- 1National Center for Emerging and Zoonotic Infectious DiseasesCenters for Disease Control and Prevention, Atlanta, GA, United States
- 2IHRC, Inc., Atlanta, GA, United States
- 3Division of Infectious Diseases and International Health, Department of Medicine, University of Virginia, Charlottesville, VA, United States
- 4Science and Technology Directorate, U.S. Department of Homeland Security, Washington, DC, United States
- 5Office of the Commissioner, U.S. Food and Drug Administration, Silver Spring, MD, United States
- 6Department of Pathology and Laboratory Medicine, Aga Khan University, Karachi, Pakistan
Objectives: The study aim was to investigate multidrug-resistant (MDR) plasmids from a collection of 10 carbapenemase-producing Klebsiella pneumoniae clinical isolates identified within the same healthcare institution in Pakistan. Full characterization of the MDR plasmids including structure, typing characteristics, and AMR content as well as determination of their plasmid-based antimicrobial susceptibility profiles were carried out.
Methods: Plasmids were isolated from 10 clinical isolates of Klebsiella pneumoniae, and from a corresponding set of Escherichia coli transconjugants, then sequenced using Nanopore/Illumina technology to generate plasmid hybrid assemblies. Full characterization of MDR plasmids, including determination of next generation sequencing (NGS)-based AMR profiles, plasmid incompatibility groups, and types, was carried out. The structure of MDR plasmids was analyzed using the Galileo AMR platform. For E. coli transconjugants, the NGS-based AMR profiles were compared to NGS-predicted AMR phenotypes and conventional broth microdilution (BMD) antimicrobial susceptibility testing (AST) results.
Results: All carbapenemase-producing K. pneumoniae isolates (carrying either blaNDM-1, or/and blaOXA-48) carried multiple AMR plasmids encoding 34 antimicrobial resistance genes (ARGs) conferring resistance to antimicrobials from 6 different classes. The plasmid incompatibility groups and types identified were: IncC (types 1 and 3), IncFIA (type 26) IncFIB, IncFII (types K1, K2, K7, and K9), IncHI1B, and IncL. None of the blaNDM-1 and blaESBL-plasmids identified in this study were previously described. Most blaNDM-1-plasmids shared identical AMR regions suggesting potential genetic material/plasmid exchange between K. pneumoniae isolates of this collection. The majority of NGS-based AMR profiles from the E. coli transconjugants correlated well with both NGS-based predicted and conventional AST results.
Conclusion: This study highlights the complexity and diversity of the plasmid-based genetic background of carbapenemase-producing clinical isolates from Pakistan. This study emphasizes the need for characterization of MDR plasmids to determine their complete molecular background and monitor AMR through plasmid transmission between multi-resistant bacterial pathogens.
Introduction
Bacterial infections caused by multidrug resistant (MDR) pathogens represent a major public health threat that jeopardizes antimicrobial therapy and its fundamental role in modern medicine (World Health Organization, 2014; CDC Report, 2019). The transfer of antimicrobial resistance (AMR) genes between bacteria of the same or different species often occurs via mobile genetic elements (MGE), such as plasmids, during horizontal gene transfer (HGT). Strategies to combat AMR include rapidly identifying MDR pathogens, elucidating disease transmission pathways for community-acquired and nosocomial infections and implementing effective prevention and infection control measures.
The continued emergence and global spread of ESBLs and carbapenemases among MDR pathogens responsible for life-threatening infections are alarming (Castanheira et al., 2019). ESBL- and carbapenemase-producing bacteria often carry additional AMR determinants that confer resistance to other classes of antimicrobials (Mączyńska et al., 2023). The 2018 isolation of a Klebsiella (K.) pneumoniae strain in the United States that was resistant to all currently available antibiotics, highlights the challenges of treating infections caused by “pan-resistant pathogens” (de Man et al., 2018).
High fidelity NGS data are critical for accurate plasmid characterization and the identification of ARG variants necessary to predict AMR profiles with high concordance to phenotypic AST results in bacteria (Neuert et al., 2018; Yee and Simner, 2022). The portable MinION (Oxford Nanopore Technologies; ONT) long-read sequencer addresses the limitations observed with many NGS technologies for plasmid sequencing (e.g.; abundance of repetitive elements), and offers a potential solution in real-time and at a much lower cost (e.g., ~$2 K for a start-up kit) for reconstructing MGEs (e.g.; plasmids) and facilitating the identification of ARGs while also helping to track plasmid dissemination and the spread of AMR (Brown et al., 2023).
Since most ARGs are located on plasmids and transmitted to other microorganisms through HGT, long-read plasmid sequencing is essential for characterizing mechanisms of resistance, and understanding the basis of plasmid dissemination, and for tracking MDR pathogens during epidemiological surveillance studies in hospital and community settings (Hendriksen et al., 2019; Hadjadj et al., 2022; Valcek et al., 2022; Yang et al., 2022). Plasmid sequence data from the original strain is used as starting material, and the plasmid of interest can then be transferred to a well-characterized bacterial strain [e.g., Escherichia (E.) coli J53] for further comprehensive analysis. Although this approach requires additional laboratory work, there are potential benefits. Following transformation of a host strain, the plasmid-mediated contributions to AMR transmission are clarified since the chromosome of the original strain is absent. Also, transferring a single plasmid allows the study of the direct phenotypic effects from the ARGs located on that specific plasmid.
Since the first identification of NDM-positive clinical Enterobacteriaceae isolates in a patient from Sweden who visited India in 2009, NDM has quickly spread worldwide due to its location on MGEs, and continuous surveillance and characterization of MGEs in regions around India where NDM originated such as Pakistan is essential for implementing control measures in hospitals and community.
The collection of 10 carbapenemase-producing (i.e., NDM- and/or OXA-48) MDR K. pneumoniae clinical isolates from Pakistan described in our previously published study (Lomonaco et al., 2018) was randomly selected with at least resistance to one carbapenem. Multiple STs were identified: ST11 (n = 3), ST14 (n = 3), ST15 (n = 1), ST101 (n = 2), and ST307 (n = 1), and identical AMR content was observed for some isolates with the same ST. The aim of the current research was to further investigate and fully characterize MDR plasmids to identify potential transmission of the same plasmids or plasmids rearrangements, between K. pneumoniae clinical isolates in this same hospital in Pakistan. Unlike our first study using Illumina sequencing which is not suitable for plasmids, ONT (long-read sequencing) was combined with Illumina (short-read sequencing) to generate long hybrid assemblies and successfully resolve MDR plasmids. We characterized the blaNDM-1 and blaOXA-48-plasmids and identified the genetic origin of AMR for 10 MDR K. pneumoniae isolates using free publicly available software tools. Using these hybrid assemblies, we: (1) determined plasmid incompatibility groups and types, (2) determined NGS-based resistance profiles and compared with NGS- and AST-phenotypes from E. coli transconjugants (3) fully characterized the genetic structure of blaNDM-1 and blaOXA-48-plasmids. This study aimed to determine genetic relatedness and transferability of AMR plasmids within this collection of clinical isolates to help monitor AMR transmission in hospitals, which constitute an important healthcare problem.
Materials and methods
Bacterial isolates
Ten MDR K. pneumoniae isolates, isolated from patients in 2013 and identified as carbapenemase-positive harboring either blaNDM-1 (n = 5), blaOXA-48 (n = 3), or both (n = 2), were obtained from the Clinical Microbiology Laboratory at the Department of Pathology and Laboratory Medicine, Aga Khan University Hospital, Karachi, Pakistan. The 10 K. pneumoniae isolates were recently described in our previous work: BL849 (CFSAN044563), BU19801 (CFSAN044564), MS84 (CFSAN044565), BL12125 (CFSAN044566), BL12456 (CFSAN044568), BA3783 (CFSAN44569), BL13802 (CFSAN044570), BA2664 (CFSAN044571), BL8800 (CFSAN044572), and BA2880 (CFSAN044573). BL12125 (CFSAN044566), BL12456 (CFSAN044568) including two pairs of isolates closely related to each other and sharing the same AMR profile: BL12125 (CFSAN044566), and BL12456 (CFSAN044568) belonging to ST14, and BL8800 (CFSAN044572), and BA2880 (CFSAN044573) belonging to ST101, and isolated from 2 specimens (blood and catheter) on the same patient (Lomonaco et al., 2018).
Transfer experiments
blaNDM-1 or blaOXA-48-plasmids from the above 10 K. pneumoniae isolates were transferred to an E. coli J53 recipient strain. Conjugal transfer of carbapenem resistance between the K. pneumoniae isolates and E. coli J53, an azide-resistant recipient strain susceptible to all antibiotics, was performed as previously described (Lascols et al., 2008). Briefly, all isolates were grown to logarithmic phase in Brain Heart Infusion broth (BHI; ThermoFisher Scientific, MA, USA), and 2 ml of the donor and the recipient strain suspensions were mixed in flasks and incubated at 37°C for 40 min without shaking. Transconjugant selection was performed on Mueller-Hinton (ThermoFisher Scientific, MA, USA) plates containing sodium azide (100 μg/ml; ThermoFisher Scientific, MA, USA) and cefotaxime (16 μg/ml) or ticarcillin (100 μg/ml; TOKU-E, WA, USA) for transfer of blaNDM-1 and/or blaOXA-48-plasmids, respectively. Plates were incubated at 37°C and inspected at 24 and 48 h for bacterial growth.
Antimicrobial susceptibility testing
Nine E. coli transconjugants were obtained: Ec_pBU19801_NDM, Ec_pMS84_NDM, Ec_pBL12125_NDM, Ec_pBL12456_NDM, Ec_pBA3783_NDM, Ec_pBL13802_NDM&OXA-48, Ec_pBA2664_OXA-48, Ec_pBL8800_OXA-48, and Ec_pBA2880_OXA-48. AST was carried out according to Clinical and Laboratory Standards Institute (CLSI) guidelines (CLSI, 2018; CLSI, 2023) and results interpreted using CLSI and European Committee on Antimicrobial Susceptibility Testing (EUCAST) breakpoints (EUCAST, 2023) for the above E. coli transconjugants.
DNA extraction
Different DNA extraction methods were used for total genomic and plasmid DNA purification. Total genomic DNA was extracted using the QIAGEN DNA Mini Kit (QIAGEN, Valencia, CA, USA) per the manufacturer’s instructions from a 10 μl loopful of cells harvested from isolated colonies grown overnight.
Plasmid DNA was extracted from 100 ml overnight culture using the QIAGEN Plasmid Midi Kit (QIAGEN, Valencia, CA, USA) per the manufacturer’s instructions. For the K. pneumoniae isolates, the QIAGEN low-copy plasmid protocol was modified: the volumes of P1, P2, and P3 buffers were doubled (8 ml instead of 4 ml). DNA concentrations and purity were measured using a Qubit 3.0 fluorometer (ThermoFisher Scientific, MA, USA) with a dsDNA Broad Range Assay Kit and Nanodrop (ThermoFisher Scientific, MA, USA), respectively. DNA quality was assessed by measuring absorbency ratios at 260 nm/280 nm and at 260 nm/230 nm to ensure that the manufacturer’s recommended ratios of 1.8 and 2.0–2.2, respectively, were met prior to sequencing library preparation.
Illumina MiSeq library preparation and sequencing
Libraries for Illumina MiSeq sequencing were prepared from total genomic DNA of each original isolate, and each E. coli transconjugant using the Nextera XT library prep kit (Illumina, San Diego, CA, USA), following the manufacturer’s instructions. DNA libraries were sequenced on a MiSeq instrument using 2×250 bp paired-end MiSeq Reagent Kit v2 (Illumina, San Diego, CA, USA) chemistry.
