- 1College of Biotechnology, Tianjin University of Science and Technology, Tianjin, China
- 2State Key Laboratory for Managing Biotic and Chemical Treats to the Quality and Safety of Agro-Products, Institute of Plant Protection and Microbiology, Zhejiang Academy of Agricultural Sciences, Hangzhou, China
Magnaporthe oryzae is a filamentous fungus that causes rice blast. Rice blast seriously threatens the safety of food production. The normal synthesis and metabolism of fatty acids are extremely important for eukaryotes, and acyl-CoA is involved in fatty acid metabolism. Acyl-CoA binding (ACB) proteins specifically bind both medium-chain and long-chain acyl-CoA esters. However, the role of the Acb protein in plant-pathogenic fungi has not yet been investigated. Here, we identified MoAcb1, a homolog of the Acb protein in Saccharomyces cerevisiae. Disruption of MoACB1 causes delayed hyphal growth, significant reduction in conidial production and delayed appressorium development, glycogen availability, and reduced pathogenicity. Using immunoblotting and chemical drug sensitivity analysis, MoAcb1 was found to be involved in endoplasmic reticulum autophagy (ER-phagy). In conclusion, our results suggested that MoAcb1 is involved in conidia germination, appressorium development, pathogenicity and autophagy processes in M. oryzae.
Introduction
The filamentous ascomycete fungus Magnaporthe oryzae, which causes rice blast, is a model fungus used to investigate the interactions between the pathogen and the host plant (Ebbole, 2007; Dean et al., 2012). Rice blast is one of the world’s most devastating crop diseases, as it drastically reduces rice production, posing a serious threat to global food security (Wilson, 2021). Rice blast fungus has a unique infection mechanism (Martin-Urdiroz et al., 2016; Di Pietro and Talbot, 2017). Glycogen and lipids are intracellular nutrients that are continuously degraded by autophagy and transported to appressoria during the continual maturation of appressoria (Fernandez and Orth, 2018). After the turgor pressure reaches its peak, penetration pegs form and invade the plant cells, causing plant necrosis (Zhu et al., 2018; Feng et al., 2021).
Acyl-coenzyme A (acyl-CoA) binding (Acb) protein is a conserved protein in mammals, insects, fungi and plants (Rosendal et al., 1993). Acb was first discovered in mouse brain tissue as an endogenous ligand for benzodiazepine receptors because of its capacity to prevent the diazepam binding inhibitor (DBI) or endozepine (EP) from attaching to brain synaptic membranes (Chen et al., 1988). The Acb1 protein was found to have the same amino acid sequence as DBI/EP (Rose et al., 1992). The most basic function of the Acb protein is to bind to acyl-CoA and regulate other metabolic pathways (Schjerling et al., 1996). The process of acylation is necessary before fatty acids may be oxidized or produced by the organism. Long-chain acyl-CoA is a byproduct of lipid metabolism that can be employed as a signaling molecule (Roduit et al., 2004), while the Acb protein is a highly specific repository and transport intermediate. Acetyl-CoA carboxylase and mitochondrial ATP/ADP translocase have been found to be shielded by the Acb protein from being inhibited by long-chain acyl-CoA esters. Acb protein also stimulates mitochondrial acyl-CoA synthase to synthesize acyl-CoA (Rasmussen et al., 1993). In both human and mouse cells, the Acb protein regulates autophagy (Duran et al., 2010), which is inhibited by both the intracellular depletion of Acb proteins and their addition to the extracellular environment. Acb protein also regulates the basic functions of many cells (Burton et al., 2005). Gaigg et al. indicated that Acb protein affects the transport of vesicles and the biosynthesis and structure of membranes (Gaigg et al., 2001). In Aspergillus oryzae, the Acb protein can specifically bind medium-chain and long-chain acyl-CoA esters without binding coenzyme A, nonesterified fatty acids, acylcarnitines, and some nucleotides (Rasmussen et al., 1993). However, in pathogenic fungi, the biological function of the Acb protein has not been elucidated.
Autophagy is an evolutionarily conserved intracellular degradation process involved in maintaining intracellular homeostasis (Glick et al., 2010; Dufey et al., 2014; Cheng et al., 2018). The MoATG1-9, MoATG12-14, MoATG16, MoATG18 of Magnaporthe oryzae participated in autophagy pathway, affecting the growth and development of conidia (Gao et al., 2013; Zhu et al., 2018). Atg8 protein is a ubiquitin-like protein composed of 121 amino acids with a size of 16 kDa, which is closely related to the synthesis of autophagosomes (Liu et al., 2010; Zheng et al., 2015). Since the synthesis and lipidation of Atg8 are enhanced under autophagy-induced conditions, it has been used by various studies as a reliable marker for autophagy induction (Ichimura et al., 2004; Yoshimoto et al., 2004; Nakatogawa et al., 2007). ER-phagy is a selective autophagic pathway used to remove misfolded or unfolded proteins within the ER in mammalian cells. Sec62 is a component of the transposon complex and has recently been recognized as an ER-phagy receptor during the stress recovery phase of the mammalian ER (Fumagalli et al., 2016). Studies have shown that Arabidopsis thaliana Sec62 is required for plant development and may act as an ER-phagy receptor in plants (Hu et al., 2020). A homolog of the S. cerevisiae Acb protein was identified in M. oryzae and named MoAcb1. We found that MoAcb1 is involved in a range of physiological activities, including appressorium formation, and pathogenicity of M. oryzae. Interestingly, we found that MoAcb1 negatively regulates ER-phagy, thus affecting the biological function of M. oryzae. Our results enrich our understanding of pathogenic fungi and provide new insights for exploring the function of M. oryzae.
Results
Identification of the Acb1 protein in Magnaporthe oryzae
We used the amino acid sequence of S. cerevisiae Acb1 to search the M. oryzae Genome Database.1 We identified an Acb1 homolog (MGG_06177) that shared 43.66% amino acid similarity with ScAcb1, which we named MoAcb1. MoAcb1 showed high homology with its orthologs in other fungi, including CoAcb1 of Colletotrichum orbiculare (66.99% identity), CeAcb1 of Caenorhabditis elegans (50.59% identity), FgAcb1 of Fusarium graminearum (63.81% identity), DmAcb1 of Drosophila melanogaster (43.68% identity), MmAcb1 of Mus musculus (40.45% identity), and NcAcb1 of Neurospora crassa (66.67% identity). The MoAcb1 protein contains the ACB domain (64aa-152aa), which is also very conserved in multiple species (Figure 1). The results show that Acb1 is well conserved in various organisms.
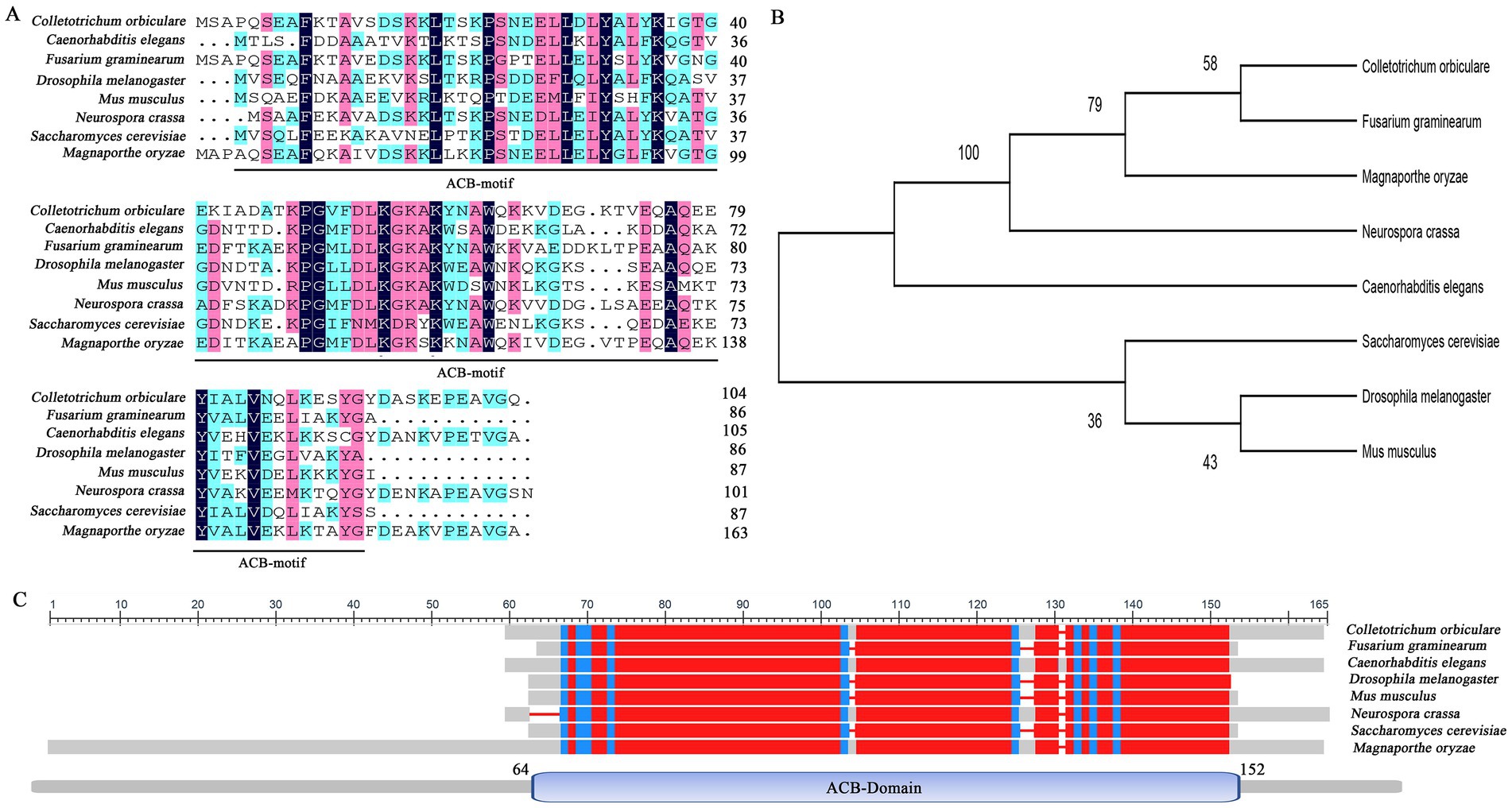
Figure 1. Sequence alignment of the amino acids of Acb1 in different species. (A) The amino acids of ACB1 domain were sequence-aligned by DNAMAN 8. Comparative sequences were obtained from Magnaporthe oryzae (MoAcb1), Colletotrichum orbiculare (TDZ22682.1), Caenorhabditis elegans (NP_491412.1), Fusarium graminearum (XP_011327983.1), Drosophila melanogaster (NP_523952.2), Mus musculus (NP_031856.1), Neurospora crassa (XP_962380.1), and Saccharomyces cerevisiae (NP_011551.3). (B) 500 bootstrapped ML (maximum likelihood) tree generated by MEGA v11. (C) The amino acids of Acb1 were sequence-aligned by SmartBLAST.
