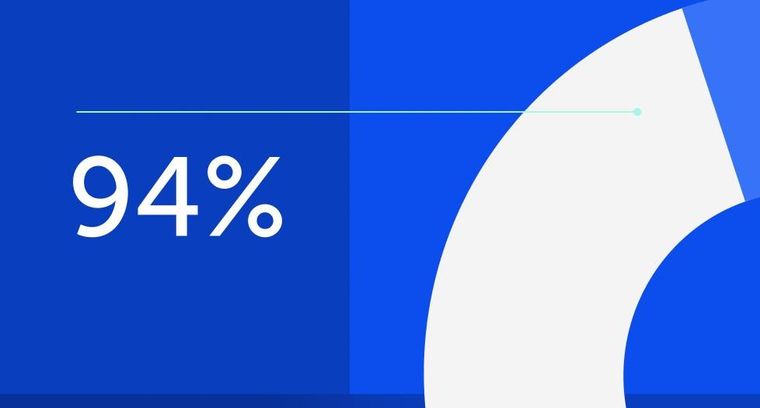
94% of researchers rate our articles as excellent or good
Learn more about the work of our research integrity team to safeguard the quality of each article we publish.
Find out more
REVIEW article
Front. Microbiol., 02 June 2023
Sec. Infectious Agents and Disease
Volume 14 - 2023 | https://doi.org/10.3389/fmicb.2023.1183247
Tuberculosis and lung cancer are, in many cases, correlated diseases that can be confused because they have similar symptoms. Many meta-analyses have proven that there is a greater chance of developing lung cancer in patients who have active pulmonary tuberculosis. It is, therefore, important to monitor the patient for a long time after recovery and search for combined therapies that can treat both diseases, as well as face the great problem of drug resistance. Peptides are molecules derived from the breakdown of proteins, and the membranolytic class is already being studied. It has been proposed that these molecules destabilize cellular homeostasis, performing a dual antimicrobial and anticancer function and offering several possibilities of adaptation for adequate delivery and action. In this review, we focus on two important reason for the use of multifunctional peptides or peptides, namely the double activity and no harmful effects on humans. We review some of the main antimicrobial and anti-inflammatory bioactive peptides and highlight four that have anti-tuberculosis and anti-cancer activity, which may contribute to obtaining drugs with this dual functionality.
Tuberculosis, a disease caused by Mycobacterium tuberculosis (Mtb), usually affects the lungs but can also affect other sites (extrapulmonary tuberculosis; Gradmann, 2006). First contact occurs when the bacillus is inhaled and it reaches the lungs. It may then be eliminated by the immune system, contained in a state of latency, or initiate an active infection. The outcome differs for each individual, depending on the immune status of the host (Ankrah et al., 2018). Common symptoms are coughing with sputum and sometimes blood, chest pain, weakness, weight loss, fever, and night sweats. These symptoms are often mild for many months, leading to delays in treatment and increasing the risk of spreading the infection to others (Bloom and Murray, 1992).
Until the COVID-19 pandemic, tuberculosis was considered the leading cause of death from a single infectious agent. According to the report by the World Health Organization, 5.8 million people were diagnosed with the disease in 2020, a lower number than in 2019, probably affected by the pandemic, but the figure rose again in 2021 to 6.4 million. With the drop in the number of diagnoses and, consequently, treatment, the number of deaths increased, to officially 1.4 million deaths in 2021. These data are a consequence of several factors associated with the COVID-19 pandemic: the health system was less capable of receiving tuberculosis cases, people had limited mobility and the symptoms of the disease and COVID were similar. It is believed that 10.6 million people contracted tuberculosis in 2021, a significantly higher number than those diagnosed. Most of these cases occurred in Southeast Asia (45%), Africa (23%) and the Western Pacific (18%). Men were the most affected by the disease (56.5% of total cases), followed by women (32.5%) and children (11%; WHO, 2022).
Lung cancer (LC) is another serious disease that affected 2.2 million people in 2020 (11.4% of all cancers), killing 1.7 million, about 18% of all cancer deaths in the world (Globocan, 2020). Currently, this type of cancer has a poor prognosis and adverse clinical outcomes, mainly because of its late diagnosis, ineffective treatment and tumor cell resistance. There are about 2.09 million cases and 1.76 million deaths every year (Arrieta et al., 2022). It is the second most common diagnosis in men and women, after prostate and breast cancer. Most LC cases occur at a mean age of 70 years and men are more affected than women (Chaitanya Thandra et al., 2021). Table 1 lists some microorganisms present in the tumor microenvironment, as well as their possible interactions with cancer cells.
To understand the entire pathophysiology of LC, one must understand the contributions of the tumor microenvironment (TME) and the host immune responses, which are interconnected with each step of the tumorigenesis of each cancer subtype (Salehi-Rad et al., 2020). The TME is the environment where the tumor is located and is formed by various types of cells, like immune and stromal cells. Additionally, the TME is composed of extracellular matrix molecules and a variety of cytokines produced by the tumoral cells (Altorki et al., 2019; Wu et al., 2021). This microenvironment can define the cells present within the tumor, as well as the cytokines and the interactions that may occur. For example, macrophages and neutrophils are involved in the mechanism of immune escape and lung cancer development. These cells produce a proinflammatory background that strongly affects the carcinogenesis and the immune-response efficiency (Altorki et al., 2019; Madeddu et al., 2022). The extracellular matrix is composed of collagens, proteoglycans and glycosaminoglycans, and it mediates the interaction between the cells in the TME. This interaction can promote carcinogenesis (Altorki et al., 2019). The TME is also important for the evasion of immune recognition. Lung cancers can alter the composition of TME to establish an immunosuppressive environment, by increasing the abundance of inhibitory molecules, such as TGT-β, IL-6 and PGE2 (Salehi-Rad et al., 2020). The genomic profile is defined by the progressive accumulation of mutations in oncogenes and tumor suppressor genes, from dysplasia and pre-neoplasia to metastatic cancer (Hanahan and Weinberg, 2011). Intrinsic genomic alterations within premalignant or neoplastic cells can reprogram the composition of the TME to facilitate carcinogenesis. Still, chronic inflammation caused by extrinsic factors, such as unresolved infections, can induce the lung TME to develop cancer progression (Dubinett, 2015). Detailed genomic profiling of LC has revealed significant heterogeneity among patients, and this has important clinical implications for the evolution of the cancer genome and acquired resistance to therapy (Salehi-Rad et al., 2020).
There are two main types of LC, called ‘small cell’ (approximately 15%) and ‘non-small cell’ (the remaining 85%). The most frequently found, non-small cell (NSCLC), is most associated with smoking but is also found in people who have never smoked, with a slightly different genomic landscape. This LC type includes subtypes with treatment and prognoses that are often similar, the main subtypes being adenocarcinoma, squamous cell carcinoma and large cell carcinoma. The small cell type (SCLC), in most cases, is a high-grade neuroendocrine tumor. This type is characterized by fast growth and metastasis, as compared with NSCLC type, and a poor clinical outcome in general (Gazdar et al., 2017; American Cancer Society, 2019). As it is a highly heterogeneous disease, molecular characterization is claimed to improve the understanding of tumor pathogenesis and thus define a personalized treatment plan (De Castro et al., 2019).
Some factors facilitate the development of this carcinoma, such as age, tobacco smoking and tuberculosis (Chaitanya Thandra et al., 2021). Several types of studies have shown that there is a positive correlation between the incidence of tuberculosis and LC, and the simultaneous occurrence of these features has increased. Tuberculosis patients have a 50% increased chance of developing LC. Those with a history of tuberculosis for more than 20 years are 2.5 times more likely to develop this disease (Sun et al., 2022). Therefore, these patients must be monitored to prevent the development of this type of cancer, since the prognosis of the disease can also be affected (Arrieta et al., 2022).
Many studies have demonstrated the relationship between chronic inflammation and cancer development. In 1863, Rudolf Virchow first identified the correlation between inflammation and cancer when he noticed that there were leukocytes in neoplastic tissues (Balkwill and Mantovani, 2001). Experimental evidence has proved that lung carcinoma can be triggered by chronic Mtb infection (Nalbandian et al., 2009). Furthermore, the most recent meta-analysis we found demonstrates that pre-existing active pulmonary TB increases the relative risk of LC, mainly squamous cell carcinoma (3,570), followed by adenocarcinoma (2,605) and small cell carcinoma (2,118). It also exhibits an increased risk of 2,746 for other histological types of LC. The authors of this meta-analysis searched for articles and abstracts published from 1987 to 2021 in different databases, and they concluded that a patient with a history of active pulmonary TB should be followed up for a longer time after the cure of pulmonary TB than patients with no such history (Abdeahad et al., 2022). Another study also showed that the incidence of LC was higher in patients with tuberculosis, with a significant increase in mortality in TB cancer patients (Kiri et al., 2009; Liang et al., 2009). In this regard, it is important to highlight that TB treatment usually takes 6–9 months of combined drug therapy. During this period, TB infection causes severe lung inflammation, which could be related to a chronic inflammatory process that has been linked to several stages of carcinogenesis (Liu et al., 2020).
Although both diseases affect the same anatomical unit of the lung, some researchers do not believe that there is clear pathogenesis between them, but rather that tuberculosis is just another direct risk factor for LC, among other widely known factors (Qin et al., 2022). As both diseases affect the same organ, the symptoms are very similar, and although it is harmful for a cancer patient to receive tuberculosis treatment (or vice versa), it is even more dangerous for a patient with both diseases to receive treatment for only one of them, as the other disease worsens without treatment (Malik et al., 2022).
The immune system plays an important role in cancer prevention; however, cancer cells can still act by preventing or inhibiting antitumor responses. Innate and adaptive immunities work by eliminating or suppressing viral infections that can induce a tumor, preventing the maintenance of an environment that favors the emergence of a tumor or identifying tumor cells and eliminating them (Shroff et al., 2022).
Mtb is an intracellular pathogen able to infect human mononuclear phagocytes, spending most of its life cycle in macrophages (Vasava et al., 2017). In addition to other very important immune cells, in the initial phase of Mtb infection, the bacillus is phagocytosed by alveolar macrophages (AM). These cells are then activated and they adopt a pro-inflammatory phenotype, recruiting more immune cells to the site of infection, among other interstitial macrophages (IM), which decrease oxidative phosphorylation, the main way of obtaining energy from AM (Tukiman, 2022). This type of energy acquisition is mainly fueled by the oxidation of fatty acids, which are abundant in lung tissue, but this makes the environment even more conducive to infection by mycobacteria, which also use fatty acids as a carbon source (Sheedy and Divangahi, 2021). IM also increases aerobic glycolysis, as in the Warburg effect, providing biosynthetic precursors necessary for rapid cell growth and proliferation (Macintyre and Rathmell, 2013). The Warburg effect has been described to explain the origin and functioning of cancer cells, and it includes the generation of pro-inflammatory cytokines and antimicrobial molecules, in addition to reactive oxygen and nitrogen species (ROS and RNS; Shi et al., 2016a). This mechanism is part of the attempt to eliminate the infection, so if aerobic glycolysis is inhibited at this stage of the infection, the resulting immunological changes lead to an increase in Mtb survival, mainly due to the reduction in pro-inflammatory IL-1β levels (Gleeson et al., 2016). Inflammatory cells produce many cytokines and chemokines that form an attractive environment for tumor development, promoting the formation of new blood vessels from existing ones, in addition to facilitating genomic instability (Coussens and Werb, 2002). In chronic inflammation, by producing ROS and RNS, most phagocytic cells induce DNA damage in proliferating cells due to the consequent production of peroxynitrite (Maeda and Akaike, 1998). If DNA damage occurs continuously and repeatedly, it may result in permanent genomic alterations, such as point mutations, deletions or rearrangements (Coussens and Werb, 2002; Figure 1).
Figure 1. Pathway triggered after infection that can result in cancer cells. The figure was created by Biorender.com, partly generated using Servier Medical Art, provided by Servier, licensed under a Creative Commons Attribution 3.0 unported license.
In the early stage of this infection, activation of the immune response with type T helper cells (Th1) and the production of IFN-γ and TNF-α are the most prominent protective mechanisms for intracellular mycobacterial killing. With the progression of the infection and the interaction between mycobacteria and immune cells, the formation of granulomas occurs. These granulomas are characterized by an extremely hypoxic and inflammatory internal region, maintained by glycolysis to kill the bacilli, and a less inflammatory peripheral region, consequently favoring the survival of the bacillus (Tukiman, 2022). Granulomas can lead to caseous lesions and cavity formation, in the latter case being extremely infectious as it is the consequence of lung tissue destruction with the formation of macroscopic open spaces that contain numerous bacilli and connect with the large airways, facilitating efficient expectoration of the bacteria (Younga and Verreck, 2012). The granuloma is characterized by glycolysis in the central region and oxidative phosphorylation in the peripheral region. These granulomas normally contain live mycobacteria, myeloid and lymphoid cells (Nalbandian et al., 2009). However, the Warburg effect was found to be reduced in the nucleus of the granuloma, suggesting that the bacillus can modulate the host’s defense mechanism to survive (Shi et al., 2016a,b). In line with this finding, Shi et al. (2016a) suggested the search for therapeutic compounds that have the potential to enhance the Warburg effect on infected macrophages to combat Mtb (Shi et al., 2016a).
