- 1Department of Laboratory Medicine, Shanghai University of Medicine and Health Sciences Affiliated Zhoupu Hospital, Shanghai, China
- 2Center for Clinical and Translational Medicine, Shanghai University of Medicine and Health Sciences, Shanghai, China
The gut microbiota varies dramatically among individuals, and changes over time within the same individual, due to diversities in genetic backgrounds, diet, nutrient supplementations and use of antibiotics. Up until now, studies on dysbiosis of microbiota have expanded to a wider range of diseases, with Akkermansia muciniphila at the cross spot of many of these diseases. A. muciniphila is a Gram-negative bacterium that produces short-chain fatty acids (SCFAs), and Amuc_1100 is one of its most highly expressed outer membrane proteins. This review aims to summarize current knowledge on correlations between A. muciniphila and involved neuropsychological diseases published in the last decade, with a focus on the potential of this bacterium and its outer membrane proteins as therapeutic targets for these diseases, on the basis of evidence accumulated from animal and clinical studies, as well as mechanisms of action from peripheral to central nervous system (CNS).
Introduction
The human gut hosts around 1013–1014 microorganisms from different species that compete, suppress and collaborate with each other to achieve delicate functions. During past decades, the knowledge on gut microbiota expanded from being merely a colonizer to being a keeper of a stable microenvironment. Importantly, these highly diverse intestinal microorganisms participated not only in maintenance of a healthy gut, but also in pathogenesis and progression of many diseases where dysbiosis occurs, via various mechanisms (Mosca et al., 2016; Hughes et al., 2018; Chen et al., 2019; Laniewski et al., 2020; Wang et al., 2021; Geng et al., 2022). Though each disease presents a unique disturbance of gut microbiota, some species appear to be involved across multiple diseases, and therefore have become targets of interest, as they may either provide an insight to a common pathogenic pathway (Makdissi et al., 2023), or hold the potential to be used as a marker or target for diagnosis or treatment of these diseases (Mamic et al., 2023). The Gram-negative anaerobe Akkermansia muciniphila, which belongs to Verrucomicrobia phylum, is one of such species that has been shown to be dysregulated in an increasing number of diseases such as metabolic disorders (Xu et al., 2020) and intestinal diseases (Liu et al., 2022).
The best-studied strain of A. muciniphila, MucT (ATCC BAA-835), was first isolated from human feces in 2004 (Derrien et al., 2004). The bacterium colonizes at the mucosal interface between the oxic intestinal epithelium and the anoxic lumen throughout the human gut (Ouwerkerk et al., 2016), and constitutes up to 5% of total bacteria in human gastrointestinal tract under basal conditions (Bellenger et al., 2019). The 16S rRNA gene sequencing has revealed that A. muciniphila colonizes early in life, develops within 1 year to a level close to that in adults, and decreases with aging (Collado et al., 2007). Since the discovery of this bacterium, numerous studies have shown its favorable role in metabolism (Plovier et al., 2017; Depommier et al., 2019; Rao et al., 2021; Daniel et al., 2023) and anti-inflammation (Grander et al., 2018; Hanninen et al., 2018; Ansaldo et al., 2019). Specifically, A. muciniphila has been consistently shown to be present with a relatively higher abundance in clinically healthy and lean individuals, compared with overweight or obese individuals (Derrien et al., 2017), with a higher abundance being correlated with a higher level of metagenome diversity (Le Chatelier et al., 2013). Importantly, A. muciniphila tends to co-exist with bacteria typically associated with a healthy human gut, such as F. prausnitzii, Gordonibacter spp. and Methanobrevibacter smithii (Dao et al., 2016), but not with those associated with unhealthy gut such as Prevotella spp. (Arumugam et al., 2011). Therefore, A. muciniphila has been proposed as a next-generation probiotic (Zhai et al., 2019). In fact, the direct administration of live (Depommier et al., 2019) or pasteurized bacterium grown on a synthetic medium (Plovier et al., 2017) were proved safe in humans. Notably, the pasteurized A. muciniphila has been approved as novel food by EFSA (Efsa Panel on Nutrition, N. F et al., 2021). A. muciniphila was detected elevated in abundance in athletes gut microbiome (Petersen et al., 2017), and could be upregulated by probiotics supplementation (Huang et al., 2020). However, contradictory results with a lower abundance of A. muciniphila were found in the rugby players and cyclists compared to controls who were not sedentary but exercised normally (Hintikka et al., 2022).
Though mainly studied in metabolic and gastrointestinal disorders previously, analysis of this species has recently been extended to neuropsychological diseases. Most of these studies have revealed a change in its relative abundance in both patients and disease models, which brings to the following questions: (i) whether the bacterium plays a unique role in pathogenesis of diseases; (ii) whether it serves as a biomarker to predict trends towards certain diseases and/or disease progression; (iii) whether modulation of this species, its outer membrane proteins or its metabolites would potentially be beneficial in the long-term management of these diseases. To answer these questions, this narrative review summarizes the correlations between A. muciniphila and neuropsychological diseases by reviewing the major findings from human and animal studies published in the last decade. Additionally, the potential therapeutic values and modulating strategies for A. muciniphila and underlying mechanisms are also discussed.
Akkermansia muciniphila and gut barrier
The adhesion study has revealed a more significant binding of A. muciniphila to human extracellular matrix protein laminin compared with its binding to bovine serum albumin (BSA; Reunanen et al., 2015). The adhesion level of A. muciniphila to intestinal epithelium and mucus was as low as <1% (Reunanen et al., 2015), and its absence of mucus-binding domain showcased in a comprehensive analysis of its genome was consistent to its mucinolytic nature (van Passel et al., 2011). Moreover, A. muciniphila was shown to bind to human enterocytes at a comparable level to the positive-control, and its binding site was expressed on epithelial cell surface at all differentiation stages (Reunanen et al., 2015). Therefore, A. muciniphila may participate in the competitive exclusion of pathogenic microorganism from sites of damage.
A. muciniphila plays an essential role in maintaining gut barrier and mucus layer integrity (Figure 1). On one hand, it facilitates mucus production (Collado et al., 2007). Thus, the protective effects from accumulation of this bacterium could be associated with thickness of the mucus layer, reduction of gut permeability, and prevention from leakage of bacterial lipopolysaccharide (LPS) into the bloodstream (Everard et al., 2013; Zhai et al., 2019). On the other hand, a thin mucus layer, often seen in diseases like inflammatory bowel disease (IBD), is considered harmful by exposing the gut mucosa to microbial antigens and leading to a more permeable barrier for endogenous proteins and endotoxin. Notably, a low abundance of A. muciniphila in the gut is often detected under these pathological conditions (Zhang et al., 2020).
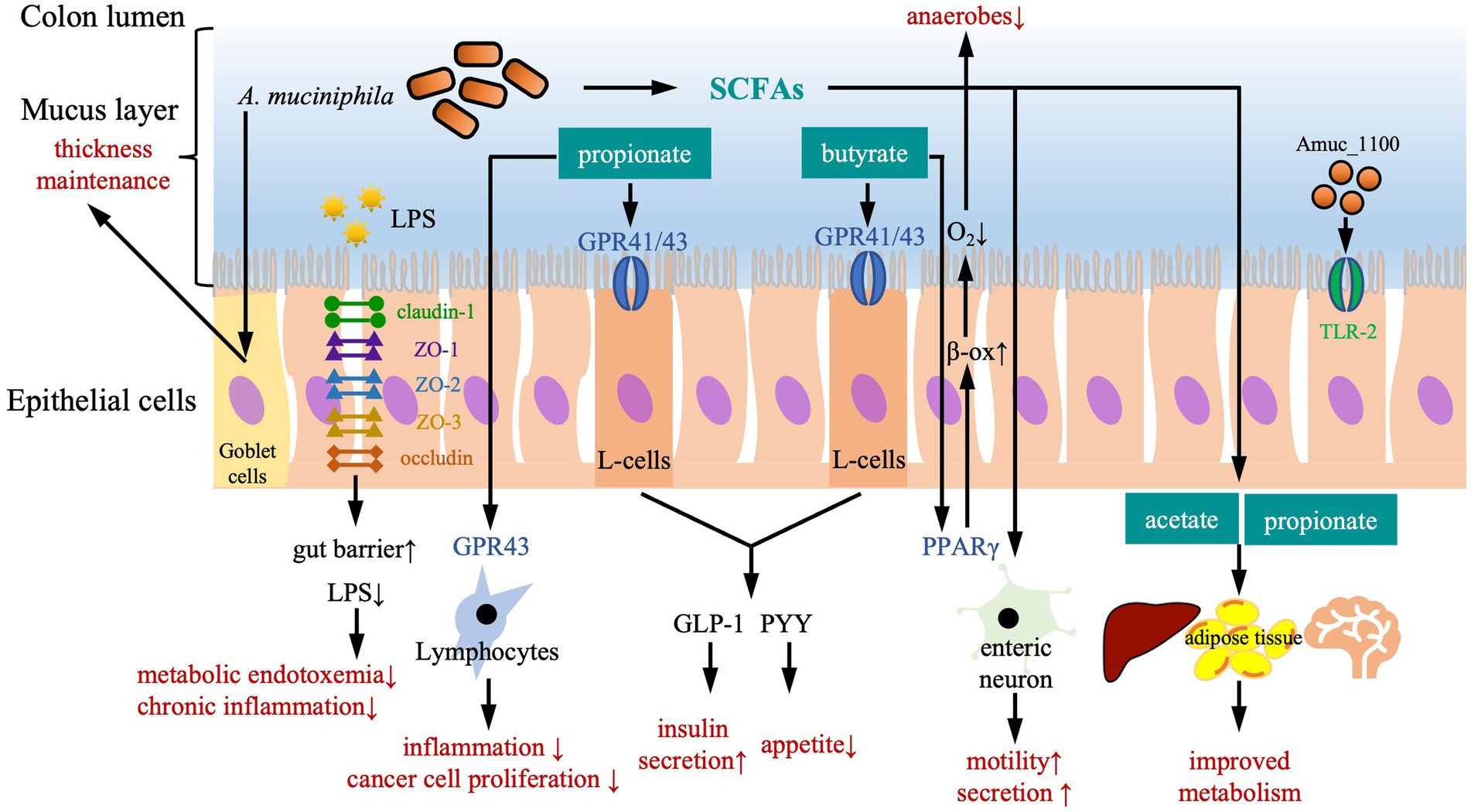
Figure 1. The protective effects of Akkermansia muciniphila against diseases. Biochemically, A. muciniphila exerts its protective function in a SCFA-dependent manner. Both butyrate and propionate bind G protein coupled receptor (GPR)-43 and GPR-41 that are expressed on the enteroendocrine L-cells, subsequently trigger secretion of gut peptides such as glucagon-like peptide-1 (GLP-1) and peptide YY (PYY), leading to a reduction of food intake and an improvement of glucose metabolism. Propionate also acts as an immune regulator by binding to GPR-43, which is expressed on lymphocytes to reduce inflammation and maintain an appropriate immune defense, as well as to reduce proliferation of cancer cells. Butyrate activates peroxisome proliferator-activated receptor-γ (PPAR-γ), leading to beta-oxidation and oxygen consumption, and maintains anaerobic condition in the gut lumen. In addition, SCFAs also trigger secretory activity and motility in intestine via stimulating enteric neuron signaling. Effects on remote organs are modulated by acetate and propionate in the circulation, leading to an improved metabolism of liver, adipose tissue and brain. Physically, A. muciniphila enhances the gut barrier to defend intrusion of harmful particles and pathogens in several ways. Firstly, A. muciniphil upregulates expression of tight-junction proteins including claudin-1, zonula occluden (ZO)-1, ZO-2, ZO-3, and occludin, improving gut barrier and reducing gut permeability for LPS. Secondly, the pili-like membrane protein (Amuc_1100) from A. muciniphila is also involved in maintaining host immunological homeostasis by activating toll-like receptor 2 (TLR-2) and improving gut barrier function. Thirdly, A. muciniphila acts on Goblet cells to stimulate the secretion of mucus and thereby maintain the thickness of mucus layer.
