 2014). Indeed, disrupting the OmpA gene decreases the minimal inhibitory concentrations (MICs) of aztreonam, chloramphenicol, and nalidixic acid by 8, 8, and 2.7-fold, respectively. This data suggests that OmpA participates in the extrusion of antibiotics from the periplasmic space through the outer membrane and couples with inner membrane efflux systems (Smani et al., 2014). In A. baumannii, OmpA serves multiple functions, both in vitro and in vivo, including adherence to epithelia, induction of epithelial cell death, drug resistance, channels for the uptake of nutrients, siderophore receptors, binding to factor H (Choi et al., 2005, 2008; Gaddy et al., 2009; Kim et al., 2009). OmpA enhances the survival and persistence of A. baumannii by facilitating biofilm formation (Gaddy et al., 2009; Shin et al., 2009). In particular, outer membrane receptor proteins are significantly upregulated in biofilm than in planktonic cultures (Shin et al., 2009). Moreover, it has been reported that overexpression of OmpA represents a significant risk factor for pneumonia, bacteremia, and enhanced mortality in patients infected with A. baumannii (Sánchez-Encinales et al., 2017). In A. baumannii, virulence factors, including OmpA and certain tissue-degrading enzymes, are delivered to host cells via OMVs (Jin et al., 2011). OMVs are spherical elements with a 20–200 nm diameter, secreted by various Gram-negative pathogenic bacteria (Kulp and Kuehn, 2010). They mainly comprise lipopolysaccharide (LPS), outer membrane and periplasmic proteins, phospholipids, and nucleic acids, representing delivery vehicles for bacterial effectors to host cells (Ellis and Kuehn, 2010). OMVs are central in delivering A. baumannii virulence factors, including OmpA, and certain tissue-degrading enzymes, such as proteases and phospholipases (Lee et al., 2017). Furthermore, OmpA has the highest content in OMVs, which is involved in the mitochondrial decomposition of the host’s cell apoptosis (Choi et al., 2005; Tiku et al., 2021).
Phospholipases are lipolytic enzyme essential for phospholipid metabolism and a major virulence factor in many Gram-negative bacteria. Phospholipids are the primary building blocks of biological membranes and a carbon and energy source in the human host. In A. baumannii, have been identified two phospholipases C (A1S_0043 and A1S_2055) and three phospholipases D (PLD1, PLD2, PLD3), all with substrate specificity toward the eukaryotic membrane component phosphatidylcholine (PC; Flores-Díaz et al., 2016). PC is abundant in eukaryotic membranes representing 50% of all phospholipids and increasing up to 80% in the lung and tracheobronchial secretions (Girod et al., 1992; Bernhard et al., 2001; Tomaras et al., 2003) Experimental evidence suggests that it may serve as a nutrient source during lung infections by pathogens like Pseudomonas aeruginosa and A. baumannii (McConnell et al., 2013; Sun et al., 2014; Özarslan et al., 2023). Phospholipids’ degradation compromises the stability of host cell membranes, interfering with cellular signaling, thus resulting in changes in the host immune response (Flores-Díaz et al., 2016). In particular, the 1,2-diacylglycerol released by cellular phospholipases C plays roles in modifying biophysical membrane properties, including charge, fluidity, and permeability, and can recruit cytosolic proteins that induce spatial reorganization of signaling complexes, which in turn affect diverse cellular processes (Toker, 2005; Flores-Díaz et al., 2016). Consequently, products generated by bacterial phospholipases could affect the immune response and promote the infection’s establishment or progression (van der Meer-Janssen et al., 2010). In A. baumannii, phospholipases D concertedly promote serum resistance, epithelial cell invasion, and in vivo pathogenesis (Jacobs et al., 2010; Stahl et al., 2015). Interestingly, PLD1 and PLD2 appear to result from a gene duplication characterized by the HxKx4Dx6GSxN (HKD) pattern similar to eukaryotic cells and required for catalytic activity (Stahl et al., 2015). Despite their similarity, PLD2 is more important for invasion and virulence than the other two PLDs (Jacobs et al., 2010; Stahl et al., 2015). Since phospholipases are conserved across numerous strains of A. baumannii and are essential for host invasion, they may represent promising targets for developing enzyme inhibitors and potential vaccine candidates to limit the impacts on human diseases (Flores-Díaz et al., 2016).
The Type II secretion system (T2SS) is a two-step process, dependent on the general secretory pathway (Sec) or the Twin-arginine (Tat) system for substrate translocation to the periplasm before secretion in the extracellular environment (Weber et al., 2017). The T2SS was first described in A. baumannii ATCC17978, with the specific apparatus encoded by genes designated, general secretory pathway (GspA-O), located in six separate operons (Eijkelkamp, 2014). Secretion of type II effector proteins includes enzymes such as lipase, elastase, alkaline phosphatase, and phospholipases, which are essential for A. baumannii virulence (Elhosseiny and Attia, 2018). In A. baumannii, major T2SS effectors include the metalloendopeptidase, CpaA, and the lipases, LipA and LipH (Johnson et al., 2016; Weber et al., 2017). Secretion of CpaA and LipA requires specific membrane-associated chaperones CpaB and LipB (Zheng et al., 2013; Harding, 2016). In particular, LipA contributes to extracellular lipolytic activity by using long-chain fatty acids as carbon sources for growth and may use fatty acids derived through lipid hydrolysis as signaling molecules allowing bacterial escape from innate immunity (Johnson et al., 2016; Lee et al., 2017). In addition, CpaA is a zinc-dependent metalloendopeptidase forming an active complex with its chaperone (CpaAB), essential for secretion. It targets the common coagulation pathway by interfering with fibrinogen, factor XII and factor V, disrupting blood clotting and allowing the dissemination and colonization of A. baumannii (Waack et al., 2018; Urusova et al., 2019). Moreover, mutations in gspD and lipA showed a significant virulence reduction in both G. mellonella and murine models (Harding, 2016; Johnson et al., 2016).
Previous studies showed that A. baumannii strains produce a type VI secretion system (T6SS) involved in interbacterial competition (Fitzsimons et al., 2018). The T6SS is a complex nanomachine structurally and mechanistically analogous to an intracellular membrane-attached contractile phage tail (Cianfanelli et al., 2016). T6SS is an efficient weapon that can inject toxic effectors into the extracellular environment or directly into eukaryotic or prokaryotic cells (Cianfanelli et al., 2016). In addition, this system is implicated in bacterial competition and DNA uptake released by the prey cells, which promotes horizontal gene transfer (HGT; Weber et al., 2017). Indeed, HGT plays a significant role in the spread of antibiotic resistance cassettes and pathogenicity islands. Therefore, the potential involvement of T6SS in acquiring antibiotic resistance in A. baumannii has attracted considerable attention (Weber et al., 2017). It remains to be determined what, if any, benefit the T6SS may provide to A. baumannii during infection. In particular, G. mellonella infected with A. baumannii defective for the T6SS did not succumb to infection as quickly as did worms infected with the wild-type but were killed to the same extent at later time points (Repizo et al., 2015).
Increasing reports of the hospital- and community-acquired MDRAB infections are accumulating worldwide (Assimakopoulos et al., 2019; Girija and Priyadharsini, 2019; Darby et al., 2023; Mangioni et al., 2023). In addition to its intrinsic resistance to antibiotics, A. baumannii can acquire new functions by HGT, enabling rapid dissemination and maintenance of resistance genes between different isolates (Decré, 2012). Indeed, the European Centre for Disease Prevention and Control’s (ECDC) reported that from 2012 to 2020 in Europe, there had been an increase of 3.4% of A. baumannii strains resistant to fluoroquinolones, aminoglycosides, and carbapenems and an alarming rise of 11.3% (217 to 2,451 isolates) in Italy only.
The quinones/fluoroquinolones are antibiotics that inhibit two enzymes involved in DNA synthesis: DNA gyrases and Topoisomerase IV. A. baumannii has genetic mutations providing resistance. Mutations in the gyrA and parC genes of the DNA gyrase subunit and Topoisomerase IV subunit C play a major role in conferring direct antibiotic resistance (Roy et al., 2021). Other important antibiotic resistance mechanisms of A. baumannii involve efflux pumps, permeability defects, and alteration of the target site (Figure 1). More generally, three resistance-nodulation cell division (RND)-family efflux pump systems, such as AdeABC, AdeFGH, and AdeIJK, and the multi-antimicrobial extrusion protein family (MATE) efflux pump in A. baumannii are overexpressed due to amino acid substitutions in their regulatory genes (Fernandez and Hancock, 2013; Sun et al., 2014; Darby et al., 2023). These two systems allow a broad spectrum of antibiotic resistance to aminoglycoside, chloramphenicol, erythromycin, tetracycline, and tigecycline (Magnet et al., 2001; Fournier et al., 2006; Vila et al., 2007). The plasmid-encoded qepA gene is an efflux pump belonging to the major facilitator superfamily that decreases susceptibility to hydrophilic fluoroquinolones, especially ciprofloxacin (Jacoby et al., 2014). With less antibiotic resistance efficiency, mutations in the aminoglycoside transferase AAC(6′)-Ib-Cr by Tryp102Arg and Asp179Typ substitution permit N-acetylation modification of two fluoroquinolones (ciprofloxacin and norfloxacin; Roy et al., 2021; Venkataramana et al., 2022).
Figure 1. The main antibiotics resistance mechanisms of Acinetobacter baumannii. The resistance mechanisms are divided into six categories. (A) The permeability defects are due to porins modification, such as the carbapenem-associated outer membrane protein (CarO) and the OMP family. (B) The one-step or two-step drug extrusion from the cytosol to the outer membrane via the efflux pumps family. Among them, the resistance-nodulation-division superfamily (RND-superfamily) takes over the drug from the cytoplasm or the periplasm by its AdeABC, AdeIJK, or AdeFGH efflux pumps system. The major facilitator superfamily (MFS; e.g., TetA, TetB, CmlA, CraA, AmvA, AbaF), the multidrug and toxic compound extrusion (MATE) transporter family (e.g., AbeM), and the small multidrug resistance (SMR) transporter (e.g., AbeS) are H+ and Na+ coupled multidrug efflux pumps at the inner membrane. (C) The hydrolysis of β-lactam antibiotics by β-lactamases. Acinetobacter baumannii β-lactamases are classified into four molecular classes: class A (e.g., TEM, GES, PER, CTX-M, SCO, VEB, KPC, CRAB enzyme family), class B (e.g., IMP, VIM, NDM, SIM enzyme family), class C (e.g., Amp family) and class D (e.g., OXA subgroups enzyme family). (D) The complete loss of LPS by inactivating the lipid A biosynthesis genes (lpxA, lpxC, and lpxD) results in colistin resistance. (E) The aminoglycoside-modifying enzymes classified in three class acetyltransferases [e.g., AAC3, AAC(6′)], adenyltransferases [e.g., ANT(2″), ANT(3″)], and phosphotransferases [e.g., APH(3″), APH(3′)]. (F) The alteration of targeted sites of TetM confers ribosomal protection against tetracycline, and GyrA subunit modification of DNA gyrase confers resistance to quinolone.
The aminoglycosides antibiotic family inhibits protein synthesis by binding to the 16S ribosomal RNA of the 30S ribosome, with high affinity. Two main mechanisms, involving aminoglycoside modifying enzymes and RNA 16S methylase modification, are associated with increased resistance. Several reports reviewing clinical A. baumannii isolates find a match in genes coding for aminoglycosides enzymes modification ant(3″)-I, aac(3)-I, aph(3′)-I, aac(6′)-Ib and aph(3′)-IIb; and a gene coding for an rRNA 16S methylase armA allowing a high antibiotic resistance (Nie et al., 2014; Hasani et al., 2016).
Carbapenems are the most important class of antibiotics against A. baumannii and, generally, for Gram-positive and negative isolates (Meletis, 2016). Indeed, carbapenems are considered the drugs of choice to treat A. baumannii infections and the first-line agents for empirical therapy in areas with low rates of resistant strains (Pandey and Cascella, 2022). However, different mechanisms of β-lactam resistance have been described resulting in overexpression of OXA β-lactamases and chromosomal cephalosporinases, which have been classified as Acinetobacter-derived cephalosporinases (ADCs; Paton et al., 1993). The ADCs overexpression is caused by an insertion sequence (ISAba1) close to these resistance genes (Heritier et al., 2006). The first ADC gene was reported in Spain in 2000 (Bou and Martínez-Beltrán, 2000). Currently, several variants have been described worldwide conferring resistance against penicillins, extended-spectrum cephalosporins, monobactam (aztreonam), and β-lactamase inhibitors (sulbactam; Rodríguez-Martínez et al., 2010; Tian et al., 2011; Kuo et al., 2015; Ingti et al., 2020). The extensive use of carbapenems has been regarded as one of the main risk factors promoting the emergence and spread of MDRAB (Garnacho-Montero et al., 2015). The most effective resistance mechanism is the acquisition of carbapenem-hydrolyzing enzymes. In CRABs, the most common are class D oxacillinases (OXA type) β-lactamases classified in subgroups, with more than 400 OXA-type enzymes identified. Specifically, OXA-23, OXA-24, OXA-51, and OXA-58 subgroups are widespread in A. baumannii (Evans et al., 2013). Nevertheless, other β-lactamases classes are involved in carbapenem resistance, such as class A β-lactamases and class B metallo-β-lactamases (MBLs; Smet et al., 2008). OXA-type β-lactamases (especially OXA-23) have also been commonly detected in cefiderocol-resistant A. baumannii clinical isolates (Iregui et al., 2020; Kohira et al., 2020; Abdul-Mutakabbir et al., 2021; Yamano et al., 2021). Moreover, PER-like β-lactamases and, to a lesser extent, NDM β-lactamases have been shown to contribute to a decreased susceptibility to cefiderocol (Poirel et al., 2021). Therefore, combined factors, including the presence of β-lactamases such as NDM-like enzymes, modification of the penicillin-binding proteins (target gene PBP-3), permeability defects associated with efflux overexpression and reduced expression or mutation of genes involved in the ion transport, might contribute to resistance to cefiderocol in A. baumannii (Malik et al., 2020; Wang et al., 2022). More seldom is the presence of mutations affecting iron transport genes (pirA and piuA) in cefiderocol-resistant A. baumannii isolates (Malik et al., 2020). The pirA and piuA genes encode components of the pyoverdine and ferric iron uptake systems, respectively. Cefiderocol is transported across the outer cell membrane via iron transporters; thus, mutations in these genes may reduce antibiotic susceptibility. Nevertheless, the finding that mutations in these iron transport genes are relatively rare in A. baumannii isolates may suggest that iron acquisition is central to A. baumannii survival and, at the same time, genes involved in drug efflux, cell envelope modification, and cell wall biosynthesis may be more efficient in providing resistance to cefiderocol (Moynié et al., 2017).
