- 1Department of Veterinary Medicine Science, College of Veterinary Medicine, Huazhong Agricultural University, Wuhan, Hubei, China
- 2National Reference Laboratory of Veterinary Drug Residues (HZAU), Wuhan, Hubei, China
- 3MOA Key Laboratory for Detection of Veterinary Drug Residues, Wuhan, Hubei, China
Salmonella spp. is one of the most important foodborne pathogens. Typhoid fever and enteritis caused by Salmonella enterica are associated with 16–33 million infections and 500,000 to 600,000 deaths annually worldwide. The eradication of Salmonella is becoming increasingly difficult because of its remarkable capacity to counter antimicrobial agents. In addition to the intrinsic and acquired resistance of Salmonella, increasing studies indicated that its non-inherited resistance, which commonly mentioned as biofilms and persister cells, plays a critical role in refractory infections and resistance evolution. These remind the urgent demand for new therapeutic strategies against Salmonella. This review starts with escape mechanisms of Salmonella against antimicrobial agents, with particular emphasis on the roles of the non-inherited resistance in antibiotic failure and resistance evolution. Then, drug design or therapeutic strategies that show impressive effects in overcoming Salmonella resistance and tolerance are summarized completely, such as overcoming the barrier of outer membrane by targeting MlaABC system, reducing persister cells by limiting hydrogen sulfide, and applying probiotics or predatory bacteria. Meanwhile, according to the clinical practice, the advantages and disadvantages of above strategies are discussed. Finally, we further analyze how to deal with this tricky problems, thus can promote above novel strategies to be applied in the clinic as soon as possible. We believed that this review will be helpful in understanding the relationships between tolerance phenotype and resistance of Salmonella as well as the efficient control of antibiotic resistance.
1. Introduction
Salmonella spp. is gram-negative, facultative anaerobes and facultative intracellular bacteria that are divided into Salmonella enterica (S. enterica) and Salmonella bongori (Ryan et al., 2017). S. enterica is estimated to cause 16–33 million infectious cases, with 500,000–600,000 annual deaths worldwide (Bula-Rudas et al., 2015). Reportedly, the confirmed number of deaths was 94,530 in the European Union in 2016 (Chlebicz and Śliżewska, 2018). 50% of US swine operations were fecal positive for Salmonella (Bearson, 2022), and the economic losses due to salmonellosis in America are estimated to exceed $ 3.5 billion annually (Kim and Isaacson, 2017). More seriously, in many African countries, the incidence of typhoid fever is above 1/1000 per year, with approximately 1% of patients’ dying (Crump and Heyderman, 2015).
Infections caused by Salmonella may be due to direct contact with infected animals and or indirect contact via their environment. In addition, Salmonella in animal intestines can transfer onto their products due to careless processing or improper hygiene (Yeh et al., 2017). Therefore, animal-derived products are an important media for Salmonella spread and infections. According to the reports, Salmonella causes over 90 million diarrhea-associated diseases annually worldwide, 85% of which are linked to animal food consumption (Huang et al., 2018). Obviously, the animal-derived products and food packaging are one of the main reasons of the salmonellosis spread.
What is more worrying is that the antibiotic resistance of Salmonella is increasing over time. Zeng et al. (2019) reported that 92 Salmonella strains isolated from 672 samples in different provinces of China showed resistance to one antimicrobial agent at least. Reportedly, the resistance of 124,347 Salmonella isolates that were reported from 1990 to 2018 worsened for all antimicrobials in all regions (Browne et al., 2020). According to the National Animal Health Monitoring System, 20% of Salmonella from swine are multidrug-resistant (resistant to ≥3 antimicrobial classes) (Bearson, 2022). Facing the present serious resistance situation, the World Health Organization (WHO) included Salmonella on the priority list for the development of new antimicrobials in 2017 (WHO, 2017). On the contrary, increasing studies indicated that the non-inherited resistance (e.g., persister cells, biofilms) of Salmonella plays a critical role in the resistant evolution and antibiotic failure. For instance, antibiotics were evidenced to show poor activity against slowly replicating Salmonella in mice (Claudi et al., 2014). In addition, Salmonella persister cells that were reported could enhance the spread of resistance plasmids in the host gut (Bakkeren et al., 2019).
However, the development of new antibiotics has entered a bottleneck period. To solve the challenge of increasing resistance in Salmonella, the use of old drugs to develop new treatment strategies is more feasible. Meanwhile, history has been shown that antibiotic resistance always occurs, which reminded that more attention has to be paid on understanding the resistant evolution processes of Salmonella. Therefore, this review starts from the combatting methods of Salmonella against antimicrobial agents, including asymmetrical outer membrane, efflux pump, and the non-inherited resistance according to the newest studies, thus to completely understand its escape mechanisms to antimicrobial agents. On this basis, the novel treatment strategies show potential in countering Salmonella resistance, such as enhancing the permeation of outer membrane (OM) by interfering MlaABC system (the phospholipid transport system maintains the asymmetry of OM), reducing persister cells by inhibition the hydrogen sulfide (H2S) producing protein, and using predatory bacteria as the biology disinfection, which are summarized. We emphasize that this review will provide new perspectives and ideas for the prevention and control of the current severe health concerns caused by Salmonella.
2. Resistance mechanisms of Salmonella
2.1. Intrinsic resistance
2.1.1. Asymmetrical outer membrane
As a gram-negative bacterium, Salmonella possesses a thick cell wall that consists of an OM, an inner membrane (IM), and a thin peptidoglycan layer, thus forming the periplasmic space (Figure 1; Pang et al., 2019). The asymmetrical outer membrane is believed to be involved in combatting antimicrobial agents. For instance, Wu et al. (2015) augured that the presence of the hydrophilic carbohydrate components of lipopolysaccharide (LPS) will form hydrated spheres, thereby limiting the movement and permeability of hydrophobic molecules on Salmonella cell membrane. At the same time, because the molecular structure of lipid A and the content of unsaturated fatty acids are lower than those of normal phospholipid bilayers, the effective packaging of lipids reduces the fluidity of the OM (Piggot et al., 2011; Khalid et al., 2015), thus limiting the permeation of hydrophobic antibiotic agents through the OM. Vancomycin, the glycopeptide class, is a classic example that is limited by OM. Vancomycin is an inhibitor of the peptidoglycan cross-linking, which is effective in gram-positive bacteria rather than in gram-negative bacteria due to the lack of permeation through the OM (Tsuchido and Takano, 1988).
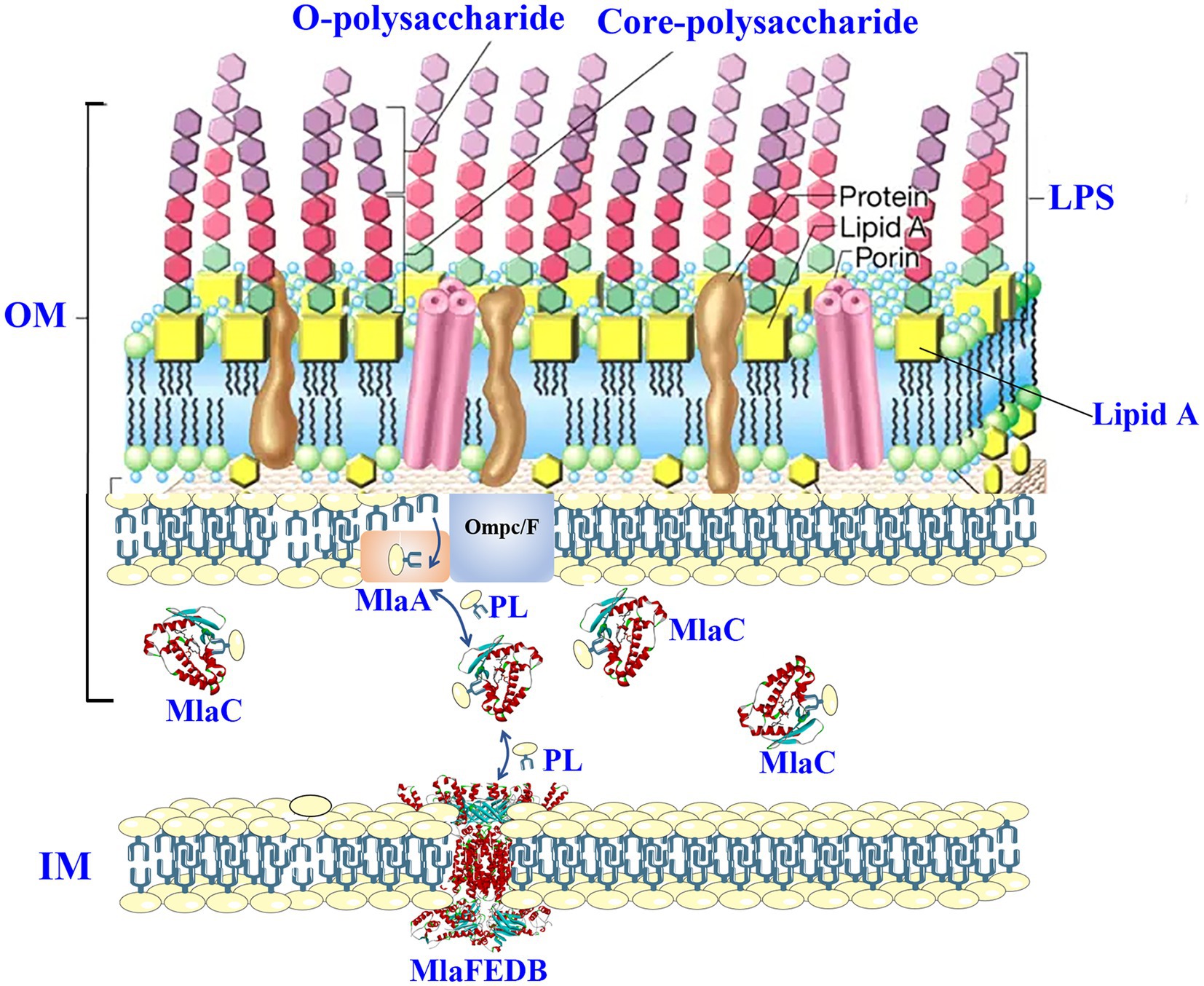
Figure 1. MlaABC transport system maintains the OM asymmetrical of gram-negative bacteria. LPS, lipopolysaccharide; PL, phospholipid; MlaC, PDB ID 7VR6; MlaFEDB, PDB ID 6Z5U.
Increasing studies proved that the asymmetrical OM is maintained by the MlaABC system, which removes and traffics phospholipids found in the outer leaflet of the OM to the IM (Figure 1; Ekiert et al., 2017; Hughes et al., 2019). These reminded that inhibitors aiming at MlaABC system of Salmonella are likely to be the potential antibiotic enhancers. Meanwhile, the agents targeting the components of OM, such as targeting phosphatidylglycerol or LPS, could disturb the asymmetrical structure, thus showing potency in improving antibacterial effects of existing antibiotics against multiple resistance Salmonella (Song et al., 2020).
2.1.2. Efflux pumps
To date, reports have shown that Salmonella expresses nine kinds of efflux pumps, namely AcrAB, AcrEF, AcrD, MdsABC, MdtABC, EmrAB, MdfA, MacAB, and MdtK (Table 1; Yamagishi et al., 2011; Sun et al., 2014; Prajapati et al., 2021). Among them, AcrAB is known to be responsible for fluoroquinolone resistance (Giraud et al., 2000; Shen et al., 2017). For instance, in our previous studies, we induced the Salmonella mutation SI3 with a high concentration of ciprofloxacin. Further studies indicated that ramA played a key role in increasing the overexpression level of the AcrAB efflux pump (Sun et al., 2011). In addition, AcrAB functions in β-lactam resistance (Giraud et al., 2000). EmrAB and AcrEF contribute to glycylcycline, triclosan, and tigecycline resistance (Rensch et al., 2014). MacAB is responsible for the efflux of macrolides and plays an important role in antioxidant stress (Yamagishi et al., 2011; Bogomolnaya et al., 2013). Song et al. (2014) reported that the overexpression of the MdsABC pump in Salmonella indicates neomycin, crystal violet, rhodamine, and gold resistance. Metal resistance is mediated by AcrD and MdtABC (Nishino et al., 2007). In addition to expelling antimicrobial compounds out of bacterial cells, efflux pumps also contribute to biofilm formation and quorum sensing (Yang et al., 2006). Baugh et al. (2012) suggested that the presence of the efflux genes acrD, emrAB, acrEF, acrB, macAB, mdsABC, mdfA, mdtK, mdtABC, and tolC resulted in the induction of biofilm formation compared with the wild-type strain.
2.1.3. Antibiotic-inactivating enzymes
The antibiotic-inactivating enzymes produced by Salmonella can decompose or modify antibiotics. For instance, Salmonella resistance to β-lactam antibiotics is commonly the result of the production of β-lactamases that are encoded by blaTEM genes. β-lactamases can break the amide bond of the β-lactam ring, thus leading to inactivation of β-lactam antibiotics (Wright, 2005). Because cephalosporins are commonly used to treat Salmonella infections in the clinic, β-lactamase plays a key role in the resistance of Salmonella. For instance, the β-lactamases produced by Salmonella strains show resistance to ceftriaxone, ceftiofur, cefoxitin, ampicillin, and amoxicillin/clavulanic acid (Coculescu et al., 2014). Wu et al. (2015) isolated 60 Salmonella strains that produced β-lactamases from 699 foodborne samples, in which the β-lactamase-encoding gene bla (TEM-1) was observed most (n = 44), followed by bla (OXA-1) (n = 38).
Aminoglycosides contain an aminocyclitol parent ring that links to amino sugars by glycosidic bonds. Aminoglycoside resistance is commonly associated with three types of aminoglycoside-modifying enzymes, including acetyltransferases (AACs), adenyltransferases (ANTs), and phosphotransferases (APHs) (Mąka and Popowska, 2016). Reportedly, in 10 Salmonella isolates with aminoglycosides resistance, the aminoglycoside-modified enzyme ANT (2″) was found (Leegaard et al., 1996).
2.2. Acquired resistance
2.2.1. Acquired resistance by mutations
Acquired resistance by mutations refers to Salmonella producing antibiotic resistance genes, which can be caused by base mismatch during replication. Base mismatch has a certain probability, though very small it occurs. Mutation in resistance genes may induce the overexpression of proteins (e.g., efflux pumps and antibiotic-inactivating enzymes), thus reducing antibiotic uptake or causing modifications to antibiotic targets (Uddin and Ahn, 2018; Pang et al., 2019).