Multiplexed nanopore library preparation and sequencing
MinION sequencing libraries were prepared from plasmid DNA preparations from both K. pneumoniae isolates and E. coli transconjugants. The SQK-RBK004 rapid barcoding kit was used according to the manufacturer’s protocol (Oxford Nanopore Technologies (ONT), Oxford, UK). The constructed library was loaded into a R9.4 (FLO-MIN106) Flow Cell on a MinION device and run with the High Accuracy BaseCaller script of MinKNOW1.5.12 for 72 h. The long reads sequencing data were stored on the MinIT, and accessible in real-time during the run.
Generation of assembly sequences
Whole genome de novo assemblies obtained from SPAdes Genome Assembler (Bankevich et al., 2012) were used for analyses. Plasmid de novo assemblies were obtained from four genome assemblers: Miniasm, WTDBG2, Canu, and Flye (Koren et al., 2017; Kolmogorov et al., 2019; Ruan and Li, 2020). Assemblers were run with default parameters, resulting in a genome size of 5.4 M as expected for K. pneumoniae isolates. Hybrid assemblies from raw MinION reads were generated first by aligning MiSeq reads to the corresponding de novo assembly using minimap2 (Li, 2018). These alignments and de novo assembly were used as input to the Pilon utility, generating the error-corrected, hybrid assembly (Walker et al., 2014). All plasmid sequences for K. pneumoniae isolates were uploaded to the publicly available NCBI BioProject PRJNA946140.
NGS-based prediction of antimicrobial resistance and plasmid and replicon-type analysis
Once NGS data are generated, AMR analysis tools were used for fully characterizing the AMR gene content and determining the plasmid types. Some software tools (e.g., ResFinder /PlasmidFinder/pMLST) rely on regular updates and curation (Bortolaia et al., 2020; Carattoli and Hasman, 2020) to accurately identify known and newly emerged ARGs ARGs/plasmid types across diverse bacterial species. To generate a comprehensive genotypic AMR profile from long-read sequences, and perform plasmid-typing, three publicly available web-based tools developed by the Center for Genomic Epidemiology (CGE): ResFinder, PlasmidFinder and pMLST were used.
ResFinder 4.01 was used to independently identify acquired AMR determinants and determine NGS-based predicted phenotypes (Bortolaia et al., 2020). Full-length AMR determinants sharing more than 98% similarity and 100% coverage with the genes of interest found in the ResFinder databases were considered “present,” and included in the organism’s NGS-based AMR profile. In case of discrepant findings, the presence/absence of an AMR gene was determined manually by conducting BLAST analysis. Each gene’s presence was interpreted as either resistant (R) or not resistant (NR) by ResFinder 4.0. All NGS-predicted phenotypes were compared with conventional BMD results for E. coli transconjugants.
Plasmid incompatibility (Inc) groups were assessed using PlasmidFinder 2.02 from CGE with default settings. Briefly, PlasmidFinder uses a curated database of known plasmid replicons for identifying plasmid incompatibility groups in Enterobacteriaceae whole genome sequences (Carattoli and Hasman, 2020). The software assigns plasmids for identification of lineages and suggests possible reference plasmids for additional analysis.
pMLST analysis was carried ou t with pMLST 2.0,3 which is a web-based tool for bacterial typing of MDR Enterobacteriaceae for rapid detection of known plasmid types (Carattoli and Hasman, 2020).
Those web-based tools (ResFinder4.0, PlasmidFinder2.0 and pMLST2.0) were used for determining AMR profiles, plasmid Inc. groups and types for each long-read sequence from the K. pneumoniae isolates and their corresponding E. coli transconjugants.
Comparative analysis of plasmid structure in E. coli transconjugants.
The analysis of the structure of the blaNDM-1 plasmids transferred to E. coli was performed using an intuitive interface for rapid annotation of plasmid sequences that does not require bioinformatics expertise and provide detailed easy-to understand diagrams (Partridge and Tsafnat, 2018).
Results
All NDM-producers carried at least two MDR plasmids, one with blaNDM-1 and one carrying one or more genes encoding other ß-lactamases, either a non-ESBL (blaTEM-1B) and/or an ESBL (blaCTX-M-15); except isolate MS84, which did not carry any blaESBL-plasmid (Table 1). All OXA-48-producers carried two to three plasmids, one with blaOXA-48, one with blaTEM-1B, and/or an additional plasmid containing ARGs ARGs such as those encoding aminoglycoside and/or rifampicin resistance (Table 1). Plasmids were transferred to E. coli J53 via conjugation to isolate blaNDM-1- and blaOXA-48-plasmids for sequencing and analysis. Overall, a total of nine E. coli transconjugants harboring either blaNDM-1 (n = 6) or blaOXA-48 (n = 3) plasmids were obtained from the 10 K. pneumoniae isolates. No transconjugant was obtained for BL849. For the two blaNDM-1/OXA-48 positive isolates (BA3783 and BL13802): only blaNDM-1-plasmid was successfully transferred from BA3783, while both blaNDM-1 and blaOXA-48-plasmids were successfully transferred from BL13802 (Table 1).
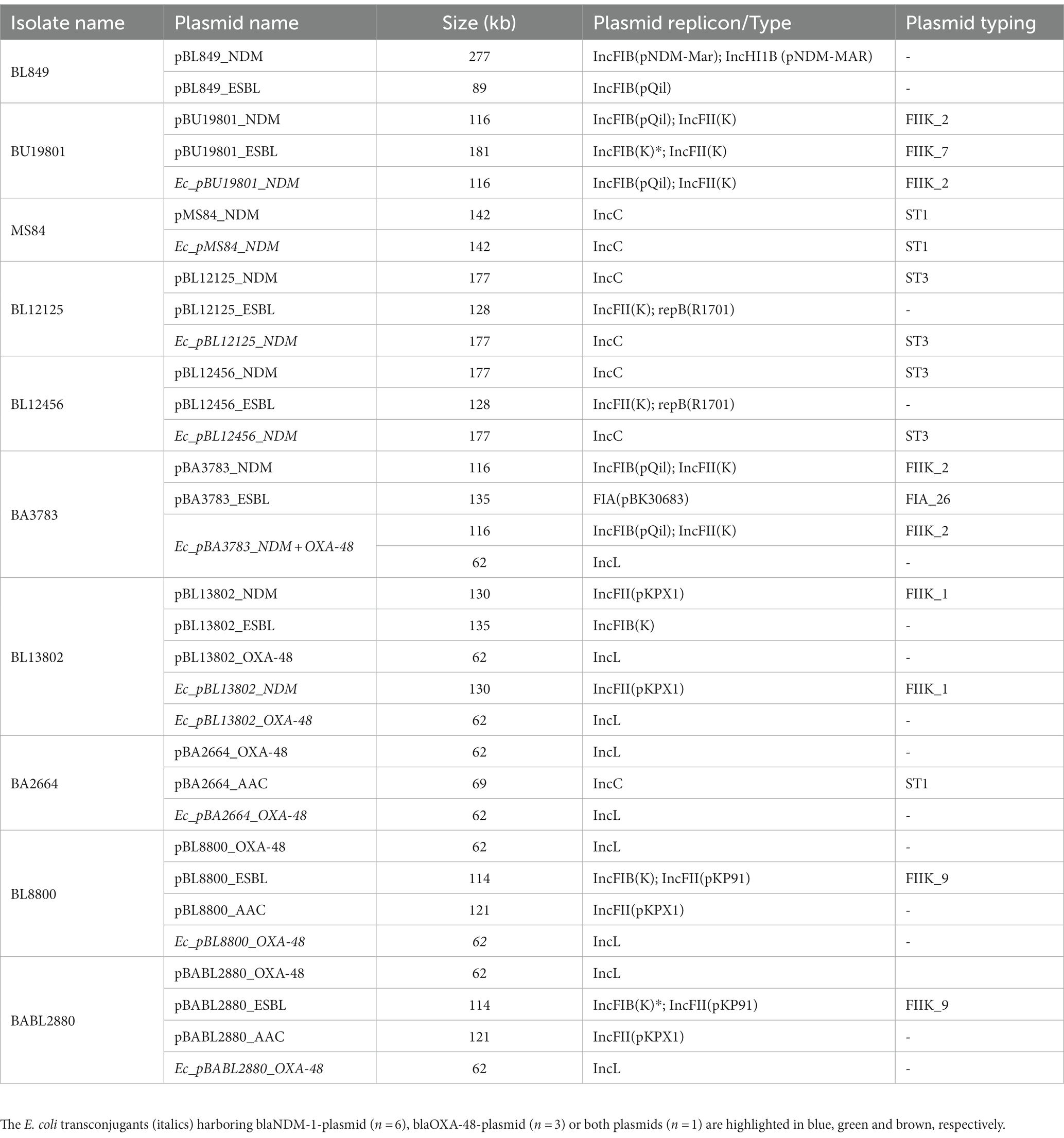
Table 1. List of MDR plasmids identified from Nanopore hybrid plasmid assemblies from 10 MDR K. pneumoniae clinical isolates and their respective E. coli transconjugants.
Comparison of assembly tools for generating hybrid plasmid assemblies
Multiple assemblers can be used for whole genome DNA assembly, but we assessed which assembler would perform best on plasmids by comparing the most common assemblers: Miniasm, WTDBG2, Canu, and Flye. Overall, six PacBio plasmid sequences were used for reference: three originating from BL12456 (BL12456_pNDM, BL12456_pESBL; Table 1), and a non-AMR plasmid, and three plasmids from MS84 (MS84_pNDM; Table 1), and two non-AMR plasmids. Canu could not accurately assemble any of the six plasmid sequences. WTDBG2 correctly assembled four plasmid sequences, and Miniasm assembled five out of six plasmid sequences. Flye accurately assembled all six plasmid sequences, thus being the assembler that produced the most accurate and reliable hybrid assemblies. Therefore, Flye was used to generate assemblies for our collection of isolates (Figure 1). This finding is consistent with a new pipeline WeFaceNano for complete ONT sequence assembly and detection of AMR in plasmids (Heikema et al., 2021).
