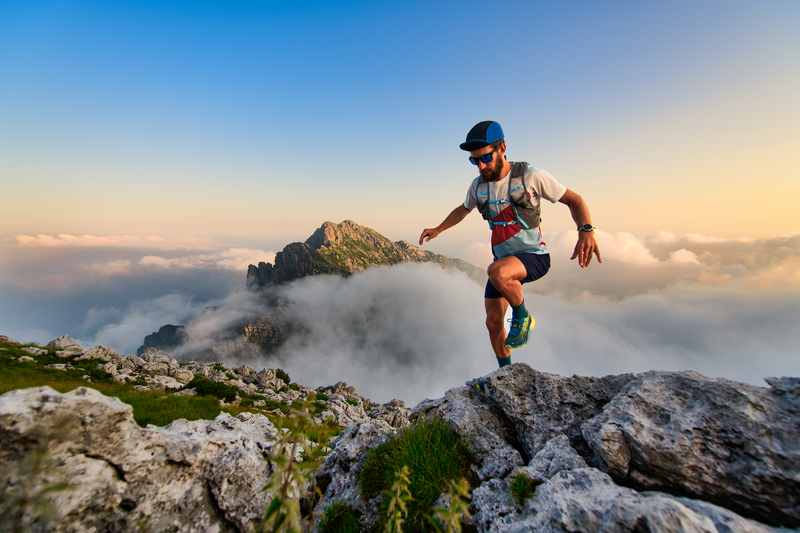
94% of researchers rate our articles as excellent or good
Learn more about the work of our research integrity team to safeguard the quality of each article we publish.
Find out more
REVIEW article
Front. Microbiol. , 09 June 2023
Sec. Infectious Agents and Disease
Volume 14 - 2023 | https://doi.org/10.3389/fmicb.2023.1196700
This article is part of the Research Topic Reviews in Microbial Pathogenesis View all 17 articles
A correction has been applied to this article in:
Corrigendum: Advancements in understanding the molecular and immune mechanisms of Bartonella pathogenicity
Bartonellae are considered to be emerging opportunistic pathogens. The bacteria are transmitted by blood-sucking arthropods, and their hosts are a wide range of mammals including humans. After a protective barrier breach in mammals, Bartonella colonizes endothelial cells (ECs), enters the bloodstream, and infects erythrocytes. Current research primarily focuses on investigating the interaction between Bartonella and ECs and erythrocytes, with recent attention also paid to immune-related aspects. Various molecules related to Bartonella’s pathogenicity have been identified. The present review aims to provide a comprehensive overview of the newly described molecular and immune responses associated with Bartonella’s pathogenicity.
Bartonellae are Gram-negative facultative intracellular bacteria (Brenner et al., 1993) that were first described in 1909. Since the last reclassification in 1993, the number of Bartonellae species has increased to 45 as of Okaro et al. (2017), and new species continue to be identified in recent years (Gutierrez et al., 2020; do Amaral et al., 2022a,b; Liu et al., 2022). Phylogenetic analyses have categorized the genus Bartonella into three phylogenetic clades, which include the honeybee symbiont Bartonella apis, the pathogenic Bartonella tamiae, and the eubartonellae, which are further separated into Bartonella australis and four distinct lineages (Segers et al., 2017). Bartonella bacilliformis and Bartonella ancashensis, which are human pathogens, belong to lineage 1. Bartonella species specific to ruminants are found in lineage 2. The most abundant Bartonella species are those in lineage 3 and 4, which infect a diverse range of mammalian hosts (Wagner and Dehio, 2019). Recently, two bat-associated Bartonella strains showed significant differences with other lineages in phylogenetic relationship analysis (Goncalves-Oliveira et al., 2023). Transmission of Bartonellae between hosts is primarily mediated by diverse blood-sucking arthropod vectors such as fleas, body lice, ticks, sandflies, and others (Breitschwerdt et al., 2010a). These pathogens have a broad range of mammalian hosts, including, but not limited to, primates, rodents, and cats (Gundi et al., 2004; Dehio, 2005). However, each Bartonella species is typically adapted to a specific mammalian host (Deng et al., 2012).
In arthropods, the life cycle of most Bartonella species is divided into replication in the midgut of intestinal tracts and spreading through excretion (Foil et al., 1998). The pathogen is shed within arthropod feces onto mammalian skin and can be superficially inoculated into the derma by scratching or biting (Okujava et al., 2014). The derma-niched Bartonella might penetrate the endothelial cells (ECs) facilitated by dendritic cells (DCs) and Bartonella effector proteins (Beps) (Siamer and Dehio, 2015; Fromm and Dehio, 2021). Subsequently, the ECs-residing bacteria enter the bloodstream, invade erythrocytes, multiply inside them, and await the next round of transmission when arthropods bite the infected mammalian host again (Harms and Dehio, 2012; Pulliainen and Dehio, 2012; Fromm and Dehio, 2021).
Bartonella bacilliformis, Bartonella quintana, and Bartonella henselae are three major species that trigger pathological angiogenesis during infection in humans. These three species are the etiological agents of Carrion’s disease, trench fever, and cat scratch disease (CSD), respectively (Rolain et al., 2004; Breitschwerdt et al., 2010a; Harms and Dehio, 2012; Liu et al., 2012). The symptoms and syndromes of bartonelloses are diverse, ranging from bacillary angiomatosis (BA), bacillary peliosis hepatis, chronic asymptomatic bacteremia, and infectious endocarditis, to neurological disorders. CSD, a relatively common zoonotic infection acquired from cats or cat fleas carrying B. henselae, is characterized by enlarged regional lymph nodes and fever, particularly in children and adolescents (Biancardi and Curi, 2014; Chang et al., 2016; Okaro et al., 2021). Atypical manifestations of CSD, such as hepatic and/or splenic lesions, discitis, granulomatous conjunctivitis, endocarditis, myocarditis, neuroretinitis, osteomyelitis, and encephalomeningitis, can mimic serious disorders. Moreover, B. henselae and B. clarridgeiae have been detected in blood samples from human donors at a Brazilian blood bank (Pitassi et al., 2015), indicating their presence in healthy humans, and highlighting the possibility of undetected cases of Bartonella infection in humans. B. bacilliformis, the pathogen of Carrion’s disease, is primarily found in the Andean valleys of South America (Sanchez Clemente et al., 2012). This disease is characterized by an acute phase, known as Oroya fever, marked by fever, pallor, hemolytic anemia, myalgia, and arthralgia, followed by a chronic phase characterized by the development of vascular proliferative lesions on the skin, known as verruga peruana, which can persist for several months or even years (Maguina et al., 2009; Harms and Dehio, 2012; Sanchez Clemente et al., 2012; Gomes et al., 2016a). B. quintana, the etiological agent of trench fever, is naturally restricted to human hosts and louse vectors. The pathogen is now frequently identified among urban homeless and marginalized populations in the United States and Europe, and is responsible for various conditions, including endocarditis, pericardial effusion, bacillary angiomatosis-peliosis, and even asymptomatic bacteremia (Leibler et al., 2016; Sasaki et al., 2021).
This review intends to summarize recent findings about the pathogenicity of Bartonella and the immune response in hosts elicited by the infection, to provide valuable insights for future research.
Blood-sucking arthropods are important for the transmission of Bartonella. Various blood-sucking arthropods have been identified as vectors capable of transmitting Bartonella, such as the sand flies Lutzomyia verrucarum for B. bacilliformis (Minnick et al., 2014), cat flea Ctenocephalides felis for B. henselae (Chomel et al., 2009; Harms and Dehio, 2012; Duscher et al., 2018), human body lice Pediculus humanus corporis for B. quintana (Byam and Lloyd, 1920), and the flea Ctenophthalmus nobilis for Bartonella grahamii and Bartonella taylorii (Bown et al., 2004). Most Bartonellae reside in the intestinal tract of arthropods, where they replicate and are eventually transmitted to mammalian hosts through vector defecation (Figure 1B; Chomel et al., 2009). Although Bartonella bacteria (such as B. henselae) typically survive in arthropod feces for several days (Higgins et al., 1996), B. quintana remains infectious for up to 1 year in louse feces (Kostrzewski, 1950; Chomel et al., 2009), making it more likely for hosts to contract an infection. Bartonellae can also infect the salivary glands of arthropods, as evidenced by the transmission of B. henselae through tick saliva to an artificial membrane feeding system (Cotte et al., 2008). Additionally, the transovarial transmission of B. henselae Marseille, Bartonella schoenbuchensis DSMZ 13525, and B. grahamii ATCC700132 from female tick Ixodes ricinus to their offspring via eggs may also occur, as Bartonella DNA has been detected in eggs laid by the three kinds of Bartonella-positive female ticks and in hatched larvae (Figure 1A; Krol et al., 2021). The spread of B. bacilliformis by L. verrucarum represents an exception of vector transmission. The current research has shown that B. bacilliformis can colonize the midgut of L. verrucarum (Battisti et al., 2015), yet the mechanism by which midgut-residing B. bacilliformis in sandflies enters human hosts remains unclear (Garcia-Quintanilla et al., 2019).
Figure 1. Model of Bartonella infection cycle. (A) The process of Bartonella transmission in the hosts. (B) The ways of Bartonellae enter the blood. There are two ways of Bartonellae with VirB/VirD4 T4SS enter the blood. The first way may be through the host’s macrophages, endothelial cells and other migration into the blood. The second way is through lymphatic circulation into the blood. Bartonellae absent of VirB/VirD4 T4SS, such as B. bacilliformis, may enter the blood with feces directly through the wound bitten by sand flies (the orange dashed line arrow). In the figure, the solid line arrow indicates the confirmed process, and the dashed line arrow indicates conjecture. Some elements in the figure were drawn by Figdraw.