In Salmonella, quinolone resistance was partly attributed to a point mutation in the gyrA gene encoding the gyrase subunit. The complex of gyrase and DNA is the main target of quinolones. Resistance mutations of the gyrA cluster in a region of the gene product between amino acids 67 and 106, called the quinolone resistance-determining region (QRDR). Hopkins et al. (2005) reported that amino acid changes at Ser-83 (change to Phe, Tyr, or Ala) or at Asp-87 (change to Gly, Asn, or Tyr) could be frequently observed in nalidixic acid-resistant Salmonella strains. In addition, an in vitro dynamic model indicated that the resistance of Salmonella against difloxacin, enrofloxacin, and marbofloxacin was targeted by a mutation in gyrA (S83F) (Lee et al., 2017).
Unlike the export action of efflux pumps, Salmonella protein channels (e.g., OmpC/F) located in the OM form an entry route for many antibiotics into cytosol. It was evidenced that OmpC and OmpF of Salmonella were responsible to the translocating of carbapenems, chloramphenicol, and cephalosporins, respectively (Medeiros et al., 1987; Armand-Lefèvre et al., 2003). That is why the changes in OmpC and OmpF, such as downregulation, mutations in the interior region, or expression of alternative porins, will develop resistance. For instance, loss of OmpC porin in Salmonella typhimurium causes increased resistance to cephalosporins (Medeiros et al., 1987).
2.2.2. Acquiring resistance genes
In addition to gene mutation, antibiotic resistance genes can be obtained from the external environment. Antibiotic resistance genes can be carried on plasmids, transposons, integrons, and prophages, and bacteria can acquire these genes via horizontal gene transfer from bacterial species and phages (Pang et al., 2019). Reportedly, Alcaine et al. (2005) proved that the isolated ceftiofur-resistant Salmonella strains in dairy farms evolved from independent horizontal gene transfer. Moreover, after 48-h incubation with chicken cecum in vitro, Salmonella Heidelberg was observed to acquire an IncK2 plasmid that carried an extended-spectrum-β-lactamase gene (bla CMY-2) (Oladeinde et al., 2019), demonstrating the possibility of resistant gene transfer in vivo.
2.3. Non-inherited resistance
Commonly, the susceptibility of bacteria to antimicrobial agents is quantified by MIC and MBC. Once the MIC is above the breakpoint, the strains are viewed as resistance. However, MIC and MBC aim at planktonic cells with normal growth rate. Unlike resistance (inherited, permanent), bacterial tolerance to antimicrobial agents is temporary and without mutations of resistant genes, which is commonly called non-inherited resistance or adaptive resistance (Fisher et al., 2017). The commonly discussed non-inherited resistance subpopulations of Salmonella are tolerant cells, persister cells, and biofilms. Among them, the form of tolerant cells and biofilms is commonly related to external stresses whose recognition may be mediated two-component systems (Murret-Labarthe et al., 2020). Given the similarity of above three phenotypes with inherited resistance, to help understanding the roles in combatting antimicrobial agents, their characteristics are concluded in Table 2 (Kim and Wood, 2017).
Owing to the slow growth rate, reduced metabolism level, low target activity, or low drug uptake of the non-inherited resistance subpopulations, the actual lethal or inhibitory effects of antimicrobial agents against them are significantly lower than that against their normal phenotype. Salmonella non-inherited resistance is recognized that can cause antibiotic treatment failure, more frequent doses of antibiotics, and longer treatment duration and have been shown to lead to the higher resistant mutation frequency (Hall and Mah, 2017; Levin-Reisman et al., 2017). Here, we discuss recent progresses in understanding the producing mechanisms of Salmonella non-inherited resistance and provide the potential therapeutic strategies.
2.3.1. Formation of biofilm
The ability of Salmonella to form biofilms in vivo was visualized by Desai et al. (2019). (Figure 2), which proved that Salmonella persistence and its asymptomatic carrier are closely related to its biofilms (Sandala et al., 2020). Reportedly, 2–5% of typhoid patients develop persistence and become asymptomatic carriers because of biofilm formation (Gunn et al., 2014). Salmonella biofilms are aggregates of Salmonella and its secretions, including extracellular DNA, exopolysaccharides, proteins, and metabolites, which are collectively referred to as extracellular polymeric substances (EPSs) (Das et al., 2013). After Salmonella attaches to the host cell surface, proliferation begins, and bacterial cells adhere to the surface irreversibly to form microcolonies. When enough individuals exist, Salmonella grows and matures from microcolonies into clusters of multilayered cells and begins to synthesize EPSs creating a biofilm. Meanwhile, some of cells within the biofilm will disperse in a planktonic state to continue to invade other host cells (Figure 3; Soto, 2013).
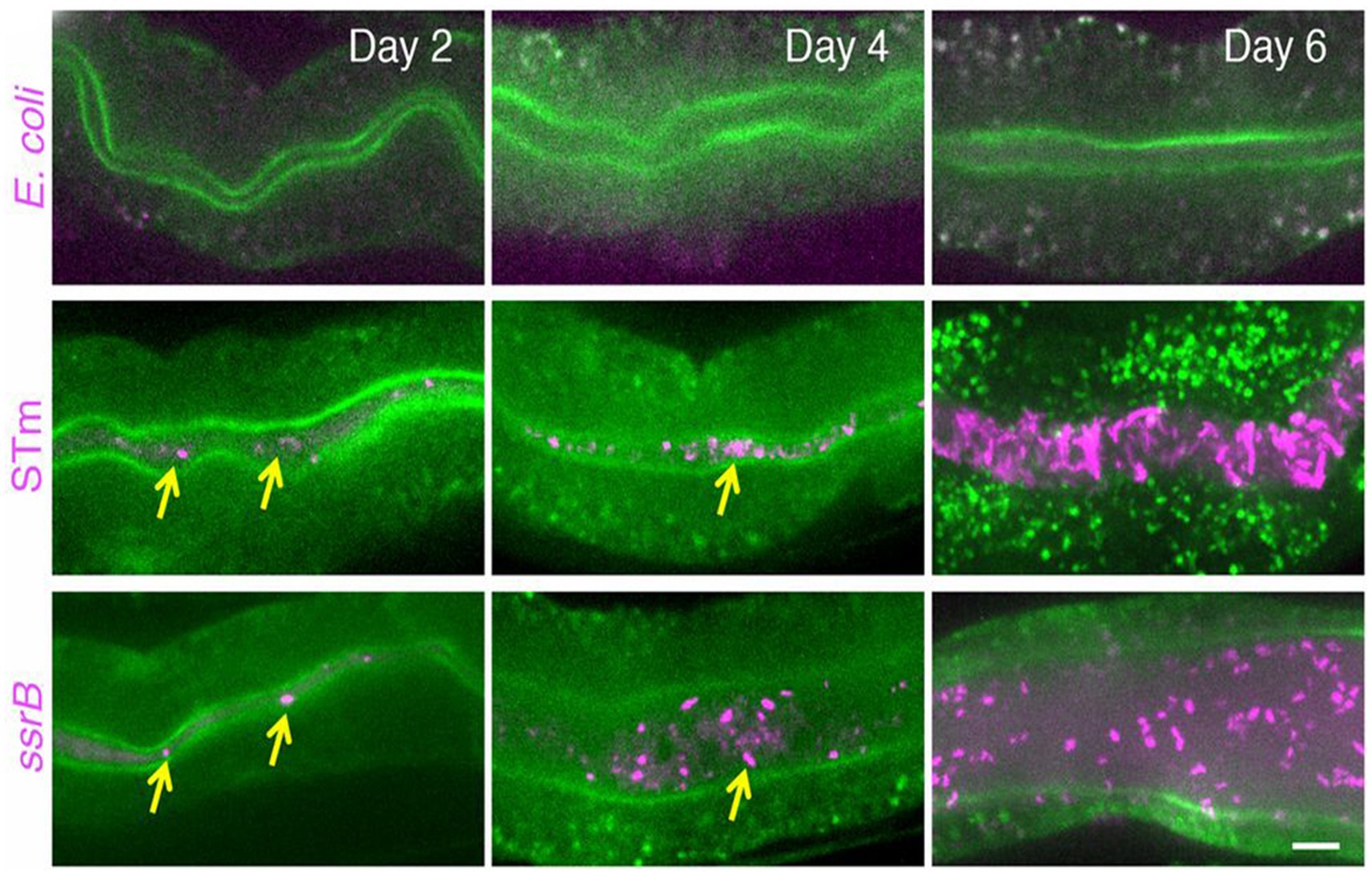
Figure 2. Salmonella forms biofilms within intestinal of Caenorhabditis elegans. Compared with E. coli, Salmonella tends to form biofilms in vivo. Reprinted with permission from Desai et al. (2019).
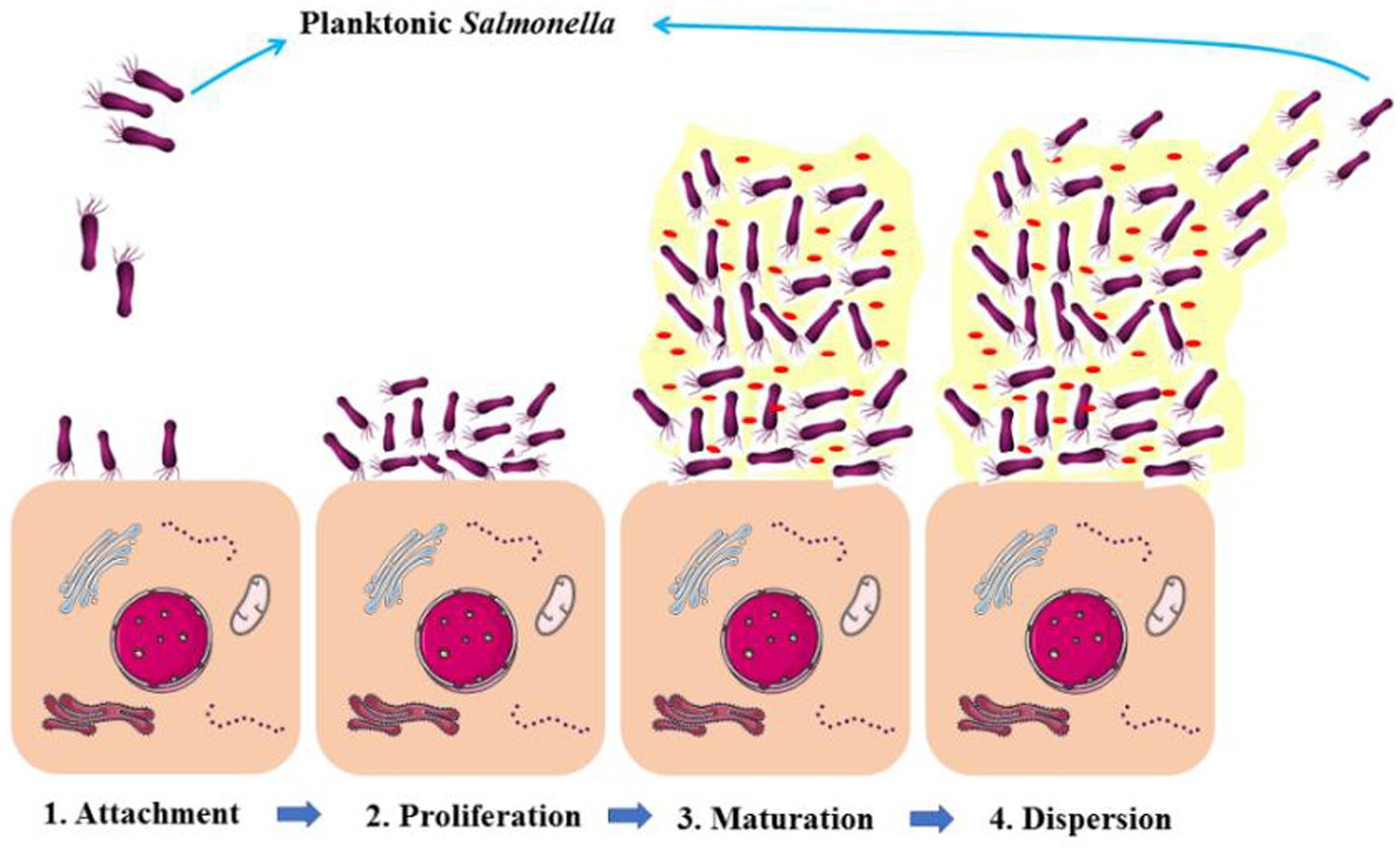
Figure 3. The schematic diagram stages of Salmonella biofilm formation. The forming of the biofilm contains four steps: (1) Salmonella attaches with the host cells; (2) proliferation; (3) biofilm maturation; (4) biofilm formed Salmonella disperse to planktonic Salmonella.
Due to the low permeability of antibiotics to biofilm matrix, the slow growth rate of the biofilms, the overexpression of efflux pumps, and the sheltering effects to persister cells (Lewis, 2010), Salmonella biofilms help the defense against antibiotics. Reportedly, González et al. (2018) found that Salmonella biofilms showed significantly higher MIC to ciprofloxacin both in vitro and in vivo. Moreover, 27 isolated S. typhi that formed biofilms displayed multidrug resistance (MDR) to doxycycline, sulfamethoxazole–trimethoprim, ciprofloxacin, ampicillin, and streptomycin (Shi et al., 2018). In addition, Salmonella can form biofilms on the surface of foods and food packaging, thus displaying resistance against disinfectants. For instance, peroxyacetic acid and acidified hypochlorite were not effective against biofilm-formed Salmonella compared with planktonic isolates (Chylkova et al., 2017). As mentioned above, food chain is an important transmission route of Salmonella, suggesting that an efficient tool for controlling Salmonella biofilms in poultry processing environments is urgently needed.
On the contrary, because of the nutrient limitation and oxygen gradients, the DNA oxidative damage of biofilms was in a high level (Hall and Mah, 2017). Therefore, Salmonella cells within biofilm show higher mutation frequency than that of planktonic cells (Hall and Mah, 2017). More importantly, unlike Pseudomonas aeruginosa and Staphylococcus aureus, Salmonella biofilm played a key role in ensuring activation of innate immunity rather than immunity escape. Desai et al.’s (2019) finding indicated that SsrB of Salmonella activated p38-MAPK innate immunity of Caenorhabditis elegans, allowing appropriate functioning of host innate immunity to eliminate the planktonic subpopulations, thus to confer itself an adaptive lifestyle advantage in persistent infections (e.g., asymptomatic carrying) because asymptomatic carriers are viewed as healthy population easily, which creates conditions for the wide spread of salmonellosis. Obviously, Salmonella biofilm status is particularly concerning in its persistent infections and pandemic (Desai et al., 2019). Therefore, the methods that can prevent the formation of Salmonella biofilms, in food chains and the clinical treatment, are worth further investigation.