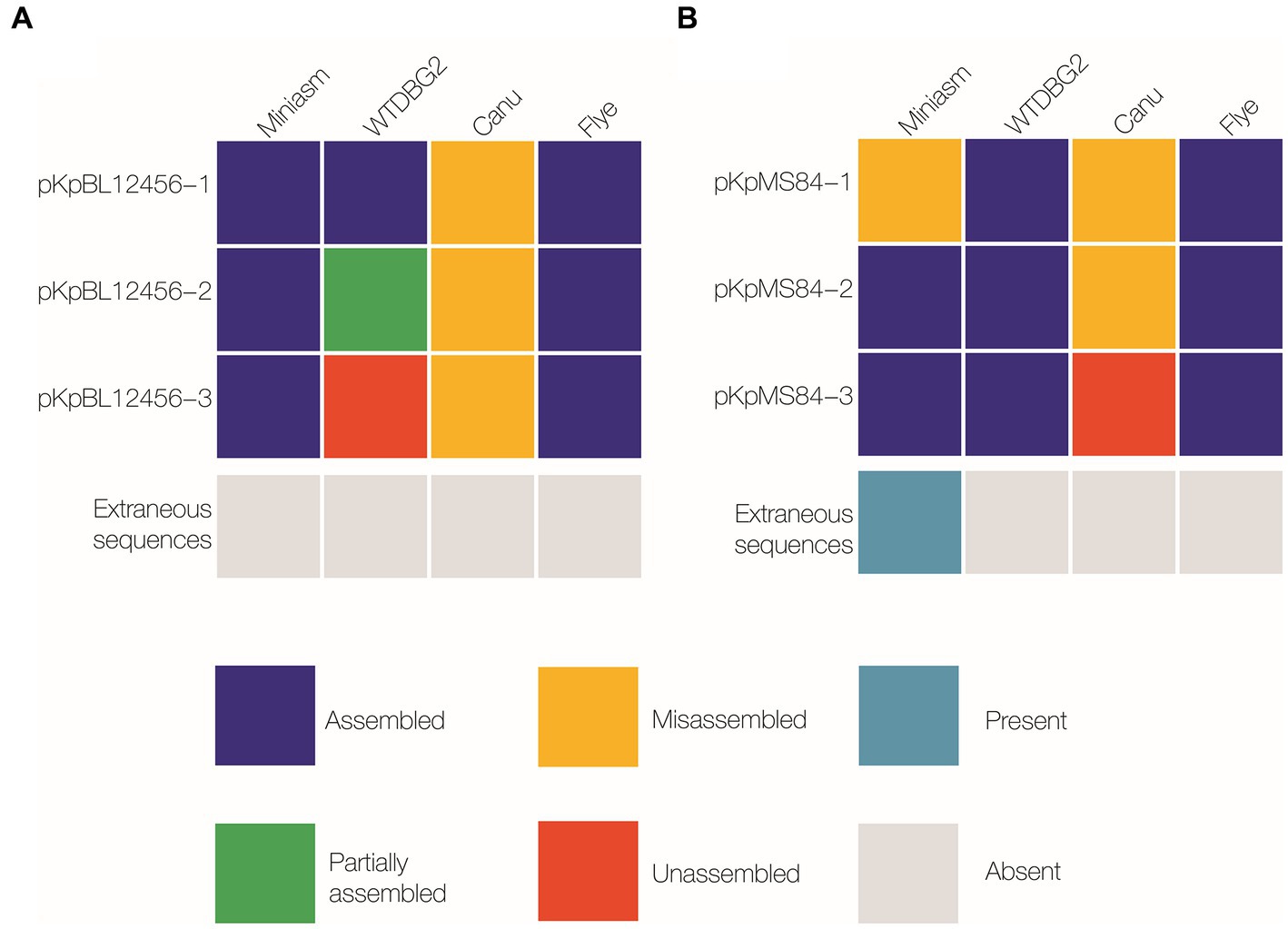
Figure 1. Comparison of plasmid-sequence assembly by Miniasm, WTDBG2, Canu, and Flye. Three plasmids were isolated from each of two K. pneumoniae strains: BL12456 (pKpBL12456-1, −2 and −3; A), and MS84 (pKpMS84-1, −2 and −3; B). Assembled was considered a complete sequence with the same nucleotide order as the reference sequence (navy); partially assembled represented a sequence that was assembled, but not as a single contig (green); misassembled included a sequence that was assembled, but the bases were in a different order than the reference sequence, or a sequence assembled multiple times resulting in an incomplete assembly (yellow); unassembled refers to a sequence that was not present in the reference assembly (orange).
Distribution and identification of plasmid-encoded AMR determinants by antimicrobial classes using ResFinder
ResFinder identified a total of 34 plasmid-mediated AMR determinants from hybrid assemblies of the 10 MDR K. pneumoniae isolates. Out of the 34 plasmid-mediated AMR determinants identified, the frequencies by antimicrobial agent classes were: β-lactams (23%, n = 8); aminoglycosides (35%, n = 12; trimethoprim and macrolides, 9% each, n = 3); 6% (fluoroquinolones, sulfamethoxazole, chloramphenicol, 6% each, n = 2). For tetracycline and rifampicin, the frequency was 3% for each (n = 1; Supplementary Figure S1). Excluding the 2 pairs of similar K. pneumoniae isolates, blaNDM-1, blaESBL, and blaOXA-48-plasmids were identified in 6, 6 and 4 out of a total of 8 K. pneumoniae isolates, respectively. Single blaNDM-1 and blaOXA-48-plasmids were identified in two K. pneumoniae isolates (BA3783 and BL13802).
A total of eight different β-lactamase genes, encoding enzymes belonging to four classes, were identified: blaNDM-1, blaOXA-10, blaCMY-6, and blaCMY-16 on NDM plasmids; blaCTX-M-15, blaOXA-1, and blaTEM-1, on blaESBL-plasmids, and blaOXA-48-plasmids (Table 2). A total of 12 different plasmid-associated aminoglycoside resistance genes were identified: aac(6′)-Ib, aph(3″)-Ib, aph(6)-Id, aadA1/A2; aph(3′)-VIb, rmtC/F, and armA on blaNDM-1-plasmids, and aac(3)-IIa/d, aac(6′)-Ib-cr, aph(3′)-Ia, aph(3″)-Ib, and aph6-Id on blaESBL-plasmids (Table 2). A total of two plasmid-mediated qnr genes were identified in four K. pneumoniae isolates (qnrB1, n = 3; qnrS1, n = 1) on blaESBL-plasmids and aac(6′)-Ib-cr was identified in 6 K. pneumoniae isolates on blaESBL-plasmids as well. Three variants of the dfrA gene (dfrA1, dfrA12, and dfrA14), which confer resistance to trimethoprim, and two variants of the sul gene (sul1 and sul2), that confer resistance to sulfamethoxazole, were detected on blaESBL-plasmids. In five K. pneumoniae isolates, dfrA and sul genes were found together on blaESBL-plasmids: dfrA1/sul1, dfrA14/sul2, dfrA14/sul1/sul2, and dfrA12/sul1, but sul1 alone was also identified on the blaNDM-1-plasmid in six K. pneumoniae isolates. The tetracycline resistance determinant tetA was identified on blaESBL-plasmids from two K. pneumoniae isolates; a third K. pneumoniae isolate possessed a tetA gene with 99.92% similarity to the reference sequence (Genbank accession number: AJ517790) on blaESBL-plasmid. The arr2 gene conferring resistance to rifampicin, was identified on blaNDM-1-plasmids in six K. pneumoniae isolates. Macrolide resistance conferred by determinants such as mphE and msrE genes were identified on blaNDM-1-plasmids in three and four K. pneumoniae isolates, respectively. mphA was identified on blaESBL-plasmid in one K. pneumoniae isolate. For chloramphenicol, cmlA5 was identified on blaNDM-1-plasmids of two K. pneumoniae isolates. ESBL plasmids carried a catB4 gene, which was subsequently found to be a truncated catB3 gene in six K. pneumoniae isolates, and catA1 was present in one K. pneumoniae isolate (data not shown).
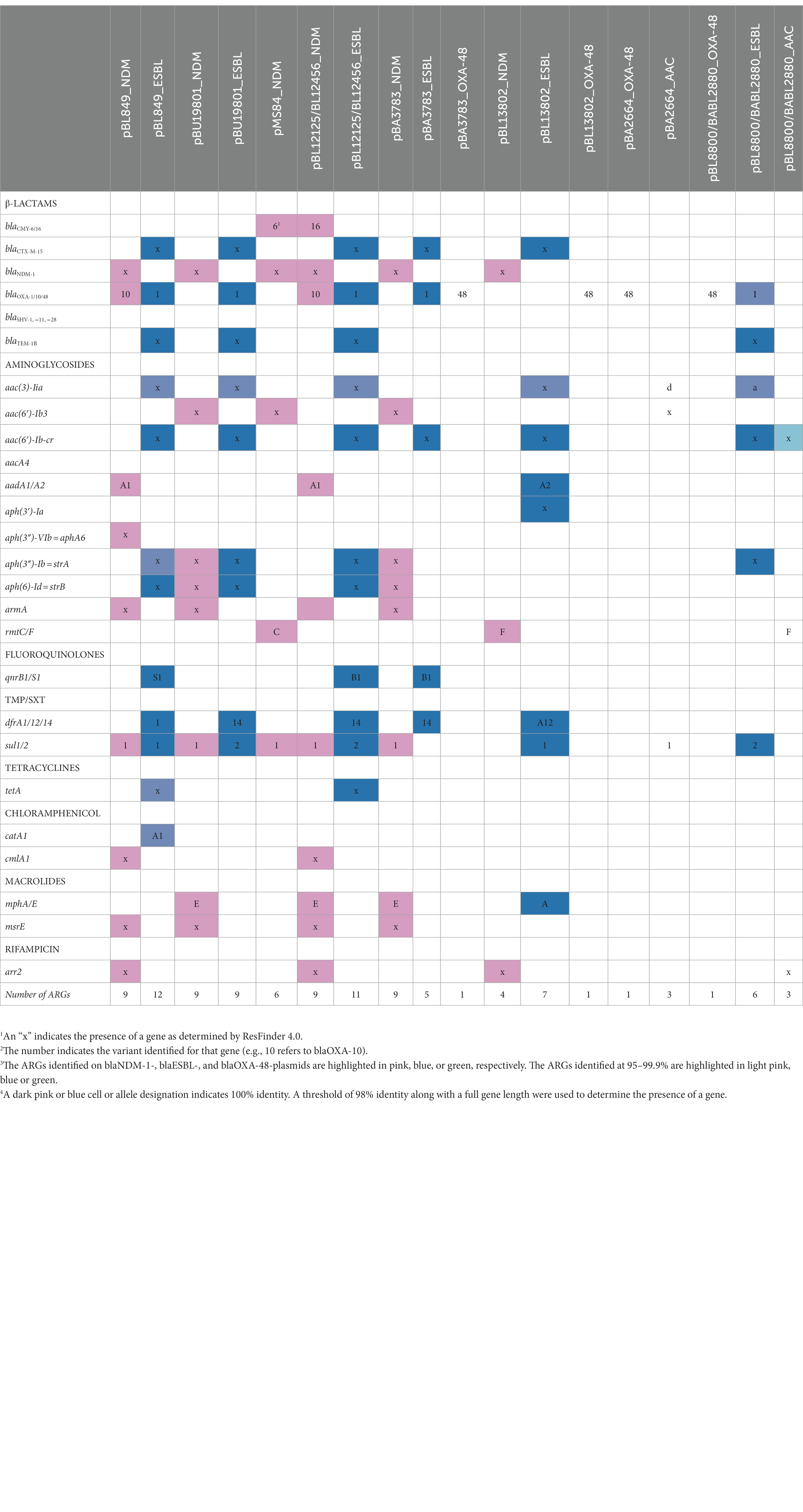
Table 2. Antimicrobial resistance determinants identified from Nanopore hybrid plasmid assemblies from 10 MDR K. pneumoniae clinical isolates.
Analysis of NGS-based genotypic AMR profiles and phenotypes from plasmids
NGS-based AMR profiles were generated from the hybrid assemblies obtained from plasmid preps from both the original K. pneumoniae isolates, and the E. coli transconjugants. The plasmids isolated from K. pneumoniae isolates are noted with a p in front of the isolate designation (e.g., pMS84) while the plasmids isolated from the E. coli transconjugants are noted with Ec before the isolate name (e.g., Ec_pMS84_NDM). The presence of both blaNDM-1 or blaOXA-48 and blaESBL plasmids in the original K. pneumoniae isolates could not explain the contribution of each plasmid in NGS-based AMR profiles, while the NGS-based AMR profiles obtained from E. coli transconjugants gave insights on the contribution of ARGs ARGs present on blaNDM-1 or blaOXA-48 plasmids. All detected ARGs ARGs, NGS-predicted AST results for K. pneumoniae isolates, and E. coli transconjugants, and AST results for E. coli transconjugants are summarized in Supplementary Table S2 (β-lactams), Supplementary Table S3 (aminoglycosides), Supplementary Table S4 (fluoroquinolones), and Supplementary Table S5 (trimethoprim/sulfamethoxazole, tetracyclines, and chloramphenicol).