Akkermansia muciniphila as an SCFAs-producing bacterium
The short-chain fatty acids (SCFAs) are generated from fermentation of dietary fibers by intestinal bacteria and are mainly composed of butyrate, propionate, and acetate. A. muciniphila mainly produces propionate and acetate, while butyrate is produced by its neighbouring symbionts (Ottman et al., 2017a). SCFAs have several beneficial effects including maintaining epithelial barrier function, diminishing oxidative DNA damage, regulating cytokine production, promoting anti-inflammation, and stimulating immune functions (Maslowski and Mackay, 2011) and metabolic health (Muller et al., 2019). The majority of butyrate is utilized in the gut, which has been shown to increase energy expenditure by promoting fat oxidation and activating brown adipose tissue (Roshanravan et al., 2017), as well as to increase A. muciniphila levels (Arora et al., 2019). Meanwhile, a large amount of acetate and a small proportion of propionate reach the circulation and improve the metabolism of liver, adipose tissue and brain (Gomes et al., 2018). Notably, A. muciniphila failed to produce propionate in the absence of vitamin B12, which is a cofactor for converting succinate to propionate via methylmalonyl-CoA synthase (Karcher et al., 2021).
As one of the key SCFAs-producing bacteria, A. muciniphila has been hypothesized to act as a protective player against a variety of central and peripheral diseases in a SCFAs-dependent manner (Cani, 2018; Caesar, 2019). The administration of this species may lead to functional symbiosis, which results in a status rich of the SCFAs. For instance, the oral administration of an A. muciniphila subtype, which produces SCFAs including acetic acid, propionic acid and isovaleric acid, has been shown to exert beneficial effects against body weight gain, hyperglycosemia and cognitive impairment in HFD-treated mice (Wu et al., 2020).
Akkermansia muciniphila and neurovascular integrity
Under pathological conditions, a variety of inflammatory stimuli, such as cytokines, LPS, amyloid β (Aβ) and tau fragments may initiate an inflammatory response in endothelial cells of the blood brain barrier (BBB), which activates a variety of signaling pathways and consequently results in neurovascular abnormalities, destruction of the BBB and activation of peripheral paracrine cells (Montagne et al., 2017). Accumulating evidence has shown a critical role of gut microbiota in regulation of neurovascular integrity. For instance, in germ-free mice, the lack of butyric acid due to deficiency of SCFAs-producing flora has led to a significant increase in BBB permeability (Braniste et al., 2014). As one of the key SCFAs-producing bacteria, the lack of A. muciniphila caused a damage to the tight junction (TJ) of the BBB and the intestinal barrier (Li et al., 2016). On the contrary, the upregulation of A. muciniphila following ketogenic diets (KD) in mice could lead to an increase in cerebral blood flow and transport of P-glycoprotein through the BBB to facilitate the clearance of Aβ (Ma et al., 2018). Therefore, interventions modulating A. muciniphila at the early stage of cognitive impairment may reduce the risk of developing Alzheimer’s disease (AD) by improving neurovascular functions.
The outer membrane protein
Amuc_1100, which is a 32 kDa pili-like protein composed of four ɑ helixes and a four-strand antiparallel β fold, is one of the most expressed outer membrane proteins of A. muciniphila (Ottman et al., 2017a). It remains stable at different temperatures and after pasteurization (Plovier et al., 2017), playing a key role in maintaining host immunological homeostasis and improving gut barrier function (Plovier et al., 2017; Ottman et al., 2017a). Specifically, the interaction between Amuc_1100 and mucin is required for the colonization of the bacterium (Shin et al., 2019). Actually, A. muciniphila could regulate the expression of TJ proteins (Occludin, zonula occluden (ZO-1) and Claudin-1) via activation of 5’AMP-activated protein kinase (AMPK) pathway by A. muciniphila-derived extracellular vesicles (AmEVs) as well as activation of Toll-like receptor 2 (TLR2) by Amuc_1100 (Ottman et al., 2017b; Chelakkot et al., 2018; Ashrafian et al., 2019). Amuc_1100 has been reported to interact with TLR2, directly and subsequently promote the expression of serotonin (5-HT) synthesis rate-limiting enzyme tryptophan hydroxylase 1 (Tph1) in Rin-14B cells and reduced the expression of the serotonin reuptake transporter (SERT) in Caco-2 cells, thereby improve the biosynthesis and extracellular availability of 5-HT (Wang et al., 2021). Recent studies in murine models have shown that the protective effects of A. muciniphila against diet-induced obesity was partially due to the interaction between Amuc_1100 and TLR2/TLR4 (Plovier et al., 2017), subsequently facilitating the production of cytokines [e.g., interleukin (IL)-6, IL-8, IL-10] (Ottman et al., 2017b) and upregulating TJ proteins (Plovier et al., 2017; Ashrafian et al., 2019). Recent studies have also suggested that Amuc_1100 may play an important role in regulating host amino acid metabolism. In a mouse model of colitis, Amuc_1100 exerted beneficial effects by reducing infiltrating macrophages and CD8+ cytotoxic T lymphocytes in the colon, and by enhancing indoleacetic acid (IAA) and indoleacrylic acid (IA) levels in the microbial tryptophan (Trp) metabolic pathway to activate aryl hydrocarbon receptor (AhR) signaling (Wang et al., 2020). In addition, Amuc_1100 could also inhibit kynurenine (Kyn) pathway by up-regulating Kyn level, down-regulating 2-picolinic acid (PIC) level, and affecting PIC/Kyn ratio (Gu et al., 2021).
Akkermansia muciniphila in neuropsychological diseases
Raised since about a decade ago, the concept of ‘microbiota-gut-brain axis’, which provides bidirectional communication pathways between the gut and the brain (Cryan and O'Mahony, 2011; Sherwin et al., 2018), has linked dysbiosis of intestinal microbiota to pathogenesis of diseases of the central nervous system (CNS). This brings new insights into the understanding of disease mechanisms, and the development of biomarkers and potential treatments of these debilitating diseases. Overall, the abundance of A. muciniphila was shown to decrease significantly in amyotrophic lateral sclerosis (ALS; Blacher et al., 2019) and neuropsychiatric disorders (NPDs; McGaughey et al., 2019; Song et al., 2019; Aatsinki et al., 2020; Park et al., 2020; Cheng et al., 2021; Ding et al., 2021), increase significantly in Multiple System Atrophy (MSA; Derrien et al., 2011; Wan et al., 2019), Multiple Sclerosis (MS; Cantarel et al., 2015; Jangi et al., 2016; Berer et al., 2017; Cekanaviciute et al., 2017, 2018; Tankou et al., 2018; Al-Ghezi et al., 2019; Probstel et al., 2020) and Parkinson’s disease (PD; Braak et al., 2006; Holmqvist et al., 2014; Keshavarzian et al., 2015; Remely et al., 2015; Sampson et al., 2016; Unger et al., 2016; Bedarf et al., 2017; Hill-Burns et al., 2017; Heintz-Buschart et al., 2018; Gorecki et al., 2019; Hertel et al., 2019; Li et al., 2019; Cirstea et al., 2020; Dodiya et al., 2020; Nishiwaki et al., 2020; Pietrucci et al., 2020; Vidal-Martinez et al., 2020; Hou et al., 2021; Jeon et al., 2021; Augustin et al., 2023), but remains ambiguous in Alzheimer’s disease (AD) and cognitive deficits (Harach et al., 2017; Vogt et al., 2017; Ma et al., 2018; Nagpal et al., 2019; Yang et al., 2019; Chen et al., 2020), autism spectrum disorder (ASD; Wang et al., 2011; De Angelis et al., 2013; Kang et al., 2013; Inoue et al., 2016; Lee et al., 2017; Strati et al., 2017; Sharon et al., 2019; Zou et al., 2020; Zurita et al., 2020) and epilepsy (Olson et al., 2018; Huang et al., 2019; Figure 2).
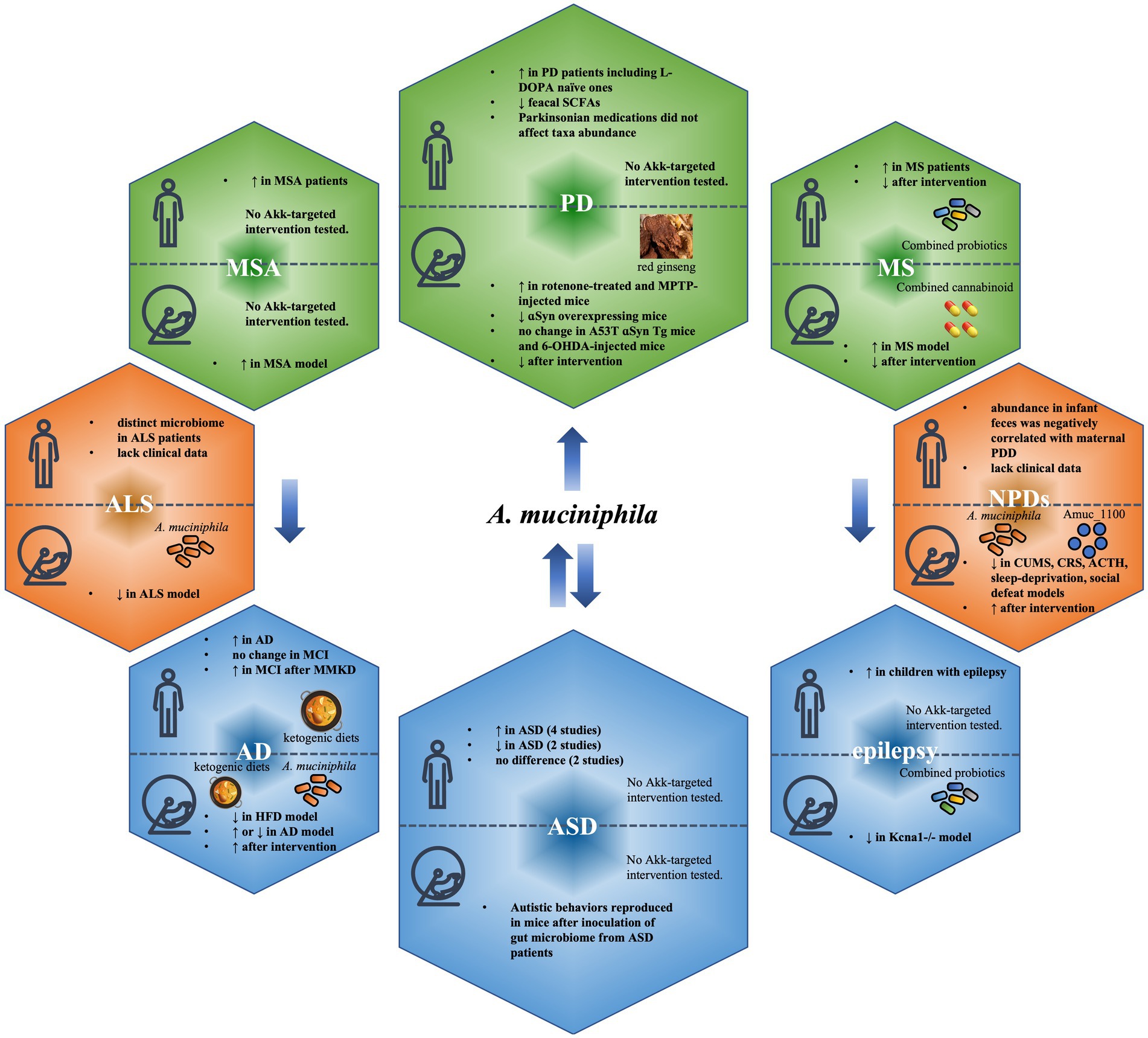
Figure 2. Change of Akkermansia muciniphila abundance in neuropsychological diseases and attempts of modulation of A. muciniphila. Overall, the abundance of A. muciniphila was shown to decrease (red boxes) significantly in amyotrophic lateral sclerosis (ALS) and neuropsychiatric disorders (NPDs), increase (green boxes) significantly in multiple system atrophy (MSA), multiple sclerosis (MS) and Parkinson’s disease (PD), but remains ambiguous (blue boxes) in Alzheimer’s disease (AD) and cognitive deficits, autism spectrum disorder (ASD) and epilepsy.