2.3.2. Persister cells
Another major tolerant strategy of Salmonella is the formation of persister cells, whose presence can be reflected by the killing curves. For instance, the more reduction in viability would not be recorded any more even under higher ampicillin concentrations or longer inhibition time (Figure 4; Lewis, 2010; Rishi et al., 2018). Persister cells not only are tolerant to high concentrations of antibiotics but can also undermine host immune defenses (Stapels et al., 2018). For instance, Salmonella persister cells reprogram macrophages by using its SPI 2 type 3 secretion system, in which the secreted effectors decreased proinflammatory innate immune responses and reduced anti-inflammatory macrophage polarization. Additionally, it was evidenced that Salmonella persister cells could help its acquired resistance in the gut (Bakkeren et al., 2019).
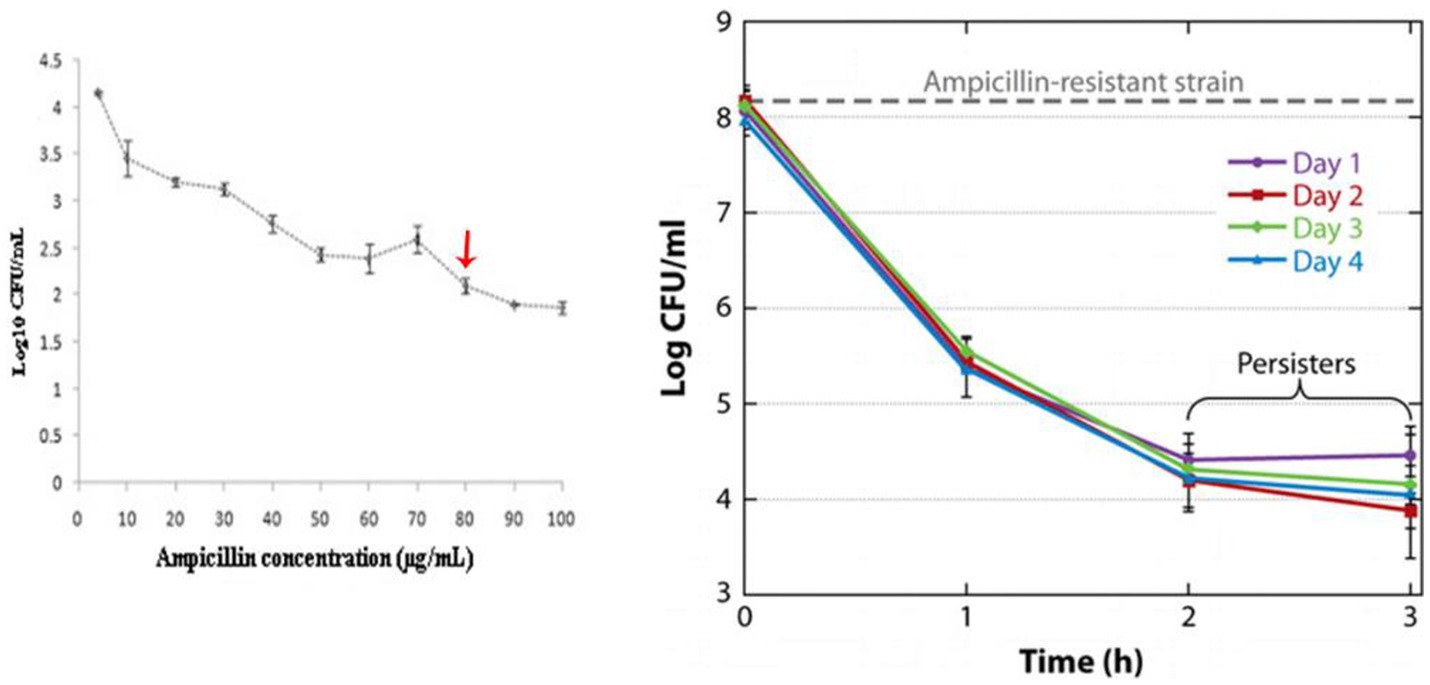
Figure 4. The tests for proving the presence of Salmonella persister cells. Reprinted with permission from Lewis (2010) and Rishi et al. (2018).
Many excellent research revealed the mechanisms of the emergence of Salmonella persister cells, which could be divided into inhibition acetylation of aminoacyl-tRNAs, reduced ATP level, and increased H2S level. For instance, Rycroft et al. (2018) proved that the inhibiting acetylation of aminoacyl-tRNAs was achieved by blocking the primary amino group of the amino acid on the charged tRNA molecule with acetyltransferase. Additionally, Lewis (2010) proposed the mechanisms of the produce of E. coli persister cells under fluoroquinolones. When fluoroquinolones damage bacterial DNA, activated RecA will activate LexA repressor in turns, in which the increased TixB effector leads to a drop in proton motive force and ATP levels, causing the decrease in metabolism level of bacteria, thus promoting the formation of persister cells (Figure 5A). In view of the inhibition mechanisms of fluoroquinolones (aiming at DNA), we argued that the formation processes of E. coli persister cells under fluoroquinolones also meet Salmonella, which reminds us of the potential of metabolism and ATP promoter in combating Salmonella persister cells. After all, treating Salmonella with the ATP synthase poison arsenate caused the increase in persister cells (Braetz et al., 2017).
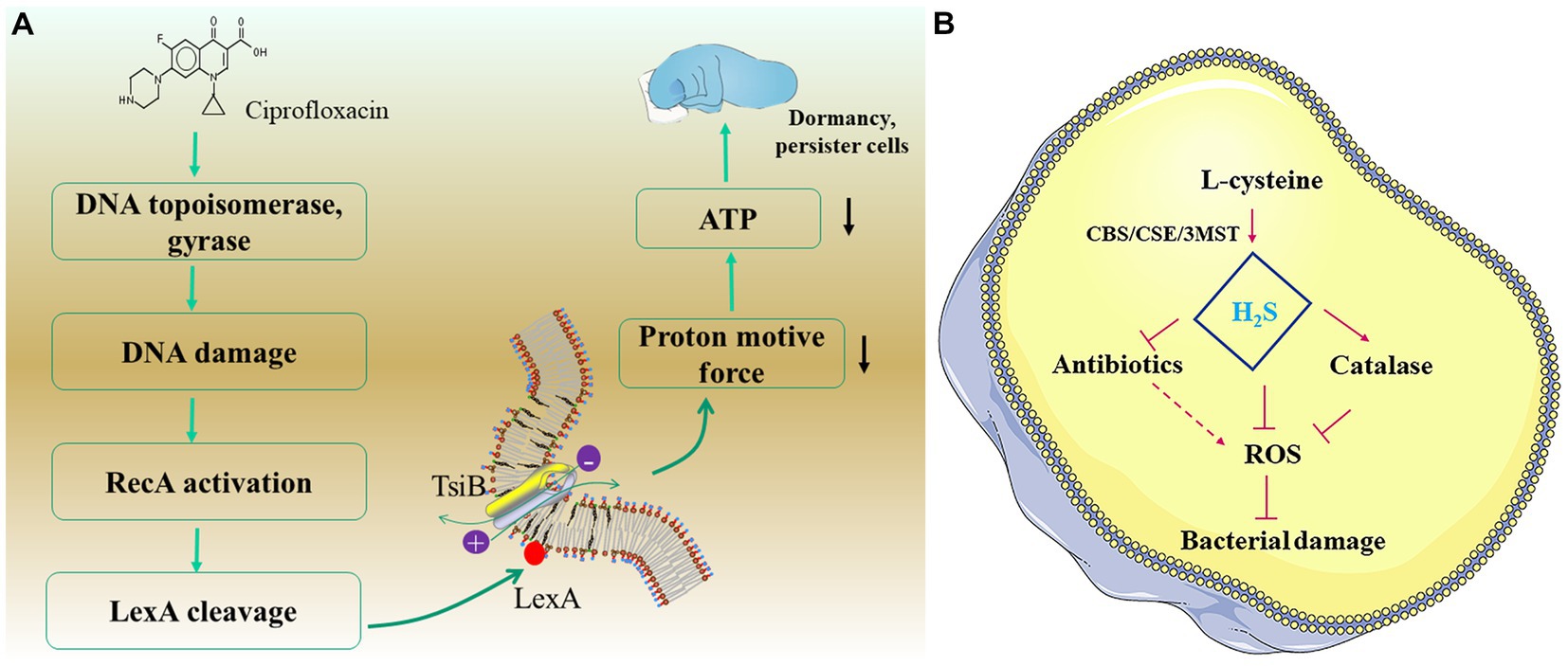
Figure 5. The two common strategies of the formation of persister cells. (A) The model of TisB protein-dependence. (B) The model of H2S-dependence.
Interestingly, recent studies suggested that the emergence of H2S may be a kind of positive defense of bacteria, which helps bacteria into a mild poisoning state. Luhachack and Nudler (2014) proved that significantly higher H2S content was detected within the persister cells of Pseudomonas aeruginosa and S. aureus than their planktonic. Recently, oxidative stress has been proposed as one of the mechanisms whereby bactericidal antibiotics (e.g., fluoroquinolones and aminoglycosides) kill bacteria (Drlica and Zhao, 2021). H2S, the reductant within bacterial cells, protects bacteria from reactive oxygen species (ROS), thus to disrupt different classes of bactericidal drugs, especially to the bactericidal antibiotics (Figure 5B).
An undoubted issue is that Salmonella persister cells can resuscitate after removal of antibiotics, thus causing refractory infections. Furthermore, even when Salmonella exited the persister cell status, they still showed tolerance to the antibiotic, suggesting the presence of a long-retention effect or “memory effect” of persister cells (Miyaue et al., 2018). Additionally, Salmonella biofilm provides a shelter to the contained persister cells for evading immune response (Balaban et al., 2004).
Overall, there is no doubt that the biofilms and persister cells of Salmonella play a critical role in combating antibiotics and resistant evolution. However, during susceptibility tests, dose regimen design and clinical medication, biofilms, tolerance, and persistence are not put on a high level. At least, yet to date, no tolerance-targeting therapeutics have been reported from the Food and Drug Administration and WHO. Following the recent advances in combating Salmonella persister cells and biofilms, better medication strategies are discussed.
3. Overcoming Salmonella resistance by compounds
3.1. Enhancing permeation to OM
As discussed above, due to the asymmetrical OM, in fact, only several antibiotics are permeable to the gram-negative pathogens. Although no specific studies focused the permeation of antibiotics against Salmonella, the studies on the validation of antibiotics accumulation in E. coli could be an indirect example that are helpful in enhancing penetration to Salmonella OM. As shown in Figure 6A, among different classes of antibiotics, only tetracycline, ciprofloxacin, and chloramphenicol showed ideal accumulation in E. coli. Once there was a lack of OmpR, the accumulation level of tetracycline, ciprofloxacin, and chloramphenicol was significantly reduced (Figure 6B). Meanwhile, combining with colistin, the accumulation level of antibiotics with low permeation was significantly increased (Figure 6C; Richter and Hergenrother, 2019).
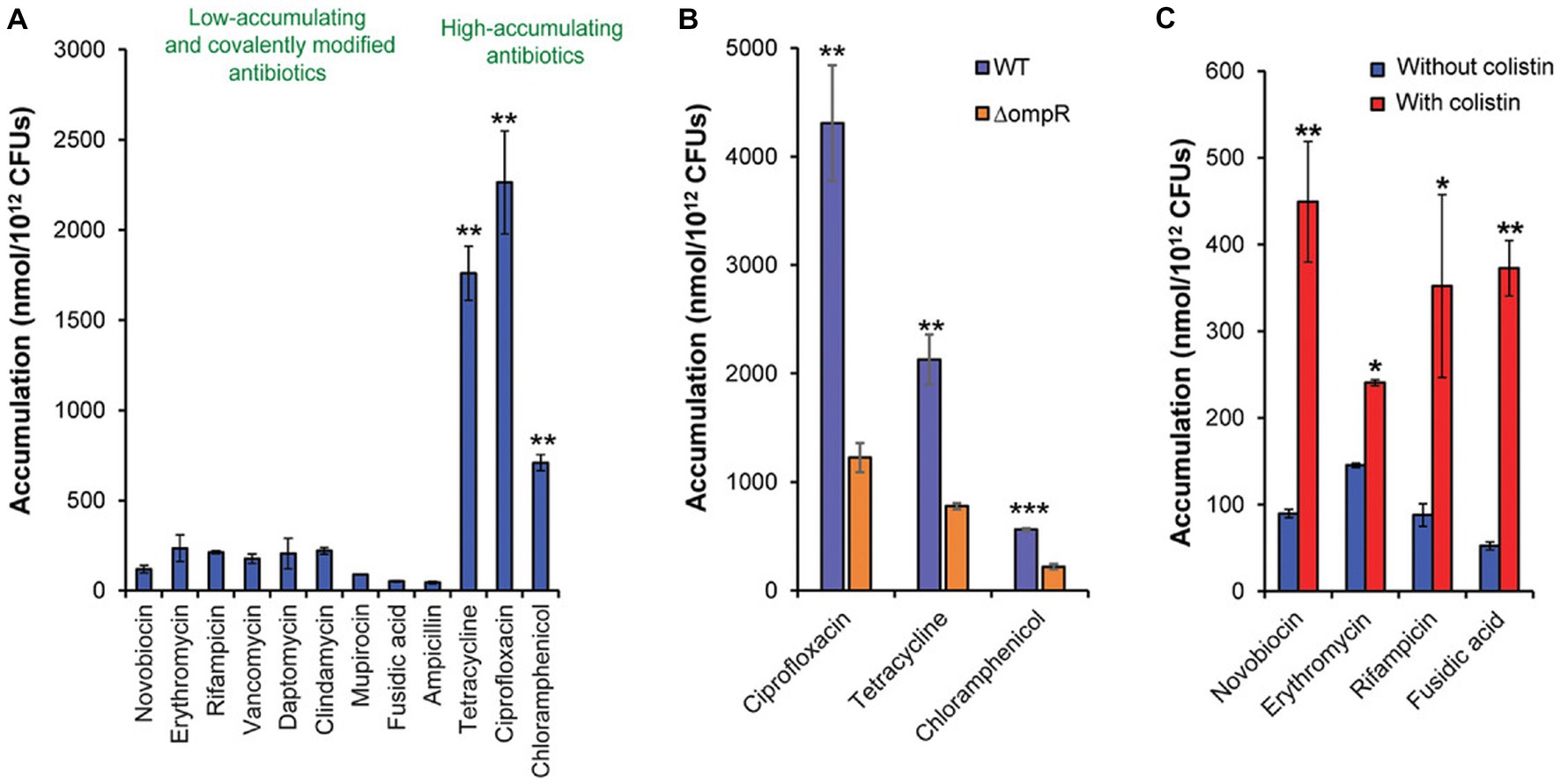
Figure 6. (A) The antibiotics with high accumulation within bacteria. (B) The roles of pore proteins in antibiotic permeation. (C) The enhancement efficiency of colisitin to permeation. Statistical significance was defined as *p < 0.05, **p < 0.01, and ***p < 0.001. (A) p values relative to the average of the low-accumulating controls; (B) p values relative to ΔompR in each group; (C) p values relative to without colistin in each group. Reprinted with permission from Richter and Hergenrother (2019).