The increase in colistin treatments after the rise of CRAB has led to a critical emergence of resistant strains, particularly in the hospital environment (Katip et al., 2021a,b). The first recorded case of a colistin-resistant Acinetobacter sp. was in 1949 in the Czech Republic (Sun et al., 2020). Currently, the high-resistant clonal lineage of A. baumannii has been described across 12 hospitals in Italy, Greece, and Spain, with resistance rates for colistin of 50% (Nowak et al., 2017). Moreover, 42% of A. baumannii isolates causing bloodstream infections in intensive care unit (ICU) patients from a Greek hospital have been found resistant to colistin and directly linked to fulminant septic shock and high mortality (Papathanakos et al., 2020). Despite that discovery, the resistance mechanisms to colistin in A. baumannii are only partially understood. Colistin is positively charged and interacts electrostatically with the negatively charged phosphate groups of lipid A, the LPS component of Gram-negative bacilli outer membrane. Colistin’s binding causes displacement of calcium (Ca2+) and magnesium (Mg2+) ions, associated with lipid A phosphoresters, thus affecting the stability of the LPS molecules. Subsequently, colistin inserts its hydrophobic terminal acyl fatty chain, causing disruption and permeabilization of the outer membrane. When permeabilization occurs, colistin penetrates the outer membrane, affecting the integrity of the inner membrane’s phospholipid bilayer, leading to membrane destabilization and cell death (Rhouma et al., 2016; Novović and Jovčić, 2023). Unlike Gram-negative bacteria such as Salmonella spp., Escherichia coli, Klebsiella pneumoniae, and Pseudomonas aeruginosa, A. baumannii does not possess a PhoP/PhoQ two-component system. The primary polymyxin resistance mechanisms in A. baumannii relies on the PmrA/PmrB two-component system. The PmrA/PmrB is a major regulatory system implied in the lipid A modification (Hua et al., 2020) and is well-characterized in E. coli, P. aeruginosa, or K. pneumoniae (Chen and Groisman, 2013). The histidine-kinase PmrB sensor reacts to various stress conditions, such as low Mg2+ and Ca2+ concentrations, acid pH, and high Fe3+ concentrations (Figure 2).
Figure 2. Model for activation of the polymyxin resistance PmrA/PmrB two-component system in Acinetobacter baumannii. Resistance to polymyxins can be induced in response to various stress conditions, such as low Mg2+ and Ca2+ concentrations, acidic pH, and high Fe3+ concentrations, which activate the two-component system PmrA/PmrB. Once activated, PmrA/PmrB upregulates pmrC gene expression, which encodes lipid A phosphoethanolamine (PEtN) transferase that promotes the addition of PEtN to lipid A. PmrC upregulates naxD, which codes for an N-acetylhexosamine deacetylase involved in the deacetylation of the β-galactosamine and Lipid A modification. Alternatively, overexpression of the eptA gene, homolog to PmrC, promotes the addition of the cationic pEtN moiety to the lipid A of LPS. Lastly, the plasmid-mediated mobile colistin resistance (mcr) genes encode a phosphoethanolamine transferase that adds PEtN to lipid A residues lowering the binding affinity of colistin to its target site.
In Gram-negative bacteria, resistance to polymyxins results mostly from LPS modifications, which is the drug target. These modifications originate from the addition of cationic groups such as 4-amino-L-arabinose (L-Ara4N) and/or phosphoethanolamine (PEtN) on the lipid A (Ezadi et al., 2019). Unlike Enterobacterales, A. baumannii lacks all the genes of the arn operon required for L-Ara4N biosynthesis. Consequently, colistin resistance is caused by the addition of PEtN to the lipid A on position 1 or 4′ by the chromosomally-encoded EptA-like phosphoethanolamine transferase by the pmrC gene (El-Sayed Ahmed et al., 2020).
Mutations in the PmrAB system have been found in a large number of colistin-resistant A. baumannii isolates. These mutations constitutively activate the PmrAB regulatory system, which in turn, upregulates the expression of the operon pmrCAB (Adams et al., 2009). The self-regulation of the pmrCAB transcription enables the modification of lipid A (Olaitan et al., 2014). The colistin resistance-related mutations in the coding sequence for the amino acids Pro102 and Ile13 of PmrA and Pro233, Thr235, and Gln270 of PmrB caused an overactivity of PmrA. These mutations in pmrA were located in the sulfatase domain, while in pmrB were in the histidine kinase domain. Mutations in pmrA-pmrB promote phosphorylation of the PmrB receptor kinase, activating PmrA. The activated PmrA modulates the expression of the pmrC gene that encodes the phosphoethanolamine transferase that catalyzes the addition of PEtN to the 1′- or 4′-phosphate group of lipid A (Sun et al., 2020). Another study, analyzing the genetic determinants associated with colistin resistance in A. baumannii isolates collected from various regions of Greece, identified additional mutations in PmrB (Glu140 or Leu178) and PmrA (Lys172 or Asp10) genes (Palmieri et al., 2020). Besides, PmrA also regulates the naxD transcription coding for an N-acetylhexosamine deacetylase which is involved in the deacetylation of the β-galactosamine modifying the Lipid A (Adams et al., 2009; Moffatt et al., 2010; Llewellyn et al., 2012; Deveson Lucas et al., 2018; Sun et al., 2020; Ilsan et al., 2021).
Recently, a plasmid-mediated resistance to polymyxin has been described in Enterobacterales. The mcr (mobile colistin resistance) genes also encode a phosphoethanolamine transferase that adds PEtN to lipid A (Partridge et al., 2018). The mcr-1 remains the predominant plasmid-mediated colistin resistance gene, while mcr-2, -3, -4, -5, -6, -7, and -8 have been detected in isolates from animals, humans, and different environments worldwide. Currently, 56 mcr variant sequences are available in GenBank (Partridge et al., 2018). The mcr genes initially found in Enterobacterales, have been only recently described on A. baumannii (Ma et al., 2019; Al-Kadmy et al., 2020), for which resistance to polymyxin was previously restricted to chromosome-encoded elements (Jeannot et al., 2017; Poirel et al., 2017; Partridge et al., 2018).
More recently, a colistin-resistant mutation has also been shown in A. baumannii by insertion into the hns gene, an H-NS family transcriptional regulator. That mutation alters the expression of more than 150 genes, including the eptA gene. Overexpression of this LPS modifying enzyme codes for EptA, a PEtN transferase homolog to PmrC, which confer colistin resistance (Deveson Lucas et al., 2018; Trebosc et al., 2019; Palmieri et al., 2020; Ilsan et al., 2021).
Instead of lipid A modification, A. baumannii can acquire resistance to colistin due to the complete loss of LPS by inactivating the lipid A biosynthesis genes (lpxA, lpxC, and lpxD; Moffatt et al., 2010; Cafiso et al., 2019). LpxA, LpxC, and LpxD are three enzymes involved in the first main steps of LPS biosynthesis of A. baumannii occurring in the cytoplasm compartment (Powers and Trent, 2018). Specifically, mutations in LpxA and LpxC can lead to modifications in the fatty acid chains of lipid A, while mutations in LpxD can affect the addition of PEtN groups to lipid A. These changes can reduce the outer membrane’s net negative charge and permeability, decreasing colistin susceptibility (Palmieri et al., 2020).
A. baumannii forms biofilms on a wide range of surfaces, including medical and ventilator-associated pneumonia (VAP), as well as on host epithelial cells leading to meningitis, pneumonia, urinary tract infection, sepsis, and other conditions (Greene et al., 2016a,b; Wong et al., 2017). Biofilm contributes to A. baumannii survival on surfaces and in dry and nutrient-deprived conditions for several weeks (Orsinger-Jacobsen et al., 2013; Chapartegui-González et al., 2018). The current understanding suggests biofilm formation in A. baumannii is a complex process mediated by a large repertoire of molecules and two-component systems (Mcconnell et al., 2013; Wong et al., 2017; Roy et al., 2022).
Generally, biofilm production relies on the initial reversible bacterial attachment to a surface in response to environmental stimuli (Toyofuku et al., 2016). Thus, early adhesion is essential in the colonization process and in establishing an A. baumannii infection. The CsuA/BABCDE chaperon-usher assembly system encodes for the bacterial pili that mediate the attachment of A. baumannii to various abiotic surfaces (Longo et al., 2014). The Csu pili comprise four protein subunits, CsuA/B, CsuA, CsuB, and CsuE, assembled via the chaperone–usher pathway (Tomaras et al., 2008). The CsuC chaperone assists the CsuA/B polymerization in forming the major pilus subunit (Pakharukova et al., 2015). In addition, CsuD functions as the usher, CsuE forms a tip adhesin, while CsuA and CsuB constitute minor pilin subunits (Tomaras et al., 2003, 2008; Pakharukova et al., 2015). Previous studies showed that the inactivation of the csuE gene abolishes pilus production and biofilm (Amala Reena et al., 2017; Ghasemi et al., 2018). However, a study conducted with 52 different clinical strains revealed that biofilm formation and the ability to attach host cells are independent abilities and not necessarily associated (Eijkelkamp et al., 2011). Indeed, most A. baumannii carry the csuA/BABCDE locus; nevertheless, a subset of clinical isolates is csu deficient, indicating that these pili may be dispensable for biofilm formation and maintenance and that other pili systems may functionally replace them (Wright et al., 2016). In A. baumannii, the expression of the csu operon is mainly regulated by the two-component system BfmRS where BfmS acts as a sensor kinase and BfmR functions as a response regulator (Tomaras et al., 2008; Gaddy and Actis, 2009). Indeed, the two-component system BfmRS is considered the master regulator of resistance to stress in A. baumannii (Law and Tan, 2022). BfmrR-P can act directly or indirectly on regulating genes for osmotic and oxidative stress, heat shock, the biosynthesis of siderophores, and the production of capsular polysaccharides, in addition to pili production. Furthermore, BfmR is also important for pellicle formation in A. baumannii (Krasauskas et al., 2019). A pellicle is an alternative biofilm growing at the air-liquid interface that may favor the colonization and persistence of A. baumannii in respiratory tracts, humidifiers, and moist surfaces (Martí et al., 2011; Nait Chabane et al., 2014).
The two-component system BfmRS is also responsible for the subsequent irreversible adhesion starting with the production of factors under the control and early extracellular DNA (eDNA) release. An early eDNA release was demonstrated to be responsible for the first tridimensional biofilm formation. Notably, eDNA release is independent from the cell lysis in the early stage of biofilm formation and is mediated by membrane vesicles (Sahu et al., 2012).
In A. baumannii, the AdeABC, AdeIJK, and AdeFGH RND-type efflux systems are critical in biofilm formation (Coyne et al., 2011). Mutant strains of AdeABC, AdeIJK, and AdeFGH efflux pumps produce a significantly lower level of biofilm than the wild-type strain (Yoon et al., 2015). Moreover, mutation of AdeABC and AdeIJK efflux pumps showed lower expression of several pilus system-encoding proteins, including CsuA/B, CsuC, and FimA. These proteins play a central role in the initial stages of adhesion, surface colonization, and biofilm maturation in A. baumannii (He et al., 2015; Shadan et al., 2023).
The AdeRS two-component system regulates the AdeABC efflux pump’s expression (Richmond et al., 2016; Xu et al., 2019). In particular, the deletions of adeRS and adeB reduced the biofilm growth of A. baumannii without affecting the number of adherent cells. This observation suggests that cells might be unable to produce a mature biofilm without this efflux pump (Richmond et al., 2016). After the initial surface attachment, biofilm maturation occurs. During this process, individual cells produce the biofilm matrix entering the irreversible attachment stage. In A. baumannii, biofilm maturation is modulated by the Biofilm-associated proteins (Bap) and their interaction with the extracellular polymeric substances (EPS; Soroosh et al., 2020; Upmanyu et al., 2022). The main elements of the A. baumannii EPS are alginates and poly-β-(1-6)-N-acetylglucosamine (PNAG) compounds that interact with each other, with ions or heterologous molecules to form an elastic structure (Marvasi et al., 2010). The pgaABCD locus is involved in the synthesis of PNAG, facilitating cell adhesion, promoting biofilm integrity, and limiting desiccation (Choi et al., 2009; Morris et al., 2019; Flannery et al., 2020). Accordingly, deleting the pgaABC genes in A. baumannii impairs biofilm formation (Choi et al., 2009). The Bap are large surface proteins orthologous to the Staphylococcus aureus Bap protein (Cucarella et al., 2001; Loehfelm et al., 2008). A type I secretion system secretes Bap. It is required in cell-to-cell adhesion and for developing higher-order structures on polystyrene and titanium (Loehfelm et al., 2008; Harding et al., 2017). Moreover, the Bap protein increases host colonization by facilitating A. baumannii adherence to human neonatal keratinocytes and bronchial epithelial cells (Brossard and Campagnari, 2012). In addition to the Bap protein, the AdeABC efflux pump, normally related to antibiotic resistance, may also contribute to biofilm maturation (Richmond et al., 2016). Notably, in mature A. baumannii biofilms, can be observed two types of colonies: the avirulent translucent (AV-T) colonies that produce dense biofilms and virulent opaque (VIR-O) colonies that exhibit low biomass but enhanced virulence in G. mellonella, increased surface motility an antibiotic resistance phenotype (Tipton et al., 2015). Several genes are linked to different genomic expression profiles. Among them, ABUW_1132, a highly conserved gene that encodes a LysR-type transcriptional regulator (LTTR) that contributes to the passage of AV-T to VIR-O; its overexpression up-regulates abaI and activates the abaI/abaR quorum sensing (QS) signal (Tierney et al., 2021). In A. baumannii, the QS system is regulated by the two-component system, AbaI/AbaR, which is homologous to the typical LuxI/LuxR system found in other Gram-negative bacteria. abaI encodes the autoinducer synthase, which catalyzes the synthesis of N-(3-hydroxy dodecanol)-L-HSL (AHL), which at high density interacts with the cognate receptor AbaR leading to downstream cellular responses (Oh and Han, 2020). Previous studies have found that abaI and abaR disruption reduces biofilm formation (Niu et al., 2008; Anbazhagan et al., 2012). Moreover, A. baumannii cultured in the presence of AHL showed increased expression of Csu pili and biofilm formation (Luo et al., 2015).
In the final stage, the cells within the biofilm disperse and colonize new surfaces. Biofilm dispersal is induced prevalently under environmental stress, including the A. baumannii SOS response, and the activation of the UmuDAb RecA-dependent repressor inactivated by RecA cleavage when DNA damage occurs. As a result, the UmuDAb mutant cannot activate the transcription of bmfR. Thus, no Csu pili or biofilm is formed (Ching et al., 2019). Notably, dispersed cells exhibit variable phenotypes, antibiotic susceptibility, transcriptomic patterns, and metabolic activities (Rumbaugh and Sauer, 2020). For example, dispersed clinical isolates of A. baumannii are more hydrophobic and adhere more efficiently to the surface than the planktonic cells (Berlanga et al., 2017). Moreover, the dispersed cells were more susceptible to ciprofloxacin and tetracycline than the same cells in the planktonic state (Berlanga et al., 2017). In contrast, another A. baumannii clinical strain disseminating from ciprofloxacin-exposed biofilms is highly resistant to ciprofloxacin, erythromycin, and tetracycline (Penesyan et al., 2019). These studies suggest that the ability of the dispersed cells to evolve, acquiring higher antibiotic resistance, could complicate the management and treatment of the infection (Law and Tan, 2022).