Amyotrophic lateral sclerosis
A. muciniphila is negatively associated with ALS, which is indicated by a gradually decreased abundance of A. muciniphila in ALS mice (Blacher et al., 2019). The same research group identified a distinct microbiome and metabolite configurations in a small cohort study comparing ALS patients with household controls (Blacher et al., 2019). However, larger scaled investigations are required to confirm such alterations in microbiome and the effectiveness of Akk-targeted interventions.
Neuropsychiatric disorders
A decreased abundance of Akkermansia has been observed in multiple depressive-like animal models (Table 1), including (i) chronic unpredictable mild stress (CUMS)-induced or chronic restraint stress (CRS)-induced mouse models (Cheng et al., 2021; Ding et al., 2021); (ii) a chronic adrenocorticotrophic hormone (ACTH)-induced depression rat model (Song et al., 2019); (iii) a water-stress-induced sleep-deprived mouse model (Park et al., 2020); (iv) a mouse model exhibiting depressive- and anxiety-like behavior following social defeat (McGaughey et al., 2019). The abundance of A. muciniphila was negatively correlated with anxiety- and depressive-like behaviors, which were indicated by sucrose preference test and open-field test (McGaughey et al., 2019).
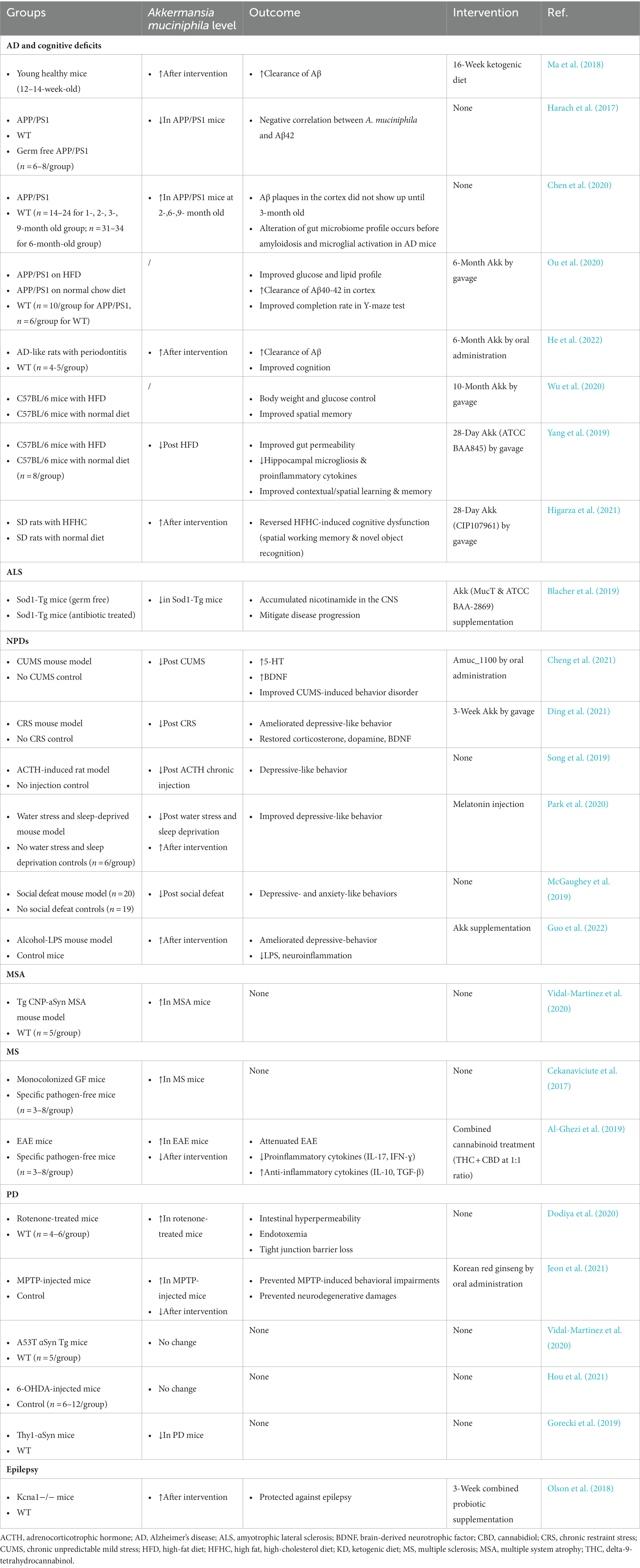
Table 1. Association between Akkermansia muciniphila and representative neurological diseases in disease models.
In human studies, the abundance of A. muciniphila in the fecal samples of 2.5 month-old infants was found negatively correlated with the symptoms of maternal prenatal psychological distress (PPD) in the FinnBrain Birth cohort study involving 398 mothers and their infants, suggesting that chronic PPD may affect the relative abundance of the species in the offsprings, which might subsequently contribute to changes in development outcomes (Aatsinki et al., 2020).
Multiple system atrophy
MSA is a sporadic, adult-onset, progressive neurodegenerative disease characterized by combinations of parkinsonian features, cerebellar ataxia, autonomic failure and pyramidal features. By metagenomic sequencing in feces of 15 MSA patients and 15 healthy controls, it showed an increase in genus Akkermansia and a decrease in genera Megamonas, Bifildobacterium, Blauta and Agregatibacter (Wan et al., 2019). The mechanisms behind an elevated level of Akkermansia in MSA remained unclear. Although Akkermansia was reported to be capable of upregulating genes involved in antigen presentation pathways, B and T cell receptor signaling, IL-4 signaling, as well as complement and coagulation cascades, it was suggested to be in pursuit of immune tolerance toward the bacteria and metabolic homeostasis of the colonized intestine (Derrien et al., 2011). Therefore, the higher abundance of this species in neurodegenerative diseases is possibly due to the tentative of the body to restore immune homeostasis, which is required to be further investigated.
Multiple sclerosis
MS is an autoimmune disease of the CNS characterized by demyelination, axonal damage and progressive neurologic disability. A. muciniphila abundance was positively associated with MS by increasing IFNɣ+ Th1 lymphocyte differentiation in vitro, indicating the crucial role of this bacterium in the pathogenesis of MS mediated via pro-inflammatory responses (Cekanaviciute et al., 2017). A. muciniphila has been shown in multiple case-controlled, cross-sectional and observational studies to increase in abundance in association with induction of pro-inflammatory responses in MS patients, compared with healthy controls (Jangi et al., 2016; Berer et al., 2017; Cekanaviciute et al., 2017, 2018; Tankou et al., 2018; Al-Ghezi et al., 2019; Probstel et al., 2020), whereas downregulation of A. muciniphila in MS has induced an anti-inflammatory immune response in the peripheral immune system, providing a potential target for MS treatment (Tankou et al., 2018; Table 2). Notably, A. muciniphila was positively associated with MS mixed cohorts consisted of untreated and under-therapy patients during disease remission, and the association between A. muciniphila and MS remained significant after correction for therapy (Jangi et al., 2016). In a recently published large-scaled clinical research (iMSMS Consortium. Electronic address: sergio.baranzini@ucsf.edu; iMSMS Consortium, 2022) on 576 MS patients and their household healthy controls, it was demonstrated a total of 16 species including A. muciniphila significantly elevated in untreated MS patients. Additionally, in the same study, A. muciniphila was also found to be significantly more represented in untreated relapsing–remitting and progressive MS patients, and but showed no difference in groups with disease modifying therapies. When transferring intestinal microbiota from MS twins to a spontaneous brain autoimmunity mouse model via fecal transplantation, microbiota from MS patients induced a significantly higher incidence of autoimmunity compared to that from their healthy co-twins (Berer et al., 2017). Unlike diabetes, disease-modifying therapies for MS have not altered the abundance of this species, compared to untreated patients (Jangi et al., 2016). In clinical practice, MS patients are often treated with glatiramer acetate (GA) and supplemented with vitamin D. Intriguingly, in a small pilot study, researchers have detected a higher enrichment of A. muciniphila in untreated MS patients after vitamin D supplementation, compared to GA-treated subjects and healthy controls (Cantarel et al., 2015), which may be indicative to the decision-making of therapeutic strategies for MS. By looking at immune transcriptional profiles in T cells and monocytes from MS patients, A. muciniphila was positively correlated with several genes from T-cells (including CASP1, TRAF5 and STAT5B) and with genes from monocytes (including MAPK14, MAPK1, LTBR, STAT5B, CASP1 and HLA-DRB1; Jangi et al., 2016).
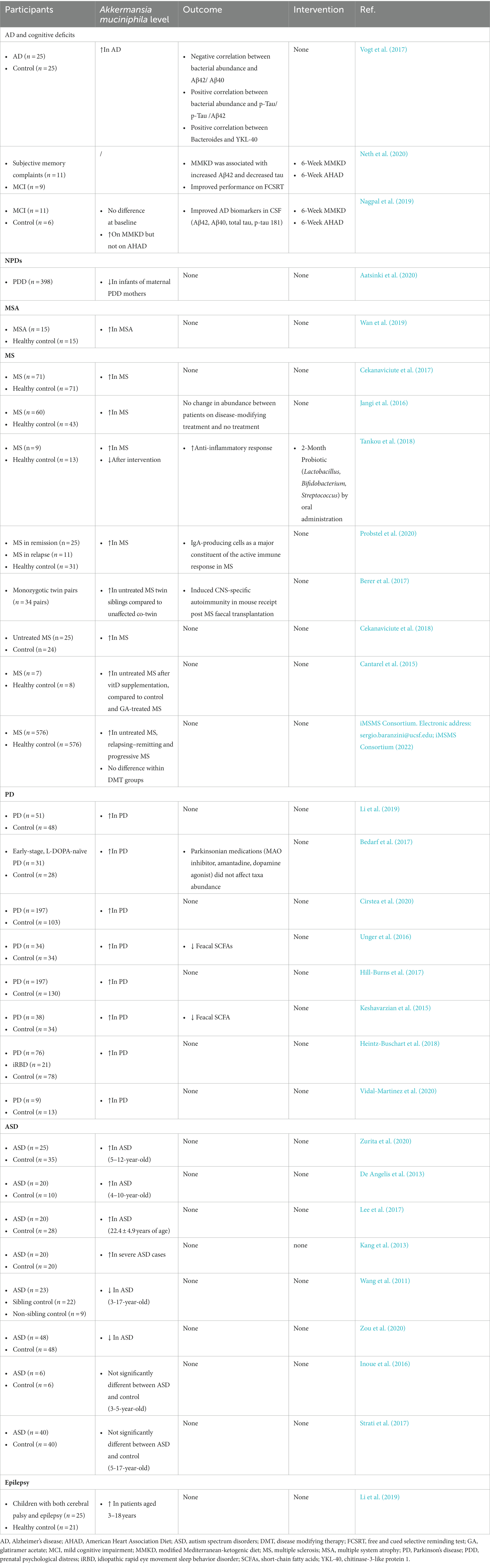
Table 2. Association between Akkermansia muciniphila and representative neuropsychological diseases in humans.