Finally, LC originating from the dysregulated chronic inflammation can also foster abundant expression of growth factors and cytokines, such as transforming growth factor beta (TGF-b) and interleukin (IL)-1b, IL-4, IL-6, IL-8, IL-10, and IL-22, which activate multiple tumorigenic pathways, such as cyclooxygenase 2 (COX-2) and nuclear factor kappa B subunit (NF-kB) to promote tumorigenesis (Dubinett, 2015). More importantly, TB also causes extensive pulmonary fibrosis, which is associated with the production of TGF-Β, IL-4 and IL-13 (Heitmann et al., 2014; George et al., 2015). Conversely, LC can decrease local immunity and reactivate a latent infection, or even increase the chances of an exogenous infection, so it is worth paying close attention to these diseases (Dobler et al., 2017).
An important epithelial growth factor produced by the mycobacteria in the early stages of tumor formation is epiregulin. In inflammatory macrophages, its production can be beneficial, as it helps to repair tissue damage; however, with the presence of persistent live pathogens, such as Mtb, this adaptive response is directed against the host, providing in this case, a potent growth factor for pre-malignant cells (Nalbandian et al., 2009). High levels of expression of this gene have been found in several cancer cell lines (Baba et al., 2000).
The effect of pulmonary TB on the epidermal growth factor receptor (EGFR) in patients with lung adenocarcinoma (LAC) was investigated in 2019, and a significantly higher mutation frequency was found than in patients without tuberculosis (Hwang et al., 2019). Mutations in this gene, which originally encodes proteins for cell proliferation and survival, are the most frequent in LAC (Jett and Carr, 2013), and TB-induced overexpression of epiregulin conferred invasive properties on cancer cells (Zhang et al., 2008).
Most lung damage resulting from an Mtb infection is the result of an intense inflammatory immune response. It has been proposed that this damage has different levels of severity depending on the variability of the genes that encode or regulate the host’s immune responses, but this hypothesis has not yet been proven (Ravimohan et al., 2018). In 2009, an Mtb genetic locus that specifically controls tissue damage and progression of pulmonary tuberculosis, sst1, was identified as an important genetic modifier that could lead to lung tumor formation (Nalbandian et al., 2009). Chai et al. (2020) demonstrated that TB and LAC share 65 similar signature genes, but emphasized the MK167 gene, which encodes Ki-67, a protein expressed exclusively in proliferating cancer cells (Chai et al., 2020). The MK167 gene was equally increased in TB and LAC groups, and it is an important mediator for Mtb-induced proliferation, migration and evasion of tumor cells. It was also discovered that this gene is regulated by an effector protein that can promote tumor development by regulating cell proliferation and migration when it enters the host cell nucleus (Wang et al., 2017). Chai et al. (2020) further suggested that the mechanism that the mycobacteria use to spread more efficiently in LC patients is through infected tumor cells, as they obtained positive mobility results in migration and invasion assays (Chai et al., 2020).
Cao et al. (2019) hypothesized that Mtb infection mediated immune response and facilitated tumor metastasis through the PD-1/PD-L1 signaling pathway (Cao et al., 2019). Mtb also reprograms macrophages during granuloma formation by inducing expression of E-cadherin into epithelioid morphology, a process analogous to epithelial-to-mesenchymal transition (EMT; Cronan et al., 2016; Ahmad et al., 2022). Further, Mtb upregulates transcription factors that induce EMT, a hallmark of carcinogenesis and metastatic progression (Hofman and Vouret-Craviari, 2012; Gupta et al., 2016). Some important up-regulated genes, such as MYBL2, BRCA-1, UBE2C, CHEK-1, CDN2A and PCNA, are common in LC and TB, mostly associated with cell cycle, checkpoints and apoptosis (Cao et al., 2019).
Peptides are molecules derived from proteins that can be obtained by hydrolysis, like bioactive peptides, or isolated from animal protein fluids (constitutive or induced) as a natural defense mechanism (Silveira et al., 2021). Membranolytic peptides can be responsible for destabilizing the cellular homeostasis of microorganisms, such as antimicrobial peptides (AMPs), and tumor cells, such as anticancer peptides (ACPs). The history of peptides began in 1922 when lysozyme was discovered (Fleming, 1922). Through proteomic characterization, lysozyme turned out to possess various AMPs with potential bactericidal activity (Ibrahim et al., 2011). AMPs are broad-spectrum biomacromolecules that, for the most part, are capable of interacting with various receptors on the cell membrane or wall, causing intra-and extracellular imbalance and later leading to death.
This type of peptide generally has less than 100-mers and is characterized by rapidly interacting with the pathogenic agent at low concentrations, decreasing the rate of induction or generation of resistance. Roque-Borda et al. (2021a) reported that the use of the antimicrobial peptide Ctx(Ile21)-Ha has great activity against Pseudomonas aeurigonosa and Acinetobacter baumannii, two of the deadliest bacteria in the world according to the WHO priority list (Roque-Borda et al., 2021b). It is known that these two bacteria can be more dangerous and deadly when they have genes for resistance to carbapenems since these drugs are used as the last lines of treatment. Da Costa de Souza et al. (2022) showed that AMPs are capable of eliminating A. baumannii and P. aeurigonosa quickly and efficiently, avoiding selective pressure and even generating better potential inhibition than conventional drugs. Although they are good alternatives because they act through interaction with microbial membranes, many AMPs have poor pharmacological profiles, and alternatives are being studied to overcome these barriers, like the chemical modification and synthesis of new peptides (Moradi et al., 2016).
The mechanism of action can be modified by targeting the bacterial membranes—producing differences in different cellular lipid compositions—or non-membrane, with intracellular targets (Preethi and Anbarasu, 2022). Thus, some modifications might result increasing antimicrobial potency, like disulfide bonds (Ravishankar et al., 2016), hydrophobicity (Chen et al., 2007), conformational freedom (Li et al., 2011) and N-Methylation (Montaser et al., 2011), but there are natural antimicrobial peptides with potential activity against Mtb infection (Preethi and Anbarasu, 2022). Accordingly, many AMPs could also eliminate cancer cells, their main objective being membrane receptors differentiated from those of healthy cells. ACPs can be classified into two large groups where their main differentiation is damage against healthy mammalian cells (Papo and Shai, 2005).
Studies on the antimicrobial effect of AMPs have also indicated a possible alternative when applied synergistically with some conventional drugs, sensitizing resistant bacteria or opening the way for the entry of obsolete drugs. Thus, a sequence M(LLKK)2M in combination with rifampicin was reported to act against resistant strains, and some strategies shown by the same authors indicated that the addition of cysteines in the sequence did not affect the activity, as compared with the addition of methionines (Khara et al., 2014).
The immunomodulatory capacity of AMPs is obviously one of the most favorable factors in the treatment of an infectious or immunosuppressive disease (Jin and Weinberg, 2019). AMPs can stimulate various molecules present in the immune system, such as chemokines, to respond to disease. Naturally, the production of AMPs occurs through the stimulation of abnormal factors such as inflammation, autoimmune diseases or co-infections (Magana et al., 2020). Previous studies have shown that the use of these peptides applied as oral additives or in localized infections was able to neutralize the disease or control the spread of pathogenic agents (Molchanova et al., 2017). The use of AMP Ctx(Ile21)-Ha had a great effect against resistant Salmonella enteritidis when pH-responsible coated microparticles were applied orally in chicks (Roque-Borda et al., 2021a) and pigs or wild-type peptide in beef cattle nutrition (Silveira et al., 2021). These studies revealed that the antimicrobial peptide was able to cross the intestinal barrier and eliminate pathogenic bacteria from systemic organs (liver > spleen > intestine; Roque-Borda et al., 2022b). In addition, depending on its microencapsulating characteristics, the peptide was capable of acting against many other intestinal infectious bacteria such as Salmonella typhimurium, Salmonella enteritidis, Salmonella Heidelberg, Salmonella Infantis (isolated from symptomatic chickens), and Escherichia coli (Roque-Borda et al., 2023).
The application of AMPs in the treatment of bacterial infections would imply their use against clinical isolates, multi-and extensively drug-resistant (M or XDR) strains such as GL13K, which had excellent activity against Acinetobacter baumannii MDR and XDR (Gorr et al., 2022), or Chex1-Arg20 hydrazide, which was also effective against the formation of biofilms of the same bacterial genus (Li et al., 2022). The formation of biofilms is another major problem when opportunistic bacteria try to restrict the passage of drugs and thus prevent their action against themselves, for which they produce various compounds based on proteins, polysaccharides and exogenous DNA; this process allows the biofilm to be more aggressive and in many cases untreatable (De Pontes et al., 2022). The alpha-helical structure and beta-sheet distribution of this peptide, as well as that of other peptides, would be related to its antimicrobial activity and its broad spectrum. This is the case of Chex1-Arg20 hydrazide rich in proline (De Pontes et al., 2022). An important group of natural AMPs belongs to the bacteriocins from the innate immunity of living beings; some bacterial communities produce them to protect themselves from other invading bacteria (Simons et al., 2020).
An interesting proposal for drug discovery and design is the use of bioinformatics tools, such as molecular docking, that predict the possible sequences with the best peptide-receptor interaction. Receptors are molecules that can be present in the bacterial membrane or in some specific component generated by its own resistance and are capable of being recognized by AMPs for possible degradation or elimination (Ali et al., 2017). Some studies have shown that molecular docking followed by an in silico ADMET study would help reduce costs and study time, since its prediction would facilitate the faster discarding of inactive molecules (Rampogu et al., 2018; Xiong et al., 2021). It was shown that this tool makes it possible to obtain promising sequences for various diseases and that many of these sequences can be found within macromolecules such as proteins or enzymes. Some enzymes produced by bacteriophages intensify the selective activity against MDR bacteria, and certain AMPs were found within these structures, which after their isolation and application demonstrated excellent activity (David et al., 1980). Thus, the lysine B fraction of bacteriophage D29 was a great source of AMPs when its composition was studied in silico, and it showed a high interaction with Mtb and cancer cell-specific receptors (Snyder et al., 2018; Dulberger et al., 2020). Table 2 shows the potential characteristics of antimicrobial peptides applied against strains of M. tuberculosis and potential candidates for new drugs.
In addition to Tables 2, 3 lists recent examples of peptides with activity against other intracellular pathogens, showing that this field of study is wide and offers many candidates for new drugs.
AMPs tend to have good antimicrobial activity, but many of them have low selectivity since they interact mainly with the ionic charges of the bacterial membrane compounds (Magana et al., 2020). However, it has been reported that their structural modification could generate better antimicrobial selectivity and decrease its cytotoxicity. Table 4 shows the factors for and against AMPs, since there are several parameters to be considered for a possible scaling strategy. AMPs tend to have multiple mechanisms of action, which allows them to be membranolytic or not at the same time, an effect that can occur when AMPs are dependent on concentration, size or structure (Zhang R. et al., 2020). Alternatively, unconventional amino acids have been used to generate mimetic peptides, where hybrid combinations or unusual amino acids are used, which will generate molecules such as peptoids (α and β), β-peptides, α-peptide/β-peptoids, α/Υ N-acylated N-aminoethyl peptides (AApeptides), oligoacyllysines (OAKs), among others (Molchanova et al., 2017).
Table 4. Strengths, weaknesses, opportunities, and threats (SWOT) analysis of peptides with therapeutic properties (Fosgerau and Hoffmann, 2015).
Due to the large number of reports on antimicrobial resistance, induced by selective pressure, crossed or naturally acquired, a possible return to the pre-antibiotic era is anticipated. This would also indicate a possible reaction in response to conventional cancer treatment and a higher rate of deaths caused by opportunistic infections. In this review, we focus on an important reason for the use of multifunctional peptides or peptides with double activity and no harmful effects on humans, such as Epinecidin-1 and its analogs. These peptides had excellent antibacterial activity against various pathogens and an anticancer effect (Neshani et al., 2019). Some articles have reported the potential of AMPs and ACPs, showing that many of them were initially AMPs and were transformed into ACPs through the use of analogs (Table 5).