MoAcb1 affects conidial formation of Magnaporthe oryzae
To investigate the biological role of MoAcb1 in M. oryzae, we generated the ∆Moacb1 mutant by homologous recombination, and then analyzed the growth and appressorium formation of different strains (Figure 2A). Compared with 70-15 and ∆Moacb1-C, the vegetative growth of the ∆Moacb1 mutant was slightly slowed by approximately 20% (Figure 2B), and the production of conidia was significantly lower than that of 70-15 (Figures 2C,E). In terms of conidial morphology, the ∆Moacb1 mutant is also significantly different from 70-15. Most conidia of the 70-15 strain have two septa, while most conidia of ∆Moacb1 mutant have only one septum or no septum, and the conidia of ∆Moacb1-C return to the normal morphology (Figures 2D,F). Since the MoAcb1 protein contains an Acb binding domain, we divided its sequence into two segments, N1 (1–189 bp) and N2 (190–456 bp), and merged it into the ∆Moacb1 mutant to obtain the ∆Moacb1-N1 and ∆Moacb1-N2 strains, respectively. Their growth rate, conidia morphology and conidiation are similar to those of the ∆Moacb1 mutant (Figures 2B–F), indicating that only the full-length complement can return to normal. Taken together, these data suggest that MoAcb1 affects the growth and conidial formation of rice blast fungus.
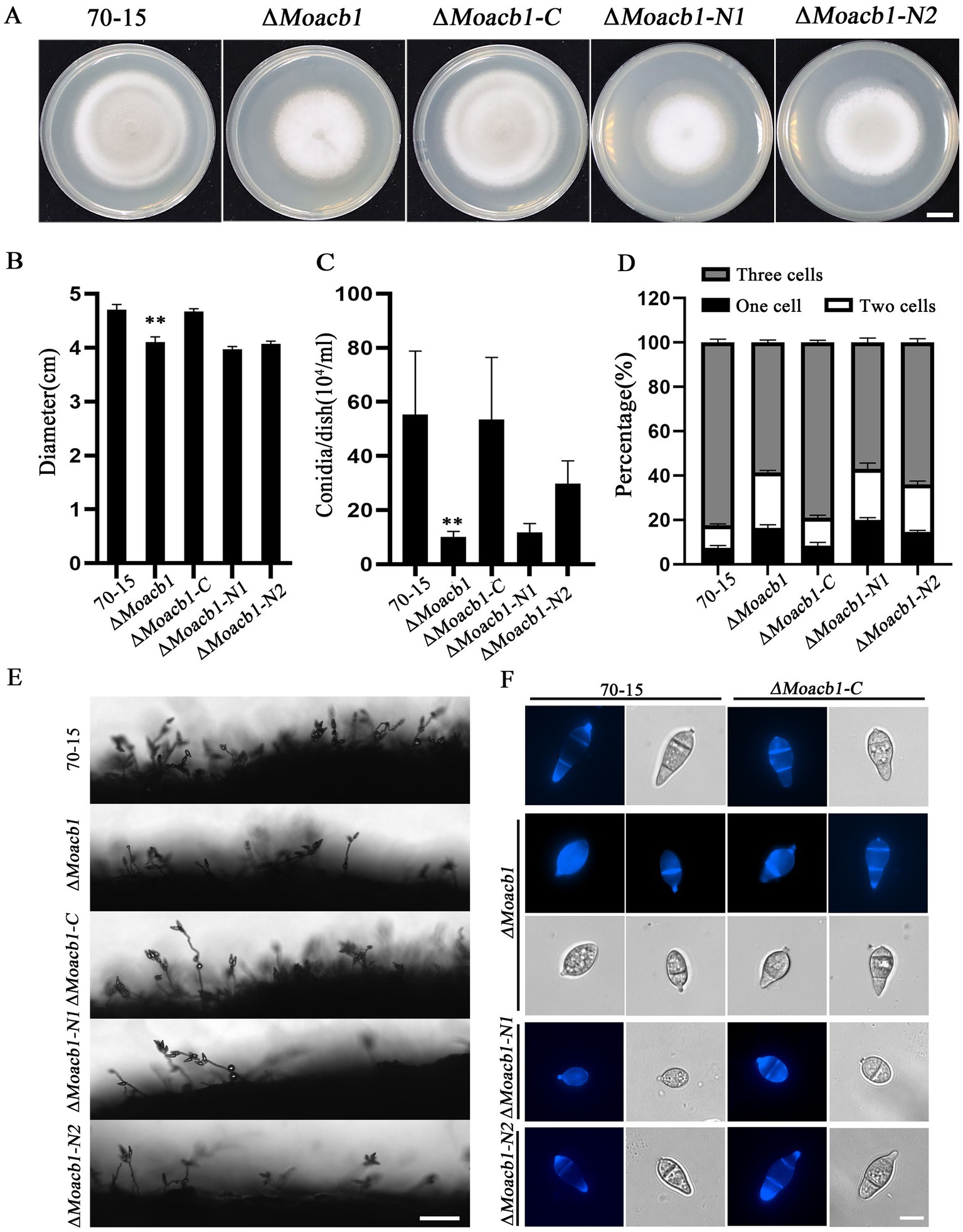
Figure 2. MoAcb1 is involved in conidial development of M. oryzae. (A) Conidial morphology of five strains grown in CM for 8 days. Bar, 1 cm. (B) Measurement and analysis of the colony diameter of five strains grown in CM for 8 days, with the standard deviation indicated by error bars. T-test was used to test for significant differences. **p < 0.01. (C) The conidial yields of five strains grown in CM for 8 days, with the standard deviation indicated by error bars. T-test test was used to test for significant differences. **p < 0.01. (D) The three different types of conidia were statistically analyzed in the five strains, with the standard deviation indicated by error bars. T-test was used to test for significant differences. (E) Observed conidiophore of the five strains under an electron microscope. Bar, 50 μm. (F) Conidia of the five strains were stained with calcofluor white (CFW), and the septum was stained blue. Bar, 10 μm.
MoAcb1 affects appressorium formation in Magnaporthe oryzae
Turgor pressure accumulation and appressorium formation are key in the infection stage (Cai et al., 2022). We analyzed these processes by observing the formation of appressoria and measuring the size of the appressorium. The results showed that appressorium formation in the ΔMoacb1 mutant was relatively delayed on the hydrophobic surface compared with that in the 70-15 and complemented strains, with a significant difference at 4 h post incubation (hpi), 8 hpi and 12 hpi but little difference at 24 hpi (Figures 3A,B). Therefore, we used 0.5–2 M glycerol for the appressorium collapse assay. By adding different concentrations of glycerol, we found that when the external glycerol concentration reached 1 M, the appressorium collapse rate of the ΔMoacb1 mutant was significantly higher than those of 70-15 and ∆Moacb1-C. Only 55% of 70-15 appressoria had collapsed, while 85% of ΔMoacb1 mutant appressoria had collapsed, indicating that the ΔMoacb1 mutant affected turgor pressure accumulation (Figures 3C,D).