The ability of A. baumannii to form biofilms has been reported as an essential factor contributing to its persistence and tolerance to antimicrobial agents (Roy et al., 2022). The proportion of A. baumannii clinical isolates that produce biofilms can vary significantly depending on the study and sample population. In a collection of 20 clinical isolates of A. baumannii, emerged that 80% of the strains formed biofilm, perhaps because of a dominant clone (Sechi et al., 2004). Bardbari et al. compared biofilm-production ability between clinical and environmental A. baumannii. In this study emerged that the majority of both clinical and environmental isolates could form varying degrees of biofilm. Specifically, the prevalence of strong biofilm producers in clinical and environmental strains was 58.7 and 31.2%, respectively (Bardbari et al., 2017). Others reported that among 154 A. baumannii isolated in Taiwan, 45.4% possessed strong biofilm formation ability (Yang et al., 2019). Moreover, among 100 A. baumannii clinical isolates from three hospitals in Iran, 58% were strong biofilm producers (Zeighami et al., 2019). Another study investigating 92 unrelated strains of A. baumannii isolated from two Spanish hospitals found that 63% of isolates formed biofilm, mainly from device-associated infections. Notably, these isolates were less frequently resistant to imipenem or ciprofloxacin than non-biofilm-forming isolates (Rodrı́guez-Bano et al., 2008).
A study from 4 Chinese hospitals analyzed the contribution of biofilm formation in the epidemic spread of A. baumannii by comparing biofilm-forming abilities and genetic characteristics of international clonal lineage II (ICL II) and non-ICL II isolates. From a total of 114 clinical A. baumannii isolates, collected from various specimens, including blood, sputum, urine, and wound, emerged that 36% of the clinical isolates were able to form biofilm, but only 19.5% were strong biofilm producers. Of the A. baumannii isolates, the biofilm formation capacity of ICL II was significantly lower than that of non-ICL II isolates. The authors concluded that biofilm formation might not be a critical factor for the epidemic spread of A. baumannii, particularly for the ICL II lineage. They suggested that other factors, such as antimicrobial resistance and virulence, could play a more critical role in the epidemic potential of A. baumannii (Hu et al., 2016). Despite the propensity to produce biofilm, the clinical impact of biofilm in A. baumannii isolates is still debated. Indeed, a recent multicenter study in Taiwan including 711 patients showed that higher APACHE II score, shock status, lack of appropriate antimicrobial therapy, and carbapenem resistance were independent risk factors of 28-day mortality in the patients with A. baumannii bacteremia but not the level of biofilm formation. In addition, biofilm formation was most commonly observed in survivors than in non-survivors (38.4% vs. 31.9%; Chiang et al., 2022). Similar results have been previously observed in a cohort of 273 patients with A. baumannii bacteremic pneumonia (Wang et al., 2018). Accordingly, other studies have shown that infections caused by biofilm-producing A. baumannii are not necessarily associated with worse clinical outcomes (Rodrı́guez-Bano et al., 2008; Wang et al., 2018). Therefore, the impact and pathogenesis of biofilm production remain elusive and, in many cases, related to the patient’s underlying condition or to the strain that causes the infection (Rodrı́guez-Bano et al., 2008; Barsoumian et al., 2015; Wang et al., 2018; Di Domenico et al., 2020, 2021).
A. baumannii is known for its ability to develop resistance to multiple antibiotics, making treatment of infections particularly challenging. In addition, the formation of biofilms further exacerbates this issue, as the extracellular matrix can act as a physical barrier, limiting the penetration of antibiotics and protecting the bacteria from the host’s immune system (Perez et al., 2007; Antunes et al., 2011). Several antibiotics and antibiotic combinations have shown promise in combating A. baumannii biofilms. However, their effectiveness may vary depending on the strain and resistance profile. The use of two or more antibiotics with different mechanisms of action can enhance the therapeutic efficacy by affecting multiple bacterial targets. In particular, the combination of colistin and rifampicin was more effective at eradicating biofilms formed by multidrug-resistant A. baumannii isolates than either antibiotic alone (Batoni et al., 2016). The antimicrobial combinations of colistin-levofloxacin, colistin-tigecycline, and tigecycline-levofloxacin or these combinations with clarithromycin were effective as lock solutions in the treatment of A. baumannii catheter-related infections (Ozbek and Mataraci, 2013). Nevertheless, candidate antibiotics were active against biofilm-embedded A. baumannii cells at 400-fold the MIC. This concentration is unachievable in human serum, making those antimicrobials an undesirable option for systemic use in A. baumannii biofilm-associated infections (Ozbek and Mataraci, 2013). Synergistic effects were also observed on biofilm-embedded carbapenem-resistant and carbapenem-susceptible A. baumannii strains. In particular, meropenem was active against biofilm-embedded carbapenem-susceptible A. baumannii, whereas meropenem plus sulbactam exhibited synergism against biofilm CRAB and caused significantly more damage to the biofilm architecture than colistin or tigecycline used alone (Wang et al., 2016). Additionally, clinical isolates of MDRAB exhibited different degrees of biofilm formation in the presence of sub-minimum inhibitory concentrations of colistin and tigecycline (Sato et al., 2018). A recent study showed that biofilm-embedded MDRAB had been eradicated with colistin but not tigecycline. Notably, the eradication increased with a combination of colistin and high concentrations of tigecycline (Sato et al., 2021). Moreover, combining azithromycin and polymyxin B displayed synergistic activity against biofilm-producing A. baumannii clinical isolates, improving antimicrobial efficacy (Peng et al., 2020). These data suggest that the effects of different antibiotics may depend on bacterial strains and the response of A. baumannii may vary under specific environmental stress conditions, such as in the presence of multiple antimicrobial agents. Nevertheless, one of the main challenges in analyzing these studies is the considerable heterogeneity in the design, methodologies, and patient populations examined (Table 1). While reflecting the field’s richness, such diversity can make it difficult to draw firm conclusions or compare findings directly across studies. Additionally, there is not yet a universally accepted definition or a standardized method for determining biofilm formation by A. baumannii. The absence of such standards introduces variability between studies and complicates the comparison of results. Furthermore, many of our findings are based on in vitro studies. While these studies provide valuable insights, they cannot fully capture the complexity of clinical infections. The behavior of A. baumannii in a real-world clinical setting can be influenced by myriad factors not present under laboratory conditions.
Table 1. Activity of different antibiotics against carbapenem-resistant Acinetobacter baumannii (CRAB).
The two most common clinical manifestations of A. baumannii are nosocomial pneumonia, particularly VAP, and bacteremia (Wong et al., 2017). While an endotracheal tube allows Acinetobacter spp. to establish biofilm facilitating its transmission and spread in the environment, the development of VAP occurs due to the aspiration of bacterial droplets directly into the alveoli. Likewise, bacteremia occurs as a hematogenous spread from pneumonia or in the presence of an infected central venous catheter. Less commonly, A. baumannii causes urinary tract infections (often associated with the presence of urinary catheters), central nervous system infections (often after neurosurgery or in the presence of external ventricular drain), wound or bone infections (often after surgery or trauma; Wong et al., 2017). Typically, infections sustained by A. baumannii occur in intensive care units, where patients are characterized by critical illness, multimorbidity, prolonged hospital stay, exposure to multiple invasive procedures, and prolonged antibiotic therapy (Ogutlu et al., 2014; Ayobami et al., 2020; Ibrahim et al., 2021).
During the COVID-19 pandemic, MDR organisms, particularly CRAB, have been increasingly reported as causative agents of secondary infections, especially in severe and critical diseases (Patel et al., 2021; Cogliati Dezza et al., 2022; Russo et al., 2022; Langford et al., 2023). Furthermore, CRAB acquisition increased during the hospital stay and accounted for high mortality rates in patients with COVID-19 (Falcone et al., 2021; Iacovelli et al., 2023). Increased antibiotic resistance, reported for clinical isolate, is even more significant in oncological patients (Ñamendys-Silva et al., 2015; Nazer et al., 2015; Cornejo-Juárez et al., 2020). A previous report highlights that among 635 oncological patients, 6.1% were infected by A. baumannii MDR (Nazer et al., 2015). An oncology department in China demonstrated that A. baumannii accounted for 9.8% of infections (Li and Wang, 2018). Two studies have shown that 19% of patients died within 72 h after A. baumannii isolation (Nazer et al., 2015; Cornejo-Juárez et al., 2020).
Therefore, CRAB represents a threat to the most vulnerable patients, contributing to the observed high mortality, which reaches values up to 50%–70% in patients with septic shock and VAP (Iovleva et al., 2022). Furthermore, despite sharing similar comorbidities and risk factors, patients infected with CRAB or XDR strains had a significantly higher mortality rate than those caused by susceptible strains (Lee et al., 2014; Lemos et al., 2014). A recent study further highlighted that the absolute excess 30-day mortality due to infection sustained by PDR A. baumannii compared to only PDR A. baumannii colonization was 34%, suggesting that one of every three treated patients would have been saved if effective drugs were available (Karakonstantis et al., 2020).
Despite being a strong biofilm producer, it has been shown that biomass production was not an independent risk factor for 28-day mortality in patients with A. baumannii bacteremia (Chiang et al., 2022). Indeed, one of the major drivers of mortality is the inappropriate initial effective therapy, which mainly depends on the high resistance level in A. baumannii. Currently, there is still no consensus on the optimal treatment of CRAB infections (Paul et al., 2022; Tiseo et al., 2022). Colistin has been considered the backbone of CRAB treatment for many years, mostly in combination with carbapenems, fosfomycin, tigecycline, or ampicillin/sulbactam or even with vancomycin and/or rifampin (Durante-Mangoni et al., 2013; Ceccarelli et al., 2015; Oliva et al., 2017; Giacobbe et al., 2020; Katip et al., 2020). Colistin is administered as an inactive prodrug, colistimethate (also known as colistin methanesulfonate, CMS). International consensus guidelines and recent studies highly recommend administering CMS as a loading dose (LD) followed by a maintenance dose for the treatment of infections due to carbapenem-resistant Gram-negative bacilli, especially in critically ill patients (Tsuji et al., 2019; Wang et al., 2022). A recent study evaluated the efficacy and safety of using a CMS LD in the treatment of critically ill patients with CRAB infections and showed higher clinical, microbiological, and 30-day survival rates in patients receiving LD compared with patients not receiving LD; however, the administration of the LD was associated with a higher risk of nephrotoxicity (Katip et al., 2021a,b).
Colistin use is limited by the risk of nephrotoxicity if administered at clinically effective dosage (Ordooei Javan et al., 2015) and the relatively poor lung epithelial lining fluid (ELF) penetration in critically ill patients (Imberti et al., 2010). Furthermore, resistance to colistin may occur in up to 30% of CRAB strains (Iovleva et al., 2022), rendering the treatment of CRAB infections even more challenging. In any case, the rate of colistin resistance is lower than that of tigecycline (45.5%; Chang et al., 2012; Muthusamy et al., 2016), suggesting this antibiotic still represents an effective antimicrobial agent against CRAB infections (Katip et al., 2021a,b).
Sulbactam is an irreversible competitive beta-lactamase inhibitor with direct antimicrobial activity thanks to its intrinsic affinity for the A. baumannii PBPs (Tamma et al., 2022). In addition, when given in high doses, sulbactam has the ability to saturate PBP-1 and PBP-3 and may therefore overcome the increasing described rates of sulbactam resistance in CRAB (Bartal et al., 2022).
In recent years, cefiderocol, a novel siderophore cephalosporin, has been approved by the Food and Drug Administration to treat serious infections caused by carbapenem-resistant Gram-negative bacteria (US Food and Drug Administration, 2019) and represented an encouraging advancement, especially for the treatment of CRAB infections. While the phase 3 randomized clinical trial CREDIBLE-CR, which compared cefiderocol with the best available therapy, showed higher mortality in the subgroup of patients with CRAB treated with cefiderocol (Bassetti et al., 2021), subsequent real-world observations from case series or observational studies showed promising results of cefiderocol in terms of efficacy (Oliva et al., 2020; Bavaro et al., 2021; Rando et al., 2021; Falcone et al., 2022) and safety (Pascale et al., 2021). This advantage was more evident in patients with bloodstream infections than those with VAP (Falcone et al., 2022), probably due to a sub-optimal penetration of cefiderocol in the ELF at current dosages (Gatti et al., 2021). However, the possibility of developing resistance to this drug under treatment, associated with an observed higher microbiological failure than the best available therapy (Falcone et al., 2022), requires caution and deserves further prospective studies to define cefiderocol optimal place in therapy toward CRAB infections (Volpicelli et al., 2021).
Given the limited therapeutic options with conventional antibiotics, there is ongoing research on alternative or adjuvant strategies for treating CRAB infections. In particular, N-acetylcysteine (NAC) exhibited high in-vitro activity against both planktonic and biofilm CRAB (Pollini et al., 2018; De Angelis et al., 2022), while a recent clinical observation showed a survival benefit of intravenous NAC addition to antibiotics in critically ill patients with CRAB septic shock (Oliva et al., 2021).
A. baumannii has emerged as an opportunistic pathogen responsible for a broad range of severe nosocomial infections. Much of A. baumannii’s success can be directly attributed to its genome plasticity, which rapidly mutates under stress. The ability to resist most last-line antimicrobial agents poses a considerable challenge, especially in critically ill patients.
In particular, the dissemination of CRAB and the increase in the use of colistin has led to a critical emergence of resistant strains. However, several virulence mechanisms beyond canonical drug resistance were recently identified, enabling A. baumannii to thrive in the healthcare environment. Indeed, it has been observed that A. baumannii can contaminate hospital surfaces or devices, caregivers’ hands, and can be spread by asymptomatically colonized persons. In addition, desiccation resistance, surface adherence, and biofilm formation make A. baumannii outbreaks in acute care hospitals difficult to control.
The environmental persistence has probably contributed to the increase in the incidence of A. baumannii from COVID-19 patients highlighting the value of appropriate prevention and control practices, particularly in open-space ICUs. During the COVID-19 pandemic, decreased vigilance for MDR control of transmissions, suspension or limitation of the hospital infection control committees, reduced surveillance, and personnel numbers likely contributed to the increase in hospital-acquired infections caused by A. baumannii. Notably, this review focuses on critically ill patients, a population particularly vulnerable to A. baumannii infections. Nevertheless, these infections also occur in other patient populations, and some of the data and conclusions herein presented may not be universally applicable.
Therefore, rapid diagnostic tests to identify and track high-risk clones, and antibiotic resistance genes, together with appropriate antibiotic regimens and strict adherence to infection control measures, may represent priorities for effectively dealing with A. baumannii infections.
IC, AO, FS, GF, MT, MP, and ED contributed to the review’s conception and design. IC, AO, FS, RP, FP, and ED researched and wrote the review. All authors contributed to the article and approved the submitted version.