Climate change indirectly affects the spread of Bartonella by perturbing the proliferation of blood-sucking arthropods (Chamberlin et al., 2002). When the number of blood-sucking arthropods increases during warm and humid conditions such as during an El Niño, the infection rate of Bartonella also seems to increase correspondingly (Chamberlin et al., 2002; Clemente et al., 2016). These findings highlight the critical role of blood-sucking arthropods in the transmission of Bartonella.
The flea C. felis is the primary means of transmitting B. henselae from cat to cat. Studies have shown that healthy cats do not contract Bartonella infection or seroconversion after sharing food or playing with highly bacteremic cats (Chomel et al., 1996; Abbott et al., 1997). However, fleas that have taken a blood meal from highly bacteremic cats can effectively transmit B. henselae to specific-pathogen-free (SPF) cats (Figure 1A; Chomel et al., 1996). Although there is no evidence to prove that B. henselae can be spread among cats through salivation, B. henselae DNA has been detected in the saliva of infected cats (Oskouizadeh et al., 2010). Bartonella may be present in the saliva of infected mammals, but the bacteria are fastidious haemophilia (Liu and Biville, 2013), and therefore, the concentration of Bartonella in the blood of a highly bacteremic host is likely higher than that in its saliva. Furthermore, different mechanisms exist in certain arthropods that enable the transmission of Bartonella from arthropods to hosts. It is important to note that the transmission of Bartonella from cat to human is usually achieved through cat scratches due to contact with B. henselae-contaminated flea feces.
The importance of some arthropod vectors for the transmission of B. henselae underscores the likelihood that this applies to most Bartonella species (Pulliainen and Dehio, 2012). After Bartonella-contaminated feces are inoculated to the wound of the host, the bacteria invade the dermis layer of the host and begin their journey of invasion (Figure 1B).
Bartonella initiates its infection cycle in a mammalian host after replication in the midgut of arthropod vectors (Harms and Dehio, 2012). When these arthropods suck blood from mammals, the affected area on the host’s skin experiences irritation, followed by scratching that can lead to the inoculation of Bartonella-containing insect feces into the dermis. Transmission of Bartonella can also occur through traumatic contact with infected animals (Chomel et al., 2009). After superficially inoculating the dermis, the bacteria sequentially access the dermal and blood-seeding niches. During dermal niche colonization, migrating cells such as DCs or macrophages are likely kidnapped by Bartonella to reach the blood-seeding niche (Fromm and Dehio, 2021). Transmission from the dermal niche to the blood-seeding niche may also occur via the lymphatic system (Hong et al., 2017). In the blood-seeding niche, the cell type is still puzzling and the bacteria most likely colonize ECs (Eicher and Dehio, 2012). It is believed that the blood-seeding niche releases bacteria into the bloodstream periodically, which invade erythrocytes and replicate until the critical limit of eight bacteria on average per erythrocyte is reached (Litwin and Johnson, 2005; Pulliainen and Dehio, 2012). After the apoptosis of infected erythrocytes, bacterial cells are released again into the bloodstream to invade new erythrocytes for replication, which may partly explain the periodic occurrence of host bacteremia after infection (Harms and Dehio, 2012). Once arthropods bite the infected mammals again, Bartonella enters the next round of transmission (Figure 1A; Harms and Dehio, 2012; Fromm and Dehio, 2021).
Bartonellae (lineage 3 and lineage 4), along with other bacteria like Helicobacter pylori, Legionella pneumophila, and Brucella spp. (Cascales and Christie, 2003; Harms et al., 2017), utilize the VirB/VirD4 type IV secretion system (T4SS) as a key virulence factor to infect humans and other mammals’ target cells (Cascales and Christie, 2003). The pathogens translocate Bartonella effector proteins (Beps) into host cells via the VirB/VirD4 T4SS, which orchestrates multiple cellular processes in host cells, including modulating the immune response and subverting cellular functions to benefit the bacterial survival and proliferation (Okujava et al., 2014; Sorg et al., 2020). The VirB/VirD4 T4SS and the translocated Beps are the best characterized Bartonella-specific virulence factors. The invasion of B. henselae into human ECs occurs in two distinct pathways, either as a single bacterium through endocytosis or as bacterial aggregates in the form of invasomes (Truttmann et al., 2011). Induced by F-actin rearrangements and stress fiber formation, the formation of invasome relies on the functional VirB/VirD4 T4SS associated with either BepG alone or the combination of BepC and BepF (Truttmann et al., 2011). BepF is comprised of three Bep intracellular delivery domains, with two non-terminal domains triggering invasome formation in conjunction with BepC (Wagner and Dehio, 2019). BepC contributes to the formation of the invasome by modulating the F-actin cytoskeleton. Recently, it has been discovered that BepC triggers stress fiber formation by activating the RhoA GTPase signaling cascade through the recruitment of the Rho guanine nucleotide exchange factor H1 (GEF-H1) to the plasma membrane (Marlaire and Dehio, 2021).
The VirB/VirD4 T4SS system also plays a crucial role in the migration of Bartonella from the dermal niche to the blood-seeding niche. It is achieved by translocating BepE into host cells, which inhibits cell fragmentation caused by BepC or other Beps and ensures the migration of Bartonella-infected DCs to deliver the pathogen from the derma site to the blood-seeding niche (Okujava et al., 2014). The distributional pattern of VirB/VirD4 T4SS and Beps is proposed to correlate with arthropod vectors’ blood-feeding behavior and the mode of bacterial transmission (Dehio and Tsolis, 2017). Bartonellae that are absent of VirB/VirD4 T4SS, such as B. bacilliformis, are exclusively transmitted by sandflies. However, sandflies display a violent mode of blood-feeding by damaging microvessels of the skin to allow them to access the freshly draining blood (Ribeiro, 1995; Serafim et al., 2021), which potentially provides a direct route for Bartonella to invade the bloodstream (Dehio and Tsolis, 2017).
Furthermore, the presence of either B. henselae or B. quintana enhances the proliferation of human ECs and prevents apoptosis. This anti-apoptotic effect is mediated by the pathogen’s BepA protein, which interacts with human adenylyl cyclase 7 (AC7), followed by elevated cAMP levels (Pulliainen et al., 2012). The prolonged survival of host cells allows for an efficient time course for intracellular bacteria to have multiple replications, which is believed to be critical for a successful infection from the primary niche to the bloodstream. However, the BepA ortholog in B. tribocorum lacks anti-apoptotic activity (Dehio, 2008), indicating that orthologous BepA proteins may have varying functions in different bacteria.
Bartonella species have been found to inhabit various host cells, including mononuclear phagocytes, CD34+ progenitor cells, and mesenchymal stromal cells (MSCs) (Mändle et al., 2005), but the vascular endothelium is considered the primary blood-seeding niche for Bartonella colonization in the mammalian host (Deng et al., 2012, 2019). To infect the ECs of blood vessels in a mammalian host, Bartonellae must first traverse the extracellular matrix of the cells. The degradation of extracellular matrix proteins has been confirmed to be facilitated by the interaction between fibrinolysis and several pathogen proteins (Lähteenmäki et al., 2001). Bartonella utilizes a metabolic enzyme known as α-enolase or phosphopyruvate hydratase, which is involved in the synthesis of pyruvate, to act as a plasminogen receptor and mediate the activation of plasmin and extracellular matrix degradation (Figure 2; Díaz-Ramos et al., 2012; Cappello et al., 2017). Plasmin, the proteolytically active form of plasminogen, is responsible for promoting fibrin dissolution in the extracellular matrix of host cells (Keragala and Medcalf, 2021). The sequence of α-enolase in B. henselae is highly homologous to that of many other Bartonella species, and they all possess typical plasminogen-binding modes. Accordingly, the α-enolase of Bartonella has been hypothesized to function as a plasminogen-binding protein, which has been recently confirmed in experiments (Deng et al., 2019). As a result, the enolase-plasminogen interaction is identified as one potential mechanism exploited by Bartonella to loosen and degrade the extracellular matrix of ECs before entering (Deng et al., 2019).
Figure 2. The mechanism of Bartonella infects vascular endothelial cells, mesenchymal stromal cells and erythrocytes. *Deformin is only found in B. bacilliformis and B. henselae.
To efficiently adhere to extracellular matrix proteins of ECs, Bartonella expresses surface adhesins, such as Bartonella adhesin A (BadA) in B. henselae (Riess et al., 2004), variably expressed outer membrane proteins (Vomps) in B. quintana (Zhang et al., 2004), and Bartonella repeat protein A (BrpA) in Bartonella vinsonii (Gilmore et al., 2005). These adhesins belong to the trimeric autotransporter adhesin (TAA) family (Linke et al., 2006). Among them, VompC confers the ability of B. quintana to bind collagen IV in extracellular matrix proteins of ECs; BadA is essential for the attachment of B. henselae to extracellular matrix proteins including collagen, laminin, and fibronectin, with fibronectin being particularly important (Kaiser et al., 2008; Muller et al., 2011). Fibronectin orchestrates various cellular processes of ECs, including cell adhesion, spreading, migration, proliferation, and apoptosis (Patten and Wang, 2021; Vaca et al., 2022). The binding of BadA to fibronectin occurs at repetitive motifs in the neck/stalk region of BadA and is a cumulative effect leading to rapid saturation (Thibau et al., 2022b). This interaction helps the bacteria to adhere to host cells. As another important virulence factor in Bartonella, BadA was described to negatively affect the Beps-translocating activity of VirB/D4 T4SS, while the function of BadA itself remain intact when both factors were co-expressed in B. henselae (Lu et al., 2013).