Some mechanisms of action based on the internalization of AMPs that are not directly related to the disruption of the membrane in cells are classified as (i) inhibitors of biosynthesis and metabolism of nucleic acids, (ii) inhibitors of biosynthesis and of protein metabolism, (iii) inhibitors of protein folding, (iv) protease inhibitors, (v) inhibitors of cell division, (vi) inhibitors of cell wall biosynthesis and (vii) binding peptides of lipopolysaccharide (Le et al., 2017). Histatins, Bactencin 7, and Apidaecin are examples that show that lipids or fatty acids can help with the translocation of AMPs within the cell membrane, which will depend on their beta or alpha-helix structure; mediated by stereospecific receptors, AMP can be located in the cytoplasm to exert their antimicrobial action (Le et al., 2017). However, this effect can limit the action of AMPs since their broad-spectrum activity can generate some type of cytotoxic or immunological response. Specifically in Mtb, the most widely used and promising strategies are focused on the search for new inducers of autophagy (Arranz-Trullén et al., 2017). Some prominent AMPs in cell permeability with intramacrophage activity against Mtb are those derived from human neutrophils, such as 1, 2, 3 (hNP1-3), lipocalin-2 and human cathelicidin, which are usually expressed when Mtb begins its extracellular multiplication (Gutsmann, 2016). These AMPs agglomerate in the bacterial cell wall, producing lesions through the formation of warts, but they also enter the cytoplasm, neutralizing bacterial DNA synthesis. Likewise, in recent years, have been reported on the multiple mechanisms of action against intramacrophagic pathogens, even Mtb with defensins, cathelicidins, hepcidin, NK-lysin, granulysin and ubiquitin, among others, demonstrating that AMPs can be selective and effective against resistant strains (Schlusselhuber et al., 2013; Baindara et al., 2016; Moussouni et al., 2019; Abraham et al., 2020; De Singulani et al., 2021).
Defensins play an important role in the innate immunology of the lung because the production of these AMPs has been reported to increase when there is colonization of Mycobacterium (Gutsmann, 2016). For example, the human β-defensins (hBD 1 or 2) can cross macrophages and phagosomes and inhibit bacterial growth. It has even been shown that their application combined with vitamin D significantly increased the inhibition percentage (Nickel et al., 2012); in the same way, prevention or prophylactic effect was achieved by combining defensins with L-isoleucine (Rivas-Santiago et al., 2015). However, it has already been demonstrated that hBD 2 can induce the proliferation of lung cancer cells through ABCG2 in a dose-and time-dependent manner (Gao et al., 2016; Ghosh et al., 2019). Laterosporulin10 is an AMP isolated from Brevibacillus sp. that has been shown to have excellent cellular permeation properties in macrophages and activity against Mtb (Baindara et al., 2016), but which at the same time induces cell apoptosis in cancer cells (HeLa cells; Baindara et al., 2017).
Some selectivity strategies have generated positive effects in relation to the hemolytic activity of the AMP and the inclusion of fatty acids is a double-edged sword since these substances can improve their antimicrobial activity in the chain but, in turn, increase their cytotoxicity or hemolytic activity. Some authors have reported that fatty acids between 8 and 10C may be more active and selective against resistant bacteria, as well as less toxic (Zhong et al., 2020). Nevertheless, these factors must be carefully studied as they depend on the hydrophobicity of the AMP and the total load that is handled. It was previously reported that the hemolytic activity and the hydrophobicity are easily predictable when the HPLC profiles are evaluated. They may be predicted by using the retention time and the % of acetonitrile, which may help to analyze the potential of different AMPs (Frederiksen et al., 2021). One of the advantages of including fatty acids is the ease of permeation of molecules within human cells. For this reason, this strategy can be initially generated with in silico molecular prediction studies with different virtual platforms. The first step would be to narrow down and select the best candidates with antimicrobial or anti-Mtb activity, as described in https://webs.iiitd.edu.in/raghava/antitbpred/ (Usmani et al., 2018), then analyze their hemolytic activity (Salem et al., 2022) and finally perform molecular docking with cell membrane proteins (Roque-Borda et al., 2023).
Another alternative would be the inclusion of cell-penetrating peptides (CPPs), making them hybrid and trying not to alter the net charge and hydrophobicity. CPPs can enter human and/or animal cells to interact with molecules internally, so many AMPs may also have permeation capacity, being able to be AMP or CPP at the same time (Xie et al., 2020). An example of hybridization was reported for Iztli Peptide 1, designed using a fraction of the Saccharomyces cerevisiae α pheromone sequence (cationic AMP) and a short CPP (without antimicrobial activity). This study showed that the hybrid AMP was capable of penetrating cells regardless of endocytosis, which paved the way for the design of new multiple-action molecules with improved cellular compatibility (Rodriguez Plaza et al., 2014). It was previously reported that the inclusion of arginines or their replacement with tryptophans could modify the behavior of AMPs by converting them into CPPs while maintaining their antimicrobial activity and their target cell selectivity (Kardani et al., 2019).
Nanotechnology as a drug delivery tool has taken on great importance during the application and transport of promising AMPs against intracellular bacteria, since many times their size and biocompatibility permeate cell membranes or easily cross them without generating an immediate immune response (Roque-Borda et al., 2022a). Nanoparticles (NP) are promising agents mostly for intramacrophage or phagosome antimicrobial action, since their construction implies the design of specific targets for their controlled release, so that large but not excessive drug concentrations can be administered (Gharatape et al., 2016). Some previous studies reported the use of polymers modified on their surface with biomacromolecules or antibodies for the recognition of proteins expressed only by tumor cells, or release conditions in slightly acidic environments that are characteristic of cancer cells (Richards et al., 2017; Marques et al., 2020). NPs can also enter infected macrophages and release AMPs to exert their action, especially when they do not have cell-penetrating characteristics (Tenland et al., 2019; Meng et al., 2023).
Lungs have historically been considered sterile in health and were initially omitted from the list of priority organ systems in the Human Microbiome Project (HMP), but subsequent studies by metagenomic analysis have since demonstrated that the lower respiratory tract is replete with diverse communities of bacteria both in health and disease (Dickson et al., 2013; Liu et al., 2020) containing about 10 to 100 bacteria per 1,000 human cells (Sze et al., 2012). Due to the importance of inflammation and cancer, the presence of microorganisms can be related to the initiation and/or progression of cancer. Some microorganisms have already been detected in patients with lung cancer. Most microorganisms colonize the tumor microenvironment, which can induce the production of inflammatory molecules (Molina-Romero et al., 2019). As shown in Table 1, some gut microbiota-related microorganisms are present in lung cancer patients.
The respiratory tract and gut can communicate with each other via some anatomical processes such as micro-aspiration and inhalation (Zhao et al., 2021). Other studies have pointed to the existence of a gut-lung axis (GLA) that can interfere with the immune responses and change the course of respiratory diseases (Enaud et al., 2020). For example, the severity of Mtb infection may be correlated with gut microbiota (Namasivayam et al., 2018). Still, some AMPs can be delivered to the gut by means of living therapies previously designed using probiotic bacteria (Geldart et al., 2016). These therapies are advantageous because probiotics help to maintain the host’s health and they are a novel approach to lung-directed treatment against TB infection (Gareau et al., 2010; Mejía-Pitta et al., 2021). Gut-related microorganisms in lung cancer can be classified into two types of relationship, namely their presence in the tumor microenvironment or a dysbiosis in the gut microbiome. Some microorganisms such as Bifidobacterium have been reported to be potential biomarkers of lung cancer, due to their decline in the gut microbiota (Zhuang et al., 2019; Zhao et al., 2021). These characteristics of the gut microbiota in lung cancer patients may affect therapy effectiveness and prognosis.
The relationship between TB and LC is the subject of much debate. The chronic inflammation caused by the colonization of active Mtb can be one of the mechanisms increasing the probability of developing this carcinoma. Chronic inflammation caused by this infection has been linked to cell dysplasia, as well as squamous LC (Broussard et al., 2009; Skowroński et al., 2015). LC can be promoted by persistent local inflammation, which contributes to damage of the pulmonary epithelium, and the cytokines released can induce the proliferation of lung epithelial cells (Ballaz and Mulshine, 2003). The main reason for describing this relationship is the development of carcinoma from TB scars (Brenner et al., 2011; Cukic, 2017). This relationship may be based on some mechanisms and interactions. For example, TB-related chronic inflammation can induce genetic mutations (Vento and Lanzafame, 2011). A close association between metastatic squamous cell carcinoma (SCC) and TB lesions has been demonstrated (Nalbandian et al., 2009). Lung parenchyma is involved in both TB and LC (Bhatt et al., 2012). The production of inflammatory cytokines can be related to cancer development (Cicėnas and Vencevičius, 2007; Engels et al., 2009). The development of the granulomas in an Mtb infection may generate a microenvironment predisposed to malignant transformation. This microenvironment may lead to changes in the epithelium of the lung caverns, calcified lymph nodes and old scars in the bronchi (Cukic, 2017). Still, the pneumocytes are infected and activated, with the induction of autophagy, secretion of pro-inflammatory mediators and cell death as outcomes (Rodrigues et al., 2020). Polverino et al. (2016) demonstrated that ligands of proliferation are more highly expressed in patients with both chronic inflammation and non-small lung cancer cells than in patients with only chronic inflammation or cancer. The expression of these ligands can be related to the progression of both, inflammation and cancer (Polverino et al., 2016).
It is important to note that cancer and TB can mimic each other at clinical, biological and radiological levels. This can be hazardous for the patient. A patient with LC but diagnosed with TB will receive chemotherapy to no effect. In fact, this therapy would deteriorate the patient’s response. On the other hand, receiving treatment for TB when the patient has cancer could lead to a complication and eventual death of the patient. Even in a concomitant condition, when a patient is correctly diagnosed with LC but also has TB, the healing process can be complicated (Malik et al., 2022).
Some reports have indicated that AMPs would be able to recognize different cancer cell receptors for efficient molecular targeting (Figure 2). Therefore, they could be a relevant alternative to the use of AMPs, for application against cancer and could be a relevant alternative against bacteria (Zhang et al., 2019).
Figure 2. Some anticancer mechanisms of antimicrobial peptides with known activity against Mycobacterium tuberculosis. The figure was created by Biorender.com, partly generated using Servier Medical Art, provided by Servier, licensed under a Creative Commons Attribution 3.0 unported license.
Kunda (2020) compiled different applications of these peptides against an unchained proliferation of non-small cell LC, because AMPs can develop similar mechanisms of action against bacteria; these peptides may interact with negatively charged molecules of cancer cells such as phosphatidylserines, negative glycoproteins and glycosaminoglycans, i.e., highly expressed in the presence of oncocells. One of the main problems with the use of AMPs in the treatment of cancer is their cellular non-specificity, which could damage healthy cells at the same time (Hilchie et al., 2019). However, there are already some strategies to improve the peptide sequence by obtaining specific target analogs that could generate better action and fewer side effects (Yang et al., 2022). Table 6 shows the main AMPs that have been relevant in the last three years, with potential anti-Mtb and anticancer activity. The results show that few studies have proven the activity for both diseases, but it is possible to obtain drugs with double function and activity.
Derived by the endogenous cationic peptide Brevinin-1 family, the B1CTcu5, of amphibian origin, was able to inhibit Mtb in culture, as well as intracellular conditions, by permeabilizing the thick cell wall of Mtb to impart bactericidal activity without causing any damage to the macrophages. B1CTcu5 can mimic the environment by adopting an alpha-helical conformation in the membrane. This characteristic contributes to the activity against prototype Gram-negative and Gram-positive bacteria. The hydrophobic interaction between amphipathic AMP and the cell membrane makes a specific peptide–lipid complex, which may produce alterations in the bacterial membrane, such as thinning, pore formation, altered curvature and localized perturbations (Abraham et al., 2020).
A recent example is LL-37, an endogenous AMP derived from Cathelicidin. It is also an endogenous cationic peptide expressed in human immune cells, with activity against extra and intracellular Mtb, mainly through the disruption of the bacterial cell wall upon binding, causing disintegration and rapid rupture within minutes. Moreover, other results the same group showed that LL-37 is internalized by macrophages and localized within the membrane of early endosomes labeled with antigen 1 protein (Deshpande et al., 2020). Other findings suggested that by “nonclassical” mechanisms, vitamin D contributes to protection against TB and can act in combination with LL-37 (Martineau et al., 2007). For lung cancer, studies have shown that LL-37 increased tumorigenicity and significantly larger tumor mass in human lung cancer cells (von Haussen et al., 2008), but analogs have significantly improved cytotoxicity against cancer cell line A549 cells (Tzitzilis et al., 2020).
Finally, in addition to the activities mentioned in Table 6, a recent study suggests a combination of amphipathic α-helical N-terminal region of cecropin A and hydrophobic N-terminal of the bee venom melittin, known as CP26. This combination is a 26-amino acid long β-helical peptide with activity against both susceptible (H37Rv) and MDR strains of Mtb (Rivas-Santiago et al., 2013), but this combination of peptides has not yet been studied against lung cancer.