This research was funded by the Italian Ministry of Health (RC 2023) and also supported by EU funding to AO within the NextGeneration EU-MUR PNRR Extended Partnership initiative on Emerging Infectious Diseases (project no. PE00000007, INF-ACT).
The authors declare that the research was conducted in the absence of any commercial or financial relationships that could be construed as a potential conflict of interest.
All claims expressed in this article are solely those of the authors and do not necessarily represent those of their affiliated organizations, or those of the publisher, the editors and the reviewers. Any product that may be evaluated in this article, or claim that may be made by its manufacturer, is not guaranteed or endorsed by the publisher.
Abdul-Mutakabbir, J. C., Nguyen, L., Maassen, P. T., Stamper, K. C., Kebriaei, R., Kaye, K. S., et al. (2021). In VitroAntibacterial activity of Cefiderocol against multidrug-resistant Acinetobacter baumannii. Antimicrob. Agents Chemother. 65:e0264620. doi: 10.1128/AAC.02646-20
Adams, M. D., Nickel, G. C., Bajaksouzian, S., Lavender, H., Murthy, A. R., Jacobs, M. R., et al. (2009). Resistance to Colistin in Acinetobacter baumannii associated with mutations in the PmrAB two-component system. Antimicrob. Agents Chemother. 53, 3628–3634. doi: 10.1128/AAC.00284-09
Al-Kadmy, I. M. S., Ibrahim, S. A., Al-Saryi, N., Aziz, S. N., Besinis, A., and Hetta, H. F. (2020). Prevalence of genes involved in Colistin resistance in Acinetobacter baumannii: First report from Iraq. Microb. Drug Resist. 26, 616–622. doi: 10.1089/mdr.2019.0243
Amala Reena, A., Subramaniyan, A., and Kanungo, R. (2017). Biofilm formation as a virulence factor of Acinetobacter baumannii: an emerging pathogen in critical care units. J. Curr. Res. Sci. Med. 3, 74–78. doi: 10.4103/jcrsm.jcrsm_66_17
Anbazhagan, D., Mansor, M., Yan, G. O., Md Yusof, M. Y., Hassan, H., and Sekaran, S. D. (2012). Detection of quorum sensing signal molecules and identification of an autoinducer synthase gene among biofilm forming clinical isolates of Acinetobacter spp. PLoS One 7:e36696. doi: 10.1371/journal.pone.0036696
Antunes, L. C., Visca, P., and Towner, K. J. (2011). Acinetobacter baumannii: evolution of a global pathogen. Pathogens Dis 71, 292–301. doi: 10.1111/j.2049-632X.2011.01264.x
Asaad, A. M., Ansari, S., Ajlan, S. E., and Awad, S. M. (2021). Epidemiology of biofilm producing Acinetobacter baumannii nosocomial isolates from a tertiary care hospital in Egypt: a cross-sectional study. Infect. Drug Resist. 14, 709–717. doi: 10.2147/IDR.S261939
Assimakopoulos, S. F., Karamouzos, V., Lefkaditi, A., Sklavou, C., Kolonitsiou, F., Christofidou, M., et al. (2019). Triple combination therapy with high-dose ampicillin/sulbactam, high-dose tigecycline and colistin in the treatment of ventilator-associated pneumonia caused by pan-drug resistant Acinetobacter baumannii: a case series study. Infez. Med. 27, 11–16.
Ayobami, O., Willrich, N., Suwono, B., Eckmanns, T., and Markwart, R. (2020). The epidemiology of carbapenem-non-susceptible Acinetobacter species in Europe: analysis of EARS-net data from 2013 to 2017. Antimicrob. Resist. Infect. Control 9:89. doi: 10.1186/s13756-020-00750-5
Bardbari, A. M., Arabestani, M. R., Karami, M., Keramat, F., Alikhani, M. Y., and Bagheri, K. P. (2017). Correlation between ability of biofilm formation with their responsible genes and MDR patterns in clinical and environmental Acinetobacter baumannii isolates. Microb. Pathog. 108, 122–128. doi: 10.1016/j.micpath.2017.04.039
Barsoumian, A. E., Mende, K., Sanchez, C. J., Beckius, M. L., Wenke, J. C., Murray, C. K., et al. (2015). Clinical infectious outcomes associated with biofilm-related bacterial infections: a retrospective chart review. BMC Infect. Dis. 15:223. doi: 10.1186/s12879-015-0972-2
Bartal, C., Rolston, K. V. I., and Nesher, L. (2022). Carbapenem-resistant Acinetobacter baumannii: colonization, infection, and current treatment options. Infect. Dis. Ther. 11, 683–694. doi: 10.1007/s40121-022-00597-w
Bassetti, M., Echols, R., Matsunaga, Y., Ariyasu, M., Doi, Y., Ferrer, R., et al. (2021). Efficacy and safety of cefiderocol or best available therapy for the treatment of serious infections caused by carbapenem-resistant gram-negative bacteria (CREDIBLE-CR): a randomised, open-label, multicentre, pathogen-focused, descriptive, phase 3 trial. Lancet Infect. Dis. 21, 226–240. doi: 10.1016/S1473-3099(20)30796-9
Batoni, G., Maisetta, G., and Esin, S. (2016). Antimicrobial peptides and their interaction with biofilms of medically relevant bacteria. Biochim. Biophys. Acta Biomembr. 1858, 1044–1060. doi: 10.1016/j.bbamem.2015.10.013
Bavaro, D. F., Belati, A., Diella, L., Stufano, M., Romanelli, F., Scalone, L., et al. (2021). Cefiderocol-based combination therapy for "difficult-to-treat" gram-negative severe infections: real-life case series and future perspectives. Antibiotics 10:652. doi: 10.3390/antibiotics10060652
Berlanga, M., Gomez-Perez, L., and Guerrero, R. (2017). Biofilm formation and antibiotic susceptibility in dispersed cells versus planktonic cells from clinical, industry, and environmental origins. Antonie Van Leeuwenhoek 110, 1691–1704. doi: 10.1007/s10482-017-0919-2
Bernhard, W., Hoffmann, S., Dombrowsky, H., Rau, G. A., Kamlage, A., Kappler, M., et al. (2001). Phosphatidylcholine molecular species in lung surfactant. Am. J. Respir. Cell Mol. Biol. 25, 725–731. doi: 10.1165/ajrcmb.25.6.4616
Boncompagni, S. R., Micieli, M., Di Maggio, T., Aiezza, N., Antonelli, A., Giani, T., et al. (2022). Activity of fosfomycin/colistin combinations against planktonic and biofilm Gram-negative pathogens. J Antimicrob Chemother. 77, 2199–2208. doi: 10.1093/jac/dkac142
Bou, G., and Martínez-Beltrán, J. (2000). Cloning, nucleotide sequencing, and analysis of the gene encoding an AmpC b-lactamase in Acinetobacter baumannii. Antimicrob. Agents Chemother. 44, 428–432. doi: 10.1128/AAC.44.2.428-432.2000
Brossard, K. A., and Campagnari, A. A. (2012). The Acinetobacter baumannii biofilm-associated protein plays a role in adherence to human epithelial cells. Infect. Immun. 80, 228–233. doi: 10.1128/IAI.05913-11
Cafiso, V., Stracquadanio, S., Lo Verde, F., Gabriele, G., Mezzatesta, M. L., Caio, C., et al. (2019). Colistin Resistant A. baumannii: genomic and transcriptomic traits acquired under Colistin therapy. Front. Microbiol. 9:3195. doi: 10.3389/fmicb.2018.03195
Ceccarelli, G., Oliva, A., D'Ettorre, G., D'Abramo, A., Caresta, E., Barbara, C. S., et al. (2015). The role of vancomycin in addition with colistin and meropenem against colistin-sensitive multidrug resistant Acinetobacter baumannii causing severe infections in a paediatric intensive care unit. BMC Infect. Dis. 15:393. doi: 10.1186/s12879-015-1133-3
Chaiben, V., Yamada, C. H., Telles, J. P., de Andrade, A. P., Arend, L. N. V. S., Ribeiro, V. S. T., et al. (2022). A carbapenem-resistant Acinetobacter baumannii outbreak associated with a polymyxin shortage during the COVID pandemic: an in vitro and biofilm analysis of synergy between meropenem, gentamicin and sulbactam. J Antimicrob Chemother. 77, 1676–1684. doi: 10.1093/jac/dkac102
Chang, K. C., Lin, M. F., Lin, N. T., Wu, W. J., Kuo, H. Y., Lin, T. Y., et al. (2012). Clonal spread of multidrug-resistant Acinetobacter baumannii in eastern Taiwan. J. Microbiol. Immunol. Infect. 45, 37–42. doi: 10.1016/j.jmii.2011.09.019
Chapartegui-González, I., Lázaro-Díez, M., Bravo, Z., Navas, J., Icardo, J. M., and Ramos-Vivas, J. (2018). Acinetobacter baumannii maintains its virulence after long-time starvation. PLoS One 13:e0201961. doi: 10.1371/journal.pone.0201961
Chen, H. D., and Groisman, E. A. (2013). The PmrA/PmrB two-component system: the major regulator of LPS modifications. Annu. Rev. Microbiol. 67, 83–112. doi: 10.1146/annurev-micro-092412-155751
Chiang, T. T., Huang, T. W., Sun, J. R., Kuo, S. C., Cheng, A., Liu, C. P., et al. (2022). Biofilm formation is not an independent risk factor for mortality in patients with Acinetobacter baumannii bacteremia. Front. Cell. Infect. Microbiol. 12:964539. doi: 10.3389/fcimb.2022.964539
Ching, C., Muller, P. A., Brychcy, M., Reverdy, A., Nguyen, B., Downs, M., et al. (2019). RecA levels modulate biofilm development in Acinetobacter baumannii. BioRxiv.
Choi, C. H., Hyun, S. H., Lee, J. Y., Lee, J. S., Lee, Y. S., Kim, S. A., et al. (2008). Acinetobacter baumannii outer membrane protein a targets the nucleus and induces cytotoxicity. Cell. Microbiol. 10, 309–319. doi: 10.1111/j.1462-5822.2007.01041.x
Choi, C. H., Lee, E. Y., Lee, Y. C., Park, T. I., Kim, H. J., Hyun, S. H., et al. (2005). Outer membrane protein 38 of Acinetobacter baumannii localizes to the mitochondria and induces apoptosis of epithelial cells. Cell. Microbiol. 7, 1127–1138. doi: 10.1111/j.1462-5822.2005.00538.x
Choi, A. H. K., Slamti, L., Avci, F. Y., Pier, G. B., and Maira-Litrán, T. (2009). The pgaABCD locus of Acinetobacter baumannii encodes the production of poly-β-1-6-N-Acetylglucosamine, which is critical for biofilm formation. J. Bacteriol. 191, 5953–5963. doi: 10.1128/JB.00647-09
Cianfanelli, F. R., Monlezun, L., and Coulthurst, S. J. (2016). Aim, load, fire: the type VI secretion system, a bacterial nanoweapon. Trends Microbiol. 24, 51–62. doi: 10.1016/j.tim.2015.10.005
Cogliati Dezza, F., Arcari, G., Alessi, F., Valeri, S., Curtolo, A., Sacco, F., et al. (2022). Clinical impact of COVID-19 on multi-drug-resistant gram-negative bacilli bloodstream infections in an intensive care unit setting: two pandemics compared. Antibiotics 11:926. doi: 10.3390/antibiotics11070926
Confer, A. W., and Ayalew, S. (2013). The OmpA family of proteins: roles in bacterial pathogenesis and immunity. Vet. Microbiol. 163, 207–222. doi: 10.1016/j.vetmic.2012.08.019
Cornejo-Juárez, P., Cevallos, M. A., Castro-Jaimes, S., Castillo-Ramírez, S., Velázquez-Acosta, C., Martínez-Oliva, D., et al. (2020). High mortality in an outbreak of multidrug resistant Acinetobacter baumannii infection introduced to an oncological hospital by a patient transferred from a general hospital. PLoS One 15:e0234684. doi: 10.1371/journal.pone.0234684
Coyne, S., Courvalin, P., and Périchon, B. (2011). Efflux-mediated antibiotic resistance in Acinetobacter spp. Antimicrob. Agents Chemother. 55, 947–953. doi: 10.1128/AAC.01388-10
Cucarella, C., Solano, C., Valle, J., Amorena, B., Lasa, I., and Penadés, J. R. (2001). Bap, a Staphylococcus aureus surface protein involved in biofilm formation. J. Bacteriol. 183, 2888–2896. doi: 10.1007/s10482-017-0919-2
Darby, E. M., Trampari, E., Siasat, P., Gaya, M. S., Alav, I., Webber, M. A., et al. (2023). Molecular mechanisms of antibiotic resistance revisited. Nat. Rev. Microbiol. 21, 280–295. doi: 10.1038/s41579-022-00820-y
De Angelis, M., Mascellino, M. T., Miele, M. C., Al Ismail, D., Colone, M., Stringaro, A., et al. (2022). High activity of N-acetylcysteine in combination with Beta-lactams against Carbapenem-resistant Klebsiella pneumoniae and Acinetobacter baumannii. Antibiotics 11:225. doi: 10.3390/antibiotics11020225
Decré, D. (2012). Acinetobacter baumannii et résistance aux antibiotiques: Un modèle d’adaptation. Revue Francophone des Laboratoires 2012, 43–52. doi: 10.1016/S1773-035X(12)71412-0
Deveson Lucas, D., Crane, B., Wright, A., Han, M. L., Moffatt, J., Bulach, D., et al. (2018). Emergence of high-level Colistin resistance in an Acinetobacter baumannii clinical isolate mediated by inactivation of the global regulator H-NS. Antimicrob. Agents Chemother. 62, e02442–e02417. doi: 10.1128/AAC.02442-17
Di Domenico, E. G., Cavallo, I., Sivori, F., Marchesi, F., Prignano, G., Pimpinelli, F., et al. (2020). Biofilm production by Carbapenem-resistant Klebsiella pneumoniae significantly increases the risk of death in oncological patients. Front. Cell. Infect. Microbiol. 10:561741. doi: 10.3389/fcimb.2020.561741
Di Domenico, E. G., Marchesi, F., Cavallo, I., Toma, L., Sivori, F., Papa, E., et al. (2021). The impact of bacterial biofilms on end-organ disease and mortality in patients with hematologic malignancies developing a bloodstream infection. Microbiol Spectr 9, e00550–e00521. doi: 10.1128/Spectrum.00550-21
Durante-Mangoni, E., Signoriello, G., Andini, R., Mattei, A., De Cristoforo, M., Murino, P., et al. (2013). Colistin and rifampicin compared with colistin alone for the treatment of serious infections due to extensively drug-resistant Acinetobacter baumannii: a multicenter, randomized clinical trial. Clin. Infect. Dis. 57, 349–358. doi: 10.1093/cid/cit253
Eijkelkamp, B. A. (2014). Comparative analysis of surface-exposed virulence factors of Acinetobacter baumannii. BMC Genomics 15:1020. doi: 10.1186/1471-2164-15-1020
Eijkelkamp, B. A., Stroeher, U. H., Hassan, K. A., Papadimitrious, M. S., Paulsen, I. T., and Brown, M. H. (2011). Adherence and motility characteristics of clinical Acinetobacter baumannii isolates. FEMS Microbiol. Lett. 323, 44–51. doi: 10.1111/j.1574-6968.2011.02362.x
Elhosseiny, N. M., and Attia, A. S. (2018). Acinetobacter: an emerging pathogen with a versatile secretome. Emerg. Microb. Infect. 7, 1–15. doi: 10.1038/s41426-018-0030-4
Ellis, T. N., and Kuehn, M. J. (2010). Virulence and immunomodulatory roles of bacterial outer membrane vesicles. Microbiol. Mol. Biol. Rev. 74, 81–94. doi: 10.1128/MMBR.00031-09
El-Sayed Ahmed, M. A. E., Zhong, L. L., Shen, C., Yang, Y., Doi, Y., and Tian, G. B. (2020). Colistin and its role in the era of antibiotic resistance: an extended review (2000-2019). Emerg Microbes Infect. 9, 868–885. doi: 10.1080/22221751.2020.1754133
Evans, B. A., Hamouda, A., and Amyes, S. G. B. (2013). The rise of carbapenem-resistant Acinetobacter baumannii. Curr. Pharm. Des. 19, 223–238.