The outer membrane protein BadA is also responsible for Bartonella-induced vasoproliferation. It activates hypoxia-inducible factor-1 and stimulates the secretion of pro-angiogenic cytokines, such as vascular endothelial growth factor (VEGF) and C-X-C motif chemokine ligand (CXCL) 8 (Riess et al., 2004; Kempf et al., 2005; McCord et al., 2006), contributing to Bartonella-induced vasoproliferation. However, the observed BadA-dependent VEGF secretion was limited to certain cells and not in B. henselae-infected ECs (Kempf et al., 2001). Another autotransporter protein, Bartonella angiogenic factor A (BafA), is identified as a key Bartonella-derived mitogenic factor (Tsukamoto et al., 2020, 2022). BafA acts as a VEGF analog that promotes angiogenesis by binding to vascular endothelial growth factor receptor-2 (VEGFR2) on the EC surface and activating the MAPK/ERK pathway, which facilitates EC proliferation, tube formation, and subsequent angiogenesis (Tsukamoto et al., 2020). BafA family proteins are common among many Bartonella species, and BafA-triggered angiogenesis plays a central role in the formation of vasoproliferative lesions during Bartonella infection. Studies have shown that B. quintana, B. henselae, and B. elizabethae induce angiogenesis and proliferation by stimulating the VEGFR2 signaling pathway through the production of BafA (Tsukamoto et al., 2020, 2022; Suzuki et al., 2023). In addition, other cell types recruited to the vasoproliferative lesions, including monocytes, macrophages, and MSCs, stimulate EC proliferation through the production of VEGF and CXCL8. In particular, MSCs distributed in various tissues, including the bone marrow and the adipose tissue, play the role of a B. henselae reservoir and modulator of EC functions. B. henselae-infected MSCs release angiogenic factors, such as CXCL8, VEGF, etc., leading to the induction of a proangiogenic phenotype in ECs. Toll-like receptor 2 (TLR2), nucleotide-binding oligomerization domain-containing protein 1 (NOD1), and epidermal growth factor receptor (EGFR) are identified as the receptors involved in the recognition of B. henselae by MSCs (Figure 2; Scutera et al., 2021). Meanwhile, B. henselae-infected MSCs also release proinflammatory chemokines, which recruit monocytes/macrophages in the vasoproliferative lesions. The angiogenic factors produced by phagocytic cells play a central role in mediating angiogenesis (Resto-Ruiz et al., 2002; McCord et al., 2006; O’Rourke et al., 2015). The main virulence factors involved in the invasion of the ECs are summarized in Table 1.
The induction of intra-erythrocytic bacteremia is a hallmark of Bartonellae infection in mammalian hosts. The infection process of erythrocytes by Bartonella is divided into three main stages: adhesion, erythrocyte deformation, and invasion, with various virulence factors involved (Table 1).
The initial step is the adhesion of Bartonella to erythrocytes. In some Bartonella species, a type of T4SS called Trw has been identified as a key factor promoting host-specific erythrocyte infection, leading to prolonged bacteremia (Frank et al., 2005). Upon attachment, Bartonellae invade and penetrate mature erythrocytes, although the exact mechanism of this process is not fully understood. However, several necessary factors have been identified. The invasion-associated locus B genes (ialB) are crucial for the invasive behavior of the bacteria. IalB protein locates on the outer membrane of B. henselae (Coleman and Minnick, 2001; Chenoweth et al., 2004), and the inner membrane of B. bacilliformis (Mitchell and Minnick, 1995). The loss of ialB does not significantly affect bacterial adhesion, yet it leads to a ten-fold reduction in the number of bacteria residing in erythrocytes (Figure 2; Deng et al., 2016). Another important factor is deformin, a small molecule with protease resistance, heat resistance, and high affinity with albumin (Iwaki-Egawa and Ihler, 1997; Hendrix and Kiss, 2003). During infection, B. bacilliformis interacts with human erythrocytes to produce trenches, pits, conical invaginations, and internal vacuoles in the erythrocyte membrane (Mernaugh and Ihler, 1992; Xu et al., 1995), which is stimulated by deformin (Figure 2). Similarly, deformation activity on the erythrocyte membrane has also been reported in B. henselae (Iwaki-Egawa and Ihler, 1997).
A recent study showed that the CAMP-like factor autotransporter (CFA) of B. taylorii is crucial for infecting erythrocytes. Mice infected with a mutant strain lacking the cfa locus remained free of bacteremia, while the wild-type strain caused infection (Siewert et al., 2022b). CFA was first identified as an autotransporter virulence protein with potential cohemolysin activity in B. henselae (Litwin and Johnson, 2005). Autotransporters are a family of proteins secreted by gram-negative bacteria through the type V secretion mechanism, and they transport themselves through the outer membrane. The extracellular passenger domain at the N-terminal region of autotransporters often binds to a β-barrel folded by the C-terminal region in the bacterial outer membrane (Tame, 2011). Comparative genomics analysis has shown that the cfa locus is present in all Eubartonellae, with a hypervariable antigenic region (Siewert et al., 2022b). Meanwhile, CFA is one of the major targets for protective neutralizing antibodies that can prevent the attachment of pathogens to erythrocytes independent of complement or Fc receptors in hosts (Siewert et al., 2022b).
The undetectable situation of Bartonella after erythrocyte invasion plays a significant role in its spread, aided by the mobility and the lack of organelles in mature red blood cells, posing challenges for treatment selection. Research on Bartonella will provide insight into intracellular parasitology.
Bartonella can cause a broad spectrum of diseases in humans, and the severity of symptoms is closely related to the immune status of the patients. It can be self-limited in immunocompetent individuals (Windsor, 2001; Gai et al., 2015), and it also can be very serious and even fatal for individuals with human immunodeficiency virus (HIV) or advanced immunosuppression (Mosepele et al., 2012). Bartonellae can establish long-lasting intraerythrocytic bacteremia, therefore, the intraerythrocytic cellular niche in host cells poses a daunting challenge to antibacterial immune defense. The lack of the major histocompatibility complex (MHC) on the cell surface of erythrocytes prevents antigen presentation, making MHC-dependent cytotoxicity an ineffective response. However, other immune responses are employed by the host to eliminate the pathogen.
Macrophages and DCs are innate immune cells that are vital for host’s defense against Bartonella invasion. In vitro, co-incubation of murine macrophage cell line J774 with B. henselae led to rapid internalization of the bacterium by the macrophages. The phagocytosis of unstimulated murine macrophages to B. henselae achieved full saturation within 4 h, accompanied by a significant increase in the expression of tumor necrosis factor α, interleukin (IL)-1β, and IL-6 by J774 (Musso et al., 2001). Following phagocytosis of B. henselae, DCs highly express the aforementioned three cytokines and upregulate the expression of CXCL8, CXCL1, and CXCL13, which recruit neutrophils and B cells to the site of infection (Vermi et al., 2006). The secretion of pro-inflammatory cytokines contributes to the formation of characteristic granuloma in CSD, which promotes the confinement of B. henselae to specific sites, limiting its spread within the host.
Mounting antibody responses that neutralize the pathogen is proved to be critical for clearing Bartonella cells in the blood of infected mice (Koesling et al., 2001). Studies have demonstrated that antibodies can prevent bacterial attachment to erythrocytes and suppress bacteremia independent of complement or Fc receptors (Pulliainen et al., 2012). The antibodies that interfere with B. taylorii adhesion to erythrocytes in vitro belong to the IgG2a and IgG3 isotypes (Siewert et al., 2022b; Figure 3). In this humoral immune defense process, the bacterial surface determinant CFA was recently identified as a target for protective antibodies (Siewert et al., 2022b). Furthermore, antibodies against B. bacilliformis could confer long-term immune protection. Individuals living in high-risk areas for B. bacilliformis have been found to possess significantly higher serum IgG levels specific to B. bacilliformis compared to those living in areas where the first outbreak of Carrion’s disease occurred (Gomes et al., 2016b). During CSD infection, IgG and IgA are the main antibodies induced, with IgG1 being the major subclass of IgG (McGill et al., 1998). Specific IgM is the predominant antibody during the acute infectious phase in B. bacilliformis-infected patients and elevated specific IgG levels indicate a history of infection (Pons et al., 2017). However, in vitro experiments have shown extensive cross-reactivity between B. henselae to various microorganisms, such as Treponema pallidum and Chlamydia group (McGill et al., 1998). The above humoral immune processes can be seen in Figure 3.
The complement system, an important part of the innate immune system, plays a key role in defending against Bartonella before it enters erythrocytes in blood vessels and other cell types in peripheral tissues. An in vitro experiment showed that the alternative pathway of the complement system was primarily involved, with activation of the classical pathway also detected when human-derived non-immune serum was exposed to B. henselae (Rodriguez-Barradas et al., 1995; Figure 3).
In addition, humoral immune defense is essential in preventing and eliminating vertical transmission of Bartonella. Vertical transmission of Bartonella exists in mice and has been reported in a patient (Breitschwerdt et al., 2010b). Siewert et al. (2022a) recently found that only B cell-deficient offspring developed persistent bacteremia upon vertical transmission of B. taylorii in mice, whereas the corresponding wild-type offspring cleared the infection and developed protective immune memory. This result severely challenges the proposal that immunological tolerance in offspring due to vertical transmission is a mechanism of Bartonella persistence (Kosoy et al., 1998).