Parkinson’s disease
Postmortem studies have proposed that ɑ-synuclein inclusions, the pathological feature of PD, may be transported from the gut to the brain via the vagus nerve (Braak et al., 2006; Holmqvist et al., 2014), raising the hypothesis that PD might begin in the gut. Since then, a growing number of studies have shown alterations in diversity and richness of gut microbiota in PD patients that may trigger ɑ-synuclein misfolding or disturb function of the enteric nervous system. A. muciniphila has been consistently found to be increased in abundance in the guts of PD patients when compared with healthy controls (Keshavarzian et al., 2015; Unger et al., 2016; Bedarf et al., 2017; Hill-Burns et al., 2017; Li et al., 2019; Cirstea et al., 2020; Nishiwaki et al., 2020; Vidal-Martinez et al., 2020), even in those at early disease stages including L-DOPA naïve cohorts (Bedarf et al., 2017), which is thought to be due to a change of feeding behaviors including caloric restriction and fasting (Remely et al., 2015; Table 2). In addition, confounding factors such as chronic constipation and drug treatments may not be excluded when interpretating the observation of increased A. muciniphila abundance in faeces of PD patients. Notably, the metabolic products SCFAs have been consistently detected to be depleted in PD despite of the increased SCFAs-producing taxa A. muciniphila, which may be caused by other flora such as Lachnospiraceae and Faecalibacterium prausnitzii (Keshavarzian et al., 2015; Sampson et al., 2016; Unger et al., 2016). The longer colonic transit time of PD may be related to the deficiency of fecal SCFAs (Augustin et al., 2023). In addition, A. muciniphila has been found to be significantly more abundant in PD patients with rapid eye movement sleep behavior disorder (RBD), compared with PD patients without RBD, and correlates with nonmotor symptoms (Heintz-Buschart et al., 2018). This is particularly important, as nonmotor symptoms may appear years before the onset of motor symptoms in PD, and at present there are no reliable early biomarkers. This raises the possibility that change in the abundance or function of A. muciniphila may potentially serve as a suitable biomarker for the early diagnosis of PD. Longitudinal prospective studies of larger cohorts of PD patients at various stages of disease may be of value. For instance, by analyzing longitudinal data from PD patients, A. muciniphila has been proposed to participate in PD pathogenesis by producing hydrogen sulfide, which is a pro-inflammatory molecule harmful to the integrity of the mucus layer (Hertel et al., 2019). In a study that used three machine learning algorithms to analyze metagenomic results from 472 PD patients and 374 healthy controls, A. muciniphila was identified as one of the most effective bacterium among the 22 bacterial families in distinguishing between predicted PD patients and controls (Pietrucci et al., 2020). Additional studies are needed to assess the effects of anti-PD medications on the abundance of gut microbiota including A. muciniphila, as no significant difference in taxa abundance has been detected following administration of parkinsonian medications, including MAO inhibitors, amantadine, and dopamine agonists (Bedarf et al., 2017). Meanwhile, the potential of gut microbiota in modifying the efficacy and/or toxicity of PD medications required to be further investigated as well.
In animal studies, the elevated abundance of A. muciniphila has only been reported in a rotenone-treated (Dodiya et al., 2020) and in an MPTP-induced mouse models (Jeon et al., 2021), which could be associated with hyper-permeability of intestine and pro-inflammatory milieu for PD exacerbation (Dodiya et al., 2020). The elevated level of A. muciniphila and its related behavioral deficits and neurodegenerative damages could be suppressed by the treatment with Korean red ginseng (Jeon et al., 2021). However, the abundance of A. muciniphila did not increase in most mouse models of PD, including Parkinsonian A53T ɑ and MSA CNP-ɑSyn Tg mouse models (Vidal-Martinez et al., 2020), 6-OHDA-induced mouse model (Hou et al., 2021), and even decreased in a human ɑ-synuclein overexpressing mouse model (Gorecki et al., 2019).
Taking all observations together, a direct role of A. muciniphila and other bacteria has not yet been confirmed in PD.
Alzheimer’s disease and cognitive deficits
Ample evidence has shown that cognitive decline presented in patients with metabolic syndrome, and metabolic syndrome is regarded as one of the key risk factors for AD. Therefore, the prevention of metabolic syndrome may protect from developing AD. AD has been proposed as ‘type 3 diabetes’, and the anti-diabetic agents (e.g., dipeptidyl peptidase-4, also known as DPP4) has been proposed as novel therapies for AD (Cheng et al., 2020). Therefore, the correlation between A. muciniphila and cognitive decline was first studied in association with metabolic symptoms in rodents. For instance, high-fat diet (HFD)-fed mice has demonstrated cognitive impairments particularly in domains of contextual/spatial learning and memory, which was associated with depletion of A. muciniphila (Yang et al., 2019). However, a 16-week intervention with ketogenic diet could significantly increase the relative abundance of A. muciniphila, hence reducing the risk for neurodegeneration by improving metabolic profile in young healthy mice aged 12–14 weeks (Ma et al., 2018). In AD rodent models, a relatively lower abundance of A. muciniphila was found in the gut of APP/PS1 transgenic mice compared to wild-type controls, and a negative correlation between the flora and Aβ42 was observed (Harach et al., 2017). However, in another study, increased abundance of A. muciniphila was detected in 2-, 6-, 9-month-old APP/PS1 mice, prior to amyloidosis and microglial activation (Chen et al., 2020). Overall, beneficial effects have been observed in these mice when boosting A. muciniphila either by oral administration or dietary intervention (Table 1).
In human studies, A. muciniphila was shown to be more abundant in very mild-to-moderate AD patients compared with controls (Vogt et al., 2017), but at a similar level between individuals with mild cognitive impairment (MCI) and normal controls (Nagpal et al., 2019).
Autism spectrum disorder
The change in abundance of A. muciniphila in ASD patients remains unclear, with increased (De Angelis et al., 2013; Kang et al., 2013; Lee et al., 2017; Zurita et al., 2020) and decreased (Wang et al., 2011; Zou et al., 2020) levels demonstrated in different clinical trials, while no significant difference (Inoue et al., 2016; Strati et al., 2017) was observed in other human studies. However, small sample size has been one of the major limitations of these studies, therefore, the findings need to be confirmed in larger cohorts. Interestingly, when a gut microbiome from human donors with ASD was transplanted to germ free mice, the typical human autistic behaviors were sufficiently reproduced in mice, with an increased abundance of A. muciniphila and reduced abundances of Bacteroidetes and Parabacteroides observed in these inoculated mice (Sharon et al., 2019).
Epilepsy
Although the abundance of A. muciniphila in animal model of epilepsy is decreased (Olson et al., 2018), children with cerebral palsy and epilepsy have shown a higher abundance of compared to healthy controls (Huang et al., 2019). It was noteworthy that the abundance of A. muciniphila could be restored in mice by a ketogenic diet to exert protective effects against seizure (Olson et al., 2018), suggesting the potential of microbial modulation as a novel anti-seizure therapy.
Akkermansia muciniphila as a potential therapeutic target for neuropsychological diseases
Given that A. muciniphila not only exists as a marker indicating a change in a disease state, but also plays a part in disease mechanisms, modifying abundance of the species may be of potential value in the treatment of various diseases. Although diet (Remely et al., 2015, 2016), exercise (Louis et al., 2016; Munukka et al., 2018), supplementations of A. muciniphila (Plovier et al., 2017; Depommier et al., 2019) or other probiotic products (Pedret et al., 2019; Tenorio-Jimenez et al., 2019), medications [e.g., antidiabetics, vancomycin or PPARɣ agonist (Wang et al., 2018; Payahoo et al., 2019; Basolo et al., 2020)] and surgeries [e.g., Roux-en-Y gastric bypass surgery, sleeve gastrectomy or duodenal/jejunal bypass surgery (Cortez et al., 2018; Sanchez-Alcoholado et al., 2019)] have all been shown to change the abundance of this species, it remains unknown whether such an increase persists and benefits individuals with neuropsychological diseases. It is noteworthy that life interventions like diets or exercises may lead to complicated microbe-microbe interactions, which are thought to be mediated by a variety of molecular and physiological mechanisms (Smid and Lacroix, 2013). Therefore, additional studies are required to figure out (i) the best method(s) for modifying A. muciniphila abundance; (ii) whether such a modification could exist in a long-term and be translated into neurological benefits; (iii) the potential of A. muciniphila as a target for the treatment of neuropsychological diseases.
Since realization of the importance of this species, studies have shown that the well-practiced interventions, such as dietary management, caloric restriction, surgical procedures, supplementation of natural products, as well as medications, all lead to an increase of A. muciniphila and associated beneficial effects in patients with metabolic syndromes. In addition, some other strategies have also been testified to increase the abundance of A. muciniphila in order to keep fitness in humans. These include: (i) a higher consumption of folate (Gurwara et al., 2019); (ii) Islamic fasting (Ozkul et al., 2019); (iii) lifestyle intervention (Guevara-Cruz et al., 2019); and (iv) a higher consumption of natural yogurt (Gonzalez et al., 2019).
Supplementation of Akkermansia muciniphila
For the APP/PS1 transgenic mouse model, supplementation of A. muciniphila for 6 months alleviated cognitive deficits (such as spatial learning impairments and memory deficits) as indicated by improved Y-maze test completion rates and shortened test completion time, with a significant reduction in amyloid-beta (Aβ) 40–42 levels in the cerebral cortex and in fasting blood glucose and blood lipid levels (Ou et al., 2020). In another study using an Alzheimer’s disease (AD)-like rat model with periodontitis, it was demonstrated that oral administration of this species significantly upregulated the abundance of other short-chain fatty acids (SCFAs)- or neurotransmitter-producing microbiomes and downregulated the abundance of pathogenic bacteria, resulting in alleviated cognitive impairments is association with reduced deposition of Aβ in both cerebral cortex and specific brain regions (He et al., 2022). These benefits in cognitive improvements following oral supplementation of A. muciniphila (or its subtype) have also been shown in mouse models with obese-induced cognitive decline, as indicated by different assessment tools such as Y-maze test (Wu et al., 2020), contextual fear-conditioning and the Barnes circular maze test (Yang et al., 2019), and novel object recognition test and spatial working memory test (Higarza et al., 2021). The mechanisms involved include that the flora restored the expression of the GluA1 and GluA2 subunits, reversed microgliosis, suppressed proinflammatory cytokines, maintained neuronal development and long-term potentiation in the hippocampus of these high-fat-diet (HFD)-fed mice (Yang et al., 2019), and the flora improved the oxidative metabolic activity in the brain by restoring the activity of the mitochondrial enzyme cytochrome C oxidase, which indicated ATP production and brain energy demand (Higarza et al., 2021). Supplementation of A. muciniphila was also shown to ameliorate chronic restraint stress (CRS)-induced (Ding et al., 2021) and alcohol-related (Guo et al., 2022) depressive-like behavior in mice, via enhancing intestinal barrier and maintaining gut microbiota homeostasis. In addition, A. muciniphila exerts beneficial effects to these depressive-like models by reducing serum LPS and neuroinflammation, normalizing the expression of depression-related genes and increasing serotonin (5-HT) levels in hippocampus (Guo et al., 2022). However, direct evidence from clinical studies is necessary to explore the therapeutic potential of A. muciniphila in depression and anxiety. Different strains have shown similar benefits. A treatment with A. muciniphila (both MucT and ATCC BAA-2869) showed similar effects of mitigation of disease progression in a mouse model of amyotrophic lateral sclerosis (ALS) by effectively increasing the nicotinamide levels in the central nervous system (CNS; Blacher et al., 2019).
A combined probiotic supplementation with other beneficial flora was also tested in various disease models (Cancello et al., 2019; Grajeda-Iglesias et al., 2021; Lee et al., 2022). In a Kcnal−/− mouse model which mimicked the associations of human KCNA1 gene variants with epilepsy and episodic ataxia, A. muciniphila together with Parabacteroides (but not alone) protected these mice against epilepsy by decreasing systemic ɣ-glutamylated ketogenic amino acids, elevating hippocampal ɣ-aminobutyric acid (GABA)/glutamate ratios and suppressing the ɣ-glutamyl transpeptidase (GGT) activity in the feaces (Olson et al., 2018).