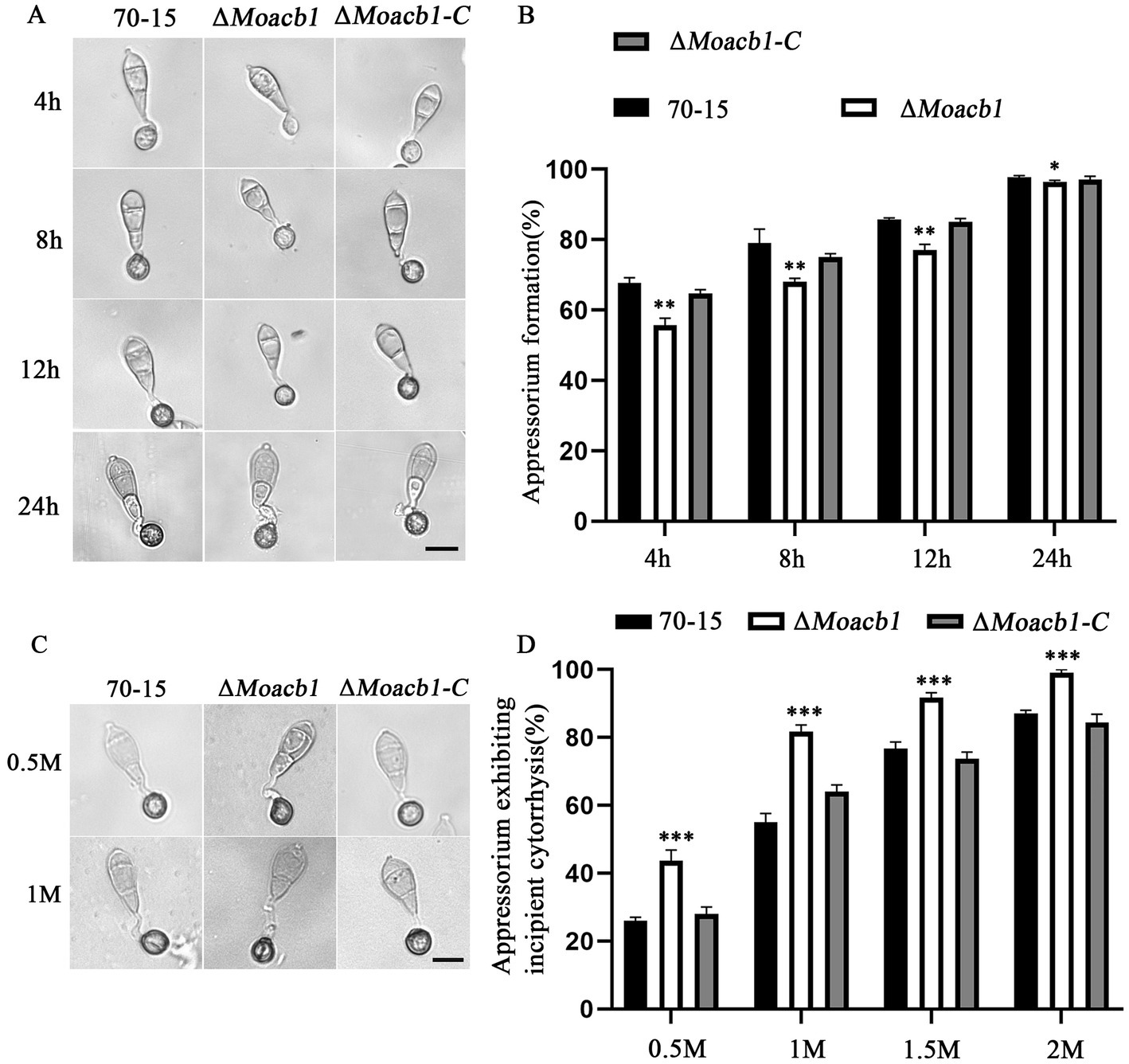
Figure 3. MoAcb1 is important for appressorium formation. (A) The conidial suspensions of the 70-15, ∆Moacb1 and ∆Moacb1-C strains were inoculated on the hydrophobic surface to photograph appressorium formation at different times. Bar, 20 μm. (B) The formation rate of the appressorium was calculated and statistically analyzed, with the standard deviation indicated by error bars. T-test was used to test for significant differences. *p < 0.05, **p < 0.01. (C) Cell collapse of the 70-15, ∆Moacb1 and ∆Moacb1-C strains in different concentrations of glycerol. Bar, 20 μm. (D) Cell collapse rates were calculated and statistically analyzed, with the standard deviation indicated by error bars. T-test test was used to test for significant differences. ***p < 0.001.
MoAcb1 affects the pathogenicity of Magnaporthe oryzae
To further explore the effect of MoAcb1 on pathogenicity, we used barley and rice leaves for pathogenicity analysis. The 70-15, ∆Moacb1 mutant and ∆Moacb1-C strains were inoculated into isolated barley leaves. The results showed that the ∆Moacb1 mutant caused only very small disease spots when compared to the severe disease spots produced by the 70-15 and complemented strains (Figure 4A). Suspensions of strain conidia (5 × 104 conidia/ml) were inoculated in barley leaves after 4 days, and the ∆Moacb1 mutant had fewer lesions than 70-15 and the complemented strains (Figure 4B). Similarly, only a few small brown spots were observed on rice leaves infected with ∆Moacb1 mutant conidia after the conidial suspension (5 × 104 conidia/ml) was sprayed onto 2-week-old susceptible rice seedlings for 7 days (Figures 4C,D). In order to further analyze the infection ability of ΔMoacb1 mutant, we observed the infection levels of strains on leaf sheath and leaf, respectively. For the convenience of statistics, invasive hyphae are divided into three types: Type 1 has only one short invasive hyphae; Type 2 has two invasive hyphae; Type 3 forms three or more long invasive hyphae. After 36 h of infection of leaves (Figure 4E) and leaf sheaths (Figure 4G), it was found that the invasive hyphae of ΔMoacb1 mutant was mainly type 1, while the invasive hyphae of wild-type 70-15 and the complemented strains was mainly type 3 (Figures 4F,H). The findings demonstrated the significance of MoAcb1 as a pathogenic component.
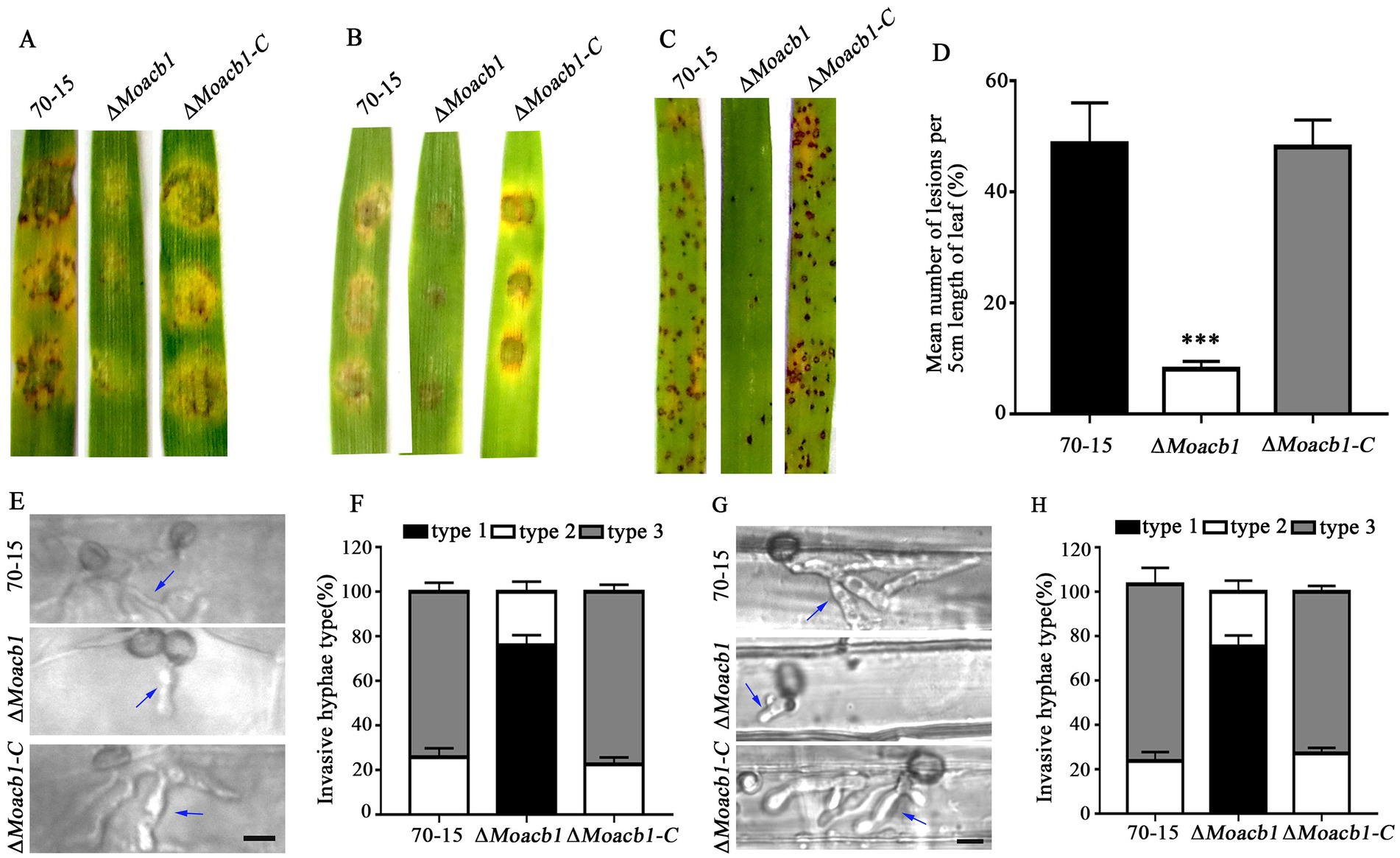
Figure 4. MoAcb1 affects the pathogenicity of M. oryzae. (A) Mycelial plugs of the 70-15, ∆Moacb1 and ∆Moacb1-C strains were placed on isolated barley leaves, and photographs of pathogenicity were taken 4 days later. (B) Conidial suspensions of the 70-15, ∆Moacb1 and ∆Moacb1-C strains were inoculated on isolated barley leaves, and photographs of pathogenicity were taken 4 days later. (C) Conidial suspensions of the 70-15, ∆Moacb1 and ∆Moacb1-C strains were sprayed on isolated rice leaves, and photographs of pathogenicity were taken 5 days later. (D) Quantification of the lesions number per 5 cm length of rice leaf. Asterisk represents significant difference (p < 0.001). (E) Pictures were taken of the leaves infected by wild type 70-15, the ∆Moacb1 mutant and complement strain at 36 hpi. The arrow points to the invasive hyphae. (F,H) Infection types produced by different strains (70-15, ∆Moacb1 and ∆Moacb1-C) were counted, and the experiment was repeated three times with three replicates each time. (G) Pictures were taken of 70-15, ∆Moacb1 and ∆Moacb1-C strains infecting leaf sheath at 36 hpi. The arrow points to the invasive hyphae.