Besides, Bartonella infection stimulates the secretion of certain cytokines in the host. Specifically, interferon γ, which activates macrophages to destroy intracellular pathogens, is highly expressed in the peripheral blood of cats with bacteremia (Kabeya et al., 2009) and in the spleen cells of B. henselae-infected mice (Kabeya et al., 2007). Additionally, innate immune defense mechanisms, such as mannan-binding proteins, may also play a role in the recognition and elimination of B. henselae (Ezekowitz and Stahl, 1988; Rodriguez-Barradas et al., 1995). In contrast, cell-mediated immune response (CMI) is crucial for eliminating pathogens when Bartonella, especially B. henselae, enters host cells (excluding erythrocytes) (Kabeya et al., 2009).
Bartonella has also developed several strategies to evade the host’s immune response. Evasion of innate immune response is a prerequisite for Bartonella to establish intracellular infection in the host. When Bartonella is inoculated at subcutaneous or intradermal sites, it is first and foremost exposed to resident DCs and macrophages. B. tribocorum has been reported to be resistant to phagocytosis in rats (Hong et al., 2017). The phagocytosis resistance is likely delivered by the structural mechanism of bacterial aggregates and is validated by the fact that a badA knock-out Bartonella strain is more susceptible to phagocytosis by macrophages (Riess et al., 2004). Even if engulfed by macrophages, Bartonella can inhibit pyroptosis and suppress the expansion of the inflammatory response (Hong et al., 2017). Alternatively, it can form a unique Bartonella-containing vacuole (BCV) that can delay lysosomal targeting and destruction (Kyme et al., 2005).
As gram-negative bacteria, Bartonellae possess a lipopolysaccharide (LPS) component on the outer membrane that is a well-known ligand for Toll-like receptor 4 (TLR4). However, Bartonella LPS is poorly recognized by TLR4, which may also contribute to the low efficiency of phagocytosis. LPS of B. quintana and B. henselae exhibits several unusual features, including a unique structure, lipid A with a long fatty acid side chain, and a lack of an O-chain polysaccharide (Zahringer et al., 2004; Popa et al., 2007; Malgorzata-Miller et al., 2016). Furthermore, the LPS of B. quintana is an antagonist of TLR4, inhibiting the expression of cytokines, including IL-1β, IL-6, and tumor necrosis factor α, generated by TLR4-linked pathways (Popa et al., 2007; Mosepele et al., 2012; Malgorzata-Miller et al., 2016). During Bartonella infection, TLR4 does not play a significant role in pathogen recognition. Instead, the host’s innate immune response is triggered through TLR2 recognition (Vermi et al., 2006; Matera et al., 2008). In the case of Candida albicans infection, TLR2 has been shown to induce IL-10 secretion and Treg cell survival, thereby inhibiting inflammation (Netea et al., 2004). However, it is still unknown whether Bartonella infection triggers a similar response.
An antagonistic mechanism against the complement system has also been identified in Bartonella. BadA is proven to be critical for bacterial resistance to the host complement system. A badA-knockout Bartonella birtlesii mutant was susceptible to mouse serum, whereas the wild-type B. birtlesii expressing active BadA was resistant, which could be neutralized by anti-BadA antibodies (Deng et al., 2012).
Antigenic variation is another efficient strategy for Bartonella to evade the host immune response (Figure 3). Two important virulence factors, BadA in B. henselae (Riess et al., 2004) and the Vomp family in B. quintana (Zhang et al., 2004), have great potential to evade the host immune response through antigenic variation. The stem domain of badA and vomp contains modular and repetitive DNA sequences that perhaps increase the recombination frequency of the corresponding genes (Linke et al., 2006), while the internal structure of the vomp locus promotes recombination and deletion of the vomp gene (Zhang et al., 2004). The variable expression of Vomp family members was detected in both macaque animal models and B. quintana-infected humans (Zhang et al., 2004), and the expression of BadA seemly exhibits the characterization of phase variation (Thibau et al., 2022a). Intriguingly, B. bacilliformis can evade Toll-like receptor 5 by possessing unique amino acid sequences in flagellin that are different from the evolutionarily conserved ones required for microbial fitness (Andersen-Nissen et al., 2005; Figure 3). Bartonella CFA, an important target of protective antibodies, has a hypervariable antigenic region in both human- and mouse-hosted Bartonella strains (Siewert et al., 2022b). This hypervariability has the potential to allow for antibody evasion in the same mammalian host infected with different Bartonella strains through multiple sequential or timewise overlapping modes.
Suppression of immune response is also employed by Bartonella to escape the host immune response. Some Bartonella species, such as B. quintana and B. henselae, can promote mononuclear cells and DCs to secrete IL-10 (Papadopoulos et al., 2001; Vermi et al., 2006; Foca et al., 2012; Schmidgen et al., 2014), which suppresses inflammation response and facilitates the continuous progression of asymptomatic pathogen infection (Kabeya et al., 2007; Couper et al., 2008). The vital function of IL-10 in Bartonella’s immune evasion has been demonstrated in mouse models, as the pathogen B. birtlesii is unable to establish bacteremia in IL-10 knockout mice (Marignac et al., 2010). What’s more, recent studies showed that BepD activated the STAT3 pathway and promoted the secretion of anti-inflammatory cytokine IL-10, which may play a role in the resistance of Bartonella to innate immune cells in the dermal niche (Fromm and Dehio, 2021). In addition, B. vinsonii reduces MHC-II expression on the surface of B cells in dogs, suggesting the declined B-cell antigen presentation to helper T cells (Pappalardo et al., 2001). In cats, post-infection with B. henselae leads to a decreased number of CD4+ cells (Kabeya et al., 2009), yet the corresponding mechanism is still unknown.
The intracellular persistence of Bartonella is another unique strategy to avoid the host humoral immune system. B. quintana, for example, colonizes intracellularly without causing hemolysis of erythrocytes, which benefits the spread through body lice and cause repeated infection (Rolain et al., 2002, 2003). Recent studies have also detected B. quintana in human dental pulp stem cells (DPSCs), and the increase of bacterial load within the cellular niche does not affect the proliferation of DPSCs (Oumarou Hama et al., 2021).
The host immune response and Bartonella immune escape competes and restricts each other as shown in Figure 3. A strong immune response can reduce the frequency of host bacteremia, leading to recovery under the combined action of specific and non-specific immunity. However, weak immune response can result in repeated and long-term Bartonella infection, which can even be life-threatening. Therefore, studying the host immune response after Bartonella infection can help understand the causes of bacillary angiomatosis, peliosis hepatis, CSD, and other diseases, and provide new ideas for alleviating the clinical symptoms of patients after Bartonella infection.
Bartonella is primarily transmitted through the feces of blood-sucking arthropods or traumatic contact with infected animals. The invasion of Bartonellae in lineage 3 and lineage 4 is facilitated by the VirB/VirD4 T4SS system and Beps protein. The pathogen’s invasion triggers the humoral and cellular immune responses of the host. Particularly, the immune system produces various antibodies to neutralize pathogens, playing a vital role in the removal of Bartonella from the bloodstream.
However, Bartonella species have developed several mechanisms to evade or resist the host’s immune response, which allow them to cause long-termed and repeated bacteremia. Some Bartonella species rely on special LPS structure or are hidden in host cells, while others stimulate the host to produce cytokines that weaken the immune response. Here we briefly summarized the recent findings on how Bartonella interacts with host ECs. Among the well-studied molecules are the trimeric family of autotransporters, α-enolase, and BafA. Additionally, Bartonella infection of MSCs can increase the susceptibility of ECs to Bartonella. The molecular involvement during erythrocyte invasion has also deepened our understanding of Bartonella infection. Investigating the mechanism of Bartonella infection on ECs and erythrocytes is crucial for comprehending Bartonella disease. Despite significant efforts, there remain numerous uncertain aspects that require further investigation, such as fully elucidating the functions of various types of molecules mentioned above. Future studies on Bartonella may require more appropriate in vitro and in vivo infection models, as well as functional genomics studies. Our efforts would be directed toward developing more effective and economical methods for detecting Bartonella in the population, as well as preventing and treating the diseases caused by Bartonella.
TL and JF contributed to the conception of the review and reviewed and revised the manuscript. XJ, YG, TL, JF, YX, JL, and JS collected and organized the data. XJ and YG wrote the manuscript. All authors contributed to the article and approved the submitted version.
The authors declare that the research was conducted in the absence of any commercial or financial relationships that could be construed as a potential conflict of interest.
All claims expressed in this article are solely those of the authors and do not necessarily represent those of their affiliated organizations, or those of the publisher, the editors and the reviewers. Any product that may be evaluated in this article, or claim that may be made by its manufacturer, is not guaranteed or endorsed by the publisher.
Abbott, R. C., Chomel, B. B., Kasten, R. W., Floyd-Hawkins, K. A., Kikuchi, Y., Koehler, J. E., et al. (1997). Experimental and natural infection with Bartonella henselae in domestic cats. Comp. Immunol. Microbiol. Infect. Dis. 20, 41–51.
Andersen-Nissen, E., Smith, K. D., Strobe, K. L., Barrett, S. L. R., Cookson, B. T., Logan, S. M., et al. (2005). Evasion of Toll-like receptor 5 by flagellated bacteria. Proc. Natl. Acad. Sci. U.S.A. 102, 9247–9252.