Supplementation of the outer membrane protein Amuc_1100
The oral administration of Amuc_1100 was reported to ameliorate chronic unpredictable mild stress (CUMS)-induced depressive-like behavior in mice, which was in association with the improvement of CUMS-triggered down-regulation of 5-hydroxytryptamine (5-HT) and brain-derived neurotrophic factor (BDNF) and up-regulation of inflammation in hippocampus (Cheng et al., 2021).
Ketogenic diets and nutrient supplementations
Ketogenic diets (KD), which mimic starvation, have been shown to exert protective effects against cognitive decline. For participants with subjective memory complaints and mild cognitive impairment (MCI), the relative abundance of A. muciniphila was increased following modified KD in association with modulated SCFAs and improved Alzheimer’s disease (AD) biomarkers in cerebrospinal fluid (CSF), along with decreased blood glucose levels, reduced body weight and prevented decline in cognitive functions (Nagpal et al., 2019; Neth et al., 2020).
The relative abundance of A. muciniphila was elevated in rats under chronic mild stress following treatments with both fish oil and olive oil, however, only fish oil treatment ameliorated depressive-like behavior (Tung et al., 2019).
Conclusion
With increasing knowledge of gut microbiota, dysbiosis of these bacteria has been recognized as a crucial player in disease mechanisms, with A. muciniphila as one of the overlapping bacteria involved in an increasing number of neuropsychological diseases. Although the exact mechanisms of the species are not fully understood, its change in abundance has been widely observed, showing a great potential as a biomarker at preclinical, early and progressed disease stages. It is worth considering whether the change of the bacterium is of predictive value. In neurological diseases, it is very intriguing that the abundance is down-regulated in ALS and NPDs, up-regulated in MSA, MS and PD, and inconsistent results have been observed in AD and cognitive deficits, ASD and epilepsy. It is strongly indicated that a reference point for change of this species may present to serve as a biomarker predicting disease progression from pre-clinical stage to clinical stage of one or more of these diseases, as well as to determine when interventions are required to be initiated. For instance, A. muciniphila holds potential as an early biomarker for the diagnosis of PD, with evidence that it is significantly related to non-motor symptoms in PD. However, due to limited studies performed, this concept needs to be confirmed with further research. In addition, a series of interventions have been proposed for A. muciniphila as a therapeutic target (Figure 2), suggesting that the species may also be used as an indicator for treatment response, as well as a target for therapeutic strategies. Though exciting potentials have been raised, cautions need to be taken. It remains unknown whether the abundance of the species vary considerably among individuals, and whether the level of abundance overlaps among different disease conditions. As A. muciniphila is sensitive to change in diet habit, exercise, nutrient supplements, medications and other medical treatments, determination of a baseline in normal cohorts remains a challenge, and requires larger-scaled studies. In addition, longitudinal studies are needed to confirm if the change of this species is transient or persistent, and whether it is suitable as a biomarker and therapeutic target.
Author contributions
FZ performed literature search, drafted the manuscript, and prepared the figures. DW initiated, supervised, edited and revised the manuscript and finalized the work for submission. All authors contributed to the article and approved the submitted version.
Funding
This work was funded by Important Weak Subject Construction Project of Shanghai Pudong New Area Health Commission (grant no. PWZbr2022-03).
Conflict of interest
The authors declare that the research was conducted in the absence of any commercial or financial relationships that could be construed as a potential conflict of interest.
Publisher’s note
All claims expressed in this article are solely those of the authors and do not necessarily represent those of their affiliated organizations, or those of the publisher, the editors and the reviewers. Any product that may be evaluated in this article, or claim that may be made by its manufacturer, is not guaranteed or endorsed by the publisher.
References
Aatsinki, A. K., Keskitalo, A., Laitinen, V., Munukka, E., Uusitupa, H. M., Lahti, L., et al. (2020). Maternal prenatal psychological distress and hair cortisol levels associate with infant fecal microbiota composition at 2.5 months of age. Psychoneuroendocrinology 119:104754. doi: 10.1016/j.psyneuen.2020.104754
Al-Ghezi, Z. Z., Busbee, P. B., Alghetaa, H., Nagarkatti, P. S., and Nagarkatti, M. (2019). Combination of cannabinoids, delta-9-tetrahydrocannabinol (THC) and cannabidiol (CBD), mitigates experimental autoimmune encephalomyelitis (EAE) by altering the gut microbiome. Brain Behav. Immun. 82, 25–35. doi: 10.1016/j.bbi.2019.07.028
Ansaldo, E., Slayden, L. C., Ching, K. L., Koch, M. A., Wolf, N. K., Plichta, D. R., et al. (2019). Akkermansia muciniphila induces intestinal adaptive immune responses during homeostasis. Science 364, 1179–1184. doi: 10.1126/science.aaw7479
Arora, T., Rudenko, O., Egerod, K. L., Husted, A. S., Kovatcheva-Datchary, P., Akrami, R., et al. (2019). Microbial fermentation of flaxseed fibers modulates the transcriptome of GPR41-expressing enteroendocrine cells and protects mice against diet-induced obesity. Am. J. Physiol. Endocrinol. Metab. 316, E453–E463. doi: 10.1152/ajpendo.00391.2018
Arumugam, M., Raes, J., Pelletier, E., Le Paslier, D., Yamada, T., Mende, D. R., et al. (2011). Enterotypes of the human gut microbiome. Nature 473, 174–180. doi: 10.1038/nature09944
Ashrafian, F., Shahriary, A., Behrouzi, A., Moradi, H. R., Keshavarz Azizi Raftar, S., Lari, A., et al. (2019). Akkermansia muciniphila-derived extracellular vesicles as a mucosal delivery vector for amelioration of obesity in mice. Front. Microbiol. 10:2155. doi: 10.3389/fmicb.2019.02155
Augustin, A., Guennec, A. L., Umamahesan, C., Kendler-Rhodes, A., Tucker, R. M., Chekmeneva, E., et al. (2023). Faecal metabolite deficit, gut inflammation and diet in Parkinson's disease: integrative analysis indicates inflammatory response syndrome. Clin. Transl. Med. 13:e1152. doi: 10.1002/ctm2.1152
Basolo, A., Hohenadel, M., Ang, Q. Y., Piaggi, P., Heinitz, S., Walter, M., et al. (2020). Effects of underfeeding and oral vancomycin on gut microbiome and nutrient absorption in humans. Nat. Med. 26, 589–598. doi: 10.1038/s41591-020-0801-z
Bedarf, J. R., Hildebrand, F., Coelho, L. P., Sunagawa, S., Bahram, M., Goeser, F., et al. (2017). Functional implications of microbial and viral gut metagenome changes in early stage L-DOPA-naive Parkinson's disease patients. Genome Med. 9:39. doi: 10.1186/s13073-017-0428-y
Bellenger, J., Bellenger, S., Escoula, Q., Bidu, C., and Narce, M. (2019). N-3 polyunsaturated fatty acids: an innovative strategy against obesity and related metabolic disorders, intestinal alteration and gut microbiota dysbiosis. Biochimie 159, 66–71. doi: 10.1016/j.biochi.2019.01.017
Berer, K., Gerdes, L. A., Cekanaviciute, E., Jia, X., Xiao, L., Xia, Z., et al. (2017). Gut microbiota from multiple sclerosis patients enables spontaneous autoimmune encephalomyelitis in mice. Proc. Natl. Acad. Sci. U. S. A. 114, 10719–10724. doi: 10.1073/pnas.1711233114
Blacher, E., Bashiardes, S., Shapiro, H., Rothschild, D., Mor, U., Dori-Bachash, M., et al. (2019). Potential roles of gut microbiome and metabolites in modulating ALS in mice. Nature 572, 474–480. doi: 10.1038/s41586-019-1443-5
Braak, H., de Vos, R. A., Bohl, J., and Del Tredici, K. (2006). Gastric alpha-synuclein immunoreactive inclusions in Meissner's and Auerbach's plexuses in cases staged for Parkinson's disease-related brain pathology. Neurosci. Lett. 396, 67–72. doi: 10.1016/j.neulet.2005.11.012
Braniste, V., Al-Asmakh, M., Kowal, C., Anuar, F., Abbaspour, A., Toth, M., et al. (2014). The gut microbiota influences blood-brain barrier permeability in mice. Sci. Transl. Med. 6:263ra158. doi: 10.1126/scitranslmed.3009759
Caesar, R. (2019). Pharmacologic and nonpharmacologic therapies for the gut microbiota in type 2 diabetes. Can. J. Diabetes 43, 224–231. doi: 10.1016/j.jcjd.2019.01.007
Cancello, R., Turroni, S., Rampelli, S., Cattaldo, S., Candela, M., Cattani, L., et al. (2019). Effect of short-term dietary intervention and probiotic mix supplementation on the gut microbiota of elderly obese women. Nutrients 11:3011. doi: 10.3390/nu11123011
Cani, P. D. (2018). Human gut microbiome: hopes, threats and promises. Gut 67, 1716–1725. doi: 10.1136/gutjnl-2018-316723
Cantarel, B. L., Waubant, E., Chehoud, C., Kuczynski, J., DeSantis, T. Z., Warrington, J., et al. (2015). Gut microbiota in multiple sclerosis: possible influence of immunomodulators. J. Investig. Med. 63, 729–734. doi: 10.1097/JIM.0000000000000192
Cekanaviciute, E., Probstel, A. K., Thomann, A., Runia, T. F., Casaccia, P., Katz Sand, I., et al. (2018). Multiple sclerosis-associated changes in the composition and immune functions of spore-forming Bacteria. mSystems 3:e00083-18. doi: 10.1128/mSystems.00083-18
Cekanaviciute, E., Yoo, B. B., Runia, T. F., Debelius, J. W., Singh, S., Nelson, C. A., et al. (2017). Gut bacteria from multiple sclerosis patients modulate human T cells and exacerbate symptoms in mouse models. Proc. Natl. Acad. Sci. U. S. A. 114, 10713–10718. doi: 10.1073/pnas.1711235114
Chelakkot, C., Choi, Y., Kim, D. K., Park, H. T., Ghim, J., Kwon, Y., et al. (2018). Akkermansia muciniphila-derived extracellular vesicles influence gut permeability through the regulation of tight junctions. Exp. Mol. Med. 50:e450. doi: 10.1038/emm.2017.282