The above results suggested that the two strategies could help the penetration of antibiotic against Salmonella OM: (a) possessing rational physicochemical properties and (b) combining with agents that aim at LPS. Many efforts have been paid in clarifying the correlation between accumulation in gram-negative pathogens and physicochemical properties of compounds. Physicochemical properties such as polarity hydrophobicity, molecular flexibility, and ring counts were recognized to be closely related to the cellular accumulation. Finally, the so called “eNTRy” rules were proposed. The small molecules possessed (a) ionizable nitrogen (primary > secondary > tertiary amines), (b) low three-dimensionality (globularity ≤0.25), and (c) relatively higher rigidity (rotatable bonds ≤5) which are most likely to accumulate in the bacterial cells (Prajapati et al., 2021). Additionally, as shown in Figure 6A, in view of the highest accumulation of ciprofloxacin (fluoroquinolones), we also guessed that -COOH might help in the penetration. The applying of these rules, such as in computer aid drug design, may promote the discovery of new compounds with high permeability to Salmonella.
In addition to design compounds rationally, the screening of MlaABC inhibitor agents is another potential strategy to increase the permeability of Salmonella OM. After all, bacterial strains with mutation of MlaABC system were evidenced to be more susceptibility. For instance, E. coli strains lack of MlaE or MlaD showed more susceptibility to EDTA and higher cellular accumulation of ethidium bromide or chlorpromazine (Tang et al., 2021). Furthermore, when clorobiocin, an inhibitor of MlaC, was combined with the human antimicrobial peptide LL-37, the antibacterial effects against Acinetobacter baumannii of the LL-37 were significantly improved (Huang et al., 2019). AA139, a synthesized peptide, targeting phospholipid transportation of bacteria, was evidenced to reverse the resistance of Klebsiella pneumoniae, Pseudomonas aeruginosa, Acinetobacter baumannii, and E. coli, while AA139 showed low spontaneous and induced resistance in vitro (Elliott et al., 2020), suggesting the huge potential of targeting MlaABC system in overcoming the Salmonella resistance.
3.2. Inhibiting efflux pumps
As mentioned above, efflux pumps of Salmonella play a critical role in reducing the cellular accumulation of antimicrobial agents, thus leading to its survival under high drug concentration. Inhibiting the activity of efflux pumps is an ideal strategy to enhance the efficiency of antimicrobial agents against Salmonella. For instance, Bonnefoy et al. (2004) provided a new β-lactamase inhibitor (AVE1330A), and when ceftazidime/AVE1330A was at a ratio of 4:1, only 2 μg/mL ceftazidime was required for the inhibition of resistant Salmonella. In addition, as mentioned above, macrolides were expelled from Salmonella cells by the MacAB efflux pump (Table 1), thus leading to Salmonella resistance to macrolides. Yamagishi et al. (2011) proved that the MacAB-expressing Salmonella strains were not sensitive to clarithromycin, azithromycin, leucomycin, or josamycin. However, after treatment with OU33858 (5-[(5-chloro-2-hydroxyphenyl) methylene]-3-propyl-2-thioxo-1,3-diazolidin-4-one, no activity against Salmonella), the growth of the MacAB-expressing Salmonella strains was clearly inhibited by the above macrolides, suggesting that OU33858 was an ideal MacAB efflux pump inhibitor. Grimsey et al. (2020) indicated that chlorpromazine and amitriptyline, substrates and inhibitors of the AcrB efflux pump, could reduce the AcrB efflux pump activity of Salmonella. It was reported that reserpine could be used as an AcrB efflux pump inhibitor of Salmonella (Shaheen et al., 2019). In addition, extracts of Artemisia tournefortiana could also reduce the expression of the AcrB efflux pump of Salmonella by downregulating the acrB gene in S. enteritidis strains (Khosravani et al., 2020).
More importantly, recent reports suggest that the EPIs show potency in limiting the horizontal transfer of resistance. Nolivos et al. (2019) found that although the resistance genes carried by plasmid could be transferred from donor bacteria to the recipient strains, the resistance protein could not be produced in the recipient strains without AcrB pump. By contrast, the resistance protein was produced in the wild-type strain. Therefore, Nolivos et al. (2019) highlighted that when we adopt the antibiotic treatment, if the inhibition method to AcrAB-TolC efflux pump was adopted at the same time, the horizontal transfer of resistance will be an uncommon issue. It suggests that the EPIs may have a second indirect effect: They can block the transfer of resistance between bacteria during antibiotic treatment, thus to limit the emergence of new resistant strains (Povolo and Ackermann, 2019). As a result, the screening of potential EPIs has got increasing attentions. According to the structure of EPIs, Lamut et al. (2019) summarized general structural characteristics of potential EPIs for us, for instance, containing at least two hydrophobic ring systems, the ring system can be quinolone, quinolone, benzene, pyridine, pyranopyridine, pyrimidine, pyridopyrimidinone, or indole.
3.3. Combatting non-inherited resistance
As mentioned above, in addition to resistant subpopulation, the biofilm and persister cell subpopulations of Salmonella, the non-normal growth or metabolism subpopulation, play a key role in combatting antibiotics and promoting resistant evolution. Consequently, the strategies on combating biofilms and persister cells are worth to be concluded.
Reportedly, Carvacrol and thymol inhibited biofilm formation of Salmonella at sub-MICs (Čabarkapa et al., 2019). Interestingly, in addition to the inhibition effect on efflux pump, efflux pump inhibitors (EPIs) were proved to possess the ability in inhibition to biofilm forming. Baugh et al. (2014) found that once the special inhibitor of AcrAB-TolC efflux pump (e.g., chlorpromazine, carbonyl cyanide m-chlorophenylhydrazone, or phenyl-arginine-naphthylamide) was inoculated with Salmonella, the biofilm forming was inhibited, by which they proposed that inhibition of efflux pump can be viewed as a strategy to prevent biofilm formation. Furthermore, Wang-Kan et al. (2017) indicated that when the acrA, acrB, or tolC gene (genes of AcrAB-TolC efflux pump) of Salmonella was deleted, compared with the wild-type Salmonella, the biofilm forming of mutant lacking strains was significantly reduced. Further analysis indicated that in mutants lacking acrB and tolC, the production of flagella genes was decreased, thus leading to the reduction of biofilm forming. As a reminder, the EPIs also possessed the inhibition ability to the biofilm of Salmonella. However, currently, there are few EPIs which can be used for medication.
On the contrary, the effects of antimicrobial agents targeting bacterial cell membrane compositions are less interfered by bacterial metabolic level and growth rate (Bahar and Ren, 2013; Rishi et al., 2018). For instance, Song et al. (2020) provided a short linear antibacterial peptide SLAP-S25, whose amino acid sequence was listed as CH3CO-Dab-Ile-Dab-Ile-Dab-dPhe-Leu-Dab-dVaL-Leu-Ala-NH2, which can be used to enhance the antibacterial activity of common antibiotics against MDR-resistant Salmonella. Mechanistic studies suggested that SLAP-S25 caused membrane damage by binding to both LPS and phosphatidylglycerol in the OM, thus allowing more antibiotics to enter the bacterial cells. As shown in Figure 4, the retention ~102 CFU/mL bacteria are always observed in the antibiotic killing (inhibiting) curves, which is viewed as persister cells. The issue that we want to discuss is that the re-growth of the rest cells will cause reinfections. Compared with traditional killing curves, once the antibiotics were combined with SLAP-S25 (≤ 4 μg/mL), 100% inhibition rate could be achieved, suggesting that SLAP-S25 will be an efficient adjuvant to help most antibiotics to clear Salmonella persister cells.
The mechanism of persister cells show tolerance to high concentrations of antibiotics is commonly recognized as its low metabolism level (Brauner et al., 2016; Kim and Wood, 2017). Changing persister cells into the state of metabolically active and is recognized to be another strategy. For instance, Rishi et al. (2018) proved that mannitol displayed activity in increasing the metabolism level in the persister cells of Salmonella. When ampicillin was combined with mannitol, an approximately 78% elimination rate to the Salmonella persister cells was observed.
Notably, due to persister cells are few fraction of the total population, combining the adjuvants that aiming at persister cells with antibiotics not always can reduce the MIC value of antibiotics. For instance, Liu et al. (2021) found that indole-3-acetic acid (IAA) could not reduce the MIC of ciprofloxacin against MRSA in vivo, but the survival rate of the infected mice was significantly enhanced by the combining of IAA and ciprofloxacin. Further studies revealed that IAA enhanced the production of ATP and ROS within MRSA persister cells. As mentioned above, the decrease of ATP in bacteria is the classic characteristic of the emergence of persister cells under pressure of fluoroquinolones (Rishi et al., 2018), which reminds that IAA is an ideal adjuvant to deal with the recalcitrant infections of Salmonella caused by persister cells. What we want to highlight is that combining persister cell activators, the lower MIC will not be observed, but the better treatment efficacy in vivo can be achieved. As a reminder, if we always aspire to the reduction in MIC values, we will miss some valid metabolism activators for Salmonella persister cells.
On the other hand, to enter slight poisoning positively by producing low level H2S keep bacteria away from the damage of antibiotics. It reminded the potential of inhibitors aiming at H2S producing proteins. Shatalin et al. (2021) proposed a small molecule, NL1, which targeted CSE (Figure 7). As shown in Figures 7C,D, when NL1 was added, the counts of Pseudomonas aeruginosa and S. aureus persister cells were significantly reduced, and the survival rate of infection mice was improved by approximately 5-fold, which showed a brilliant prospect of the inhibitor agents targeting CSE in combatting persister cells of Salmonella.
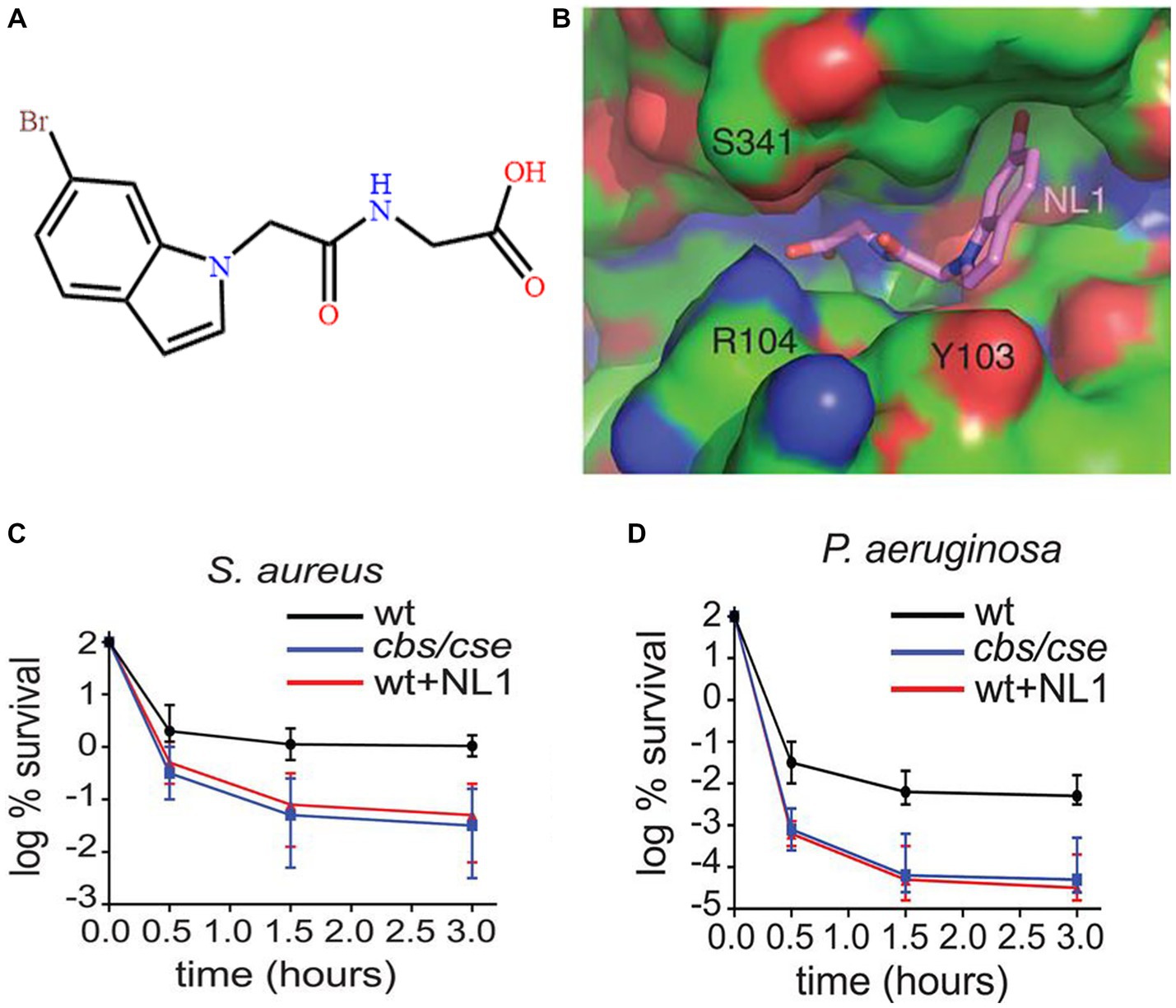
Figure 7. The effects of NL1 in reducing persister cells. (A) The construction of NL1. (B) View of NL1 (sticks) in the CSE binding pocket. (C) The effects of NL1 in reducing persister cells of S. aureus. (D) The effects of NL1 in reducing persister cells of Pseudomonas aeruginosa. Reprinted with permission from Shatalin et al. (2021).
4. Overcoming Salmonella resistance by bacteria
4.1. Probiotics
The most proposed common groups of probiotics are Lactobacilli and Bifidobacteria. In addition, E. coli, Saccharomyces cerevisiae var., and Bacillus coagulans have been commercialized as probiotic products (Gut et al., 2018). As well known, the successful colonization of Salmonella is the first step of infections. Without colonization, further proliferation, biofilm formation, and intracellular survival will not occur. The main mechanisms by which probiotics can prevent resistant Salmonella infections were included as follows: (a) competitively inhibiting Salmonella colonization on the surface of host cells; (b) producing inhibitory agents to interfere with biofilm formation; (c) antibacterial molecules released by probiotics can kill resistant Salmonella directly; and (d) inhibiting resistant Salmonella by enhancing systemic immunity (Figure 8; Gut et al., 2018). Among the above mechanisms, competitive colonization inhibition is the most common. Although most studies do not focused on the elimination of resistant Salmonella, no evidence has shown that the colonization processes between resistant and sensitive Salmonella are different. The progresses of probiotics against Salmonella are concluded in Table 3.