Battisti, J. M., Lawyer, P. G., and Minnick, M. F. (2015). Colonization of Lutzomyia verrucarum and Lutzomyia longipalpis sand flies (Diptera: Psychodidae) by Bartonella bacilliformis, the etiologic agent of carrion’s disease. PLoS Negl. Trop. Dis. 9:e0004128. doi: 10.1371/journal.pntd.0004128
Biancardi, A. L., and Curi, A. L. (2014). Cat-scratch disease. Ocul. Immunol. Inflamm. 22, 148–154. doi: 10.3109/09273948.2013.833631
Bown, K. J., Bennet, M., and Begon, M. (2004). Flea-borne Bartonella grahamii and Bartonella taylorii in bank voles. Emerg. Infect. Dis. 10, 684–687.
Breitschwerdt, E. B., Maggi, R. G., Chomel, B. B., and Lappin, M. R. (2010a). Bartonellosis: an emerging infectious disease of zoonotic importance to animals and human beings. J. Vet. Emerg. Crit. Care 20, 8–30. doi: 10.1111/j.1476-4431.2009.00496.x
Breitschwerdt, E. B., Maggi, R. G., Farmer, P., and Mascarelli, P. E. (2010b). Molecular evidence of perinatal transmission of Bartonella vinsonii subsp. berkhoffii and Bartonella henselae to a child. J. Clin. Microbiol. 48, 2289–2293. doi: 10.1128/JCM.00326-10
Brenner, D. J., O’Connor, S. P., Winkler, H. H., and Steigerwalt, A. G. (1993). Proposals to unify the genera Bartonella and Rochalimaea, with descriptions of Bartonella quintana comb. nov., Bartonella vinsonii comb. nov., Bartonella henselae comb. nov., and Bartonella elizabethae comb. nov., and to remove the family Bartonellaceae from the order Rickettsiales. Int. J. Syst. Bacteriol. 43, 777–786. doi: 10.1099/00207713-43-4-777
Byam, W., and Lloyd, L. (1920). Trench fever: its epidemiology and endemiology. Proc. R. Soc. Med. 13, 1–27.
Cappello, P., Principe, M., Bulfamante, S., and Novelli, F. (2017). Alpha-Enolase (ENO1), a potential target in novel immunotherapies. Front. Biosci. 22:944–959.
Cascales, E., and Christie, P. J. (2003). The versatile bacterial type IV secretion systems. Nat. Rev. Microbiol. 1, 137–149. doi: 10.1038/nrmicro753
Chamberlin, J., Laughlin, L. W., Romero, S., Solórzano, N., Gordon, S., Andre, R. G., et al. (2002). Epidemiology of endemic Bartonella bacilliformis: a prospective cohort study in a Peruvian mountain valley community. J. Infect. Dis. 186, 983–990.
Chang, C. C., Lee, C. J., Ou, L. S., Wang, C. J., and Huang, Y. C. (2016). Disseminated cat-scratch disease: case report and review of the literature. Paediatr. Int. Child Health 36, 232–234. doi: 10.1179/2046905515Y.0000000005
Chenoweth, M. R., Greene, C. E., Krause, D. C., and Gherardini, F. C. (2004). Predominant outer membrane antigens of Bartonella henselae. Infect. Immun. 72, 3097–3105. doi: 10.1128/IAI.72.6.3097-3105.2004
Chomel, B. B., Boulouis, H. J., Breitschwerdt, E. B., Kasten, R. W., Vayssier-Taussat, M., Birtles, R. J., et al. (2009). Ecological fitness and strategies of adaptation of Bartonella species to their hosts and vectors. Vet. Res. 40:29. doi: 10.1051/vetres/2009011
Chomel, B. B., Kasten, R. W., Floyd-Hawkins, K., Chi, B., Yamamoto, K., Roberts-Wilson, J., et al. (1996). Experimental transmission of Bartonella henselae by the cat flea. J. Clin. Microbiol. 34, 1952–1956.
Clemente, N. S., Ugarte-Gil, C., Solorzano, N., Maguiña, C., and Moore, D. (2016). An outbreak of Bartonella bacilliformis in an endemic andean community. PLoS One. 11:e0150525. doi: 10.1371/journal.pone.0150525
Coleman, S. A., and Minnick, M. F. (2001). Establishing a direct role for the Bartonella bacilliformis invasion-associated locus B (IalB) protein in human erythrocyte parasitism. Infect. Immun. 69, 4373–4381.
Cotte, V., Bonnet, S., Le Rhun, D., Le Naour, E., Chauvin, A., Boulouis, H. J., et al. (2008). Transmission of Bartonella henselae by Ixodes ricinus. Emerg. Infect. Dis. 14, 1074–1080. doi: 10.3201/eid1407.071110
Couper, K. N., Blount, D. G., and Riley, E. M. (2008). IL-10: the master regulator of immunity to infection. J. Immunol. 180, 5771–5777. doi: 10.4049/jimmunol.180.9.5771
Dehio, C. (2005). Bartonella–host-cell interactions and vascular tumour formation. Nat. Rev. Microbiol. 3, 621–631. doi: 10.1038/nrmicro1209
Dehio, C. (2008). Infection-associated type IV secretion systems of Bartonella and their diverse roles in host cell interaction. Cell. Microbiol. 10, 1591–1598. doi: 10.1111/j.1462-5822.2008.01171.x
Dehio, C., and Tsolis, R. M. (2017). Type IV effector secretion and subversion of host functions by Bartonella and Brucella species. Curr. Top. Microbiol. Immunol. 413, 269–295. doi: 10.1007/978-3-319-75241-9_11
Deng, H., Le Rhun, D., Buffet, J. P., Cotte, V., Read, A., Birtles, R. J., et al. (2012). Strategies of exploitation of mammalian reservoirs by Bartonella species. Vet. Res. 43:15. doi: 10.1186/1297-9716-43-15
Deng, H., Pang, Q., Xia, H., Le Rhun, D., Le Naour, E., Yang, C., et al. (2016). Identification and functional analysis of invasion associated locus B (IalB) in Bartonella species. Microb. Pathog. 98, 171–177. doi: 10.1016/j.micpath.2016.05.007
Deng, H., Wu, S., Song, Q., Zhang, J., Sang, F., Sun, X., et al. (2019). Cloning and identification of Bartonella alpha-enolase as a plasminogen-binding protein. Microb. Pathog. 135:103651. doi: 10.1016/j.micpath.2019.103651
Díaz-Ramos, A., Roig-Borrellas, A., García-Melero, A., and López-Alemany, R. (2012). α-Enolase, a multifunctional protein: its role on pathophysiological situations. J. Biomed. Biotechnol. 2012, 156795. doi: 10.1155/2012/156795
do Amaral, R. B., Cardozo, M. V., Varani, A. M., Furquim, M. E. C., Dias, C. M., Assis, W. O., et al. (2022a). First report of Bartonella spp. in marsupials from Brazil, with a description of Bartonella harrusi sp. nov. and a new proposal for the taxonomic reclassification of species of the Genus Bartonella. Microorganisms 10:1609. doi: 10.3390/microorganisms10081609
do Amaral, R. B., Cardozo, M. V., Varani, A. M., Goncalves, L. R., Furquim, M. E. C., Dias, C. M., et al. (2022b). Bartonella machadoae sp. nov. isolated from wild rodents in the Pantanal wetland. Acta Trop. 229:106368. doi: 10.1016/j.actatropica.2022.106368
Duscher, G. G., Hodzic, A., Potkonjak, A., Leschnik, M. W., and Spergser, J. (2018). Bartonella henselae and rickettsia felis detected in cat fleas (Ctenocephalides felis) derived from Eastern Austrian Cats. Vector Borne Zoonotic Dis 18, 282–284. doi: 10.1089/vbz.2017.2215
Eicher, S. C., and Dehio, C. (2012). Bartonella entry mechanisms into mammalian host cells. Cell. Microbiol. 14, 1166–1173. doi: 10.1111/j.1462-5822.2012.01806.x
Ezekowitz, R. A., and Stahl, P. D. (1988). The structure and function of vertebrate mannose lectin-like proteins. J. Cell Sci. Suppl. 9, 121–133.