Chen, Y. Y., Chen, D. Q., Chen, L., Liu, J. R., Vaziri, N. D., Guo, Y., et al. (2019). Microbiome-metabolome reveals the contribution of gut-kidney axis on kidney disease. J. Transl. Med. 17:5. doi: 10.1186/s12967-018-1756-4
Chen, Y., Fang, L., Chen, S., Zhou, H., Fan, Y., Lin, L., et al. (2020). Gut microbiome alterations precede cerebral amyloidosis and microglial pathology in a mouse model of Alzheimer's disease. Biomed. Res. Int. 2020:8456596. doi: 10.1155/2020/8456596
Cheng, Q., Cheng, J., Cordato, D., and Gao, J. (2020). Can dipeptidyl peptidase-4 inhibitors treat cognitive disorders? Pharmacol. Ther. 212:107559. doi: 10.1016/j.pharmthera.2020.107559
Cheng, R., Xu, W., Wang, J., Tang, Z., and Zhang, M. (2021). The outer membrane protein Amuc_1100 of Akkermansia muciniphila alleviates the depression-like behavior of depressed mice induced by chronic stress. Biochem. Biophys. Res. Commun. 566, 170–176. doi: 10.1016/j.bbrc.2021.06.018
Cirstea, M. S., Yu, A. C., Golz, E., Sundvick, K., Kliger, D., Radisavljevic, N., et al. (2020). Microbiota composition and metabolism are associated with gut function in Parkinson's disease. Mov. Disord. 35, 1208–1217. doi: 10.1002/mds.28052
Collado, M. C., Derrien, M., Isolauri, E., de Vos, W. M., and Salminen, S. (2007). Intestinal integrity and Akkermansia muciniphila, a mucin-degrading member of the intestinal microbiota present in infants, adults, and the elderly. Appl. Environ. Microbiol. 73, 7767–7770. doi: 10.1128/AEM.01477-07
Cortez, R. V., Petry, T., Caravatto, P., Pessoa, R., Sanabani, S. S., Martinez, M. B., et al. (2018). Shifts in intestinal microbiota after duodenal exclusion favor glycemic control and weight loss: a randomized controlled trial. Surg. Obes. Relat. Dis. 14, 1748–1754. doi: 10.1016/j.soard.2018.07.021
Cryan, J. F., and O'Mahony, S. M. (2011). The microbiome-gut-brain axis: from bowel to behavior. Neurogastroenterol. Motil. 23, 187–192. doi: 10.1111/j.1365-2982.2010.01664.x
Daniel, N., Gewirtz, A. T., and Chassaing, B. (2023). Akkermansia muciniphila counteracts the deleterious effects of dietary emulsifiers on microbiota and host metabolism. Gut 72, 906–917. doi: 10.1136/gutjnl-2021-326835
Dao, M. C., Everard, A., Aron-Wisnewsky, J., Sokolovska, N., Prifti, E., Verger, E. O., et al. (2016). Akkermansia muciniphila and improved metabolic health during a dietary intervention in obesity: relationship with gut microbiome richness and ecology. Gut 65, 426–436. doi: 10.1136/gutjnl-2014-308778
De Angelis, M., Piccolo, M., Vannini, L., Siragusa, S., De Giacomo, A., Serrazzanetti, D. I., et al. (2013). Fecal microbiota and metabolome of children with autism and pervasive developmental disorder not otherwise specified. PLoS One 8:e76993. doi: 10.1371/journal.pone.0076993
Depommier, C., Everard, A., Druart, C., Plovier, H., Van Hul, M., Vieira-Silva, S., et al. (2019). Supplementation with Akkermansia muciniphila in overweight and obese human volunteers: a proof-of-concept exploratory study. Nat. Med. 25, 1096–1103. doi: 10.1038/s41591-019-0495-2
Derrien, M., Belzer, C., and de Vos, W. M. (2017). Akkermansia muciniphila and its role in regulating host functions. Microb. Pathog. 106, 171–181. doi: 10.1016/j.micpath.2016.02.005
Derrien, M., Van Baarlen, P., Hooiveld, G., Norin, E., Muller, M., and de Vos, W. M. (2011). Modulation of mucosal immune response, tolerance, and proliferation in mice colonized by the Mucin-degrader Akkermansia muciniphila. Front. Microbiol. 2:166. doi: 10.3389/fmicb.2011.00166
Derrien, M., Vaughan, E. E., Plugge, C. M., and de Vos, W. M. (2004). Akkermansia muciniphila gen. Nov., sp. nov., a human intestinal mucin-degrading bacterium. Int. J. Syst. Evol. Microbiol. 54, 1469–1476. doi: 10.1099/ijs.0.02873-0
Ding, Y., Bu, F., Chen, T., Shi, G., Yuan, X., Feng, Z., et al. (2021). A next-generation probiotic: Akkermansia muciniphila ameliorates chronic stress-induced depressive-like behavior in mice by regulating gut microbiota and metabolites. Appl. Microbiol. Biotechnol. 105, 8411–8426. doi: 10.1007/s00253-021-11622-2
Dodiya, H. B., Forsyth, C. B., Voigt, R. M., Engen, P. A., Patel, J., Shaikh, M., et al. (2020). Chronic stress-induced gut dysfunction exacerbates Parkinson's disease phenotype and pathology in a rotenone-induced mouse model of Parkinson's disease. Neurobiol. Dis. 135:104352. doi: 10.1016/j.nbd.2018.12.012
Efsa Panel on Nutrition, N. FFood, A., Turck, D., Bohn, T., Castenmiller, J., De Henauw, S., et al. (2021). Safety of pasteurised Akkermansia muciniphila as a novel food pursuant to regulation (EU) 2015/2283. EFSA J. 19:e06780. doi: 10.2903/j.efsa.2021.6780
Everard, A., Belzer, C., Geurts, L., Ouwerkerk, J. P., Druart, C., Bindels, L. B., et al. (2013). Cross-talk between Akkermansia muciniphila and intestinal epithelium controls diet-induced obesity. Proc. Natl. Acad. Sci. U. S. A. 110, 9066–9071. doi: 10.1073/pnas.1219451110
Geng, J., Ni, Q., Sun, W., Li, L., and Feng, X. (2022). The links between gut microbiota and obesity and obesity related diseases. Biomed. Pharmacother. 147:112678. doi: 10.1016/j.biopha.2022.112678
Gomes, A. C., Hoffmann, C., and Mota, J. F. (2018). The human gut microbiota: metabolism and perspective in obesity. Gut Microbes 9, 308–325. doi: 10.1080/19490976.2018.1465157
Gonzalez, S., Fernandez-Navarro, T., Arboleya, S., de Los Reyes-Gavilan, C. G., Salazar, N., and Gueimonde, M. (2019). Fermented dairy foods: impact on intestinal microbiota and health-linked biomarkers. Front. Microbiol. 10:1046. doi: 10.3389/fmicb.2019.01046
Gorecki, A. M., Preskey, L., Bakeberg, M. C., Kenna, J. E., Gildenhuys, C., MacDougall, G., et al. (2019). Altered gut microbiome in Parkinson's disease and the influence of lipopolysaccharide in a human alpha-Synuclein over-expressing mouse model. Front. Neurosci. 13:839. doi: 10.3389/fnins.2019.00839
Grajeda-Iglesias, C., Durand, S., Daillere, R., Iribarren, K., Lemaitre, F., Derosa, L., et al. (2021). Oral administration of Akkermansia muciniphila elevates systemic antiaging and anticancer metabolites. Aging (Albany NY) 13, 6375–6405. doi: 10.18632/aging.202739
Grander, C., Adolph, T. E., Wieser, V., Lowe, P., Wrzosek, L., Gyongyosi, B., et al. (2018). Recovery of ethanol-induced Akkermansia muciniphila depletion ameliorates alcoholic liver disease. Gut 67, 891–901. doi: 10.1136/gutjnl-2016-313432
Gu, Z., Pei, W., Shen, Y., Wang, L., Zhu, J., Zhang, Y., et al. (2021). Akkermansia muciniphila and its outer protein Amuc_1100 regulates tryptophan metabolism in colitis. Food Funct. 12, 10184–10195. doi: 10.1039/d1fo02172a
Guevara-Cruz, M., Flores-Lopez, A. G., Aguilar-Lopez, M., Sanchez-Tapia, M., Medina-Vera, I., Diaz, D., et al. (2019). Improvement of lipoprotein profile and metabolic Endotoxemia by a lifestyle intervention that modifies the gut microbiota in subjects with metabolic syndrome. J. Am. Heart Assoc. 8:e012401. doi: 10.1161/JAHA.119.012401
Guo, D., Park, C., Li, Y., Li, B., Yang, Q., Deng, Y., et al. (2022). Akkermansia muciniphila ameliorates depressive disorders in a murine alcohol-LPS (mALPS) model. Food Funct. 13, 12766–12776. doi: 10.1039/d2fo01478e
Gurwara, S., Ajami, N. J., Jang, A., Hessel, F. C., Chen, L., Plew, S., et al. (2019). Dietary nutrients involved in one-carbon metabolism and colonic mucosa-associated gut microbiome in individuals with an Endoscopically Normal Colon. Nutrients 11:613. doi: 10.3390/nu11030613
Hanninen, A., Toivonen, R., Poysti, S., Belzer, C., Plovier, H., Ouwerkerk, J. P., et al. (2018). Akkermansia muciniphila induces gut microbiota remodelling and controls islet autoimmunity in NOD mice. Gut 67, 1445–1453. doi: 10.1136/gutjnl-2017-314508
Harach, T., Marungruang, N., Duthilleul, N., Cheatham, V., Mc Coy, K. D., Frisoni, G., et al. (2017). Reduction of Abeta amyloid pathology in APPPS1 transgenic mice in the absence of gut microbiota. Sci. Rep. 7:41802. doi: 10.1038/srep41802
He, X., Yan, C., Zhao, S., Zhao, Y., Huang, R., and Li, Y. (2022). The preventive effects of probiotic Akkermansia muciniphila on D-galactose/AlCl3 mediated Alzheimer's disease-like rats. Exp. Gerontol. 170:111959. doi: 10.1016/j.exger.2022.111959
Heintz-Buschart, A., Pandey, U., Wicke, T., Sixel-Doring, F., Janzen, A., Sittig-Wiegand, E., et al. (2018). The nasal and gut microbiome in Parkinson's disease and idiopathic rapid eye movement sleep behavior disorder. Mov. Disord. 33, 88–98. doi: 10.1002/mds.27105
Hertel, J., Harms, A. C., Heinken, A., Baldini, F., Thinnes, C. C., Glaab, E., et al. (2019). Integrated analyses of microbiome and longitudinal Metabolome data reveal microbial-host interactions on sulfur metabolism in Parkinson's disease. Cell Rep. 29:e1768. doi: 10.1016/j.celrep.2019.10.035
Higarza, S. G., Arboleya, S., Arias, J. L., Gueimonde, M., and Arias, N. (2021). Akkermansia muciniphila and environmental enrichment reverse cognitive impairment associated with high-fat high-cholesterol consumption in rats. Gut Microbes 13, 1–20. doi: 10.1080/19490976.2021.1880240
Hill-Burns, E. M., Debelius, J. W., Morton, J. T., Wissemann, W. T., Lewis, M. R., Wallen, Z. D., et al. (2017). Parkinson's disease and Parkinson's disease medications have distinct signatures of the gut microbiome. Mov. Disord. 32, 739–749. doi: 10.1002/mds.26942
Hintikka, J. E., Munukka, E., Valtonen, M., Luoto, R., Ihalainen, J. K., Kallonen, T., et al. (2022). Gut microbiota and serum Metabolome in elite cross-country skiers: a controlled study. Meta 12:335. doi: 10.3390/metabo12040335
Holmqvist, S., Chutna, O., Bousset, L., Aldrin-Kirk, P., Li, W., Bjorklund, T., et al. (2014). Direct evidence of Parkinson pathology spread from the gastrointestinal tract to the brain in rats. Acta Neuropathol. 128, 805–820. doi: 10.1007/s00401-014-1343-6
Hou, Y. F., Shan, C., Zhuang, S. Y., Zhuang, Q. Q., Ghosh, A., Zhu, K. C., et al. (2021). Gut microbiota-derived propionate mediates the neuroprotective effect of osteocalcin in a mouse model of Parkinson's disease. Microbiome 9:34. doi: 10.1186/s40168-020-00988-6