The antimicrobial peptides can also be designed to combat bacteria and cancer cells through re-engineering into anticancer peptides. Both bacteria and cancer cells possess an electronegative surface that the peptides can break because they are cationic amphiphiles. Aronson et al. (2020) tested this hypothesis with an AMP originally designed to kill M. tuberculosis and found a powerful result against ovarian cancer, opening new paths to study alternatives that are also potent against lung cancer (Aronson et al., 2020).
The most debated hypothesis is that an Mtb infection can trigger such a complex inflammatory environment in the lung that it unintentionally induces normal cells to differentiate into cancer cells, initiating lung cancer. Many researchers are studying this link, but more experimental data are needed to establish a clear mechanism between the diseases, especially through the study of clinical-human samples. A few other researchers suggest the opposite, namely that there is a predisposition to Mtb infection precisely because of a depressed immune system and tissue affected by the growth of cancer cells, but these studies have not found an accurate mechanism proving this relationship.
Regardless of the disease that initially affected the patient, diagnostic methods for differentiating them are extremely necessary because they have similar symptoms, confusing and/or masking the secondary disease, which, in turn, can go untreated or treated incorrectly, thus worsening the clinical scenario. In addition, there is a need for new treatment alternatives that combat both diseases developed in the same organ, so that there is no unnecessary overlapping of drugs, causing greater toxicity or even antagonistic effects.
We report on four alternatives of antimicrobial peptides with current relevance, which were able to show activity against the mycobacteria as well as against lung cancer. Examples of peptides from the same class with action on TB and LC have been presented. However, no derivative or analog has yet been found that manages to act on both diseases for concomitant treatment, which opens up a field of study with infinite possibilities for alterations and combinations. These alternatives need more investment and further studies to prove the dual activity and develop dual-function drugs that minimize the abandonment of therapy when pulmonary diseases coexist.
GP: bibliographic survey, bibliography analysis, discussion, and manuscript elaboration. LP, MR, FD, and PB: bibliographic survey and manuscript elaboration. CR-B and FP: supervision, idea, bibliography analysis, discussion, and manuscript elaboration. All authors contributed to the article and approved the submitted version.
This study was supported by the São Paulo Research Foundation (FAPESP): Research Grant: 2020/13497-4, 2022/09728-6, 2021/14603-5 and 2020/16573-3. National Council for Scientific and Technological Development (CNPq): Productivity Research Fellows (PQ CNPq): 305408/2022-4. This study was financed in part by the Coordenação de Aperfeiçoamento de Pessoal de Nível Superior - Brasil (CAPES) - Finance Code 001.
The authors declare that the research was conducted in the absence of any commercial or financial relationships that could be construed as a potential conflict of interest.
All claims expressed in this article are solely those of the authors and do not necessarily represent those of their affiliated organizations, or those of the publisher, the editors and the reviewers. Any product that may be evaluated in this article, or claim that may be made by its manufacturer, is not guaranteed or endorsed by the publisher.
Abdeahad, H., Salehi, M., Yaghoubi, A., Aalami, A. H., Aalami, F., and Soleimanpour, S. (2022). Previous pulmonary tuberculosis enhances the risk of lung cancer: systematic reviews and meta-analysis. Infect. Dis. 54, 255–268. doi: 10.1080/23744235.2021.2006772
Abraham, P., Jose, L., Maliekal, T. T., Kumar, R. A., and Kumar, K. S. (2020). B1CTcu5: A frog-derived brevinin-1 peptide with anti-tuberculosis activity. Peptides 132:170373. doi: 10.1016/j.peptides.2020.170373
Ahmad, F., Rani, A., Alam, A., Zarin, S., Pandey, S., Singh, H., et al. (2022). Macrophage: A cell with many faces and functions in tuberculosis. Front. Immunol. 13:747799. doi: 10.3389/fimmu.2022.747799
Alhakamy, N. A., Okbazghi, S. Z., Alfaleh, M. A., Abdulaal, W. H., Bakhaidar, R. B., Alselami, M. O., et al. (2022). Wasp venom peptide improves the proapoptotic activity of alendronate sodium in A549 lung cancer cells. PLoS One 17:e0264093. doi: 10.1371/journal.pone.0264093
Ali, M. R., Sadoqi, M., Møller, S. G., Boutajangout, A., and Mezei, M. (2017). Assessing the binding of cholinesterase inhibitors by docking and molecular dynamics studies. J. Mol. Graph. Model. 76, 36–42. doi: 10.1016/j.jmgm.2017.06.027
Alonso, S., Pethe, K., Russell, D. G., and Purdy, G. E. (2007). Lysosomal killing of Mycobacterium mediated by ubiquitin-derived peptides is enhanced by autophagy. Proc. Natl. Acad. Sci. 104, 6031–6036. doi: 10.1073/pnas.0700036104
Altorki, N. K., Markowitz, G. J., Gao, D., Port, J. L., Saxena, A., Stiles, B., et al. (2019). The lung microenvironment: an important regulator of tumour growth and metastasis. Nat. Rev. Cancer 19, 9–31. doi: 10.1038/s41568-018-0081-9
American Cancer Society. (2019). Lung Cancer. Available at: https://www.cancer.org/cancer/lung-cancer.html (Accessed January 03, 2023).
Ankrah, A. O., Glaudemans, A. W. J. M., Maes, A., Van de Wiele, C., Dierckx, R. A. J. O., Vorster, M., et al. (2018). Tuberculosis. Semin. Nucl. Med. 48, 108–130. doi: 10.1053/j.semnuclmed.2017.10.005
Aronson, M. R., Dahl, E. S., Halle, J. A., Simonson, A. W., Gogal, R. A., Glick, A. B., et al. (2020). Re-engineering antimicrobial peptides into Oncolytics targeting drug-resistant ovarian cancers. Cell. Mol. Bioeng. 13, 447–461. doi: 10.1007/s12195-020-00626-z
Arranz-Trullén, J., Lu, L., Pulido, D., Bhakta, S., and Boix, E. (2017). Host antimicrobial peptides: the promise of new treatment strategies against tuberculosis. Front. Immunol. 8:1499. doi: 10.3389/fimmu.2017.01499
Arrieta, O., Molina-Romero, C., Cornejo-Granados, F., Marquina-Castillo, B., Avilés-Salas, A., López-Leal, G., et al. (2022). Clinical and pathological characteristics associated with the presence of the IS6110 Mycobacterim tuberculosis transposon in neoplastic cells from non-small cell lung cancer patients. Sci. Rep. 12:2210. doi: 10.1038/s41598-022-05749-z
Baba, I., Shirasawa, S., Iwamoto, R., Okumura, K., Tsunoda, T., Nishioka, M., et al. (2000). Involvement of deregulated epiregulin expression in tumorigenesis in vivo through activated Ki-Ras signaling pathway in human colon cancer cells. Cancer Res. 60, 6886–6889.
Baindara, P., Gautam, A., Raghava, G. P. S., and Korpole, S. (2017). Anticancer properties of a defensin like class IId bacteriocin Laterosporulin 10. Sci. Rep. 7:46541. doi: 10.1038/srep46541
Baindara, P., Singh, N., Ranjan, M., Nallabelli, N., Chaudhry, V., Pathania, G. L., et al. (2016). Laterosporulin 10: a novel defensin like class IId bacteriocin from Brevibacillus sp. strain SKDU10 with inhibitory activity against microbial pathogens. Microbiology 162, 1286–1299. doi: 10.1099/mic.0.000316
Balkwill, F., and Mantovani, A. (2001). Inflammation and cancer: back to Virchow? Lancet 357, 539–545. doi: 10.1016/S0140-6736(00)04046-0
Ballaz, S., and Mulshine, J. L. (2003). The potential contributions of chronic inflammation to lung carcinogenesis. Clin. Lung Cancer 5, 46–62. doi: 10.3816/CLC.2003.n.021
Bhatt, M., Kant, S., and Bhaskar, R. (2012). Pulmonary tuberculosis as differential diagnosis of lung cancer. South Asian J. Cancer 01, 36–42. doi: 10.4103/2278-330X.96507
Bloom, B. R., and Murray, C. J. L. (1992). Tuberculosis: commentary on a reemergent killer. Science 257, 1055–1064. doi: 10.1126/science.257.5073.1055
Brenner, D. R., McLaughlin, J. R., and Hung, R. J. (2011). Previous lung diseases and lung Cancer risk: a systematic review and Meta-analysis. PLoS One 6:e17479. doi: 10.1371/journal.pone.0017479
Broussard, G. W., Norris, M. B., Schwindt, A. R., Fournie, J. W., Winn, R. N., Kent, M. L., et al. (2009). Chronic Mycobacterium marinum infection acts as a tumor promoter in Japanese Medaka (Oryzias latipes). Comp. Biochem. Physiol. Part C Toxicol. Pharmacol. 149, 152–160. doi: 10.1016/j.cbpc.2008.09.011
Cameron, S. J. S., Lewis, K. E., Huws, S. A., Hegarty, M. J., Lewis, P. D., Pachebat, J. A., et al. (2017). A pilot study using metagenomic sequencing of the sputum microbiome suggests potential bacterial biomarkers for lung cancer. PLoS One 12:e0177062. doi: 10.1371/journal.pone.0177062
Cao, S., Li, J., Lu, J., Zhong, R., and Zhong, H. (2019). Mycobacterium tuberculosis antigens repress Th1 immune response suppression and promotes lung cancer metastasis through PD-1/PDl-1 signaling pathway. Cell Death Dis. 10:44. doi: 10.1038/s41419-018-1237-y
Chai, Q., Lu, Z., Liu, Z., Zhong, Y., Zhang, F., Qiu, C., et al. (2020). Lung gene expression signatures suggest pathogenic links and molecular markers for pulmonary tuberculosis, adenocarcinoma and sarcoidosis. Commun. Biol. 3:604. doi: 10.1038/s42003-020-01318-0
Chaitanya Thandra, K., Barsouk, A. A., Saginala, K., Sukumar Aluru, J., and Barsouk, A. A. (2021). Epidemiology of lung cancer. Współczesna Onkol. 25, 45–52. doi: 10.5114/wo.2021.103829
Chaudhary, S., Ali, Z., Tehseen, M., Haney, E. F., Pantoja-Angles, A., Alshehri, S., et al. (2023). Efficient in planta production of amidated antimicrobial peptides that are active against drug-resistant ESKAPE pathogens. Nat. Commun. 14:1464. doi: 10.1038/s41467-023-37003-z
Cheah, Y.-H., Liu, C.-Y., Yip, B.-S., Wu, C.-L., Peng, K.-L., and Cheng, J.-W. (2022). Strategy to enhance anticancer activity and induced immunogenic cell death of antimicrobial peptides by using non-nature amino acid substitutions. Biomedicine 10:1097. doi: 10.3390/biomedicines10051097
Chen, Y., Guarnieri, M. T., Vasil, A. I., Vasil, M. L., Mant, C. T., and Hodges, R. S. (2007). Role of peptide hydrophobicity in the mechanism of action of α-helical antimicrobial peptides. Antimicrob. Agents Chemother. 51, 1398–1406. doi: 10.1128/AAC.00925-06
Chen, J., Zhang, C.-Y., Chen, J.-Y., Seah, R. W. X., Zhang, L., Ma, L., et al. (2023). Host defence peptide LEAP2 contributes to antimicrobial activity in a mustache toad (Leptobrachium liui). BMC Vet. Res. 19:47. doi: 10.1186/s12917-023-03606-3
Chen, X., Zou, X., Qi, G., Tang, Y., Guo, Y., Si, J., et al. (2018). Roles and mechanisms of human cathelicidin LL-37 in Cancer. Cell. Physiol. Biochem. 47, 1060–1073. doi: 10.1159/000490183