Ezadi, F., Ardebili, A., and Mirnejad, R. (2019). Antimicrobial susceptibility testing for Polymyxins: challenges, issues, and recommendations. J. Clin. Microbiol. 57, e01390–e01318. doi: 10.1128/JCM.01390-18
Eze, E. C., Chenia, H. Y., and El Zowalaty, M. E. (2018). Acinetobacter baumannii biofilms: effects of physicochemical factors, virulence, antibiotic resistance determinants, gene regulation, and future antimicrobial treatments. Infect Drug Resist. 11, 2277–2299. doi: 10.2147/IDR.S169894
Falcone, M., Tiseo, G., Giordano, C., Leonildi, A., Menichini, M., Vecchione, A., et al. (2021). Predictors of hospital-acquired bacterial and fungal superinfections in COVID-19: a prospective observational study. J. Antimicrob. Chemother. 76, 1078–1084. doi: 10.1093/jac/dkaa530
Falcone, M., Tiseo, G., Leonildi, A., Della Sala, L., Vecchione, A., Barnini, S., et al. (2022). Cefiderocol-compared to Colistin-based regimens for the treatment of severe infections caused by Carbapenem-resistant Acinetobacter baumannii. Antimicrob. Agents Chemother. 66:e0214221. doi: 10.1128/aac.02142-21
Fernandez, L., and Hancock, R. (2013). Adaptive and mutational resistance: role of porins and efflux pumps in drug resistance. Clin. Microbiol. Rev. 25, 661–681. doi: 10.1128/CMR.00043-12
Fitzsimons, T. C., Lewis, J. M., Wright, A., Kleifeld, O., Schittenhelm, R. B., Powell, D., et al. (2018). Identification of novel Acinetobacter baumannii type VI secretion system antibacterial effector and immunity pairs. Infect. Immun. 86, e00297–e00218. doi: 10.1128/IAI.00297-18
Flannery, A., le Berre, M., Pier, G. B., O’Gara, J. P., and Kilcoyne, M. (2020). Glycomics microarrays reveal differential in situ presentation of the biofilm polysaccharide poly-N-acetylglucosamine on Acinetobacter baumannii and Staphylococcus aureus cell surfaces. Int. J. Mol. Sci. 21:2465. doi: 10.3390/ijms21072465
Flores-Díaz, M., Monturiol-Gross, L., Naylor, C., Alape-Girón, A., and Flieger, A. (2016). Bacterial sphingomyelinases and phospholipases as virulence factors. Microbiol. Mol. Biol. Rev. 80, 597–628. doi: 10.1128/MMBR.00082-15
Fournier, P. E., Vallenet, D., Barbe, V., Audic, S., Ogata, H., Poirel, L., et al. (2006). Comparative genomics of multidrug resistance in Acinetobacter baumannii. PLoS Genet. 2, 32–34. doi: 10.1371/journal.pgen.0020007
Gaddy, J. A., and Actis, L. A. (2009). Regulation of Acinetobacter baumannii biofilm formation. Future Microbiol. 4, 273–278. doi: 10.2217/fmb.09.5
Gaddy, J. A., Tomaras, A. P., and Actis, L. A. (2009). The Acinetobacter baumannii 19606 OmpA protein plays a role in biofilm formation on abiotic surfaces and in the interaction of this pathogen with eukaryotic cells. Infect. Immun. 77, 3150–3160. doi: 10.1128/IAI.00096-09
Garnacho-Montero, J., Amaya-Villar, R., Ferrándiz-Millón, C., Díaz-Martín, A., López-Sánchez, J. M., and Gutiérrez-Pizarraya, A. (2015). Optimum treatment strategies for carbapenem-resistant Acinetobacter baumannii bacteremia. Expert Rev. Anti Infect. Ther. 13, 769–777. doi: 10.1586/14787210.2015.1032254
Gatti, M., Bartoletti, M., Cojutti, P. G., Gaibani, P., Conti, M., Giannella, M., et al. (2021). A descriptive case series of pharmacokinetic/pharmacodynamic target attainment and microbiological outcome in critically ill patients with documented severe extensively drug-resistant Acinetobacter baumannii bloodstream infection and/or ventilator-associated pneumonia treated with cefiderocol. J. Glob. Antimicrob. Resist. 27, 294–298. doi: 10.1016/j.jgar.2021.10.014
Ghasemi, E., Ghalavand, Z., Goudarzi, H., Yeganeh, F., Hashemi, A., Dabiri, H., et al. (2018). Phenotypic and genotypic investigation of biofilm formation in clinical and environmental isolates of Acinetobacter baumannii. Arch. Clin. Infect. Dis. 13:e12914. doi: 10.5812/archcid.12914
Giacobbe, D. R., Saffioti, C., Losito, A. R., Rinaldi, M., Aurilio, C., Bolla, C., et al. (2020). Use of colistin in adult patients: a cross-sectional study. J Glob Antimicrob Resist. 20, 43–49. doi: 10.1016/j.jgar.2019.06.009
Giammanco, A., Cala, C., Fasciana, T., and Dowzicky, M. J. (2017). Global assessment of the activity of tigecycline against multidrug-resistant gram-negative pathogens between 2004 and 2014 as part of the tigecycline evaluation and surveillance trial. mSphere 2, e00310–e00316. doi: 10.1128/mSphere.00310-16
Girija, S., and Priyadharsini, J. (2019). CLSI based antibiogram profile and the detection of MDR and XDR strains of Acinetobacter baumannii isolated from urine samples. Med. J. Islam Repub. Iran 33:3. doi: 10.34171/mjiri.33.3
Girod, S., Galabert, C., Lecuire, A., Zahm, J. M., and Puchelle, E. (1992). Phospholipid composition and surface-active properties of tracheobronchial secretions from patients with cystic fibrosis and chronic obstructive pulmonary diseases. Pediatr. Pulmonol. 13, 22–27. doi: 10.1002/ppul.1950130107
Gonzalez-Villoria, A. M., and Valverde-Garduno, V. (2016). Antibiotic-resistant Acinetobacter baumannii increasing success remains a challenge as a nosocomial pathogen. J Pathog 2016:7318075. doi: 10.1155/2016/7318075
Greene, C., Vadlamudi, G., Newton, D., Foxman, B., and Xi, C. (2016a). The influence of biofilm formation and multidrug resistance on environmental survival of clinical and environmental isolates of Acinetobacter baumannii. Am. J. Infect. Control 44, e65–e71. doi: 10.1016/j.ajic.2015.12.012
Greene, C., Wu, J., Rickard, A. H., and Xi, C. (2016b). Evaluation of the ability of Acinetobacter baumannii to form biofilms on six different biomedical relevant surfaces. Lett. Appl. Microbiol. 63, 233–239. doi: 10.1016/j.ajic.2015.12.012
Hamidian, M., and Nigro, S. J. (2019). Emergence, molecular mechanisms and global spread of carbapenem-resistant Acinetobacter baumannii. Microb Genom. 5:e000306. doi: 10.1099/mgen.0.000306
Harding, C. M. (2016). Medically relevant Acinetobacter species require a type ii secretion system and specific membrane-associated chaperones for the export of multiple substrates and full virulence. PLoS Pathog. 12:e1005391. doi: 10.1371/journal.ppat.1005391
Harding, C. M., Hennon, S. W., and Feldman, M. F. (2018). Uncovering the mechanisms of Acinetobacter baumannii virulence. Nat. Rev. Microbiol. 16, 91–102. doi: 10.1038/nrmicro.2017.148
Harding, C. M., Pulido, M. R., Di Venanzio, G., Kinsella, R. L., Webb, A. I., Scott, N. E., et al. (2017). Pathogenic Acinetobacter species have a functional type I secretion system and contact-dependent inhibition systems. J. Biol. Chem. 292, 9075–9087. doi: 10.1074/jbc.M117.781575
Hasani, A., Sheikhalizadeh, V., Ahangarzadeh Rezaee, M., Rahmati-Yamchi, M., Hasani, A., Ghotaslou, R., et al. (2016). Frequency of aminoglycoside-modifying enzymes and ArmA among different sequence groups of Acinetobacter baumannii in Iran. Microb. Drug Resist. 22, 347–353. doi: 10.1089/mdr.2015.0254
He, X., Lu, F., Yuan, F., Jiang, D., Zhao, P., Zhu, J., et al. (2015). Biofilm formation caused by clinical Acinetobacter baumannii isolates is associated with overexpression of the AdeFGH efflux pump. Antimicrob. Agents Chemother. 59, 4817–4825. doi: 10.1128/AAC.00877-15
Heritier, C., Poirel, L., and Nordmann, P. (2006). Cephalosporinase overexpression resulting from insertion of ISAba1 in Acinetobacter baumannii. Clin. Microbiol. Infect. 12:123e30. doi: 10.1111/j.1469-0691.2005.01320.x
Hu, Y., He, L., Tao, X., Meng, F., and Zhang, J. (2016). Biofilm may not be necessary for the epidemic spread of Acinetobacter baumannii. Sci. Rep. 6:32066. doi: 10.1038/srep32066
Hua, J., Jia, X., Zhang, L., and Li, Y. (2020). The characterization of two-component system PmrA/PmrB in Cronobacter sakazakii. Front. Microbiol. 11:903. doi: 10.3389/fmicb.2020.00903
Iacovelli, A., Oliva, A., Siccardi, G., Tramontano, A., Pellegrino, D., Mastroianni, C. M., et al. (2023). Risk factors and effect on mortality of superinfections in a newly established COVID-19 respiratory sub-intensive care unit at University Hospital in Rome. BMC Pulm. Med. 23:30. doi: 10.1186/s12890-023-02315-9
Ibrahim, S., Al-Saryi, N., Al-Kadmy, I. M. S., and Aziz, S. N. (2021). Multidrug-resistant Acinetobacter baumannii as an emerging concern in hospitals. Mol. Biol. Rep. 48, 6987–6998. doi: 10.1007/s11033-021-06690-6
Ilsan, N. A., Lee, Y. J., Kuo, S. C., Lee, I. H., and Huang, T. W. (2021). Antimicrobial resistance mechanisms and virulence of Colistin- and Carbapenem-resistant Acinetobacter baumannii isolated from a teaching Hospital in Taiwan. Microorganisms 9:1295. doi: 10.3390/microorganisms9061295
Imberti, R., Cusato, M., Villani, P., Carnevale, L., Iotti, G. A., Langer, M., et al. (2010). Steady-state pharmacokinetics and BAL concentration of colistin in critically ill patients after IV colistin methanesulfonate administration. Chest 138, 1333–1339. doi: 10.1378/chest.10-0463
Ingti, B., Upadhyay, S., Hazarika, M., Khyriem, A. B., Paul, D., Bhattacharya, P., et al. (2020). Distribution of carbapenem-resistant Acinetobacter baumannii with blaADC-30 and induction of ADC-30 in response to beta-lactam antibiotics. Res. Microbiol. 171, 128–133. doi: 10.1016/j.resmic.2020.01.002
Iovleva, A., Mustapha, M. M., Griffith, M. P., Komarow, L., Luterbach, C., Evans, D. R., et al. (2022). Carbapenem-Resistant Acinetobacter baumannii in U.S. Hospitals: Diversification of Circulating Lineages and Antimicrobial Resistance. MBio 13:e0275921. doi: 10.1128/mbio.02759-21
Iregui, A., Khan, Z., Landman, D., and Quale, J. (2020). Activity of Cefiderocol against Enterobacterales, Pseudomonas aeruginosa, and Acinetobacter baumannii endemic to medical Centers in new York City. Microb. Drug Resist. 26, 722–726. doi: 10.1089/mdr.2019.0298
Jacobs, A. C., Hood, I., Boyd, K. L., Olson, P. D., Morrison, J. M., Carson, S., et al. (2010). Inactivation of phospholipase D diminishes Acinetobacter baumannii pathogenesis. Infect. Immun. 78, 1952–1962. doi: 10.1128/IAI.00889-09
Jacoby, G. A., Strahilevitz, J., and Hooper, D. C. (2014). Plasmid-mediated quinolone resistance. Microbiol Spectr 2:10. doi: 10.1128/microbiolspec.PLAS-0006-2013
Jaruratanasirikul, S., Vattanavanit, V., Samaeng, M., Nawakitrangsan, M., and Sriwiriyajan, S. (2019). Pharmacokinetics of imipenem in critically ill patients with life-threatening severe infections during support with extracorporeal membrane oxygenation. Clin. Drug Investig. 39, 787–798. doi: 10.1007/s40261-019-00796-3
Jeannot, K., Bolard, A., and Plésiat, P. (2017). Resistance to polymyxins in gram-negative organisms. Int. J. Antimicrob. Agents 49, 526–535. doi: 10.1016/j.ijantimicag.2016.11.029
Jin, J. S., Kwon, S. O., Moon, D. C., Gurung, M., Lee, J. H., Kim, S. I., et al. (2011). Acinetobacter baumannii secretes cytotoxic outer membrane protein a via outer membrane vesicles. PLoS One 6:e17027. doi: 10.1371/journal.pone.0017027
Johnson, T. L., Waack, U., Smith, S., Mobley, H., and Sandkvist, M. (2016). Acinetobacter baumannii is dependent on the type II secretion system and its substrate LipA for lipid utilization and in vivo fitness. J. Bacteriol. 198, 711–719. doi: 10.1128/JB.00622-15
Karakonstantis, S., Gikas, A., Astrinaki, E., and Kritsotakis, E. I. (2020). Excess mortality due to pandrug-resistant Acinetobacter baumannii infections in hospitalized patients. J. Hosp. Infect. 106, 447–453. doi: 10.1016/j.jhin.2020.09.009
Katip, W., Uitrakul, S., and Oberdorfer, P. A. (2020). Comparison of Colistin versus Colistin plus Meropenem for the treatment of Carbapenem-resistant Acinetobacter baumannii in critically ill patients: a propensity score-matched analysis. Antibiotics 9:647. doi: 10.3390/antibiotics9100647