Resistance to β-lactams
All five K. pneumoniae isolates (BL849, BU19801, MS84, BL12125/456) carrying blaNDM-1 were resistant to all classes of β-lactam antimicrobials. Among these isolates, three (BL849, BL12125, and BL12456) carried up to five β-lactamase genes: one blaCTX-M-15 ESBL gene, one blaOXA-1 along with blaTEM-1B on the blaESBL-plasmid, and blaOXA-10 on the blaNDM-1-plasmid, including two isolates that were found to carry the blaCMY-16 AmpC β-lactamase gene on the blaNDM-1-plasmid. BU19801 carried three β-lactamase genes (blaCTX-M-15, blaOXA-1, and blaTEM-1B) on the blaESBL-plasmid while MS84 carried in addition to blaNDM-1 only one gene variant of AmpC β-lactamase (blaCMY-6) on the blaNDM-1-plasmid. Since most β-lactamase genes were located on the blaESBL-plasmid, when blaNDM-1 was transferred by itself, the E. coli transconjugants were susceptible to aztreonam which agrees with the definition of NDM-1 producers except for BL12125 and BL12456. When additional β-lactamase genes were transferred, such as blaCMY-16 and blaOXA-1 (BL12125 and BL12456), the E. coli transconjugant isolates were resistant to all β-lactams tested by both the NGS-based approach and confirmed by BMD.
For most blaNDM-1-positive E. coli transconjugants, the NGS-based AMR profiles were in concordance with conventional AST results, and consistent with the characteristics of NDM-1 producers [example: NDM-1 producers hydrolyze all ß-lactams except aztreonam (ATM)]. However, the absence of blaCTX-M-15 was interpreted by ResFinder as not resistant to ceftriaxone (CRO) and ATM for five blaNDM-1-positive (Ec_pBU19801_NDM, Ec_pMS84_NDM, Ec_pBL12125/456_NDM, Ec_pBA3783_NDM, and Ec_pBL13802_NDM) and two blaOXA-48-positive E. coli transconjugants (Ec_pBA2664_OXA-48, Ec_pBL8800/pBABL2880_OXA-48) respectively, and one K. pneumoniae isolate (MS84). MICs for CRO and ATM were ≥32 μg/ml for those K. pneumoniae/E. coli isolates which is interpreted as resistant (≥4 μg/ml) by CLSI (2023), therefore those NGS-based results did not match BMD results (Supplementary Table S1).
Among the three blaOXA-48-positive K. pneumoniae isolates, two (BL8800 and BA2880) carried both blaTEM-1, and blaOXA-1 located on a blaESBL-plasmid and were resistant to penicillins [ampicillin (AMP), amoxicillin-clavulanic acid (AMC), ampicillin-sulbactam (SAM), piperacillin-tazobactam (TZP)], cephalosporins [cefazolin (CZ), cefotaxime (CTX), ceftazidime (CAZ), CRO, cefepime (FEP)], and ATM and yet were susceptible to doripenem (DOR), meropenem (MEM) and non-susceptible to imipenem (IMP). One K. pneumoniae isolate (BA2664) did not carry any blaESBL-plasmid and was resistant to all ß-lactams except CAZ and ATM.
The three E. coli transconjugant isolates that carried the blaOXA-48 plasmid were also resistant to the first generation of β-lactams, but not to the more recent generations of cephalosporins (FOX, CTX, CAZ, CRO, and FEP) and carbapenems (IPM, MEM, ERT) except DOR. This susceptibility profile is consistent with the observation that OXA-48 producers hydrolyze carbapenems at a low level (Poirel et al., 2012). However, in two instances (Ec_pBL8800/BABL2880_OXA-48), the presence of blaOXA-48 was interpreted by ResFinder as resistant for IPM and MER, which does not agree with MICs for IPM and MEM of 1 and 2 μg/ml (susceptible), respectively (Supplementary Table S1).
Resistance to aminoglycosides
A total of 11 different genes responsible for high-level aminoglycoside resistance: (i) N-acetyltransferases (AAC): aac(3)-IIa, aac(6′)-Ib-cr; (ii) aminoglycoside-O-nucleotidyltransferases (ANT): aadA1, and aadA2; (iii) aminoglycoside-O-phosphotransferases (APH): aph(3′)-Ia, aph(3″)-Ib, aph(6)-Id, aph(3′)-VIb; n = 4; and (iv) 16S rRNA methylases (armA, rmtC or rmtF) were identified in E. coli transconjugants (Supplementary Table S2). All of the E. coli transconjugants carrying blaNDM-1-plasmids were resistant to AMK (MIC > 64 μg/ml), GM (MIC > 16 μg/ml), and TOB (MIC > 16 μg/ml) by ResFinder and confirmed by BMD.
Out of the six blaNDM-1-positive E. coli transconjugants, each of the blaNDM-1-plasmids carried at least one AMR gene conferring resistance to amikacin (AMK), gentamicin (GM) and tobramycin (TOB). Four carried aac(6′)-Ib-cr either solely (n = 2) or with armA (n = 2), rmtC (n = 1), or rmtF (n = 1). In addition to all the ARGs identified on the NDM plasmids, most K. pneumoniae isolates harbored additional ARGs conferring resistance to aminoglycosides such as aac3-IIa on the blaESBL-plasmids except MS84 and BA3783. Ec_pBA2664_OXA-48 did not carry any aminoglycoside resistance gene except aac3-IId located on a non-blaESBL-plasmid but was called resistant by ResFinder, which did not agree with MICs of susceptible of 8 μg/ml.
Other ARGs conferring resistance to STR (not tested by BMD) such as aadA2 and aph(3′)-Ia were identified on the blaESBL-plasmid (pBL13802) of a single K. pneumoniae isolate. The aph(3″)-Ib gene also called strA was identified on four blaESBL-plasmids (pBL849_ESBL, pBU19801_ESBL, pBL12125/456_ESBL and pBL8800/BABL2880_ESBL) and two blaNDM-1-plasmid (pBU19801_NDM and pBA3783_NDM). aph(6)-Id also called strB was identified on the same above plasmids except for pBL8800/BABL2880_NDM for which aph(6)-Id was missing. Two E. coli transconjugants carried aadA1 and two carried both aph(3″)-Ib and aph(6)-Id.
Resistance to fluoroquinolones
All nine E. coli transconjugants that were susceptible to CIP (MICs ≤0.25 μg/ml) did not carry any aac(6′)-Ib-cr, qnrB, or qnrS. However, ResFinder identified a truncated version of aac(6′)-Ib-cr-EF636461 (519 bp) in 4/9 E. coli transconjugants (Supplementary Table S3) which were categorized as resistant. This observation suggests that this truncated aac(6′)-Ib-cr may not confer resistance to fluoroquinolones and should be removed from the ResFinder database until confirmation.
Resistance to trimethoprim-sulfamethoxazole (cotrimoxazole), tetracyclines, and chloramphenicol
Modified dihydrofolate reductase (DHFR) and dihydropteroate synthetase (DHPS), enzymes encoded by dfr and sul, respectively, are involved in resistance to cotrimoxazole (SXT; trimethoprim + sulfamethoxazole; Ramirez and Tolmasky, 2010). The five E. coli transconjugants carrying blaNDM-1-plasmid that harbored sul1 without any dfr genes likely explained their susceptibility to SXT. The absence of the dfr/sul genes on blaNDM-1-plasmid for four E. coli transconjugants was consistent with the observed susceptibility to SXT. However, Resfinder does not propose any interpretation categorization for SXT susceptibilities. The absence of the tetA gene on blaNDM-1-plasmid was consistent with the observed susceptibility to TET by AST. Two E. coli transconjugants, carrying cmlA5 on the blaNDM-1 plasmid, were susceptible to CHL (MIC = 8 μg/ml) but categorized as resistant to CHL by Resfinder (Supplementary Table S4).
Structure of MDR plasmids identified in MDR Klebsiella pneumoniae clinical isolates
The structures of each plasmid transferred via conjugation and identified in E. coli transconjugants were consistent with the plasmids identified in the K. pneumoniae study isolates (100% similarity). The blaNDM-1-plasmid was the only plasmid that was transferred for BA3783 while both blaNDM-1- and blaOXA-48-plasmids were transferred for BL13802. Lastly, no transconjugant was obtained for BL849. The incompatibility groups identified for (1) blaNDM-, (2) blaESBL, and (3) blaOXA-48-plasmids were: (1) IncFIB, IncHI1B, IncFII, and IncC; (2) IncFIB; and IncFII; and (3) IncL, respectively. The pMLST types identified were IncC11 and IncC3 (n = 2), IncFII2 and IncFII1 among blaNDM-1-plasmids and were IncFIIK7, IncFIIK9, and IncFIA26 among blaESBL-plasmids (Table 1).
NDM-producers (n = 7): BL849, BU19801, MS84, BL12125, BL12456, BA3783, BL13802
Among the seven blaNDM-1-positive K. pneumoniae isolates, the number of AMR determinants encoded on blaNDM-1- and blaESBL-plasmids varied from 4 to 10, and 7–12 genes, respectively. Whole-plasmid hybrid sequencing identified seven blaNDM-1-plasmids of various sizes ranging from 117 to 277 kb: pBL849_NDM (277 kb), pBU19801_NDM (181 kb), pMS84_NDM (142 kb), pBL12125_NDM and pBL12456_NDM (177 kb), pBA3783_NDM (117 kb), and pBL13802_NDM (130 kb). Among the seven blaNDM-1-plasmids, IncC was the most common incompatibility group identified (n = 3), followed by IncFIB (n = 2), IncFII (n = 1), and IncFIB/IncHI1B (n = 1; Table 1).
BLAST analysis of the complete nucleotide sequences of MDR plasmids identified in this study found zero exact matches to previously reported MDR plasmids for five K. pneumoniae isolates: pBL849_NDM, pBU19801_NDM, pBL12125/456_NDM and pBA3783_NDM. However, pMS84_NDM and pBL13802_NDM each shared high similarity with two plasmids previously described, pB557-NDM-KX786648 (142 kb) and pKX-1-NDM-AP012055 (250 kb), respectively (Figures 2, 3).