Subcellular localization of MoAcb1 protein
To explore the intracellular localization of the MoAcb1 protein, We observed the MoAcb1-GFP strain under a Laser confocal microscope and MoAcb1-GFP was found in the ER. Collecting conidia from complete medium (CM) and stained with ER tracker. As shown in Figure 5, we observed that the conidial cells contained multiple fluorescent spots that overlapped with the ER tracker. The experimental results demonstrated that MoAcb1 is mainly localized in the ER of M. oryzae.
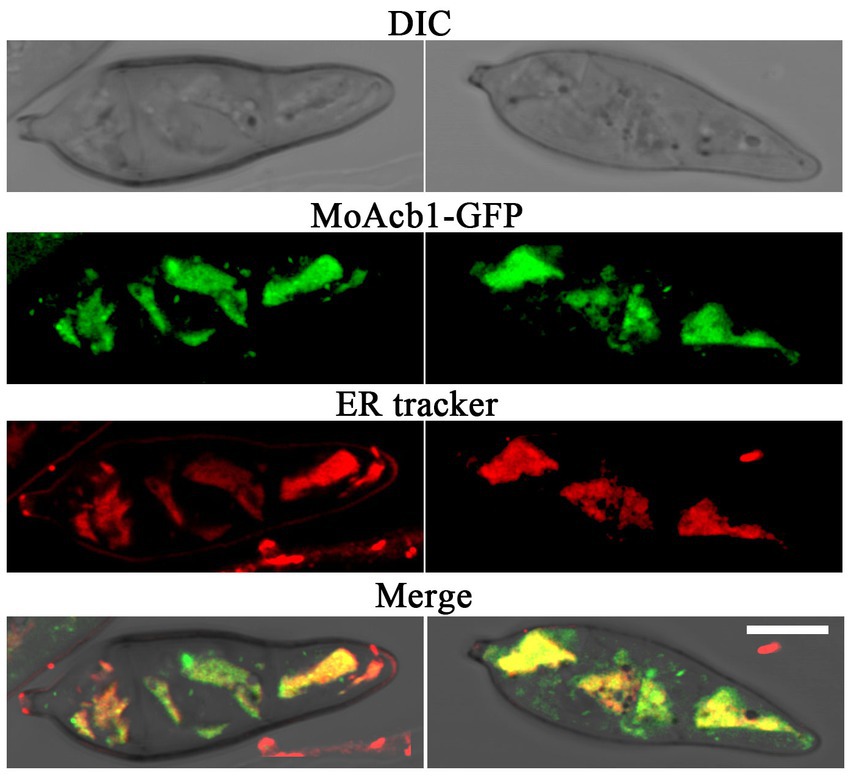
Figure 5. Subcellular localization of MoAcb1. Colocalization of MoAcb1-GFP and ER tracker Red in the conidia. Bar, 5 μm.
Deletion of MoACB1 delays glycogen utilization and degradation
In M. oryzae, to raise the turgor pressure of the appressoria and speed up penetration into the host (Thines et al., 2000), the lipids and glycogen accumulated in conidia are degraded and then transported through germ tubes to the appressoria (Howard et al., 1991; Wilson and Talbot, 2009). Due to the impaired appressorium turgor pressure of the ∆Moacb1 mutant, we decided to study the distribution of glycogen and lipid droplets of the ∆Moacb1 mutant during appressorium formation. Conidial germination was induced on the hydrophobic surface, and after KI/I2 staining, it was observed that there was abundant glycogen in the conidia, germ tubes and nascent appressorium of the 70-15 and ∆Moacb1 mutant strains at 0 h and 4 h, but the utilization of glycogen in the conidia in the ∆Moacb1 mutant was significantly hindered at 8 hpi (Figure 6A). In wild-type 70-15, glycogen in 65.3% of conidia is utilized, while only 26% of the conidia glycogen in the ∆Moacb1 mutant were utilized (Figure 6C). At 24 hpi, 70.0% of the 70-15 appressoria were degraded and utilized, while 55.6% of the appressoria of the ∆Moacb1 mutant were degraded and utilized (Figure 6B). Unlike for glycogen, the strains did not differ significantly in lipid droplet translocation. The results showed that MoAcb1 is essential for the utilization and degradation of glycogen.
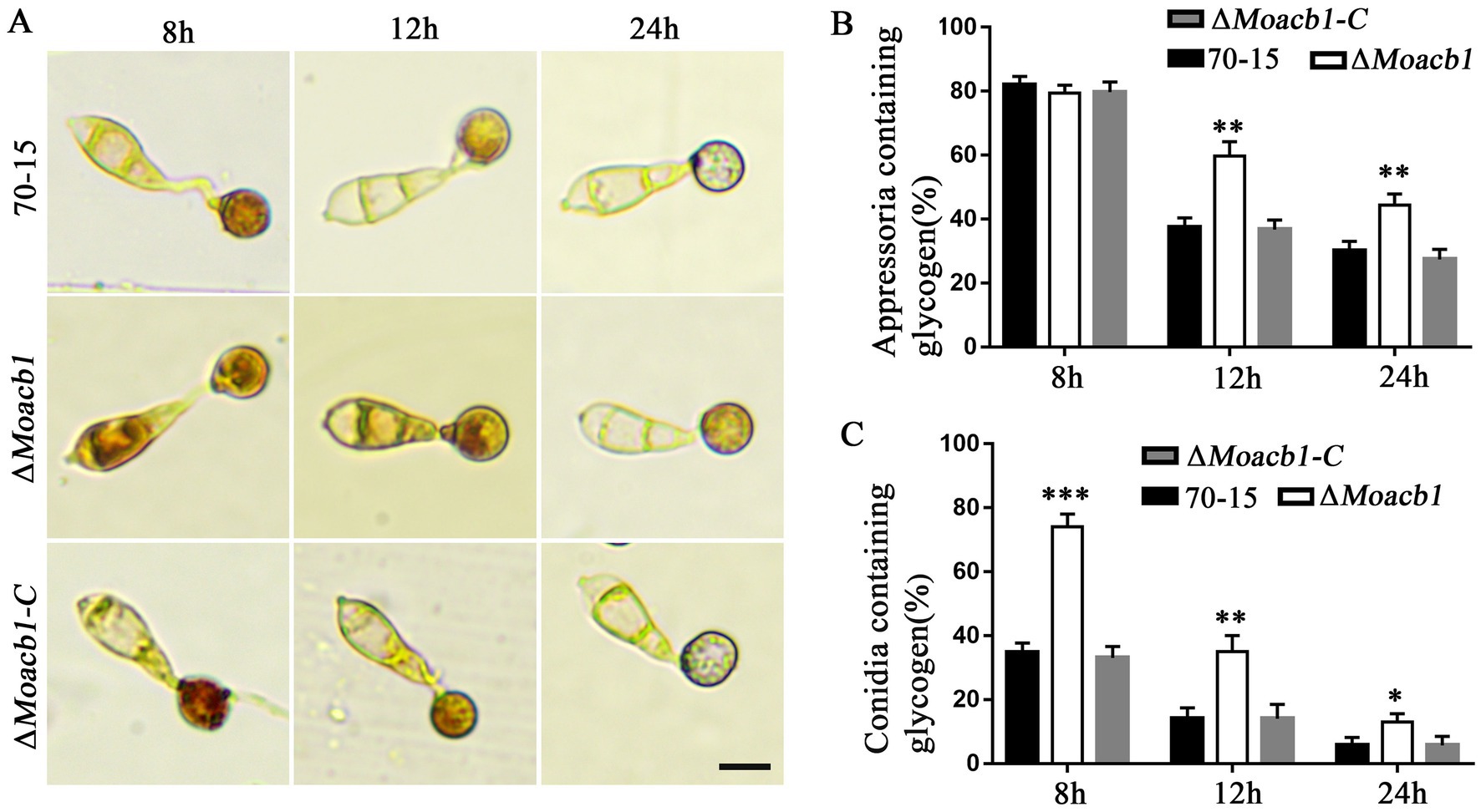
Figure 6. Glycogen utilization during appressorium development. (A) Distribution of glycogen during appressorium development. Samples were stained with KI/I2 solution at different time periods, and glycogen is dark brown under the microscope. Bar, 10 μm. (B) Proportion of appressoria containing glycogen, with standard deviation indicated by error bars. T-test was used to test for significant differences. **p < 0.01. (C) Proportion of conidia containing glycogen, with standard deviation indicated by error bars. T-test test was used to test for significant differences. *p < 0.05, **p < 0.01, ***p < 0.001.