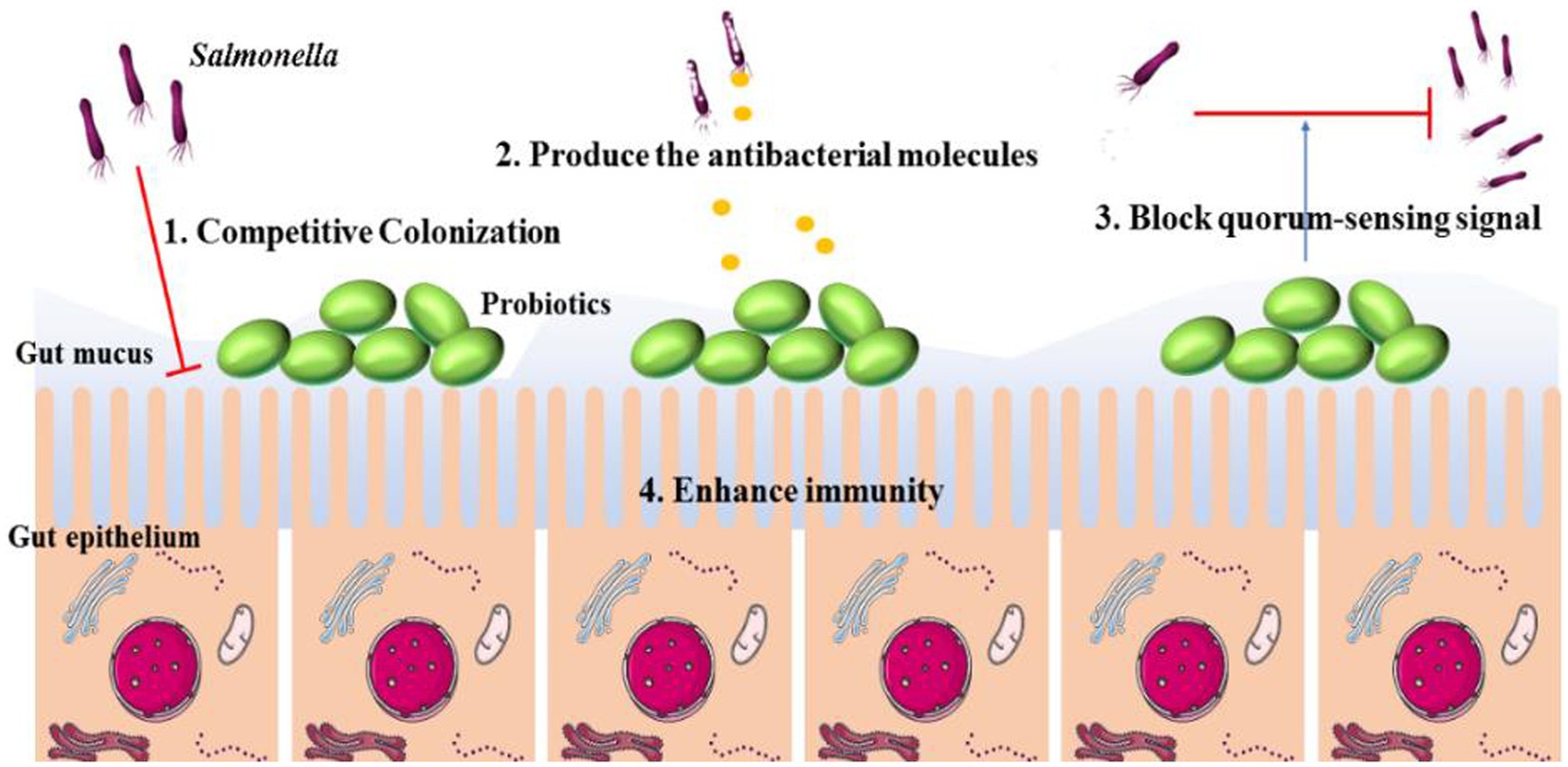
Figure 8. The mechanisms of intestine probiotic population prevent the colonization and invasion of Salmonella. The main mechanisms include: (1) competitive colonization; (2) produce the antibacterial molecules; (3) block quorum-sensing signal; (4) enhance host immunity.
Previously, competitive colonization was thought to occur when the Salmonella colonization sites in the intestinal epithelium were occupied by probiotics. For instance, Vuotto et al. (2014) indicated that the attachment site of Salmonella on CaCo-2 cells was displaced by Bifidobacterium longum Bar 33. Recently, some studies reported that the adhesion of Salmonella on the surface of probiotics was one of the mechanisms to reduce Salmonella colonization. The close contact of S. typhi and Saccharomyces boulardii (S. boulardii) was confirmed by transmission electron microscopy (Figure 9; Martins et al., 2010). S. typhi colonized the gastrointestinal tract (GIT) of mice, but when the infected mice were administered S. boulardii, the bacterial cells clustered around the yeast cells, suggesting the adhesion action of S. typhi to S. boulardii (Pontier-Bres et al., 2014). Importantly, S. boulardii does not bind to the GIT, and after transitory retention in the GIT, the bound Salmonella will be excreted in the feces (Czerucka et al., 2007). It is speculated that the mechanism of binding is due to the presence of mannose-specific adhesion/receptors (fimbriae) on the cell wall of Salmonella, which can bind to mannose on the cell wall of yeast (Gut et al., 2018). Therefore, when yeast is used to prevent or control Salmonella infections, the intake of food- or beverage-rich sugars should be reduced.
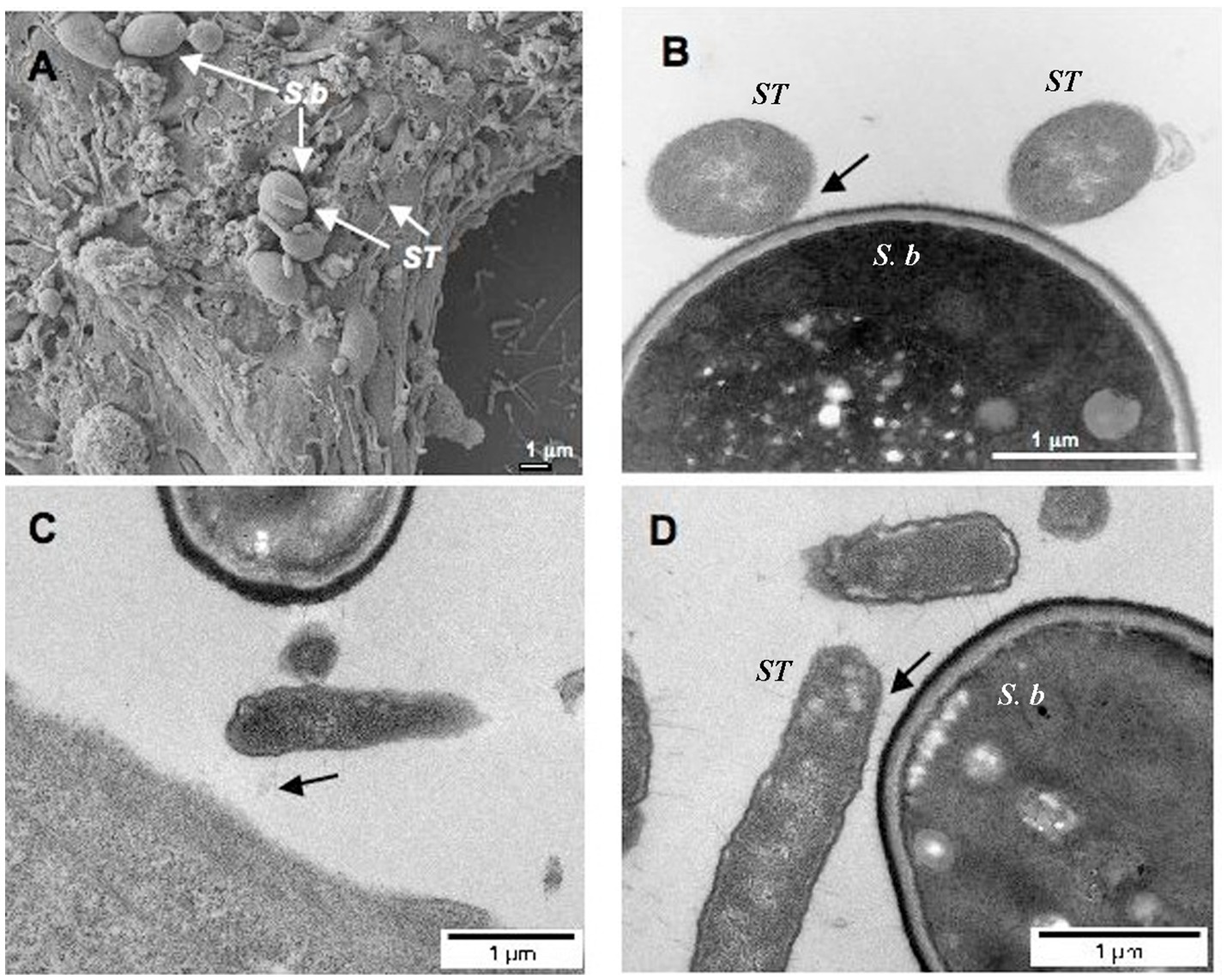
Figure 9. The imagines of S. Typhimurium (ST) binding to S. boulardii (Sb) cell wall. (A) Scanning electron micrograph of T84 cells infected by ST in the presence of Sb. (B) Transmission electron microscopy. (C, D) Transmission electron micrograph after red ruthenium staining. N=3. Black arrows show the binding of bacteria to the yeast. Reprinted with permission from Martins et al. (2010), licensed under CC-BY 4.0.
Because of the natural antibiotic resistance and immunity escape, Salmonella biofilms are difficult to be cleared. It was reported that several enhancement mechanisms are associated with the inhibition of probiotics against biofilm formation. One of the mechanisms is that probiotics can produce enzymes to interfering Salmonella biofilm. Alpha-amylase, an enzyme produced by yeast cells, was reported to prevent biofilm formation of Salmonella (Sadekuzzaman et al., 2018). Moreover, the biomolecules produced by probiotics could destroy the construction of the bacterial biofilm. For instance, the exopolysaccharide produced by Lactobacillus spp. showed inhibitory activity against biofilms of Salmonella (Xu et al., 2020). Additionally, lectin-like protein (Llp) 1 and Llp2 produced by Lactobacillus rhamnosus GG showed pore-like action on Salmonella biofilms. More interestingly, neither Llp1 nor Llp2 could prevent biofilm formation of other pathogens, suggesting that the Llp1 and Llp2 lectins may have the Salmonella-specific activity (Petrova et al., 2016).
Although the probiotic method, which treats Salmonella with bacteria, showed exciting in combating resistant Salmonella, there are few commercial probiotic products that are used for the treatment of Salmonella infections (Ghosh et al., 2019). One of the reasons is the lower treatment efficacy. For instance, the results of probiotic feeding indicated that probiotics had the potential to enhance the luminal microbiota; however, the amount of S. typhi found in the feces was not significantly reduced (Khan and Chousalkar, 2019). On the contrary, it is not easy to screen an activity probiotic against Salmonella. To simplify the screening processes of efficient probiotics, fecal transplant treatment (FTT) technology that transplants the microbiome from a healthy donor into a diseased gut is an alternative strategy. Because efficient probiotics are contained in the feces of healthy donors, FTT therapy has shown promise in clearing colonization with multidrug-resistant Enterobacteriaceae such as E. coli, Salmonella, and methicillin-resistant S. aureus (Piewngam et al., 2018), especially for the treatment of Clostridium difficile infections. However, few studies of FTT against Salmonella infections in humans and in veterinary clinics are reported. We argue that the FTT method is a potential breaker to combat resistant Salmonella. At least in the veterinary clinic, the feces of healthy animals can be mixed with feed to control animals’ Salmonella infections.
4.2. Predatory bacteria
Predatory bacteria are commonly called “killer of gram-negative bacteria.” Being different from phages, they multiply only after entering gram-negative pathogens (e.g., E. coli, Salmonella) (Kadouri et al., 2013). Common predatory bacteria are Bdellovibrio and Bdellovibrio-like organisms, such as Bdellovibrio bacteriovorus and Micavibrio aeruginosavorus (Negus et al., 2017). The invasion processes and life cycle of Bdellovibrio bacteriovorus in gram-negative are displayed in Figure 10 (Sockett and Lambert, 2004; Cavallo et al., 2021). During predation, predatory bacteria seek for prey by flagellum propelling. Once preys are caught, the location between predatory bacteria with prey occurs. During attachment, predatory bacteria swim at high speeds (~160 μm/s) to penetrate a prey cell. Then, predatory bacteria establish themselves within the prey cell periplasm by remodeling the wall and attaching to the cytoplasmic membrane of the prey cells. After that, predatory bacteria produce several progeny cells by using the nutrients within prey cells. Finally, when nutrients within prey cell are exhausted, the division cells format flagellum to exit old prey cells and start to seek for further prey.
Due to its specificity and efficiency, prey ability to gram-negative, predatory bacteria is called “alive antibiotics” (Cavallo et al., 2021). The studies on using predatory bacteria to combat Salmonella are excited. Atterbury et al. (2011) proved that after oral administration of Bdellovibrio, the amount of S. enteritidis in the chicken gut was significantly reduced. As mentioned above, during invasion, predatory bacteria can form a local pore on the cell wall of bacterial pathogens, suggesting that the enhancement of antibiotic intracellular delivery could be achieved by combining predatory bacteria with antibiotics. Shatzkes et al. (2017) proved that the antibacterial activity of predatory bacteria against resistant E. coli was the same as that against sensitive E. coli, which also demonstrated the antibacterial potential of predatory bacteria against resistant Salmonella.
As mentioned above, the cell wall damaging agents are one of the potential measures to combat the Salmonella-tolerant cells. Being different from most antibiotics, the predation ability (bactericidal capacity) of predatory bacteria is not limited by the growth state of Salmonella, that is, when predatory bacteria are applied to treat salmonellosis, the few tolerant cell formation can be expected. For instance, the enzymes produced by predatory bacteria can help the penetration to bacterial biofilms (Wucher et al., 2021). Importantly, this reminds the advantage of predatory bacteria in eliminating Salmonella biofilms attach on the surface of food (Sun et al., 2017).