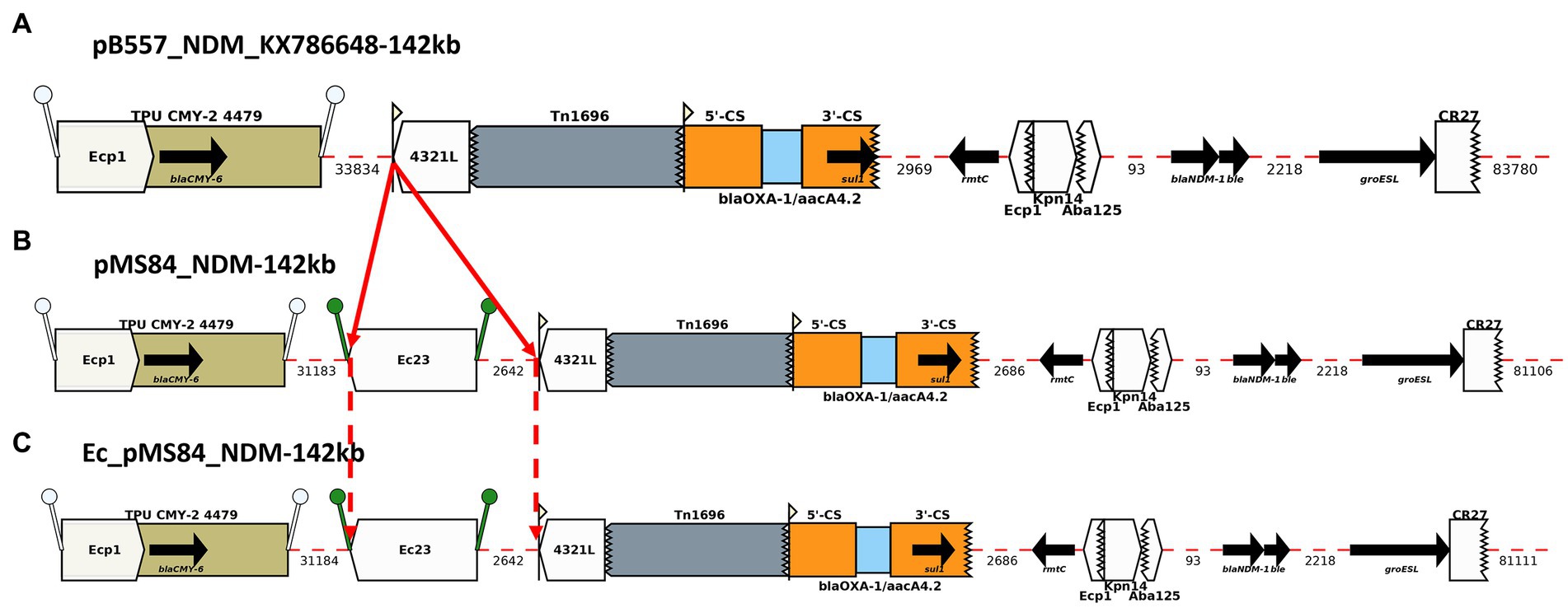
Figure 2. Annotation Diagram of (A) pB557-NDM_KX786648, (B) MS84_pNDM, and (C) Ec_MS84_pNDM using Galileo AMR platform. Gaps >50 (base pairs) bp are indicated by dashed red lines and the length in bp is given. Genes features (e.g., blaCMY-6, sul1, rmtC, blaNDM-1) are shown by arrows; gene cassettes (e.g., blaOXA-1/aacA4.2) by pale blue boxes; the CS of integrons as orange boxes; and IS (e.g., ISEc23) as white block arrows labelled with the IS number/name and the pointed end indicating IRR. Unit transposons (e.g., Tn1696) are shown as boxes of different colors and their IR are shown as flags, with the flat side at the outer boundary of the transposon. Truncated features (e.g., Tn1696) are shown with a jagged edge on the truncated side(s). Direct repeats flanking ISs are shown as ‘lollipops’ of the same color ISEcp1 (white) and ISEc23(green). Here, an ISEc23 has been inserted in MS84_pNDM and Ec_MS84_pNDM compared to pB557-NDM-KX786648.
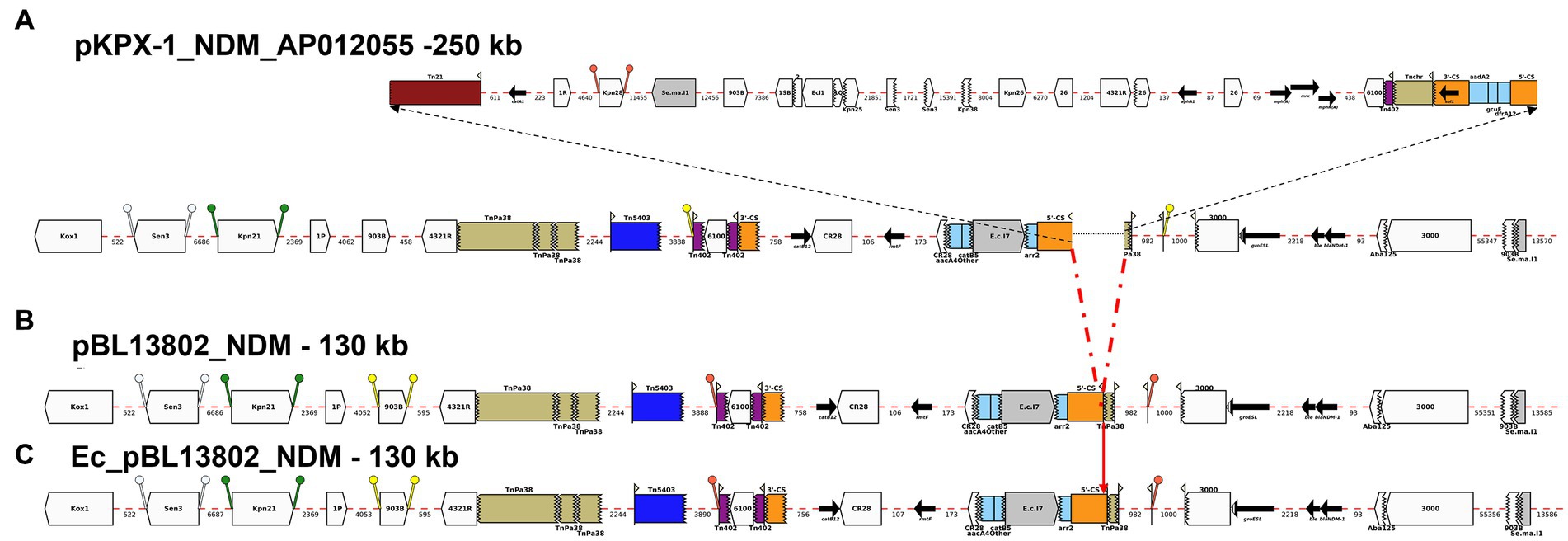
Figure 3. Annotation Diagram of (A) pKPX-1-NDM_AP012055, (B) BL13802_pNDM, and (C) Ec_BL13802_pNDM using Galileo AMR platform. Gaps >50 (base pairs) bp are indicated by dashed red lines and the length in bp is given. Genes features (e.g., catB12, rmtF, blaNDM-1) are shown by arrows; gene cassettes (catB3/aacA4/arr2) by pale blue boxes; the CS of integrons as orange boxes; and IS (e.g., IS3000) as white block arrows labelled with the IS number/name and the pointed end indicating IRR. Unit transposons are shown as boxes of different colors (e.g., Tn5403, blue, Tn40, dark purple) and their IR are shown as flags, with the flat side at the outer boundary of the transposon. Truncated features (e.g., 3’-CS) are shown with a jagged edge on the truncated side(s). Direct repeats flanking ISs are shown as ‘lollipops’ of the same color ISKpn21 (green) and IS903B (yellow). Here, a 46-kb region containing aacA4, catB3, and arr2 cassettes is found in pKPX-1-A012055 upstream of blaNDM-1 both in BL13802_pNDM, and Ec_BL13802_pNDM.
pMS84_NDM shared 100% identity with pB557-NDM_KX786648 (GenBank accession number: KX786648), a blaNDM-1-plasmid isolated from an Enterobacter cloacae clinical isolate in China in 2016. An ISEc23 (5 kb) was inserted downstream the ISEcp1/blaCMY-6 module and upstream of a Tn1696 transposon in pMS84_NDM (Figure 2). The ARGs identified on this blaNDM-1-plasmid were: blaCMY-6, sul1, rmtC, and blaNDM-1.
pBL13802_NDM was identical to part of the 250-kb total sequence (99.98% similarity) of plasmid pKPX-1-NDM-AP012055 (Genbank accession number: AP012055), a plasmid isolated from K. pneumoniae strain KPX-1, obtained from a Taiwanese patient with a hospitalization history in New Delhi (Huang et al., 2013). pBL13802_NDM lacked a 120-kb region from pKPX-1 plasmid. pBL13802_NDM contained a 22-kb AMR region, also found in pKPX-1-AP012055, composed of several gene cassettes, such as catB3, aac(6′)-Ib, aacA4 and arr2, as well as a region containing blaNDM-1 (Figure 3).
BLAST analysis identified regions/AMR modules that were present in several of the study plasmids: Modules A-D and N0-N3. The AMR modules identified in this study are illustrated in Figures 4, 5, respectively. Module A is composed of a complete gene cassette of 5 ARGs: 3’-CS-[arr2/cmlA5/blaOXA-10/aadA1/sul1]-5’-CS. Module B has armA, msrE-like, and mphE (2 copies) genes, along with multiple insertion sequences (IS; ISEc28, ISEc29, and ISKpn21). Module C contains two ARGs: a truncated aac(6′)-Ib-cr and sul1. Module D is composed of ISKpn25 along with two strA/strB ARGs (Figure 4).
Module N0 here is composed of blaNDM-1, bleMBL, and groESL which is consistent with the backbone of a truncated ΔTn125 transposon previously described in multiple blaNDM-1-plasmids (Dong et al., 2019). Prior studies showed that ISAba125 mobilized “en bloc” both blaNDM-1 and bleomycin bleMBL genes which originated from the same progenitor (Poirel et al., 2011). blaNDM-1 is mainly and widely spread by an ISAba125-bounded composite transposon Tn125 (Poirel et al., 2012). Module N1 is a section downstream of Module N with multiple truncated IS (ISCr27/ISCRp4/IS3000) along with a complete sequence of IS3000 and IS26. Module N2 is composed of Module N except groESL is flanked upstream of ISAba125 by a set of three ARGs (e.g., blaOXA-10, aadA1, and sul1). Lastly, Module N3 is very similar with Module N1 with an addition of IS5 upstream of ISAba125 (Figure 5).
Among the seven blaNDM-1-positive K. pneumoniae: pBL849_NDM and pBL12125/456_NDM, shared modules A and B and some variations of module N (N1, BL849 and N2, BL12125/456) while pBU19801_NDM and pBA3783_NDM, shared the exact same modules: B, C, D and N3 (Figure 6).
pBL849_NDM, and pBL12125/456_NDM shared a common set of seven ARGs (Table 2) including arr-2, cmlA5, aadA1, sul1 as part of Module A, and armA, msrE, and mphE (2 copies for pBL849_NDM) as part of Module B. Also, pBL849_NDM and pBL12125/456_NDM carried different genes or IS around blaNDM-1 (Module N1 for pBL849_NDM and Module N2 for pBL12125/456_NDM; Figure 6).