Katip, W., Uitrakul, S., and Oberdorfer, P. (2021a). Clinical efficacy and nephrotoxicity of the loading dose Colistin for the treatment of Carbapenem-resistant Acinetobacter baumannii in critically ill patients. Pharmaceutics. 14:31. doi: 10.3390/pharmaceutics14010031
Katip, W., Uitrakul, S., and Oberdorfer, P. (2021b). Short-course versus long-course Colistin for treatment of Carbapenem-Resistant A. baumannii in cancer patient. Antibiotics 10:484. doi: 10.3390/antibiotics10050484
Kim, S. W., Choi, C. H., Moon, D. C., Jin, J. S., Lee, J. H., Shin, J. H., et al. (2009). Serum resistance of Acinetobacter baumannii through the binding of factor H to outer membrane proteins. FEMS Microbiol. Lett. 301, 224–231. doi: 10.1111/j.1574-6968.2009.01820.x
Kohira, N., Hackel, M. A., Ishioka, Y., Kuroiwa, M., Sahm, D. F., Sato, T., et al. (2020). Reduced susceptibility mechanism to cefiderocol, a siderophore cephalosporin, among clinical isolates from a global surveillance program (SIDERO-WT-2014). J. Glob. Antimicrob. Resist. 22, 738–741. doi: 10.1016/j.jgar.2020.07.009
Krasauskas, R., Skerniškytė, J., Armalytė, J., and Sužiedėlienė, E. (2019). The role of Acinetobacter baumannii response regulator BfmR in pellicle formation and competitiveness via contact-dependent inhibition system. BMC Microbiol. 19:241. doi: 10.1186/s12866-019-1621-5
Kulp, A., and Kuehn, M. J. (2010). Biological functions and biogenesis of secreted bacterial outer membrane vesicles. Annu. Rev. Microbiol. 64, 163–184. doi: 10.1146/annurev.micro.091208.073413
Kuo, S. C., Lee, Y. T., Yang Lauderdale, T. L., Huang, W. C., Chuang, M. F., and Chen, C. P. (2015). Contribution of Acinetobacter-derived cephalosporinase-30 to sulbactam resistance in Acinetobacter baumannii. Front. Microbiol. 6:231. doi: 10.3389/fmicb.2015.00231
Langford, B. J., So, M., Simeonova, M., Leung, V., Lo, J., Kan, T., et al. (2023). Antimicrobial resistance in patients with COVID-19: a systematic review and meta-analysis. Lancet Microbe 4:e179. doi: 10.2139/ssrn.4099404
Law, S. K. K., and Tan, H. S. (2022). The role of quorum sensing, biofilm formation, and iron acquisition as key virulence mechanisms in Acinetobacter baumannii and the corresponding anti-virulence strategies. Microbiol. Res. 260:127032. doi: 10.1016/j.micres.2022.127032
Lee, H. Y., Chen, C. L., Wu, S. R., Huang, C. W., and Chiu, C. H. (2014). Risk factors and outcome analysis of Acinetobacter baumannii complex bacteremia in critical patients. Crit. Care Med. 42, 1081–1088. doi: 10.1097/CCM.0000000000000125
Lee, C. R., Lee, J. H., Park, M., Park, K. S., Bae, I. K., Kim, S., et al. (2017). Biology of Acinetobacter baumannii: pathogenesis, antibiotic resistance mechanisms, and prospective treatment options. Front. Cell. Infect. Microbiol. 7:55. doi: 10.3389/fcimb.2017.00055
Lemos, E. V., de la Hoz, F. P., Einarson, T. R., McGhan, W. F., Quevedo, E., Castañeda, C., et al. (2014). Carbapenem resistance and mortality in patients with Acinetobacter baumannii infection: systematic review and meta-analysis. Clin. Microbiol. Infect. 20, 416–423. doi: 10.1111/1469-0691.12363
Li, F., and Wang, Z. (2018). Increasing rates of Acinetobacter baumannii infection and resistance in an oncology department. J. Cancer Res. Ther. 14, 68–71. doi: 10.4103/jcrt.JCRT_737_17
Llewellyn, A. C., Zhao, J., Song, F., Parvathareddy, J., Xu, Q., Napier, B. A., et al. (2012). NaxD is a deacetylase required for lipid a modification and Francisella pathogenesis. Mol. Microbiol. 86, 611–627. doi: 10.1111/mmi.12004
Lob, S. H., Hoban, D. J., Sahm, D. F., and Badal, R. E. (2016). Regional differences and trends in antimicrobial susceptibility of Acinetobacter baumannii. Int. J. Antimicrob. Agents 47, 317–323. doi: 10.1016/j.ijantimicag.2016.01.015
Loehfelm, T. W., Luke, N. R., and Campagnari, A. A. (2008). Identification and characterization of an Acinetobacter baumannii biofilm-associated protein. J. Bacteriol. 190, 1036–1044. doi: 10.1128/JB.01416-07
Longo, F., Vuotto, C., and Donelli, G. (2014). Biofilm formation in Acinetobacter baumannii. New Microbiol. 37, 119–127. doi: 10.2147/IDR.S332051
Luo, L. M., Wu, L. J., Xiao, Y. L., Zhao, D., Chen, Z. X., Kang, M., et al. (2015). Enhancing pili assembly and biofilm formation in Acinetobacter baumannii ATCC19606 using non-native acyl-homoserine lactones. BMC Microbiol. 15:62. doi: 10.1186/s12866-015-0397-5
Ma, C., and McClean, S. (2021). Mapping global prevalence of Acinetobacter baumannii and recent vaccine development to tackle it. Vaccine 9:570. doi: 10.3390/vaccines9060570
Ma, F., Shen, C., Zheng, X., Liu, Y., Chen, H., Zhong, L., et al. (2019). Identification of a novel plasmid carrying mcr-4.3 in an Acinetobacter baumannii strain in China. Antimicrob. Agents Chemother. 63, e00133–e00119. doi: 10.1128/AAC.00133-19
Magill, S. S., Edwards, J. R., Bamberg, W., Beldavs, Z. G., Dumyati, G., Kainer, M. A., et al. (2014). Multistate point-prevalence survey of health care-associated infections. N. Engl. J. Med. 370, 1198–1208. doi: 10.1056/NEJMoa1306801
Magnet, S., Courvalin, P., and Lambert, T. J. (2001). Resistance-nodulation-cell division-type efflux pump involved in aminoglycoside resistance in Acinetobacter baumannii strain BM4454. Antimicrob. Agents Chemother. 45, 3375–3380. doi: 10.1128/AAC.45.12.3375-3380.2001
Malik, S., Kaminski, M., Landman, D., and Quale, J. (2020). Cefiderocol resistance in Acinetobacter baumannii: roles of the ß-lactamases, siderophore receptors, and penicillin-binding protein 3. Antimicrob. Agents Chemother. 64, e01221–e01220. doi: 10.1128/AAC.01221-20
Mangioni, D., Fox, V., Chatenoud, L., Bolis, M., Bottino, N., Cariani, L., et al. (2023). Genomic characterization of Carbapenem-resistant Acinetobacter baumannii (CRAB) in mechanically ventilated COVID-19 patients and impact of infection control measures on reducing CRAB circulation during the second wave of the SARS-CoV-2 pandemic in Milan, Italy. Microbiol Spectr. 11:e0020923. doi: 10.1128/spectrum.00209-23
Martí, S., Rodríguez-Bano, J., Catel-Ferreira, M., Jouenne, T., Vila, J., Seifert, H., et al. (2011). Biofilm formation at the solid-liquid and air-liquid interfaces by Acinetobacter species. BMC. Res. Notes 4:5. doi: 10.1186/1756-0500-4-5
Marvasi, M., Visscher, P. T., and Casillas Martinez, L. (2010). Exopolymeric substances (EPS) from Bacillus subtilis: polymers and genes encoding their synthesis. FEMS Microbiol. Lett. 313, 1–9. doi: 10.1111/j.1574-6968.2010.02085.x
McConnell, M. J., Actis, L., and Pachon, J. (2013). Acinetobacter baumannii: human infections, factors contributing to pathogenesis and animal models. FEMS Microbiol. Rev. 37, 130–155. doi: 10.1111/j.1574-6976.2012.00344.x
Meletis, G. (2016). Carbapenem resistance: overview of the problem and future perspectives. Ther Adv Infect Dis 3, 15–21. doi: 10.1177/2049936115621709
Moffatt, J. H., Harper, M., Harrison, P., Hale, J. D. F., Vinogradov, E., Seemann, T., et al. (2010). Colistin resistance in Acinetobacter baumannii is mediated by complete loss of lipopolysaccharide production. Antimicrob. Agents Chemother. 54, 4971–4977. doi: 10.1128/AAC.00834-10
Moon, D. C., Choi, C. H., Lee, J. H., Choi, C. W., Kim, H. Y., Park, J. S., et al. (2012). Acinetobacter baumannii outer membrane protein a modulates the biogenesis of outer membrane vesicles. J. Microbiol. 50, 155–160. doi: 10.1007/s12275-012-1589-4
Morris, F. C., Dexter, C., Kostoulias, X., Uddin, M. I., and Peleg, A. Y. (2019). The mechanisms of disease caused by Acinetobacter baumannii. Front. Microbiol. 10:1601. doi: 10.3389/fmicb.2019.01601
Moynié, L., Luscher, A., Rolo, D., Pletzer, D., Tortajada, A., Weingart, H., et al. (2017). Structure and function of the PiuA and PirA Siderophore-drug receptors from Pseudomonas aeruginosa and Acinetobacter baumannii. Antimicrob. Agents Chemother. 61, e02531–e02516. doi: 10.1128/AAC.02531-16
Muthusamy, D., Sudhishnaa, S., and Boppe, A. (2016). Invitro activities of Polymyxins and rifampicin against Carbapenem resistant Acinetobacter baumannii at a tertiary care hospital from South India. J. Clin. Diagn. Res. 10, DC15–DC18. doi: 10.7860/JCDR/2016/19968.8535
Nait Chabane, Y., Marti, S., Rihouey, C., Alexandre, S., Hardouin, J., Lesouhaitier, O., et al. (2014). Characterisation of pellicles formed by Acinetobacter baumannii at the air-liquid interface. PLoS One 9:660. doi: 10.1371/journal.pone.0111660
Ñamendys-Silva, S. A., Correa-García, P., García-Guillén, F. J., González-Herrera, M. O., Pérez-Alonso, A., Texcocano-Becerra, J., et al. (2015). Outcomes of critically ill cancer patients with Acinetobacter baumannii infection. World J Crit Care Med 4, 258–264. doi: 10.5492/wjccm.v4.i3.258
Nazer, L. H., Kharabsheh, A., Rimawi, D., Mubarak, S., and Hawari, F. (2015). Characteristics and outcomes of Acinetobacter baumannii infections in critically ill patients with cancer: a matched case–control study. Microb. Drug Resist. 21, 556–561. doi: 10.1089/mdr.2015.0032
Nie, L., Lv, Y., Yuan, M., Hu, X., Nie, T., Yang, X., et al. (2014). Genetic basis of high-level aminoglycoside resistance in Acinetobacter baumannii from Beijing, China. Acta Pharm. Sin. B 4, 295–300. doi: 10.1016/j.apsb.2014.06.004
Niu, C., Clemmer, K. M., Bonomo, R. A., and Rather, P. N. (2008). Isolation and characterization of an autoinducer synthase from Acinetobacter baumannii. J. Bacteriol. 190, 3386–3392. doi: 10.1128/JB.01929-07
Novović, K., and Jovčić, B. (2023). Colistin resistance in Acinetobacter baumannii: molecular mechanisms and epidemiology. Antibiotics 12:516. doi: 10.3390/antibiotics12030516
Nowak, J., Zander, E., Stefanik, D., Higgins, P. G., Roca, I., Vila, J., et al. (2017). High incidence of pandrug-resistant Acinetobacter baumannii isolates collected from patients with ventilator-associated pneumonia in Greece, Italy and Spain as part of the MagicBullet clinical trial. J. Antimicrob. Chemother. 72, 3277–3282. doi: 10.1093/jac/dkx322
Ogutlu, A., Guclu, E., Karabay, O., Utku, A. C., Tuna, N., and Yahyaoglu, M. (2014). Effects of Carbapenem consumption on the prevalence of Acinetobacter infection in intensive care unit patients. Ann. Clin. Microbiol. Antimicrob. 13:7. doi: 10.1186/1476-0711-13-7
Oh, M. H., and Han, K. (2020). AbaR is a LuxR type regulator essential for motility and the formation of biofilm and pellicle in Acinetobacter baumannii. Genes Genomics. 42, 1339–1346. doi: 10.1007/s13258-020-01005-8
Olaitan, A. O., Morand, S., and Rolain, J. M. (2014). Mechanisms of polymyxin resistance: acquired and intrinsic resistance in bacteria. Front. Microbiol. 5:643. doi: 10.3389/fmicb.2014.00643
Oliva, A., Bianchi, A., Russo, A., Ceccarelli, G., Cancelli, F., Aloj, F., et al. (2021). Effect of N-acetylcysteine Administration on 30-day mortality in critically ill patients with septic shock caused by Carbapenem-resistant Klebsiella pneumoniae and Acinetobacter baumannii: a retrospective case-control study. Antibiotics 10:271. doi: 10.3390/antibiotics10030271
Oliva, A., Ceccarelli, G., De Angelis, M., Sacco, F., Miele, M. C., Mastroianni, C. M., et al. (2020). Cefiderocol for compassionate use in the treatment of complicated infections caused by extensively and pan-resistant Acinetobacter baumannii. J. Glob. Antimicrob. Resist. 23, 292–296. doi: 10.1016/j.jgar.2020.09.019
Oliva, A., Cipolla, A., Vullo, V., Venditti, M., Mastroianni, C. M., and Falcone, M. (2017). Clinical and in vitro efficacy of colistin plus vancomycin and rifampin against colistin-resistant Acinetobacter baumannii causing ventilator-associated pneumonia. New Microbiol. 40, 205–207.