Huang, C., Li, Y., Feng, X., Li, D., Li, X., Ouyang, Q., et al. (2019). Distinct gut microbiota composition and functional category in children with cerebral palsy and epilepsy. Front. Pediatr. 7:394. doi: 10.3389/fped.2019.00394
Huang, W. C., Pan, C. H., Wei, C. C., and Huang, H. Y. (2020). Lactobacillus plantarum PS128 improves physiological adaptation and performance in triathletes through gut microbiota modulation. Nutrients 12:2315. doi: 10.3390/nu12082315
Hughes, H. K., Rose, D., and Ashwood, P. (2018). The gut microbiota and Dysbiosis in autism Spectrum disorders. Curr. Neurol. Neurosci. Rep. 18:81. doi: 10.1007/s11910-018-0887-6
iMSMS Consortium. Electronic address: sergio.baranzini@ucsf.edu; iMSMS Consortium (2022). Gut microbiome of multiple sclerosis patients and paired household healthy controls reveal associations with disease risk and course. Cell 185, 3467–3486.e16. doi: 10.1016/j.cell.2022.08.021
Inoue, R., Sakaue, Y., Sawai, C., Sawai, T., Ozeki, M., Romero-Perez, G. A., et al. (2016). A preliminary investigation on the relationship between gut microbiota and gene expressions in peripheral mononuclear cells of infants with autism spectrum disorders. Biosci. Biotechnol. Biochem. 80, 2450–2458. doi: 10.1080/09168451.2016.1222267
Jangi, S., Gandhi, R., Cox, L. M., Li, N., von Glehn, F., Yan, R., et al. (2016). Alterations of the human gut microbiome in multiple sclerosis. Nat. Commun. 7:12015. doi: 10.1038/ncomms12015
Jeon, H., Bae, C. H., Lee, Y., Kim, H. Y., and Kim, S. (2021). Korean red ginseng suppresses 1-methyl-4-phenyl-1,2,3,6-tetrahydropyridine-induced inflammation in the substantia nigra and colon. Brain Behav. Immun. 94, 410–423. doi: 10.1016/j.bbi.2021.02.028
Kang, D. W., Park, J. G., Ilhan, Z. E., Wallstrom, G., Labaer, J., Adams, J. B., et al. (2013). Reduced incidence of Prevotella and other fermenters in intestinal microflora of autistic children. PLoS One 8:e68322. doi: 10.1371/journal.pone.0068322
Karcher, N., Nigro, E., Puncochar, M., Blanco-Miguez, A., Ciciani, M., Manghi, P., et al. (2021). Genomic diversity and ecology of human-associated Akkermansia species in the gut microbiome revealed by extensive metagenomic assembly. Genome Biol. 22:209. doi: 10.1186/s13059-021-02427-7
Keshavarzian, A., Green, S. J., Engen, P. A., Voigt, R. M., Naqib, A., Forsyth, C. B., et al. (2015). Colonic bacterial composition in Parkinson's disease. Mov. Disord. 30, 1351–1360. doi: 10.1002/mds.26307
Laniewski, P., Ilhan, Z. E., and Herbst-Kralovetz, M. M. (2020). The microbiome and gynaecological cancer development, prevention and therapy. Nat. Rev. Urol. 17, 232–250. doi: 10.1038/s41585-020-0286-z
Le Chatelier, E., Nielsen, T., Qin, J., Prifti, E., Hildebrand, F., Falony, G., et al. (2013). Richness of human gut microbiome correlates with metabolic markers. Nature 500, 541–546. doi: 10.1038/nature12506
Lee, Y., Byeon, H. R., Jang, S. Y., Hong, M. G., Kim, D., Lee, D., et al. (2022). Oral administration of Faecalibacterium prausnitzii and Akkermansia muciniphila strains from humans improves atopic dermatitis symptoms in DNCB induced NC/Nga mice. Sci. Rep. 12:7324. doi: 10.1038/s41598-022-11048-4
Lee, Y., Park, J. Y., Lee, E. H., Yang, J., Jeong, B. R., Kim, Y. K., et al. (2017). Rapid assessment of microbiota changes in individuals with autism Spectrum disorder using Bacteria-derived membrane vesicles in urine. Exp Neurobiol 26, 307–317. doi: 10.5607/en.2017.26.5.307
Li, C., Cui, L., Yang, Y., Miao, J., Zhao, X., Zhang, J., et al. (2019). Gut microbiota differs between Parkinson's disease patients and healthy controls in Northeast China. Front. Mol. Neurosci. 12:171. doi: 10.3389/fnmol.2019.00171
Li, J., Lin, S., Vanhoutte, P. M., Woo, C. W., and Xu, A. (2016). Akkermansia muciniphila protects against atherosclerosis by preventing metabolic Endotoxemia-induced inflammation in Apoe−/− mice. Circulation 133, 2434–2446. doi: 10.1161/CIRCULATIONAHA.115.019645
Liu, M. J., Yang, J. Y., Yan, Z. H., Hu, S., Li, J. Q., Xu, Z. X., et al. (2022). Recent findings in Akkermansia muciniphila-regulated metabolism and its role in intestinal diseases. Clin. Nutr. 41, 2333–2344. doi: 10.1016/j.clnu.2022.08.029
Louis, S., Tappu, R. M., Damms-Machado, A., Huson, D. H., and Bischoff, S. C. (2016). Characterization of the gut microbial Community of Obese Patients Following a weight-loss intervention using whole Metagenome shotgun sequencing. PLoS One 11:e0149564. doi: 10.1371/journal.pone.0149564
Ma, D., Wang, A. C., Parikh, I., Green, S. J., Hoffman, J. D., Chlipala, G., et al. (2018). Ketogenic diet enhances neurovascular function with altered gut microbiome in young healthy mice. Sci. Rep. 8:6670. doi: 10.1038/s41598-018-25190-5
Makdissi, S., Parsons, B. D., and Di Cara, F. (2023). Towards early detection of neurodegenerative diseases: a gut feeling. Front. Cell Dev. Biol. 11:1087091. doi: 10.3389/fcell.2023.1087091
Mamic, P., Snyder, M., and Tang, W. H. W. (2023). Gut microbiome-based Management of Patients with Heart Failure: JACC review topic of the week. J. Am. Coll. Cardiol. 81, 1729–1739. doi: 10.1016/j.jacc.2023.02.045
Maslowski, K. M., and Mackay, C. R. (2011). Diet, gut microbiota and immune responses. Nat. Immunol. 12, 5–9. doi: 10.1038/ni0111-5
McGaughey, K. D., Yilmaz-Swenson, T., Elsayed, N. M., Cruz, D. A., Rodriguiz, R. M., Kritzer, M. D., et al. (2019). Relative abundance of Akkermansia spp. and other bacterial phylotypes correlates with anxiety- and depressive-like behavior following social defeat in mice. Sci. Rep. 9:3281. doi: 10.1038/s41598-019-40140-5
Montagne, A., Zhao, Z., and Zlokovic, B. V. (2017). Alzheimer's disease: a matter of blood-brain barrier dysfunction? J. Exp. Med. 214, 3151–3169. doi: 10.1084/jem.20171406
Mosca, A., Leclerc, M., and Hugot, J. P. (2016). Gut microbiota diversity and human diseases: should we reintroduce key predators in our ecosystem? Front. Microbiol. 7:455. doi: 10.3389/fmicb.2016.00455
Muller, M., Hernandez, M. A. G., Goossens, G. H., Reijnders, D., Holst, J. J., Jocken, J. W. E., et al. (2019). Circulating but not faecal short-chain fatty acids are related to insulin sensitivity, lipolysis and GLP-1 concentrations in humans. Sci. Rep. 9:12515. doi: 10.1038/s41598-019-48775-0
Munukka, E., Ahtiainen, J. P., Puigbo, P., Jalkanen, S., Pahkala, K., Keskitalo, A., et al. (2018). Six-week endurance exercise alters gut Metagenome that is not reflected in systemic metabolism in over-weight women. Front. Microbiol. 9:2323. doi: 10.3389/fmicb.2018.02323
Nagpal, R., Neth, B. J., Wang, S., Craft, S., and Yadav, H. (2019). Modified Mediterranean-ketogenic diet modulates gut microbiome and short-chain fatty acids in association with Alzheimer's disease markers in subjects with mild cognitive impairment. EBioMedicine 47, 529–542. doi: 10.1016/j.ebiom.2019.08.032
Neth, B. J., Mintz, A., Whitlow, C., Jung, Y., Solingapuram Sai, K., Register, T. C., et al. (2020). Modified ketogenic diet is associated with improved cerebrospinal fluid biomarker profile, cerebral perfusion, and cerebral ketone body uptake in older adults at risk for Alzheimer's disease: a pilot study. Neurobiol. Aging 86, 54–63. doi: 10.1016/j.neurobiolaging.2019.09.015
Nishiwaki, H., Ito, M., Ishida, T., Hamaguchi, T., Maeda, T., Kashihara, K., et al. (2020). Meta-analysis of gut Dysbiosis in Parkinson's disease. Mov. Disord. 35, 1626–1635. doi: 10.1002/mds.28119
Olson, C. A., Vuong, H. E., Yano, J. M., Liang, Q. Y., Nusbaum, D. J., and Hsiao, E. Y. (2018). The gut microbiota mediates the anti-seizure effects of the Ketogenic diet. Cells 174:497. doi: 10.1016/j.cell.2018.06.051
Ottman, N., Geerlings, S. Y., Aalvink, S., de Vos, W. M., and Belzer, C. (2017a). Action and function of Akkermansia muciniphila in microbiome ecology, health and disease. Best Pract. Res. Clin. Gastroenterol. 31, 637–642. doi: 10.1016/j.bpg.2017.10.001
Ottman, N., Reunanen, J., Meijerink, M., Pietila, T. E., Kainulainen, V., Klievink, J., et al. (2017b). Pili-like proteins of Akkermansia muciniphila modulate host immune responses and gut barrier function. PLoS One 12:e0173004. doi: 10.1371/journal.pone.0173004
Ou, Z., Deng, L., Lu, Z., Wu, F., Liu, W., Huang, D., et al. (2020). Protective effects of Akkermansia muciniphila on cognitive deficits and amyloid pathology in a mouse model of Alzheimer's disease. Nutr. Diabetes 10:12. doi: 10.1038/s41387-020-0115-8
Ouwerkerk, J. P., van der Ark, K. C. H., Davids, M., Claassens, N. J., Finestra, T. R., de Vos, W. M., et al. (2016). Adaptation of Akkermansia muciniphila to the Oxic-anoxic Interface of the mucus layer. Appl. Environ. Microbiol. 82, 6983–6993. doi: 10.1128/AEM.01641-16
Ozkul, C., Yalinay, M., and Karakan, T. (2019). Islamic fasting leads to an increased abundance of Akkermansia muciniphila and Bacteroides fragilis group: a preliminary study on intermittent fasting. Turk J Gastroenterol 30, 1030–1035. doi: 10.5152/tjg.2019.19185
Park, Y. S., Kim, S. H., Park, J. W., Kho, Y., Seok, P. R., Shin, J. H., et al. (2020). Melatonin in the colon modulates intestinal microbiota in response to stress and sleep deprivation. Intest Res 18, 325–336. doi: 10.5217/ir.2019.00093
Payahoo, L., Khajebishak, Y., Alivand, M. R., Soleimanzade, H., Alipour, S., Barzegari, A., et al. (2019). Investigation the effect of oleoylethanolamide supplementation on the abundance of Akkermansia muciniphila bacterium and the dietary intakes in people with obesity: a randomized clinical trial. Appetite 141:104301. doi: 10.1016/j.appet.2019.05.032
Pedret, A., Valls, R. M., Calderon-Perez, L., Llaurado, E., Companys, J., Pla-Paga, L., et al. (2019). Effects of daily consumption of the probiotic Bifidobacterium animalis subsp. lactis CECT 8145 on anthropometric adiposity biomarkers in abdominally obese subjects: a randomized controlled trial. Int. J. Obes. 43, 1863–1868. doi: 10.1038/s41366-018-0220-0
Petersen, L. M., Bautista, E. J., Nguyen, H., Hanson, B. M., Chen, L., Lek, S. H., et al. (2017). Community characteristics of the gut microbiomes of competitive cyclists. Microbiome 5:98. doi: 10.1186/s40168-017-0320-4