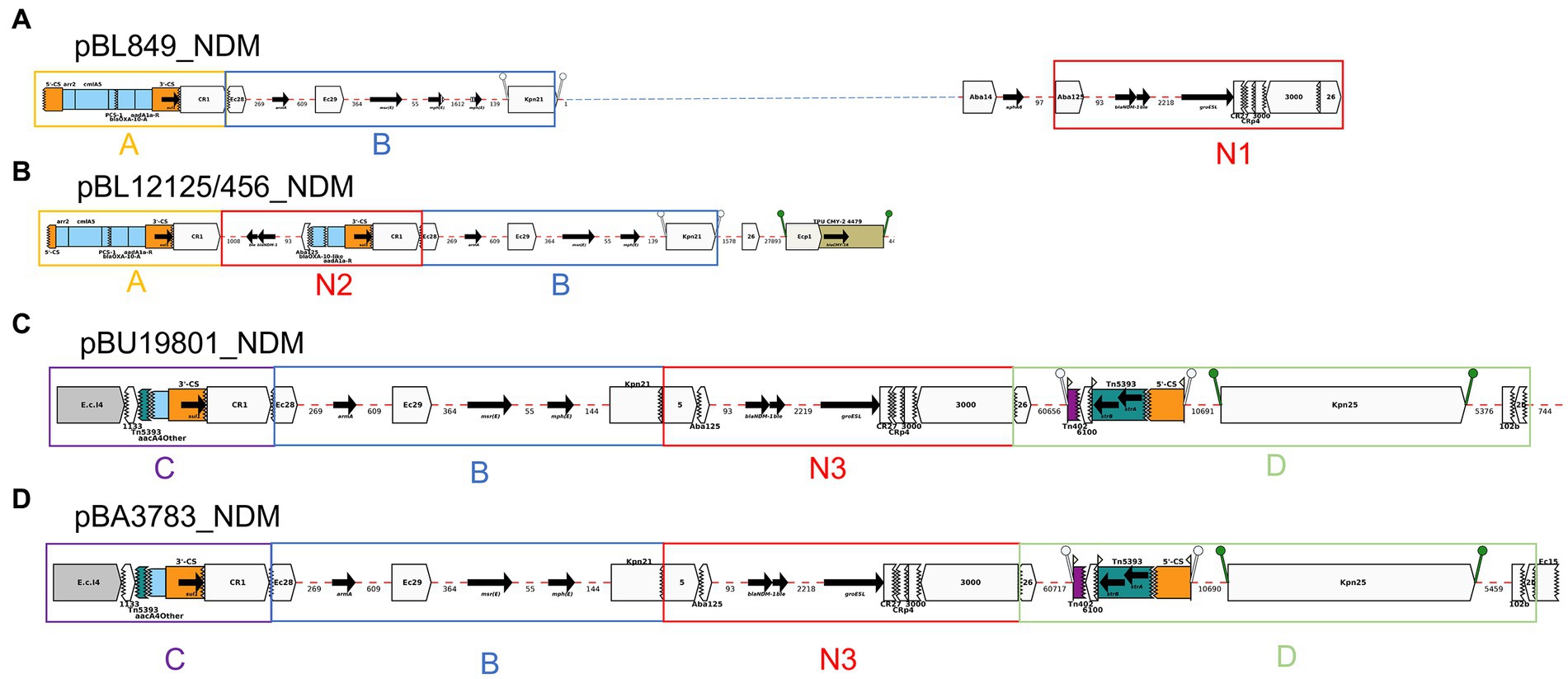
Figure 6. Annotation Diagrams of pNDM-plasmids (A) BL849_pNDM, (B) BL12125/456_pNDM, (C) BU19801_pNDM, and (D) BA3783_pNDM using Galileo AMR platform. Gaps >50 bp are indicated by dashed red lines and the length in bp is given. Genes features (e.g., armA, sul1, blaNDM-1) are shown by arrows; gene cassettes (arr2/OXA-10/catB3) by pale blue boxes; the CS of integrons as orange boxes; and IS (e.g., IS3000) as white block arrows labelled with the IS number/name and the pointed end indicating IRR. Unit transposons are shown as boxes of different colors (e.g., Tn5393, green) and their IR are shown as flags, with the flat side at the outer boundary of the transposon. Truncated features (e.g., 3’-CS) are shown with a jagged edge on the truncated side(s). Direct repeats flanking ISs are shown as ‘lollipops’ of the same color ISKpn21 (green). Modules A, B, C and D are represented by yellow, blue, purple, and light green boxes. Modules N1, N2 and N3 comprising blaNDM-1 are represented by red boxes.
pBU19801_NDM and pBA3783_NDM shared a common set of eight ARGs (Table 2) including truncated-aac(6′)-Ib-cr and sul1 (Module C); armA, msrE, and mphE (Module B), and aph(3″)-Ib (or strA), aph(6)-Id (or strB; Module D) along with Module N3 (Figure 6). pBL12125/456 had the same AMR profiles for both blaNDM-1-(10 ARGs) and blaESBL-(11 ARGs) plasmids. pBL12125/456_NDM also contained blaCMY-16-like downstream of module B which was not present in pBL849_NDM. Conversely, pBL849_NDM also contained an additional AMR gene, aph(3″)-VIb (=aph6) upstream of Module N1 which was not present in pBL12125_NDM and pBL12456_NDM (Table 2; Figure 6).
Module A was shared by pBL849_NDM, pBL12125/456_NDM (n = 3) while module B was shared by pBL849_NDM, pBL12125/456_NDM, pBU19801_NDM, and pBA3783_NDM (n = 5). Modules C and D were shared by pBU19801_NDM and pBA3783_NDM (n = 2). Module N0 was shared by all five K. pneumoniae NDM-1 producers including modifications for pBL849_NDM (Module N1) pBL12125_NDM and pBL12456_NDM (Module N2), and pBU19801_NDM and pBA3783_NDM (Module N3; Figure 6).
OXA-48-producers (n = 5): BA3783, BL13802, BA2664, BL8800, and BA2880
All OXA-48 producers shared the same blaOXA-48-plasmid, which does not carry additional ARGs (Figure 7). All five blaOXA-48-plasmids were positive for IncL. BLAST analyses of the plasmid sequence revealed high similarity (99.99%) between the current 62-kb blaOXA-48-plasmid and pRJ-119-2, first blaOXA-48-positive plasmid isolated in China in 2016 (Figure 6). Tn1999, also named Tn1999.1, consists of two copies of the insertion sequence IS1999 surrounding blaOXA-48: one copy inserted 26 bp upstream of blaOXA-48 and another copy downstream of blaOXA-48. In our sequences, the insertion of IS1R into IS1999 upstream of blaOXA-48 indicates the presence of a Tn1999.2 variant (Giani et al., 2012; Figure 7).
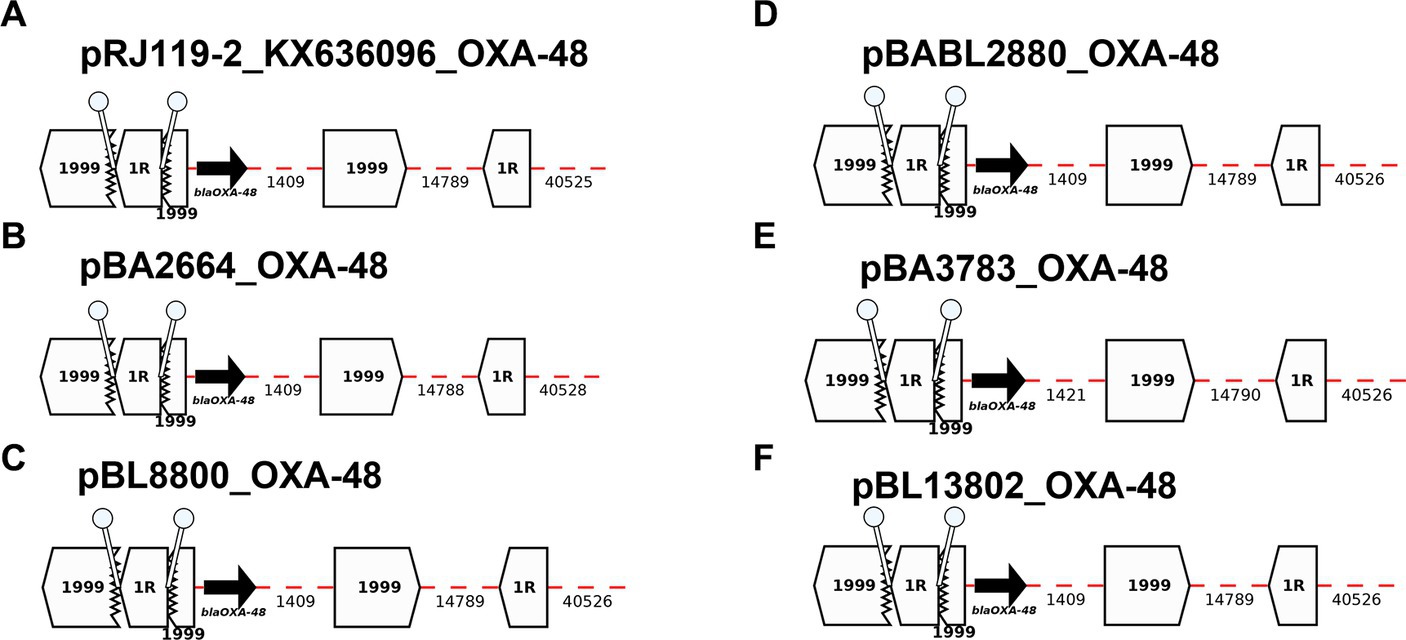
Figure 7. Annotation Diagrams of OXA-48-plasmids (A) pRJ119-1-OXA-48_KX636096, (B) pBA2664-NDM, (C) pBL8800_OXA-48, (D) pBABL2880_OXA-48, (E) pBA3783-OXA-48, and (F) pBL13802_OXA-48 using Galileo AMR platform. Gaps >50 bp are indicated by dashed red lines and the length in bp is given. Genes features (i.e., blaOXA-48) are shown by arrows, and IS (e.g., IS1999, IS1) as white block arrows labelled with the IS number/name and the pointed end indicating IRR. Truncated features (e.g., IS1999) are shown with a jagged edge on the truncated side(s). Direct repeats flanking ISs are shown as ‘lollipops’ of the same color IS1R (white). Here, the blaOXA-48 module found in all isolates are the same and identical to the module identified in pRJ119-2_KX636096.
ESBL-plasmids: BL849, BU19801, MS84, BL12125, BL12456, BA3783, BL13802, BL8800, and BA2880
Among the 10 blaNDM-1 and/or blaOXA-48-positive K. pneumoniae isolates, eight blaESBL-plasmids were characterized by hybrid sequencing. K. pneumoniae isolates pMS84 and pBA2664 did not carry blaESBL-plasmids. Among the eight blaESBL-plasmids, IncFIB was the most common incompatibility group identified with 100% identity (n = 2) followed by IncFII (n = 1). As far as incompatibility groups with an identity >96%, the IncFIB/IncFII combination was the most common (n = 3), followed by IncFII (n = 1) and FIA (n = 1; Table 1).
Five blaESBL-plasmids identified in this study were highly comparable to some regions of previously described blaESBL-plasmids. However, none of the prior blaESBL-plasmids shared the exact same sequence structure with the blaESBL-plasmids described herein. Also, 3/8 blaESBL-plasmids shared identity in a region that did not contain ARGs: BU19801_pESBL (99.97%, a 180-kb region of K. pneumoniae E16KP0258 chromosome, Genbank accession number: CP052272), BL8800/BABL2880_pESBL (99.98%, a 75-kb region of K. pneumoniae69 p69-1, Genbank accession number: CP025457; data not shown).
pBL849_ESBL (89 kb) was highly similar to some sections of the p4_1_2.2 plasmid from K. pneumoniae strain 4/1-2 (99.98%, 96 kb, Genbank accession number: CP023841), isolated in Sweden in 2018 including evidence of rearrangements between regions. pBL12125_ESBL and pBL12456_ESBL (127 kb) were both comparable at 99.95% to part of pG747, a 151 kb plasmid isolated from the K. pneumoniae strain G747 in Maryland (USA) in 2018 (Genbank accession number: CP034137). pBA3783_ESBL (135 kb) was highly comparable (99.97%) to part of the p2K157 plasmid (157 kb) isolated from the K. pneumoniae strain KP69 (Genbank accession number: CP054291). pBL13802_ESBL (189 kb) was almost identical (99.99%) to part of a 180-kb plasmid that was present in a K. pneumoniae strain named K. pneumoniae_Goe_588–1 (Genbank accession number: CP018693), which was isolated in Germany in 2016. Also, pBL12125/456_ESBL, pBU19801_ESBL and pBL849_ESBL shared a common set of 7 ARGs including aph(3″)-Ib (or strA), aph(6)-Id (or strB), blaTEM-1B, blaCTX-M-15, blaOXA-1, and aac(6′)-Ib-cr (data not shown).
Discussion
Understanding the evolution and spread of MDR bacteria is essential to countering the serious global threat posed by these organisms. The presence of ARGs on mobile genetic elements, strongly increases the risk of transferring resistance between different bacterial genera, including among those established in hospital environments. The development of novel sequencing methods and user-friendly web-based tools that fully characterize plasmids found in MDR pathogens is crucial for detecting and tracking the spread of AMR determinants across communities and preventing outbreaks.