Ordooei Javan, A., Shokouhi, S., and Sahraei, Z. (2015). A review on colistin nephrotoxicity. Eur. J. Clin. Pharmacol. 71, 801–810. doi: 10.1007/s00228-015-1865-4
Orsinger-Jacobsen, S. J., Patel, S. S., Vellozzi, E. M., Gialanella, P., Nimrichter, L., Miranda, K., et al. (2013). Use of a stainless-steel washer platform to study Acinetobacter baumannii adhesion and biofilm formation on abiotic surfaces. Microbiology 159, 2594–2604. doi: 10.1099/mic.0.068825-0
Özarslan, T. O., Sirmatel, F., Karabörk, S. O., Düzcü, S. E., and Astarci, H. M. (2023). Acinetobacter baumannii pneumonia increases surfactant proteins SP-A, SP-B, and SP-D levels, while decreasing SP-C level in bronchoalveolar lavage in rats. Microbes Infect. 25:105064. doi: 10.1016/j.micinf.2022.105064
Ozbek, B., and Mataraci, E. (2013). In vitro effectiveness of colistin, tigecycline, and levofloxacin alone and combined with clarithromycin and/or heparin as lock solutions against embedded Acinetobacter baumannii strains. J. Antimicrob. Chemother. 68, 827–830. doi: 10.1093/jac/dks472
Pakharukova, N., Tuittila, M., Paavilainen, S., and Zavialov, A. (2015). Crystallization and preliminary X-ray diffraction analysis of the Csu pili CsuC-CsuA/B chaperone-major subunit pre-assembly complex from Acinetobacter baumannii. Acta Crystallogr. F. Struct. Biol. Commun. 71, 770–774. doi: 10.1107/S2053230X15007955
Palmieri, M., D’Andrea, M. M., Pelegrin, A. C., Perrot, N., Mirande, C., Blanc, B., et al. (2020). Abundance of Colistin-resistant, OXA-23- and ArmA-producing Acinetobacter baumannii belonging to international clone 2 in Greece. Front. Microbiol. 11:668. doi: 10.3389/fmicb.2020.00668
Pandey, N., and Cascella, M. (2022). “Beta lactam antibiotics” in StatPearls (Treasure Island (FL): StatPearls Publishing).
Papathanakos, G., Andrianopoulos, I., Papathanasiou, A., Priavali, E., Koulenti, D., and Koulouras, V. (2020). Colistin-resistant Acinetobacter Baumannii Bacteremia: a serious threat for critically ill patients. Microorganisms 8:287. doi: 10.3390/microorganisms8020287
Park, J. S., Lee, W. C., Choi, S., Yeo, K. J., Song, J. H., Han, Y. H., et al. (2011). Overexpression, purification, crystallization and preliminary X-ray crystallographic analysis of the periplasmic domain of outer membrane protein a from Acinetobacter baumannii. Acta Crystallogr. Sect. F Struct. Biol. Cryst. Commun. 67, 1531–1533. doi: 10.1107/S1744309111038401
Park, J. S., Lee, W. C., Yeo, K. J., Ryu, K. S., Kumarasiri, M., Hesek, D., et al. (2012). Mechanism of anchoring of OmpA protein to the cell wall peptidoglycan of the gram-negative bacterial outer membrane. FASEB J. 26, 219–228. doi: 10.1096/fj.11-188425
Partridge, S. R., Di Pilato, V., Doi, Y., Feldgarden, M., Haft, D. H., Klimke, W., et al. (2018). Proposal for assignment of allele numbers for mobile colistin resistance (mcr) genes. J. Antimicrob. Chemother. 73, 2625–2630. doi: 10.1093/jac/dky262
Pascale, R., Pasquini, Z., Bartoletti, M., Caiazzo, L., Fornaro, G., Bussini, L., et al. (2021). Cefiderocol treatment for carbapenem-resistant Acinetobacter baumannii infection in the ICU during the COVID-19 pandemic: a multicentre cohort study. JAC Antimicrob. Resist. 3:dlab174. doi: 10.1093/jacamr/dlab174
Patel, A., Emerick, M., Cabunoc, M. K., Williams, M. H., Preas, M. A., Schrank, G., et al. (2021). Rapid spread and control of multidrug-resistant gram-negative bacteria in COVID-19 patient care units. Emerg. Infect. Dis. 27, 1234–1237. doi: 10.3201/eid2704.204036
Paton, R., Miles, R. S., Hood, J., Amyes, S. G., Miles, R. S., and Amyes, S. G. (1993). ARI 1: b-lactamase-mediated imipenem resistance in Acinetobacter baumannii. Int. J. Antimicrob. Agents 2:81e7. doi: 10.1016/0924-8579(93)90045-7
Paul, M., Carrara, E., Retamar, P., Tängdén, T., Bitterman, R., Bonomo, R. A., et al. (2022). European Society of Clinical Microbiology and Infectious Diseases (ESCMID) guidelines for the treatment of infections caused by multidrug-resistant gram-negative bacilli (endorsed by European society of intensive care medicine). Clin. Microbiol. Infect. 28, 521–547. doi: 10.1016/j.cmi.2021.11.025
Peleg, A. Y., Seifert, H., and Paterson, D. L. (2008). Acinetobacter baumannii: emergence of a successful pathogen. Clin. Microbiol. Rev. 21, 538–582. doi: 10.1128/CMR.00058-07
Penesyan, A., Nagy, S. S., Kjelleberg, S., Gillings, M. R., and Paulsen, I. T. (2019). Rapid microevolution of biofilm cells in response to antibiotics. NPJ Biofilms Microbiomes 5:34. doi: 10.1038/s41522-019-0108-3
Peng, Q., Lin, F., and Ling, B. (2020). In vitro activity of biofilm inhibitors in combination with antibacterial drugs against extensively drug-resistant Acinetobacter baumannii. Sci. Rep. 10:18097. doi: 10.1038/s41598-020-75218-y
Perez, F., Hujer, A. M., Hujer, K. M., Decker, B. K., Rather, P. N., and Bonomo, R. A. (2007). Global challenge of multidrug-resistant Acinetobacter baumannii. Antimicrob. Agents Chemother. 51, 3471–3484. doi: 10.1128/AAC.01464-06
Piperaki, E. T., Tzouvelekis, L. S., Miriagou, V., and Daikos, G. L. (2019). Carbapenem-resistant Acinetobacter baumannii: in pursuit of an effective treatment. Clin. Microbiol. Infect. 25, 951–957. doi: 10.1016/j.cmi.2019.03.014
Poirel, L., Jayol, A., and Nordmann, P. (2017). Polymyxins: antibacterial activity, susceptibility testing, and resistance mechanisms encoded by plasmids or chromosomes. Clin. Microbiol. Rev. 30, 557–596. doi: 10.1128/CMR.00064-16
Poirel, L., Sadek, M., and Nordmann, P. (2021). Contribution of PER-type and NDM-type β-lactamases to Cefiderocol resistance in Acinetobacter baumannii. Antimicrob. Agents Chemother. 65:e0087721. doi: 10.1128/AAC.00877-21
Pollini, S., Boncompagni, S., Di Maggio, T., Di Pilato, V., Spanu, T., Fiori, B., et al. (2018). In vitro synergism of colistin in combination with N-acetylcysteine against Acinetobacter baumannii grown in planktonic phase and in biofilms. J. Antimicrob. Chemother. 73, 2388–2395. doi: 10.1093/jac/dky185
Pour, N. K., Dusane, D. H., Dhakephalkar, P. K., Zamin, F. R., Zinjarde, S. S., and Chopade, B. A. (2011). Biofilm formation by Acinetobacter baumannii strains isolated from urinary tract infection and urinary catheters. FEMS Immunol. Med. Microbiol. 62, 328–338. doi: 10.1111/j.1574-695X.2011.00818.x
Powers, M. J., and Trent, M. S. (2018). Expanding the paradigm for the outer membrane: Acinetobacter baumannii in the absence of endotoxin. Mol. Microbiol. 107, 47–56. doi: 10.1111/mmi.13872
Pybus, C. A., Felder-Scott, C., Obuekwe, V., and Greenberg, D. E. (2021). Cefiderocol Retains Antibiofilm Activity in Multidrug-Resistant Gram-Negative Pathogens. Antimicrob Agents Chemother. 65, e01194–e01120. doi: 10.1128/AAC.01194-20
Rando, E., Segala, F. V., Vargas, J., Seguiti, C., De Pascale, G., Murri, R., et al. (2021). Cefiderocol for severe Carbapenem-Resistant A. baumannii pneumonia: towards the comprehension of its place in therapy. Antibiotics 11:3. doi: 10.3390/antibiotics11010003
Repizo, G. D., Gagné, S., Foucault-Grunenwald, M. L., Borges, V., Charpentier, X., Limansky, A. S., et al. (2015). Differential role of the T6SS in Acinetobacter baumannii virulence. PLoS One 10:e0138265. doi: 10.1371/journal.pone.0138265
Rhouma, M., Beaudry, F., Thériault, W., and Letellier, A. (2016). Colistin in pig production: chemistry, mechanism of antibacterial action, microbial resistance emergence, and one health perspectives. Front. Microbiol. 7:1789. doi: 10.3389/fmicb.2016.01789
Richmond, G. E., Evans, L. P., Anderson, M. J., Wand, M. E., Bonney, L. C., Ivens, A., et al. (2016). The Acinetobacter baumannii two-component system AdeRS regulates genes required for multidrug efflux, biofilm formation, and virulence in a strain-specific manner. MBio 7:e00430. doi: 10.1128/mBio.00430-16
Roca, I., Espinal, P., Vila-Farres, X., and Vila, J. (2012). The Acinetobacter baumannii oxymoron: commensal hospital dweller turned pan-drug-resistant menace. Front. Microbiol. 3:148. doi: 10.3389/fmicb.2012.00148
Rodrı́guez-Bano, J., Martı́, S., Soto, S., Fernández-Cuenca, F., Cisneros, J. M., Pachón, J., et al. (2008). Biofilm formation in Acinetobacter baumannii: associated features and clinical implications. Clin. Microbiol. Infect. 14, 276–278. doi: 10.1111/j.1469-0691.2007.01916.x
Rodríguez-Martínez, J. M., Nordmann, P., Ronco, E., and Poirel, L. (2010). Extended-spectrum cephalosporinase in Acinetobacter baumannii. Antimicrob. Agents Chemother. 54:3484e8. doi: 10.1128/AAC.00050-10
Roy, S., Chatterjee, S., Bhattacharjee, A., Chattopadhyay, P., Saha, B., Dutta, S., et al. (2021). Overexpression of efflux pumps, mutations in the pumps’ regulators, chromosomal mutations, and AAC(6′)-Ib-cr are associated with fluoroquinolone resistance in diverse sequence types of neonatal Septicaemic Acinetobacter baumannii: a 7-year single Center study. Front. Microbiol. 12:602724. doi: 10.3389/fmicb.2021.602724
Roy, S., Chowdhury, G., Mukhopadhyay, A. K., Dutta, S., and Basu, S. (2022). Convergence of biofilm formation and antibiotic resistance in Acinetobacter baumannii infection. Front. Med. 9:793615. doi: 10.3389/fmed.2022.793615
Rumbaugh, K. P., and Sauer, K. (2020). Biofilm dispersion. Nat. Rev. Microbiol. 18, 571–586. doi: 10.1038/s41579-020-0385-0
Russo, A., Gavaruzzi, F., Ceccarelli, G., Borrazzo, C., Oliva, A., Alessandri, F., et al. (2022). Multidrug-resistant Acinetobacter baumannii infections in COVID-19 patients hospitalized in intensive care unit. Infection 50, 83–92. doi: 10.1007/s15010-021-01643-4
Sahu, P. K., Iyer, P. S., Oak, A. M., Pardesi, K. R., and Chopade, B. A. (2012). Characterization of eDNA from the clinical strain Acinetobacter baumannii AIIMS 7 and its role in biofilm formation. Sci. World J. 2012:973436. doi: 10.1100/2012/973436
Sánchez-Encinales, V., Álvarez-Marín, R., Pachón-Ibáñez, M. E., Fernández-Cuenca, F., Pascual, A., Garnacho-Montero, J., et al. (2017). Overproduction of outer membrane protein a by Acinetobacter baumannii as a risk factor for nosocomial pneumonia, Bacteremia, and mortality rate increase. J Infect Dis 215, 966–974. doi: 10.1093/infdis/jix010
Sato, Y., Ubagai, T., Tansho-Nagakawa, S., Yoshino, Y., and Ono, Y. (2021). Effects of colistin and tigecycline on multidrug-resistant Acinetobacter baumannii biofilms: advantages and disadvantages of their combination. Sci. Rep. 11:11700. doi: 10.1038/s41598-021-90732-3
Sato, Y., Unno, Y., Ubagai, T., and Ono, Y. (2018). Sub-minimum inhibitory concentrations of colistin and polymyxin B promote Acinetobacter baumannii biofilm formation. PLoS One 13, e0194556. doi: 10.1371/journal.pone.0194556
Sechi, L. A., Karadenizli, A., Deriu, A., Zanetti, S., Kolayli, F., Balikci, E., et al. (2004). PER-1 type beta-lactamase production in Acinetobacter baumannii is related to cell adhesion. Med. Sci. Monit. 10, CR180–CR184.