As one of the foodborne pathogens, animal-derived food (e.g., meat, milk, and egg) is a common route of Salmonella transmission. Meanwhile, due to the presence of co-resistance between disinfectants with antibiotics, the use of disinfectants in environment promotes the development of Salmonella resistance. The application potential of predatory bacteria being used as a biology disinfection agent is obtaining more and more attention. Waso et al. (2021) proposed that compared with using chemistry disinfectants and antiseptics, the predatory bacteria method shows more superiority in limiting the emergence of co-resistance against disinfectants and Salmonella and reducing biofilms, suggesting the application potential of predatory bacteria in food packaging and environmental disinfection.
As mentioned above, one of the important resistance mechanisms of Salmonella is that it can obtain resistance genes by horizontal spread, which is called acquired resistance. Fortunately, because of its kinds of enzymes (e.g., nuclease), predatory bacteria showed enormous potential in the degradation of antibiotics resistant genes (Bratanis et al., 2017; Bratanis and Lood, 2019). As a reminder, if predatory bacteria were used to treat Salmonella infections, not only could the resistance Salmonella be killed, but also the horizontal spread of resistant genes could be limited. As proposed by Sockett and Lambert (2004), due to the poor segregation during the co-encytic multichromosomal bdelloplast stage and highly motile as well as fast-swimming, one of the native characteristics of predatory bacteria is that they can reduce the opportunities for conjugative transfer of resistant genes from other bacteria species.
Reportedly, Bdellovibrio was isolated from mammalian feces, suggesting the probable presence of predatory bacteria in the mammalian intestine (Schwudke et al., 2001), that is, predatory bacteria are probable safe to mammalian. However, studies on predatory bacteria are very limited. Their biosafety remains a concern that needs further evaluation.
5. Concluding remarks and further prospects
Salmonella infections severely threaten human health, and increasing antibiotic resistance continuously leads to a worsening situation. The antibiotic resistance of Salmonella is multifactorial in that it can occur through intrinsic (OM limitation, efflux pumps and antibiotic-inactivating enzymes) and acquired (mutations and acquisition of resistance genes) mechanisms. Meanwhile, biofilms and persister cells of Salmonella play a critical role in antimicrobial agent escaping, resistant evolution, and pandemic. Due to the strong abilities of Salmonella in combating antimicrobial agents, traditional antibiotic therapy is difficult to control its spread and resistant development. To solve the challenges caused by Salmonella resistance, this review included some alternative therapeutic strategies that have the potential to combat the resistance of Salmonella.
However, most of strategies only are validated in vitro. Regardless of small compounds, polypeptide, probiotics, or predatory bacteria, only a suitable dose could have enough antibacterial effect on resistant Salmonella in vivo. Therefore, how to design a suitable dose is worth to be discussed. Only then can these potential resistance breakers be safely applied in the clinic. Because of the large experimental animal uses in conventional PK-PD methods during dose design, the more economical hollow fiber model has received increasing attention (Ferro et al., 2015), which has been qualified by the European Medicines Agency as a methodology for use in support of selection and development of anti-tuberculosis regimens (Cavaleri and Manolis, 2015) Reportedly, the acquired resistance degrees of MDR Mycobacterium tuberculosis under different doses of levofloxacin were evaluated by a hollow fiber model; thus, the optimized antibacterial and prevention mutation dose of levofloxacin was provided (Arber, 2014), suggesting that the treatment efficacy of antibiotics plus antibiotics and antibiotics plus adjuvants against Salmonella could also be studied by the hollow fiber infection model (Srivastava et al., 2017). Furthermore, even the efficient inoculation number of probiotics and predatory bacteria can also be designed via a hollow fiber infection model by monitoring the number of Salmonella versus time (D’ambrosio et al., 2022).
More important issues of probiotics and predatory bacteria are their retention in vivo and potential biological risks. After probiotics and predatory bacteria being used for treatment, how to elimination them from body is a widely concerned problem (Ghosh et al., 2019). Recent report indicated that chronological lifespan (CLS) of E. coli could be shorted 33% by deleting the methyltransferase gene (ubiG) (Figure 11A), while the regulation was evidenced to be a robust, strain-independent regulation (Guo et al., 2022). This suggests that the CLS of probiotics and predatory bacteria may also be controlled (Figure 11B). In this case, once the treatment time required for Salmonella infections is met, probiotics or predatory are automatic death in vivo. By this way, the potential biological risks and resistance spread risks of probiotics and predatory will not be an issue any more.
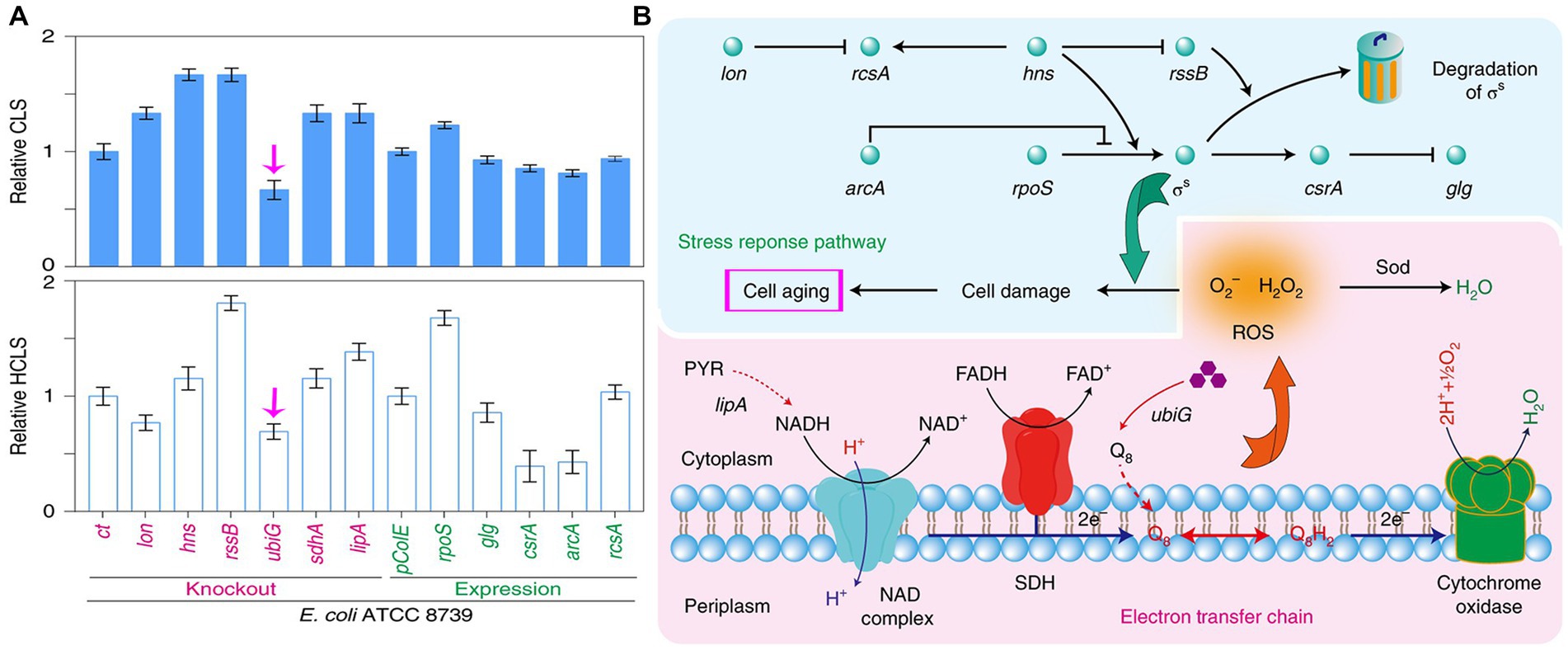
Figure 11. Regulating chronological lifespan (CLS) of bacteria by genetic manipulation. (A) Knockout ubiG gene of E. coli could short its CLS and half-chronological lifespan (HCLS). (B) The modulation of probiotics or predator bacteria lifespan may be achieved by regulating the electron transfer chain and stress response pathways. Reprinted with permission from Guo et al. (2022), authorized by Nat. Catal.
Finally, as proved by Liu et al. (2021), persister cells as a small part of population, combining antibiotics with persister cells inhibitors not always causes a lower MIC value, but better treatment efficacy in vivo can be achieved. We want to highlight is that the goal during antibiotic chemotherapy is the cure of infections, and the traditional view only focusing on the MIC decrease in vitro is the time to be changed. After all, the cure rates are not only related to the susceptibility of bacterial pathogens to antibiotics but also the presence of the non-inherited phenotype (Zappala, 2004; Shatalin et al., 2021).
Author contributions
KZ and LH: conceptualization. XZ, LS, XX, and WM: methodology. KM, LS, XZ, XX, and WM: investigation. KZ: writing—original draft. KZ and LH: writing—reviewing and editing. LH: funding acquisition and supervision. All authors have read and agreed to the published version of the manuscript.
Funding
This research was funded by the National key research and development program (2022YFD1800402) and the National Natural Science Foundation of China (32273063).
Conflict of interest
The authors declare that the research was conducted in the absence of any commercial or financial relationships that could be construed as a potential conflict of interest.
Publisher’s note
All claims expressed in this article are solely those of the authors and do not necessarily represent those of their affiliated organizations, or those of the publisher, the editors and the reviewers. Any product that may be evaluated in this article, or claim that may be made by its manufacturer, is not guaranteed or endorsed by the publisher.
References
Alcaine, S. D., Sukhnanand, S. S., Warnick, L. D., Su, W. L., McGann, P., McDonough, P., et al. (2005). Ceftiofur-resistant Salmonella strains isolated from dairy farms represent multiple widely distributed subtypes that evolved by independent horizontal gene transfer. Antimicrob. Agents Chemother. 49, 4061–4067. doi: 10.1128/AAC.49.10.4061-4067.2005
Arber, W. (2014). Horizontal gene transfer among bacteria and its role in biological evolution. Life 4, 217–224. doi: 10.3390/life4020217
Armand-Lefèvre, L., Leflon-Guibout, V., Bredin, J., Barguellil, F., Amor, A., Pagès, J. M., et al. (2003). Imipenem resistance in Salmonella enterica serovar wien related to porin loss and cmy-4 β-lactamase production. Antimicrob. Agents Chemother. 47, 1165–1168. doi: 10.1128/AAC.47.3.1165-1168.2003
Atterbury, R. J., Hobley, L., Till, R., Lambert, C., Capeness, M. J., Lerner, T. R., et al. (2011). Effects of orally administered Bdellovibrio bacteriovorus on the well-being and Salmonella colonization of young chicks. Appl. Environ. Microbiol. 77, 5794–5803. doi: 10.1128/AEM.00426-11
Bahar, A. A., and Ren, D. (2013). Antimicrobial peptides. Pharmaceuticals 6, 1543–1575. doi: 10.3390/ph6121543
Bakkeren, E., Huisman, J. S., Fattinger, S. A., Hausmann, A., Furter, M., Egli, A., et al. (2019). Salmonella persisters promote the spread of antibiotic resistance plasmids in the gut. Nature 573, 276–280. doi: 10.1038/s41586-019-1521-8
Balaban, N. Q., Merrin, J., Chait, R., Kowalik, L., and Leibler, S. (2004). Bacterial persistence as a phenotypic switch. Science 305, 1622–1625. doi: 10.1126/science.1099390
Baugh, S., Ekanayaka, A. S., Piddock, L. J., and Webber, M. A. (2012). Loss of or inhibition of all multidrug resistance efflux pumps of Salmonella enterica serovar Typhimurium results in impaired ability to form a biofilm. J. Antimicrob. Chemother. 67, 2409–2417. doi: 10.1093/jac/dks228
Baugh, S., Phillips, C. R., Ekanayaka, A. S., Piddock, L. J., and Webber, M. A. (2014). Inhibition of multidrug efflux as a strategy to prevent biofilm formation. J. Antimicrob. Chemoth. 69, 673–681. doi: 10.1093/jac/dkt420
Bearson, S. M. D. (2022). Salmonella in swine: prevalence, multidrug resistance, and vaccination strategies. Annu. Rev. Anim. Biosci. 10, 373–393. doi: 10.1146/annurev-animal-013120-043304
Bogomolnaya, L. M., Andrews, K. D., Talamantes, M., Maple, A., Ragoza, Y., Vazquez-Torres, A., et al. (2013). The ABC-type efflux pump MacAB protects Salmonella enterica serovar typhimurium from oxidative stress. MBio 4, e00630–e00613. doi: 10.1128/mBio.00630-13
Bonnefoy, A., Dupuis-Hamelin, C., Steier, V., Delachaume, C., Seys, C., Stachyra, T., et al. (2004). In vitro activity of AVE1330A an innovative broad-spectrum non-beta-lactam beta-lactamase inhibitor. J. Antimicrob. Chemother. 54, 410–417. doi: 10.1093/jac/dkh358
Braetz, S., Schwerk, P., Thompson, A., Tedin, K., and Fulde, M. (2017). The role of ATP pools in persister cell formation in (fluoro)quinolone-susceptible and -resistant strains of Salmonella enterica ser. Typhimurium. Vet. Microbiol. 210, 116–123. doi: 10.1016/j.vetmic.2017.09.007
Bratanis, E., and Lood, R. (2019). A novel broad-spectrum Elastase-like serine protease from the predatory bacterium Bdellovibrio bacteriovorus facilitates elucidation of site-specific IgA glycosylation pattern. Front. Microbiol. 10:971. doi: 10.3389/fmicb.2019.00971
Bratanis, E., Molina, H., Naegeli, A., Collin, M., and Lood, R. (2017). BspK, a serine protease from the predatory bacterium Bdellovibrio bacteriovorus with utility for analysis of therapeutic antibodies. Appl. Environ. Microbiol. 83, e03037–e03016. doi: 10.1128/AEM.03037-16
Brauner, A., Fridman, O., Gefen, O., and Balaban, N. Q. (2016). Distinguishing between resistance, tolerance and persistence to antibiotic treatment. Nat. Rev. Microbiol. 14, 320–330. doi: 10.1038/nrmicro.2016.34
Browne, A. J., Kashef Hamadani, B. H., Kumaran, E. A. P., Rao, P., Longbottom, J., Harriss, E., et al. (2020). Drug-resistant enteric fever worldwide, 1990 to 2018: a systematic review and meta-analysis. BMC Med. 18:1. doi: 10.1186/s12916-019-1443-1
Bula-Rudas, F. J., Rathore, M. H., and Maraqa, N. F. (2015). Salmonella infections in childhood. Adv. Pediatr. Infect. Dis. 62, 29–58. doi: 10.1016/j.yapd.2015.04.005
Čabarkapa, I., Čolović, R., Đuragić, O., Popović, S., Kokić, B., and Milanov, D. (2019). Anti-biofilm activities of essential oils rich in carvacrol and thymol against Salmonella Enteritidis. Biofouling 35, 361–375. doi: 10.1080/08927014.2019.1610169
Cavaleri, M., and Manolis, E. (2015). Hollow fiber system model for tuberculosis: the European medicines agency experience. Clin. Infect. Dis. 61, S1–S4. doi: 10.1093/cid/civ484
Cavallo, F. M., Jordana, L., Friedrich, A. W., Glasner, C., and van Dijl, J. M. (2021). Bdellovibrio bacteriovorus: a potential ‘living antibiotic’ to control bacterial pathogens. Crit. Rev. Microbiol. 47, 630–646. doi: 10.1080/1040841X.2021.1908956
Chlebicz, A., and Śliżewska, K. (2018). Campylobacteriosis, Salmonellosis, Yersiniosis, and Listeriosis as zoonotic foodborne diseases: a review. Int. J. Environ. Res. Public Health 15:863. doi: 10.3390/ijerph15050863
Chylkova, T., Cadena, M., Ferreiro, A., and Pitesky, M. (2017). Susceptibility of Salmonella biofilm and planktonic bacteria to common disinfectant agents used in poultry processing. J. Food Prot. 80, 1072–1079. doi: 10.4315/0362-028X.JFP-16-393
Claudi, B., Spröte, P., Chirkova, A., Personnic, N., Zankl, J., Schürmann, N., et al. (2014). Phenotypic variation of Salmonella in host tissues delays eradication by antimicrobial chemotherapy. Cells 158, 722–733. doi: 10.1016/j.cell.2014.06.045
Coculescu, B. I., Palade, A. M., and Purcarea, V. L. (2014). Multiresistance to antibiotics of Salmonella enterica serovar Typhimurium strains producing extended spectrum beta-lactamases (ESBLs). J. Med. Life 2, 80–82.