MoAcb1 affects the utilization of certain carbon and nitrogen sources by Magnaporthe oryzae
Since MoAcb1 is an acyl-CoA-binding protein, it specifically binds medium-chain and long-chain acyl-CoA esters and regulates lipid metabolism to some extent (Mogensen et al., 1987). Therefore, we performed experiments on the utilization of a variety of nonfermented carbon sources by the 70-15 and ∆Moacb1 mutant. For this purpose, we replaced 1% of the glucose in the base minimal medium (MM) with other carbon sources, including sodium acetate, olive oil, palmitic acid, ferulic acid, and tetradecanoic acid (Supplementary Figure S1A). The results showed that the ∆Moacb1 mutant had different utilization rates of the different carbon sources. The utilization rate of the carbon sources was calculated by inoculating the three strains (70-15, ∆Moacb1 and ∆Moacb1-C) on MM with 1% glucose from a carbon source as the control. The results showed that the utilization rate of the ∆Moacb1 mutant for three of the carbon sources was higher than that of 70-15, and the growth-promoting effects were 74.3% for sodium acetate, 72.2% for olive oil, 72.3% for palmitic acid. However, the utilization rate of the tetradecanoic acid by ∆Moacb1 mutant was lower than that by 70-15 (Supplementary Figure S1B). The results indicated that the deletion of the MoACB1 gene affects the utilization of different carbon sources.
After analyzing the carbon source utilization of the mutant, we hypothesized that the gene also affected nitrogen source utilization. The NaNO3 concentration of the only nitrogen source in the MM was changed to 10 mM as a control, and NaNO2, (NH4)2SO4, NH4NO3, glutamine (Gln) and histidine (His) were selected as the test nitrogen sources (Supplementary Figure S1C). The data were analyzed, and it was found that the utilization rate by the ΔMoacb1 mutant of (NH4)2SO4, NH4NO3 and His was higher than that by the 70-15 strain, showing a growth-promoting effect. The utilization rate of NaNO2 and Gln by the ΔMoacb1 mutant was lower than that by the 70-15 strain, showing a growth-inhibiting effect (Supplementary Figure S1D). These results indicated that the deletion of the MoACB1 gene also affects the utilization of different nitrogen sources.
MoAcb1 is involved in the regulation of hypertonic stress responses and DNA replication stress responses
The key of M. oryzae infection is its ability to resist external environmental stresses (Li et al., 2012). To detect strain sensitivity to hypertonic stress, 70-15, ΔMoacb1 mutant and ∆Moacb1-C were inoculated on CM plates containing 0.5 M potassium chloride, 1 M sucrose, 0.5 M sodium chloride, and 1 M sorbitol (Supplementary Figure S2A). Using CM as a control, the inhibition rate was calculated, and the experimental data were calculated from the results of three independent experiments. As shown in Supplementary Figure S2C, the loss of the MoACB1 gene resulted in increased resistance to ionic hyperosmotic stress and enhanced growth fitness, indicating that the MoACB1 gene plays an important role in adaptation to hyperosmotic stress.
The completion of DNA replication controls the initial development of appressorium and the appearance of penetration pegs (Saunders et al., 2010; Oses-Ruiz et al., 2017). Hydroxyurea (HU) and methyl mesylate (MMS) are DNA damage agents. To elucidate the cellular function of MoAcb1 in M. oryzae, we investigated the sensitivity of the ∆Moacb1 mutant to HU and MMS. ∆Moacb1 mutant and ∆Moacb1-C and 70-15 were inoculated on CM plates containing 20 mM HU and 0.02% MMS. The inhibition rate was calculated by using CM as a control. Loss of the MoACB1 gene increased strain resistance to DNA-damaging agents and enhanced growth fitness. In particular, the growth rate of the ∆Moacb1 mutant inoculated on HU was significantly higher than that of strain 70-15, which was more responsive to DNA replication pressure, indicating that MoACB1 played a role in the DNA replication stress response (Supplementary Figures S2B,D).
MoAcb1 is involved in the regulation of ER-phagy
To test whether MoAcb1 regulates autophagy, we used Western blot to detect the content of full-length GFP-MoAtg8 and free GFP in the 70-15 and ∆Moacb1 mutant (Nair et al., 2012). Under vegetative conditions, autophagy was weak, with few free GFP bands. Therefore, we tested the GFP-MoAtg8 activity induced under nitrogen starvation at 3 h and 6 h, respectively. The free GFP bands increased when the 70-15 and ∆Moacb1 mutant were induced by nitrogen starvation medium (SD-N); however, there was no significant difference between the mutant and 70-15 (Supplementary Figure S3). Since this experiment found that MoAcb1 is mainly localized in the ER, We further detected whether ER-phagy was affected in the ΔMoacb1 mutant. Strains were inoculated on CM containing 2 mM dithiothreitol (DTT, a chemical inducer of ER-phagy) and cultured for 8 days. The ∆Moacb1 mutant strain showed greater resistance and grew better than the 70-15 and ∆Moacb1-C strain (Figures 7A,B). Next, we transferred GFP- MoSec62 (ER-phagy marker) into the 70-15 and ∆Moacb1 mutant and found that 70-15 free GFP bands were not detected and that the GFP- MoSec62 band of the ∆Moacb1 mutant was stronger than that of 70-15. After induction in liquid CM containing 5 mM DTT for 3 h and 6 h, respectively, the free GFP bands were increased in 70-15, and the GFP- MoSec62 band in the ∆Moacb1 mutant remained numerous (Figure 7C). The results showed that the ER-phagy of the ∆Moacb1 mutant was strengthened, and MoAcb1 played a negative role in regulating ER-phagy in M. oryzae.
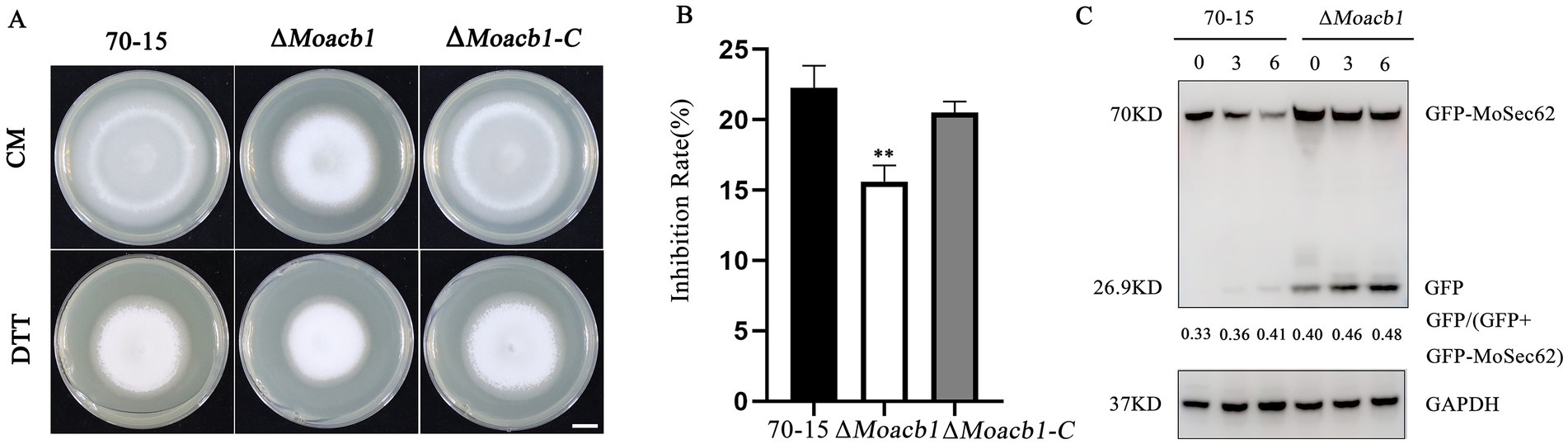
Figure 7. MoAcb1 participated in the analysis of ER autophagy. (A) The 70-15, ∆Moacb1 and ∆Moacb1-C strains were inoculated on CM plates containing 2 mM DTT at 25°C for 8 days. Bar, 1 cm. (B) Relative inhibition rate of the 70-15, the ∆Moacb1 and ∆Moacb1-C strains on CM medium containing 2 mM DTT, with the standard deviation indicated by error bars. T-test was used to test for significant differences. **p < 0.01. (C) Immunoblot analysis of MoSec62-GFP proteolysis in the 70-15 and ∆Moacb1 mutant strains.