Cicėnas, S., and Vencevičius, V. (2007). Lung cancer in patients with tuberculosis. World J. Surg. Oncol. 5:22. doi: 10.1186/1477-7819-5-22
Coussens, L. M., and Werb, Z. (2002). Inflammation and cancer. Nature 420, 860–867. doi: 10.1038/nature01322
Coyotl, E. A. P., Palacios, J. B., Muciño, G., Moreno-Blas, D., Costas, M., Montes, T. M., et al. (2020). Antimicrobial peptide against mycobacterium tuberculosis that activates autophagy is an effective treatment for tuberculosis. Pharmaceutics 12, 1–24. doi: 10.3390/pharmaceutics12111071
Cronan, M. R., Beerman, R. W., Rosenberg, A. F., Saelens, J. W., Johnson, M. G., Oehlers, S. H., et al. (2016). Macrophage epithelial reprogramming underlies mycobacterial granuloma formation and promotes infection. Immunity 45, 861–876. doi: 10.1016/j.immuni.2016.09.014
Cukic, V. (2017). The association between lung carcinoma and tuberculosis. Med. Arch. 71:212. doi: 10.5455/medarh.2017.71.212-214
Da Costa de Souza, G., Roque-Borda, C. A., and Pavan, F. R. (2022). Beta-lactam resistance and the effectiveness of antimicrobial peptides against KPC-producing bacteria. Drug Dev. Res. 83, 1534–1554. doi: 10.1002/ddr.21990
Daletos, G., Kalscheuer, R., Koliwer-Brandl, H., Hartmann, R., de Voogd, N. J., Wray, V., et al. (2015). Callyaerins from the marine sponge Callyspongia aerizusa: cyclic peptides with Antitubercular activity. J. Nat. Prod. 78, 1910–1925. doi: 10.1021/acs.jnatprod.5b00266
David, H., Clavel, S., and Clement, F. (1980). Adsorption and growth of the bacteriophage D29 in selected mycobacteria. Ann. l’Institut Pasteur/Virol. 131, 167–184. doi: 10.1016/0769-2617(80)90031-3
De Castro, G. Jr., Harada, G., and De Mello, E. S. (2019). The importance of molecular characterization in lung cancer. J. Bras. Pneumol. 45:139. doi: 10.1590/1806-3713/e20190139
De Pontes, J. T. C., Toledo Borges, A. B., Roque-Borda, C. A., and Pavan, F. R. (2022). Antimicrobial peptides as an alternative for the eradication of bacterial biofilms of multi-drug resistant Bacteria. Pharmaceutics 14:642. doi: 10.3390/pharmaceutics14030642
De Singulani, J. L., Oliveira, L. T., Ramos, M. D., Fregonezi, N. F., Gomes, P. C., Galeane, M. C., et al. (2021). The antimicrobial peptide MK58911-NH 2 acts on planktonic, biofilm, and Intramacrophage cells of Cryptococcus neoformans. Antimicrob. Agents Chemother. 65:21. doi: 10.1128/AAC.00904-21
Deshpande, D., Grieshober, M., Wondany, F., Gerbl, F., Noschka, R., Michaelis, J., et al. (2020). Super-resolution microscopy reveals a direct interaction of intracellular Mycobacterium tuberculosis with the antimicrobial peptide LL-37. Int. J. Mol. Sci. 21:6741. doi: 10.3390/ijms21186741
Dickson, R. P., Erb-Downward, J. R., and Huffnagle, G. B. (2013). The role of the bacterial microbiome in lung disease. Expert Rev. Respir. Med. 7, 245–257. doi: 10.1586/ers.13.24
Dobler, C. C., Cheung, K., Nguyen, J., and Martin, A. (2017). Risk of tuberculosis in patients with solid cancers and haematological malignancies: a systematic review and meta-analysis. Eur. Respir. J. 50:1700157. doi: 10.1183/13993003.00157-2017
Dong, Z., Yin, Y., Luo, J., Li, B., Lou, F., Wang, Q., et al. (2022). An FGFR1-binding peptide modified liposome for si RNA delivery in lung Cancer. Int. J. Mol. Sci. 23:8380. doi: 10.3390/ijms23158380
Drab, E., and Sugihara, K. (2020). Cooperative function of LL-37 and HNP1 protects mammalian cell membranes from lysis. Biophys. J. 119, 2440–2450. doi: 10.1016/j.bpj.2020.10.031
Du, Y., Lin, X., Feng, Q., Pan, X., Song, S., and Yang, J. (2022). Inhibition of human lung cancer cells by anti-p21Ras sc Fv mediated by the activatable cell-penetrating peptide. Anti-Cancer Drugs 33, e562–e572. doi: 10.1097/CAD.0000000000001180
Dulberger, C. L., Rubin, E. J., and Boutte, C. C. (2020). The mycobacterial cell envelope — a moving target. Nat. Rev. Microbiol. 18, 47–59. doi: 10.1038/s41579-019-0273-7
Enaud, R., Prevel, R., Ciarlo, E., Beaufils, F., Wieërs, G., Guery, B., et al. (2020). The gut-lung Axis in health and respiratory diseases: A place for inter-organ and inter-kingdom Crosstalks. Front. Cell. Infect. Microbiol. 10:9. doi: 10.3389/fcimb.2020.00009
Engels, E. A., Shen, M., Chapman, R. S., Pfeiffer, R. M., Yu, Y.-Y., He, X., et al. (2009). Tuberculosis and subsequent risk of lung cancer in Xuanwei. China. Int. J. Cancer 124, 1183–1187. doi: 10.1002/ijc.24042
Fleming, A. (1922). On a remarkable bacteriolytic element found in tissues and secretions. Proceedings of the Royal Society of London. Series B, Containing Papers of a Biological Character, 93, 306–317.
Fosgerau, K., and Hoffmann, T. (2015). Peptide therapeutics: current status and future directions. Drug Discov. Today 20, 122–128. doi: 10.1016/j.drudis.2014.10.003
Frederiksen, N., Louka, S., Mudaliar, C., Domraceva, I., Kreicberga, A., Pugovics, O., et al. (2021). Peptide/β-Peptoid hybrids with ultrashort PEG-like moieties: effects on hydrophobicity, antibacterial activity and hemolytic properties. Int. J. Mol. Sci. 22:7041. doi: 10.3390/ijms22137041
Gao, C., Yue, W., Tian, H., Li, L., Li, S., and Si, L. (2016). Human beta-defensin 2 promotes the proliferation of lung cancer cells through ATP-binding cassette transporter G2. Int. J. Clin. Exp. Pathol. 9, 5944–5949.
Gareau, M. G., Sherman, P. M., and Walker, W. A. (2010). Probiotics and the gut microbiota in intestinal health and disease. Nat. Rev. Gastroenterol. Hepatol. 7, 503–514. doi: 10.1038/nrgastro.2010.117
Gazdar, A. F., Bunn, P. A., and Minna, J. D. (2017). Small-cell lung cancer: what we know, what we need to know and the path forward. Nat. Rev. Cancer 17, 725–737. doi: 10.1038/nrc.2017.87
Geldart, K., Forkus, B., McChesney, E., McCue, M., and Kaznessis, Y. (2016). pMPES: A modular peptide expression system for the delivery of antimicrobial peptides to the site of gastrointestinal infections using probiotics. Pharmaceuticals 9:60. doi: 10.3390/ph9040060
George, P. J., Pavan Kumar, N., Jaganathan, J., Dolla, C., Kumaran, P., Nair, D., et al. (2015). Modulation of pro-and anti-inflammatory cytokines in active and latent tuberculosis by coexistent Strongyloides stercoralis infection. Tuberculosis 95, 822–828. doi: 10.1016/j.tube.2015.09.009
Gharatape, A., Davaran, S., Salehi, R., and Hamishehkar, H. (2016). Engineered gold nanoparticles for photothermal cancer therapy and bacteria killing. RSC Adv. 6, 111482–111516. doi: 10.1039/C6RA18760A
Ghavami, S., Asoodeh, A., Klonisch, T., Halayko, A. J., Kadkhoda, K., Kroczak, T. J., et al. (2008). Brevinin-2R1 semi-selectively kills cancer cells by a distinct mechanism, which involves the lysosomal-mitochondrial death pathway. J. Cell. Mol. Med. 12, 1005–1022. doi: 10.1111/j.1582-4934.2008.00129.x
Ghosh, S. K., McCormick, T. S., and Weinberg, A. (2019). Human Beta Defensins and Cancer: contradictions and common ground. Front. Oncol. 9:341. doi: 10.3389/fonc.2019.00341
Gleeson, L. E., Sheedy, F. J., Palsson-McDermott, E. M., Triglia, D., O’Leary, S. M., O’Sullivan, M. P., et al. (2016). Cutting edge: Mycobacterium tuberculosis induces aerobic glycolysis in human alveolar macrophages that is required for control of intracellular bacillary replication. J. Immunol. 196, 2444–2449. doi: 10.4049/jimmunol.1501612
Globocan (2020). Number of new cases in 2020, both sexes, all ages. Glob. Cancer Obs. Available at: https://gco.iarc.fr/today (Accessed January 03, 2023).
Gomez Rodriguez, Y., Oliva Arguelles, B., Riera-Romo, M., Fernandez-De-Cossio, J., Garay, H. E., Fernandez Masso, J., et al. (2022). Synergic effect of anticancer peptide CIGB-552 and cisplatin in lung cancer models. Mol. Biol. Rep. 49, 3197–3212. doi: 10.1007/s11033-022-07152-3
Gorr, S.-U., Brigman, H. V., Anderson, J. C., and Hirsch, E. B. (2022). The antimicrobial peptide DGL13K is active against drug-resistant gram-negative bacteria and sub-inhibitory concentrations stimulate bacterial growth without causing resistance. PLoS One 17:e0273504. doi: 10.1371/journal.pone.0273504
Gradmann, C. (2006). Robert Koch and the white death: from tuberculosis to tuberculin. Microbes Infect. 8, 294–301. doi: 10.1016/j.micinf.2005.06.004
Greathouse, K. L., White, J. R., Vargas, A. J., Bliskovsky, V. V., Beck, J. A., von Muhlinen, N., et al. (2018). Interaction between the microbiome and TP53 in human lung cancer. Genome Biol. 19:123. doi: 10.1186/s13059-018-1501-6
Gupta, P. K., Tripathi, D., Kulkarni, S., and Rajan, M. G. R. (2016). Mycobacterium tuberculosis H37Rv infected THP-1 cells induce epithelial mesenchymal transition (EMT) in lung adenocarcinoma epithelial cell line (A549). Cell. Immunol. 300, 33–40. doi: 10.1016/j.cellimm.2015.11.007
Gusman, H., Travis, J., Helmerhorst, E. J., Potempa, J., Troxler, R. F., and Oppenheim, F. G. (2001). Salivary Histatin 5 is an inhibitor of both host and bacterial enzymes implicated in periodontal disease. Infect. Immun. 69, 1402–1408. doi: 10.1128/IAI.69.3.1402-1408.2001
Gutsmann, T. (2016). Interaction between antimicrobial peptides and mycobacteria. Biochim. Biophys. Acta-Biomembr. 1858, 1034–1043. doi: 10.1016/j.bbamem.2016.01.031
Hajisharifi, Z., Piryaiee, M., Mohammad Beigi, M., Behbahani, M., and Mohabatkar, H. (2014). Predicting anticancer peptides with Chou′s pseudo amino acid composition and investigating their mutagenicity via Ames test. J. Theor. Biol. 341, 34–40. doi: 10.1016/j.jtbi.2013.08.037
Han, F.-F., Liu, Y.-F., Xie, Y.-G., Gao, Y.-H., Luan, C., and Wang, Y.-Z. (2011). Antimicrobial peptides derived from different animals: comparative studies of antimicrobial properties, cytotoxicity and mechanism of action. World J. Microbiol. Biotechnol. 27, 1847–1857. doi: 10.1007/s11274-010-0643-9
Hanahan, D., and Weinberg, R. A. (2011). Hallmarks of cancer: the next generation. Cells 144, 646–674. doi: 10.1016/j.cell.2011.02.013
Hassan, R. R., Abdulaqadir, S. Z., Rashid, R. F., and Hassan, A. O. (2021). In vitro experiments of prokaryotic and eukaryotic antimicrobial peptide cytotoxicity in intestinal epithelial cells. Int. J. Hortic. Agric. Food Sci. 5, 06–14. doi: 10.22161/ijhaf.5.6.2
Heitmann, L., Abad Dar, M., Schreiber, T., Erdmann, H., Behrends, J., Mckenzie, A. N., et al. (2014). The IL −13/ IL-4R α axis is involved in tuberculosis-associated pathology. J. Pathol. 234, 338–350. doi: 10.1002/path.4399
Henao Arias, D. C., Toro, L. J., Téllez Ramirez, G. A., Osorio-Méndez, J. F., Rodríguez-Carlos, A., Valle, J., et al. (2021). Novel antimicrobial cecropins derived from O. curvicornis and D. satanas dung beetles. Peptides 145:170626. doi: 10.1016/j.peptides.2021.170626