Shadan, A., Pathak, A., Ma, Y., Pathania, R., and Singh, R. P. (2023). Deciphering the virulence factors, regulation, and immune response to Acinetobacter baumannii infection. Front. Cell. Infect. Microbiol. 13:1053968. doi: 10.3389/fcimb.2023.1053968
Shin, J. H., Lee, H. W., Kim, S. M., and Kim, J. (2009). Proteomic analysis of Acinetobacter baumannii in biofilm and planktonic growth mode. J. Microbiol. 47, 728–735. doi: 10.1007/s12275-009-0158-y
Smani, Y., Fabrega, A., Roca, I., Sanchez-Encinales, V., Vila, J., and Pachon, J. (2014). Role of OmpA in the multidrug resistance phenotype of Acinetobacter baumannii. Antimicrob. Agents Chemother. 58, 1806–1808. doi: 10.1128/AAC.02101-13
Smani, Y., McConnell, M. J., and Pachón, J. (2012). Role of fibronectin in the adhesion of Acinetobacter baumannii to host cells. PLoS One 7:e33073. doi: 10.1371/journal.pone.0033073
Smet, A., Martel, A., Persoons, D., Dewulf, J., Heyndrickx, M., Catry, B., et al. (2008). Diversity of extended-Spectrum β-lactamases and class C β-lactamases among cloacal Escherichia coli isolates in Belgian broiler farms. Antimicrob. Agents Chemother. 52, 1238–1243. doi: 10.1128/AAC.01285-07
Soroosh, M., Furmanek-Blaszk, B., Łupkowska, A., Kuczynska-Wisnik, D., Stojowska-Swedrzynska, K., and Laskowsk, E. (2020). Mechanisms protecting Acinetobacter baumannii against multiple stresses triggered by the host immune response, antibiotics and outside-host environment. Int. J. Mol. Sci. 21:5498. doi: 10.3390/ijms21155498
Stahl, J., Bergmann, H., Göttig, S., Ebersberger, I., and Averhoff, B. (2015). Acinetobacter baumannii virulence is mediated by the concerted action of three phospholipases D. PLoS One 10:e0138360. doi: 10.1371/journal.pone.0138360
Sugawara, E., and Nikaido, H. (2012). OmpA is the principal nonspecific slow porin of Acinetobacter baumannii. J. Bacteriol. 194, 4089–4096. doi: 10.1128/JB.00435-12
Sun, Z., Kang, Y., Norris, M. H., Troyer, R. M., Son, M. S., Schweizer, H. P., et al. (2014). Blocking phosphatidylcholine utilization in Pseudomonas aeruginosa via mutagenesis of fatty acid, glycerol, and choline degradation pathways, confirms the importance of this nutrient source in vivo. PLoS One 9:e103778. doi: 10.1371/journal.pone.0103778
Sun, B., Liu, H., Jiang, Y., Shao, L., Yang, S., and Chen, D. (2020). New mutations involved in Colistin resistance in Acinetobacter baumannii. MSphere 5, e00895–e00819. doi: 10.1128/mSphere.00895-19
Tacconelli, E., Carrara, E., Savoldi, A., Harbarth, S., Mendelson, M., Monnet, D. L., et al. (2018). Discovery, research, and development of new antibiotics: the WHO priority list of antibiotic-resistant bacteria and tuberculosis. Lancet Infect Dis. 18, 318–327. doi: 10.1016/S1473-3099(17)30753-3
Tamma, P. D., Aitken, S. L., Bonomo, R. A., Mathers, A. J., van Duin, D., and Clancy, C. J. (2022). Infectious Diseases Society of America guidance on the treatment of AmpC β-lactamase-producing Enterobacterales, Carbapenem-resistant Acinetobacter baumannii, and Stenotrophomonas maltophilia infections. Clin. Infect. Dis. 74, 2089–2114. doi: 10.1093/cid/ciab1013
Tayabali, A. F., Nguyen, K. C., Shwed, P. S., Crosthwait, J., Coleman, G., and Seligy, V. L. (2012). Comparison of the virulence potential of Acinetobacter strains from clinical and environmental sources. PLoS One 7:e37024. doi: 10.1371/journal.pone.0037024
Thompson, M. G., Black, C. C., Pavlicek, R. L., Honnold, C. L., Wise, M. C., Alamneh, Y. A., et al. (2014). Validation of a novel murine wound model of Acinetobacter baumannii infection. Antimicrob. Agents Chemother. 58, 1332–1342. doi: 10.1128/AAC.01944-13
Tian, G. B., Adams-Haduch, J. M., Taracila, M., Bonomo, R. A., Wang, H. N., and Doi, Y. (2011). Extended-spectrum AmpC cephalosporinase in Acinetobacter baumannii: ADC-56 confers resistance to cefepime. Antimicrob. Agents Chemother. 55:4922e5. doi: 10.1128/AAC.00704-11
Tierney, A. R. P., Chin, C. Y., Weiss, D. S., and Rather, P. N. (2021). A LysR-type transcriptional regulator controls multiple phenotypes in Acinetobacter baumannii. Front. Cell. Infect. Microbiol. 11:778331. doi: 10.3389/fcimb.2021.778331
Tiku, V. (2022). Acinetobacter baumannii: virulence strategies and host Defense mechanisms. DNA Cell Biol. 41, 43–48. doi: 10.1089/dna.2021.0588
Tiku, V., Kofoed, E. M., Yan, D., Kang, J., Xu, M., Reichelt, M., et al. (2021). Outer membrane vesicles containing OmpA induce mitochondrial fragmentation to promote pathogenesis of Acinetobacter baumannii. Sci. Rep. 11:618. doi: 10.1038/s41598-020-79966-9
Tipton, K. A., Dimitrova, D., and Rather, P. N. (2015). Phase-variable control of multiple phenotypes in Acinetobacter baumannii strain AB5075. J. Bacteriol. 197, 2593–2599. doi: 10.1128/JB.00188-15
Tiseo, G., Brigante, G., Giacobbe, D. R., Maraolo, A. E., Gona, F., Falcone, M., et al. (2022). Diagnosis and management of infections caused by multidrug-resistant bacteria: guideline endorsed by the Italian Society of Infection and Tropical Diseases (SIMIT), the Italian Society of Anti-Infective Therapy (SITA), the Italian Group for Antimicrobial Stewardship (GISA), the Italian Association of Clinical Microbiologists (AMCLI) and the Italian Society of Microbiology (SIM). Int. J. Antimicrob. Agents 60:106611. doi: 10.1016/j.ijantimicag.2022.106611
Toker, A. (2005). The biology and biochemistry of diacylglycerol signalling. EMBO Rep. 6, 310–314. doi: 10.1038/sj.embor.7400378
Tomaras, A. P., Dorsey, C. W., Edelmann, R. E., and Actis, L. A. (2003). Attachment to and biofilm formation on abiotic surfaces by Acinetobacter baumannii: involvement of a novel chaperone-usher pili assembly system. Microbiology 149, 3473–3484. doi: 10.1099/mic.0.26541-0
Tomaras, A. P., Flagler, M. J., Dorsey, C. W., Gaddy, J. A., and Actis, L. A. (2008). Characterization of a two-component regulatory system from Acinetobacter baumannii that controls biofilm formation and cellular morphology. Microbiology 154, 3398–3409. doi: 10.1099/mic.0.2008/019471-0
Toyofuku, M., Inaba, T., Kiyokawa, T., Obana, N., Yawata, Y., and Nomura, N. (2016). Environmental factors that shape biofilm formation. Biosci. Biotechnol. Biochem. 80:1058701. doi: 10.1080/09168451.2015.1058701
Trebosc, V., Gartenmann, S., Tötzl, M., Lucchini, V., Schellhorn, B., Pieren, M., et al. (2019). Dissecting Colistin resistance mechanisms in extensively drug-resistant Acinetobacter baumannii clinical isolates. MBio 10, e01083–e01019. doi: 10.1128/mBio.01083-19
Tsuji, B. T., Pogue, J. M., Zavascki, A. P., Paul, M., Daikos, G. L., Forrest, A., et al. (2019). International consensus guidelines for the optimal use of the Polymyxins: endorsed by the American College of Clinical Pharmacy (ACCP), European Society of Clinical Microbiology and Infectious Diseases (ESCMID), Infectious Diseases Society of America (IDSA), International Society for Anti-infective Pharmacology (ISAP), Society of Critical Care Medicine (SCCM), and Society of Infectious Diseases Pharmacists (SIDP). Pharmacotherapy 39, 10–39. doi: 10.1002/phar.2209
Upmanyu, K., Haq, Q. M., and Singh, R. (2022). Factors mediating Acinetobacter baumannii biofilm formation: opportunities for developing therapeutics. Curr Res Microbial Sci 3:100131. doi: 10.1016/j.crmicr.2022.100131
Uppalapati, S. R., Sett, A., and Pathania, R. (2020). The outer membrane proteins OmpA, CarO, and OprD of Acinetobacter baumannii Confer a two-pronged Defense in facilitating its success as a potent human pathogen. Front. Microbiol. 11:589234. doi: 10.3389/fmicb.2020.589234
Urusova, D. V., Kinsella, R. L., Salinas, N. D., Haurat, M. F., Feldman, M. F., and Tolia, N. H. (2019). The structure of Acinetobacter-secreted protease CpaA complexed with its chaperone CpaB reveals a novel mode of a T2SS chaperone–substrate interaction. J. Biol. Chem. 294, 13344–13354. doi: 10.1074/jbc.RA119.009805
US Food and Drug Administration. (2019). Highlights of prescribing information: Fetroja (Cefiderocol). Available at: https://www.accessdata.fda.gov/drugsatfda_docs/label/2019/209445s000lbl.pdf.
Van der Meer-Janssen, Y. P., Van Galen, J., Batenburg, J. J., and Helms, J. B. (2010). Lipids in host-pathogen interactions: pathogens exploit the complexity of the host cell lipidome. Prog. Lipid Res. 49, 1–26. doi: 10.1016/j.plipres.2009.07.003
Venkataramana, G. P., Lalitha, A. K. V., Mariappan, S., and Sekar, U. (2022). Plasmid-mediated fluoroquinolone resistance in Pseudomonas aeruginosa and Acinetobacter baumannii. J Lab Physicians. 14, 271–277. doi: 10.1055/s-0042-1742636
Vila, J., Martí, S., and Sanchez-Céspedes, J. J. (2007). Porins, efflux pumps and multidrug resistance in Acinetobacter baumannii. J. Antimicrob. Chemother. 59, 1210–1215. doi: 10.1093/jac/dkl509
Volpicelli, L., Venditti, M., Ceccarelli, G., and Oliva, A. (2021). Place in therapy of the newly available armamentarium for multi-drug-resistant gram-negative pathogens: proposal of a prescription algorithm. Antibiotics 10:1475. doi: 10.3390/antibiotics10121475
Waack, U., Warnock, M., Yee, A., Huttinger, Z., Smith, S., Kumar, A., et al. (2018). CpaA is a glycan-specific Adamalysin-like protease secreted by Acinetobacter baumannii that inactivates coagulation factor XII. MBio 9, e01606–e01618. doi: 10.1128/mBio.01606-18
Wang, Y. C., Huang, T. W., Yang, Y. S., Kuo, S. C., Chen, C. T., Liu, C. P., et al. (2018). Biofilm formation is not associated with worse outcome in Acinetobacter baumannii bacteraemic pneumonia. Sci. Rep. 8:7289. doi: 10.1038/s41598-018-25661-9
Wang, Y. C., Kuo, S. C., Yang, Y. S., Lee, Y. T., Chiu, C. H., Chuang, M. F., et al. (2016). Individual or combined effects of meropenem, imipenem, sulbactam, colistin, and tigecycline on biofilm-embedded Acinetobacter baumannii and biofilm architecture. Antimicrob. Agents Chemother. 60, 4670–4676. doi: 10.1128/AAC.00551-16
Wang, S. H., Yang, K. Y., Sheu, C. C., Chen, W. C., Chan, M. C., Feng, J. Y., et al. (2022). The necessity of a loading dose when prescribing intravenous colistin in critically ill patients with CRGNB-associated pneumonia: a multi-center observational study. Crit. Care 26:91. doi: 10.1186/s13054-022-03947-9
Wang, C., Yang, D., Wang, Y., and Ni, W. (2022). Cefiderocol for the treatment of multidrug-resistant gram-negative bacteria: a systematic review of currently available evidence. Front. Pharmacol. 13:896971. doi: 10.3389/fphar.2022.896971
Weber, B. S., Kinsella, R. L., Harding, C. M., and Feldman, M. F. (2017). The secrets of Acinetobacter secretion. Trends Microbiol. 25, 532–545. doi: 10.1016/j.tim.2017.01.005
Weinberg, S. E., Villedieu, A., Bagdasarian, N., Karah, N., Teare, L., and Elamin, W. F. (2020). Control and management of multidrug resistant Acinetobacter baumannii: a review of the evidence and proposal of novel approaches. Infect Prev Pract. 2:100077. doi: 10.1016/j.infpip.2020.100077
Wences, M., Wolf, E. R., Li, C., Singh, N., Bah, N., Tan, X., et al. (2022). Combatting Planktonic and Biofilm Populations of Carbapenem-Resistant Acinetobacter baumannii with Polymyxin-Based Combinations. Antibiotics (Basel). 11:959. doi: 10.3390/antibiotics11070959
Whiteway, C., Breine, A., Philippe, C., and Van der Henst, C. (2022). Acinetobacter baumannii. Trends Microbiol. 30, 199–200. doi: 10.1016/j.tim.2021.11.008
Wong, D., Nielsen, T. B., Bonomo, R. A., Pantapalangkoor, P., Luna, B., and Spellberg, B. (2017). Clinical and pathophysiological overview of Acinetobacter infections: a century of challenges. Clin. Microbiol. Rev. 30, 409–447. doi: 10.1128/CMR.00058-16
Wright, M. S., Iovleva, A., Jacobs, M. R., Bonomo, R. A., and Adams, M. D. (2016). Genome dynamics of multidrug-resistant Acinetobacter baumannii during infection and treatment. Genome Med. 8:26. doi: 10.1186/s13073-016-0279-y
Xu, C. F., Bilya, S. R., and Xu, W. (2019). adeABC efflux gene in Acinetobacter baumannii. N. Microbes N. Infect. 30:100549. doi: 10.1016/j.nmni.2019.100549
Yamano, Y., Ishibashi, N., Kuroiwa, M., Takemura, M., Sheng, W. H., and Hsueh, P. R. (2021). Characterisation of cefiderocol-non-susceptible Acinetobacter baumannii isolates from Taiwan. J. Glob. Antimicrob. Resist. 28, 120–124. doi: 10.1016/j.nmni.2019.100549
Yang, C. H., Su, P. W., Moi, S. H., and Chuang, L. Y. (2019). Biofilm formation in Acinetobacter baumannii: genotype-phenotype correlation. Molecules 24:1849. doi: 10.3390/molecules24101849
Yoon, E. J., Chabane, Y. N., Goussard, S., Snesrud, E., Courvalin, P., Dé, E., et al. (2015). Contribution of resistance-nodulation-cell division efflux systems to antibiotic resistance and biofilm formation in Acinetobacter baumannii. MBio 6, e00309–e00315. doi: 10.1128/mBio.00309-15
Zeighami, H., Valadkhani, F., Shapouri, R., Samadi, E., and Haghi, F. (2019). Virulence characteristics of multidrug resistant biofilm forming Acinetobacter baumannii isolated from intensive care unit patients. BMC Infect. Dis. 19:629. doi: 10.1186/s12879-019-4272-0
Keywords: Acinetobacter baumannii, cancer, biofilm, skin and soft-tissue infections, colistin, carbapenem, crab, cefiderocol
Citation: Cavallo I, Oliva A, Pages R, Sivori F, Truglio M, Fabrizio G, Pasqua M, Pimpinelli F and Di Domenico EG (2023) Acinetobacter baumannii in the critically ill: complex infections get complicated. Front. Microbiol. 14:1196774. doi: 10.3389/fmicb.2023.1196774
Received: 30 March 2023; Accepted: 05 June 2023;
Published: 22 June 2023.
Edited by:
Axel Cloeckaert, Institut National de recherche pour l’agriculture, l’alimentation et l’environnement (INRAE), FranceReviewed by:
Wasan Katip, Chiang Mai University, ThailandCopyright © 2023 Cavallo, Oliva, Pages, Sivori, Truglio, Fabrizio, Pasqua, Pimpinelli and Di Domenico. This is an open-access article distributed under the terms of the Creative Commons Attribution License (CC BY). The use, distribution or reproduction in other forums is permitted, provided the original author(s) and the copyright owner(s) are credited and that the original publication in this journal is cited, in accordance with accepted academic practice. No use, distribution or reproduction is permitted which does not comply with these terms.
*Correspondence: Enea Gino Di Domenico, ZW5lYS5kaWRvbWVuaWNvQHVuaXJvbWExLml0
Disclaimer: All claims expressed in this article are solely those of the authors and do not necessarily represent those of their affiliated organizations, or those of the publisher, the editors and the reviewers. Any product that may be evaluated in this article or claim that may be made by its manufacturer is not guaranteed or endorsed by the publisher.
Research integrity at Frontiers
Learn more about the work of our research integrity team to safeguard the quality of each article we publish.