Crump, J. A., and Heyderman, R. S. (2015). A perspective on invasive salmonella disease in Africa. Clin. Infect. Dis. 61, S235–S240. doi: 10.1093/cid/civ709
Czerucka, D., Piche, T., and Rampal, P. (2007). Review article, yeast as probiotics-Saccharomyces boulardii. Aliment. Pharmacol. Ther. 26, 767–778. doi: 10.1111/j.1365-2036.2007.03442.x
D’ambrosio, S., Ventrone, M., Fusco, A., Casillo, A., Dabous, A., Cammarota, M., et al. (2022). Limosilactobacillus fermentum from buffalo milk is suitable for potential biotechnological process development and inhibits Helicobacter pylori in a gastric epithelial cell model. Biotechnol. Rep. 34:e00732. doi: 10.1016/j.btre.2022.e00732
Das, T., Sehar, S., and Manefield, M. (2013). The roles of extracellular DNA in the structural integrity of extracellular polymeric substance and bacterial biofilm development. Environ. Microbiol. Rep. 5, 778–786. doi: 10.1111/1758-2229.12085
Desai, S. K., Padmanabhan, A., Harshe, S., Zaidel-Bar, R., and Kenney, L. J. (2019). Salmonella biofilms program innate immunity for persistence in Caenorhabditis elegans. Proc. Natl. Acad. Sci. U. S. A. 116, 12462–12467. doi: 10.1073/pnas.1822018116
Drlica, K., and Zhao, X. (2021). Bacterial death from treatment with fluoroquinolones and other lethal stressors. Expert Rev. Anti-Infect. Ther. 19, 601–618. doi: 10.1080/14787210.2021.1840353
Ekiert, D. C., Bhabha, G., Isom, G. L., Greenan, G., Ovchinnikov, S., Henderson, I. R., et al. (2017). Architectures of lipid transport systems for the bacterial outer membrane. Cells 169, 273–285.e17. doi: 10.1016/j.cell.2017.03.019
Elliott, A. G., Huang, J. X., Neve, S., Zuegg, J., Edwards, I. A., Cain, A. K., et al. (2020). An amphipathic peptide with antibiotic activity against multidrug-resistant Gram-negative bacteria. Nat. Commun. 11:3184. doi: 10.1038/s41467-020-16950-x
Ferro, B. E., Srivastava, S., Deshpande, D., Sherman, C. M., Pasipanodya, J. G., van Soolingen, D., et al. (2015). Amikacin pharmacokinetics/pharmacodynamics in a novel hollow-fiber Mycobacterium abscessus disease model. Antimicrob. Agents Chemother. 60, 1242–1248. doi: 10.1128/AAC.02282-15
Fisher, R. A., Gollan, B., and Helaine, S. (2017). Persistent bacterial infections and persister cells. Nat. Rev. Microbiol. 15, 453–464. doi: 10.1038/nrmicro.2017.42
Forkus, B., Ritter, S., Vlysidis, M., Geldart, K., and Kaznessis, Y. N. (2017). Antimicrobial probiotics reduce Salmonella enterica in Turkey gastrointestinal tracts. Sci. Rep. 7:40695. doi: 10.1038/srep40695
Ghosh, C., Sarkar, P., Issa, R., and Haldar, J. (2019). Alternatives to conventional antibiotics in the era of antimicrobial resistance. Trends Microbiol. 27, 323–338. doi: 10.1016/j.tim.2018.12.010
Giraud, E., Cloeckaert, A., Kerboeuf, D., and Chaslus-Dancla, E. (2000). Evidence for active efflux as the primary mechanism of resistance to ciprofloxacin in Salmonella enterica serovar typhimurium. Antimicrob. Agents Chemother. 44, 1223–1228. doi: 10.1128/aac.44.5.1223-1228.2000
González, J. F., Alberts, H., Lee, J., Doolittle, L., and Gunn, J. S. (2018). Biofilm formation protects Salmonella from the antibiotic ciprofloxacin in vitro and in vivo in the mouse model of chronic carriage. Sci. Rep. 8:222. doi: 10.1038/s41598-017-18516-2
Grimsey, E. M., Fais, C., Marshall, R. L., Ricci, V., Ciusa, M. L., Stone, J. W., et al. (2020). Chlorpromazine and amitriptyline are substrates and inhibitors of the AcrB multidrug efflux pump. MBio 11, e00465–e00420. doi: 10.1128/mBio.00465-20
Gunn, J. S., Marshall, J. M., Baker, S., Dongol, S., Charles, R. C., and Ryan, E. T. (2014). Salmonella chronic carriage, epidemiology, diagnosis, and gallbladder persistence. Trends Microbiol. 22, 648–655. doi: 10.1016/j.tim.2014.06.007
Guo, L., Qi, M., Gao, C., Ye, C., Hu, G., Song, W., et al. (2022). Engineering Escherichia coli lifespan for enhancing chemical production. Nat. Catal. 3, 307–318. doi: 10.1038/s41929-019-0411-7
Gut, A. M., Vasiljevic, T., Yeager, T., and Donkor, O. N. (2018). Salmonella infection-prevention and treatment by antibiotics and probiotic yeasts, a review. Microbiology 164, 1327–1344. doi: 10.1099/mic.0.000709
Hall, C. W., and Mah, T. F. (2017). Molecular mechanisms of biofilm-based antibiotic resistance and tolerance in pathogenic bacteria. FEMS Microbiol. Rev. 41, 276–301. doi: 10.1093/femsre/fux010
Higgins, S. E., Erf, G. F., Higgins, J. P., Henderson, S. N., Wolfenden, A. D., Gaona-Ramirez, G., et al. (2007). Effect of probiotic treatment in broiler chicks on intestinal macrophage numbers and phagocytosis of Salmonella enteritidis by abdominal exudate cells. Poult. Sci. 86, 2315–2321. doi: 10.3382/ps.2007-00123
Hopkins, K. L., Davies, R. H., and Threlfall, E. J. (2005). Mechanisms of quinolone resistance in Escherichia coli and Salmonella: recent developments. Int. J. Antimicrob. Agents 25, 358–373. doi: 10.1016/j.ijantimicag.2005.02.006
Huang, Y. M., Munguia, J., Miao, Y., Nizet, V., and McCammon, J. A. (2019). Docking simulation and antibiotic discovery targeting the MlaC protein in gram-negative bacteria. Chem. Biol. Drug Des. 93, 647–652. doi: 10.1111/cbdd.13462
Huang, C., Shi, J., Ma, W., Li, Z., Wang, J., Li, J., et al. (2018). Isolation characterization and application of a novel specific Salmonella bacteriophage in different food matrices. Food Res. Int. 111, 631–641. doi: 10.1016/j.foodres.2018.05.071
Hughes, G. W., Hall, S. C. L., Laxton, C. S., Sridhar, P., Mahadi, A. H., Hatton, C., et al. (2019). Evidence for phospholipid export from the bacterial inner membrane by the Mla ABC transport system. Nat. Microbiol. 4, 1692–1705. doi: 10.1038/s41564-019-0481-y
Kadouri, D. E. K., Shanks, R. M., and Doi, Y. (2013). Predatory bacteria: a potential ally against multidrug-resistant Gram-negative pathogens. PLoS One 8:e63397. doi: 10.1371/journal.pone.0063397
Khalid, S., Berglund, N. A., Holdbrook, D. A., Leung, Y. M., and Parkin, J. (2015). The membranes of Gram-negative bacteria, progress in molecular modelling and simulation. Biochem. Soc. Trans. 43, 162–167. doi: 10.1042/BST20140262
Khan, S., and Chousalkar, K. K. (2019). Short-term feeding of probiotics and synbiotics modulates caecal microbiota during Salmonella Typhimurium infection but does not reduce shedding and invasion in chickens. Appl. Microbiol. Biotechnol. 104, 319–334. doi: 10.1007/s00253-019-10220-7
Khosravani, M., Soltan Dallal, M. M., and Norouzi, M. (2020). Phytochemical composition and anti-efflux pump activity of hydroalcoholic, aqueous, and hexane extracts of artemisia tournefortiana in ciprofloxacin-resistant strains of Salmonella enterica serotype Enteritidis. Iran. J. Public Health 49, 134–144. doi: 10.18502/ijph.v49i1.3060
Kim, H. B., and Isaacson, R. E. (2017). Salmonella in swine: microbiota interactions. Annu. Rev. Anim. Biosci. 5, 43–63. doi: 10.1146/annurev-animal-022516-022834
Kim, J. S., and Wood, T. K. (2017). Tolerant, growing cells from nutrient shifts are not persister cells. MBio 8, e00354–e00317.
Lamut, A., Peterlin Mašič, L., Kikelj, D., and Tomašič, T. (2019). Efflux pump inhibitors of clinically relevant multidrug resistant bacteria. Med. Res. Rev. 39, 2460–2504. doi: 10.1002/med.21591
Lee, S. J., Awji, E. G., Park, N. H., and Park, S. C. (2017). Using in vitro dynamic models to evaluate fluoroquinolone activity against emergence of resistant Salmonella enterica Serovar Typhimurium. Antimicrob. Agents Chemother. 61, e01756–e01716. doi: 10.1128/AAC.01756-16
Leegaard, T. M., van Gestel, M. H., Petit, P. L., and van de Klundert, J. A. (1996). Antibiotic resistance mechanisms in Salmonella species causing bacteraemia in Malawi and Kenya. APMIS 104, 302–306. doi: 10.1111/j.1699-0463.1996.tb00721.x
Levin-Reisman, I., Ronin, I., Gefen, O., Braniss, I., Shoresh, N., and Balaban, N. Q. (2017). Antibiotic tolerance facilitates the evolution of resistance. Science 355, 826–830. doi: 10.1126/science.aaj2191
Lewis, K. (2010). Persister cells. Annu. Rev. Microbiol. 64, 357–372. doi: 10.1146/annurev.micro.112408.134306
Liu, Y., Yang, K., Jia, Y., Shi, J., Tong, Z., Fang, D., et al. (2021). Gut microbiome alterations in high-fat-diet-fed mice are associated with antibiotic tolerance. Nat. Microbiol. 6, 874–884. doi: 10.1038/s41564-021-00912-0
Luhachack, L., and Nudler, E. (2014). Bacterial gasotransmitters: an innate defense against antibiotics. Curr. Opin. Microbiol. 21, 13–17. doi: 10.1016/j.mib.2014.06.017
Mąka, Ł., and Popowska, M. (2016). Antimicrobial resistance of Salmonella spp. isolated from food. Rocz. Panstw. Zakl. Hig. 67, 343–358.