Hilchie, A. L., Hoskin, D. W., and Power Coombs, M. R. (2019). Anticancer activities of natural and synthetic peptides. Adv. Exp. Med. Biol., 131–147. doi: 10.1007/978-981-13-3588-4_9
Hofman, P., and Vouret-Craviari, V. (2012). Microbes-induced EMT at the crossroad of inflammation and cancer. Gut Microbes 3, 176–185. doi: 10.4161/gmic.20288
Horváti, K., Bacsa, B., Mlinkó, T., Szabó, N., Hudecz, F., Zsila, F., et al. (2017). Comparative analysis of internalisation, haemolytic, cytotoxic and antibacterial effect of membrane-active cationic peptides: aspects of experimental setup. Amino Acids 49, 1053–1067. doi: 10.1007/s00726-017-2402-9
Hosgood, H. D., Sapkota, A. R., Rothman, N., Rohan, T., Hu, W., Xu, J., et al. (2014). The potential role of lung microbiota in lung cancer attributed to household coal burning exposures. Environ. Mol. Mutagen. 55, 643–651. doi: 10.1002/em.21878
Hwang, I. K., Paik, S. S., and Lee, S. H. (2019). Impact of pulmonary tuberculosis on the EGFR mutational status and clinical outcome in patients with lung adenocarcinoma. Cancer Res. Treat. 51, 158–168. doi: 10.4143/crt.2018.084
Ibrahim, H. R., Imazato, K., and Ono, H. (2011). Human lysozyme possesses novel antimicrobial peptides within its N-terminal domain that target bacterial respiration. J. Agric. Food Chem. 59, 10336–10345. doi: 10.1021/jf2020396
Ikonomova, S. P., Moghaddam-Taaheri, P., Wang, Y., Doolin, M. T., Stroka, K. M., Hube, B., et al. (2020). Effects of histatin 5 modifications on antifungal activity and kinetics of proteolysis. Protein Sci. 29, 480–493. doi: 10.1002/pro.3767
Jett, J. R., and Carr, L. L. (2013). Targeted therapy for non–small cell lung Cancer. Am. J. Respir. Crit. Care Med. 188, 907–912. doi: 10.1164/rccm.201301-0189PP
Jiang, M., Fang, X., Ma, L., Liu, M., Chen, M., Liu, J., et al. (2022). A nucleus-targeting peptide antagonist towards EZH2 displays therapeutic efficacy for lung cancer. Int. J. Pharm. 622:121894. doi: 10.1016/j.ijpharm.2022.121894
Jiang, Y., Huang, W., Sun, X., Yang, X., Wu, Y., Shi, J., et al. (2022). DTX-P7, a peptide–drug conjugate, is highly effective for non-small cell lung cancer. J. Hematol. Oncol. 15:73. doi: 10.1186/s13045-022-01274-8
Jin, G., and Weinberg, A. (2019). Human antimicrobial peptides and cancer. Semin. Cell Dev. Biol. 88, 156–162. doi: 10.1016/j.semcdb.2018.04.006
Kaewjanthong, P., Sooksai, S., Sasano, H., Hutvagner, G., Bajan, S., McGowan, E., et al. (2022). Cell-penetrating peptides containing the progesterone receptor polyproline domain inhibits EGF signaling and cell proliferation in lung cancer cells. PLoS One 17:e0264717. doi: 10.1371/journal.pone.0264717
Kalita, A., Verma, I., and Khuller, G. K. (2004). Role of human neutrophil peptide–1 as a possible adjunct to Antituberculosis chemotherapy. J. Infect. Dis. 190, 1476–1480. doi: 10.1086/424463
Kardani, K., Milani, A., Shabani, H. S., and Bolhassani, A. (2019). Cell penetrating peptides: the potent multi-cargo intracellular carriers. Expert Opin. Drug Deliv. 16, 1227–1258. doi: 10.1080/17425247.2019.1676720
Khara, J. S., Wang, Y., Ke, X.-Y., Liu, S., Newton, S. M., Langford, P. R., et al. (2014). Anti-mycobacterial activities of synthetic cationic α-helical peptides and their synergism with rifampicin. Biomaterials 35, 2032–2038. doi: 10.1016/j.biomaterials.2013.11.035
Kiri, V. A., Soriano, J. B., Visick, G., and Fabbri, L. M. (2009). Recent trends in lung cancer and its association with COPD: an analysis using the UK GP research database. Prim. Care Respir. J. 19, 57–61. doi: 10.4104/pcrj.2009.00048
Kunda, N. K. (2020). Antimicrobial peptides as novel therapeutics for non-small cell lung cancer. Drug Discov. Today 25, 238–247. doi: 10.1016/j.drudis.2019.11.012
Laroumagne, S., Salinas-Pineda, A., Hermant, C., Murris, M., Gourraud, P.-A., Do, C., et al. (2011). Incidence et caractéristiques des colonisations des voies respiratoires lors du diagnostic de cancer bronchique: étude rétrospective de 388 cas. Rev. Mal. Respir. 28, 328–335. doi: 10.1016/j.rmr.2010.05.020
Le, C.-F., Fang, C.-M., and Sekaran, S. D. (2017). Intracellular targeting mechanisms by antimicrobial peptides. Antimicrob. Agents Chemother. 61:16. doi: 10.1128/AAC.02340-16
Li, W., Lin, F., Hung, A., Barlow, A., Sani, M.-A., Paolini, R., et al. (2022). Enhancing proline-rich antimicrobial peptide action by homodimerization: influence of bifunctional linker. Chem. Sci. 13, 2226–2237. doi: 10.1039/D1SC05662J
Li, Y.-B., Xie, Y.-Y., Du, N.-N., Lu, Y., Xu, H.-Z., Wang, B., et al. (2011). Synthesis and in vitro antitubercular evaluation of novel sansanmycin derivatives. Bioorg. Med. Chem. Lett. 21, 6804–6807. doi: 10.1016/j.bmcl.2011.09.031
Liang, H.-Y., Li, X.-L., Yu, X.-S., Guan, P., Yin, Z.-H., He, Q.-C., et al. (2009). Facts and fiction of the relationship between preexisting tuberculosis and lung cancer risk: a systematic review. Int. J. Cancer 125, 2936–2944. doi: 10.1002/ijc.24636
Liu, N.-N., Ma, Q., Ge, Y., Yi, C.-X., Wei, L.-Q., Tan, J.-C., et al. (2020). Microbiome dysbiosis in lung cancer: from composition to therapy. NPJ Precis Oncologia 4:33. doi: 10.1038/s41698-020-00138-z
Lu, J., Xu, H., Xia, J., Ma, J., Xu, J., Li, Y., et al. (2020). D-and unnatural amino acid substituted antimicrobial peptides with improved proteolytic resistance and their proteolytic degradation characteristics. Front. Microbiol. 11:3030. doi: 10.3389/fmicb.2020.563030
Lungu, P. S., Kilembe, W., Lakhi, S., Sukwa, T., Njelesani, E., Zumla, A. I., et al. (2022). A comparison of vitamin D and cathelicidin (LL-37) levels between patients with active TB and their healthy contacts in a high HIV prevalence setting: a prospective descriptive study. Trans. R. Soc. Trop. Med. Hyg. 116, 336–343. doi: 10.1093/trstmh/trab126
Macintyre, A. N., and Rathmell, J. C. (2013). Activated lymphocytes as a metabolic model for carcinogenesis. Cancer Metab. 1:5. doi: 10.1186/2049-3002-1-5
Madeddu, C., Donisi, C., Liscia, N., Lai, E., Scartozzi, M., and Macciò, A. (2022). EGFR-mutated non-small cell lung Cancer and resistance to immunotherapy: role of the tumor microenvironment. Int. J. Mol. Sci. 23:6489. doi: 10.3390/ijms23126489
Maeda, H., and Akaike, T. (1998). Nitric oxide and oxygen radicals in infection, inflammation, and cancer. Biochemistry (Mosc) 63, 854–865.
Magana, M., Pushpanathan, M., Santos, A. L., Leanse, L., Fernandez, M., Ioannidis, A., et al. (2020). The value of antimicrobial peptides in the age of resistance. Lancet Infect. Dis. 20, e216–e230. doi: 10.1016/S1473-3099(20)30327-3
Malik, A. A., Sheikh, J. A., Ehtesham, N. Z., Hira, S., Hasnain, S. E., and Hasnain, E. (2022). Can Mycobacterium tuberculosis infection lead to cancer? Call for a paradigm shift in understanding TB and cancer. Int. J. Med. Microbiol. 312:151558. doi: 10.1016/j.ijmm.2022.151558
Mao, Q., Jiang, F., Yin, R., Wang, J., Xia, W., Dong, G., et al. (2018). Interplay between the lung microbiome and lung cancer. Cancer Lett. 415, 40–48. doi: 10.1016/j.canlet.2017.11.036
Marin-Luevano, S. P., Rodriguez-Carlos, A., Jacobo-Delgado, Y., Valdez-Miramontes, C., Enciso-Moreno, J. A., and Rivas-Santiago, B. (2021). Steroid hormone modulates the production of cathelicidin and human β-defensins in lung epithelial cells and macrophages promoting Mycobacterium tuberculosis killing. Tuberculosis 128, 1472–9792. doi: 10.1016/j.tube.2021.102080
Marques, A. C., Costa, P. J., Velho, S., and Amaral, M. H. (2020). Functionalizing nanoparticles with cancer-targeting antibodies: A comparison of strategies. J. Control. Release 320, 180–200. doi: 10.1016/j.jconrel.2020.01.035
Martineau, A. R., Wilkinson, K. A., Newton, S. M., Floto, R. A., Norman, A. W., Skolimowska, K., et al. (2007). IFN-γ-and TNF-independent vitamin D-inducible human suppression of mycobacteria: the role of cathelicidin LL-37. J. Immunol. 178, 7190–7198. doi: 10.4049/jimmunol.178.11.7190
Mejía-Pitta, A., Broset, E., and de la Fuente-Nunez, C. (2021). Probiotic engineering strategies for the heterologous production of antimicrobial peptides. Adv. Drug Deliv. Rev. 176:113863. doi: 10.1016/j.addr.2021.113863
Meng, Z., Pan, L., Qian, S., Yang, X., Pan, L., Chi, R., et al. (2023). Antimicrobial peptide nanoparticles coated with macrophage cell membrane for targeted antimicrobial therapy of sepsis. Mater. Des. 229:111883. doi: 10.1016/j.matdes.2023.111883
Molchanova, N., Hansen, P., and Franzyk, H. (2017). Advances in development of antimicrobial Peptidomimetics as potential drugs. Molecules 22:1430. doi: 10.3390/molecules22091430
Molina-Romero, C., Arrieta, O., and Hernández-Pando, R. (2019). Tuberculosis and lung cancer. Salud Publica Mex. 61:286. doi: 10.21149/10090
Montaser, R., Paul, V. J., and Luesch, H. (2011). Pitipeptolides C–F, antimycobacterial cyclodepsipeptides from the marine cyanobacterium Lyngbya majuscula from Guam. Phytochemistry 72, 2068–2074. doi: 10.1016/j.phytochem.2011.07.014
Moradi, S. V., Hussein, W. M., Varamini, P., Simerska, P., and Toth, I. (2016). Glycosylation, an effective synthetic strategy to improve the bioavailability of therapeutic peptides. Chem. Sci. 7, 2492–2500. doi: 10.1039/C5SC04392A
Moussouni, M., Nogaret, P., Garai, P., Ize, B., Vivès, E., and Blanc-Potard, A.-B. (2019). Activity of a synthetic peptide targeting MgtC on Pseudomonas aeruginosa Intramacrophage survival and biofilm formation. Front. Cell. Infect. Microbiol. 9:84. doi: 10.3389/fcimb.2019.00084
Mustafa, G., Mehmood, R., Mahrosh, H. S., Mehmood, K., and Ahmed, S. (2022). Investigation of plant antimicrobial peptides against selected pathogenic bacterial species using a peptide-protein docking approach. Biomed. Res. Int. 2022, 1–11. doi: 10.1155/2022/1077814
Nagaraj, G., Dhanusu, S., Nachiappan, D. M., and Vellaichamy, E. (2022). C-type natriuretic peptide (CNP) induces cell death and sensitizes the effect of cisplatin in human non-small cell lung Cancer cells (A549). Int. J. Pept. Res. Ther. 28:112. doi: 10.1007/s10989-022-10420-2
Nalbandian, A., Yan, B.-S., Pichugin, A., Bronson, R. T., and Kramnik, I. (2009). Lung carcinogenesis induced by chronic tuberculosis infection: the experimental model and genetic control. Oncogene 28, 1928–1938. doi: 10.1038/onc.2009.32
Namasivayam, S., Sher, A., Glickman, M. S., and Wipperman, M. F. (2018). The microbiome and tuberculosis: early evidence for cross talk. MBio 9:18. doi: 10.1128/mBio.01420-18
Neshani, A., Zare, H., Akbari Eidgahi, M. R., Khaledi, A., and Ghazvini, K. (2019). Epinecidin-1, a highly potent marine antimicrobial peptide with anticancer and immunomodulatory activities. BMC Pharmacol. Toxicol. 20:33. doi: 10.1186/s40360-019-0309-7