Foca, A., Liberto, M. C., Quirino, A., and Matera, G. (2012). Lipopolysaccharides: from Erinyes to Charites. Mediators Inflamm. 2012:684274. doi: 10.1155/2012/684274
Foil, L., Andress, E., Freeland, R. L., Roy, A. F., Rutledge, R., Triche, P. C., et al. (1998). Experimental infection of domestic cats with Bartonella henselae by inoculation of Ctenocephalides felis (Siphonaptera: Pulicidae) feces. J. Med. Entomol. 35, 625–628. doi: 10.1093/jmedent/35.5.625
Frank, A. C., Alsmark, C. M., Thollesson, M., and Andersson, S. G. (2005). Functional divergence and horizontal transfer of type IV secretion systems. Mol. Biol. Evol. 22, 1325–1336. doi: 10.1093/molbev/msi124
Fromm, K., and Dehio, C. (2021). The impact of Bartonella VirB/VirD4 Type IV secretion system effectors on eukaryotic host cells. Front. Microbiol. 12:762582. doi: 10.3389/fmicb.2021.762582
Gai, M., d’Onofrio, G., di Vico, M. C., Ranghino, A., Nappo, A., Diena, D., et al. (2015). Cat-scratch disease: case report and review of the literature. Transplant Proc. 47, 2245–2247. doi: 10.1016/j.transproceed.2015.07.014
Garcia-Quintanilla, M., Dichter, A. A., Guerra, H., and Kempf, V. A. J. (2019). Carrion’s disease: more than a neglected disease. Parasit Vectors 12, 141. doi: 10.1186/s13071-019-3390-2
Gilmore, R. D. Jr., Bellville, T. M., Sviat, S. L., and Frace, M. (2005). The Bartonella vinsonii subsp. arupensis immunodominant surface antigen BrpA gene, encoding a 382-kilodalton protein composed of repetitive sequences, is a member of a multigene family conserved among Bartonella species. Infect. Immun. 73, 3128–3136. doi: 10.1128/IAI.73.5.3128-3136.2005
Gomes, C., Martinez-Puchol, S., Ruiz-Roldan, L., Pons, M. J., Del Valle Mendoza, J., and Ruiz, J. (2016a). Development and characterisation of highly antibiotic resistant Bartonella bacilliformis mutants. Sci. Rep. 6:33584. doi: 10.1038/srep33584
Gomes, C., Palma, N., Pons, M. J., Magallon-Tejada, A., Sandoval, I., Tinco-Valdez, C., et al. (2016b). Succinyl-CoA synthetase: new antigen candidate of Bartonella bacilliformis. PLoS Negl. Trop. Dis. 10:e0004989. doi: 10.1371/journal.pntd.0004989
Goncalves-Oliveira, J., Gutierrez, R., Schlesener, C. L., Jaffe, D. A., Aguilar-Setien, A., Boulouis, H. J., et al. (2023). Genomic characterization of three novel Bartonella strains in a rodent and two bat species from Mexico. Microorganisms 11:340. doi: 10.3390/microorganisms11020340
Gundi, V. A., Davoust, B., Khamis, A., Boni, M., Raoult, D., and La Scola, B. (2004). Isolation of Bartonella rattimassiliensis sp. nov. and Bartonella phoceensis sp. nov. from European Rattus norvegicus. J. Clin. Microbiol. 42, 3816–3818. doi: 10.1128/JCM.42.8.3816-3818.2004
Gutierrez, R., Shalit, T., Markus, B., Yuan, C., Nachum-Biala, Y., Elad, D., et al. (2020). Bartonella kosoyi sp. nov. and Bartonella krasnovii sp. nov., two novel species closely related to the zoonotic Bartonella elizabethae, isolated from black rats and wild desert rodent-fleas. Int. J. Syst. Evol. Microbiol. 70, 1656–1665. doi: 10.1099/ijsem.0.003952
Harms, A., and Dehio, C. (2012). Intruders below the radar: molecular pathogenesis of Bartonella spp. Clin. Microbiol. Rev. 25, 42–78. doi: 10.1128/CMR.05009-11
Harms, A., Segers, F. H., Quebatte, M., Mistl, C., Manfredi, P., Korner, J., et al. (2017). Evolutionary dynamics of pathoadaptation revealed by three independent acquisitions of the VirB/D4 type IV secretion system in Bartonella. Genome Biol. Evol. 9, 761–776. doi: 10.1093/gbe/evx042
Hendrix, L. R., and Kiss, K. (2003). Studies on the identification of deforming factor from Bartonella bacilliformis. Ann. N.Y. Acad. Sci. 990, 596–604. doi: 10.1111/j.1749-6632.2003.tb07433.x
Higgins, J. A., Radulovic, S., Jaworski, D. C., and Azad, A. F. (1996). Acquisition of the cat scratch disease agent Bartonella henselae by cat fleas (Siphonaptera:Pulicidae). J. Med. Entomol. 33, 490–495. doi: 10.1093/jmedent/33.3.490
Hong, J., Li, Y., Hua, X., Bai, Y., Wang, C., Zhu, C., et al. (2017). Lymphatic circulation disseminates Bartonella infection into bloodstream. J. Infect. Dis. 215, 303–311. doi: 10.1093/infdis/jiw526
Iwaki-Egawa, S., and Ihler, G. M. (1997). Comparison of the abilities of proteins from Bartonella bacilliformis and Bartonella henselae to deform red cell membranes and to bind to red cell ghost proteins. FEMS Microbiol. Lett. 157, 207–217. doi: 10.1111/j.1574-6968.1997.tb12775.x
Kabeya, H., Umehara, T., Okanishi, H., Tasaki, I., Kamiya, M., Misawa, A., et al. (2009). Experimental infection of cats with Bartonella henselae resulted in rapid clearance associated with T helper 1 immune responses. Microbes Infect. 11, 716–720. doi: 10.1016/j.micinf.2009.03.008
Kabeya, H., Yamasaki, A., Ikariya, M., Negishi, R., Chomel, B. B., and Maruyama, S. (2007). Characterization of Th1 activation by Bartonella henselae stimulation in BALB/c mice: inhibitory activities of interleukin-10 for the production of interferon-gamma in spleen cells. Vet. Microbiol. 119, 290–296. doi: 10.1016/j.vetmic.2006.08.010
Kaiser, P. O., Riess, T., Wagner, C. L., Linke, D., Lupas, A. N., Schwarz, H., et al. (2008). The head of Bartonella adhesin A is crucial for host cell interaction of Bartonella henselae. Cell. Microbiol. 10, 2223–2234. doi: 10.1111/j.1462-5822.2008.01201.x
Kempf, V. A., Lebiedziejewski, M., Alitalo, K. Wälzlein, J. H., Ehehalt, U., Fiebig, J., et al. (2005). Activation of hypoxia-inducible factor-1 in bacillary angiomatosis: evidence for a role of hypoxia-inducible factor-1 in bacterial infections. Circulation 111, 1054–1062. doi: 10.1161/01.CIR.0000155608.07691.B
Kempf, V. A., Volkmann, B., Schaller, M., Sander, C. A., Alitalo, K., Riess, T., et al. (2001). Evidence of a leading role for VEGF in Bartonella henselae-induced endothelial cell proliferations. Cell. Microbiol. 3, 623–632. doi: 10.1046/j.1462-5822.2001.00144.x
Keragala, C. B., and Medcalf, R. L. (2021). Plasminogen: an enigmatic zymogen. Blood 137, 2881–2889. doi: 10.1182/blood.2020008951
Koesling, J., Aebischer, T., Falch, C., Schulein, R., and Dehio, C. (2001). Cutting edge: antibody-mediated cessation of hemotropic infection by the intraerythrocytic mouse pathogen Bartonella grahamii. J. Immunol. 167, 11–14. doi: 10.4049/jimmunol.167.1.11
Kosoy, M. Y., Regnery, R. L., Kosaya, O. I., Jones, D. C., Marston, E. L., and Childs, J. E. (1998). Isolation of Bartonella spp. from embryos and neonates of naturally infected rodents. J. Wildl. Dis. 34, 305–309.
Kostrzewski, J. (1950). [Epidemiology of trench fever]. Medycyna Doswiadczalna I Mikrobiologia 2, 19–51.
Krol, N., Militzer, N., Stobe, E., Nijhof, A. M., Pfeffer, M., Kempf, V. A. J., et al. (2021). Evaluating transmission paths for three different Bartonella spp. in ixodes ricinus ticks using artificial feeding. Microorganisms 9:901. doi: 10.3390/microorganisms9050901
Kyme, P. A., Haas, A., Schaller, M., Peschel, A., Iredell, J., and Kempf, V. A. (2005). Unusual trafficking pattern of Bartonella henselae -containing vacuoles in macrophages and endothelial cells. Cell. Microbiol. 7, 1019–1034. doi: 10.1111/j.1462-5822.2005.00531.x
Lähteenmäki, K., Kuusela, P., and Korhonen, T. K. (2001). Bacterial plasminogen activators and receptors. FEMS Microbiol. Rev. 25, 531–552. doi: 10.1111/j.1574-6976.2001.tb00590.x
Leibler, J. H., Zakhour, C. M., Gadhoke, P., and Gaeta, J. M. (2016). Zoonotic and vector-borne infections among urban homeless and marginalized people in the United States and Europe, 1990-2014. Vector Borne Zoonotic Dis. 16, 435–444. doi: 10.1089/vbz.2015.1863
Linke, D., Riess, T., Autenrieth, I. B., Lupas, A., and Kempf, V. A. (2006). Trimeric autotransporter adhesins: variable structure, common function. Trends Microbiol. 14, 264–270. doi: 10.1016/j.tim.2006.04.005
Litwin, C. M., and Johnson, J. M. (2005). Identification, cloning, and expression of the CAMP-like factor autotransporter gene (cfa) of Bartonella henselae. Infect. Immun. 73, 4205–4213. doi: 10.1128/IAI.73.7.4205-4213.2005
Liu, M., and Biville, F. (2013). Managing iron supply during the infection cycle of a flea borne pathogen, Bartonella henselae. Front. Cell. Infect. Microbiol. 3:60. doi: 10.3389/fcimb.2013.00060
Liu, Q., Eremeeva, M. E., and Li, D. (2012). Bartonella and Bartonella infections in China: from the clinic to the laboratory. Comp. Immunol. Microbiol. Infect. Dis. 35, 93–102. doi: 10.1016/j.cimid.2012.01.002