Discussion
Numerous studies have shown that acyl-CoA binding protein, which is frequently present in eukaryotic cells, has a high affinity for acyl-CoA. Acb protein participates in fatty acid oxidation in addition to transporting acyl-CoA (Sumper et al., 1969; Rasmussen et al., 1994). Fatty acid metabolism takes a variety of forms, which helps to show the variety of Acb protein gene functions and the flexibility of Acb protein genes in subcellular localization (Cohen Simonsen et al., 2003). Acb protein homologs were identified in animals, plants, fungi, protists and 11 eubacterial species. However, there are few studies on the function of the Acb protein in plant-pathogenic fungi. Whether the Acb1 protein plays a conserved function in blast fungus is still unknown. We knocked out MoACB1 in wild-type 70-15 and found that MoAcb1 is involved in conidiation, appressorium development, and pathogenicity in M. oryzae.
Conidial germination in rice blast fungus produces appressoria. To achieve plant infection in the absence of exogenous nutrients, the appressorium uses autophagy pathway to breakdown the nutrients in conidia, continuously accumulates glycerol, and then produces enough turgor pressure to breach the host plant’s cuticle (Qu et al., 2020). In our study, the number of conidia produced by the ∆Moacb1 mutant was significantly reduced, and the proportion of conidia with abnormal morphology was significantly increased. As shown in Figure 2C, the ∆Moacb1-N2 could partly rescue the phenotype of conidia production, We suspect that it may be related to the fact that the ∆Moacb1-N2 contains an Acb-binding domain. This indicated that ACB domain affected the conidiation of M. oryzae. In addition, the degradation rate of glycogen in the ∆Moacb1 mutant was slowed, and the turgor pressure was also significantly reduced. The ∆Moacb1 mutant almost completely lost its virulence. Acb protein partly regulates the β oxidation of fatty acids, the elongation of fatty acid chains, and lipid metabolism (Andersen et al., 1991). Therefore, we replaced the carbon sources in MM with different fatty acids, and we observed that the deletion of the MoACB1 gene affected the utilization of different carbon sources by M. oryzae, indicating that the function of MoACB1 of affecting the utilization of fatty acids was conserved in rice blast fungus.
Autophagy is an evolutionarily conserved intracellular degradation process in which some damaged proteins or organelles are encapsulated by double-membrane autophagy vesicles, then delivered to lysosomes or vacuoles to be degraded and cycled (Zhou et al., 2018; Zhu et al., 2021), thereby fulfilling the metabolic needs of the cells and the renewal of certain organelles. According to the different substrates, autophagy can be divided into macroautophagy and selective autophagy (Wang et al., 2022). In Fusarium graminearum, autophagy is required for normal vegetative growth, complete virulence, and toxin biosynthesis (Lv et al., 2017). In human and mouse cells, the Acb protein also regulates autophagy. Both intracellular depletion of the Acb protein and its addition to the extracellular environment inhibit autophagy (Knudsen et al., 1994). In our study, it was discovered that there was no discernible difference between the wild-type and the ∆Moacb1 mutant in the rate of degradation of the autophagy-tagged protein GFP-MoAtg8, proving that MoAcb1 was not involved in macroautophagy. To maintain homeostasis in the ER, the endoplasmic reticulum associated protein degradation mechanism is initiated. In this study, We found that ∆Moacb1 mutant was more resistant in CM plates containing 2 mM DTT, stimulating ER-phagy. As shown in Figure 7C, compared with 70-15, GFP-MoSec62 in ∆Moacb1 mutant degrades more, We suspected that mutant require higher ER-phagy to maintain homeostasis.
In our study, we found that MoAcb1 is involved in ER-phagy, but the specific mechanism is unclear and needs to be further studied. MoAcb1 plays an important role in conidial growth, appressorium formation, hypertonic stress and pathogenicity of M. oryzae, providing a reference for subsequent exploration of other research aspects.
Materials and methods
Fungal strains and growth conditions
In this experiment, M. oryzae strain 70-15 was always used as the wild-type strain, and the mutant strain ∆Moacb1 and the complemented strain ∆Moacb1-C were obtained by gene knockout. Unless stated, all strains were grown on CM plates and then grown in a 25°C incubator for 6–8 days (Yang et al., 2010). In the experiment to explore the ability to resist external environmental stress, the designated strains were inoculated on CM plates containing 0.5 M sodium chloride, 1 M sorbitol, 0.5 M potassium chloride and 1 M sucrose, cultivated at 25°C for 7 days and photographed. All assays in this study were performed in triplicate.
Fluorescence localization
The conidia of MoAcb1-GFP strain were washed after 8 days of culture in CM. The conidia were stained with 1 μM ER-tracker (Invitrogen, America, E34250) dye solution at 28°C for 20 min and then fluorescence was observed with a laser confocal microscopy (LSM 880).
Gene knockout and mutant complementation
Using a high-throughput gene knockout system (Lu et al., 2014), the MoACB1 gene was knocked out, and then the copy of this gene was inserted into the deleted mutant to obtain the ∆Moacb1-C complement strain. To construct knockout vectors PKO3A, containing a suicide gene, HSVtk, we amplified upstream and downstream fragments of the target gene with primers and ligated the hygromycin B resistance gene (HPH) as a selectable marker (Supplementary Figure S4B; Liu et al., 2021). All these fragments were cloned using Phanta Max Ultra-Fidelity DNA Polymerase (Vazyme, China) and ligated into the HindIII/XbaI-cut linearized vector pKO3A (Lu et al., 2014). The recombination plasmids were transferred into Agrobacterium tumefaciens and the knockout was performed by ATMT (Agrobacterium tumefaciens mediated transformation) methods. The mutants were screened with CM plates containing the corresponding resistance and further verified by PCR. During the experiment, all PCR products and linearized vectors were verified by agarose gel electrophoresis (Supplementary Figure S4A) and then purified by a DNA gel extraction kit (Axygen, Hangzhou, China). In addition, qPCR confirmed only one copy of the HPH gene in MoABC1 deletion mutant (Supplementary Figure S4D) and the MoACB1 gene could not detected in MoACB1 deletion mutant (Supplementary Figure S4C). Relative expression was calculated according to 2−ΔΔCT. Actin gene was used as the reference gene of qPCR. For the complementation assay, the target gene MoACB1 was amplified with the upstream promoter, fused with the PKD5-GFP vector containing sulfonylurea resistance gene (SUR), and transferred into the ΔMoacb1 mutant to obtain the complement strain ΔMoacb1-C. Complement strains were identified by phenometric analysis, fluorescence observation and qPCR. The PCR primers used in this study are listed in Table 1.
Phenotypic characterization
For vegetative growth and conidiation experiments, the strains were grown in CM. Eight days after inoculation, colony diameter and conidiation were determined. For the conidial germination test, the conidia were washed out and diluted to 5 × 104 cells/ml, and the suspensions were induced on the hydrophobic membrane for 4 h, 6 h, 8 h, and 24 h (Kong et al., 2012).
For pathogenicity assays, isolated barley leaves were inoculated with 70-15, ∆Moacb1 and ∆Moacb1-C mycelial plugs and conidial suspensions (5 × 104 cells/ml). The cells were cultured at 25°C for 4 days, and the diseased spots were observed and photographed (Kim et al., 2005). Moreover, we performed live-cell imaging using isolated barley leaves and leaf sheaths to observe how invasive hyphae developed within the host cell. Conidia suspension was inoculated in leaves and leaf sheaths and cultured in a wet box to observe and recorded the infection at different time periods (Zhou et al., 2009; Fernandez and Wilson, 2014). As previously mentioned, we measured the turgor pressure of appressoria using glycerol solutions of 0.5 M, 1.0 M, 1.5 M, and 2.0 M. Glycogen was stained with KI/I2 solution to detect its breakdown. Each of the aforementioned experiments was carried out three times.
Nutrient replacement and growth stress test
To test whether there is a difference in the utilization of different nutrients by mutants, we replaced the carbon and nitrogen sources in MM, respectively. In the carbon source utilization experiment, 1% glucose in the medium is replaced by sodium acetate, olive oil, palmitic acid, ferulic acid, and tetradecanoic acid, respectively. In the nitrogen source utilization experiment, 10 mM of NaNO3 is replaced by NaNO2, (NH4)2SO4, NH4NO3, Gln and His, respectively. The strain is incubated on plates for 8 days and the diameter of each sample is measured to calculate the growth rate. The procedure was repeated 3 times for each strain.
To test the response of mutants to hyperosmotic stress, CM containing 0.5 M potassium chloride, 1 M sucrose, 0.5 M sodium chloride, and 1 M sorbitol was prepared. To test the susceptibility of mutants to DNA damage reagents, CM containing 20 mM HU and 0.02%MMS was prepared. The strains were incubated on plates for 8 days and the diameter of each sample was measured to calculate the inhibition rate. The procedure was repeated 3 times for each strain.
Autophagy induction and western blot analysis
To detect autophagy flux, we transferred GFP-MoAtg8 into 70-15 and ∆Moacb1 mutant. The transferred strains were cultured in liquid CM at 25°C and 150 rpm for 48 h, followed by induction in SD-N medium for 3 h and 6 h to induce autophagy. To induce ER-phagy, GFP-MoSec62 was transferred into 70-15 and ∆Moacb1 mutant. The mycelia of strain were transferred into SD-N with 5 mM DTT, the culture conditions are the same as above. For autophagy and ER-phagy, the free GFP and fusion bands were detected by GFP antibody (Huabiao, Hangzhou, China) with 12% SDS-PAGE. The protein GAPDH was used as a loading control.