Nickel, D., Busch, M., Mayer, D., Hagemann, B., Knoll, V., and Stenger, S. (2012). Hypoxia triggers the expression of human β Defensin 2 and antimicrobial activity against Mycobacterium tuberculosis in human macrophages. J. Immunol. 188, 4001–4007. doi: 10.4049/jimmunol.1100976
Pandit, G., Sarkar, T. S. R. V., Debnath, S., Satpati, P., and Chatterjee, S. (2022). Delineating the mechanism of action of a protease resistant and salt tolerant synthetic antimicrobial peptide against Pseudomonas aeruginosa. ACS Omega 7, 15951–15968. doi: 10.1021/acsomega.2c01089
Papo, N., and Shai, Y. (2005). Host defense peptides as new weapons in cancer treatment. C. Cell. Mol. Life Sci. 62, 784–790. doi: 10.1007/s00018-005-4560-2
Patil, S. M., and Kunda, N. K. (2022a). Anticancer activity of D-LAK-120A, an antimicrobial peptide, in non-small cell lung cancer (NSCLC). Biochimie 201, 7–17. doi: 10.1016/j.biochi.2022.06.011
Patil, S. M., and Kunda, N. K. (2022b). Nisin ZP, an antimicrobial peptide, induces cell death and inhibits non-small cell lung Cancer (NSCLC) progression in vitro in 2D and 3D cell culture. Pharm. Res. 39, 2859–2870. doi: 10.1007/s11095-022-03220-2
Pilaniya, V., Gera, K., Kunal, S., and Shah, A. (2016). Pulmonary tuberculosis masquerading as metastatic lung disease. Eur. Respir. Rev. 25, 97–98. doi: 10.1183/16000617.00002315
Polverino, F., Laucho-Contreras, M., Rojas Quintero, J., Divo, M., Pinto-Plata, V., Sholl, L., et al. (2016). Increased expression of A proliferation-inducing ligand (APRIL) in lung leukocytes and alveolar epithelial cells in COPD patients with non small cell lung cancer: a possible link between COPD and lung cancer? Multidiscip. Respir. Med. 11:17. doi: 10.1186/s40248-016-0051-6
Poore, G. D., Kopylova, E., Zhu, Q., Carpenter, C., Fraraccio, S., Wandro, S., et al. (2020). Microbiome analyses of blood and tissues suggest cancer diagnostic approach. Nature 579, 567–574. doi: 10.1038/s41586-020-2095-1
Portell-Buj, E., Vergara, A., Alejo, I., López-Gavín, A., Monté, M. R., San Nicolás, L., et al. (2019). In vitro activity of 12 antimicrobial peptides against Mycobacterium tuberculosis and Mycobacterium avium clinical isolates. J. Med. Microbiol. 68, 211–215. doi: 10.1099/jmm.0.000912
Preethi, A. R., and Anbarasu, A. (2022). Antimicrobial peptides as Immunomodulators and Antimycobacterial agents to combat Mycobacterium tuberculosis: a critical review. Probiotics Antimicrob. Proteins. doi: 10.1007/s12602-022-10018-6
Qin, Y., Chen, Y., Chen, J., Xu, K., Xu, F., and Shi, J. (2022). The relationship between previous pulmonary tuberculosis and risk of lung cancer in the future. Infect. Agent. Cancer 17:20. doi: 10.1186/s13027-022-00434-2
Ramírez-Carreto, S., Jiménez-Vargas, J. M., Rivas-Santiago, B., Corzo, G., Possani, L. D., Becerril, B., et al. (2015). Peptides from the scorpion Vaejovis punctatus with broad antimicrobial activity. Peptides 73, 51–59. doi: 10.1016/j.peptides.2015.08.014
Rampogu, S., Zeb, A., Baek, A., Park, C., Son, M., and Lee, K. W. (2018). Discovery of potential plant-derived peptide Deformylase (PDF) inhibitors for multidrug-resistant Bacteria using computational studies. J. Clin. Med. 7:563. doi: 10.3390/jcm7120563
Rao Muvva, J., Ahmed, S., Rekha, R. S., Kalsum, S., Groenheit, R., Schön, T., et al. (2021). Immunomodulatory agents combat multidrug-resistant tuberculosis by improving antimicrobial immunity. J. Infect. Dis. 224, 332–344. doi: 10.1093/infdis/jiab100
Ravimohan, S., Kornfeld, H., Weissman, D., and Bisson, G. P. (2018). Tuberculosis and lung damage: from epidemiology to pathophysiology. Eur. Respir. Rev. 27:170077. doi: 10.1183/16000617.0077-2017
Ravishankar, S., Ambady, A., Swetha, R. G., Anbarasu, A., Ramaiah, S., and Sambandamurthy, V. K. (2016). Essentiality assessment of Cysteinyl and Lysyl-tRNA Synthetases of Mycobacterium smegmatis. PLoS One 11:e0147188. doi: 10.1371/journal.pone.0147188
Richards, D. A., Maruani, A., and Chudasama, V. (2017). Antibody fragments as nanoparticle targeting ligands: a step in the right direction. Chem. Sci. 8, 63–77. doi: 10.1039/C6SC02403C
Rivas-Santiago, B., Rivas Santiago, C. E., Castañeda-Delgado, J. E., León-Contreras, J. C., Hancock, R. E. W., and Hernandez-Pando, R. (2013). Activity of LL-37, CRAMP and antimicrobial peptide-derived compounds E2, E6 and CP26 against Mycobacterium tuberculosis. Int. J. Antimicrob. Agents 41, 143–148. doi: 10.1016/j.ijantimicag.2012.09.015
Rivas-Santiago, B., Rivas-Santiago, C., Sada, E., and Hernández-Pando, R. (2015). Prophylactic potential of defensins and L-isoleucine in tuberculosis household contacts: an experimental model. Immunotherapy 7, 207–213. doi: 10.2217/imt.14.119
Rodrigues, T. S., Conti, B. J., Fraga-Silva, T. F. D. C., Almeida, F., and Bonato, V. L. D. (2020). Interplay between alveolar epithelial and dendritic cells and Mycobacterium tuberculosis. J. Leukoc. Biol. 108, 1139–1156. doi: 10.1002/JLB.4MR0520-112R
Rodriguez Plaza, J. G., Morales-Nava, R., Diener, C., Schreiber, G., Gonzalez, Z. D., Lara Ortiz, M. T., et al. (2014). Cell penetrating peptides and cationic antibacterial peptides. J. Biol. Chem. 289, 14448–14457. doi: 10.1074/jbc.M113.515023
Rodríguez-Carlos, A., Jacobo-Delgado, Y. M., Santos-Mena, A. O., and Rivas-Santiago, B. (2021). Modulation of cathelicidin and defensins by histone deacetylase inhibitors: A potential treatment for multi-drug resistant infectious diseases. Peptides 140:170527. doi: 10.1016/j.peptides.2021.170527
Roque-Borda, C. A., Bento da Silva, P., Rodrigues, M. C., Di Filippo, L. D., Duarte, J. L., Chorilli, M., et al. (2022a). Pharmaceutical nanotechnology: antimicrobial peptides as potential new drugs against WHO list of critical, high, and medium priority bacteria. Eur. J. Med. Chem. 241:114640. doi: 10.1016/j.ejmech.2022.114640
Roque-Borda, C. A., De Souza Saraiva, M. M., Monte, D. F. M., Rodrigues Alves, L. B., De Almeida, A. M., Ferreira, T. S., et al. (2022b). HPMCAS-coated alginate microparticles loaded with Ctx(Ile 21)-ha as a promising antimicrobial agent against Salmonella Enteritidis in a chicken infection model. ACS Infect. Dis. 8, 472–481. doi: 10.1021/acsinfecdis.1c00264
Roque-Borda, C. A., Pereira, L. P., Guastalli, E. A. L., Soares, N. M., Mac-Lean, P. A. B., Salgado, D. D., et al. (2021a). HPMCP-coated microcapsules containing the Ctx(Ile21)-ha antimicrobial peptide reduce the mortality rate caused by resistant Salmonella Enteritidis in laying hens. Antibiotics 10:616. doi: 10.3390/antibiotics10060616
Roque-Borda, C. A., Saraiva, M. D. M. S., Macedo Junior, W. D., Márquez Montesinos, J. C. E., Meneguin, A. B., Toledo Borges, A. B., et al. (2023). Chitosan and HPMCAS double-coating as protective systems for alginate microparticles loaded with Ctx(Ile21)-ha antimicrobial peptide to prevent intestinal infections. Biomaterials 293:121978. doi: 10.1016/j.biomaterials.2022.121978
Roque-Borda, C. A., Silva, H. R. L., Crusca Junior, E., Serafim, J. A., Meneguin, A. B., Chorilli, M., et al. (2021b). Alginate-based microparticles coated with HPMCP/AS cellulose-derivatives enable the Ctx(Ile21)-ha antimicrobial peptide application as a feed additive. Int. J. Biol. Macromol. 183, 1236–1247. doi: 10.1016/j.ijbiomac.2021.05.011
Roshanak, S., Shahidi, F., Tabatabaei Yazdi, F., Javadmanesh, A., and Movaffagh, J. (2021). Buforin I an alternative to conventional antibiotics: evaluation of the antimicrobial properties, stability, and safety. Microb. Pathog. 161:105301. doi: 10.1016/j.micpath.2021.105301
Rungsa, P., Peigneur, S., Jangpromma, N., Klaynongsruang, S., Tytgat, J., and Daduang, S. (2022). In silico and in vitro structure–activity relationship of Mastoparan and its analogs. Molecules 27:561. doi: 10.3390/molecules27020561
Salehi-Rad, R., Li, R., Paul, M. K., Dubinett, S. M., and Liu, B. (2020). The biology of lung Cancer: development of more effective methods for prevention, diagnosis, and treatment. Clin. Chest Med. 41, 25–38. doi: 10.1016/j.ccm.2019.10.003
Salem, M., Keshavarzi Arshadi, A., and Yuan, J. S. (2022). AMPDeep: hemolytic activity prediction of antimicrobial peptides using transfer learning. BMC Bioinformatics 23:389. doi: 10.1186/s12859-022-04952-z
Samuchiwal, S. K., Tousif, S., Singh, D. K., Kumar, A., Ghosh, A., Bhalla, K., et al. (2014). A peptide fragment from the human COX3 protein disrupts association of Mycobacterium tuberculosisvirulence proteins ESAT-6 and CFP10, inhibits mycobacterial growth and mounts protective immune response. BMC Infect. Dis. 14:355. doi: 10.1186/1471-2334-14-355
Schlusselhuber, M., Torelli, R., Martini, C., Leippe, M., Cattoir, V., Leclercq, R., et al. (2013). The equine antimicrobial peptide eCATH1 is effective against the facultative intracellular pathogen Rhodococcus equi in mice. Antimicrob. Agents Chemother. 57, 4615–4621. doi: 10.1128/AAC.02044-12
Sengkhui, S., Klubthawee, N., and Aunpad, R. (2023). A novel designed membrane-active peptide for the control of foodborne Salmonella enterica serovar typhimurium. Sci. Rep. 13:3507. doi: 10.1038/s41598-023-30427-z
Sharma, R., Raghav, R., Priyanka, K., Rishi, P., Sharma, S., Srivastava, S., et al. (2019). Exploiting chitosan and gold nanoparticles for antimycobacterial activity of in silico identified antimicrobial motif of human neutrophil peptide. Sci. Rep. 9, 7866–7878. doi: 10.1038/s41598-019-44256-6
Sheedy, F. J., and Divangahi, M. (2021). Targeting immunometabolism in host defence against Mycobacterium tuberculosis. Immunology 162, 145–159. doi: 10.1111/imm.13276
Shi, L., Eugenin, E. A., and Subbian, S. (2016a). Immunometabolism in tuberculosis. Front. Immunol. 7:150. doi: 10.3389/fimmu.2016.00150
Shi, L., Salamon, H., Eugenin, E. A., Pine, R., Cooper, A., and Gennaro, M. L. (2016b). Infection with Mycobacterium tuberculosis induces the Warburg effect in mouse lungs. Sci. Rep. 5:18176. doi: 10.1038/srep18176
Shroff, G. S., Strange, C. D., Altan, M., Carter, B. W., Ahuja, J., Godoy, M. C. B., et al. (2022). Post-immunotherapy imaging in lung cancer. Clin. Radiol. 77, 44–57. doi: 10.1016/j.crad.2021.05.003
Silveira, R. F., Roque-Borda, C. A., and Vicente, E. F. (2021). Antimicrobial peptides as a feed additive alternative to animal production, food safety and public health implications: an overview. Anim. Nutr. 7, 896–904. doi: 10.1016/j.aninu.2021.01.004
Simons, A., Alhanout, K., and Duval, R. E. (2020). Bacteriocins, antimicrobial peptides from bacterial origin: overview of their biology and their impact against multidrug-resistant Bacteria. Microorganisms 8:639. doi: 10.3390/microorganisms8050639