Pietrucci, D., Teofani, A., Unida, V., Cerroni, R., Biocca, S., Stefani, A., et al. (2020). Can gut microbiota be a good predictor for Parkinson's disease? A machine learning approach. Brain Sci 10:242. doi: 10.3390/brainsci10040242
Plovier, H., Everard, A., Druart, C., Depommier, C., Van Hul, M., Geurts, L., et al. (2017). A purified membrane protein from Akkermansia muciniphila or the pasteurized bacterium improves metabolism in obese and diabetic mice. Nat. Med. 23, 107–113. doi: 10.1038/nm.4236
Probstel, A. K., Zhou, X., Baumann, R., Wischnewski, S., Kutza, M., Rojas, O. L., et al. (2020). Gut microbiota-specific IgA(+) B cells traffic to the CNS in active multiple sclerosis. Sci Immunol 5:eabc7191. doi: 10.1126/sciimmunol.abc7191
Rao, Y., Kuang, Z., Li, C., Guo, S., Xu, Y., Zhao, D., et al. (2021). Gut Akkermansia muciniphila ameliorates metabolic dysfunction-associated fatty liver disease by regulating the metabolism of L-aspartate via gut-liver axis. Gut Microbes 13, 1–19. doi: 10.1080/19490976.2021.1927633
Remely, M., Hippe, B., Geretschlaeger, I., Stegmayer, S., Hoefinger, I., and Haslberger, A. (2015). Increased gut microbiota diversity and abundance of Faecalibacterium prausnitzii and Akkermansia after fasting: a pilot study. Wien. Klin. Wochenschr. 127, 394–398. doi: 10.1007/s00508-015-0755-1
Remely, M., Hippe, B., Zanner, J., Aumueller, E., Brath, H., and Haslberger, A. G. (2016). Gut microbiota of obese, type 2 diabetic individuals is enriched in Faecalibacterium prausnitzii, Akkermansia muciniphila and Peptostreptococcus anaerobius after weight loss. Endocr. Metab. Immune Disord. Drug Targets 16, 99–106. doi: 10.2174/1871530316666160831093813
Reunanen, J., Kainulainen, V., Huuskonen, L., Ottman, N., Belzer, C., Huhtinen, H., et al. (2015). Akkermansia muciniphila adheres to enterocytes and strengthens the integrity of the epithelial cell layer. Appl. Environ. Microbiol. 81, 3655–3662. doi: 10.1128/AEM.04050-14
Roshanravan, N., Mahdavi, R., Alizadeh, E., Jafarabadi, M. A., Hedayati, M., Ghavami, A., et al. (2017). Effect of butyrate and inulin supplementation on glycemic status, lipid profile and glucagon-like peptide 1 level in patients with type 2 diabetes: a randomized double-blind, placebo-controlled trial. Horm. Metab. Res. 49, 886–891. doi: 10.1055/s-0043-119089
Sampson, T. R., Debelius, J. W., Thron, T., Janssen, S., Shastri, G. G., Ilhan, Z. E., et al. (2016). Gut microbiota regulate motor deficits and Neuroinflammation in a model of Parkinson's disease. Cells 167:e1412. doi: 10.1016/j.cell.2016.11.018
Sanchez-Alcoholado, L., Gutierrez-Repiso, C., Gomez-Perez, A. M., Garcia-Fuentes, E., Tinahones, F. J., and Moreno-Indias, I. (2019). Gut microbiota adaptation after weight loss by roux-en-Y gastric bypass or sleeve gastrectomy bariatric surgeries. Surg. Obes. Relat. Dis. 15, 1888–1895. doi: 10.1016/j.soard.2019.08.551
Sharon, G., Cruz, N. J., Kang, D. W., Gandal, M. J., Wang, B., Kim, Y. M., et al. (2019). Human gut microbiota from autism Spectrum disorder promote behavioral symptoms in mice. Cells 177:e1617. doi: 10.1016/j.cell.2019.05.004
Sherwin, E., Dinan, T. G., and Cryan, J. F. (2018). Recent developments in understanding the role of the gut microbiota in brain health and disease. Ann. N. Y. Acad. Sci. 1420, 5–25. doi: 10.1111/nyas.13416
Shin, J., Noh, J. R., Chang, D. H., Kim, Y. H., Kim, M. H., Lee, E. S., et al. (2019). Elucidation of Akkermansia muciniphila probiotic traits driven by Mucin depletion. Front. Microbiol. 10:1137. doi: 10.3389/fmicb.2019.01137
Smid, E. J., and Lacroix, C. (2013). Microbe-microbe interactions in mixed culture food fermentations. Curr. Opin. Biotechnol. 24, 148–154. doi: 10.1016/j.copbio.2012.11.007
Song, J., Ma, W., Gu, X., Zhao, L., Jiang, J., Xu, Y., et al. (2019). Metabolomic signatures and microbial community profiling of depressive rat model induced by adrenocorticotrophic hormone. J. Transl. Med. 17:224. doi: 10.1186/s12967-019-1970-8
Strati, F., Cavalieri, D., Albanese, D., De Felice, C., Donati, C., Hayek, J., et al. (2017). New evidences on the altered gut microbiota in autism spectrum disorders. Microbiome 5:24. doi: 10.1186/s40168-017-0242-1
Tankou, S. K., Regev, K., Healy, B. C., Tjon, E., Laghi, L., Cox, L. M., et al. (2018). A probiotic modulates the microbiome and immunity in multiple sclerosis. Ann. Neurol. 83, 1147–1161. doi: 10.1002/ana.25244
Tenorio-Jimenez, C., Martinez-Ramirez, M. J., Del Castillo-Codes, I., Arraiza-Irigoyen, C., Tercero-Lozano, M., Camacho, J., et al. (2019). Lactobacillus reuteri V3401 reduces inflammatory biomarkers and modifies the gastrointestinal microbiome in adults with metabolic syndrome: the PROSIR study. Nutrients 11:1761. doi: 10.3390/nu11081761
Tung, T. H., Tung, Y. T., Lin, I. H., Shih, C. K., Nguyen, N. T. K., Shabrina, A., et al. (2019). Fish oil, but not olive oil, ameliorates depressive-like behavior and gut microbiota Dysbiosis in rats under chronic mild stress. Biomol. Ther. 9:516. doi: 10.3390/biom9100516
Unger, M. M., Spiegel, J., Dillmann, K. U., Grundmann, D., Philippeit, H., Burmann, J., et al. (2016). Short chain fatty acids and gut microbiota differ between patients with Parkinson's disease and age-matched controls. Parkinsonism Relat. Disord. 32, 66–72. doi: 10.1016/j.parkreldis.2016.08.019
van Passel, M. W., Kant, R., Zoetendal, E. G., Plugge, C. M., Derrien, M., Malfatti, S. A., et al. (2011). The genome of Akkermansia muciniphila, a dedicated intestinal mucin degrader, and its use in exploring intestinal metagenomes. PLoS One 6:e16876. doi: 10.1371/journal.pone.0016876
Vidal-Martinez, G., Chin, B., Camarillo, C., Herrera, G. V., Yang, B., Sarosiek, I., et al. (2020). A pilot microbiota study in Parkinson's disease patients versus control subjects, and effects of FTY720 and FTY720-Mitoxy therapies in Parkinsonian and multiple system atrophy mouse models. J. Parkinsons Dis. 10, 185–192. doi: 10.3233/JPD-191693
Vogt, N. M., Kerby, R. L., Dill-McFarland, K. A., Harding, S. J., Merluzzi, A. P., Johnson, S. C., et al. (2017). Gut microbiome alterations in Alzheimer's disease. Sci. Rep. 7:13537. doi: 10.1038/s41598-017-13601-y
Wan, L., Zhou, X., Wang, C., Chen, Z., Peng, H., Hou, X., et al. (2019). Alterations of the gut microbiota in multiple system atrophy patients. Front. Neurosci. 13:1102. doi: 10.3389/fnins.2019.01102
Wang, L., Christophersen, C. T., Sorich, M. J., Gerber, J. P., Angley, M. T., and Conlon, M. A. (2011). Low relative abundances of the mucolytic bacterium Akkermansia muciniphila and Bifidobacterium spp. in feces of children with autism. Appl. Environ. Microbiol. 77, 6718–6721. doi: 10.1128/AEM.05212-11
Wang, Q., Luo, Y., Ray Chaudhuri, K., Reynolds, R., Tan, E. K., and Pettersson, S. (2021). The role of gut dysbiosis in Parkinson's disease: mechanistic insights and therapeutic options. Brain 144, 2571–2593. doi: 10.1093/brain/awab156
Wang, Z., Saha, S., Van Horn, S., Thomas, E., Traini, C., Sathe, G., et al. (2018). Gut microbiome differences between metformin- and liraglutide-treated T2DM subjects. Endocrinol Diabetes Metab 1:e00009. doi: 10.1002/edm2.9
Wang, L., Tang, L., Feng, Y., Zhao, S., Han, M., Zhang, C., et al. (2020). A purified membrane protein from Akkermansia muciniphila or the pasteurised bacterium blunts colitis associated tumourigenesis by modulation of CD8(+) T cells in mice. Gut 69, 1988–1997. doi: 10.1136/gutjnl-2019-320105
Wu, F., Guo, X., Zhang, M., Ou, Z., Wu, D., Deng, L., et al. (2020). An Akkermansia muciniphila subtype alleviates high-fat diet-induced metabolic disorders and inhibits the neurodegenerative process in mice. Anaerobe 61:102138. doi: 10.1016/j.anaerobe.2019.102138
Xu, Y., Wang, N., Tan, H. Y., Li, S., Zhang, C., and Feng, Y. (2020). Function of Akkermansia muciniphila in obesity: interactions with lipid metabolism, immune response and gut systems. Front. Microbiol. 11:219. doi: 10.3389/fmicb.2020.00219
Yang, Y., Zhong, Z., Wang, B., Xia, X., Yao, W., Huang, L., et al. (2019). Early-life high-fat diet-induced obesity programs hippocampal development and cognitive functions via regulation of gut commensal Akkermansia muciniphila. Neuropsychopharmacology 44, 2054–2064. doi: 10.1038/s41386-019-0437-1
Zhai, Q., Feng, S., Arjan, N., and Chen, W. (2019). A next generation probiotic, Akkermansia muciniphila. Crit. Rev. Food Sci. Nutr. 59, 3227–3236. doi: 10.1080/10408398.2018.1517725
Zhang, T., Li, P., Wu, X., Lu, G., Marcella, C., Ji, X., et al. (2020). Alterations of Akkermansia muciniphila in the inflammatory bowel disease patients with washed microbiota transplantation. Appl. Microbiol. Biotechnol. 104, 10203–10215. doi: 10.1007/s00253-020-10948-7
Zou, R., Xu, F., Wang, Y., Duan, M., Guo, M., Zhang, Q., et al. (2020). Changes in the gut microbiota of children with autism Spectrum disorder. Autism Res. 13, 1614–1625. doi: 10.1002/aur.2358
Keywords: Akkermansia muciniphila , Amuc_1100, neuropsychological disease, probiotics, treatment
Citation: Zhang F and Wang D (2023) Potential of Akkermansia muciniphila and its outer membrane proteins as therapeutic targets for neuropsychological diseases. Front. Microbiol. 14:1191445. doi: 10.3389/fmicb.2023.1191445
Edited by:
Karolina Skonieczna-Żydecka, Pomeranian Medical University, PolandReviewed by:
Kiran Veer Sandhu, University College Cork, IrelandRichard Agans, Air Force Research Laboratory, United States
Peter Suenaert, SparkClin, Belgium
Copyright © 2023 Zhang and Wang. This is an open-access article distributed under the terms of the Creative Commons Attribution License (CC BY). The use, distribution or reproduction in other forums is permitted, provided the original author(s) and the copyright owner(s) are credited and that the original publication in this journal is cited, in accordance with accepted academic practice. No use, distribution or reproduction is permitted which does not comply with these terms.
*Correspondence: Dali Wang, d2FuZ2RsQHN1bWhzLmVkdS5jbg==