Data availability statement
The original contributions presented in the study are included in the article/Supplementary material, further inquiries can be directed to the corresponding author.
Author contributions
LL, X-MZ, and J-DB designed all the experiments. L-HZ and HL participated in data statistical analysis. NC wrote the first draft. LL, X-MZ, and F-CL provided fund support. All authors contributed to the article and approved the submitted version.
Funding
This work is supported by grants (32200162 to LL, 31970140 to F-CL, 32100159 to X-MZ) from the National Natural Science Foundation of China.
Conflict of interest
The authors declare that the research was conducted in the absence of any commercial or financial relationships that could be construed as a potential conflict of interest.
Publisher’s note
All claims expressed in this article are solely those of the authors and do not necessarily represent those of their affiliated organizations, or those of the publisher, the editors and the reviewers. Any product that may be evaluated in this article, or claim that may be made by its manufacturer, is not guaranteed or endorsed by the publisher.
Supplementary material
The Supplementary material for this article can be found online at: https://www.frontiersin.org/articles/10.3389/fmicb.2023.1179536/full#supplementary-material
Footnotes
References
Andersen, K. V., Ludvigsen, S., Mandrup, S., Knudsen, J., and Poulsen, F. M. (1991). The secondary structure in solution of acyl-coenzyme A binding protein from bovine liver using 1H nuclear magnetic resonance spectroscopy. Biochemistry 30, 10654–10663. doi: 10.1021/bi00108a008
Burton, M., Rose, T. M., Færgeman, N. J., and Knudsen, J. (2005). Evolution of the acyl-CoA binding protein (ACBP). Biochem. J. 392, 299–307. doi: 10.1042/BJ20050664
Cai, Y. Y., Wang, J. Y., Wu, X. Y., Liang, S., Zhu, X. M., Li, L., et al. (2022). MoOpy2 is essential for fungal development, pathogenicity, and autophagy in Magnaporthe oryzae. Environ. Microbiol. 24, 1653–1671. doi: 10.1111/1462-2920.15949
Chen, Z. W., Agerberth, B., Gell, K., Andersson, M., Mutt, V., Ostenson, C. G., et al. (1988). Isolation and characterization of porcine diazepam-binding inhibitor, a polypeptide not only of cerebral occurrence but also common in intestinal tissues and with effects on regulation of insulin release. Eur. J. Biochem. 174, 239–244. doi: 10.1111/j.1432-1033.1988.tb14088.x
Cheng, J., Yin, Z., Zhang, Z., and Liang, Y. (2018). Functional analysis of MoSnf7 in Magnaporthe oryzae. Fungal Genet. Biol. 121, 29–45. doi: 10.1016/j.fgb.2018.09.005
Cohen Simonsen, A., Bernchou Jensen, U., Faergeman, N. J., Knudsen, J., and Mouritsen, O. G. (2003). Acyl-coenzyme A organizes laterally in membranes and is recognized specifically by acyl-coenzyme A binding protein. FEBS Lett. 552, 253–258. doi: 10.1016/S0014-5793(03)00970-0
Dean, R., Van Kan, J. A., Pretorius, Z. A., Hammond-Kosack, K. E., Di Pietro, A., Spanu, P. D., et al. (2012). The Top 10 fungal pathogens in molecular plant pathology. Mol. Plant Pathol. 13, 414–430. doi: 10.1111/j.1364-3703.2011.00783.x
Di Pietro, A., and Talbot, N. J. (2017). Fungal pathogenesis: combatting the oxidative burst. Nat. Microbiol. 2:17095. doi: 10.1038/nmicrobiol.2017.95
Dufey, E., Sepúlveda, D., Rojas-Rivera, D., and Hetz, C. (2014). Cellular mechanisms of endoplasmic reticulum stress signaling in health and disease. 1. An overview. Am. J. Physiol. Cell Physiol. 307, C582–C594. doi: 10.1152/ajpcell.00258.2014
Duran, J. M., Anjard, C., Stefan, C., Loomis, W. F., and Malhotra, V. (2010). Unconventional secretion of Acb1 is mediated by autophagosomes. J. Cell Biol. 188, 527–536. doi: 10.1083/jcb.200911154
Ebbole, D. J. (2007). Magnaporthe as a model for understanding host-pathogen interactions. Annu. Rev. Phytopathol. 45, 437–456. doi: 10.1146/annurev.phyto.45.062806.094346
Feng, W., Yin, Z., Wu, H., Liu, P., Liu, X., Liu, M., et al. (2021). Balancing of the mitotic exit network and cell wall integrity signaling governs the development and pathogenicity in Magnaporthe oryzae. PLoS Pathog. 17:e1009080. doi: 10.1371/journal.ppat.1009080
Fernandez, J., and Orth, K. (2018). Rise of a cereal killer: the biology of Magnaporthe oryzae biotrophic growth. Trends Microbiol. 26, 582–597. doi: 10.1016/j.tim.2017.12.007
Fernandez, J., and Wilson, R. A. (2014). Characterizing roles for the glutathione reductase, thioredoxin reductase and thioredoxin peroxidase-encoding genes of Magnaporthe oryzae during rice blast disease. PLoS One 9:e87300. doi: 10.1371/journal.pone.0087300
Fumagalli, F., Noack, J., Bergmann, T. J., Cebollero, E., Pisoni, G. B., Fasana, E., et al. (2016). Translocon component Sec62 acts in endoplasmic reticulum turnover during stress recovery. Nat. Cell Biol. 18, 1173–1184. doi: 10.1038/ncb3423
Gaigg, B., Neergaard, T. B. F., Schneiter, R., Hansen, J. K., Færgeman, N. J., Jensen, N. A., et al. (2001). Depletion of acyl-coenzyme A-binding protein affects sphingolipid synthesis and causes vesicle accumulation and membrane defects in Saccharomyces cerevisiae. Mol. Biol. Cell 12, 1147–1160. doi: 10.1091/mbc.12.4.1147
Gao, H. M., Liu, X. G., Shi, H. B., Lu, J. P., Yang, J., Lin, F. C., et al. (2013). MoMon1 is required for vacuolar assembly, conidiogenesis and pathogenicity in the rice blast fungus Magnaporthe oryzae. Res. Microbiol. 164, 300–309. doi: 10.1016/j.resmic.2013.01.001
Glick, D., Barth, S., and Macleod, K. F. (2010). Autophagy: cellular and molecular mechanisms. J. Pathol. 221, 3–12. doi: 10.1002/path.2697
Howard, R. J., Ferrari, M. A., Roach, D. H., and Money, N. P. (1991). Penetration of hard substrates by a fungus employing enormous turgor pressures. Proc. Natl. Acad. Sci. U. S. A. 88, 11281–11284. doi: 10.1073/pnas.88.24.11281
Hu, S., Ye, H., Cui, Y., and Jiang, L. (2020). AtSec62 is critical for plant development and is involved in ER-phagy in Arabidopsis thaliana. J. Integr. Plant Biol. 62, 181–200. doi: 10.1111/jipb.12872
Ichimura, Y., Imamura, Y., Emoto, K., Umeda, M., Noda, T., and Ohsumi, Y. (2004). In vivo and in vitro reconstitution of Atg8 conjugation essential for autophagy. J. Biol. Chem. 279, 40584–40592. doi: 10.1074/jbc.M405860200
Kim, S., Ahn, I. P., Rho, H. S., and Lee, Y. H. (2005). MHP1, a Magnaporthe grisea hydrophobin gene, is required for fungal development and plant colonization. Mol. Microbiol. 57, 1224–1237. doi: 10.1111/j.1365-2958.2005.04750.x
Knudsen, J., Faergeman, N. J., Skøtt, H., Hummel, R., Børsting, C., Rose, T. M., et al. (1994). Yeast acyl-CoA-binding protein: acyl-CoA-binding affinity and effect on intracellular acyl-CoA pool size. Biochem. J. 302, 479–485. doi: 10.1042/bj3020479
Kong, L. A., Yang, J., Li, G. T., Qi, L. L., Zhang, Y. J., Wang, C. F., et al. (2012). Different chitin synthase genes are required for various developmental and plant infection processes in the rice blast fungus Magnaporthe oryzae. PLoS Pathog. 8:e1002526. doi: 10.1371/journal.ppat.1002526
Li, G., Zhou, X., and Xu, J. R. (2012). Genetic control of infection-related development in Magnaporthe oryzae. Curr. Opin. Microbiol. 15, 678–684. doi: 10.1016/j.mib.2012.09.004
Liu, T. B., Liu, X. H., Lu, J. P., Zhang, L., Min, H., and Lin, F. C. (2010). The cysteine protease MoAtg4 interacts with MoAtg8 and is required for differentiation and pathogenesis in Magnaporthe oryzae. Autophagy 6, 74–85. doi: 10.4161/auto.6.1.10438