Singh, S., Yabaji, S. M., Ali, R., Srivastava, K. K., and Haq, W. (2019). Synthesis and biological activity of Ub2 derived peptides as potential host-directed antitubercular therapy. Chem. Biol. Drug Des. 94, 1330–1338. doi: 10.1111/cbdd.13508
Skowroński, M., Iwanik, K., Halicka, A., and Barinow-Wojewódzki, A. (2015). Squamous cell lung Cancer in a male with pulmonary tuberculosis. Adv. Respir. Med. 83, 298–302. doi: 10.5603/PiAP.2015.0049
Snyder, K. M., Hullsiek, R., Mishra, H. K., Mendez, D. C., Li, Y., Rogich, A., et al. (2018). Expression of a recombinant high affinity IgG fc receptor by engineered NK cells as a docking platform for therapeutic mAbs to target Cancer cells. Front. Immunol. 9:2873. doi: 10.3389/fimmu.2018.02873
Song, L., Chen, X., Liu, Y., Wang, H., and Li, J. (2022). Synthetic polymer material modified by d-peptide and its targeted application in the treatment of non-small cell lung cancer. Int. J. Pharm. 619:121651. doi: 10.1016/j.ijpharm.2022.121651
Sun, W., Zhang, L., Liang, J., Li, X., Yang, Y., Sun, W., et al. (2022). Comparison of clinical and imaging features between pulmonary tuberculosis complicated with lung cancer and simple pulmonary tuberculosis: a systematic review and meta-analysis. Epidemiol. Infect. 150:e43. doi: 10.1017/S0950268822000176
Suttmann, H., Retz, M., Paulsen, F., Harder, J., Zwergel, U., Kamradt, J., et al. (2008). Antimicrobial peptides of the Cecropin-family show potent antitumor activity against bladder cancer cells. BMC Urol. 8:5. doi: 10.1186/1471-2490-8-5
Sze, M. A., Dimitriu, P. A., Hayashi, S., Elliott, W. M., McDonough, J. E., Gosselink, J. V., et al. (2012). The lung tissue microbiome in chronic obstructive pulmonary disease. Am. J. Respir. Crit. Care Med. 185, 1073–1080. doi: 10.1164/rccm.201111-2075OC
Tenland, E., Pochert, A., Krishnan, N., Umashankar Rao, K., Kalsum, S., Braun, K., et al. (2019). Effective delivery of the anti-mycobacterial peptide NZX in mesoporous silica nanoparticles. PLoS One 14:e0212858. doi: 10.1371/journal.pone.0212858
Tipgomut, C., Wongprommoon, A., Takeo, E., Ittiudomrak, T., Puthong, S., and Chanchao, C. (2018). Melittin induced G1 cell cycle arrest and apoptosis in Chago-K1 human bronchogenic carcinoma cells and inhibited the differentiation of THP-1 cells into tumour-associated macrophages. Asian Pacific J. Cancer Prev. 19, 3427–3434. doi: 10.31557/APJCP.2018.19.12.3427
Tsay, J.-C. J., Wu, B. G., Badri, M. H., Clemente, J. C., Shen, N., Meyn, P., et al. (2018). Airway microbiota is associated with upregulation of the PI3K pathway in lung Cancer. Am. J. Respir. Crit. Care Med. 198, 1188–1198. doi: 10.1164/rccm.201710-2118OC
Tukiman, M. H. (2022). Immunometabolism of immune cells in mucosal environment drives effector responses against Mycobacterium tuberculosis. Int. J. Mol. Sci. 23–8531. doi: 10.3390/ijms23158531
Tzitzilis, A., Boura-Theodorou, A., Michail, V., Papadopoulos, S., Krikorian, D., Lekka, M. E., et al. (2020). Cationic amphipathic peptide analogs of cathelicidin LL-37 as a probe in the development of antimicrobial/anticancer agents. J. Pept. Sci. 26:3254. doi: 10.1002/psc.3254
Usmani, S. S., Bhalla, S., and Raghava, G. P. S. (2018). Prediction of Antitubercular peptides from sequence information using ensemble classifier and hybrid features. Front. Pharmacol. 9:954. doi: 10.3389/fphar.2018.00954
Vasava, M. S., Bhoi, M. N., Rathwa, S. K., Borad, M. A., Nair, S. G., and Patel, H. D. (2017). Drug development against tuberculosis: past, present and future. Indian J. Tuberc. 64, 252–275. doi: 10.1016/j.ijtb.2017.03.002
Vento, S., and Lanzafame, M. (2011). Tuberculosis and cancer: a complex and dangerous liaison. Lancet Oncol. 12, 520–522. doi: 10.1016/S1470-2045(11)70105-X
von Haussen, J., Koczulla, R., Shaykhiev, R., Herr, C., Pinkenburg, O., Reimer, D., et al. (2008). The host defence peptide LL-37/hCAP-18 is a growth factor for lung cancer cells. Lung Cancer 59, 12–23. doi: 10.1016/j.lungcan.2007.07.014
Wang, J., Ge, P., Qiang, L., Tian, F., Zhao, D., Chai, Q., et al. (2017). The mycobacterial phosphatase PtpA regulates the expression of host genes and promotes cell proliferation. Nat. Commun. 8:244. doi: 10.1038/s41467-017-00279-z
Wang, J., Ma, K., Ruan, M., Wang, Y., Li, Y., Fu, Y. V., et al. (2018). A novel cecropin B-derived peptide with antibacterial and potential anti-inflammatory properties. PeerJ 6:e5369. doi: 10.7717/peerj.5369
Wei, X.-B., Wu, R.-J., Si, D.-Y., Liao, X.-D., Zhang, L.-L., and Zhang, R.-J. (2016). Novel hybrid peptide Cecropin A (1–8)-LL37 (17–30) with potential antibacterial activity. Int. J. Mol. Sci. 17:983. doi: 10.3390/ijms17070983
Wiman, E., Zattarin, E., Aili, D., Bengtsson, T., Selegård, R., and Khalaf, H. (2023). Development of novel broad-spectrum antimicrobial lipopeptides derived from plantaricin NC8 β. Sci. Rep. 13:4104. doi: 10.1038/s41598-023-31185-8
Wu, J., Li, L., Zhang, H., Zhao, Y., Zhang, H., Wu, S., et al. (2021). A risk model developed based on tumor microenvironment predicts overall survival and associates with tumor immunity of patients with lung adenocarcinoma. Oncogene 40, 4413–4424. doi: 10.1038/s41388-021-01853-y
Xie, J., Bi, Y., Zhang, H., Dong, S., Teng, L., Lee, R. J., et al. (2020). Cell-penetrating peptides in diagnosis and treatment of human diseases: from preclinical research to clinical application. Front. Pharmacol. 11:697. doi: 10.3389/fphar.2020.00697
Xiong, G., Wu, Z., Yi, J., Fu, L., Yang, Z., Hsieh, C., et al. (2021). ADMETlab 2.0: an integrated online platform for accurate and comprehensive predictions of ADMET properties. Nucleic Acids Res. 49, W5–W14. doi: 10.1093/NAR/GKAB255
Yan, X., Yang, M., Liu, J., Gao, R., Hu, J., Li, J., et al. (2015). Discovery and validation of potential bacterial biomarkers for lung cancer. Am. J. Cancer Res. 5, 3111–3122.
Yang, M., Zhang, C., Zhang, M. Z., and Zhang, S. (2018). Beta-defensin derived cationic antimicrobial peptides with potent killing activity against gram negative and gram positive bacteria. BMC Microbiol. 18:54. doi: 10.1186/s12866-018-1190-z
Yang, D., Zhu, L., Lin, X., Zhu, J., Qian, Y., Liu, W., et al. (2022). Therapeutic effects of synthetic triblock amphiphilic short antimicrobial peptides on human lung adenocarcinoma. Pharmaceutics 14:929. doi: 10.3390/pharmaceutics14050929
Younga, D., and Verreck, F. A. W. (2012). Creativity in tuberculosis research and discovery. Tuberculosis 92, 14–16. doi: 10.1016/S1472-9792(12)70006-9
Yu, G., Gail, M. H., Consonni, D., Carugno, M., Humphrys, M., Pesatori, A. C., et al. (2016). Characterizing human lung tissue microbiota and its relationship to epidemiological and clinical features. Genome Biol. 17:163. doi: 10.1186/s13059-016-1021-1
Zhang, R., Fan, X., Jiang, X., Zou, M., Xiao, H., and Wu, G. (2020). Multiple mechanisms of the synthesized antimicrobial peptide TS against gram-negative Bacteria for high efficacy antibacterial action in vivo. Molecules 26:60. doi: 10.3390/molecules26010060
Zhang, J., Iwanaga, K., Choi, K. C., Wislez, M., Raso, M. G., Wei, W., et al. (2008). Intratumoral Epiregulin is a marker of advanced disease in non–small cell lung Cancer patients and confers invasive properties on EGFR-mutant cells. Cancer Prev. Res. 1, 201–207. doi: 10.1158/1940-6207.CAPR-08-0014
Zhang, Y., Li, T., Hu, Y., Chen, J., He, Y., Gao, X., et al. (2022). Co-delivery of doxorubicin and curcumin via cRGD-peptide modified PEG-PLA self-assembly nanomicelles for lung cancer therapy. Chinese Chem. Lett. 33, 2507–2511. doi: 10.1016/j.cclet.2021.11.076
Zhang, C., Yang, M., and Ericsson, A. C. (2019). Antimicrobial peptides: potential application in liver Cancer. Front. Microbiol. 10:1257. doi: 10.3389/fmicb.2019.01257
Zhang, J., Zhang, H., Liu, M., Lan, Y., Sun, H., Mai, K., et al. (2020). Short-chain fatty acids promote intracellular bactericidal activity in head kidney macrophages from turbot (Scophthalmus maximus L.) via hypoxia inducible factor-1α. Front. Immunol. 11:536. doi: 10.3389/fimmu.2020.615536
Zhao, Y., Liu, Y., Li, S., Peng, Z., Liu, X., Chen, J., et al. (2021). Role of lung and gut microbiota on lung cancer pathogenesis. J. Cancer Res. Clin. Oncol. 147, 2177–2186. doi: 10.1007/s00432-021-03644-0
Zhao, W., Xue, Y., Zhang, Y., Zhu, Y., Chen, Z., and Zhao, X. (2022). A peptide translated from circPPP1R12A promotes the malignancy of non-small cell lung cancer cells through AKT signaling pathway. J. Clin. Lab. Anal. 36:e24644. doi: 10.1002/jcla.24644
Zhong, C., Zhu, N., Zhu, Y., Liu, T., Gou, S., Xie, J., et al. (2020). Antimicrobial peptides conjugated with fatty acids on the side chain of D-amino acid promises antimicrobial potency against multidrug-resistant bacteria. Eur. J. Pharm. Sci. 141:105123. doi: 10.1016/j.ejps.2019.105123
Zhou, Y., Zou, Y., Yang, M., Mei, S., Liu, X., Han, H., et al. (2022). Highly potent, selective, biostable, and cell-permeable cyclic D -peptide for dual-targeting therapy of lung Cancer. J. Am. Chem. Soc. 144, 7117–7128. doi: 10.1021/jacs.1c12075
Keywords: Mycobacterium tuberculosis, lung cancer, peptides, multifunctional peptides, treatment
Citation: Polinário G, Primo LMDG, Rosa MABC, Dett FHM, Barbugli PA, Roque-Borda CA and Pavan FR (2023) Antimicrobial peptides as drugs with double response against Mycobacterium tuberculosis coinfections in lung cancer. Front. Microbiol. 14:1183247. doi: 10.3389/fmicb.2023.1183247
Received: 09 March 2023; Accepted: 16 May 2023;
Published: 02 June 2023.
Edited by:
Michal Letek, Universidad de León, SpainReviewed by:
Alvaro Mourenza Flórez, University of Southern California, United StatesCopyright © 2023 Polinário, Primo, Rosa, Dett, Barbugli, Roque-Borda and Pavan. This is an open-access article distributed under the terms of the Creative Commons Attribution License (CC BY). The use, distribution or reproduction in other forums is permitted, provided the original author(s) and the copyright owner(s) are credited and that the original publication in this journal is cited, in accordance with accepted academic practice. No use, distribution or reproduction is permitted which does not comply with these terms.
*Correspondence: Cesar Augusto Roque-Borda, cm9xdWViLmNlc2FyQGdtYWlsLmNvbQ==; Fernando Rogério Pavan, ZmVybmFuZG8ucGF2YW5AdW5lc3AuYnI=
Disclaimer: All claims expressed in this article are solely those of the authors and do not necessarily represent those of their affiliated organizations, or those of the publisher, the editors and the reviewers. Any product that may be evaluated in this article or claim that may be made by its manufacturer is not guaranteed or endorsed by the publisher.
Research integrity at Frontiers
Learn more about the work of our research integrity team to safeguard the quality of each article we publish.