Martins, F. S., Dalmasso, G., Arantes, R. M., Doye, A., Lemichez, E., Lagadec, P., et al. (2010). Interaction of Saccharomyces boulardii with Salmonella enterica serovar Typhimurium protects mice and modifies T84 cell response to the infection. PLoS One 5:e8925. doi: 10.1371/journal.pone.0008925
Medeiros, A. A., O’Brien, T. F., Rosenberg, E. Y., and Nikaido, H. (1987). Loss of OmpC porin in a strain of Salmonella typhimurium causes increased resistance to cephalosporins during therapy. J. Infect. Dis. 156, 751–757. doi: 10.1093/infdis/156.5.751
Menconi, A., Wolfenden, A. D., Shivaramaiah, S., Terraes, J. C., Urbano, T., Kuttel, J., et al. (2011). Effect of lactic acid bacteria probiotic culture for the treatment of Salmonella enterica serovar Heidelberg in neonatal broiler chickens and turkey poults. Poult. Sci. 90, 561–565. doi: 10.3382/ps.2010-01220.5
Miyaue, S., Suzuki, E., Komiyama, Y., Kondo, Y., Morikawa, M., and Maeda, S. (2018). Bacterial memory of persisters, bacterial persister cells can retain their phenotype for days or weeks after withdrawal from colony-biofilm culture. Front. Microbiol. 9:1396. doi: 10.3389/fmicb.2018.01396
Murret-Labarthe, C., Kerhoas, M., Dufresne, K., and Daigle, F. (2020). New roles for two-component system response regulators of Salmonella enterica Serovar Typhi during host cell interactions. Microorganisms 8:722. doi: 10.3390/microorganisms8050722
Negus, D., Moore, C., Baker, M., Raghunathan, D., Tyson, J., and Sockett, R. E. (2017). Predator versus pathogen: how does predatory Bdellovibrio bacteriovorus interface with the challenges of killing gram-negative pathogens in a host setting? Annu. Rev. Microbiol. 71, 441–457. doi: 10.1146/annurev-micro-090816-093618
Nishino, K., Nikaido, E., and Yamaguchi, A. (2007). Regulation of multidrug efflux systems involved in multidrug and metal resistance of Salmonella enterica serovar Typhimurium. J. Bacteriol. 189, 9066–9075. doi: 10.1128/JB.01045-07
Nolivos, S., Cayron, J., Dedieu, A., Page, A., Delolme, F., and Lesterlin, C. (2019). Role of AcrAB-TolC multidrug efflux pump in drug-resistance acquisition by plasmid transfer. Science 364, 778–782. doi: 10.1126/science.aav6390
Oelschlaeger, T. A. (2010). Mechanisms of probiotic actions-A review. Int. J. Med. Microbiol. 300, 57–62. doi: 10.1016/j.ijmm.2009.08.005
Oladeinde, A., Cook, K., Lakin, S. M., Woyda, R., Abdo, Z., Looft, T., et al. (2019). Horizontal gene transfer and acquired antibiotic resistance in Salmonella enterica serovar Heidelberg following in vitro incubation in broiler ceca. Appl. Environ. Microbiol. 85, e01903–e01919. doi: 10.1128/AEM.01903-19
Pang, Z., Raudonis, R., Glick, B. R., Lin, T. J., and Cheng, Z. (2019). Antibiotic resistance in Pseudomonas aeruginosa, mechanisms and alternative therapeutic strategies. Biotechnol. Adv. 37, 177–192. doi: 10.1016/j.biotechadv.2018.11.013
Petrova, M. I., Imholz, N. C., Verhoeven, T. L., Balzarini, J., Van Damme, E. J., Schols, D., et al. (2016). Lectin-like molecules of lactobacillus rhamnosus GG inhibit pathogenic Escherichia coli and Salmonella biofilm formation. PLoS One 11:e0161337. doi: 10.1371/journal.pone.0161337
Piewngam, P., Zheng, Y., Nguyen, T. H., Dickey, S. W., Joo, H. S., Nguyen, T. H., et al. (2018). Pathogen elimination by probiotic Bacillus via signalling interference. Nature 562, 532–537. doi: 10.1038/s41586-018-0616-y
Piggot, T. J., Holdbrook, D. A., and Khalid, S. (2011). Electroporation of the E. coli and S. aureus membranes, molecular dynamics simulations of complex bacterial membranes. J. Phys. Chem. B 115, 13381–13388. doi: 10.1021/jp207013v
Pontier-Bres, R., Munro, P., Boyer, L., Anty, R., Imbert, V., Terciolo, C., et al. (2014). Saccharomyces boulardii modifies Salmonella typhimurium traffic and host immune responses along the intestinal tract. PLoS One 9:e103069. doi: 10.1371/journal.pone.0103069
Povolo, V. R., and Ackermann, M. (2019). Disseminating antibiotic resistance during treatment. Science 364, 737–738. doi: 10.1126/science.aax6620
Prajapati, J. D., Kleinekathöfer, U., and Winterhalter, M. (2021). How to enter a Bacterium: bacterial porins and the permeation of antibiotics. Chem. Rev. 121, 5158–5192. doi: 10.1021/acs.chemrev.0c01213
Rensch, U., Nishino, K., Klein, G., and Kehrenberg, C. (2014). Salmonella enterica serovar Typhimurium multidrug efflux pumps EmrAB and AcrEF support the major efflux system AcrAB in decreased susceptibility to triclosan. Int. J. Antimicrob. Agents 44, 179–180. doi: 10.1016/j.ijantimicag.2014.04.015
Richter, M. F., and Hergenrother, P. J. (2019). The challenge of converting Gram-positive-only compounds into broad-spectrum antibiotics. Ann. N. Y. Acad. Sci. 1435, 18–38. doi: 10.1111/nyas.13598
Rishi, P., Bhagat, N. R., Thakur, R., and Pathania, P. (2018). Tackling Salmonella persister cells by antibiotic-nisin combination via mannitol. Indian J. Microbiol. 58, 239–243. doi: 10.1007/s12088-018-0713-5
Rokana, N., Singh, R., Mallappa, R. H., Batish, V. K., and Grover, S. (2016). Modulation of intestinal barrier function to ameliorate Salmonella infection in mice by oral administration of fermented milks produced with Lactobacillus plantarum MTCC 5690 -a probiotic strain of Indian gut origin. J. Med. Microbiol. 65, 1482–1493. doi: 10.1099/jmm.0.000366
Ryan, M. P., O’Dwyer, J., and Adley, C. C. (2017). Evaluation of the complex nomenclature of the clinically and veterinary significant pathogen Salmonella. Biomed. Res. Int. 2017:3782182. doi: 10.1155/2017/3782182
Rycroft, J. A., Gollan, B., Grabe, G. J., Hall, A., Cheverton, A. M., Larrouy-Maumus, G., et al. (2018). Activity of acetyltransferase toxins involved in Salmonella persister formation during macrophage infection. Nat. Commun. 9:1993. doi: 10.1038/s41467-018-04472-6
Sadekuzzaman, M., Mizan, M. F. R., Yang, S., Kim, H. S., and Ha, S. D. (2018). Application of bacteriophages for the inactivation of Salmonella spp. in biofilms. Food Sci. Technol. Int. 24, 424–433. doi: 10.1177/1082013218763424
Sandala, J. L., Eichar, B. W., Kuo, L. G., Hahn, M. M., Basak, A. K., Huggins, W. M., et al. (2020). A dual-therapy approach for the treatment of biofilm-mediated Salmonella gallbladder carriage. PLoS Pathog. 16:e1009192. doi: 10.1371/journal.ppat.1009192
Schwudke, D., Strauch, E., Krueger, M., and Appel, B. (2001). Taxonomic studies of predatory Bdellovibrios based on 16S rRNA analysis, ribotyping and the hit locus and characterization of isolates from the gut of animals. Syst. Appl. Microbiol. 24, 385–394. doi: 10.1078/0723-2020-00042
Shaheen, A., Afridi, W. A., Mahboob, S., Sana, M., Zeeshan, N., Ismat, F., et al. (2019). Reserpine is the new addition into the repertoire of AcrB efflux pump inhibitor. Mol. Biol. 53, 674–684. doi: 10.1134/S0026898419040128
Shatalin, K., Nuthanakanti, A., Kaushik, A., Shishov, D., Peselis, A., Shamovsky, I., et al. (2021). Inhibitors of bacterial H2S biogenesis targeting antibiotic resistance and tolerance. Science 372, 1169–1175. doi: 10.1126/science.abd8377
Shatzkes, K., Tang, C., Singleton, E., Shukla, S., Zuena, M., Gupta, S., et al. (2017). Effect of predatory bacteria on the gut bacterial microbiota in rats. Sci. Rep. 7:43483. doi: 10.1038/srep43483
Shen, J., Yang, B., Gu, Q., Zhang, G., Yang, J., Xue, F., et al. (2017). The role of AcrAB-TolC efflux pump in mediating fluoroquinolone resistance in naturally occurring Salmonella isolates from China. Foodborne Pathog. Dis. 14, 728–734. doi: 10.1089/fpd.2017.2291
Shi, H., Zhou, X., Zou, W., Wang, Y., Lei, C., Xiang, R., et al. (2018). Co-occurrence of biofilm formation and quinolone resistance in Salmonella enterica serotype typhimurium carrying an IncHI2-type oqxAB-positive plasmid. Microb. Pathog. 123, 68–73. doi: 10.1016/j.micpath.2018.06.006
Sockett, R. E., and Lambert, C. (2004). Bdellovibrio as therapeutic agents: a predatory renaissance? Nat. Rev. Microbiol. 2, 669–675. doi: 10.1038/nrmicro959
Song, S., Hwang, S., Lee, S., Ha, N. C., and Lee, K. (2014). Interaction mediated by the putative tip regions of MdsA and MdsC in the formation of a Salmonella-specific tripartite efflux pump. PLoS One 9:24. doi: 10.1371/journal.pone.0100881
Song, M., Liu, Y., Huang, X., Ding, S., Wang, Y., Shen, J., et al. (2020). A broad-spectrum antibiotic adjuvant reverses multidrug-resistant Gram-negative pathogens. Nat. Microbiol. 5, 1040–1050. doi: 10.1038/s41564-020-0723-z
Soto, S. M. (2013). Role of efflux pumps in the antibiotic resistance of bacteria embedded in a biofilm. Virulence 4, 223–229. doi: 10.4161/viru.23724
Srivastava, S., Deshpande, D., and Gumbo, T. (2017). Failure of the azithromycin and ethambutol combination regimen in the hollow-fiber system model of pulmonary Mycobacterium avium infection is due to acquired resistance. J. Antimicrob. Chemother. 72, i20–i23. doi: 10.1093/jac/dkx303
Stapels, D. A. C., Hill, P. W. S., Westermann, A. J., Fisher, R. A., Thurston, T. L., Saliba, A. E., et al. (2018). Salmonella persisters undermine host immune defenses during antibiotic treatment. Science 362, 1156–1160. doi: 10.1126/science.aat7148
Sun, Y., Dai, M., Hao, H., Wang, Y., Huang, L., Almofti, Y. A., et al. (2011). The role of RamA on the development of ciprofloxacin resistance in Salmonella enterica serovar Typhimurium. PLoS One 6:e23471. doi: 10.1371/journal.pone.0023471
Sun, J., Deng, Z., and Yan, A. (2014). Bacterial multidrug efflux pumps: mechanisms, physiology and pharmacological exploitations. Biochem. Biophys. Res. Commun. 453, 254–267. doi: 10.1016/j.bbrc.2014.05.090
Sun, Y., Ye, J., Hou, Y., Chen, H., Cao, J., and Zhou, T. (2017). Predation efficacy of Bdellovibrio bacteriovorus on multidrug-resistant clinical pathogens and their corresponding biofilms. Jpn. J. Infect. Dis. 70, 485–489. doi: 10.7883/yoken.JJID.2016.405
Tang, X., Chang, S., Qiao, W., Luo, Q., Chen, Y., Jia, Z., et al. (2021). Structural insights into outer membrane asymmetry maintenance in Gram-negative bacteria by MlaFEDB. Nat. Struct. Mol. Biol. 28, 81–91. doi: 10.1038/s41594-020-00532-y
Tsuchido, T., and Takano, M. (1988). Sensitization by heat treatment of Escherichia coli K-12 cells to hydrophobic antibacterial compounds. Antimicrob. Agents Chemother. 32, 1680–1683. doi: 10.1128/AAC.32.11.1680
Uddin, M. J., and Ahn, J. (2018). Characterization of β-lactamase- and efflux pump-mediated multiple antibiotic resistance in Salmonella Typhimurium. Food Sci. Biotechnol. 27, 921–928. doi: 10.1007/s10068-018-0317-1
Vuotto, C., Longo, F., and Donelli, G. (2014). Probiotics to counteract biofilmassociated infections, promising and conflicting data. Int. J. Oral Sci. 6, 189–194. doi: 10.1038/ijos.2014.52
Wang-Kan, X., Blair, J. M. A., Chirullo, B., Betts, J., La Ragione, R. M., Ivens, A., et al. (2017). Lack of AcrB efflux function confers loss of virulence on Salmonella enterica Serovar Typhimurium. MBio 8, e00968–e00917. doi: 10.1128/mBio.00968-17
Waso, M., Reyneke, B., Havenga, B., Khan, S., and Khan, W. (2021). Insights into Bdellovibrio spp. mechanisms of action and potential applications. World J. Microbiol. Biotechnol. 37:85. doi: 10.1007/s11274-021-03054-x
WHO (2017). “World Health Organization” in Global priority list of antibiotic-resistant bacteria to guide research discovery and development of new antibiotics (Geneva: World Health Organization)
Wright, G. D. (2005). Bacterial resistance to antibiotics, enzymatic degradation and modification. Adv. Drug Deliv. Rev. 57, 1451–1470. doi: 10.1016/j.addr.2005.04.002
Wu, H., Wang, Y., Wu, Y., Qiao, J., Li, H., Zheng, S., et al. (2015). Emergence of β-lactamases and extended-spectrum β-lactamases (ESBLs) producing Salmonella in retail raw chicken in China. Foodborne Pathog. Dis. 12, 228–234. doi: 10.1089/fpd.2014.1859
Wucher, B. R., Elsayed, M., Adelman, J. S., Kadouri, D. E., and Nadell, C. D. (2021). Bacterial predation transforms the landscape and community assembly of biofilms. Curr. Biol. 31, 2643–2651.e3. doi: 10.1016/j.cub.2021.03.036
Xu, X., Peng, Q., Zhang, Y., Tian, D., Zhang, P., Huang, Y., et al. (2020). Antibacterial potential of a novel Lactobacillus casei strain isolated from Chinese northeast sauerkraut and the antibiofilm activity of its exopolysaccharides. Food Funct. 11, 4697–4706. doi: 10.1039/d0fo00905a
Yamagishi, A., Nakano, S., Yamasaki, S., and Nishino, K. (2011). AcrA dependency of the AcrD efflux pump in Salmonella enterica serovar Typhimurium. J. Antibiot. 64, 433–437. doi: 10.1038/ja.2011.28
Yang, S., Lopez, C. R., and Zechiedrich, E. L. (2006). Quorum sensing and multidrug transporters in Escherichia coli. Proc. Natl. Acad. Sci. 103, 2386–2391. doi: 10.1073/pnas.0502890102
Yeh, Y., Purushothaman, P., Gupta, N., Ragnone, M., Verma, S. C., and de Mello, A. S. (2017). Bacteriophage application on red meats and poultry, effects on Salmonella population in final ground products. Meat Sci. 127, 30–34. doi: 10.1016/j.meatsci.2017.01.001
Zappala, R. (2004). The population biology of antibiotic treatment failure in immunecompetent mice. Ph.D. Dissertation Atlanta, GA Emory University
Keywords: Salmonella, resistance, tolerance, out membrane, probiotics, predatory bacteria, clinical application
Citation: Zhou K, Sun L, Zhang X, Xu X, Mi K, Ma W, Zhang L and Huang L (2023) Salmonella antimicrobials inherited and the non-inherited resistance: mechanisms and alternative therapeutic strategies. Front. Microbiol. 14:1176317. doi: 10.3389/fmicb.2023.1176317
Edited by:
Octavio Luiz Franco, Catholic University of Brasilia (UCB), BrazilReviewed by:
Changyong Cheng, Zhejiang A&F University, ChinaAlexandro Rodríguez-Rojas, University of Veterinary Medicine Vienna, Austria
Copyright © 2023 Zhou, Sun, Zhang, Xu, Mi, Ma, Zhang and Huang. This is an open-access article distributed under the terms of the Creative Commons Attribution License (CC BY). The use, distribution or reproduction in other forums is permitted, provided the original author(s) and the copyright owner(s) are credited and that the original publication in this journal is cited, in accordance with accepted academic practice. No use, distribution or reproduction is permitted which does not comply with these terms.
*Correspondence: Lingli Huang, aHVhbmdsaW5nbGlAbWFpbC5oemF1LmVkdS5jbg==