Here, we characterized the plasmid structure in a collection of blaNDM-1 and blaOXA-48-harboring MDR K. pneumoniae isolated in Karachi, Pakistan using a hybrid NGS approach combining Nanopore long-read sequencing data with Illumina short-reads data. We also isolated blaNDM-1-plasmids of interest via conjugation to study correlation between plasmid-targeted AMR profiles and their associated susceptibility profiles. The genotypic AMR profiles of the E. coli transconjugants harboring only blaNDM-1-plasmids highlights the impact of blaNDM-1 and ARGs present on that same plasmid and co-transferred.
Several different types of plasmids associated with the Enterobacteriaceae have been reported to harbor blaNDM, including IncA/C, IncFII subtypes, IncH types, IncL/M, IncN and IncX (Ho et al., 2012; Partridge and Iredell, 2012; Carattoli, 2013). The variety of incompatibility groups and pMLST identified in our plasmids collection (IncC (types 1 and 3), IncFIA (type 26) IncFIB, IncFII (types K1, K2, K7, K9), IncHI1B, and IncL) are consistent with this observation.
All seven blaNDM-1-plasmids identified in our study were unique, however plasmids from isolates BL12125/BL12456 harbored the same IncC3 plasmid. As discussed previously (Lomonaco et al., 2018), BL12125 and BL12456 were identified as related strains ST14 sharing the same AMR profile, the same AST phenotype, and the same plasmid incompatibility group and plasmid type (IncC3). In both cases, blaNDM-1 is surrounded by a duplication of a set of ARGs (blaOXA-10, aadA1, and sul1 including two copies of ISCR1) suggesting insertion events. A common IncL blaOXA-48-plasmid was also found to be shared by isolates BL8800/BA2880. Long read sequencing confirmed that the plasmids identified in BL12125 and BL12456 are identical. Additionally, BL8800 and BA2880 were found to carry the same blaESBL-plasmid (IncFIIK9; 6 ARGs) and IncL blaOXA-48-plasmid, suggesting again that these 2 pairs of isolates are equivalent but obtained from different sites of infection in a single patient as previously reported by Lomonaco et al. (2018).
The blaNDM-1-plasmids isolated from BU19801 (ST307; IncFIIK2) and BA3783 (ST14, IncFIIK2) were 100% identical except for the insertion of a 746-bp truncated ISEc15 region on pBA3783_NDM, suggesting that the genetic rearrangements did not impact AMR profiles. However, both isolates BU19801 and BA3783 do share the same plasmid incompatibility group and type (IncFIIK2), AMR profiles, and AST phenotypes which strongly suggests that those two K. pneumoniae isolates may have previously shared the exact same plasmid.
Long-read technology has significantly enhanced the usefulness of NGS data to accurately predict AMR profiles, detect the presence of similar plasmids in different isolates, and identify genetic rearrangement events. For instance, pBL849 [IncFIB(pNDM-Mar)/IncHI1B(pNDM-MAR), pBL12125/456 (IncC3)] shared 3 AMR modules that lead to the same AMR profiles but carried both different non-AMR regions and incompatibility groups. This observation suggests that their respective plasmids are different.
pMS84_NDM and pBL13802_NDM were similar to two blaNDM-1-plasmids described previously. pMS84_NDM, which was IncC1-positive, shared 100% identity with an IncA/C2 blaNDM-1-plasmid (GenBank accession number: C050164) isolated from a K. pneumoniae strain from Hong-Kong in 2020. The ISEc23 element found in pMS84_NDM suggests that their respective plasmids are related. pBL13802_NDM, which was IncFII(pKPX1)/type 1-positive, shared 99.98% identity with the pKPX-1 plasmid identified from a K. pneumoniae clinical isolate obtained from a rectal swab in Taiwan in 2016 (Huang et al., 2013). BL13802_pNDM and pKPX-1, shared a ~130-kb region comprising a stretch of AMR determinants, but a 121-kb region neighboring blaNDM-1, and filled with a succession of IS elements, was missing from pBL13802_NDM. The KPX strain originated from a Taiwanese patient with a hospitalization history in New Delhi (Huang et al., 2013), which may suggest that those two plasmids are also related.
The blaOXA-48-plasmids (IncL) identified in this study were identical to the pRJ119-2 plasmid reported in 2020 as the first blaOXA-48-plasmid in China (Genbank accession number: KX636096). Finally, while some large AMR regions have been reported, all blaESBL-plasmids identified in this study have not been previously described. The AMR features were observed in multiple rearrangements which underscores the complexity of molecular background and importance of mobility of ARGs via horizontal transfer.
All blaNDM-1-positive E. coli transconjugants were resistant to most β-lactams, and all aminoglycosides while these transconjugants were susceptible to CIP, SXT, TET, and CHL. All E. coli transconjugants were susceptible to ATM as expected for NDM producers except for Ec_BL12125/456 due to the presence of blaOXA-10 on the blaNDM-1-plasmid. All blaOXA-48-positive E. coli transconjugants were resistant to ampicillin, β-lactams/β-lactamase inhibitor combinations (AMC, TZP, and SAM), but susceptible to fluoroquinolones, other β-lactams and aminoglycosides.
Overall, the NGS/RF-phenotypes-based approach performed well in comparison with BMD for E. coli transconjugants except for a few instances: CRO, ATM, and CHL. The potential hydrolysis of CRO (MIC >32 μg/ml) by the NDM enzyme was apparently not accounted for by ResFinder. The OXA-48 enzyme, which is not a particularly efficient carbapenemase often referred to “phantom menace” or “hidden threat” (Poirel et al., 2012; Bakthavatchalam et al., 2016) is difficult to detect and often missed by routine diagnostics was detected by ResFinder both genotypically and phenotypically. Both K. pneumoniae isolates harboring the cmlA5 gene (BL12125/456) were classified as susceptible by ResFinder and BMD. In both study isolates, two important components of the class 1 integron described by Revathi et al. (2013) were altered: (1) truncated integrase (intI1) and (2) absence of the Pc promoter for the cmlA5 gene cassette. This finding suggests that cmlA5 may not be expressed in this integron. Lastly, all E. coli transconjugants carrying qnrB/S or aac(6′)-Ib-cr were susceptible to fluoroquinolones (CIP), which implies that the high-level fluoroquinolone resistance phenotypes observed in our previous study (Lomonaco et al., 2018) are most likely due to chromosomal mutations on genes encoding DNA gyrase and topoisomerases IV, respectively.
It has been well-established that genetic-based screening approaches informed by high-quality sequencing data have potential to be useful to rapidly detect the most common and well-described mechanisms of resistance which correlate with phenotypes for the major classes of antimicrobials. However, the current bioinformatics landscape lacks a standardized and well-curated database with expert-defined rules for AMR gene inclusion/exclusion (e.g., truncated genes). Moreover, our findings reiterate that the presence/detection of ARGs does not necessarily yield a resistant phenotype, as observed for qnrB1, aac(6′)-Ib-cr and cmlA5.
In this study, bacterial conjugation provided additional information on phenotypes of targeted plasmids but one of the limitations is that plasmids over ~200 kb may be difficult to transfer (e.g., BL249, 277 kb). This study did not aim to investigate the transfer of plasmids between isolates, since this collection did not include all carbapenemase-positive Enterobacteriaceae samples from the same period. However, this study highlights how comprehensive characterization of AMR plasmids may offer insights to rapidly respond to outbreaks and help early implementation of effective infection controls procedures.
The use of ONT/Illumina sequencing is crucial for accurate and complete plasmid characterization in research laboratories, but ONT sequencing could also provide preliminary results within healthcare institutions to inform and fortify infection control measures while waiting for AST results that would confirm expression of the ARGs detected by NGS. Nanopore offers a specific workflow for plasmids that has the potential to identify pathogens, AMR determinants, and plasmid classification and/or structural features within minutes (Tamma et al., 2019; Neal-McKinney et al., 2021).
Further comprehensive plasmid analysis including a hybrid approach can be performed for epidemiologic purposes. Real-time sequencing approaches could enable MDR plasmid characterization and analysis as new isolates are collected. To maximize impact, the data could be used to build a curated AMR-based plasmid database, that combines AMR profiles and metadata. Overall, such a resource would provide scientists and clinicians a powerful infection control surveillance system that tracks plasmid-based AMR transmission in real time.
This study highlights the complexity and diversity of molecular background of pathogens that produce carbapenemases along with ESBL or AmpC ß-lactamases, which are challenging for clinicians. The spread of plasmid-mediated AMR determinants between pathogens emerging as MDR bacteria is a major threat worldwide, and rapid tracking of such pathogens is warranted. This study emphasizes the value of rapid, real-time sequencing of AMR plasmids to predict NGS-based AST profiles, and to provide insights on their dissemination especially during outbreaks.
Data availability statement
The datasets presented in this study can be found in online repositories. The names of the repository/repositories and accession number(s) can be found in the article/Supplementary material.
Author contributions
CL, MC, MH, EK, KA, DH, SP, and DS contributed to conception and design of the study. CL, BC, DF, and MC performed all lab experiments. AC and LR performed the bioinformatics data analysis. CL wrote the first draft of the manuscript. All authors contributed to the article and approved the submitted version.
Funding
This project was supported by the Department of Homeland Security through an interagency agreement HSHQPM-14-X-00116 with the Centers for Disease Control and Prevention. MC and MH received additional support from the U.S. National Institutes of Health grants R21 AI139947 and R01 AI150941. The findings and conclusions in this report are those of the authors and do not necessarily represent the views or official positions of the Department of Health and Human Services, Centers for Disease Control and Prevention, U.S. Food and Drug Administration and the Department of Homeland Security. The use of trade names is for identification only and does not imply endorsement.
Acknowledgments
The authors thank Linda Weigel for spearheading this project with external CDC collaborators, and Sara Lomonaco for her valuable scientific input for this project. A special thank you to David Lonsway and his team from the Division of Healthcare Quality Promotion at the Centers for Disease Control and Prevention for providing the antibiotic panels for BMD. We also thank Tom de Man for his appreciated assistance in bioinformatics.
Conflict of interest
The authors declare that the research was conducted in the absence of any commercial or financial relationships that could be construed as a potential conflict of interest.
Publisher’s note
All claims expressed in this article are solely those of the authors and do not necessarily represent those of their affiliated organizations, or those of the publisher, the editors and the reviewers. Any product that may be evaluated in this article, or claim that may be made by its manufacturer, is not guaranteed or endorsed by the publisher.