Liu, X., Pan, X., Chen, D., Yin, C., Peng, J., Shi, W., et al. (2021). Prp19-associated splicing factor Cwf15 regulates fungal virulence and development in the rice blast fungus. Environ. Microbiol. 23, 5901–5916. doi: 10.1111/1462-2920.15616
Lu, J., Cao, H., Zhang, L., Huang, P., and Lin, F. (2014). Systematic analysis of Zn2Cys6 transcription factors required for development and pathogenicity by high-throughput gene knockout in the rice blast fungus. PLoS Pathog. 10:e1004432. doi: 10.1371/journal.ppat.1004432
Lv, W., Wang, C., Yang, N., Que, Y., Talbot, N. J., and Wang, Z. (2017). Genome-wide functional analysis reveals that autophagy is necessary for growth, sporulation, deoxynivalenol production and virulence in Fusarium graminearum. Sci. Rep. 7:11062. doi: 10.1038/s41598-017-11640-z
Martin-Urdiroz, M., Oses-Ruiz, M., Ryder, L. S., and Talbot, N. J. (2016). Investigating the biology of plant infection by the rice blast fungus Magnaporthe oryzae. Fungal Genet. Biol. 90, 61–68. doi: 10.1016/j.fgb.2015.12.009
Mogensen, I. B., Schulenberg, H., Hansen, H. O., Spener, F., and Knudsen, J. (1987). A novel acyl-CoA-binding protein from bovine liver. Effect on fatty acid synthesis. Biochem. J. 241, 189–192. doi: 10.1042/bj2410189
Nair, U., Yen, W. L., Mari, M., Cao, Y., Xie, Z., Baba, M., et al. (2012). A role for Atg8-PE deconjugation in autophagosome biogenesis. Autophagy 8, 780–793. doi: 10.4161/auto.19385
Nakatogawa, H., Ichimura, Y., and Ohsumi, Y. (2007). Atg8, a ubiquitin-like protein required for autophagosome formation, mediates membrane tethering and hemifusion. Cells 130, 165–178. doi: 10.1016/j.cell.2007.05.021
Oses-Ruiz, M., Sakulkoo, W., Littlejohn, G. R., Martin-Urdiroz, M., and Talbot, N. J. (2017). Two independent S-phase checkpoints regulate appressorium-mediated plant infection by the rice blast fungus Magnaporthe oryzae. Proc. Natl. Acad. Sci. U. S. A. 114, E237–E244. doi: 10.1073/pnas.1611307114
Qu, Y., Wang, J., Zhu, X., Dong, B., Liu, X., Lu, J., et al. (2020). The P5-type ATPase Spf1 is required for development and virulence of the rice blast fungus Pyricularia oryzae. Curr. Genet. 66, 385–395. doi: 10.1007/s00294-019-01030-5
Rasmussen, J. T., FÆrgeman, N. J., Kristiansen, K., and Knudsen, J. (1994). Acyl-CoA-binding protein (ACBP) can mediate intermembrane acyl-CoA transport and donate acyl-CoA for beta-oxidation and glycerolipid synthesis. Biochem. J. 299, 165–170. doi: 10.1042/bj2990165
Rasmussen, J. T., Rosendal, J., and Knudsen, J. (1993). Interaction of acyl-CoA binding protein (ACBP) on processes for which acyl-CoA is a substrate, product or inhibitor. Biochem. J. 292, 907–913. doi: 10.1042/bj2920907
Roduit, R., Nolan, C., Alarcon, C., Moore, P., Barbeau, A., Delghingaro-Augusto, V., et al. (2004). A role for the malonyl-CoA/long-chain acyl-CoA pathway of lipid signaling in the regulation of insulin secretion in response to both fuel and nonfuel stimuli. Diabetes 53, 1007–1019. doi: 10.2337/diabetes.53.4.1007
Rose, T. M., Schultz, E. R., and Todaro, G. J. (1992). Molecular cloning of the gene for the yeast homolog (ACB) of diazepam binding inhibitor/endozepine/acyl-CoA-binding protein. Proc. Natl. Acad. Sci. U. S. A. 89, 11287–11291. doi: 10.1073/pnas.89.23.11287
Rosendal, J., Ertbjerg, P., and Knudsen, J. (1993). Characterization of ligand binding to acyl-CoA-binding protein. Biochem. J. 290, 321–326. doi: 10.1042/bj2900321
Saunders, D. G., Aves, S. J., and Talbot, N. J. (2010). Cell cycle-mediated regulation of plant infection by the rice blast fungus. Plant Cell 22, 497–507. doi: 10.1105/tpc.109.072447
Schjerling, C. K., Hummel, R., Hansen, J. K., Børsting, C., Mikkelsen, J. M., Kristiansen, K., et al. (1996). Disruption of the gene encoding the acyl-CoA-binding protein (ACB1) perturbs acyl-CoA metabolism in Saccharomyces cerevisiae. J. Biol. Chem. 271, 22514–22521. doi: 10.1074/jbc.271.37.22514
Sumper, M., Riepertinger, C., Lynen, F., and Oesterhelt, D. (1969). Synthesis of various carboxylic acids by the fatty acid synthetase multienzyme complex of yeast and the explanation for their structure. Eur. J. Biochem. 10, 377–387. doi: 10.1111/j.1432-1033.1969.tb00701.x
Thines, E., Weber, R. W., and Talbot, N. J. (2000). MAP kinase and protein kinase A-dependent mobilization of triacylglycerol and glycogen during appressorium turgor generation by Magnaporthe grisea. Plant Cell 12, 1703–1718. doi: 10.1105/tpc.12.9.1703
Wang, J., Huang, Z., Huang, P., Wang, Q., Li, Y., Liu, X. H., et al. (2022). The plant homeodomain protein Clp1 regulates fungal development, virulence, and autophagy homeostasis in Magnaporthe oryzae. Microbiol Spectr 10:e0102122. doi: 10.1128/spectrum.01021-22
Wilson, R. A. (2021). Magnaporthe oryzae. Trends Microbiol. 29, 663–664. doi: 10.1016/j.tim.2021.03.019
Wilson, R. A., and Talbot, N. J. (2009). Under pressure: investigating the biology of plant infection by Magnaporthe oryzae. Nat. Rev. Microbiol. 7, 185–195. doi: 10.1038/nrmicro2032
Yang, J., Zhao, X., Sun, J., Kang, Z., Ding, S., Xu, J. R., et al. (2010). A novel protein Com1 is required for normal conidium morphology and full virulence in Magnaporthe oryzae. Mol. Plant-Microbe Interact. 23, 112–123. doi: 10.1094/MPMI-23-1-0112
Yoshimoto, K., Hanaoka, H., Sato, S., Kato, T., Tabata, S., Noda, T., et al. (2004). Processing of ATG8s, ubiquitin-like proteins, and their deconjugation by ATG4s are essential for plant autophagy. Plant Cell 16, 2967–2983. doi: 10.1105/tpc.104.025395
Zheng, W., Zhou, J., He, Y., Xie, Q., Chen, A., Zheng, H., et al. (2015). Retromer is essential for autophagy-dependent plant infection by the rice blast fungus. PLoS Genet. 11:e1005704. doi: 10.1371/journal.pgen.1005704
Zhou, Z., Li, G., Lin, C., and He, C. (2009). Conidiophore stalk-less1 encodes a putative zinc-finger protein involved in the early stage of conidiation and mycelial infection in Magnaporthe oryzae. Mol. Plant-Microbe Interact. 22, 402–410. doi: 10.1094/MPMI-22-4-0402
Zhou, W., Shi, W., Xu, X. W., Li, Z. G., Yin, C. F., Peng, J. B., et al. (2018). Glutamate synthase MoGlt1-mediated glutamate homeostasis is important for autophagy, virulence and conidiation in the rice blast fungus. Mol. Plant Pathol. 19, 564–578. doi: 10.1111/mpp.12541
Zhu, X., Li, L., Wang, J., Zhao, L., Shi, H., Bao, J., et al. (2021). Vacuolar protein-sorting receptor MoVps13 regulates conidiation and pathogenicity in rice blast fungus Magnaporthe oryzae. J Fungi (Basel) 7:1084. doi: 10.3390/jof7121084
Keywords: Magnaporthe oryzae, acyl-coenzyme A binding protein, appressorium development, pathogenicity, ER-phagy
Citation: Cao N, Zhu X-M, Bao J-D, Zhu L-H, Liu H, Lin F-C and Li L (2023) Acyl-coenzyme A binding protein MoAcb1 regulates conidiation and pathogenicity in Magnaporthe oryzae. Front. Microbiol. 14:1179536. doi: 10.3389/fmicb.2023.1179536
Edited by:
Gang Yu, Shanghai Jiao Tong University, ChinaReviewed by:
Jingtao Li, Qingdao Agricultural University, ChinaHuanbin Shi, Chinese Academy of Agricultural Sciences, China
Jian Li, Shandong Academy of Agricultural Sciences, China
Copyright © 2023 Cao, Zhu, Bao, Zhu, Liu, Lin and Li. This is an open-access article distributed under the terms of the Creative Commons Attribution License (CC BY). The use, distribution or reproduction in other forums is permitted, provided the original author(s) and the copyright owner(s) are credited and that the original publication in this journal is cited, in accordance with accepted academic practice. No use, distribution or reproduction is permitted which does not comply with these terms.
*Correspondence: Lin Li, MjE2MTYxNDNAemp1LmVkdS5jbg==