Liu, Y., Chen, J., Lang, H., and Zheng, H. (2022). Bartonella choladocola sp. nov. and Bartonella apihabitans sp. nov., two novel species isolated from honey bee gut. Syst. Appl. Microbiol. 45:126372. doi: 10.1016/j.syapm.2022.126372
Lu, Y. Y., Franz, B., Truttmann, M. C., Riess, T., Gay-Fraret, J., Faustmann, M., et al. (2013). Bartonella henselae trimeric autotransporter adhesin BadA expression interferes with effector translocation by the VirB/D4 type IV secretion system. Cell Microbiol. 15, 759–778. doi: 10.1111/cmi.12070
Maguina, C., Guerra, H., and Ventosilla, P. (2009). Bartonellosis. Clin. Dermatol. 27, 271–280. doi: 10.1016/j.clindermatol.2008.10.006
Malgorzata-Miller, G., Heinbockel, L., Brandenburg, K., van der Meer, J. W., Netea, M. G., and Joosten, L. A. (2016). Bartonella quintana lipopolysaccharide (LPS): structure and characteristics of a potent TLR4 antagonist for in-vitro and in-vivo applications. Sci. Rep. 6:34221. doi: 10.1038/srep34221
Mändle, T., Einsele, H., Schaller, M., Neumann, D., Vogel, W., Autenrieth, I. B., et al. (2005). Infection of human CD34+ progenitor cells with Bartonella henselae results in intraerythrocytic presence of B. henselae. Blood 106, 1215–1222. doi: 10.1182/blood-2004-12-4670
Marignac, G., Barrat, F., Chomel, B., Vayssier-Taussat, M., Gandoin, C., Bouillin, C., et al. (2010). Murine model for Bartonella birtlesii infection: new aspects. Comp. Immunol. Microbiol. Infect. Dis. 33, 95–107. doi: 10.1016/j.cimid.2008.07.011
Marlaire, S., and Dehio, C. (2021). Bartonella effector protein C mediates actin stress fiber formation via recruitment of GEF-H1 to the plasma membrane. PLoS Pathog. 17:e1008548. doi: 10.1371/journal.ppat.1008548
Matera, G., Liberto, M. C., Joosten, L. A., Vinci, M., Quirino, A., Pulicari, M. C., et al. (2008). The Janus face of Bartonella quintana recognition by Toll-like receptors (TLRs): a review. Eur. Cytokine Netw. 19, 113–118. doi: 10.1684/ecn.2008.0128
McCord, A. M., Resto-Ruiz, S. I., and Anderson, B. E. (2006). Autocrine role for interleukin-8 in Bartonella henselae-induced angiogenesis. Infect. Immun. 74, 5185–5190. doi: 10.1128/IAI.00622-06
McGill, S. L., Regnery, R. L., and Karem, K. L. (1998). Characterization of human immunoglobulin (Ig) isotype and IgG subclass response to Bartonella henselae infection. Infect. Immun. 66, 5915–5920. doi: 10.1128/IAI.66.12.5915-5920.1998
Mernaugh, G., and Ihler, G. M. (1992). Deformation factor: an extracellular protein synthesized by Bartonella bacilliformis that deforms erythrocyte membranes. Infect. Immun. 60, 937–943. doi: 10.1128/iai.60.3.937-943.1992
Minnick, M. F., Anderson, B. E., Lima, A., Battisti, J. M., Lawyer, P. G., and Birtles, R. J. (2014). Oroya fever and verruga peruana: bartonelloses unique to South America. PLoS Negl. Trop. Dis. 8:e2919. doi: 10.1371/journal.pntd.0002919
Mitchell, S. J., and Minnick, M. F. (1995). Characterization of a two-gene locus from Bartonella bacilliformis associated with the ability to invade human erythrocytes. Infect. Immun. 63, 1552–1562. doi: 10.1128/iai.63.4.1552-1562.1995
Mosepele, M., Mazo, D., and Cohn, J. (2012). Bartonella infection in immunocompromised hosts: immunology of vascular infection and vasoproliferation. Clin. Dev. Immunol. 2012:612809. doi: 10.1155/2012/612809
Muller, N. F., Kaiser, P. O., Linke, D., Schwarz, H., Riess, T., Schafer, A., et al. (2011). Trimeric autotransporter adhesin-dependent adherence of Bartonella henselae, Bartonella quintana, and Yersinia enterocolitica to matrix components and endothelial cells under static and dynamic flow conditions. Infect. Immun. 79, 2544–2553. doi: 10.1128/IAI.01309-10
Musso, T., Badolato, R., Ravarino, D., Stornello, S., Panzanelli, P., Merlino, C., et al. (2001). Interaction of Bartonella henselae with the murine macrophage cell line J774: infection and proinflammatory response. Infect. Immun. 69, 5974–5980. doi: 10.1128/IAI.69.10.5974-5980.2001
Netea, M. G., Sutmuller, R., Hermann, C., Van der Graaf, C. A., Van der Meer, J. W., van Krieken, J. H., et al. (2004). Toll-like receptor 2 suppresses immunity against Candida albicans through induction of IL-10 and regulatory T cells. J. Immunol. 172, 3712–3718. doi: 10.4049/jimmunol.172.6.3712
O’Rourke, F., Mändle, T., Urbich, C., Dimmeler, S., Michaelis, U. R., Brandes, R. P., et al. (2015). Reprogramming of myeloid angiogenic cells by Bartonella henselae leads to microenvironmental regulation of pathological angiogenesis. Cell Microbiol. 17, 1447–1463. doi: 10.1111/cmi.12447
Okaro, U., Addisu, A., Casanas, B., and Anderson, B. (2017). Bartonella species, an emerging cause of blood-culture-negative endocarditis. Clin. Microbiol. Rev. 30, 709–746. doi: 10.1128/CMR.00013-17
Okaro, U., George, S., and Anderson, B. (2021). What is in a cat scratch? Growth of Bartonella henselae in a biofilm. Microorganisms 9:835. doi: 10.3390/microorganisms9040835
Okujava, R., Guye, P., Lu, Y. Y., Mistl, C., Polus, F., Vayssier-Taussat, M., et al. (2014). A translocated effector required for Bartonella dissemination from derma to blood safeguards migratory host cells from damage by co-translocated effectors. PLoS Pathog. 10:e1004187. doi: 10.1371/journal.ppat.1004187
Oskouizadeh, K., Zahraei-Salehi, T., and Aledavood, S. (2010). Detection of Bartonella henselae in domestic cats’ saliva. Iran J. Microbiol. 2, 80–84.
Oumarou Hama, H., Hamada, A., Aboudharam, G., Ghigo, E., and Drancourt, M. (2021). Human dental pulp stem cells: a sanctuary for relapsing Bartonella quintana. Microb. Pathog. 153:104797. doi: 10.1016/j.micpath.2021.104797
Papadopoulos, N. G., Gourgiotis, D., Bossios, A., Fretzayas, A., Moustaki, M., and Karpathios, T. (2001). Circulating cytokines in patients with cat scratch disease. Clin. Infect. Dis. 33, e54–56.
Pappalardo, B. L., Brown, T. T., Tompkins, M., and Breitschwerdt, E. B. (2001). Immunopathology of Bartonella vinsonii (berkhoffii) in experimentally infected dogs. Vet. Immunol. Immunopathol. 83, 125–147.
Patten, J., and Wang, K. (2021). Fibronectin in development and wound healing. Adv. Drug Deliv. Rev. 170, 353–368. doi: 10.1016/j.addr.2020.09.005
Pitassi, L. H., de Paiva Diniz, P. P., Scorpio, D. G., Drummond, M. R., Lania, B. G., Barjas-Castro, M. L., et al. (2015). Bartonella spp. bacteremia in blood donors from Campinas. Brazil. PLoS Negl. Trop. Dis. 9:e0003467. doi: 10.1371/journal.pntd.0003467
Pons, M. J., Gomes, C., Aguilar, R., Barrios, D., Aguilar-Luis, M. A., Ruiz, J., et al. (2017). Immunosuppressive and angiogenic cytokine profile associated with Bartonella bacilliformis infection in post-outbreak and endemic areas of Carrion’s disease in Peru. PLoS Negl. Trop. Dis. 11:e0005684. doi: 10.1371/journal.pntd.0005684
Popa, C., Abdollahi-Roodsaz, S., Joosten, L. A., Takahashi, N., Sprong, T., Matera, G., et al. (2007). Bartonella quintana lipopolysaccharide is a natural antagonist of Toll-like receptor 4. Infect. Immun. 75, 4831–4837. doi: 10.1128/IAI.00237-07
Pulliainen, A. T., and Dehio, C. (2012). Persistence of Bartonella spp. stealth pathogens: from subclinical infections to vasoproliferative tumor formation. FEMS Microbiol. Rev. 36, 563–599. doi: 10.1111/j.1574-6976.2012.00324.x
Pulliainen, A. T., Pieles, K., Brand, C. S., Hauert, B., Bohm, A., Quebatte, M., et al. (2012). Bacterial effector binds host cell adenylyl cyclase to potentiate Galphas-dependent cAMP production. Proc. Natl. Acad. Sci. U.S.A. 109, 9581–9586. doi: 10.1073/pnas.1117651109
Resto-Ruiz, S. I., Schmiederer, M., Sweger, D., Newton, C., Klein, T. W., Friedman, H., et al. (2002). Induction of a potential paracrine angiogenic loop between human THP-1 macrophages and human microvascular endothelial cells during Bartonella henselae infection. Infect. Immun. 70, 4564–4570. doi: 10.1128/IAI.70.8.4564-4570.2002
Ribeiro, J. M. (1995). Blood-feeding arthropods: live syringes or invertebrate pharmacologists? Infect. Agents Dis. 4, 143–152.
Riess, T., Andersson, S. G., Lupas, A., Schaller, M., Schäfer, A., Kyme, P., et al. (2004). Bartonella adhesin A mediates a proangiogenic host cell response. J. Exp. Med. 200, 1267–1278. doi: 10.1084/jem.20040500
Rodriguez-Barradas, M. C., Bandres, J. C., Hamill, R. J., Trial, J., Clarridge, J. E., Baughn, R. E., et al. (1995). In vitro evaluation of the role of humoral immunity against Bartonella henselae. Infect. Immun. 63, 2367–2370.