Supplementary material
The Supplementary material for this article can be found online at: https://www.frontiersin.org/articles/10.3389/fmicb.2023.1192097/full#supplementary-material
Footnotes
References
Bakthavatchalam, Y. D., Anandan, S., and Veeraraghavan, B. (2016). Laboratory detection and clinical implication of oxacillinase-48 like Carbapenemase: the hidden threat. J. Global Infect. Dis. 8, 41–50. doi: 10.4103/0974-777X.176149
Bankevich, A., Nurk, S., Antipov, D., Gurevich, A. A., Dvorkin, M., Kulikov, A. S., et al. (2012). SPAdes: a new genome assembly algorithm and its applications to single-cell sequencing. J. Comput. Biol. 19, 455–477. doi: 10.1089/cmb.2012.0021
Bortolaia, V., Kaas, R. S., Ruppe, E., Roberts, M. C., Schwarz, S., Cattoir, V., et al. (2020). ResFinder 4.0 for predictions of phenotypes from genotypes. J. Antimicrob. Chemother. 75, 3491–3500. doi: 10.1093/jac/dkaa345
Brown, S. D., Dreolini, L., Wilson, J. F., Balasundaram, M., and Holt, R. A. (2023). Complete sequence verification of plasmid DNA using the Oxford Nanopore Technologies' MinION device. BMC Bioinform. 24:116. doi: 10.1186/s12859-023-05226-y
Carattoli, A. (2013). Plasmids and the spread of resistance. Int. J. Med. Microbiol. 303, 298–304. doi: 10.1016/j.ijmm.2013.02.001
Carattoli, A., and Hasman, H. (2020). PlasmidFinder and in Silico pMLST: identification and typing of plasmid replicons in whole-genome sequencing (WGS). Methods Mol. Biol. 2075, 285–294. doi: 10.1007/978-1-4939-9877-7_20
Castanheira, M., Deshpande, L. M., Mendes, R. E., Canton, R., Sader, H. S., and Jones, R. N. (2019). Variations in the occurrence of resistance phenotypes and Carbapenemase genes among Enterobacteriaceae isolates in 20 years of the SENTRY antimicrobial surveillance program. Open Forum Infect. Dis. 6, S23–S33. doi: 10.1093/ofid/ofy347
CLSI (2018). Methods for dilution antimicrobial susceptibility tests for Bacteria that grow aerobically; Approved Standards—11th Edition. CLSI standard M07, Wayne, PA. Clinical and Laboratory Standards Institute.
CLSI (2023). Performance standards for antimicrobial susceptibility testing, 33rd Edition. CLSI Supplement M100, Clinical and Laboratory Standards Institute.
de Man, T. J. B., Lutgring, J. D., Lonsway, D. R., Anderson, K. F., Kiehlbauch, J. A., Chen, L., et al. (2018). Genomic analysis of a Pan-resistant isolate of Klebsiella pneumoniae, United States 2016. MBio 9, 1–7. doi: 10.1128/mBio.00440-18
Dong, D., Li, M., Liu, Z., Feng, J., Jia, N., Zhao, H., et al. (2019). Characterization of a NDM-1- encoding plasmid pHFK418-NDM from a clinical Proteus mirabilis isolate harboring two novel transposons, Tn6624 and Tn6625. Front. Microbiol. 10:2030. doi: 10.3389/fmicb.2019.02030
EUCAST (2023). Breakpoint tables for interpretation of MICs and zone diameters, version 13. Available at: http://www.eucast.org/clinical_breakpoints/ (Accessed January 02, 2023).
Giani, T., Conte, V., di Pilato, V., Aschbacher, R., Weber, C., Larcher, C., et al. (2012). Escherichia coli from Italy producing OXA-48 carbapenemase encoded by a novel Tn1999 transposon derivative. Antimicrob. Agents Chemother. 56, 2211–2213. doi: 10.1128/AAC.00035-12
Hadjadj, L., Cassir, N., Saïdani, N., Hoffman, C., Brouqui, P., Astoul, P., et al. (2022). Outbreak of carbapenem-resistant enterobacteria in a thoracic-oncology unit through clonal and plasmid-mediated transmission of the bla (OXA-48) gene in southern France. Front. Cell. Infect. Microbiol. 12:1048516. doi: 10.3389/fcimb.2022.1048516
Heikema, A. P., Jansen, R., Hiltemann, S. D., Hays, J. P., and Stubbs, A. P. (2021). WeFaceNano: a user-friendly pipeline for complete ONT sequence assembly and detection of antibiotic resistance in multi-plasmid bacterial isolates. BMC Microbiol. 21:171. doi: 10.1186/s12866-021-02225-y
Hendriksen, R. S., Bortolaia, V., Tate, H., Tyson, G. H., Aarestrup, F. M., and McDermott, P. F. (2019). Using genomics to track global antimicrobial resistance. Front. Public Health 7:242. doi: 10.3389/fpubh.2019.00242
Ho, P. L., Li, Z., Lo, W. U., Cheung, Y. Y., Lin, C. H., Sham, P. C., et al. (2012). Identification and characterization of a novel incompatibility group X3 plasmid carrying bla NDM-1 in Enterobacteriaceae isolates with epidemiological links to multiple geographical areas in China. Emerg. Microbes Infect. 1:e39. doi: 10.1038/emi.2012.37
Huang, T. W., Chen, T. L., Chen, Y. T., Lauderdale, T. L., Liao, T. L., Lee, Y. T., et al. (2013). Copy number change of the NDM-1 sequence in a multidrug-resistant Klebsiella pneumoniae clinical isolate. PLoS One 8:e62774. doi: 10.1371/journal.pone.0062774
Kolmogorov, M., Yuan, J., Lin, Y., and Pevzner, P. A. (2019). Assembly of long, error-prone reads using repeat graphs. Nat. Biotechnol. 37, 540–546. doi: 10.1038/s41587-019-0072-8
Koren, S., Walenz, B. P., Berlin, K., Miller, J. R., Bergman, N. H., and Phillippy, A. M. (2017). Canu: scalable and accurate long-read assembly via adaptive k-mer weighting and repeat separation. Genome Res. 27, 722–736. doi: 10.1101/gr.215087.116
Lascols, C., Podglajen, I., Verdet, C., Gautier, V., Gutmann, L., Soussy, C. J., et al. (2008). A plasmid-borne Shewanella algae gene, qnrA3, and its possible transfer in vivo between Kluyvera ascorbata and Klebsiella pneumoniae. J. Bacteriol. 190, 5217–5223. doi: 10.1128/JB.00243-08
Li, H. (2018). Minimap2: pairwise alignment for nucleotide sequences. Bioinformatics 34, 3094–3100. doi: 10.1093/bioinformatics/bty191
Lomonaco, S., Crawford, M. A., Lascols, C., Timme, R. E., Anderson, K., Hodge, D. R., et al. (2018). Resistome of carbapenem- and colistin-resistant Klebsiella pneumoniae clinical isolates. PLoS One 13:e0198526. doi: 10.1371/journal.pone.0198526
Mączyńska, B., Frej-Mądrzak, M., Sarowska, J., Woronowicz, K., Choroszy-Król, I., and Jama-Kmiecik, A. (2023). Evolution of antibiotic resistance in Escherichia coli and Klebsiella pneumoniae clinical isolates in a multi-profile hospital over 5 years (2017-2021). J. Clin. Med. 12, 1–7. doi: 10.3390/jcm12062414
Neal-McKinney, J. M., Liu, K. C., Lock, C. M., Wu, W. H., and Hu, J. (2021). Comparison of MiSeq, MinION, and hybrid genome sequencing for analysis of Campylobacter jejuni. Sci. Rep. 11:5676. doi: 10.1038/s41598-021-84956-6
Neuert, S., Nair, S., Day, M. R., Doumith, M., Ashton, P. M., Mellor, K. C., et al. (2018). Prediction of phenotypic antimicrobial resistance profiles from whole genome sequences of non-typhoidal Salmonella enterica. Front. Microbiol. 9:592. doi: 10.3389/fmicb.2018.00592
Partridge, S. R., and Iredell, J. R. (2012). Genetic contexts of blaNDM-1. Antimicrob. Agents Chemother. 56, 6065–6067. doi: 10.1128/AAC.00117-12
Partridge, S. R., and Tsafnat, G. (2018). Automated annotation of mobile antibiotic resistance in gram-negative bacteria: the multiple antibiotic resistance annotator (MARA) and database. J. Antimicrob. Chemother. 73, 883–890. doi: 10.1093/jac/dkx513
Poirel, L., Bonnin, R. A., Boulanger, A., Schrenzel, J., Kaase, M., and Nordmann, P. (2012). Tn125-related acquisition of blaNDM-like genes in Acinetobacter baumannii. Antimicrob. Agents Chemother. 56, 1087–1089. doi: 10.1128/AAC.05620-11
Poirel, L., Dortet, L., Bernabeu, S., and Nordmann, P. (2011). Genetic features of blaNDM-1-positive Enterobacteriaceae. Antimicrob. Agents Chemother. 55, 5403–5407. doi: 10.1128/AAC.00585-11
Poirel, L., Potron, A., and Nordmann, P. (2012). OXA-48-like carbapenemases: the phantom menace. J. Antimicrob. Chemother. 67, 1597–1606. doi: 10.1093/jac/dks121
Ramirez, M. S., and Tolmasky, M. E. (2010). Aminoglycoside modifying enzymes. Drug Resist. Updat. 13, 151–171. doi: 10.1016/j.drup.2010.08.003
Revathi, G., Siu, L. K., Lu, P. L., and Huang, L. Y. (2013). First report of NDM-1-producing Acinetobacter baumannii in East Africa. Int. J. Infect. Dis. 17, e1255–e1258. doi: 10.1016/j.ijid.2013.07.016
Ruan, J., and Li, H. (2020). Fast and accurate long-read assembly with wtdbg2. Nat. Methods 17, 155–158. doi: 10.1038/s41592-019-0669-3
Tamma, P. D., Fan, Y., Bergman, Y., Pertea, G., Kazmi, A. Q., Lewis, S., et al. (2019). Applying rapid whole-genome sequencing to predict phenotypic antimicrobial susceptibility testing results among Carbapenem-resistant Klebsiella pneumoniae clinical isolates. Antimicrob. Agents Chemother. 63, 1–12. doi: 10.1128/AAC.01923-18
Valcek, A., Nesporova, K., Whiteway, C., de Pooter, T., de Coster, W., Strazisar, M., et al. (2022). Genomic analysis of a strain collection containing multidrug-, extensively drug-, Pandrug-, and Carbapenem-resistant modern clinical isolates of Acinetobacter baumannii. Antimicrob. Agents Chemother. 66:e0089222. doi: 10.1128/aac.00892-22
Walker, B. J., Abeel, T., Shea, T., Priest, M., Abouelliel, A., Sakthikumar, S., et al. (2014). Pilon: an integrated tool for comprehensive microbial variant detection and genome assembly improvement. PLoS One 9:e112963. doi: 10.1371/journal.pone.0112963
Yang, M., Xu, G., Ruan, Z., and Wang, Y. (2022). Genomic characterization of a multidrug-resistant Escherichia coli isolate co-carrying Bla (NDM-5) and Bla (CTX-M-14) genes recovered from a pediatric patient in China. Infect. Drug Resist. 15, 6405–6412. doi: 10.2147/IDR.S388797
Keywords: Klebsiella pneumoniae , AMR determinants, MDR plasmids, nanopore hybrid assemblies, AMR data analysis
Citation: Lascols C, Cherney B, Conley AB, Rishishwar L, Crawford MA, Morse SA, Fisher DJ, Anderson K, Hodge DR, Pillai SP, Hughes MA, Khan E and Sue D (2023) Investigation of multidrug-resistant plasmids from carbapenemase-producing Klebsiella pneumoniae clinical isolates from Pakistan. Front. Microbiol. 14:1192097. doi: 10.3389/fmicb.2023.1192097
Edited by:
Isabel Henriques, University of Coimbra, PortugalReviewed by:
João Pedro Rueda Furlan, University of São Paulo, BrazilNadezhda Fursova, State Research Center for Applied Microbiology and Biotechnology, Russia
Copyright © 2023 Lascols, Cherney, Conley, Rishishwar, Crawford, Morse, Fisher, Anderson, Hodge, Pillai, Hughes, Khan and Sue. This is an open-access article distributed under the terms of the Creative Commons Attribution License (CC BY). The use, distribution or reproduction in other forums is permitted, provided the original author(s) and the copyright owner(s) are credited and that the original publication in this journal is cited, in accordance with accepted academic practice. No use, distribution or reproduction is permitted which does not comply with these terms.
*Correspondence: Christine Lascols, Y2xhc2NvbHNAY2RjLmdvdg==