Rolain, J. M., Brouqui, P., Koehler, J. E., Maguina, C., Dolan, M. J., and Raoult, D. (2004). Recommendations for treatment of human infections caused by Bartonella species. Antimicrob. Agents Chemother. 48, 1921–1933. doi: 10.1128/aac.48.6.1921-1933.2004
Rolain, J. M., Foucault, C., Guieu, R., La Scola, B., Brouqui, P., and Raoult, D. (2002). Bartonella quintana in human erythrocytes. Lancet 360, 226–228. doi: 10.1016/s0140-6736(02)09462-x
Rolain, J. M., Maurin, M., Mallet, M. N., Parzy, D., and Raoult, D. (2003). Culture and antibiotic susceptibility of Bartonella quintana in human erythrocytes. Antimicrob. Agents Chemother. 47, 614–619. doi: 10.1128/AAC.47.2.614-619.2003
Sanchez Clemente, N., Ugarte-Gil, C. A., Solorzano, N., Maguina, C., Pachas, P., Blazes, D., et al. (2012). Bartonella bacilliformis: a systematic review of the literature to guide the research agenda for elimination. PLoS Negl. Trop. Dis. 6:e1819. doi: 10.1371/journal.pntd.0001819
Sasaki, T., Adachi, T., Itoh, K., Matsuoka, M., Yamagishi, T., Hirao, M., et al. (2021). Detection of Bartonella quintana Infection among the homeless population in Tokyo, Japan, from 2013-2015. Jpn J. Infect. Dis. 74, 411–415. doi: 10.7883/yoken.JJID.2020.505
Schmidgen, T., Kaiser, P. O., Ballhorn, W., Franz, B., Gottig, S., Linke, D., et al. (2014). Heterologous expression of Bartonella adhesin A in Escherichia coli by exchange of trimeric autotransporter adhesin domains results in enhanced adhesion properties and a pathogenic phenotype. J. Bacteriol. 196, 2155–2165. doi: 10.1128/JB.01461-13
Scutera, S., Mitola, S., Sparti, R., Salvi, V., Grillo, E., Piersigilli, G., et al. (2021). Bartonella henselae persistence within mesenchymal stromal cells enhances endothelial cell activation and infectibility that amplifies the angiogenic process. Infect. Immun. 89:e0014121. doi: 10.1128/IAI.00141-21
Segers, F. H. I. D., Kešnerová, L., Kosoy, M., and Engel, P. (2017). Genomic changes associated with the evolutionary transition of an insect gut symbiont into a blood-borne pathogen. ISME J. 11, 1232–1244. doi: 10.1038/ismej.2016.201
Serafim, T. D., Coutinho-Abreu, I. V., Dey, R., Kissinger, R., Valenzuela, J. G., Oliveira, F., et al. (2021). Leishmaniasis: the act of transmission. Trends Parasitol. 37, 976–987. doi: 10.1016/j.pt.2021.07.003
Siamer, S., and Dehio, C. (2015). New insights into the role of Bartonella effector proteins in pathogenesis. Curr. Opin. Microbiol. 23, 80–85. doi: 10.1016/j.mib.2014.11.007
Siewert, L. K., Dehio, C., and Pinschewer, D. D. (2022a). Adaptive immune defense prevents Bartonella persistence upon trans-placental transmission. PLoS Pathog. 18:e1010489. doi: 10.1371/journal.ppat.1010489
Siewert, L. K., Korotaev, A., Sedzicki, J., Fromm, K., Pinschewer, D. D., and Dehio, C. (2022b). Identification of the Bartonella autotransporter CFA as a protective antigen and hypervariable target of neutralizing antibodies in mice. Proc. Natl. Acad. Sci. U.S.A. 119:e2202059119. doi: 10.1073/pnas.2202059119
Sorg, I., Schmutz, C., Lu, Y. Y., Fromm, K., Siewert, L. K., Bogli, A., et al. (2020). A Bartonella effector acts as signaling hub for intrinsic STAT3 activation to trigger anti-inflammatory responses. Cell Host Microbe 27, 476–485.e7. doi: 10.1016/j.chom.2020.01.015
Suzuki, N., Kumadaki, K., Tatematsu, K., Doi, Y., and Tsukamoto, K. (2023). The autotransporter BafA contributes to the proangiogenic potential of Bartonella elizabethae. Microbiol. Immunol. 67, 248–257. doi: 10.1111/1348-0421.13057
Tame, J. R. (2011). Autotransporter protein secretion. Biomol. Concepts 2, 525–536. doi: 10.1515/bmc.2011.045
Thibau, A., Hipp, K., Vaca, D. J., Chowdhury, S., Malmstrom, J., Saragliadis, A., et al. (2022a). Long-read sequencing reveals genetic adaptation of Bartonella Adhesin A among different Bartonella henselae isolates. Front. Microbiol. 13:838267. doi: 10.3389/fmicb.2022.838267
Thibau, A., Vaca, D. J., Bagowski, M., Hipp, K., Bender, D., Ballhorn, W., et al. (2022b). Adhesion of Bartonella henselae to fibronectin is mediated via repetitive motifs present in the stalk of Bartonella Adhesin A. Microbiol. Spectr. 10:e0211722. doi: 10.1128/spectrum.02117-22
Truttmann, M. C., Rhomberg, T. A., and Dehio, C. (2011). Combined action of the type IV secretion effector proteins BepC and BepF promotes invasome formation of Bartonella henselae on endothelial and epithelial cells. Cell. Microbiol. 13, 284–299. doi: 10.1111/j.1462-5822.2010.01535.x
Tsukamoto, K., Kumadaki, K., Tatematsu, K., Suzuki, N., and Doi, Y. (2022). The passenger domain of Bartonella bacilliformis BafA promotes endothelial cell angiogenesis via the VEGF receptor signaling pathway. mSphere 7:e0008122. doi: 10.1128/msphere.00081-22
Tsukamoto, K., Shinzawa, N., Kawai, A., Suzuki, M., Kidoya, H., Takakura, N., et al. (2020). The Bartonella autotransporter BafA activates the host VEGF pathway to drive angiogenesis. Nat. Commun. 11:3571. doi: 10.1038/s41467-020-17391-2
Vaca, D. J., Thibau, A., Leisegang, M. S., Malmstrom, J., Linke, D., Eble, J. A., et al. (2022). Interaction of Bartonella henselae with fibronectin represents the molecular basis for adhesion to host cells. Microbiol. Spectr. 10:e0059822. doi: 10.1128/spectrum.00598-22
Vermi, W., Facchetti, F., Riboldi, E., Heine, H., Scutera, S., Stornello, S., et al. (2006). Role of dendritic cell-derived CXCL13 in the pathogenesis of Bartonella henselae B-rich granuloma. Blood 107, 454–462. doi: 10.1182/blood-2005-04-1342
Wagner, A., and Dehio, C. (2019). Role of distinct type-IV-secretion systems and secreted effector sets in host adaptation by pathogenic Bartonella species. Cell. Microbiol. 21:e13004. doi: 10.1111/cmi.13004
Windsor, J. J. (2001). Cat-scratch disease: epidemiology, aetiology and treatment. Br. J. Biomed. Sci. 58, 101–110.
Xu, Y. H., Lu, Z. Y., and Ihler, G. M. (1995). Purification of deformin, an extracellular protein synthesized by Bartonella bacilliformis which causes deformation of erythrocyte membranes. Biochim. Biophys. Acta 1234, 173–183. doi: 10.1016/0005-2736(94)00271-p
Zahringer, U., Lindner, B., Knirel, Y. A., van den Akker, W. M., Hiestand, R., Heine, H., et al. (2004). Structure and biological activity of the short-chain lipopolysaccharide from Bartonella henselae ATCC 49882T. J. Biol. Chem. 279, 21046–21054. doi: 10.1074/jbc.M313370200
Keywords: Bartonella, blood-sucking arthropods, endothelial cells, erythrocytes, antibody, immune escape
Citation: Jin X, Gou Y, Xin Y, Li J, Sun J, Li T and Feng J (2023) Advancements in understanding the molecular and immune mechanisms of Bartonella pathogenicity. Front. Microbiol. 14:1196700. doi: 10.3389/fmicb.2023.1196700
Received: 30 March 2023; Accepted: 23 May 2023;
Published: 09 June 2023.
Edited by:
Daniel Pletzer, University of Otago, New ZealandReviewed by:
Burt Anderson, University of South Florida, United StatesCopyright © 2023 Jin, Gou, Xin, Li, Sun, Li and Feng. This is an open-access article distributed under the terms of the Creative Commons Attribution License (CC BY). The use, distribution or reproduction in other forums is permitted, provided the original author(s) and the copyright owner(s) are credited and that the original publication in this journal is cited, in accordance with accepted academic practice. No use, distribution or reproduction is permitted which does not comply with these terms.
*Correspondence: Tingting Li, bGl0dEBsenUuZWR1LmNu; Jie Feng, amZlbmdAbHp1LmVkdS5jbg==
†These authors have contributed equally to this work and share first authorship
Disclaimer: All claims expressed in this article are solely those of the authors and do not necessarily represent those of their affiliated organizations, or those of the publisher, the editors and the reviewers. Any product that may be evaluated in this article or claim that may be made by its manufacturer is not guaranteed or endorsed by the publisher.
Research integrity at Frontiers
Learn more about the work of our research integrity team to safeguard the quality of each article we publish.